- 1Institut des Sciences de la Mer de Rimouski, Université du Québec à Rimouski, Rimouski, QC, Canada
- 2Centre for Ocean and Atmospheric Sciences, School of Environmental Sciences, University of East Anglia, Norwich, United Kingdom
- 3Centre for Environment, Fisheries and Aquaculture Science (Cefas), Lowestoft, United Kingdom
- 4Scottish Association for Marine Science, Scottish Marine Institute, Oban, United Kingdom
- 5Department of Marine Sciences, University of Gothenburg, Gothenburg, Sweden
- 6Laboratoire d'Océanographie et de Climatologie (LOCEAN), Institut Pierre Simon Laplace (IPSL), Observatoire Ecce Terra, Sorbonne Univ-CNRS-IRD-MNHN, UMR 7159, Paris, France
Ocean gliders are quiet, buoyancy-driven, long-endurance, profiling autonomous platforms. Gliders therefore possess unique advantages as platforms for Passive Acoustic Monitoring (PAM) of the marine environment. In this paper, we review available glider platforms and passive acoustic monitoring systems, and explore current and potential uses of passive acoustic monitoring-equipped gliders for the study of physical oceanography, biology, ecology and for regulatory purposes. We evaluate limiting factors for passive acoustic monitoring glider surveys, such as platform-generated and flow noise, weight, size and energy constraints, profiling ability and slow movement. Based on data from 34 passive acoustic monitoring glider missions, it was found that <13% of the time spent at sea was unsuitable for passive acoustic monitoring measurements, either because of surface communications or glider manoeuvre, leaving the remainder available for subsequent analysis. To facilitate the broader use of passive acoustic monitoring gliders, we document best practices and include workarounds for the typical challenges of a passive acoustic monitoring glider mission. Three research priorities are also identified to improve future passive acoustic monitoring glider observations: 1) Technological developments to improve sensor integration and preserve glider endurance; 2) improved sampling methods and statistical analysis techniques to perform population density estimation from passive acoustic monitoring glider observations; and 3) calibration of the passive acoustic monitoring glider to record absolute noise levels, for anthropogenic noise monitoring. It is hoped this methodological review will assist glider users to broaden the observational capability of their instruments, and help researchers in related fields to deploy passive acoustic monitoring gliders in their studies.
1 Introduction
Sound can travel tens to thousands of kilometres in the ocean, whereas light only reaches up to a hundred metres. For this reason, many marine species have evolved to use sound for foraging, communication and navigation. Passive acoustic monitoring (PAM) entails recording sounds to infer information about their sources. PAM is a powerful way to monitor the ocean in a non-invasive way. Analysis of the underwater soundscape allows detection, identification and localisation of a wide variety of sound sources which are illustrated in Figure 1. Whether purposefully or not, most marine anthropogenic and biological activities, as well as some natural processes, emit sounds (Figure 1), respectively classified as anthropophony, biophony, and geophony (Krause 2008).
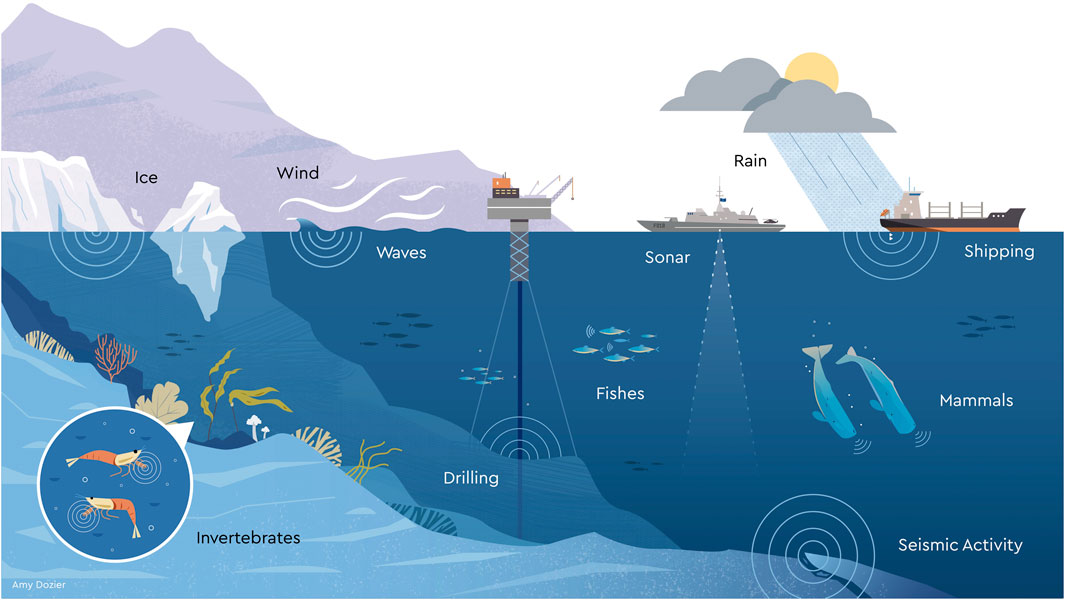
FIGURE 1. Main sources contributing to the underwater soundscape. Sources contributing to biophony: Marine mammals, fishes and invertebrates. Sources contributing to geophony: Wind, waves, rain, ice and seismic activity. Sources contributing to anthropophony: Marine traffic, active acoustics systems (e.g., sonar, seabed imaging), and offshore platforms and pile driving. Figure by Amy Dozier. Published with permission.
Anthropophony consists of sound contributions from industrial, military and leisure activities such as shipping (Merchant et al., 2012), seismic surveys (Guerra et al., 2011; Guan et al., 2015; Nowacek et al., 2015), pile driving (Bailey et al., 2010; Thompson et al., 2013), anti-submarine warfare (Ricks et al., 2012), seabed characterization (Harrison and Simons 2002; Quijano et al., 2012), and active sonar (Dolman et al., 2011), causing anthropogenic underwater noise pollution (Williams et al., 2015; Haver et al., 2017; Merchant et al., 2018). Analysis of the anthropophony allows quantification of the associated pressure on the oceanic environment, widely affecting marine life (Van der Graaf et al., 2012).
Analysis of the biophony allows estimation of, for example, whale population density (Marques et al., 2013), population abundance (Lewis et al., 2018), seasonality (Stafford et al., 2007) and behaviour (Wahlberg 2002; Miller and Miller 2018). Seal calls present unique signatures enabling individuals to be identified (Charrier et al., 2017). Analysis of sounds from fish (Bolgan et al., 2018; Di Iorio et al., 2018), shrimp (Everest 1947; Johnson et al., 1947) and sea urchins (Radford et al., 2008b) provide valuable information about habitats and ecosystem health (Radford et al., 2008a; Harris et al., 2016).
Analysis of the geophony allows measurement of sea surface wind speed and rainfall rate (Vagle et al., 1990; Nystuen 1996; Cauchy et al., 2018). Low frequency sounds generated by seismic activity can be detected from thousands of kilometres away (McGuire et al., 2005). Submarine volcanoes (Matsumoto et al., 2011), ice shelf calving events (Dziak et al., 2019) and turbidity currents (Hatcher 2017) can be monitored through their contribution to the underwater soundscape.
PAM systems are usually deployed on moorings, enabling persistent observation of their surroundings over long durations (months to years). Moored PAM systems are commonly used for underwater noise monitoring (Merchant et al., 2016), observation of cetacean presence (Miller and Miller 2018) and monitoring of evolutions of the soundscape (Erbe et al., 2015). PAM systems can be towed behind a survey ship, away from propeller, engine and hull-radiated noise (Lasky et al., 2004). Towed PAM systems provide a real-time data stream that can be analysed onboard by acoustic experts, routinely used for anti-submarine warfare (Lemon 2004) and monitoring of cetacean population abundance, density and distribution estimation (ACCOBAMS Survey Initiative, www.accobams.org).
Ocean gliders are highly suitable for PAM applications. They glide quietly through water, without any propulsion noise; they collect hydrographic profiles, from which sound velocity profiles can be calculated (this is important information for modelling sound propagation); raw acoustic data can be accessed after recovery of the glider; they can carry one or several PAM sensors, offering multiple acoustic monitoring possibilities. Flow noise, generated by turbulent water flow around the glider’s hull, is of similar magnitude to flow noise observed on moored PAM systems (Erbe et al., 2015; Dos Santos et al., 2016; Fregosi et al., 2020). Ocean gliders have been equipped with custom-built PAM systems and on-board processing capability for near real-time detection of beaked whales (Klinck et al., 2012) and baleen whales (Baumgartner et al., 2013; Baumgartner et al., 2020), demonstrating the opportunity to use PAM gliders as a component of operational whale monitoring observatories. Multi-channel systems have been used to demonstrate the ability to track sperm whales (Kusel et al., 2017) and perform tactical maritime surveillance operations (Tesei et al., 2015). Autonomous PAM systems have been successfully attached on gliders, to observe soundscape variability along the glider’s track (Wall et al., 2017), to map fish activity along cross-shelf transects in the Gulf of Mexico (Wall et al., 2012), to observe sperm whale populations in the Mediterranean Sea (Cauchy et al., 2020) and measure surface wind speed (Cauchy et al., 2018). They have been identified as suitable for long term acoustic population monitoring (Verfuss et al., 2019).
There is a growing literature of primary studies applying PAM techniques on ocean gliders, but this work has yet to be reviewed and consolidated. The purpose of this study is to highlight the opportunities (and pitfalls) of PAM glider applications and to review the specific methodological considerations that PAM glider users should be aware of. Our hope is that this work might help to enhance interactions between the ocean glider and underwater acoustics scientific communities and support the broader use of PAM gliders, so that the great potential of this technology might be more fully realised.
2 Technology: Current state-of-the-art
2.1 Ocean gliders
Ocean gliders are autonomous underwater vehicles performing successive dives along a predefined trajectory, using satellite positioning and communication while at the surface to transfer data back to shore and update their mission plan if required. Over the past decades, ocean gliders have demonstrated their ability to fill gaps in the global ocean observing systems (Testor et al., 2010; Testor et al., 2019). They provide high-resolution measurements that allow observation of submesoscale and mesoscale (1–100 km) processes, difficult to resolve with traditional observing platforms. They can target identified geographical areas where coverage from Argo floats is insufficient (e.g., coastal regions, boundary currents, water transformation areas). They are unaffected by storms and are used to provide prediction and observation of tropical storms (Glenn et al., 2016). They can travel near and under ice (Lee et al., 2017). They can carry a wide range of sensors simultaneously measuring physical, chemical and biological properties of the water column (Testor et al., 2019) to study the marine ecosystems health.
Ocean gliders are driven by buoyancy changes, controlled by pumping oil in and out of an external bladder or by using a piston, inducing a vertical motion in the water column between the surface and a specified depth. Fixed wings, fins and a specifically profiled body convert their vertical velocity (0.1–0.2 m s-1) into horizontal velocity (0.2–0.4 m s-1) following V-shape profiles of typical glide angles of 15°–30° to horizontal. Pitch is controlled by moving the internal battery. Steering is obtained by either roll adjustments via rotating the internal battery or a rudder, depending on the glider type, while a compass is used for underwater navigation. An ocean glider’s usual diving pattern is composed of repeated cycles of three successive steady phases: Two profiling phases, descent and ascent, where the glider is collecting scientific measurements, and a communication phase, where the glider stays afloat at the surface communicating with land via satellite and updating its location using GPS (Figure 2). The two profiling phases are the main phases of interest, where the glider is able to steer and make progress towards its next waypoint, scientific data can be collected throughout the profile with no additional energy needed for propulsion.
At the time of writing, the market is dominated by three types of ocean gliders that are commercially available: Seaglider is developed by the University of Washington and commercialised by Huntington Ingalls, SeaExplorer is developed by Alseamar, while Slocum is developed by Teledyne. Two models of Slocum, deep (max depth 1,000 m) and coastal (max depth 150 m) are commercially available, the coastal version being equipped with an optimised buoyancy piston allowing increased manoeuvrability in shallow waters. Each manufacturer makes their own design choices (Table 1), but all share similar dive cycle and operating and propulsion principles.
2.2 PAM systems
2.2.1 Self-contained PAM systems
Self-contained PAM systems are autonomous recorders equipped with their own battery and memory that follow a programmed sampling mission. Raw data are accessed and processed after recovery of the instrument. They are usually composed of a main pressure housing, containing the electronics, memory and battery, with one or more tethered hydrophones and optional external battery packs. Many different specifications are available, depending on the expected application and the associated size, weight and energy constraints. When considering adding a self-contained system externally to an ocean glider, weight is the critical constraint. Propulsion relies on small buoyancy changes (800–1,000 cm3) and energy consumption for travel is directly proportional to buoyancy changes, requiring the glider to be carefully ballasted to the same density as seawater at the mission location. Therefore, any weight added needs to be compensated for, by addition of high-density foam of the equivalent buoyancy inside the glider’s fairing. Although there are no hard limits regarding how much an external sensor package can protrude, it is beneficial to limit vehicle drag to maximise travel efficiency and save power. As examples of large sensors being integrated to gliders, the nitrate sensor Deep SUNA and turbulence sensor MicroRider-1000 weigh, respectively 500 g and 0 g in water for 1,500 and 5,500 cm3 displacement (Satlantic deep SUNA manual, SAT-DN-00627, Rev. E, 2014-Dec-01, MicroRider-1000 Datasheet, 2014-08). Finally, the pressure rating of the system can be a limiting factor. Depending on water depth and sampling plan, ocean gliders perform successive dives from the surface up to 1,000 m deep (even up to 6,000 m for recently developed deep gliders), submitting the attached system to a repeated mechanical stress caused by pressure variations. Compact self-contained PAM systems, such as the SoundTrap ST300 (www.oceansonics.co.nz), D-Tag (Johnson and Tyack 2003), and Acousonde (Burgess 2010) with external battery pack have been successfully deployed on gliders for defence (Nott 2015), ecosystem monitoring trial (Suberg et al., 2014), marine mammal monitoring (Cauchy et al., 2020; Thums et al., 2022) and weather observation through ambient noise (WOTAN) (Cauchy et al., 2018) applications.
Self-contained PAM systems run on their own batteries, leaving the glider’s endurance unaffected. The success of many oceanographic glider missions relies on their ability to make sustained observations over long periods (months), to monitor intra-seasonal variability, to be able to travel to and from remote locations or across oceanic basins and to reduce logistical costs. Such missions can only afford to run a limited number of sensors but can accommodate self-contained, energy self-sufficient, PAM systems with no negative impacts on endurance. Compact self-contained PAM systems have limited endurance (Table 2). However, they offer the opportunity to configure a duty cycle, spreading the available recording time over a longer period to match the glider’s endurance. A delayed start mode allows configuration of a future date and time for the recording cycle to start, focusing effort on when the glider reaches its target area for example.
2.2.2 Integrated PAM systems
Over the past decade, PAM systems have been integrated into ocean gliders. At the time of writing, each of the three commercially available glider manufacturer offers an option for an integrated PAM system developed by JASCO (www.jasco.com, Table 2). Integrated PAM systems are powered by the glider’s batteries and controlled by the glider’s operating system. They can communicate data to the glider system, enabling a level of real-time data transmission, and can receive sampling instructions from the pilot. Examples of glider missions can be found in the literature, using different versions of commercially integrated PAM systems for marine mammal monitoring studies. Seagliders have been equipped with the WISPR board (Fregosi et al., 2020; Cauchy et al., 2020), developed by Embedded Ocean Systems, and with the AMAR G4 (Aniceto et al., 2020), developed by JASCO. Slocums have been equipped with the OceanObserver (Kowarski et al., 2020), also developed by JASCO. Integrated PAM systems offer high quality acoustic recordings (Table 2), and the most recent version offers onboard processing capability and the ability to transmit whale detection information via satellite communication in near real time (Kowarski et al., 2020). They can be controlled remotely, benefitting from satellite connection through the glider. Acoustic sampling can therefore be adapted to the location of the glider, the battery usage and the observed conditions. They can be automatically turned off when the glider is at surface, or during the bottom inflection phase, known to be unsuitable for PAM applications because of the buoyancy pump noise. The four published integrated PAM glider studies (Aniceto et al., 2020; Fregosi et al., 2020; Kowarski et al., 2020; Cauchy et al., 2020) suggest an endurance of 14–45 days while recording up to 16 days of acoustic data (Table 3), which is significantly shorter that the expected endurance of ocean gliders (Table 1).

TABLE 3. Summary of the integrated PAM glider missions: Platform and system used, duration of the mission and time recorded.
3 Challenges
3.1 Platform noise
Ocean gliders generate noise when performing manoeuvres, interacting with their environment or collecting scientific measurements. Platform noise received level on the PAM system is high, due to transmission via solid vibration between the noise source and the receiver. Glider-generated noise varies greatly during a dive cycle. Most of a glider’s manoeuvres—and therefore platform noise - occur during the transition between two successive steady phases, as illustrated in Figure 3, where the spectrogram corresponds to a full dive cycle similar to the one presented in Figure 2. Each of the steady phases and transitions presents a specific platform noise regime, due to the glider’s behaviour and combination of systems in use (Table 4).
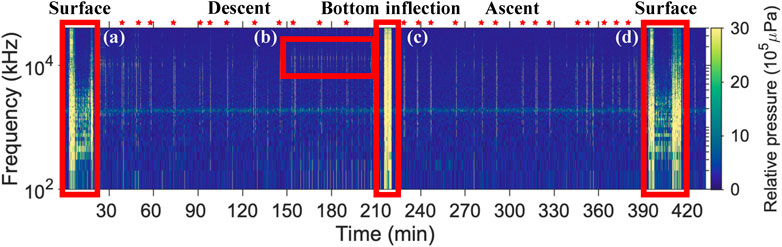
FIGURE 3. Spectrogram of a typical Seaglider dive cycle, recorded using an Acousonde. (A,D) succession of surface manoeuvre, communication phase and start dive transition. (B) Train of 13 kHz altimeter pings. (C) Pump noise during bottom inflection phase. The red stars at the top show the mid-profile adjustments of the glider’s attitude (pitch, roll and/or pump). The constant 2 kHz noise throughout is an electrical noise from the Acousonde.
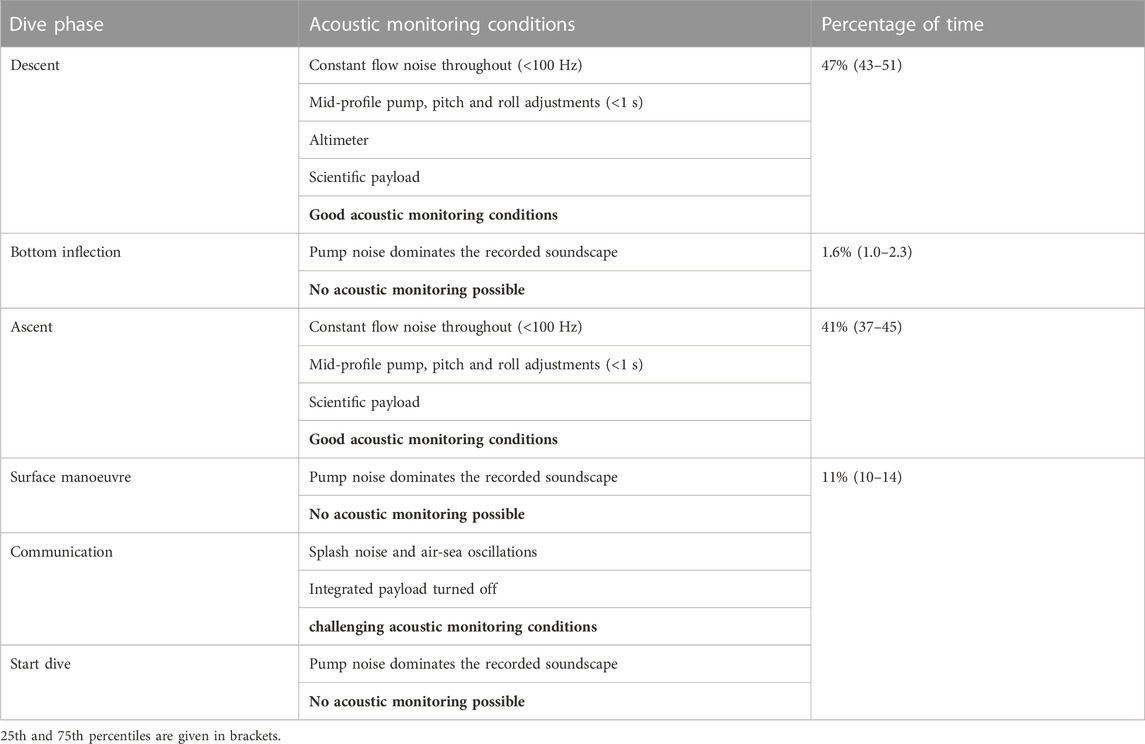
TABLE 4. Sources of platform noise and impact on the recorded soundscape for each phase of a dive, and median percentage of time spent in each phase.
Glider flight characteristics were analysed from past glider missions from the UEA glider group (7 missions using Seagliders) and the MOOSE network (27 missions using Slocum gliders). These glider missions did not include acoustic recordings. Information contained in mission data files was used to identify the dive phases and transitions. Time spent at surface was detected using a depth threshold of 5 m (Figure 2). Bottom inflection phase was detected from change in volume in the bladder around the depth maximum. Descent and ascent profiles were defined as the phases between the surface and the bottom inflection, as described in the dive cycle schematics (Figure 2). The time spent at surface, descending, in bottom inflection phase and ascending was extracted for each glider mission to provide quantitative observations of the relative importance of each of these phases and transitions, and the implications for PAM applications (Table 4).
3.1.1 Surface phase
The surface phase comprises a surface manoeuvre, a communication phase and a start dive transition (Figure 2). The succession of three different acoustic signatures can be seen on the spectrogram in both the surface phases (Figures 3A, D). The surface manoeuvre sets the glider in a position for good communication, raising the antenna high above the sea surface (highly positive buoyancy, downward pitch) (Figure 2). Noise production during this transition is dominated by the loud broadband noise of the buoyancy pump, covering the whole recorded spectrum (1–62 kHz) and completely masking the underwater soundscape (Figures 3A, D). During the communication phase, the glider stays afloat at the surface. Noise is generated by the interaction of the glider with the sea surface. Splash sounds of waves hitting the hull and the PAM sensor oscillating between in-air and underwater positions generate a mix of multiple broadband, impulsive sounds (Figures 3A, D). Finally, the start dive transition modifies the glider’s navigation parameters from the communication phase (highly positive buoyancy, downward pitch) to the descent phase (negative buoyancy, downward pitch) (Figure 2). Noise production during this transition is dominated by the broadband loud noise of the buoyancy pump, covering the whole recorded spectrum (1 Hz–62 kHz) and completely masking the underwater soundscape (Figures 3A, D). The surface phase is therefore considered unsuitable for PAM applications.
The surface phase is necessary for the glider to receive piloting instructions, transfer data to land and acquire its GPS location. It is also a phase where the glider is in danger of getting hit by a boat or covered in ice, the glider is not manoeuvrable and drifts with the surface currents, and the glider is not usually collecting any scientific data. Ocean glider pilots usually intend to minimise the time spent at surface by limiting the amount of data transmitted, and this is particularly important for PAM missions. Piloting files, transmitted from the pilot to the glider, should be reduced to the bare minimum size. The amount of scientific data transmitted from the glider to the pilot can be minimised. Scientific data files can for example be downsampled before transmission. It is also possible to perform successive dives without surfacing. Time spent at surface is directly affected by the quality of the communication, which is dependent on antenna performance, sea state, the iridium satellite constellation and weather. The surface phase is the main source of acoustic monitoring time loss, representing 11% of the time spent by the glider at sea during the 34 glider missions analysed (Table 4).
3.1.2 Bottom inflection
The bottom inflection, at the end of the descent phase (negative buoyancy, downward pitch), requires activation of the buoyancy engine and the pitch motor to initiate the ascent phase (positive buoyancy, upward pitch) (Figure 2). Noise generated by the buoyancy engine covers the whole recorded spectrum (1 Hz–62 kHz), completely masking the underwater soundscape (Figure 3C). The bottom inflection phase is therefore considered non-suitable for PAM applications. Acoustic monitoring time loss due to bottom inflection noise however is marginal, this phase representing only 1.6% of the time spent by the glider at sea during the 34 glider missions analysed (Table 4), but this proportion increases in shallow regions.
3.1.3 Profiling phases
Descent and ascent profiling phases are similar, with the glider passively gliding through the water column relying solely on the change in buoyancy imparted during the preceding surface or bottom inflection phase (Figure 2). Mid-profile adjustments of the glider’s buoyancy, pitch, roll or rudder may occur, for steering and control of the vertical speed, generating platform noise (Figure 3). Such adjustments are of short duration (<10 s) and their occurrence are logged in the glider’s data files. It is therefore easy to detect and remove the samples corrupted by the associated platform noise from the acoustic analysis, to avoid artificial increase of sound levels or false detections of events. The implied reduction of the overall monitoring time is marginal. In the example dive shown in Figure 3, mid-profile adjustments represent ∼1% of the recorded time (26 10-second segments out of a ∼400-min dive). The occurrence rate of mid-profile adjustments can be modified by the pilots, or even deactivated, although with an obvious reduction in the navigational capability.
The glider’s altimeter is often used during the descent to detect and avoid the seabed by triggering the bottom inflection (Figure 2). Recently developed under-ice navigation capability, for Seagliders, activates the altimeter during the ascent to similarly detect and avoid ice. The acoustic characteristics of the altimeter ping (central frequency, duration, intensity) are known and constant throughout the mission. The central frequency depends on glider type and configuration, 10–25 kHz for Seagliders, 200 kHz for Slocum and 675 kHz for SeaExplorer gliders. It is therefore easy to assess whether an acoustic analysis is likely to be affected by altimeter noise and mitigate the effects. When studying marine mammal echolocation clicks for example, altimeter ping parameters can be provided to the detection software, or the operator in charge of data annotation, to be removed from the analysis to avoid false detections. When studying sound level in a frequency band affected by altimeter noise, timestamps for each altimeter ping can be extracted from the glider’s data file, and corrupted samples removed from the analysis. It is also possible to deactivate the altimeter during descent when the bathymetry is well known or much deeper than the glider’s profiling depth, or during ascent in ice free conditions. Descent and ascent phases are highly suitable for PAM applications. They represent, respectively 47% and 41% of the time spent by the glider at sea during the 34 glider missions analysed (Table 4). In total, 87% of a glider’s time spent at sea offers excellent conditions for PAM applications.
3.1.4 Thrusters
Thrusters have recently been added to ocean gliders as an option, increasing travelling speed when necessary (coping with strong currents, or arriving at their waypoint in a timely fashion) and allowing horizontal motion, with detrimental effects on PAM applications. Thrusters have been used on a Slocum glider equipped with an Acousonde during a trial experiment, where the glider was configured to perform multiple (6) shallow dives (to a depth of 50 m) between the communication phases (Figures 4A, C), with continuous use of thrusters, to escape strong coastal currents. The measured soundscape is largely dominated by thruster-generated noise (Figure 4). The thrusters generated constant broadband noise, masking most of the soundscape in the 200 Hz–20 kHz frequency band, as shown in Figure 4B. It is clear that the use of thrusters is not compatible with most PAM glider applications and should therefore be avoided when possible on PAM gliders, or switched off during PAM recordings.
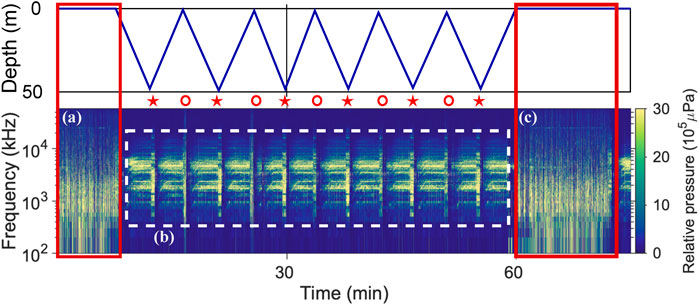
FIGURE 4. Thruster noise recorded with an Acousonde mounted on a Slocum glider. Top panel shows the diving pattern of the glider, performing six successive 50-m dives between each communication phase (A,C). Bottom panel shows the spectrogram of the recordings. Bottom and surface inflection noise are identified by red stars and circles on the top of the spectrogram. (B) Thruster noise is continuously recorded in the 200–20 kHz frequency band.
3.2 Flow noise
Flow noise is generated by the water flow around the glider’s hull. Slow speed through water (∼0.25 m s-1) and an optimised streamlined shape lead to reduced flow noise. Flow noise has however been shown to be a possible issue for PAM applications focusing on weak and low frequency (<100 Hz) signals (Dos Santos et al., 2016; Fregosi et al., 2020). It is possible to reduce the glider’s speed through water to reduce flow noise, as shown in a recent study (Fregosi et al., 2020). However, reduced glider speeds may have adverse effects on manoeuvrability, ability to cope with currents and reliability of some other scientific measurements from instruments such as un-pumped CTD or turbulence sensors. It is also worth noting that reducing a glider’s speed is a well-known way to increase its endurance, therefore usually optimised by pilots whether or not the glider operates a PAM system. Descent and ascent profiles are typically very similar but may differ based on piloting decisions or inaccuracy of the buoyancy engine and asymmetries in drag, leading to different speeds and therefore flow noise levels, as reported in previous studies (Matsumoto et al., 2015; Fregosi et al., 2020). Such asymmetries are usually consistent throughout a glider mission, but vary randomly from one mission to another, which explains the dispersion observed in the time spent during ascent and descent phases during the 34 glider missions analysed (Table 4), as well as the two opposite observations from two single deployment studies, low frequency noise level being found higher (Matsumoto et al., 2015) or lower (Fregosi et al., 2020) during ascent than descent. This speed variability between ascent and descent highlights the importance of fine tuning the piloting parameters, aiming for slow and constant speed through the water to control flow noise variations, simplifying further analysis and processing.
Alternative behaviours have been implemented to reduce flow noise. Gliders can adjust their buoyancy to become neutrally buoyant at a predefined depth, then loiter (no vertical velocity, drifting behaviour following the surrounding water mass), generating no flow noise. They can also be programmed to land and rest on the seabed, with possible flow noise from the current and noise from benthic life depending on the landing site. As yet, there are no reports of these behaviours’ use during a PAM glider mission in the peer reviewed literature.
3.3 Payload noise
Gliders can carry multiple instruments, from a large and expanding range. Common oceanographic sensors, such as conductivity, temperature and depth (CTD), chlorophyll a, optical backscatter, fluorometers, photosynthetically active radiation (PAR) and dissolved oxygen sensors have been used on ocean gliders simultaneously with PAM observations without any interference reported. Sensors based on active acoustic technology, such as acoustic Doppler current profilers (ADCP) or echosounders, will necessarily have an impact on acoustic measurements, depending on their emitting frequency. No reports of simultaneous use of active and passive acoustics payload on a glider have been found in the literature. Payloads of increasing complexity, such as nitrate and turbulence sensors, water samplers and Lab-on-a-Chip biogeochemical measurement systems, have recently been adapted for use on ocean gliders. Moving parts, pumps and more generally complex systems are likely to generate noise when they operate. Care must be taken when selecting the suite of sensors to deploy on a glider to anticipate potential noise contamination. When operating incompatible payloads, synchronised duty cycles to activate systems at different times is a good mitigation solution. This is not an issue specific to PAM applications; turbulence sensors, for example, are sensitive to any sensor modifying the water flow around the hull or generating vibrations.
3.4 Sampling pattern
PAM gliders are of increasing interest to the bioacoustics community. Ocean gliders’ ability to perform sustained long-term observation along a predefined, purposefully-designed mission have the potential to significantly improve population monitoring (Verfuss et al., 2019). A glider’s slow speed (∼20 km a day), however, and the combination of horizontal and vertical movement, can be a challenge when developing new usage. For density estimates of marine mammal populations, for example, ship-borne surveys make the assumption that the animal’s speed is slow compared with the vessel’s speed (Marques et al., 2013), ensuring that animals would not be counted multiple times. Such an assumption is largely violated in the case of a PAM glider observing mobile marine species, which could introduce an overestimation bias. Optimized statistical approaches need to be developed to extend standard density estimation methods to PAM glider surveys (Marques et al., 2013; Fregosi et al., 2022).
Similar challenges have been tackled in the past by physical oceanographers, who pioneered the use of ocean gliders. Mission plans and glider behaviours have been adapted to observations of various ocean processes (e.g., fronts, boundary currents, eddies) and have the potential to improve bioacoustics observations. Ocean gliders are often used to follow a simple pattern, with a shape and size that can be adapted to the expected variability of the observed process or species (Figure 5). They can be programmed to perform dives around a single waypoint, maintaining a virtual mooring pattern (Figure 5), providing time-series of single point observation with a time resolution depending on the dive duration (∼2 h for 1,000 m profiles). Analysis of bioacoustics observations from such a glider mission can be based on techniques developed for fixed point observatories (Marques et al., 2013; Verfuss et al., 2019). Repeated cross slope sections (Figure 5) in the northwestern Mediterranean Sea allowed observation of the along-slope Northern Current (Carret et al., 2019) with a weekly to bi-weekly resolution. They also allowed observations of the variability of marine life detection with distance to the slope (Cauchy et al., 2020). In the case of a glider mission monitoring an oceanographic eddy or marine life around a seamount, following a butterfly pattern allows collection of observations along two axes (Figure 5).
3.5 Vertical profiling
Underwater noise levels vary with measurement depth, due to complex acoustic propagation processes including reflection on the seabed and the surface, and refraction between water masses of different densities (Urick and Kuperman 1989). The profiling behaviour of ocean gliders offers the opportunity to repeatedly measure noise levels at each depth along their trajectory. The resulting acoustic time series therefore includes effects of the varying measurement depth that must be considered in the analysis.
For WOTAN applications, the noise propagates vertically from a source distributed at the surface. Vagle et al. (1990) proposed a model for vertical propagation of surface noise that can be used, using the local sound velocity profile measured by the glider, to correct the effects of the varying measurement depth from a PAM glider (Cauchy et al., 2018). For monitoring of the marine life, long range propagation effects, such as waveguide propagation along the deep sound channel may affect the detection range. For measurement of anthropogenic noise, in-situ measurement of noise level at multiple depth can improve the estimation of exposure of the marine species depending on their depth in the water column. For vertically mobile species, such as marine mammals, a PAM glider could be configured to simulate animal behaviour, offering the opportunity to integrate received levels along a 3D trajectory.
4 Opportunities and applications
4.1 Integration and endurance
State-of-the-art integrated PAM systems offer promising functionalities. The ability to run onboard data processing tools enables communication of observations in real-time. Remote piloting can focus the sampling effort on locations of interest (e.g., when the glider reaches its study area) and to adapt to real-time observations (e.g., when a targeted event is detected). Integration into the glider’s operating system allows for increased data quality by acquiring data only when no platform noise is emitted. However, a review of the examples of integrated PAM glider missions found in the literature suggests technical challenges and avenues for endurance improvement.
Ocean glider endurance is a major strength as an ocean observing platform, stemming from their power efficient design. Ocean glider batteries store a limited quantity of energy (Table 1), shared between propulsion, navigation and communication, and collection of scientific data. Mission durations of 60 days are often exceeded. For a 6-kWh battery, such endurance requires an average power draw of ∼4 W, and typical oceanographic glider surveys aim for 1–2 W (P. Testor, personal communication). The suite of sensors usually deployed on ocean gliders has been adapted to this constraint, drawing minimal power. For example, the unpumped Seabird Glider Payload CTD draws 175 mW when sampling at 1 Hz (Janzen and Creed 2011) and the unpumped CTD RBR Legato draws 45 mW when sampling at 2 Hz (www.rbr-global.com/products/oem/rbrlegato, accessed on 08/02/2022). The latest available version of integrated PAM systems, however, draws an estimated power of 2–3 W (Kowarski et al., 2020), which represents 50%–75% of the available power. Continuous use of this system therefore induces reduction of the overall endurance by 33%–43%. Acceptability of such endurance reduction depends on the location and primary objective of a glider mission. Current integrated PAM systems seem adapted to only certain types of glider observations, such as routine or operational observations with easy access to the deployment and recovery sites and that can accommodate shorter missions. They are necessary to applications requiring onboard processing and real-time communication (e.g., robots4whales.whoi.edu, Kowarski et al., 2020). However, many ocean glider missions rely on glider endurance, to reduce costs and risks of deployment and recovery operations and to safely operate in remote locations or in adverse weather conditions. The scientific community would benefit from the availability of a low-power integrated PAM system that could be easily added to the usual ocean glider payload while preserving its endurance. The global ocean glider fleet is increasingly important. Testor et al. (2019) presented results from 25 boundary current sections sustained for a minimum of 1 year, and for as long as 12 years, and expect this number to increase to 100 sustained sections by 2030. Widespread use of PAM glider systems will benefit biology, physical oceanography, and regulatory applications, providing sustained acoustic monitoring of key areas that are potentially hard to reach with the usual moored PAM systems, and with increased spatial resolution.
Software integration of the PAM system could be improved, to offer more versatile configuration options, and therefore reduce energy consumption of the PAM system. The integrated PAM systems used in the four reviewed studies (Aniceto et al., 2020; Fregosi et al., 2020; Kowarski et al., 2020; Cauchy et al., 2020) can be turned off during surface and bottom inflection phases, known to be unsuitable for PAM applications (see above). They offer the option to be turned on or off during descent and ascent phases independently. A depth threshold can be set up to trigger the recording. Such configurations allow to collect a maximum of one recording sample per glider profile (∼2 h for 1,000 m profiles). All three options were used for a bioacoustics survey (Aniceto et al., 2020), recording continuously during descent to 200 m only, collecting 108 h of data during a 40 days (284 dive cycles) PAM glider mission. The resulting sampling effort is 11% of the mission time, recording one 23-min sample every dive cycle (∼4 h for 1,000 m dives). Using a self-contained PAM system, with more versatile configuration options, recordings of 1 min every 10 min have been used for WOTAN (Cauchy et al., 2018) and marine mammal (Cauchy et al., 2020) monitoring applications. The resulting sampling effort is similar (10%) but spreading evenly shorter samples with a higher repetition rate allowed observation with a much higher time resolution (10 min), throughout the mission. The use of such duty cycles, however, comes with an energy cost due to power consumption and battery discharge when the system is in idle mode. For example, the estimated power draw of a SoundTrap ST400 is 40 mW when recording at 192 kHz, and 4 mW when in idle mode, according to the manufacturer (SoundTrap ST600/ST400 deployment endurance estimator, V4.0 June 2022). In this case, recordings of 1 min every 10 min lead to a loss of almost half of the possible continuous recording time.
4.2 Measurement of absolute sound levels
An ocean glider is not transparent to underwater acoustic waves. The dry compartment, an air-filled cavity hosting the electronics and the batteries encapsulated in a carbon fibre or aluminium hull, is likely to modify the local acoustic field through scattering and reflection effects, as previously documented for a larger autonomous underwater vehicle, comprising two air-filled glass spheres (Lepper and D’Spain, 2007). The resulting interference pattern is complex and depends on the mechanical characteristics of the body (shape and material, specific to each glider make and configuration). The effects on acoustic measurements also depend on the location of the hydrophone and are different for each frequency and angle of arrival of the measured sound. Knowledge of the interference pattern, caused by scattering of the acoustic waves from the glider’s body, will improve source localisation techniques (Lepper and D’Spain, 2007) and is necessary to achieve calibrated sound level measurements from a glider. To the authors’ knowledge, there have been no published studies of the local modification of the acoustic field by a glider’s body and the associated effect on the measured sound level. Such a study would require either costly in-situ measurement, in a large instrumented basin capable of hosting the glider in a controlled acoustic environment (Lepper and D’Spain, 2007), or modelling of the vibro-acoustic behaviour of the glider’s body.
Not all PAM applications need absolute sound level measurements. Software for annotation, detection and classification of bioacoustics signals (e.g., Raven (Charif et al., 2004) or PAMGuard (Gillespie et al., 2008)) typically use relative sound levels (Merchant et al., 2015). Wind speed measurement can be achieved using relative sound levels, provided in-situ observation of the wind speed can be used to calibrate the relationship between the relative sound level measured and the wind speed (Cauchy et al., 2018). However, acquisition of absolute sound level is required for noise pollution monitoring.
5 Conclusion and outlook
We have reviewed the existing PAM glider literature and summarised basic knowledge needed to understand potential use of PAM gliders and consider planning of future PAM glider surveys. We have also identified promising features, key challenges and desirable developments for PAM glider applications to reach their full potential. For application to physical oceanography, PAM gliders provide information about the surface weather conditions (Cauchy et al., 2018; Cazau et al., 2019) co-located with collection of hydrographic profiles, allowing promising applications for storm monitoring and quantification of air-sea interaction processes such as wind-driven mixing or air-sea gas exchange. For bioacoustics, PAM glider observations are well adapted to long term population monitoring (Verfuss et al., 2019; Cauchy et al., 2020) and have the potential to provide density estimations (Fregosi et al., 2022). Onboard processing with real-time transmission of animal detection has reached operational level, used by Canadian and United States authorities to trigger slowdowns of marine traffic when North Atlantic right whales are detected (robots4whales.whoi.edu). Multiple hydrophones have been used on an ocean glider, demonstrating the ability estimate animals’ location and track their movements, using time difference of arrival methods (Kusel et al., 2017).
Preserving ocean gliders’ endurance is key to enabling broad use of PAM systems on gliders. Low power consumption is a very critical characteristic for any sensor to be used routinely in a large variety of glider missions. Commercially available self-contained PAM sensors can be used on ocean gliders, with no noticeable effects on endurance. For integrated PAM systems, however, developments are needed to improve power efficiency. Versatile configuration options would allow a great improvement with minimal technological development. Current glider-integrated PAM systems allow to stop the recording based on glider location and depth, to focus sampling effort on mission relevant observations; and dive cycle phase, to avoid recording of glider-generated noise. Future glider-integrated PAM systems should allow to add a duty cycling behaviour, to spread the sampling effort on a larger time scale. Many applications, such as WOTAN, population monitoring and anthropogenic noise monitoring do not need continuous recording.
Anthropogenic underwater noise is a global issue, with a need for better monitoring and description identified by the scientific community and governing bodies (e.g., International Maritime Organisation, EU’s Marine Strategy Framework Directive, iqoe.org). Widespread use of PAM gliders would improve monitoring in the same way that ocean gliders fill gaps in ocean observing systems. PAM gliders could collect sustained observations in marine protected areas, near identified sources of anthropogenic noise (e.g., offshore works, industrial harbour, shipping lane). Because of their vertical profiling ability, PAM glider observations allow to carry measurements in three spatial dimensions, at a horizontal scale that is clearly not possible to obtain with moored observations. However, the ability to collect absolute sound level measurement is necessary to quantify levels of noise pollution and ambient noise. It is critical to further explore the vibroacoustic behaviour of a glider’s body, to provide comprehensive description and correction of the resulting modification of the measured acoustic field.
Finally, onboard processing and real-time transmission are key characteristics for operational applications (robots4whales.whoi.edu, Kowarski et al., 2020) and have the potential to benefit to many others. The ability to process raw acoustic data onboard allows extraction of key parameters (e.g., sound levels on prespecified frequency band, automatic detections), summarising the information at a fraction of the data volume. Real-time data transmission provides feedback on the data collected by the PAM glider and the opportunity to adapt the sampling strategy to improve monitoring. Real-time transmission of whale call detection could trigger continuous recording, to provide extended observation of behaviour and social interaction without significantly reducing the PAM glider’s endurance. Real-time transmission of rain detection could trigger modification of the glider’s diving depth, providing subsurface observations at an increased time resolution. Real-time transmission of sound level would allow to adapt the PAM glider’s route to improve monitoring around a noise source.
Author contributions
PC wrote the first manuscript draft, with KH, NM, DR, BQ, and PT contributing to all sections of the manuscript early drafts. PC, KH, BQ, and PT designed and carried out the PAM glider data collection. All authors contributed to the article and approved the submitted version.
Funding
This research was funded by the Natural Environment Research Council (Grant NE/N012070/1) and the Engineering and Physical Sciences Research Council, via the NEXUSS Centre of Doctoral Training in the Smart and Autonomous Observation of the Environment, and as part of the Cefas−UEA strategic alliance (Cefas Seedcorn SP002).
Acknowledgments
We acknowledge the staff of the French National Pool of Gliders (DT-INSU/CNRS-CETSM/Ifremer) for the sustained glider deployments carried out in the framework of MOOSE, as well as the intensive deployments during this 2012–2013 DEWEX experiment. We thank captains, crews as well as UEA scientists, engineers, technicians and students who participated in the cruises and autonomous platform deployments and piloting. The work presented here was initiated during PC’s PhD and partially appears in his PhD thesis (Cauchy 2021).
Conflict of interest
The authors declare that the research was conducted in the absence of any commercial or financial relationships that could be construed as a potential conflict of interest.
Publisher’s note
All claims expressed in this article are solely those of the authors and do not necessarily represent those of their affiliated organizations, or those of the publisher, the editors and the reviewers. Any product that may be evaluated in this article, or claim that may be made by its manufacturer, is not guaranteed or endorsed by the publisher.
References
Aniceto, A. S., Pedersen, G., Primicerio, R., Biuw, M., Lindstrøm, U., and Camus, L. (2020). Arctic marine data collection using oceanic gliders: Providing ecological context to cetacean vocalizations. Front. Mar. Sci. 7, 1–11. doi:10.3389/fmars.2020.585754
Bailey, H., Senior, B., Simmons, D., Rusin, J., Picken, G., and Thompson, P. M. (2010). Assessing underwater noise levels during pile-driving at an offshore windfarm and its potential effects on marine mammals. Mar. Pollut. Bull. 60, 888–897. doi:10.1016/j.marpolbul.2010.01.003
Baumgartner, M. F., Bonnell, J., Corkeron, P. J., others, , Hotchkin, C., Hodges, B. A., et al. (2020). Slocum gliders provide accurate near real-time estimates of baleen whale presence from human-reviewed passive acoustic detection information. Front. Mar. Sci. 7, 100. doi:10.3389/fmars.2020.00100
Baumgartner, M. F., Fratantoni, D. M., Hurst, T. P., Brown, M. W., Cole, T. V. N., Van Parijs, S. M., et al. (2013). Real-time reporting of baleen whale passive acoustic detections from ocean gliders. J. Acoust. Soc. Am. 134, 1814–1823. doi:10.1121/1.4816406
Bolgan, M., Amorim, M. C. P., Fonseca, P. J., Di Iorio, L., and Parmentier, E. (2018). Acoustic complexity of vocal fish communities: A field and controlled validation. Sci. Rep. 8, 10559. doi:10.1038/s41598-018-28771-6
Burgess, W. C. 2010. Development of a wideband acoustic recording tag to assess the acoustic behavior of marine wildlife. GREENERIDGE SCIENCES INC SANTA BARBARA CA.
Carret, A., Birol, F., Estournel, C., Zakardjian, B., and Testor, P. (2019). Synergy between in situ and altimetry data to observe and study Northern Current variations (NW Mediterranean Sea). Ocean. Sci. 15, 269–290. doi:10.5194/os-15-269-2019
Cauchy, P., Heywood, K. J., Merchant, N. D., Queste, B. Y., and Testor, P. (2018). Wind speed measured from underwater gliders using passive acoustics. J. Atmos. Ocean. Technol. 35, 2305–2321. doi:10.1175/JTECH-D-17-0209.1
Cauchy, P., Heywood, K. J., Risch, D., Merchant, N. D., Queste, B. Y., and Testor, P. (2020). Sperm whale presence observed using passive acoustic monitoring from gliders of opportunity. Endanger. Species Res. 42, 133–149. doi:10.3354/esr01044
Cauchy, P. (2021). Ocean of sound: Underwater gliders observing the oceanic environment. Doctoral thesis. Norwich, UK: University of East Anglia.
Cazau, D., Bonnel, J., and Baumgartner, M. F. (2019). Wind speed estimation using acoustic underwater glider in a near-shore marine environment. IEEE Trans. Geosci. Remote Sens. 57, 2097–2106. doi:10.1109/TGRS.2018.2871422
Charif, R. A., Clark, C. W., and Fristrup, K. M. (2004). Raven 1.2 user’s manual. Ithaca, NY: Cornell Lab. Ornithol., 1–167.
Charrier, I., Marchesseau, S., Dendrinos, P., Tounta, E., and Karamanlidis, A. A. (2017). Individual signatures in the vocal repertoire of the endangered Mediterranean monk seal: New perspectives for population monitoring. Endanger. Species Res. 32, 459–470. doi:10.3354/esr00829
Di Iorio, L., Raick, X., Parmentier, E., Boissery, P., Valentini-Poirier, C.-A., and Gervaise, C. (2018). in ‘ posidonia meadows calling’: A ubiquitous fish sound with monitoring potential. Editors N. Pettorelli, and N. Merchant, 4, 248–263. Remote Sens. Ecol. Conserv. doi:10.1002/rse2.72
Dolman, S. J., Evans, P. G. H., Notarbartolo-di-Sciara, G., and Frisch, H. (2011). Active sonar, beaked whales and European regional policy. Mar. Pollut. Bull. 63, 27–34. doi:10.1016/j.marpolbul.2010.03.034
Dos Santos, F. A., São Thiago, P. M., De Oliveira, A. L. S., Barmak, R., Lima, J. A. M., De Almeida, F. G., et al. (2016). Investigating flow noise on underwater gliders acoustic data. J. Acoust. Soc. Am. 140, 3409. doi:10.1121/1.4970954
Dziak, R. P., Lee, W. S., Haxel, J. H., Matsumoto, H., Tepp, G., Lau, T. K., et al. (2019). Hydroacoustic, meteorologic and seismic observations of the 2016 nansen ice shelf calving event and iceberg formation. Front. Earth Sci. 7, 1–12. doi:10.3389/feart.2019.00183
Erbe, C., Verma, A., McCauley, R., Gavrilov, A., and Parnum, I. (2015). The marine soundscape of the Perth Canyon. Prog. Oceanogr. 137, 38–51. doi:10.1016/j.pocean.2015.05.015
Everest, F. a., Young, R. W., and Johnson, M. W. (1947). Acoustical characteristics of noise produced by snapping shrimp. J. Acoust. Soc. Am. 19, 726. doi:10.1121/1.1916566
Fregosi, S., Harris, D. V., Matsumoto, H., Mellinger, D. K., Martin, S. W., Matsuyama, B., et al. (2022). Detection probability and density estimation of fin whales by a Seaglider. J. Acoust. Soc. Am. 152, 2277–2291. doi:10.1121/10.0014793
Fregosi, S., Harris, D. V., Matsumoto, H., others, , Negretti, C., Moretti, D. J., et al. (2020). Comparison of fin whale 20 Hz call detections by deep-water mobile autonomous and stationary recorders. J. Acoust. Soc. Am. 147, 961–977. doi:10.1121/10.0000617
Gillespie, D., Gordon, J. C. D., McHugh, R., and others, (2008). Pamguard: Semiautomated, open source software for real-time acoustic detection and localisation of cetaceans. Proc. Inst. Acoust. 30, 54–62. doi:10.1121/1.4808713
Glenn, S. M., Miles, T. N., Seroka, G. N., Xu, Y., Forney, R. K., Yu, F., et al. (2016). Stratified coastal ocean interactions with tropical cyclones. Nat. Commun. 7, 10887. doi:10.1038/ncomms10887
Guan, S., Vignola, J., Judge, J., and Turo, D. (2015). Airgun inter-pulse noise field during a seismic survey in an Arctic ultra shallow marine environment. J. Acoust. Soc. Am. 138, 3447–3457. doi:10.1121/1.4936904
Guerra, M., Thode, A. M., Blackwell, S. B., and Michael Macrander, A. (2011). Quantifying seismic survey reverberation off the alaskan North slope. J. Acoust. Soc. Am. 130, 3046–3058. doi:10.1121/1.3628326
Harris, S. A., Shears, N. T., and Radford, C. A. (2016). Ecoacoustic indices as proxies for biodiversity on temperate reefs. Methods Ecol. Evol. 7, 713–724. doi:10.1111/2041-210X.12527
Harrison, C. H., and Simons, D. G. (2002). Geoacoustic inversion of ambient noise: A simple method. J. Acoust. Soc. Am. 112, 1377–1389. doi:10.1121/1.1506365
Hatcher, M. G. (2017). Ambient noise from turbidity currents in howe sound. Master thesis. Halifax, NS, Canada: Dalhousie University.
Haver, S. M., Klinck, H., Nieukirk, S. L., Matsumoto, H., Dziak, R. P., and Miksis-Olds, J. L. (2017). The not-so-silent world: Measuring arctic, equatorial, and antarctic soundscapes in the atlantic ocean. Deep. Res. Part I Oceanogr. Res. Pap. 122, 95–104. doi:10.1016/j.dsr.2017.03.002
Janzen, C. D., and Creed, E. L. (2011). Physical oceanographic data from Seaglider trials in stratified coastal waters using a new pumped payload CTD. Ocean. - MTS/IEEE Kona, Progr. B. doi:10.23919/oceans.2011.6107290
Johnson, M. P., and Tyack, P. L. (2003). A digital acoustic recording tag for measuring the response of wild marine mammals to sound. IEEE J. Ocean. Eng. 28, 3–12. doi:10.1109/JOE.2002.808212
Johnson, M. W., Everest, F. A., and Young, R. W. (1947). The role of snapping shrimp (Crangon and synalpheus) in the production of underwater noise in the sea. Biol. Bull. 93, 122–138. doi:10.2307/1538284
Klinck, H., Mellinger, D. K., Klinck, K., others, , Luby, J. C., Jump, W. A., et al. (2012). Near-real-time acoustic monitoring of beaked whales and other cetaceans using a SeagliderTM. PLoS One 7, e36128. doi:10.1371/journal.pone.0036128
Kowarski, K. A., Gaudet, B. J., Cole, A. J., Maxner, E. E., Turner, S. P., Martin, S. B., et al. (2020). Near real-time marine mammal monitoring from gliders: Practical challenges, system development, and management implications. J. Acoust. Soc. Am. 148, 1215–1230. doi:10.1121/10.0001811
Krause, B. (2008). Anatomy of the soundscape: Evolving perspectives. AES J. Audio Eng. Soc. 56, 73–80.
Kusel, E. T., Munoz, T., Siderius, M., Mellinger, D. K., and Heimlich, S. (2017). Marine mammal tracks from two-hydrophone acoustic recordings made with a glider. Ocean. Sci. 13, 273–288. doi:10.5194/os-13-273-2017
Lasky, M., Doolittle, R. D., Simmons, B. D., and Lemon, S. G. (2004). Recent progress in towed hydrophone array research. IEEE J. Ocean. Eng. 29, 374–387. doi:10.1109/JOE.2004.829792
Lee, C. M., Thomson, J., Cho, K. H., and others, (2017). An autonomous approach to observing the seasonal ice zone in the Western arctic. Oceanography 30, 56–68. doi:10.5670/OCEANOG.2017.222
Lemon, S. G. (2004). Towed-Array history, 1917–2003. IEEE J. Ocean. Eng. 29, 365–373. doi:10.1109/JOE.2004.829791
Lepper, P. A., and D’Spain, G. L. (2007). Measurement and modeling of the acoustic field near an underwater vehicle and implications for acoustic source localization. J. Acoust. Soc. Am. 122, 892–905. doi:10.1121/1.2749410
Lewis, T., Boisseau, O., Danbolt, M., others, , Leaper, R., Matthews, J., et al. (2018). Abundance estimates for sperm whales in the Mediterranean Sea from acoustic line-transect surveys. J. Cetacean Res. Manag. 18, 103–117. doi:10.47536/jcrm.v18i1.437
Marques, T. A., Thomas, L., Martin, S. W., Mellinger, D. K., Ward, J. A., Moretti, D. J., et al. (2013). Estimating animal population density using passive acoustics. Biol. Rev. 88, 287–309. doi:10.1111/brv.12001
Matsumoto, H., Haxel, J. H., Dziak, R. P., Bohnenstiehl, D. R., and Embley, R. W. (2011). Mapping the sound field of an erupting submarine volcano using an acoustic glider. J. Acoust. Soc. Am. 129, EL94–EL99. doi:10.1121/1.3547720
Matsumoto, H., Haxel, J., and Turpin, A. (2015), Simultaneous operation of mobile acoustic recording systems off the Washington coast for cetacean studies. Oceans 2015. MTS/IEEE Washington. IEEE, 1–7. others.
McGuire, J. J., Boettcher, M. S., and Jordan, T. H. (2005). Erratum: Foreshock sequences and short-term earthquake predictability on East Pacific Rise transform faults. Nature 435, 528. doi:10.1038/nature03621
Merchant, N. D., Blondel, P., Dakin, D. T., and Dorocicz, J. (2012). Averaging underwater noise levels for environmental assessment of shipping. J. Acoust. Soc. Am. 132, EL343–EL349. doi:10.1121/1.4754429
Merchant, N. D., Brookes, K. L., Faulkner, R. C., Bicknell, A. W. J., Godley, B. J., and Witt, M. J. (2016). Underwater noise levels in UK waters. Sci. Rep. 6, 36942. doi:10.1038/srep36942
Merchant, N. D., Faulkner, R. C., and Martinez, R. (2018). Marine noise budgets in practice. Conserv. Lett. 11, e12420–e12428. doi:10.1111/conl.12420
Merchant, N. D., Fristrup, K. M., Johnson, M. P., Tyack, P. L., Witt, M. J., Blondel, P., et al. (2015). Measuring acoustic habitats. Methods Ecol. Evol. 6, 257–265. doi:10.1111/2041-210X.12330
Miller, B. S., and Miller, E. J. (2018). The seasonal occupancy and diel behaviour of Antarctic sperm whales revealed by acoustic monitoring. Sci. Rep. 8, 5429–5512. doi:10.1038/s41598-018-23752-1
Nott, B. J. (2015). Long-endurance maritime surveillance with ocean glider networks. Monterey, California: Naval Postgraduate School.
Nowacek, D. P., Clark, C. W., Mann, D., others, , Rosenbaum, H. C., Golden, J. S., et al. (2015). Marine seismic surveys and ocean noise: Time for coordinated and prudent planning. Front. Ecol. Environ. 13, 378–386. doi:10.1890/130286
Nystuen, J. A. (1996). Acoustical rainfall analysis: Rainfall drop size distribution using the underwater sound field. J. Atmos. Ocean. Technol. 13, 74–84. doi:10.1175/1520-0426(1996)013<0074:ARARDS>2.0.CO;2
Quijano, J. E., Dosso, S. E., Dettmer, J., Zurk, L. M., Siderius, M., and Harrison, C. H. (2012). Bayesian geoacoustic inversion using wind-driven ambient noise. J. Acoust. Soc. Am. 131, 2658–2667. doi:10.1121/1.3688482
Radford, C. A., Jeffs, A. G., Tindle, C. T., and Montgomery, J. C. (2008a). Temporal patterns in ambient noise of biological origin from a shallow water temperate reef. Oecologia 156, 921–929. doi:10.1007/s00442-008-1041-y
Radford, C. A., Jeffs, A., Tindle, C., and Montgomery, J. C. (2008b). Resonating sea urchin skeletons create coastal choruses. Mar. Ecol. Prog. Ser. 362, 37–43. doi:10.3354/meps07444
Ricks, R., Grimmett, D., and Wakayama, C. (2012). Passive acoustic tracking for cueing a multistatic active acoustic tracking system. Oceans - Yeosu, 1–7. Program Book - OCEANS 2012 MTS/IEEE Yeosu: The Living Ocean and Coast - Diversity of Resources and Sustainable Activities. IEEE.
Stafford, K. M., Mellinger, D. K., Moore, S. E., and Fox, C. G. (2007). Seasonal variability and detection range modeling of baleen whale calls in the Gulf of Alaska, 1999–2002. J. Acoust. Soc. Am. 122, 3378–3390. doi:10.1121/1.2799905
Suberg, L., Wynn, R. B., Van Der Kooij, J., Fernand, L., Fielding, S., Guihen, D., et al. (2014). Assessing the potential of autonomous submarine gliders for ecosystem monitoring across multiple trophic levels (plankton to cetaceans) and pollutants in shallow shelf seas. Methods Oceanogr. 10, 70–89. doi:10.1016/j.mio.2014.06.002
Tesei, A., Been, R., Williams, D., Cardeira, B., Galletti, D., Cecchi, D., et al. (2015). Passive acoustic surveillance of surface vessels using tridimensional array on an underwater glider. OCEANS 2015 - Genova. IEEE, 1–8.
Testor, P., Meyers, G., Pattiaratchi, C. B., and others, (2010). “Gliders as a component of future observing systems,” in Proceedings of OceanObs’09: Sustained Ocean Observations and Information for Society (Venice, Italy: European Space Agency), 961–978.
Testor, P., Turpin, V., DeYoung, B., others, , Hayes, D., Lee, C. M., et al. (2019). OceanGliders: A component of the integrated goos. Front. Mar. Sci. 6, 1–32. doi:10.3389/fmars.2019.00422
Thompson, P. M., Hastie, G. D., Nedwell, J., Barham, R., Brookes, K. L., Cordes, L. S., et al. (2013). Framework for assessing impacts of pile-driving noise from offshore wind farm construction on a harbour seal population. Environ. Impact Assess. Rev. 43, 73–85. doi:10.1016/j.eiar.2013.06.005
Thums, M., Ferreira, L. C., Jenner, C., others, , Harris, D., Davenport, A., et al. (2022). Pygmy blue whale movement, distribution and important areas in the Eastern Indian Ocean. Glob. Ecol. Conserv. 35, e02054. doi:10.1016/j.gecco.2022.e02054
Urick, R. J., and Kuperman, W. A. (1989). Ambient noise in the sea. J. Acoust. Soc. Am. 86, 1626. doi:10.1121/1.398683
Vagle, S., Large, W. G., and Farmer, D. M. (1990). An evaluation of the WOTAN technique of inferring oceanic winds from underwater ambient sound. J. Atmos. Ocean. Technol. 7, 576–595. doi:10.1175/1520-0426(1990)007<0576:AEOTWT>2.0.CO;2
Van der Graaf, A. J., Ainslie, M. A., and André, M. 2012. European marine strategy framework directive good environmental status (MSFD-GES).
Verfuss, U. K., Aniceto, A. S., Harris, D. V., others, , Fielding, S., Jimenez, G., et al. (2019). A review of unmanned vehicles for the detection and monitoring of marine fauna. Mar. Pollut. Bull. 140, 17–29. doi:10.1016/J.MARPOLBUL.2019.01.009
Wahlberg, M. (2002). The acoustic behaviour of diving sperm whales observed with a hydrophone array. J. Exp. Mar. Bio. Ecol. 281, 53–62. doi:10.1016/S0022-0981(02)00411-2
Wall, C. C., Mann, D. A., Lembke, C., Taylor, C., He, R., and Kellison, T. (2017). Mapping the soundscape off the southeastern USA by using passive acoustic glider technology. Mar. Coast. Fish. 9, 23–37. doi:10.1080/19425120.2016.1255685
Wall, C., Lembke, C., and Mann, D. (2012). Shelf-scale mapping of sound production by fishes in the eastern Gulf of Mexico, using autonomous glider technology. Mar. Ecol. Prog. Ser. 449, 55–64. doi:10.3354/meps09549
Keywords: glider, ocean gliders, PAM glider, passive acoustic monitoring (PAM), good practices, soundscape, underwater noise, ambient noise
Citation: Cauchy P, Heywood KJ, Merchant ND, Risch D, Queste BY and Testor P (2023) Gliders for passive acoustic monitoring of the oceanic environment. Front. Remote Sens. 4:1106533. doi: 10.3389/frsen.2023.1106533
Received: 23 November 2022; Accepted: 26 January 2023;
Published: 09 February 2023.
Edited by:
Xiuming Wang, Institute of Acoustics (CAS), ChinaReviewed by:
Jaroslaw Tegowski, University of Gdansk, PolandElias Fakiris, University of Patras, Greece
Copyright © 2023 Cauchy, Heywood, Merchant, Risch, Queste and Testor. This is an open-access article distributed under the terms of the Creative Commons Attribution License (CC BY). The use, distribution or reproduction in other forums is permitted, provided the original author(s) and the copyright owner(s) are credited and that the original publication in this journal is cited, in accordance with accepted academic practice. No use, distribution or reproduction is permitted which does not comply with these terms.
*Correspondence: Pierre Cauchy, cGllcnJlX2NhdWNoeUB1cWFyLmNh