An updated review of the salient geomedical aspects of mercury for enhancement of data quality in simulation modelling and other prognostic applications: Africa case descriptions
- Faculty of Natural Sciences, Mangosuthu University of Technology, Umlazi, KwaZulu Natal, South Africa
Mercury (Hg) pollution is of global concern. Despite the prolificity of research in the past two decades or so, there are still several uncertainties and variabilities in our knowledge of both the element’s exposure dynamics and its health effects. Understanding the intricacies of the element’s emissions-to-impact path, for instance, is rendered intractable by its varied environmental fate and the overarching influence of environmental, geochemical, biological and socioeconomic drivers. In this paper, an updated synopsis of the relevant and more important geomedical characteristics of Hg is considered to constitute part of the provision of high-quality input data needed in Hg simulation modelling studies, and other applications such as the provision of long-term data necessary for evaluating the effectiveness of regulatory measures at various scales. A critical overview is presented on the importance of data quality in parameterisation, and validation of Hg simulation models and other related applications. In this connection, the dearth of modern measurements of Hg abundance in crustal rocks and other Earth materials which needs to be set prior to simulation as well as in modelling source to sink transfers in the Hg cycle, is highlighted. An improved input data quality would also foster the production of model outcomes that are accurate enough for applications in design of better exposure-limiting strategies; and in providing insights on how the course of diagnosis and treatment currently proffered by physicians for Hg-induced maladies, can be revised or expanded. Model results derived from high-quality input datasets also have a high potential for providing forecasting capabilities to inform policy.
1 Introduction
Mercury is a naturally occurring element. For hundreds of years, it has been directly dispersed by humans into aquatic and terrestrial ecosystems through activities such as biomass burning, mining of metal sulphide ores, use in precious metal extraction, and many other materials where it is present in significant amounts (e.g., paint, electronic devices and chlor-alkali plants). Its release and exposure to humans represent one of the most serious environmental contamination problems known. There are also natural sources of Hg release, including weathering of rocks, volcanic activity and forest fires. Mercury can evaporate from the ocean into the atmosphere, and be deposited back into the oceans or onto land.
Worldwide health concerns on Hg, centre on human consumption of seafood, mainly fish, contaminated with methyl Hg (MeHg), with neurotoxicity being the most important of these concerns. Methyl Hg easily reaches the bloodstream and is distributed to all tissues and can cross the normally protective blood-brain barrier to enter the brain. Mercury that enters the body is of especial concern to pregnant women and women of childbearing age, because it can readily move through the placenta to foetuses and their developing brains. Other effects of Hg exposure in humans include kidney damage, renal impairment, gastrointestinal, genetic, cardiovascular and developmental disorders, and even death in extreme cases.
Despite the prolificity of research worldwide on Hg exposure, there still exist several gaps in our understanding of the element’s emissions-to-impact path, as well as the dynamic interactions during metabolic processes that lead to maladies. For instance, there are significant gaps in the knowledge of chemical processes affecting Hg atmospheric transport and deposition, characteristics of the air-surface exchange and processes responsible for re-emission of Hg to the atmosphere (Travnikov et al., 2013; Leiva González et al., 2022); and properly designed simulation modelling experiments with high quality input data would go a long way in helping to bridge these knowledge gaps. The assemblage of a high quality geomedical database for Hg would also enhance our predictive capacity for future modifications in Hg pertubations in the environment under climate change, such as the still unknown direction of Hg ocean - air fluxes (See, e.g., Custódio et al., 2022) and the consequent implications for the elements’ toxicokinetics.
1.1 Mercury simulation modelling
A simplified and commonly used definition of ‘simulation’ was given by Banks et al. (2010, p. 3), viz: “an approximate imitation of the operation of a process or system”. Model simulation analyses are increasingly being conducted in many contexts, such as in medical studies on explaining a system or examining how the system will look in the future or how it behaved in past times (See, e.g., Greenberg et al., 2022; Shi et al., 2022; Wallett et al., 2022). The accuracy of the simulation outcomes, however, is a much-debated issue; but there is little doubt that it depends to a large extent on the quality of the input data (Ekström et al. (2021), defined here as ‘a measure of the condition of data with regard to factors such as completeness, consistency, accuracy and reliability, and whether or not it is up-to-date’ (Hassenstein and Vanella, 2022). These were therefore the factors taken into account (in the present critical overview) in compiling the geomedically relevant attributes of Hg, making use of the most authentic and up-to-date sources of reference available as of 2022/2023.
A number of important studies have recently been conducted on the development of a general model to assess the impact of data quality on the accuracy of model output (See, e.g., Hasan and Padman, 2006; Ekström et al., 2021; Wang et al., 2023). Indeed, as Forbus and Berleant (2022) recently retorted: “A simulation model output is only as good as the data input that drives it, and without taking the time to ensure quality input data, the output may not yield usable results.” Data are regarded to be of high quality if they correctly represent the real-world situation to which they refer.
1.2 Input data management
Skoogh and Johansson (2008) define ‘input data management’ as: “… the entire process of preparing quality assured, and simulation adapted, representations of all relevant input data parameters for simulation models.
The cruciality of the input data quality in the data management process is underscored by its role in model realisation and verification. A high-quality input data ensure that the right information is made available in the right format and at the right time. Protocols for simulation model verification and validation are a vibrant field of ongoing academic study, research and development in simulations technology and practice.
In this paper, a brief updated synopsis of pertinent geomedical attributes of Hg is given to foster their proper understanding, but more particularly, to provide the basis for the development of high-quality input data for performing model simulation experiments and analyses, such as evaluating model fits for HgII solubility by comparing modelled and observed distribution of HgII between solid/adsorbed and aqueous phases (Amde et al., 2016; Liem-Nguyen, 2016; Geng et al., 2023).
Successful simulation modelling of dynamic and complex processes such as chemical transport of Hg, has demonstrably high potential for helping us better understand the element’s emissions-to-impact path and exposure dynamics. Such robust model outputs have the further potential of opening the doors for improved exposure prevention protocols and better diagnosis and therapy for maladies resulting from Hg exposure and its effect on vulnerable ecosystems.
Finally, the latest advances in Hg research (as of 2022/2023) in Africa (where research gaps on Hg transportation dynamics, exposure routes and toxicokinetics are widest), as reflected in key papers in reputable journals or book chapters, are given, and suggested areas of future Hg research in the field of Medical Geology are listed.
2 Sources
Mercury is emitted by natural sources, such as volcanoes, geothermal springs, geologic deposits, and the oceans; and by human activities. Human-related sources primarily include coal combustion, waste incineration, industrial uses, and mining.
In the environment, Hg occurs mainly in the form of inorganic Hg. Inorganic Hg species enter the air from the mining of deposits of ores that contain Hg, from the emissions of coal-fired power plants, from burning municipal and medical waste, from the production of cement, and from uncontrolled releases in factories that use Hg (ATSDR, reviewed 2015). Most models evaluating sources of Hg in the oceans have been built using input data on Hg deposited directly from the atmosphere. However, a new study now shows that rivers are actually the main source of this toxic element along the world’s coasts (Liu et al., 2021).
Relatively recently, Ehrlich and Newman (2008) gave an average crustal abundance of Hg by mass of only 0.08 ppm. There is a dearth of recent estimates of Hg content in the various components of the Earth’s crust (igneous, metamorphic and sedimentary rocks), as the following sections (I to III) clearly show.
2.1 Igneous rocks
Igneous rocks are the basic sources of Hg (USGS, 1970). These (igneous) rocks generally contain less than 200 ppb of Hg and 100 ppb on average, except for alkalic igneous rocks and deep-seated eclogites and kimberlites that average several hundred ppb Hg.
The following world averages are from Jonasson and Boyle (1972): Granite, granodiorite, 62 ppb; Acid extrusives, 62 ppb; Alkali-rich rocks (syenite, nepheline syenite, phonolite), 150 ppb; Diorite, 38 ppb; Andesite, 66 ppb; Basalt, 20 ppb; Ultrabasic rocks (dunite and peridotite), 168 ppb.
2.2 Sedimentary rocks
Most sedimentary rocks have Hg contents less than 200 ppb Hg and generally average less than 100 ppb, except for shales and clays, for which the data show considerable variation with average contents of a few hundred ppb Hg. Certain organic-rich shales may reach concentrations of up to 10,000 ppb or more of Hg (USGS, 1970; Loukola-Ruskeeniemi, 1990; Zhu et al., 2021).
The following world averages are from Jonasson and Boyle (1972), except the value for ‘coal’ which is from Swaine (1990): Sandstone, arkose, 55 ppb; Shale, 67 ppb; Limestone/dolomite, 40 ppb; Coal (coal burnt in power stations of EU countries - domestic and imported), 300 ppb.
In addition to organic-rich shales, other rocks with abnormally high Hg contents are known to exist (USGS, 1970).
2.3 Metamorphic rocks
The Hg content of metamorphic rocks varies very widely; but are generally much lower than (the Hg content) of sedimentary rocks, probably as a result of the loss of Hg during high-pressure and high-temperature conditions during metamorphism (Chen et al., 2022). Again, as is the case of other rock types, there is a dearth of recent measurements of the Hg content of metamorphic rocks. The following values are from Jonasson and Boyle (1972): Amphibolite, 50 ppb; Schist, 100 ppb; Gneiss, 50 ppb.
2.4 Minerals
There are quite a number of Hg-bearing minerals, but only a few occur abundantly in nature. The most common mineral containing Hg in ore deposits is cinnabar, or Hg sulphide (HgS), but naturally occurring elemental Hg, or quicksilver is also found in some Hg deposits, e.g., some cinnabar deposits. Metacinnabar, a Hg sulphide mineral and a high temperature polymorph of cinnabar (trigonal structure) is also sometimes found (Warr, 2021). It occurs with cinnabar in Hg deposits and is associated with native Hg and a host of other sulphide minerals (HM, 2001 - 2005). Mercury readily combines with Cl, S, and other elements, and subsequently weathers to form inorganic salts (O’Connor et al., 2019; US EPA, 2021a). Calomel, a Hg chloride mineral with formula Hg₂Cl₂ also occurs as a secondary mineral which forms as an alteration product in some Hg deposits (HM, 2001 - 2005).
2.5 Soils
Interest in soil Hg research has been growing over the last few years (O’Connor et al., 2019), a development, which according to Olson et al. (2022), is driven by the importance of soil pools within the broader global Hg cycling frame. Comprehensive and quantitative estimates of Hg in soil pools are needed. The paucity of data on Hg cycling in regional soil pools, according to Olson et al. (2022), is attributed mainly to the heterogeneity of soils and the difficulty often experienced in their sampling, factors that pose challenges in obtaining accurate pool estimates, especially as spatial scale increases. As a result, reliable sampled soil data, which are needed for critical scientific applications such as policy formulation and remediation efforts, are rarely available beyond the local level.
Mercury is naturally present in soils at concentrations (given in earlier estimates) ranging between 0.003 and 4.6 ppm (Steinnes, 1997)—but in most cases, it is present below 0.5 ppm (Schluter, 1993); whereas more recent estimates give, for contaminated sites, concentrations in the range of 11,500 to 14,000 ppm (Gray et al., 2002; Nekulita et al., 2005; Gworek et al., 2020; Morosini et al., 2021; Frey et al., 2022). An earlier (1970) average value of 100 ppb, and range, 30–500 ppb, were given for the Hg content of typical soils by USGS, who also noted that these values vary within relatively narrow limits (USGS, 1970). Soil concentrations of Hg are extremely high in the vicinity of HgS deposits (See, e.g., Gray et al., 2015; Samaniego et al., 2020). It is estimated that in such highly contaminated soils, generally less than 2% of the Hg present is available for plants (See, e.g., Dago et al., 2014), with maximum Hg accumulation generally decreasing in the order: root, stem and leaf (See: Khan et al., 2015).
2.6 Plants
According to earlier estimates by the USGS (1970), most plants are likely to contain less than 500 ppb of Hg. Terrestrial plants, just like aquatic organisms, absorb minor elements (including Hg) from the soils in which they grow at rates depending on various pedological aspects as well as the genetic characteristics of the plants. Unlike aquatic organisms, there seems to be little tendency for terrestrial plants to concentrate Hg above background levels. In terrestrial ecosystems, plant foliage is known to play a vital role in accumulating Hg from the atmosphere and transferring it to soils (Sun and Branfireun, 2022).
There are certain plants referred to as “hyperaccumulators” belonging to unrelated families, and able to grow in metalliferous soils, accumulating very high levels of toxic metals in their aerial parts, far above the levels found in the majority of species without showing any phytotoxic effects (Pasricha et al., 2021; Chamba-Eras et al., 2022). These plants, according to Rascio and Navari-Izzo (2011) are characterised by three basic traits: “a strongly enhanced rate of heavy metal uptake, a faster root-to-shoot translocation and a greater ability to detoxify and sequester heavy metals in leaves.” Skuza et al. (2022) review the natural plant mechanisms by which increased toxic metal accumulation and tolerance are attained as well as factors that influence the plant hyperaccumulator capacity. The hyperaccumulator Cardamine violifolia, for example, was found to accumulate Hg in its roots and above ground parts at concentrations of up to six million ppb (Cui et al., 2022), and is considered to be a useful plant for phytoremediating Hg-contaminated farmlands.
It is important to be able to quantify global fluxes of Hg in vegetation, because, up to two-thirds of terrestrial Hg emissions are deposited back onto land, largely through vegetation uptake of Hg (Zhou et al., 2021). Thankfully, a number of recent studies (e.g., Gworek et al., 2020; Feinberg et al., 2022; Fisher et al., 2022) have been conducted on Hg flows in the various vegetation compartments; though more needs to be done to better constrain vegetation uptake mechanisms in order to understand vegetation cycling. Modelling of vegetation Hg cycling using improved input data would enable more precise quantification of global vegetation impacts.
2.7 Food crops and other major dietary sources
The Provisional Tolerable Weekly Intake (PTWI) of Hg for adults recommended by the European Environment and Health Information System (ENHIS, 2007) in 2007 is 0.0016 (ppm) body weight (not isolated dietary intake value).
The content of Hg in most foodstuffs is normally below the level of detection [usually 20 ng/g (20 ppb)] fresh weight (WHO, 2000).
Fish and marine mammals are known to be among the primary sources of Hg worldwide (e.g., Evers and Sunderland, 2019), Hg being present in the form of methyl Hg compounds (70%–90% of the total) (WHO, 2000). Despite this knowledge, there is a glaring data deficit on Hg levels in fish and other forms of seafood (Capodiferro et al., 2022; Basu et al., 2023), which underlines the importance of collecting site-specific Hg abundance data in fish and other forms of seafood from around the world, as Hg levels in fish vary widely across and within species (See: UNEP, 2019a). Mercury content of edible fish tissues for various species can vary from 50 to 1,400 ppb fresh weight (IPCS, 2003; Raihan et al., 2020), depending on factors such as pH, the redox potential of the water and the species, age and size of the fish (WHO, 2000). However, Hg content of fish from contaminated aquatic environments can be as high as 10 ppm (IPCS, 2003)
Though consumption fish and other seafoods is the most well-known dietary predictor of Hg biomarker concentrations, a number of researchers, e.g., Wells et al. (2020) consider the consumption of wine, rice, vegetables/vegetable oil, or liquor to be potential contributors to Hg toxicity, especially among non-seafood consumers.
2.8 Natural waters
Mercury content in rainwater generally falls within the range of 0.005–0.1 ppb, but mean levels as low as 0.001 ppb have been reported (IPCS, 1990). Rain washes Hg from the atmosphere just as it does certain other atmospheric components.
Naturally occurring levels of Hg in groundwater and surface waters are generally less than 0.5 ppb, although in areas where we have Hg mineralisation (HgS) higher levels are usually encountered. A lower value of less than 0.1 ppb was given by USGS (1970) for surface waters, barring the influence of recent anthropogenic pollution or extraneous geological conditions. This figure reflects the relatively low concentration of Hg in rainwater and the relatively tight bonding of Hg in organic and inorganic materials over which the water passes in its environmental cycling (USGS, 1970). More recently, Siudek et al. (2015) reported mean values of rainwater of 8.3 ng L−1 (0.0083 ppb) with standard deviation of ±3.7; and related the distribution of Hg species to both the emission source and the atmospheric processes [See Section 2.10 (Air)], this article.
Higher concentrations of Hg are generally found in groundwaters because of the longer contact times with mineral grains, as well as the influence of other geo-environmental factors.
In the study of bays by Soerensen et al. (2017), MeHg was found to represent from only 4%–4.5% of total Hg in freshwater, in general; and only 2.1% in offshore saltwater. In 2014, Lamborg et al. estimated the total amount of anthropogenic Hg in the global ocean to be 290 ± 80 million moles, about two-thirds of which resides in water shallower than a thousand metres.
Earlier on, in 1983, Nishimura et al. (1983) had determined the total Hg content of 342 seawater samples collected in the Bering Sea, North and South Pacific, Japan Sea, East and South China Seas, and Indian Ocean, and obtained concentrations of “total” Hg ranging from 0.003 to 0.006 ppb with an arithmetic mean of 0.0053 ppb, and a geometric mean of 0.005 ppb, for 70 percent of the total number of samples analysed.
2.9 Drinking water
Mercury in drinking water is usually in the range 0.005–0.1 ppb (the same range as for rainwater), the average value being about 0.25 ppb (IPCS, 1990). The forms of Hg occurring in drinking water are not known with certainty, but in unpolluted drinking water, most of the Hg is thought to be in the form of inorganic Hg (Hg2+) (See, e.g., WHO, 2005); but this amount is unlikely to engender direct risk to humans, in terms of the maximum allowable concentration (MAC) for drinking water set by recognised institutions such as US EPA (cf., MAC of 2 ppb, US EPA, 2021b). However, certain individuals who drink water containing Hg2+ considerably above the MAC for many years could experience a variety of symptoms, which according to Teixeira et al. (2018) could include oxidative stress as well as necrosis and apoptosis processes, which kill cells. The cell death is considered the main factor responsible for fine motor control changes (Figure 1).
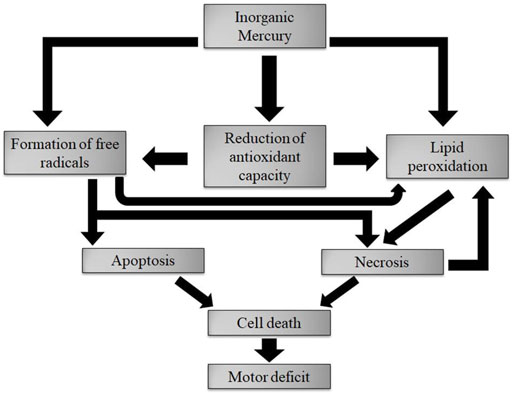
FIGURE 1. Long term effects of excessive amounts of inorganic Hg. Reproduced from Teixeira et al. (2018), Figure 10.
2.10 Air
The primary transport pathway of Hg emissions is the atmosphere, whereas the redistribution of Hg in terrestrial, freshwater, and marine ecosystems and the production of MeHg are driven by land and ocean processes (Drisscol et al., 2013). The range of Hg concentrations in air has been put at 2–10 ng/m3 by WHO (2003). Wohlgemuth et al. (2020) have given average concentration ranges of Hg in Basel, Switzerland, of from 2.0 to 10.8 ng m−3 indoors and from 1.8 to 2.5 ng m−3 outdoors, indicating that households are a net source of Hg to the urban atmosphere and exceed outdoor Hg levels by a factor of two on average.
Because of the tendency of Hg to vaporise, Hg in the atmosphere measured at ground level near Hg ore deposits (cf., HgS) may contain as much as 20,000 ng/m3 of Hg in air (USGS, 1970). This airborne mercury can fall to the ground in raindrops, in dust, or simply due to gravity (known as “air deposition”).
Few data are available on the speciation of Hg in the atmosphere, but practically all of the Hg emitted or re-emitted into the atmosphere is thought to be gas phase elemental Hg, with very small amounts of gas phase Hg2+ compounds and particulate bound Hg (II) (Friedly et al., 2003; ATSDR, 2022).
The amount of Hg deposited in any particular area depends on the amount of Hg that is released from local, regional, national, and international sources. Siudek et al. (2015) attributed elevated Hg concentration in rainwater and, consequently, higher deposition ratio, to the effect of intensive anthropogenic emissions (domestic heating) and/or photochemical reactions.
3 Associations (synergism/antagonism)
Since elemental Hg is a liquid at room temperature, its gas phase is very important geochemically, for some of its compounds have relatively high vapour pressures.
Mercury is a chalcophile element (having affinity for a sulphide phase) and extremely active biologically. A complex relationship exists between Hg and Se (also primarily chalcophilic in character) with reference to toxicity effects (Zampetti and Brandt, 2023), with co-exposure leading to reduced toxicity (antagonism; See, e,g., Oliveira et al., 2017; Tinggi and Perkins, 2022), enhanced toxicity (synergism), or no effect at all (See: Wyatt et al., 2016). Different interactions may be related to chemical speciation, concentration, and method of administration (Dang and Wang, 2011); but these have not been thoroughly studied.
According to Timmerman and Omaye (2021), it is likely that the toxicity of Hg can result in Se deficiency, in that the affinity of Hg for Se is strong enough to cause deactivation of Se-dependent enzymes and proteins. Conversely, Se compounds can have prophylactic or antidotal effects, whereby the adverse toxicity action of Hg exposure can be prevented or reversed (Timmerman and Omaye, 2021). According to Gochfeld and Burger (2021) the high mutual binding affinity of Hg and Se is the reason that each can reduce the toxicity of the other. This is an area that needs further extensive research, as results obtained so far have been inconclusive.
Other metals/metalloids shown to have synergistic and/or antagonistic relationship with Hg include - Cr: e.g., synergistic action with Hg on fish (See: Selvan et al., 2012; Dwivedi et al., 2012); As: e.g., synergistic action in attenuating canonical and non-canonical NLRP3 inflammasome activation (See: Ahn et al., 2018); Pb: combined exposure (synergism) to Pb, inorganic Hg and MeHg showing deviation from additivity for cardiovascular toxicity in rats (See: Dwivedi et al., 2012) and Ni: synergistic effect of Ni and Hg on fatty acid composition in the muscle of fish Lates calcarifer (See: Senthamilselvan et al., 2016).
4 Chemical forms or speciation in soil and aqueous phases
Before working out the degree of mobility, availability, and toxicity of Hg it is of critical importance to determine its speciation (See: Amde et al., 2016; Natasha et al., 2020). In nature, Hg exists in three oxidation states, viz., metallic or elemental Hg (Hg°), mercurous ion (Hg2 2+), and mercuric ion (Hg2+). These oxidation states determine the properties and behaviour of Hg in the environment as well as in metabolic processes in humans, other animals and plants. Inorganic Hg1+ species can also occur but are generally believed to play an inconsiderable role in natural systems; it is found mainly as shorter-lived intermediates in redox cycling between Hg° and Hg2+ species.
All three forms of Hg convey some degree of toxicity to life forms - including humans - but it is the Hg compounds containing the mercuric ion that are the most toxic, especially the organic-mercury compound, methyl mercury (CH3Hg+) or simply, MeHg.
Mercury has seven stable isotopes, making it unique among the heavy elements - these isotopes are: Hg196, Hg198, Hg199, Hg200, Hg201, Hg202 and Hg204, and they span a relative mass difference of 4%. On this score, Bergquist and Blum (2009) recognised the great promise held by Hg isotope systematics - both mass-dependent (MDF) and mass-independent fractionations (MIF) - for tracking this toxin’s geochemical transformations, and by extension, its adverse metabolic interactions (See Section 8, on: “Metabolic function (clinical toxicity)”, this article. As of today, however, we have only just begun exploring the potential of using Hg stable isotope signatures to provide new insights into Hg biogeochemistry (AuYang et al., 2022; McLagan et al., 2022; Ecley et al., 2023).
5 Environmental circulation and impacts of climate change
A lot has been written about the biogeochemical cycling of Hg in terms of our understanding of several aspects of the cycle, such as oxidation processes in the atmosphere, land-atmosphere and ocean-atmosphere cycling, and methylation processes in the ocean. Some of the more recent accounts include those of Gustin et al. (2020), Chételat et al. (2022) and Wang et al. (2022).
Most of the Hg that enters the atmosphere (from mining deposits of ores that contain Hg, from the emissions of coal-fired power plants, from burning municipal and medical waste, from cement production, and from uncontrolled releases in factories that use Hg), is in the form of metallic (Hg°) and inorganic compounds (ATSDR, reviewed 2015). The fate of Hg after it is emitted depends on several factors such as: the form of Hg emitted, location of the emission source, the height above the landscape from which the Hg is emitted (e.g., the height of a power-plant stack), the surrounding terrain, and the weather. Redeposition of Hg0 mainly as Hg++ (because Hg0 is oxidised in the atmosphere) takes place on terrestrial surfaces by dry deposition, including foliar uptake, sorption, and gas dissolution (Enrico et al., 2016). The ultimate deposition of Hg, probably as HgS ore, is believed to be in ocean sediments (Sanei et al., 2021; Zaferani and Biester, 2021), where part of the inorganic Hg emitted becomes oxidised to Hg++ and then methylated or in other ways transformed into organomercurials (WHO, 2000; Ignatavičius et al., 2022).
Some of the effects of climate change on the environmental perturbations of Hg include: its impact on Hg deposition, viz., a synchronous change in global Hg deposition as reported by Li et al. (2020); changes in cycling of Hg, engendering an increase in MeHg and hence increased contamination (See: Fahnestock et al., 2019; Zhou et al., 2021; Chai et al., 2022; Chételat et al., 2022; Wang et al., 2022).); and increase in Hg uptake in fish and marine mammals in regions experiencing glacial retreat (See, e.g., Wang et al., 2020; McKinney et al., 2022).
6 Mobility, mode of entry into food chains and bioavailability
The solubility, mobility as well as availability of Hg in soils are quite low, and dependent primarily on factors such as soil texture, the amount of organic matter and soil pH (See, e.g., Różański et al., 2016; Gworek et al., 2020). A wide array of physical, chemical, biological and isotopic exchange methods has been developed to estimate the (bio)-available pools of Hg in soil in an attempt to offer a plausible assessment of its risks. Unfortunately, many of these methods do not mirror the (bio)-available pools of soil Hg and suffer from technical drawbacks (Huang et al., 2020).
The main reservoir of Hg in aquatic environments appear to be sediments, which may also serve as a source of Hg to aquatic food webs (See: Jonsson et al., 2022). In aqueous systems and anoxic sediments, the geochemical forms of Hg (and subsequent bioavailability) are controlled primarily by reactions between Hg (II), inorganic sulphides, and natural organic matter (Hsu-Kim et al., 2013). Inorganic Hg compounds are, in general, water soluble with a bioavailability of 7%–15% after ingestion (Park and Zheng, 2012).
Plants differ (from species to species) in their ability to take up Hg and can develop a tolerance to high concentrations on contaminated sites, with corresponding elevated concentrations in edible parts compared with natural soils (Kabata-Pendias and Mukherjee, 2007).
Studying the process of methylation of inorganic forms of Hg constitutes the first step in understanding the process of bioaccumulation of MeHg in aquatic food webs (Figure 2). Because the process is primarily mediated by anaerobic bacteria (Hsu-Kim et al., 2013) knowledge about the bioavailability of inorganic Hg to these microbes is important in understanding of MeHg bioaccumulation in fish and other high trophic-level species (See, e.g., Li et al., 2021).
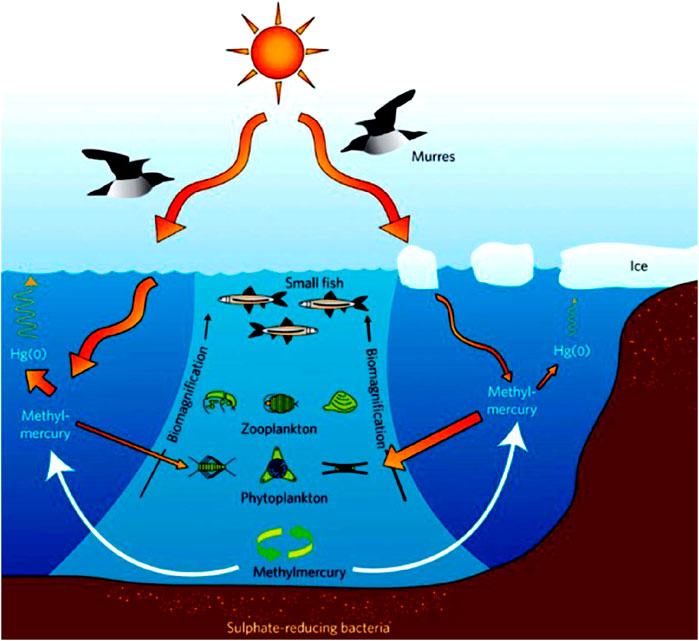
FIGURE 2. Marine Hg breakdown. Reproduced from Blum, (2011), with permission from Springer Nature.
As already stated, the dominant route of exposure of humans and other higher order wildlife to MeHg is through the ingestion of fish and seafood products, with most fish - both freshwater and saltwater - containing varying levels of MeHg, which can enter and accumulate in the food chain (Ullrich et al., 2001; Al-Sulaiti et al., 2022). Although Hg is not known to be an essential part of the food chain, it is assimilated by organisms living in environments which contain it.
7 Absorption and distribution
The absorption, transport and distribution of Hg are dependent on the Hg species. The main entry route of elemental Hg (Hg0) into the body is inhalation, with up to 80% of the inhaled vapours directly entering the bloodstream (Martinez-Finley and Aschner, 2014). Absorption of elemental Hg by the gastrointestinal tract, however, is weak (less than 0.01% in rats) (WHO, 2000; Fowler and Zalups, 2022; US EPA, 2022). Upon absorption, elemental Hg enters all tissues and accumulates in the central nervous system and kidneys. Inorganic mercury (HgS) has a lower liposolubility, engendering lower organism absorption and limited passage through the blood-brain barrier (Lohren et al., 2016).
Oral exposure is the primary route for inorganic Hg salts (Park and Zheng, 2012). Mercury absorption from oral ingestion also depends on the form of Hg. Absorption and accumulation of Hg in its metallic form have already been described at the beginning of Section 7 (this Section).
Dermal penetration is usually not a significant route of exposure to inorganic Hg (Bjørklund et al., 2019; Tincu et al., 2022).
The main route of absorption of organic Hg (MeHg) is the GI, but some absorption of MeHg through the skin and the lungs also occurs (Posin et al., 2022).
Once absorbed, the majority of Hg2+ accumulates in the kidney and liver (Martinez-Finley and Aschner, 2014; Zhao et al., 2022). Subsequent to absorption into the circulation, MeHg enters erythrocytes where a substantial amount binds to haemoglobin and a lesser amount to plasma proteins. A small amount of Hg (some 10% of the burden of MeHg) gets to the brain where demethylation to an inorganic Hg form proceeds slowly. Methylmercury readily crosses the placenta to the foetus, where deposition within the developing foetal brain can take place (See: ATSDR, reviewed 2015; Bjørklund et al., 2019) with serious neurological consequences for the newborn, though other recent studies (e.g., Dack et al., 2022) have ruled out the likelihood of dietary Hg exposure during pregnancy being a risk factor for low neurodevelopmental functioning in early childhood.
8 Metabolic function (clinical toxicity)
The toxicity of Hg is strongly influenced by microbially mediated biotransformation between its organic (MeHg) and inorganic [Hg (II) and Hg0] forms.
8.1 Mechanism of toxicity
Several mechanisms have been invoked in the study of Hg toxicity. These include alteration or disruption in the regulation of intracellular Ca homeostasis, cytoskeleton, mitochondrial function, oxidative stress, neurotransmitter release, and DNA methylation (ATSDR, 2022) with Hg binding to thiolate anions thought to underlie many of these alterations or disruptions. Once absorbed, elemental and inorganic Hg enter an oxidation-reduction cycle. Mercury is absorbed in the elemental state and is oxidised to the mercuric form (Hg++) in the red blood cells and tissues, in a process that takes only minutes (Park and Zheng, 2012; Abdullah, 2020).
According to Rand Lab, URMC (2022, evaluating the potential for MeHg toxicity relies on the accurate prediction of the body burden of Hg that results from eating fish (Figure 3). As already indicated, exposure to MeHg through the consumption of seafood and fish can seriously affect foetal neurobehavioral development (i.e., motor impairment) even when MeHg levels in maternal blood are quite low, say around 5 μg/L, which the mother tolerates well (See: Bjørklund et al., 2022).
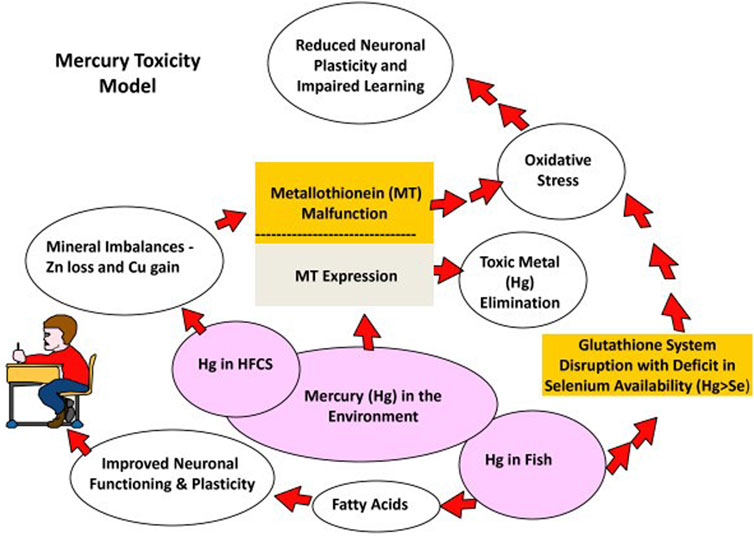
FIGURE 3. Mercury toxicity model. Reproduced from Dufault et al. (2009), Figure 1.
The Hg body burden is directly determined by the slow elimination kinetics of MeHg in the human body (t1/2–50 days) (Rand Lab, URMC, 2022). Existing studies are limited in size and number, but sufficient evidence now exists to show that the biological half-life of MeHg in humans can vary widely (t1/2 = 30 to >150 days).
As of 2022, the Rand Lab, URMC studies continue to characterise MeHg metabolism and kinetics in people, focussing on the most vulnerable populations, viz., pregnant women and young children. In parallel, these researchers are investigating the gut microbiome in more depth for its role in the biotransformation process. Rand Lab, URMC data are being used to inform development of a physiologically based pharmacokinetic (PBPK) model in silico capable of predicting MeHg kinetics in humans (Rand Lab, URMC, 2022).
Bjørklund et al. (2022) summarise current knowledge on the mechanisms of MeHg toxicity during the period of nervous system development and give examples of herbal substances with neuroprotective effects that can be utilised for development of natural drugs for mitigating neurotoxicity symptoms caused by MeHg or other Hg compounds. Pan et al. (2022) review current status of research on neurodevelopmental toxicity of MeHg exposure during pregnancy and the molecular mechanisms of related pathways.
Impairment of extra-cerebellar functions can result from the actions of MeHg on thiol groups in the neuronal cytoskeleton as well as on mitochondrial enzymes and induction of disturbances of glutamate signalling (Bjørklund et al., 2022). Antunes dos Santos et al. (2018) review the general interactions between MeHg with regulators of the antioxidant response system that are rich in thiol and selenol groups such as glutathione (GSH), and the selenoenzymes thioredoxin reductase (TrxR) and glutathione peroxidase (Gpx).
In experiments to decipher the molecular mechanism of MeHg-induced pancreatic β-cell cytotoxicity, Yang et al. (2022) found that the induction of ROS-activated JNK (c-Jun N-terminal kinase) signalling is a crucial mechanism in MeHg-induced mitochondria- and ER stress-dependent apoptosis, ultimately leading to β-cell death.
9 Supplementation
Mercury is not classified as an essential element and no known benefits have been reported for life processes in animals and plants.However, a number of studies have revealed the presence of Hg in significant quantities in herbal dietary supplements and in several therapeutic preparations (See, e.g., Puścion-Jakubik et al., 2021; Brodzial-Dopierala et al., 2018), such as to warrant further research that would possibly lead to implementation of a stricter quality control of dietary supplements. The use of Hg in traditional medicine is also becoming widespread (See, e.g., Street et al., 2015).
Reported therapeutic uses appearing in the literature include various medications, ointments, dental fillings, contact lenses and cosmetics. A number of instruments for medical applications such as thermometers and sphygmomanometers also make use of Hg (See: Iqbal and Asmat, 2012), though a number of healthcare institutions and clinicians worldwide have been calling for a replacement of these instruments even before the year 2010 (See, e.g., Buchanan et al., 2011; WHO, 2015; Asayama et al., 2016).
10 Reduction of body burden/elimination
Excretion of Hg takes place primarily through the urine and faeces, with smaller amounts leaving the body through exhaled breath, sweat, and saliva.Following short-term exposure to Hg vapour [elemental Hg (Hg°)], about a third of the absorbed Hg will be eliminated in unchanged form through exhalation, while the rest will be eliminated mainly through the faeces (Ishihara, 2000; Abass et al., 2018; Paduraru et al., 2022).
Another form of excretion, albeit an undesirable one, is through lactation (ATSDR, reviewed 2015). Wu et al. (2022) have found that AnGongNiuHuang Pill (AGNHP) (a Hg-containing traditional Chinese drug for the treatment of “febrile disease”) increased blood Hg absorption and reduced urinary Hg excretion. Other studies, e.g., the one by Li et al. (2012) have noted that organic Se supplementation could increase Hg excretion and decrease urinary malondialdehyde and 8-hydroxy-2-deoxyguanosine levels in local residents. For synergistic/antagonistic relationships between Se and Hg, see Section 3: “Associations (synergism/antagonism)”, this article.
11 Analytical determination
Mercury concentrations in humans and other animals have been determined in blood, urine, body tissues, hair, breast milk, and umbilical cord blood. Most of the earlier methods used atomic absorption spectrometry (AAS), atomic fluorescence spectrometry (AFS), or neutron activation analysis (NAA), although mass spectrometry (MS), spectrophotometry, and anodic stripping voltammetry (ASV) have also been employed. The most commonly used method, pre-2000s was cold vapour (CV) AAS introduced in 1968 by Hatch and Ott (1968). As this technique (CV AAS) went through further development, it became possible to reliably determine Hg concentrations below the ppb level (>76% recovery) through either direct reduction of the sample or reduction subsequent to pre-digestion (See: Risher, 2003).
Today, the concentration of Hg can be accurately determined in air, water, soil, and biological samples (blood, urine, tissue, hair, breast milk, and breath) by a variety of analytical methods. Most of these methods are for total Hg (inorganic as well as organic Hg compounds) and are based on wet oxidation followed by a reduction step. However, relatively modern methods also exist for the separate determination of inorganic Hg compounds and organic Hg compounds (See, e.g., Risher, 2003).
There continues to be a huge and continuous interest in improvements on the determination of Hg and its speciation, mainly due to the element’s high toxicity and biomagnification (Liem-Nguyen, 2016). Developments in analytical procedures for Hg, its speciation analysis and its applications in environmental and biological studies since the year 2008 were extensively reviewed by Gao et al. (2012). Despite these relatively recent advances in analytical techniques, interpretation of Hg geochemistry in environmental systems still remains a challenge (See, e.g., McLagan et al., 2022). This is largely due to our inability to identify specific Hg transformation processes and species using established analytical methods in Hg geochemistry (total Hg and Hg speciation). Recently, Yu et al. (2019) and Favilli et al. (2022), respectively reviewed and critically examined different approaches for the quantification of Hg species in different samples using HPLC-ICP-MS.
Further improvements in analytical techniques for determining Hg in biological and environmental samples are now particularly focussed on the attainment of lower detection limits and ease of operation of analytical instrumentation, as exemplified in the following overview which encapsulates selected works that represent these developments:
• In 2022, Santos et al. (2022) described a simple and cost-effective wet digestion method for the determination of total Hg in samples of small plankton material using a cold vapor atomic fluorescence spectroscopic (CV AFS) technique.
• Ignatavičius et al. (2022) used chemical vapour generation, inductively coupled plasma mass spectrometry, to develop a simple and quick method for measuring MeHg.
• Suárez-Criado et al. (2022) have compared the GC-ICP-MS, GC-EI-MS and GC-EI-MS/MS methods for the determination of methylmercury, ethylmercury and inorganic Hg in biological samples by triple spike species-specific isotope dilution mass spectrometry.
• Alzahrani et al. (2023) determined the concentration of Hg(II) ions in the presence of the Schiff base ligand, 2-((5-(2-hydroxy-3-methoxybenzylideneamino)–2H-1,2,4-triazole-3-ylimino)methyl)-6-methoxyphenol (HMBT), at pH 10 using the so-called Briton Robinson Buffer.
12 Current status of geomedical research on mercury toxicity in Africa
In this section, a brief mention is made of selected recent geomedical research on Hg toxicity in Africa, focussing on work done in the last two decades (post-2000), or so. Most of the research on Hg exposure in Africa has been done in South Africa, despite the existence of multiple sources of Hg pollution in several other African countries. Much of this research centres around the impact of Hg exposure during alluvial Au mining, which is also a common activity in a number of countries across the Continent. A few selected examples follow:
Bonzongo et al. (2004) present a case study on health impacts due to Hg exposure in artisanal Au mining in Ghana, West Africa. Oosthuizen et al. (2010) studied Hg exposure in a low-income community in South Africa associated with Au mining activities to determine whether significant exposure to Hg occurs. Spiegel (2009) looked at socio-economic dimensions of Hg pollution abatement in artisanal mining communities in Sub-Saharan Africa; and in 2013, Lusilao-Makiese et al. (2013) assessed the impact of historical use of Hg for the extraction of Au in watersheds from an abandoned mine in Randfontein, a town located some 45 km west of Johannesburg (South Africa).
In 2007, Learner (2007) reported on the development of the South African Mercury Assessment (SAMA) Programme during the Stockholm Water Week that year. The research areas addressed in the SAMA Programme included: “(a) regulatory framework; (b) analytical methods; (c) source, speciation, fate, and transport; and d) impacts (ecological and human health)”. In 2010, Papu-Zamxaka et al. (2010) looked at the consequences of Hg contamination in the vicinity of a Hg processing plant in the KwaZulu-Natal Province of South Africa.
In 2011, Walters et al. (2011) reviewed the status of Hg as a pollutant in the aquatic ecosystems of South Africa.
In 2011, AJUA Environmental Consultants prepared on behalf of the Department of Environmental Affairs of South Africa, a report entitled: “Situation analysis of mercury in South Africa: Development of an inventory of mercury uses and sources”. Mercury release calculations used UNEP (2011) Toolkit level 1 (revised January 2011) were based on the mass balance principle, where the quality of input data impinged strongly on the system outputs. A further review of this ‘Toolkit’ appeared in 2019 (UNEP, 2019b). In 2014, Hanna et al. (2015) compiled published data on Hg contamination in African freshwater fishes, invertebrates, and plankton, and examined factors that may be responsible for concentrating Hg in these organisms. Despite revealing strong trends in Hg concentration, the work revealed a marked paucity of data on a number of other aspects of Hg dynamics in Africa.
The work of Street et al., in 2015 looked at the perceptions and practices in the use of metallic Hg by South African traditional health practitioners, stressing the need for putting in place, public education messages and regulatory measures.
In 2015, Bosch et al. (2016) outlined gaps in the then current knowledge and research on the accumulation of metal contaminants including Hg in commercially consumed marine fish worldwide and especially in South Africa, that affect both the fishing industry and fish consumers.
In 2016, Garnham and Langerman (2016) calculated the amount of Hg emitted from each of the South Africa Electricity Supply Commission (Eskom) coal-fired power stations, based on the amount of coal burnt and the Hg content in the coal. These authors calculated Emission Reduction Factors (ERF’s) from two sources, in working out the co-benefit derived from the emission control technologies at the stations.
In 2019, Belelie et al. (2019) performed a pilot study to characterise ambient total gaseous Hg concentrations over the South African Highveld (a portion of the South African inland plateau). In the same year (2019), Panichev et al. carried out large-scale assessment of atmospheric air pollution by Hg using the lichen Parmelia caperata as biological indicator. In the study by Panichev et al. (2019), five provinces of South Africa were sampled between 2013 and 2017; and the lichens analysed to provide time-integrated data, which correspond to the mean Hg concentration in air at a specific location over a long time period. Nevondo et al. (2019) suggested that Hg coming from contaminated groundwater from landfill leachate seepage could carry significant amounts of Hg, especially in landfills that are not lined with a geomembrane.
Bieser et al. (2020) investigated sources and sinks of Hg at Cape Point, South Africa by combining atmospheric Hg data with a trajectory model; and found that continent is the major sink, and the ocean, especially its warm regions (i.e., the Agulhas Current), is the major source for Hg.
Astolfi et al. (2020) used Hg in hair as biomarkers for understanding of Hg exposure in a population living in Asmara, capital of Eritrea; and to evaluate the influence of certain demographical factors on the Hg levels in hair. These authors (Astolfi et al. (2020)) found hair Hg concentration to be significantly higher among women compared to men (p < 0.001), underlining once again the need for routine biomonitoring surveys and the promotion of health education by municipal authorities, decision makers and workers.
Bredenkamp’s 2021 ‘Thesis’ (North-West University, South Africa) pointed out the paucity of long-term measurements of atmospheric Hg at inland background sites in South Africa; and held that such measurements, in conjunction with data from the coastal CP GAW (Cape Point Global Atmosphere Watch) station will be essential in providing credible estimates on background atmospheric Hg concentrations in South Africa; as well as contribute to formulation of cogent policies and standards on Hg emission limits.
In 2022, Elumalai et al. (2022) performed a chemometric analysis of exposure and human health on tourist beaches in Durban, South Africa, coming up with observations that lend credence to the criticality of erecting long-term monitoring schemes for Hg in order to provide requisite data for formulating improved public health management strategies. The emergence of relatively new techniques such as Hg stable isotopes now enables us perform more accurate biomonitoring exercises (See: Li et al., 2022).
Ansah et al. (2022) constructed a model for Hg in soil and Hg leaching to waters for use in assessing present and future pollution levels for the environment in Ghana, a country where artisanal small scale gold mining (ASGM) activities are intense, and the use of Hg in the extraction process, widespread. The model, which according to Ansah et al. (2022), can be run with a 5 × 5 km2 scale resolution and a yearly or monthly time-step; and is considered to be an important tool for policy support in future regulation of the mining sector.
A noteworthy case description can be given of Cato Ridge, a small industrial village in the KwaZulu Natal Province of South Africa. Mercury waste shipments from other countries have been received at a Cato-Ridge plant owned by Thor Chemicals in South Africa, a subsidiary of Thor Chemicals, Inc. of Great Britain (See, e.g., Phalane and Steady, 2009). Investigation into the international shipment of hazardous wastes has focused attention on the consignment and its delivery to South Africa. This Firm has been accused of poisoning workers and putting surrounding communities at risk from Hg exposure. As of December, 2,000, the Hg wastes were still stockpiled at Thor Chemicals, Inc., in Cato-Ridge. Research on the fate of residual Hg at Cato-Ridge is perhaps a worthwhile proposition.
13 Other suggested areas for further research
Mercury pollution is a global problem, not least in the Continent of Africa. Despite the prolificity of research in the past two decades or so, there are still several uncertainties and variabilities in our knowledge of both the element’s exposure dynamics and its health effects.
Understanding the intricacies of the element’s emissions-to-impact path is rendered intractable by its varied environmental fate and the overarching influences of environmental, geological/geochemical, biological and socioeconomic drivers (See: Budnik and Casteleyn, 2019). This knowledge is vital as it carries the potential of swaying the course of diagnosis and treatment proffered by medics for Hg-induced maladies. In this context, the use of simulation modelling techniques is thought to carry a high potential for eliminating or reducing some of these knowledge gaps.
Here, a list comprised of key uncertainties, future research needs, and recommendations is presented to assist researchers in readily identifying important gaps in knowledge in the various intricacies of Hg exposure and its outcomes. A comprehensive list of the more recent references is given, to aid the search process of those researchers who seek additional information on areas not covered in this article.
• The evaluation of the content of Hg in the Earth’s crust as well as in the different rock types is critical in any simulation modelling of the elements’ source-emission-impact path. Many Hg simulation models still use Hg concentration values in source rocks that were determined prior to the 2000s (e.g., Turekian and Wedepohl, 1961; Jonasson and Boyle, 1972), when instrument detection limits and operating parameters were not yet at their optimum; nor were techniques for data interpretation and compilation at their most refined.
• The accuracy of Hg content in source rocks as input data in Hg simulation modelling is important in determining the validity of model output and the conclusions reached. The dearth of modern analytical data on Hg content of source rocks for simulation modelling and other studies of Hg toxicity remains unsettled and awaits further research.
• Attempts at controlling Hg emissions have been on-going since the mid-1980s. However, we do not know whether reductions achieved by some countries have been offset by the increase in emissions from rapidly industrialising countries. In addition, a major challenge facing scientists now is to ascertain links between Hg in the atmosphere, deposition, and ecosystem contamination (See: Pirrone et al., 2010; Sundseth et al., 2017).
• Interpretation of Hg geochemistry in environmental systems continues to be problematic (See, e.g., McLagan et al., 2022). This is largely due to our inability to identify specific Hg transformation processes and species using modern analytical tools in Hg geochemistry (total Hg and Hg speciation) (See Section 11, “Analytical Determination”, this article). Research on these processes is vital to our understanding of Hg speciation, bioavailability, bioaccessibility and distribution of Hg that enters the body.
• Links between fully coupled biogeochemical models with biological interactions in food webs should be unveiled to provide the basis for designing future policies.
• There is an extreme paucity of measurements of reactive Hg worldwide (See: AMAP/UNEP, 2019); in many countries, including those in Africa these measurements are virtually non-existent.
• There is an urgent need for research on the effectiveness of implementation of solutions to reduce the emissions and exposure to Hg as a global environmental pollutant. Mechanisms for testing the efficiency of implementation of the Minamata Convention in Africa and other parts of the world, are still needed. Such research data should be translated swiftly into management tools for local policymakers and health professionals, with particular attention paid to the major differences in Hg contamination across Africa and other parts of the world (See: Budnik and Casteleyn, 2019).
• The Minamata Convention includes provisions to reduce emissions of Hg to the atmosphere with the overarching goal of reducing Hg in the environment. A major setback for scientists and policymakers is the inability to gauge the effectuality of actions put in place by policymakers in response to the Convention, which relies on firm understanding of the element’s sources, distribution, transformation and fate.
• Many faunal groups are clearly vulnerable to Hg contamination, but the myriad of relationships existing between Hg and faunal biology remains poorly construed (See, e.g., Seewagen, 2010). From a conservation standpoint, we need a proper understanding of the threats facing faunal populations. The taxonomic groups, geographic ranges, life history stages, habitat associations, and foraging behaviours of several fauna that are significantly threatened by Hg pollution need to be more precisely identified. Successful conservation strategies are dependent on a comprehensive understanding of the threats facing populations. Additional research on how Hg is impacting faunal groups will hugely benefit our efforts at conservation.
• As for biota, there is the need to further elucidate the physiological mechanisms that control the accumulation, distribution, and toxicity of Hg by coupling new techniques with Hg stable isotopes (See, e.g., Mi-Ling et al., 2022), taking full cognisance of the fact that in biotic systems, processes do not operate in isolation. Many of the processes affecting the conversion of inorganic Hg to MeHg and subsequent uptake in biota are poorly understood, particularly in marine ecosystems (See, e.g., Hsu-Kim et al., 2013; Lamborg et al., 2014).
• The chemistry of Hg in oceans is still not fully understood, cf., precise mechanisms behind the methylation process. Basic questions remain unanswered regarding the geochemical forms of Hg that persist in anoxic settings, the mode of assimilation by methylating bacteria in the ocean, and the biochemical pathway by which these microorganisms produce and break down MeHg (See: Hsu-Kim et al., 2013). These are questions, whose answers are needed to inform policymakers and develop long-term strategies for controlling Hg contamination.
• Mercury science and policy will likely benefit from furthering the development of Hg research at the food-environment nexus and integrating seafood safety and food security themes and approaches into the framework of the Minamata Convention (See: Pacyna, 2020).
• Recent research has established the uniqueness of the chemical interactions between Hg and Se. The Hg capturing capacity of Se is several times higher than that of S compounds and results in inactive complexes that can be exploited in preventing or reversing the severe toxic action of Hg. Future work can target engineering technology that can exploit the Hg capturing ability of Se in reducing the toxicity of Hg in the environment (See, e.g., Castriotta et al., 2020; Timmerman and Omaye, 2021). The role of technological development in contributing to the reduction of Hg exposure and improvement of environmental responsibility need further study.
• The suggestion by Nevondo et al. (2019) that Hg coming from contaminated groundwater from landfill leachate seepage could carry significant amounts of Hg deserves further research, since several African rural communities living near landfill sites depend on groundwater for drinking purposes. Additional studies to identify pathways of release from contaminated sites to water and air, are needed.
• Since consumption of seafood, particularly fish, has been established as the most common form of human exposure to MeHg, the topic has ignited a surge in research, especially the relationship between maternal fish intake and the health effects on the developing foetus. Despite these research efforts, the Hg concentrations in fish in Africa and in many other parts of the world remain unknown (See, e.g., Pandey and Shrivastav, (2012). There is therefore the need for additional data on Hg content in fish to provide consumers and government regulators with accurate information upon which to base consumption guidelines. A major achievement in the effort to reduce Hg in the environment would be to provide policymakers with reliable data on the relationship between anthropogenic emissions and the resulting concentrations of Hg in seafood eaten by humans.
• The magnitude of uncertainty and variability of Hg and MeHg toxicokinetics in highly-exposed population groups such as pregnant women and children needs further study.
• Abundant evidence now exists to support the thesis that Hg toxicity is complex; hence, Martinez-Finley and Aschner (2014) have proposed that genetic analyses and testing with low-level Hg species could likely unveil new insights into the mechanism of toxicity. Studies (both genetic and behavioural) suggest that the extent of disease may be modulated not only by levels of Hg uptake, but also by age (both of exposure and at testing) and genetic background (Martinez-Finley and Aschner, 2014).
• Further gaps in our knowledge of Hg cycling were pointed out by Cossa et al. (2022), in their work on the Mediterranean Sea, in particular. These gaps include “… temporal variations in air-sea exchange, hydrothermal and cold seep inputs, point sources, submarine groundwater discharge, and exchanges between margins and the open sea.” These authors also pointed out the need for long-term observations and dedicated high-resolution Earth System Models for future assessment of global change impacts under the Minamata Convention Hg policy.
• Innovative and holistic ways of addressing the above “bulleted” issues regarding Hg pollution and health effects will be required within the framework of the Minamata Convention. This is so, because the highly complex nature of Hg cycling and exposure dynamics require that multitiered risk assessments that focus on environmental health and human health separately will need to be brought together.
• As this article was being written (2022/2023), work was still ongoing on evaluating the completeness of our understanding of Hg as a global environmental pollutant; and on the best approaches for developing and implementing solutions to reduce the emissions and exposure to this pollutant. This was the principal mandate of the International Conference on Mercury as a Global Pollutant (ICMGP), the 14th ICMGP which took place in Krakow, Poland in September 2019. Major subjects of discussions at this Conference centred around the improvement of our knowledge on Hg sources, fate, impacts, and emission control options under the broad topic: “Bridging knowledge on global mercury with environmental responsibility, human welfare and policy response”. Sadly, though, at this meeting, it was consensual that global anthropogenic emissions of Hg to the environment were still rising.
Author contributions
The author confirms being the sole contributor of this work and has approved it for publication.
Acknowledgments
Data acquisition and compilation were supported by the Research Directorate of the Mangosuthu University of Technology. Office space and writing facilities were provided by the management and staff of the Gooderson Tropicana Hotel in Durban, South Africa. I am truly grateful to these institutions.
Conflict of interest
The author declares that the research was conducted in the absence of any commercial or financial relationships that could be construed as a potential conflict of interest.
Publisher’s note
All claims expressed in this article are solely those of the authors and do not necessarily represent those of their affiliated organizations, or those of the publisher, the editors and the reviewers. Any product that may be evaluated in this article, or claim that may be made by its manufacturer, is not guaranteed or endorsed by the publisher.
References
Abass, K., Huusko, A., Knutsen, H. K., Nieminen, P., Myllynen, P., Meltzer, H. M., et al. Quantitative estimation of mercury intake by toxicokinetic modelling based on total mercury levels in humans. Environ. Int. (2018) 114:1–11. doi:10.1016/j.envint.2018.02.028
Abdullah, N. K. Mercury in the diet: Absorption and bioaccessibility. Open Access Libr. J. (2020) 7(10):1–15. doi:10.4236/oalib.1106666
Ahn, H., Kim, J., Kang, S. G., Yoon, S., Ko, H-J., Kim, P. H., et al. Mercury and arsenic attenuate canonical and non-canonical NLRP3 inflammasome activation. Sci. Rep. (2018) 8:13659. doi:10.1038/s41598-018-31717-7
Al-Sulaiti, M. M., Soubra, L., and Al-Ghouti, M. A. The causes and effects of mercury and methylmercury contamination in the marine environment: A review. Curr. Pollut. Rep. (2022) 8:249–272. doi:10.1007/s40726-022-00226-7
Alzahrani, L., El-Ghamry, H. A., Saber, A. L., and Mohammed, G. I. Spectrophotometric determination of Mercury(II) ions in laboratory and Zamzam water using bis Schiff base ligand based on 1,2,4-Triazole-3,5-diamine and o-Vaniline. Arabian J. Chem. (2023) 16:104418. doi:10.1016/j.arabjc.2022.104418
AMAP/UNEP (Arctic Monitoring and Assessment Programme/United Nations Environment Programme) (2019). Technical background report to the global mercury assessment 2018. Oslo, Norway, AMAP/UNEP. 1- 430. Available at: https://www.amap.no/documents/download/3409/inline (Accessed 31.12.2022)
Amde, M., Yin, Y., Zhang, D., and Liu, J. Methods and recent advances in speciation analysis of mercury chemical species in environmental samples: A review. Chem. Speciat. Bioavailab. (2016) 28(1 - 4):51–65. doi:10.1080/09542299.2016.1164019
Ansah, E., Bak, J. L., Sørensen, P., and Darko, G. Modelling mercury concentration in Ghanaian soil. Chemosphere (2022) 307(1):135553. doi:10.1016/j.chemosphere.2022.135553
Antunes dos Santos, A., Ferrer, B., Marques Gonçalves, F., Tsatsakis, A. M., Renieri, E. A., Skalny, A. V., et al. Oxidative stress in methylmercury-induced cell Toxicity. Toxics (2018) 6:47. doi:10.3390/toxics6030047
Asayama, K., Ohkubo, T., Hoshide, S., Kario, K., Ohya, Y., Rakugi, H., et al. From mercury sphygmomanometer to electric device on blood pressure measurement: Correspondence of Minamata convention on mercury. Hypertens. Res. (2016) 39:179–182. doi:10.1038/hr.2015.158
Astolfi, M. L., Protano, C., Marconi, E., Massimi, L., Piamonti, D., Brunori, M., et al. Biomonitoring of mercury in hair among a group of Eritreans (Africa). Int. J. Environ. Res. Public Health (2020) 17(6):1911. doi:10.3390/ijerph17061911
ATSDR (Agency for Toxic Substances and Disease Registry). Mercury: Mercury overviews, ToxZine (2015). Available at: https://www.atsdr.cdc.gov/sites/toxzine/mercury_toxzine.html#:∼:text=Most%20of%20the%20metallic%20mercury,body%20in%20the%20exhaled%20breath (Accessed 07 11, 2022).
ATSDR (Agency for Toxic Substances and Disease Registry). Toxicological profile for mercury (draft for public comment) (2022). Available at: https://www.atsdr.cdc.gov/toxprofiles/tp46.pdf (Accessed 07 11, 2022).
AuYang, D., Chen, J., Zheng, W., Lang, Y., Wang, Y., Wang, Z., et al. Seasonal variations of the mercury multiple isotopic compositions of subrural and urban aerosols highlight an additional atmospheric Hg0 oxidation pathway. Front. Environ. Sci. Sect. Biogeochem. Dyn. (2022) 9:773327. doi:10.3389/fenvs.2021.773327
Banks, J., Carson, J. S., Nelson, B., and Nicol, D. M. Discrete-even system simulation. 5th edition. Hoboken, NJ, USA: Prentice-Hall (2010). p. 622.
Basu, N., Bastiansz, A., Dórea, J. G., Fujimura, M., Horvat, M., Shroff, E., et al. Our evolved understanding of the human health risks of mercury. Ambio (2023). Advance online publication. doi:10.1007/s13280-023-01831-6
Belelie, M. D., Piketh, S. J., Burger, R. P., Venter, A. D., and Naidoo, M. Characterisation of ambient total gaseous mercury concentrations over the South African Highveld. Atmos. Pollut. Res. (2019) 10:12–23. doi:10.1016/j.apr.2018.06.001
Bergquist, B. A., and Blum, J. D. The odds and evens of mercury isotopes: Applications of mass-dependent and mass-independent isotope fractionation. Elements (2009) 5:353–357. doi:10.2113/gselements.5.6.353
Bieser, J., Angot, H., Slemr, F., and Martin, L. Atmospheric mercury in the southern hemisphere - Part 2: Source apportionment analysis at Cape point station, South Africa. Atmos. Chem. Phys. (2020) 20:10427–10439. doi:10.5194/acp-20-10427-2020
Bjørklund, G., Antonyak, H., Polishchuk, A., Semenova, Y., Lesiv, M., Lysiuk, R., et al. Effect of methylmercury on fetal neurobehavioral development: An overview of the possible mechanisms of toxicity and the neuroprotective effect of phytochemicals. Archives Toxicol. (2022) 96:3175–3199. doi:10.1007/s00204-022-03366-3
Bjørklund, G., Chirumbolo, S., Dadar, M., Pivina, L., LindhButnariu, M., Aaseth, J., et al. Mercury exposure and its effects on fertility and pregnancy outcome. Basic Clin. Pharmacol. Toxicol. (2019) 125:317–327. doi:10.1111/bcpt.13264
Bonzongo, J. C. J., Donkor, A. K., Nartey, V. K., and Lacerda, L. D. Mercury pollution in Ghana: A case study of environmental impacts of artisanal gold mining in sub-saharan Africa. In: L. Drude de Lacerda, R. E. Santelli, E. K. Duursma, and J. J. Abrão, editors. Environmental geochemistry in tropical and subtropical environments. Environmental science. Berlin, Heidelberg: Springer (2004). doi:10.1007/978-3-662-07060-4_12
Bosch, A. C., O'Neill, B., Sigge, G. O., Kerwath, S. E., and Hoffman, L. C. Heavy metals in marine fish meat and consumer health: A review. J. Sci. Food Agric. (2016) 96:32–48. doi:10.1002/jsfa.7360
Bredenkamp, L. Background ambient atmospheric mercury concentrations for the South African interior. Master of Science Dissertation. Kirkland, Washington: Environmental Sciences, North-West University (2021). Available at: https://repository.nwu.ac.za/bitstream/handle/10394/37866/Bredenkamp%20L%2023488697.pdf?sequence=1 (Accessed 07 13, 2022).
Brodziak-Dopierała, B., Fischer, A., Szczelina, W., and Stojko, J. The content of mercury in herbal dietary supplements. Biol. Trace Elem. Res. (2018) 185(1):236–243. doi:10.1007/s12011-018-1240-2
Buchanan, S., Orris, P., and Karliner, J. Alternatives to the mercury sphygmomanometer. Journa;l Public Health Policy (2011) 32(1):107–120. doi:10.1057/jphp.2010.38
Budnik, L. T., and Casteleyn, L. Mercury pollution in modern times and its socio-medical consequences. Sci. total Environ. (2019) 654:720–734. doi:10.1016/j.scitotenv.2018.10.408
Capodiferro, M., Marco, E., and Grimalt, J. O. Wild fish and seafood species in the Western Mediterranean Sea with low safe mercury concentrations. Environ. Pollut. (2022) 314:120274. doi:10.1016/j.envpol.2022.120274
Castriotta, L., Rosolen, V., Biggeri, A., Ronfani, L., Catelan, D., Mariuz, M., et al. The role of mercury, selenium and the Se-Hg antagonism on cognitive neurodevelopment: A 40-month follow-up of the Italian mother-child phime cohort. Int. J. Hyg. Environ. Health (2020) 230:113604. doi:10.1016/j.ijheh.2020.113604
Chai, L., Zhou, Y., and Wang, X. Impact of global warming on regional cycling of mercury and persistent organic pollutants on the Tibetan plateau: Current progress and future prospects. Environ. Sci. Process. Impacts (2022) 10:1616. doi:10.1039/D1EM00550B
Chamba-Eras, I., Griffith, D. M., Kalinhoff, C., Ramírez, J., and Gázquez, M. J. Native hyperaccumulator plants with differential phytoremediation potential in an artisanal gold mine of the Ecuadorian Amazon. Plants (2022) 11:1186. doi:10.3390/plants11091186
Chen, D., Ren, D., Deng, C., Tian, Z., and Yin, R. Mercury loss and isotope fractionation during high-pressure and high-temperature processing of sediments: Implication for the behaviors of mercury during metamorphism. Geochimica Cosmochimica Acta (2022) 334:231–240. doi:10.1016/j.gca.2022.08.010
Chételat, J., McKinney, M. A., Amyot, M., Dastoor, A., Douglas, T. A., Heimbürger-Boavida, L. E., et al. Climate change and mercury in the Arctic: Abiotic interactions. Sci. Total Environ. (2022) 824:153715. doi:10.1016/j.scitotenv.2022.153715
Cossa, D., Knoery, J., Bănaru, D., Harmelin-Vivien, M., Sonke, J. E., Hedgecock, I. M., et al. Mediterranean mercury assessment 2022: An updated budget, health consequences, and research perspectives. Environ. Sci. Technol. Am. Chem. Soc. (2022) 56(7):3840–3862. doi:10.1021/acs.est.1c03044
Cui, L., Tian, X., Xie, H., Cong, X., Cui, L., Wu, H., et al. Cardamine violifolia as a potential Hg hyperaccumulator and the cellular responses. Sci. Total Environ. (2022) 863:160940. Advance online publication. doi:10.1016/j.scitotenv.2022.160940
Custódio, D., Pfaffhuber, K. A., Spain, T. G., Pankratov, F. F., Strigunova, I., Molepo, K., et al. Odds and ends of atmospheric mercury in europe and over the North atlantic ocean: Temporal trends of 25 years of measurements. Atmos. Chem. Phys. (2022) 22:3827–3840. doi:10.5194/acp-22-3827-2022
Dack, K., Fell, M., Taylor, C. M., Havdahl, A., and Lewis, S. J. Prenatal mercury exposure and neurodevelopment up to the age of 5 years: A systematic review. Int. J. Environ. Res. Public Health (2022) 19:1976. doi:10.3390/ijerph19041976
Dago, A., González, I., Ariño, C., Martínez-Coronado, A., Higueras, P., Díaz-Cruz, J. M., et al. Evaluation of mercury stress in plants from the Almadén mining district by analysis of phytochelatins and their Hg complexes. Environ. Sci. Technol. (2014) 8:6256–6263. doi:10.1021/es405619y
Dang, F., and Wang, W-X. Antagonistic interaction of mercury and selenium in a marine fish is dependent on their chemical species. Environ. Sci. Technol. (2011) 45(7):3116–3122. doi:10.1021/es103705a
Driscoll, C. T., Mason, R. P., Chan, H. M., Jacob, D. J., and Pirrone, N. Mercury as a global pollutant: Sources, pathways, and effects. Environ. Sci. Technol. (2013) 47(10):4967–4983. doi:10.1021/es305071v
Dufault, R., Schnoll, R., Lukiw, W. J., LeBlanc, B., Cornett, C., Patrick, L., et al. Mercury exposure, nutritional deficiencies and metabolic disruptions may affect learning in children. Behav. Brain Funct. (2009) 5:44. doi:10.1186/1744-9081-5-44
Dwivedi, S., Chezhian, A., Kabilan, N., and Kumar, T. S. Synergistic effect of mercury and chromium on the histology and physiology of fish, Tilapia Mossambica (Peters, 1852) and Lates calcarifer Calcarifer (Bloch, 1790). Toxicol. Int. (2012) 19(3):235–240. doi:10.4103/0971-6580.103655
Eckley, C. S., Luxton, T. P., Janssen, S., Eagles-Smith, C., Luxton, T. P., Hoffman, J., et al. Using mercury stable isotope fractionation to identify the contribution of historical mercury mining sources present in downstream water, sediment and fish. Front. Environ. Chem. Sect. Inorg. Pollut. (2023) 4:2023. doi:10.3389/fenvc.2023.1096199
Ehrlich, H. L., and Newman, D. K. Geomicrobiology. Boca Raton, FL, USA: CRC Press (2008). p. 265. ISBN 978-0-8493-7906-2.
Ekström, T., Burke, S., Wiktorsson, M., Hassanie, S., Harderup, L. E., and Arfvidsson, J. Evaluating the impact of data quality on the accuracy of the predicted energy performance for a fixed building design using probabilistic energy performance simulations and uncertainty analysis. Energy Build. (2021) 249:111205. doi:10.1016/j.enbuild.2021.111205
Elumalai, V., Sujitha, S. B., and Jonathan, M. P. Mercury pollution on tourist beaches in Durban, South Africa: A chemometric analysis of exposure and human health. Mar. Pollut. Bull. (2022) 180:113742. doi:10.1016/j.marpolbul.2022.113742
ENHIS (European Environment and Health Information System). Exposure of children to chemical hazards in food. Fact Sheet No. 4.4, May 2007. CODE: RPG4_Food_Ex1 (2007). Available at: https://www.euro.who.int/__data/assets/pdf_file/0003/97446/4.4.pdf (Accessed 07 17, 2022).
Enrico, M., Le Roux, G., Marusczak, N., Heimbürger, L. E., Claustres, A., Fu, X., et al. Atmospheric mercury transfer to peat bogs dominated by gaseous elemental mercury dry deposition. Environ. Sciience Technol. (2016) 50:2405–2412. doi:10.1021/acs.est5b06058
Evers, D. C., and Sunderland, E. Technical information report on mercury monitoring in biota: Proposed components towards a strategic long-term plan for monitoring mercury in fish and wildlife globally. Geneva, Switzerland: UN Environment Programme, Chemicals and Health Branch (2019). p. 40. Available at: https://wedocs.unep.org/bitstream/handle/20.500.11822/30821/Report_Moni_Biota.pdf?sequence=1&isAllowed=y (Accessed 02 25, 2023).
Fahnestock, M. F., Bryce, J. G., McCalley, C. K., Montesdeoca, M., Bai, S., Li, Y., et al. Mercury reallocation in thawing subarctic peatlands. Geochem. Perspect. Lett. (2019) 33:33–38. doi:10.7185/geochemlet.1922
Favilli, L., Giacomino, A., Malandrino, M., Inaudi, P., Diana, A., and Abollino, O. Strategies for mercury speciation with single and multi-element approaches by HPLC-ICP-MS. Front. Chem. (2022) 10:1082956. doi:10.3389/fchem.2022.1082956
Feinberg, A., Dlamini, T., Jiskra, M., Shah, V., and Selin., N. E. N. E. Evaluating atmospheric mercury (Hg) uptake by vegetation in a chemistry-transport model. Environ. Sci. Process. Impacts (2022) 24:1303–1318. doi:10.1039/D2EM00032F
Fischer, A., and Brodziak-Dopierała, B. The Mercury concentration in spice plants. Processes (2022) 10:1954. doi:10.3390/pr10101954
Forbus, J. J., and Berleant, D. Discrete-event simulation in healthcare settings: A review. Modelling (2022) 3(4):417–433. doi:10.3390/modelling3040027
Fowler, B. A., and Zalups, R. K. Mercury. In: G. F. Nordberg, and M. Costa, editors. Handbook on the toxicology of metals, volume II: Specific metals: Chapter 22. Cambridge, MA, USA: Academic Press (2022). p. 539–599. doi:10.1016/B978-0-12-822946-0.00020-9
Frey, B., Rast, B. M., Qi, W., Stierli, B., and Brunner, I. Long-term mercury contamination does not affect the microbial gene potential for C and N cycling in soils but enhances detoxification gene abundance. Front. Microbiol. (2022) 13:1034138. doi:10.3389/fmicb.2022.1034138
Friedli, H. R., Radke, L. F., Prescott, R., Hobbs, P. V., and Sinha, P. Mercury emissions from the Aug 2001 wildfires in Washington State and an agricultural waste fire in Oregon and atmospheric mercury budget estimates. Glob. Biogeochem. Cycles (2003) 17:1039–1047. doi:10.1029/2002GB001972
Gao, Y., Shi, Z., Long, Z., Wu, P., Zheng, C., and Hou, X. Determination and speciation of mercury in environmental and biological samples by analytical atomic spectrometry. Microchem. J. (2012) 103:1–14. doi:10.1016/j.microc.2012.02.001
Garnham, B., and Langerman, K. Mercury emissions from South Africa’s coal-fired power stations. Clean Air J. (2016) 26(2):14–20. doi:10.17159/2410-972X/2016/v26n2a
Geng, C., Lin, R., Yang, P., Liu, P., Guo, L., Cui, B., et al. Highly selective adsorption of Hg (II) from aqueous solution by three-dimensional porous N-doped starch-based carbon. Environ. Sci. Pollut. Res. (2023). doi:10.1007/s11356-023-26002-8
Gochfeld, M., and Burger, J. Mercury interactions with selenium and sulfur and the relevance of the Se:Hg molar ratio to fish consumption advice. Environ. Sci. Pollut. Res. Int. (2021) 15:18407–18420. doi:10.1007/s11356-021-12361-7
Gray, J. E., Crock, J. G., and Lasorsa, B. K. Mercury methylation at mercury mines in the humboldt river basin, Nevada, USA. Geochem. Explor. Environ. Anal. (2002) 2(2):143–149. doi:10.1144/1467-787302-017
Gray, J. E., Theodorakos, P. M., Fey, D. L., and Krabbenhoft, D. P. Mercury concentrations and distribution in soil, water, mine waste leachates, and air in and around mercury mines in the Big Bend region, Texas, USA. Environ. Geochem. Health (2015) 37(1):35–48. doi:10.1007/s10653-014-9628-1
Greenberg, A. L., Syed, S. M., Alseidi, A. A., O’Sullivan, P. S., and Chern, H. Communicating from a distance: Medical student perspectives from a robotic bedside assist simulation. Glob. Surg. Educ. (2022) 1:53. doi:10.1007/s44186-022-00052-x
Gustin, M., Bank, M., Bishop, K., Bowman, K., Branfireun, B., Chételat, J., et al. Mercury biogeochemical cycling: A synthesis of recent scientific advances. Sci. Total Environ. (2020) 737:139619. doi:10.1016/j.scitotenv.2020.139619
Gworek, B., Dmuchowski, W., and Baczewska-Dąbrowska, A. H. Mercury in the terrestrial environment: A review. Environ. Sci. Eur. (2020) 32:128. doi:10.1186/s12302-020-00401-x
Hanna, D. E. L., Solomon, C. T., Poste, A. E., Buck, D. G., and Chapman, L. J. A review of mercury concentrations in freshwater fishes of Africa: Patterns and predictors. Environ. Toxicol. Chem. (2015) 34:215–223. doi:10.1002/etc.2818
Hasan, S., and Padman, R. Analyzing the effect of data quality on the accuracy of clinical decision support systems: A computer simulation approach. AMIA Annu. Symp. Proc. AMIA Symp. (2006) 2006:324–328. Available at: https://www.ncbi.nlm.nih.gov/pmc/articles/PMC1839724/(Accessed 10 10, 2022).
Hassenstein, M. J., and Vanella, P. Data quality - concepts and problems. Encyclopaedia (2022) 2:498–510. doi:10.3390/encyclopedia2010032
Hatch, W. R., and Ott, W. L. Determination of submicrogram quantities of mercury by atomic absorption spectrophotometry. Anal. Chem. (1968) 40(14):2085–2087. doi:10.1021/ac50158a025
HM (The Handbook of Mineralogy). Metacinnabar. c 2001-2005 Mineral Data Publishing, version 1. In: The handbook of mineralogy (2005). Available at: https://rruff.info/doclib/hom/metacinnabar.pdf (Accessed 12 29, 2022).
Hsu-Kim, H., Kucharzyk, K. H., Zhang, T., and Deshusses, M. A. Mechanisms regulating mercury bioavailability formethylating microorganisms in the aquatic environment: A critical review. Environ. Sci. Technol. (2013) 47(6):2441–2456. doi:10.1021/es304370g
Huang, J. H., Shetaya, W. H., and Osterwalder, S. Determination of (bio)-available mercury in soils: A review. Environ. Pollut. (2020) 263:114323. doi:10.1016/j.envpol.2020.114323
Ignatavičius, G., Unsal, M. H., Busher, P. E., Wolkowicz, S., Satkūnas, J., Šulijienė, G., et al. Geochemistry of mercury in soils and water sediments. AIMS Environ. Sci. (2022) 9(3):277–297. doi:10.3934/environsci.2022019
IPCS (International Programme on Chemical Safety). Elemental mercury and inorganic mercury compounds. Geneva, Switzerland: World Health Organization (2003). Available at: https://apo.who.int/publications/i/item/elemental-mercury-and-inorganic-mercury-compounds-human-health-aspects (Accessed 12 30, 2022).
IPCS (International Programme on Chemical Safety). Methylmercury; environmental health criteria 101. Geneva: International Programme on Chemical Safety World Health Organisation (1990). Methylmercury (EHC 101, 1990) (inchem.org) (accessed 17.07.2022).
Iqbal, K., and Asmat, M. Uses and effects of mercury in medicine and dentistry. J. Ayub Med. Coll. Abbottabad (JAMC) (2012) 24(3-4):204–207. Available at: https://www.ayubmed.edu.pk/JAMC/24-3/Kefi.pdf (Accessed 07 19, 2022).
Ishihara, N. Excretion of methyl mercury in human feces. Archives Environ. Health (2000) 55(1):44–47.doi:10.1080/00039890009603384
Jonasson, I. R., and Boyle, R. W. Geochemistry of mercury and origins of natural contamination in the environment. Cim. Bull. (1972) 1972:32–39. Available at: https://www.onemine.org/document/abstract.cfm?docid=246477 (Accessed 07 09, 2022).
Jonsson, S., Liem-Nguyen, V., Andersson, A., Skyllberg, U., Nilsson, M. B., Lundberg, E., et al. Geochemical and dietary drivers of mercury bioaccumulation in estuarine benthic invertebrates. Environ. Sci. Technol. (2022) 56(14):10141–10148. doi:10.1021/acs.est.2c03265
Kabata-Pendias, A., and Mukherjee, A. B. Plants. In: A. Kabata-Pendias, and A. B. Mukherjee, editors. Trace elements from soil to human. Berlin, Heidelberg: Springer (2007). p. 57–65. doi:10.1007/978-3-540-32714-1_6
Lamborg, C., Bowman, K., Hammerschmidt, C., Gilmour, C., Munson, K., Selin, N., et al. Mercury in the anthropocene ocean. Oceanography (2014) 27(1):76–87. doi:10.5670/oceanog.2014.11
Leaner, J. Focus on CSIR research in pollution waste: South African mercury assessment (SAMA) programme. 2007 Stockholm World Water Week, 13 - 17 August 2007, pp 1. CSIR Nat. Resour. Environ. (2007) 2007. Available at: http://hdl.handle.net/10204/1123 (Accessed 07 12, 2022).
Leiva González, J., Diaz-Robles, L. A., Cereceda-Balic, F., Pino-Cortés, E., and Campos, V. Atmospheric modellingof mercury in the southern hemisphere and future research needs: A review. Atmos. (Basel). (2022) 13:1226. doi:10.3390/atmos13081226
Li, C., Xu, Z., Luo, K., Chen, Z., Xu, X., Xu, C., et al. Biomagnification and trophic transfer of total mercury and methylmercury in a sub-tropical montane forest food web, southwest China. Chemosphere (2021) 277:130371. doi:10.1016/j.chemosphere.2021.130371
Li, F., Ma, C., and Zhang, P. Mercury deposition, climate change and anthropogenic activities: A review. Front. Earth Sci. Sec Quat. Sci. Geomorphol. Paleoenviron. (2020) 8. doi:10.3389/feart.2020.00316
Li, M. L., Kwon, S. Y., Poulin, B. A., Tsui, M. T. K., Motta, L. C., and Cho, M. Internal dynamics and metabolism of mercury in biota: A review of insights from mercury stable isotopes. Environ. Sci. Technol. (2022) 56(13):9182–9195. doi:10.1021/acs.est.1c08631
Li, Y. F., Dong, Z., Chen, C., Li, B., Gao, Y., Qu, L., et al. Organic selenium supplementation increases mercury excretion and decreases oxidative damage in long-term mercury-exposed residents from Wanshan, China. Environ. Sci. Technol. (2012) 46(20):11313–11318. doi:10.1021/es302241v
Liem-Nguyen, V. Determination of mercury chemical speciation in the presence of low molecular mass thiols and its importance for mercury methylation (2016). Available at: https://www.diva-portal.org/smash/get/diva2:925704/FULLTEXT01.pdf (Accessed 12 13, 2022).
Liu, M., Zhang, Q., Maavara, T., Liu, S., Wang, X., and Raymond, P. Rivers as the largest source of mercury to coastal oceans worldwide. Nat. Geosci. (2021) 14:672–677. doi:10.1038/s41561-021-00793-2
Lohren, H., Bornhorst, J., Fitkau, R., Pohl, G., Galla, H. J., and Schwerdtle, T. Effects on and transfer across the blood-brain barrier in vitro-Comparison of organic and inorganic mercury species. BMC Pharmacol. Toxicol. (2016) 17(1):63. doi:10.1186/s40360-016-0106-5
Loukola-Ruskeeniemi, K. Metalliferous black shales - a probable source of mercury in pike in Lake Kolmisoppi, Sotkamo, Finland. Bull. Geol. Soc. Finl. (1990) 62:167–175. doi:10.17741/bgsf/62.2.007
Lusilao-Makiese, J., Cukrowska, E., Tessier, E., Amouroux, D., and Weiersbye, I. The impact of post gold mining on mercury pollution in the West Rand region, Gauteng, South Africa. J. Geochem. Explor. (2013) 134:111–119. doi:10.1016/j.gexplo.2013.08.010
Martinez-Finley, E. J., and Aschner, M. Recent advances in mercury research. Curr. Environ. Health Rep. (2014) 1(2):163–171. doi:10.1007/s40572-014-0014-z
McKinney, M. A., Chételat, J., Burke, S. M., Elliott, K. H., Fernie, K. J., Houde, M., et al. Climate change and mercury in the Arctic: Biotic interactions. Sci. Total Environ. (2022) 834:155221. doi:10.1016/j.scitotenv.2022.155221
McLagan, D. S., Schwab, L., Wiederhold, J. G., Chen, L., Pietrucha, J., Kraemer, S. M., et al. Demystifying mercury geochemistry in contaminated soil-groundwater systems with complementary mercury stable isotope, concentration, and speciation analyses. Environ. Sci. Process. impacts (2022). Advance online publication. doi:10.1039/d1em00368b
Mi-Ling, L., Kwon, S. Y., Poulin, B. A., Tsui, M. T., Motta, L. C., and Cho, M. Internal dynamics and metabolism of mercury in biota: A review of insights from mercury stable isotopes. Environ. Sci. Technol. (2022) 56(13):9182–9195. doi:10.1021/acs.est.1c08631
Morosini, C., Terzaghi, E., Raspa, G., Zanardini, E., Anelli, S., Armiraglio, S., et al. Mercury vertical and horizontal concentrations in agricultural soils of a historically contaminated site: Role of soil properties, chemical loading, and cultivated plant species in driving its mobility. Environ. Pollut. (2021) 285:117467. doi:10.1016/j.envpol.2021.117467
Natasha, N., Shahid, M., Khalid, S., Bibi, I., Bundschuh, J., Niazi, N. K., et al. 2020. A critical review of mercury speciation, bioavailability, toxicity and detoxification in soil-plant environment: Ecotoxicology and health risk assessment. Sci. Total Environ. 711, 134749. doi:10.1016/j.scitotenv.2019.134749
Neculita, C. M., Zagury, G. J., and Deschenes, L. Mercury speciation in highly contaminated soils from chlor-alkali plants using chemical extractions. J. Environ. Qual. (2005) 34(1):255–262. doi:10.2134/jeq2005.0255a
Nevondo, V., Malehase, T., Daso, A. P., and Okonkwo, O. J. Leachate seepage from landfill: A source of groundwater mercury contamination in South Africa. Water Sa. (2019) 45(2):225–231. doi:10.4314/wsa.v45i2.09
Nishimura, M., Konishi, S., Matsunaga, K., Hata, K., and Kosuga, T. Mercury concentration in the ocean. J. Oceanogr. Soc. Jpn. (1983) 39:295–300. doi:10.1007/BF02071824
O’Connor, D., Hou, D., Ok, Y. S., Mulder, J., Duan, L., Wu, Q., et al. Mercury speciation, transformation, and transportation in soils, atmospheric flux, and implications for risk management: A critical review. Environ. Int. (2019) 126:747–761. doi:10.1016/j.envint.2019.03.019
Oliveira, C.-S., Piccoli, B.-C., Aschner, M., and Rocha, J. B. T. Chemical speciation of selenium and mercury as determinant of their neurotoxicity. In: A. Schousboe, editor. Advances in neurobiology, Vol. 18. New York: Springer New York LLC (2017). p. 53–83. doi:10.1007/978-3-319-60189-2_4
Olson, C. I., Geyman, B. M., Thackray, C. P., Krabbenhoft, D. P., Tate, M. T., Sunderland, E. M., et al. Mercury in soils of the conterminous United States: Patterns and pools. Environ. Res. Lett. (2022) 17(7):074030. doi:10.1088/1748-9326/ac79c2
Oosthuizen, M. A., John, J., and Somerset, V. Mercury exposure in a low-income community in South Africa. SAMJ South Afr. Med. J. (2010) 100(6):366–371. doi:10.7196/samj.3863
Pacyna, J. M. Recent advances in mercury research. Sci. total Environ. (2020) 738:139955. doi:10.1016/j.scitotenv.2020.139955
Paduraru, E., Iacob, D., Rarinca, V., Rusu, A., Jijie, R., Ilie, O.-D., et al. Comprehensive review regarding mercury poisoning and its complex involvement in Alzheimer’ Disease. Int. J. Mol. Scences (2022) 23:1992. doi:10.3390/ijms23041992
Palmieri, J. R., Guthrie, T., Kaur, G., Collins, E., and Benjamin, B. Implications and significance of methyl mercury toxicity and exposure in rice and fish. J. Drug Metabolism Toxicol. (2021) 12(3):258. Available at: https://www.longdom.org/open-access/implications-and-significance-of-methyl-mercury-toxicity-and-exposure-in-riceand-fish-82690.html#ai (Accessed 12 16, 2022).
Pan, J., Li, X., Wei, Y., Ni, L., Xu, B., Deng, Y., et al. Advances on the influence of methylmercury exposure during neurodevelopment. Chem. Res. Toxicol. (2022) 35(1):43–58. doi:10.1021/acs.chemrestox.1c00255
Pandey, G., and Shrivastav, A. B. Contamination of mercury in fish and its toxicity to both fish and humans: An overview. Int. Res. J. Pharm. (2012) 3:44–47. Available at: https://www.researchgate.net/publication/270173968_Contamination_of_mercury_in_fish_and_its_toxicity_to_both_fish_and_humans_An_overview/link/54a250d40cf267bdb902cde8/download (Accessed 07 21, 2022).
Panichev, N., Mokgalaka, N., and Panicheva, S. Assessment of air pollution by mercury in South African provinces using lichens Parmelia caperata as bioindicators. Environ. Geochem. Health (2019) 41:2239–2250. doi:10.1007/s10653-019-00283-w
Papu-Zamxaka, V., Mathee, A., Harpham, T., Barnes, B., Röllin, H., Lyons, M., et al. Elevated mercury exposure in communities living alongside the Inanda Dam, South Africa. J. Environ. Monit. JEM (2010) 12(2):472–477. doi:10.1039/b917452d
Park, J. D., and Zheng, W. Human exposure and health effects of inorganic and elemental mercury. J. Prev. Med. Public Health Korean Soc. Prev. Med. (2012) 45(6):344–352. doi:10.3961/jpmph.2012.45.6.344
Pasricha, S., Mathur, V., Garg, A., Lenka, S., Verma, K., and Agarwal, S. Molecular mechanisms underlying heavy metal uptake, translocation and tolerance in hyperaccumulators: An analysis: Heavy metal tolerance inhyperaccumulators. Environ. Challenges (2021) 4:100197. doi:10.1016/j.envc.2021.100197
Phalane, M. F., and Steady, F. C. Nuclear energy, hazardous waste, health, and environmental Justice in South Africa. Chapter 8. In: F. C. Steady, editor. Environmental justice in the new millennium: Global perspectives on race, ethnicity and human rights. New York: Palgrave Macmillan (2009). p. 189–201. Available at: http://ndl.ethernet.edu.et/bitstream/123456789/5351/1/158.pdf.pdf#page=193 (Accessed 07 12, 2022).
Pirrone, N., Cinnirella, S., Feng, X., Finkelman, R. B., Friedli, H. R., Leaner, J., et al. Global mercury emissions to the atmosphere from anthropogenic and natural sources. Atmos. Chem. Phys. (2010) 10(13):5951–5964.
Posin, S. L., Kong, E. L., and Sharma, S. Mercury toxicity. In: StatPearls [internet]. Treasure Island, FL: StatPearls Publishing (2022). Available at: https://www.ncbi.nlm.nih.gov/books/NBK499935/(Accessed 12 30, 2022).
Puścion-Jakubik, A., Mielech, A., Abramiuk, D., Iwaniuk, M., Grabia, M., Bielecka, J., et al. Mercury content in dietary supplements from Poland containing ingredients of plant origin: A safety assessment. Front. Pharmacol. (2021) 12:738549. doi:10.3389/fphar.2021.738549
Qing, Y., Li, Y., Yang, J., Li, S., Gu, K., Bao, Y., et al. Risk assessment of mercury through dietary exposure in China. Environ. Pollut. (2022) 312:120026. doi:10.1016/j.envpol.2022.120026
Raihan, S. M., Moniruzzaman, M., Park, Y., Lee, S., and Bai, S. C. Evaluation of dietary organic and inorganic mercury threshold levels on induced mercury toxicity in a marine fish model. Animals An Open Access J. MDPI (2020) 10(3):405. doi:10.3390/ani10030405
Rand Lab (URMC) (University of Rochester Medical Centre). Methylmercury metabolism and elimination status (MerMES) in humans (2022). Available at: https://www.urmc.rochester.edu/labs/rand/projects/methylmercury-metabolism-elimination.aspx (Accessed 07 11, 2022).
Rascio, N., and Navari-Izzo, F. Heavy metal hyperaccumulating plants: How and why do they do it? And what makes them so interesting? Plant Sci. An Int. J. Exp. Plant Biol. (2011) 180(2):169–181. doi:10.1016/j.plantsci.2010.08.016
Reeves, R. D., Baker, A. J. M., Jaffré, T., Erskine, P. D., Echevarria, G., and van der Ent, A. A global database for plants that hyperaccumulate metal and metalloid trace elements. New Phytol. (2018) 218:407–411. doi:10.1111/nph.14907
Risher, J. F. Elemental mercury and inorganic mercury compounds: Human health aspects. In: Concise international chemical assessment document 50. Geneva: World Health Organisation (2003). Available at: https://apps.who.int/iris/bitstream/handle/10665/42607/9241530502.pdf?sequence=1&isAllowed=y (Accessed 07 11, 2022).
Różański, S. Ł., Castejón, J. M. P., and Fernández, G. G. Bioavailability and mobility of mercury in selected soil profiles. Environ. Earth Sci. (2016) 75:1065. doi:10.1007/s12665-016-5863-3
Samaniego, J., Gibaga, C. R., Tanciongco, A., and Rastrullo, R. Total mercury in soils and sediments in the vicinity of abandoned mercury mine area in Puerto Princesa city, Philippines. Appl. Sci. (2020) 10:4599. doi:10.3390/app10134599
Sanei, H., Outridge, P. M., Oguri, K., Stern, G. A., Thamdrup, B., Wenzhöfer, F., et al. High mercury accumulation in deep-ocean hadal sediments. Sci. Rep. (2021) 11(1):10970. doi:10.1038/s41598-021-90459-1
Santos, J. P., Mehmeti, L., and Slaveykova, V. I. Simple acid digestion procedure for the determination of total mercury in plankton by cold vapor atomic fluorescence spectroscopy. Methods Protoc. (2022) 5(2):29. doi:10.3390/mps5020029
Schluter, K. The fate of mercury in soil: A review of current knowledge. In: Soil and groundwater research report IV, technical report EUR 14666 EN. Luxembourg, UK: Commission of the European Communities (1993). URL unavailable.
Seewagen, C. L. Threats of environmental mercury to birds: Knowledge gaps and priorities for future research. Bird. Conserv. Int. (2010) 20:112–123. doi:10.1017/S095927090999030X
Selvan, D. S., Chezhian, A., Kabilan, N., and Kumar, T. Synergistic effect of mercury and chromium on the histology and physiology of fish, Tilapia Mossambica (Peters, 1852) and Lates calcarifer calcarifer (Bloch, 1790). Toxicol. Int. (2012) 19:235–240. doi:10.4103/0971-6580.103655
Senthamilselvan, D., Chezhian, A., and Suresh, E. Synergistic effect of nickel and mercury on fatty acid composition in the muscle of fish Lates calcarifer. J. Fish. Aquatic Sci. (2016) 11:77–84. doi:10.3923/jfas.2016.77.84
Shi, Y., Fan, F., and Zhang, Z. Simulation of performance evaluation model for medical-elderly care integrated institutions based on system dynamics. BMC Health Serv. Res. (2022) 22:1451. doi:10.1186/s12913-022-08835-0
Siudek, P., Falkowska, L., Brodecka, A., Kowalski, A., Frankowski, M., and Siepak, J. Mercury in precipitation over the coastal zone of the southern Baltic Sea, Poland. Environ. Sci. Pollut. Res. Int. (2015) 22(4):2546–2557. doi:10.1007/s11356-014-3537-9
Skoogh, A., and Johansson, B. A methodology for input data management in discrete event simulation projects. In: 2008 Winter Simulation Conference. Miami, FL, USA: IEEE (2008). p. 1727–1735. doi:10.1109/WSC.2008.4736259
Skuza, L., Szućko-Kociuba, I., Filip, E., and Bożek, I. Natural molecular mechanisms of plant hyperaccumulation and hypertolerance towards heavy metals. Int. Mol. Sci. (2022) 23(16):9335. doi:10.3390/ijms23169335
Soerensen, A. L., Schartup, A. T., Skrobonja, A., and Björn, E. Organic matter drives high interannual variability in methylmercury concentrations in a subarctic coastal sea. Environ. Pollut. (2017) 229:531–538. doi:10.1016/j.envpol.2017.06.008
Spiegel, S. J. Socioeconomic dimensions of mercury pollution abatement: Engaging artisanal mining communities in Sub-Saharan Africa. Ecol. Econ. (2009) 68:3072–3083. doi:10.1016/j.ecolecon.2009.07.015
Steinnes, E. Mercury. In: B. J. Alloway, editor. Heavy metals in soils. 2nd edition. London, UK: Blackie Academics and Professional Press (1997). p. 245–259.
Street, R. A., Kabera, G. M., and Connolly, C. Metallic mercury use by South African traditional health practitioners: Perceptions and practices. Environ. Health (2015) 14:67. doi:10.1186/s12940-015-0053-4
Suárez-Criado, L., Rodriguez-Cea, A., Rodriguez-Gonzalez, P., Garcia Alonso, J. I., Queipo-Abad, S., Cea, A. R., et al. Comparison of GC-ICP-MS, GC-EI-MS and GC-EI-MS/MS for the determination of methylmercury, ethylmercury and inorganic mercury in biological samples by triple spike species-specific isotope dilution mass spectrometry. J. Anal. Atomic Spectrom. (2022) 37:1462–1470. doi:10.1039/d2ja00086e (
Sun, T., and Branfireun, B. A. Plant mercury accumulation and litter input to a Northern Sedge-dominated Peatland. EGUsphere (2022). [preprint]. doi:10.5194/egusphere-2022-632
Sundseth, K., Pacyna, J. M., Pacyna, E. G., Pirrone, N., and Thorne, R. J. Global sources and pathways of mercury in the context of human health. Int. J. Environ. Res. Public Health (2017) 14(1):105. doi:10.3390/ijerph14010105
Teixeira, F. B., de Oliveira, A., Leão, L., Fagundes, N., Fernandes, R. M., Fernandes, L., et al. Exposure to inorganic mercury causes oxidative stress, cell death, and functional deficits in the motor cortex. Front. Mol. Neurosci. (2018) 11:125. doi:10.3389/fnmol.2018.00125
Timmerman, R., and Omaye, S. Selenium’s utility in mercury toxicity: A mini-review. Food Nutr. Sci. (2021) 12:124–137. doi:10.4236/fns.2021.122011
Țincu, R. C., Cobilinsch, C., Majd, J., and Țincu, I. F. Low level mercury exposure effects on fertility. Proc. Romanian Acad. Ser. B (2022) 24(1):85–90. Available at: https://acad.ro/sectii2002/proceedingsChemistry/doc2022-1/Art12.pdf (Accessed 12 30, 2022).
Tinggi, U., and Perkins, A. V. Selenium status: Its interactions with dietary mercury exposure and implications in human health. Nutrients (2022) 14:5308. doi:10.3390/nu14245308
Travnikov, O., Hedgecock, I., Matthias, V., Dastoor, A., and Lin, C. Mercury simulations within GMOS: Analysis of short-term observational episodes. E3S Web Conf. (2013) 1:17006. doi:10.1051/e3sconf/20130117006
Turekian, K. K., and Wedepohl, K. H. Distribution of the elements in some major units of the earth's crust. Geol. Soc. Am. Bull. (1961) 72:175–192. doi:10.1130/0016-7606(1961)72[175:doteis]2.0.co;2
Ullrich, S. M., Tanton, T. W., and Abdrashitova, S. A. Mercury in the aquatic environment: A review of factors affecting methylation. Crit. Rev. Environ. Sci. Technol. (2001) 31(3):241–293. doi:10.1080/20016491089226
UNEP (United Nations Environment Pogramme). Global mercury assessment 2018 (2019a). Available at: https://www.unep.org/resources/publication/global-mercury-assessment-2018 (Accessed 02 27, 2022).
UNEP (United Nations Environment Programme). Mercury inventory Toolkit. Geneva: UNEP (2011). Available at: https://www.unep.org/explore-topics/chemicals-waste/what-we-do/mercury/mercury-inventory-toolkit (Accessed 12 17, 2022).
UNEP (United Nations Environment Programme). Toolkit for identification and quantification of mercury releases reference report and guideline for inventory level 2 version 1.5 november 2019 (2019b). Available at: https://wedocs.unep.org/bitstream/handle/20.500.11822/30684/HgTlktRef.pdf (Accessed 12 17, 2022).
US EPA. Systematic review protocol for the inorganic mercury salts IRIS assessment (preliminary assessment materials). EPA/635/R-20/239, 2021. Washington, DC: U.S. Environmental Protection Agency (2021b). Available at: https://cfpub.epa.gov/ncea/iris_drafts/recordisplay.cfm?deid=349284 (Accessed 12 29, 2022).
US EPA (United States Environmenta Protection Agency). Health effects of exposures to mercury (2022). Available at: https://www.epa.gov/mercury/health-effects-exposures-mercury (Accessed 02 28, 2023).
US EPA (United States Environmental Protection Agency). What EPA is doing to reduce mercury pollution, and exposures to mercury (2021a). Available at: https://www.epa.gov/mercury/what-epa-doing-reduce-mercury-pollution-and-exposures-mercury#understanding (Accessed 07 23, 2022).
USGS (United States Geological Survey). Mercury in the environment. Geological survey professional paper 713 (1970). Available at: https://pubs.usgs.gov/pp/0713/report.pdf (Accessed 07 09, 2022).
Wallett, L., Chen, W., Thomas, L., Blaggan, P., Ooi, E., Zhou, D., et al. Developing a simulation-based learning model for acute medical education during COVID-19 pandemic with simulation via instant messaging - Birmingham advance (SIMBA). BMJ Open Qual. (2022) 11(2):e001565. doi:10.1136/bmjoq-2021-001565
Walters, C. R., Somerset, V. S., Leaner, J. J., and Nel, J. M. A review of mercury pollution in South Africa: Current status. J. Environ. Sci. Health A Tox Hazard Subst. Environ. Eng. (2011) 46(10):1129–1137. doi:10.1080/10934529.2011.590729
Wang, J., Liu, Y., Li, P., Lin, Z., Sindakis, S., and Aggarwal, S. Overview of data quality: Examining the dimensions, antecedents, and impacts of data quality. J. Knowl. Econ. (2023). doi:10.1007/s13132-022-01096-6
Wang, J., Ma, L. Q., Letcher, R., Bradford, S. A., Feng, F., and Rinklebe, J. Biogeochemical cycle of mercury and controlling technologies: Publications in critical reviews in environmental science & technology in the period of 2017 - 2021. Crit. Rev. Environ. Sci. Technol. (2022) 52:2071210. doi:10.1080/10643389.2022.2071210
Wang, L., Han, J., Katuwal, H. B., Xia, P., Xu, X., Feng, X., et al. Occurrence of total mercury and methylmercury in rice: Exposure and health implications in Nepal. Ecotoxicol. Environ. Saf. (2021) 228:113019. Advance online publication. doi:10.1016/j.ecoenv.2021.113019
Wang, X., Luo, J., Yuan, W., Lin, C-J., Wang, F., Liu, C., et al. Global warming accelerates uptake of atmospheric mercury in regions experiencing glacier retreat. PNAS (2020) 117(4):2049–2055. doi:10.1073/pnas.1906930117
Warr, L. N. IMA–CNMNC approved mineral symbols. Mineral. Mag. (2021) 85(3):291. doi:10.1180/mgm.2021.43
Wells, E. M., Kopylev, L., Nachman, R., Radke, E. G., and Segal, D. Seafood, wine, rice, vegetables, and other food items associated with mercury biomarkers among seafood and non-seafood consumers: Nhanes 2011-2012. J. Expo. Sci. Environ. Epidemiol. (2020) 30(3):504–514. doi:10.1038/s41370-020-0206-6
WHO (World Health Organisation). Developing national strategies for phasing out mercury containing thermometers and sphygmomanometers in health care, including in the context of the Minamata convention on mercury: Key considerations and step-by-step guidance (2015). Available at: https://www.euro.who.int/__data/assets/pdf_file/0006/295611/Phasing-Out-Mercury-containing-thermometers-sphygmomanometers-HC-en.pdf (Accessed 02 26, 2023).
WHO (World Health Organisation). Mercury in drinking-water; background document for development of WHO guidelines for drinking-water quality. Geneva: Document WHO/SDE/WSH/03.04/10 (2003). Available at: https://cdn.who.int/media/docs/default-source/wash-documents/wash-chemicals/mercury-2003.pdf?sfvrsn=f2804f45_3 (Accessed 07 17, 2022).
WHO (World Health Organisation). Mercury in drinking-water. Background document for development of WHO guidelines for drinking-water quality. Geneva, Switzerland: WHO/SDE/WSH/05.08/10 (2005). Available at: https://www.who.int/docs/default-source/wash-documents/wash-chemicals/mercury-background-document.pdf?sfvrsn=9b117325_4#:∼:text=If%20a%20level%20in%20drinking,the%20range%202%E2%80%9320%20%C2%B5g (Accessed 07 23, 2022).
WHO (World Health Organisation). Mercury. Chapter 6.9: Air quality guidelines. 2nd Edition. Copenhagen, Denmark: Regional Office for Europe (2000). Microsoft Word - 6.9-Mercury.doc (who.int) (accessed 10.07.2022).
Wohlgemuth, L., McLagan, D., Flückiger, B., Vienneau, D., and Osterwalder, S. Concurrently measured concentrations of atmospheric mercury in indoor (household) and outdoor air of Basel, Switzerland. Environ. Sci. Technol. Lett. (2020) 7(4):234–239. doi:10.1021/acs.estlett.0c00110
Wu, X., Zong, Z., Lin, K., Lin, K., Liu, X., Wu, Z., et al. Comparative pharmacokinetics and urinary excretion of arsenic and mercury after oral administration of realgar, cinnabar and AnGongNiuHuang Pill to rats. Front. Pharmacol. (2022) 13. doi:10.3389/fphar.2022.967608
Wyatt, L. H., Diringer, S. E., Rogers, L. A., Hsu-Kim, H., Pan, W. K., and Meyer, J. N. Antagonistic growth effects of mercury and selenium in Caenorhabditis elegans are chemical-species-dependent and do not depend on internal Hg/Se ratios. Environ. Sci. Technol. (2016) 50(6):3256–3264. doi:10.1021/acs.est.5b06044
Yang, C. Y., Liu, S. H., Su, C. C., Fang, K. M., Yang, T. Y., Liu, J. M., et al. Methylmercury induces mitochondria- and endoplasmic reticulum stress-dependent pancreatic β-Cell apoptosis via an oxidative stress-mediated JNK signaling pathway. Int. J. Mol. Sci. (2022) 23:2858. doi:10.3390/ijms23052858
Yu, X., Liu, C., Guo, Y., and Deng, T. Speciation analysis of trace arsenic, mercury, selenium and antimony in environmental and biological samples based on hyphenated techniques. Molecules (2019) 24:926. doi:10.3390/molecules24050926
Zaferani, S., and Biester, H. Mercury accumulation in marine sediments - a comparison of an upwelling area and two large river Mouths. Front. Mar. Sci. (2021) 8:732720. doi:10.3389/fmars.2021.732720
Zampetti, C. J., and Brandt, J. E. Co-Considering selenium concentrations alters mercury-based fish and seafood consumption advice: A data compilation and critical assessment. Environ. Sci. Technol. Lett. (2023) 10(2):179–185. doi:10.1021/acs.estlett.2c00925
Zhao, M., Li, Y., and Wang, Z. Mercury and mercury-containing preparations: History of use, clinical applications, Pharmacology, toxicology and pharmacokinetics in traditional Chinese medicine. Front. Pharmacol. (2022) 13:807807. doi:10.3389/fphar.2022.807807
Zhou, J., Obrist, D., Dastoor, A., Jiskra, M., and Ryzhkov, A. Vegetation uptake of mercury and impacts on global cycling. Nat. Rev. Earth Environ. (2021) 2:269–284. doi:10.1038/s43017-021-00146-y
Keywords: mercury, uncertainties, geomedical characteristics, exposure, input data quality, simulation analysis, model validation, addressing the issues
Citation: Davies TC (2023) An updated review of the salient geomedical aspects of mercury for enhancement of data quality in simulation modelling and other prognostic applications: Africa case descriptions. Front. Anal. Sci. 3:1069678. doi: 10.3389/frans.2023.1069678
Received: 14 October 2022; Accepted: 13 March 2023;
Published: 19 April 2023.
Edited by:
Anamika Shrivastava, Amity University, IndiaReviewed by:
Jeferson Luis Franco, Federal University of Pampa, BrazilValentina Rimondi, Università di Firenze, Italy
Copyright © 2023 Davies. This is an open-access article distributed under the terms of the Creative Commons Attribution License (CC BY). The use, distribution or reproduction in other forums is permitted, provided the original author(s) and the copyright owner(s) are credited and that the original publication in this journal is cited, in accordance with accepted academic practice. No use, distribution or reproduction is permitted which does not comply with these terms.
*Correspondence: Theophilus C. Davies, theo.clavellpr3@gmail.com, davies.theophilus@mut.ac.za,
†ORCID: Theophilus C. Davies, orcid.org/0000-0002-8299-1121