- Science and Technology Research Laboratories, Japan Broadcasting Corporation, Tokyo, Japan
This study investigates the effects of multimodal cues on visual field guidance in 360° virtual reality (VR). Although this technology provides highly immersive visual experiences through spontaneous viewing, this capability can disrupt the quality of experience and cause users to miss important objects or scenes. Multimodal cueing using non-visual stimuli to guide the users’ heading, or their visual field, has the potential to preserve the spontaneous viewing experience without interfering with the original content. In this study, we present a visual field guidance method that imparts auditory and haptic stimulations using an artificial electrostatic force that can induce a subtle “fluffy” sensation on the skin. We conducted a visual search experiment in VR, wherein the participants attempted to find visual target stimuli both with and without multimodal cues, to investigate the behavioral characteristics produced by the guidance method. The results showed that the cues aided the participants in locating the target stimuli. However, the performance with simultaneous auditory and electrostatic cues was situated between those obtained when each cue was presented individually (medial effect), and no improvement was observed even when multiple cue stimuli pointed to the same target. In addition, a simulation analysis showed that this intermediate performance can be explained by the integrated perception model; that is, it is caused by an imbalanced perceptual uncertainty in each sensory cue for orienting to the correct view direction. The simulation analysis also showed that an improved performance (synergy effect) can be observed depending on the balance of the uncertainty, suggesting that a relative amount of uncertainty for each cue determines the performance. These results suggest that electrostatic force can be used to guide 360° viewing in VR, and that the performance of visual field guidance can be improved by introducing multimodal cues, the uncertainty of which is modulated to be less than or comparable to that of other cues. Our findings on the conditions that modulate multimodal cueing effects contribute to maximizing the quality of spontaneous 360° viewing experiences with multimodal guidance.
1 Introduction
The presentation of multimodal sensory information in virtual reality (VR) can considerably enhance the sense of presence and immersion. In daily life, we perceive the surrounding physical world through multiple senses, such as visual, auditory, and haptic senses, and interact with it based on these perceptions (Gibson, 1979; Flach and Holden, 1998; Dalgarno and Lee, 2010). Therefore, introducing multimodal stimulations into VR can enhance realism and significantly improve the experience. In fact, numerous studies have reported the benefits of multimodal VR (Mikropoulos and Natsis, 2011; Murray et al., 2016; Wang et al., 2016; Martin et al., 2022; Melo et al., 2022).
Head-mounted displays (HMDs) offer a highly immersive visual experience by spontaneously allowing users to view a 360° visual world; however, this feature may disrupt the 360° viewing experience, causing users to miss important objects or scenes that are located outside their visual field, thereby resulting in the “out-of-view” problem (Gruenefeld, El Ali, et al., 2017b). In 360° VR, the visual field of the user is defined by the viewport of the HMD. As nothing is presented outside the viewport, users have no opportunity for perception without changing the head direction. To address this problem, the presentation of arrows (Lin Y.-C et al., 2017; Schmitz et al., 2020; Wallgrun et al., 2020), peripheral flickering (Schmitz et al., 2020; Wallgrun et al., 2020), and picture-in-picture previews and thumbnails (Lin Y. T. et al., 2017; Yamaguchi et al., 2021) have been employed and shown to guide the gaze and visual attention effectively. However, these approaches also exhibit the problem of inevitably interfering with the video content, potentially disrupting the spontaneous viewing experience and mitigating the benefit of 360° video viewing (Sheikh et al., 2016; Pavel et al., 2017; Tong et al., 2019). Addressing this problem will significantly improve the 360° video viewing experience, especially for VR content with fixed time events, such as live scenes, movies, and dramas.
Several studies have explored the potential of multimodal stimuli to guide user behavior in 360° VR while preserving the original content (Rothe et al., 2019; Malpica, Serrano, Allue, et al., 2020b). Diegetic cues based on non-visual sensory stimuli such as directional sound emanating from a VR scene provide natural and intuitive guidance that feels appropriate in VR settings (Nielsen et al., 2016; Sheikh et al., 2016; Rothe et al., 2017; Rothe and Hußmann, 2018; Tong et al., 2019), exhibiting good compatibility with immersive 360° video viewing. At present, audio output is usually supported by any available HMDs and is the most common cue for visual field guidance. Because visual field guidance using non-visual stimuli is expected to provide high-quality VR experiences (Rothe et al., 2019), extensive research on various multimodal stimulation methods, including haptic stimulation, can aid the design of better VR experiences.
This study introduces electrostatic force stimuli to guide user behavior in selecting visual images that are displayed on the HMD (Figure 1). Previous studies have shown that applying an electrostatic force to the human body can induce a “fluffy” haptic sensation (Fukushima and Kajimoto, 2012a; 2012b; Suzuki et al., 2020; Karasawa and Kajimoto, 2021). Unlike some species of fish, amphibians, and mammals, humans do not possess electroreceptive abilities that allow them to perceive electric fields directly (Proske et al., 1998; Newton et al., 2019; Hüttner et al., 2023). However, as discussed in Karasawa and Kajimoto (2021), the haptic sensations that are produced through electrostatic stimulation are strongly related to the hair on the skin. Therefore, humans can indirectly perceive electrostatic stimulation through cutaneous mechanoreceptors (Horch et al., 1977; Johnson, 2001; Zimmerman et al., 2014), which are primarily stimulated by hair movements owing to electrostatic forces. Perceiving the physical world through cutaneous haptic sensations is a common experience in daily life, such as feeling the movement of air, and is expected to be a candidate method to guide user behavior naturally.
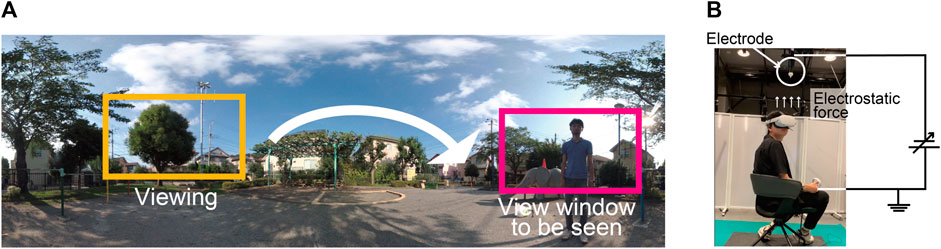
Figure 1. Visual field guidance in 360° VR using electrostatic force stimuli to mitigate the out-of-view problem. (A) Gentle visual field guidance. A user is viewing the scene depicted in the orange frame, whereas an important situation exists in the scene depicted in the red frame. Guiding the visual field to the proper direction will improve the user experience. (B) Haptic stimulus presentation using electrostatic forces. Electrostatic force helps the user to discover the important scene without affecting the original 360° VR content.
Many studies have proposed various methods of providing haptic sensations for visual field guidance, such as vibrations (Matsuda et al., 2020), normal forces on the face (Chang et al., 2018), and muscle stimulation (Tanaka et al., 2022), demonstrating that multimodal stimulation can improve the VR experience. Electrostatic force stimulation also provides haptic sensations, but can stimulate a relatively large area of the human body in a “fluffy” and subtle manner, which differs significantly from stimuli produced by other tactile stimulation methods, such as direct vibration stimulation through actuators. Karasawa and Kajimoto (2021) showed that electrostatic force stimulation can provide a feeling of presence. Previously, Slater (2009) and Slater et al. (2022) provided two views of immersive VR experiences, namely, place illusion (PI) and plausibility illusion (Psi), which refer to the sensation of being in a real place and the illusion that the depicted scenario is actually occurring, respectively. In this sense, the effects of haptic stimulation on user experiences in VR belong to Psi. Such fluffy, subtle stimulation of the skin by electrostatic force has the potential to simulate the sensations of airflow, chills, and goosebumps, which are common daily-life experiences. The introduction of such modalities will enhance the plausibility of VR and lead to better VR experiences.
In this study, we presented electrostatic force stimuli using corona discharge, which is a phenomenon wherein ions are continuously emitted from a needle electrode at high voltages, allowing the provision of stimuli from a distance. Specifically, we placed the electrode above the user’s head to stimulate a large area, from the head to the body (Figure 1B). Previous studies have employed plate- or pole-shaped electrodes to present such stimuli (Fukushima and Kajimoto, 2012a; 2012b; Karasawa and Kajimoto, 2021) and required the user to place their forearm close to the electrodes of the stimulation device, thereby limiting their body movement. The force becomes imperceptible even if the body parts are located 10 cm from the electrode (Karasawa and Kajimoto, 2021). As a typical VR user moves more than this distance, these conventional methods are not suitable for some VR applications that require physical movement. In addition, these devices are too bulky to be worn on the body. The proposed method can potentially overcome this limitation of distance and provide haptic sensations to VR users from a distance, thereby enabling the use of electrostatic force stimulation for visual field guidance in VR.
We evaluated the proposed visual field guidance method using multimodal cues in a psychophysical experiment. Previous studies have systematically evaluated visual field guidance using visual cues (Gruenefeld, Ennenga, et al., 2017a; Gruenefeld, El Ali, et al., 2017b; Danieau et al., 2017; Gruenefeld et al., 2018; 2019; Harada and Ohyama, 2022) in VR versions of visual search experiments (Treisman and Gelade, 1980; McElree and Carrasco, 1999). This study similarly investigated the effects of multimodal cues on visual searching.
Although numerous studies have shown that multiple modalities in VR can significantly improve the immersive experience (Ranasinghe et al., 2017; 2018; Cooper et al., 2018), it is unclear whether visual field guidance can also be improved by introducing multiple non-overt cues. We believe that multiple overt cues, such as visual arrows and halos, would help users to perform search tasks. However, this is not necessarily true for non-overt, subtle, and vague cues. Although guidance through subtle cues can minimize content intrusion (Bailey et al., 2009; Nielsen et al., 2016; Sheikh et al., 2016; Bala et al., 2019), it is not always guaranteed to be effective (Rothe et al., 2018). However, employing multiple subtle cues and integrating them into a coherent cue may provide effective overall guidance. In this study, in addition to electrostatic forces, we introduced weak auditory stimuli as subtle environmental cues to investigate the interaction effects of electrostatic and auditory cues on the guidance performance in VR as well as whether they improve, worsen, or have no effect on the guidance performance.
The nature of multimodal perception, which involves the integration of various sensory inputs to produce a coherent perception, has been understood using statistical models, such as maximum likelihood estimation and integration based on Bayes’ theorem (Ernst and Banks, 2002; Ernst, 2006; 2007; Spence, 2011). Although such computational modeling approaches are also expected to aid in comprehending the underlying mechanisms of multimodal cueing effects on visual field guidance, to the best of our knowledge, this aspect remains unexplored. Therefore, we adopted a similar approach using computational models and investigated the effects of various cueing conditions on visual field guidance. Thus, this study offers a detailed understanding of multimodal visual field guidance and knowledge for predicting user behavior under various cue conditions.
We first introduce electrostatic force and auditory stimuli as multimodal cues in a visual search task and then show that electrostatic force can potentially address the out-of-view problem. Because auditory stimuli have been commonly used in previous studies to guide user behavior (Walker and Lindsay, 2003; Rothe et al., 2017; Bala et al., 2018; Malpica, Serrano, Allue, et al., 2020a; Malpica, Serrano, Gutierrez, et al., 2020b; Chao et al., 2020; Masia et al., 2021), a baseline is provided for comparisons. In the visual search task, the participants were instructed to find a specific visual target as quickly as possible in 360° VR, both with and without sensory cues. We anticipated that the cueing would reduce the cumulative travel angles associated with updating the head direction during the search. Therefore, a comparison of the task performances in each condition revealed the effect of multimodal cueing on the visual field guidance.
In this study, we hypothesized that performance with multimodal cueing in the visual search task in VR would show one of the following three effects: 1) a performance improvement compared to that with electrostatic force or auditory cues (synergy effect); 2) the same performance as that with the better cue, not considering the performance worth the other cue (masking effect); and 3) performance between the individual performances with each cue (medial effect). We conducted a psychophysical experiment to investigate which of these effects were observed with multimodal cues. Subsequently, through the psychophysical experiment and an additional simulation analysis, we demonstrated that both the synergy and medial effects can be observed depending on the balance of perceptual uncertainties for each cue and the variance in the selection of the head direction. Finally, we investigated the conditions for effective multimodal visual field guidance.
2 Materials and methods
2.1 Visual search experiment with multimodal cues
This subsection describes the experiment that was conducted to investigate the effects of visual field guidance on visual search performance in 360° VR using haptic and auditory cues. In addition, the multimodal effects of simultaneous cueing using haptic and auditory stimuli were investigated. The search performance was measured based on the travel angles, which are the cumulative rotation angles of the head direction, as described in detail in Section 2.2.1.1. Finally, we determined which of the effects, namely, synergy, medial, or masking, were likely by comparing the travel angles obtained in each cue condition.
2.1.1 Participants
Fifteen participants (seven male, eight female; aged 21–33 years, mean: 24.4) were recruited for this experiment. All participants had normal or corrected-to-normal vision. Two participants were excluded because their psychological thresholds for the electrostatic force stimuli were too high and exceeded the intensity range that our apparatus could present. Informed consent was obtained from all participants, and the study design was approved by the ethics committee of the Science and Technology Research Laboratories, Japan Broadcasting Corporation.
2.1.2 Apparatus
A corona charging gun (GC90N, Green Techno, Japan) was used to present electrostatic force stimuli. This device comprises a needle-shaped electrode (ion-emitting gun) and a high-voltage power supply unit (rated voltage range: 0 to −90 [kV]). The electrostatic force intensity was modulated by adjusting the applied voltage. The gun was hung from the ceiling and placed approximately 50 cm above the participant’s head, as shown in Figure 1B. In addition, the participant wore a wristband attached to the ground to avoid accidental shocks owing to unintentional charging.
A standalone HMD, Meta Quest 2 (Meta, United States), was used to present the 360° visual images and auditory stimuli, and the controller joystick (for the right hand) was used to collect the responses. The HMD communicated with the corona charging gun via an Arduino-based microcomputer (M5Stick-C PLUS, M5Stack Technology, China) to control the analog inputs for the gun. The delay between the auditory and electrostatic force stimuli was a maximum of 20 ms, which was sufficiently small to perform the task. The participants viewed the 360° images while sitting in a swivel chair to facilitate viewing. They wore wired earphones (SE215, SURE, United States), which were connected to the HMD and used to present auditory stimuli using functions provided in Unity (Unity Technologies, United States) throughout the experiment, even when no auditory stimuli were presented. The experimental room was soundproof. Participant safety was duly considered; the floor was covered with an electrically grounded conductive mat, which collected ions that were not meant for the participant, thereby preventing unintentional charging of other objects in the room.
2.1.3 Stimuli
2.1.3.1 Visual stimuli
The target and distractor stimuli were presented in a VR environment implemented in Unity (2021.3.2 f1). The target stimulus included a randomly selected white symbol among “├“, “┤“, “┬“, and “┴,” whereas the distractor stimuli included white “┼” symbols. These stimuli were displayed on a gray background and distributed within a range of -10°–10° relative to each intersection of the latitudes and longitudes of a sphere with a 5-m radius that was centered at the origin. The referential latitudes and longitudes were placed at each 36° position of the horizontal 360° view and 22.5° positions between the elevation angles of −45° and 45°. Thus, 1 target and 39 distractor stimuli were presented at 10 × 4 locations. The stimuli sizes were randomly selected from visual angles ranging from 2.86° ± 1.43°, both horizontally and vertically. The difficulty of the task was modulated by varying the stimulus size and placement and the parameter values were selected based on our preliminary experiments.
2.1.3.2 Electrostatic force stimuli
In this study, the electrostatic force stimuli are referred to as haptic stimuli induced by the corona charging gun. The electrostatic force intensity was determined based on the gun voltage. We selected the physical intensity of the electrostatic force for each participant based on their psychological threshold; the intensity ranged from zero to twice the threshold. Thus, we ensured that the stimulus intensity was psychologically equivalent among all participants. The threshold
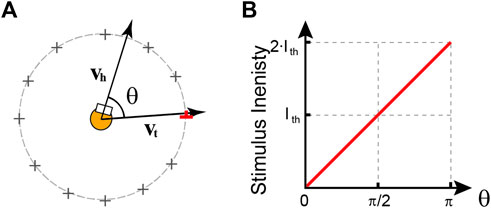
Figure 2. Stimulus intensity modulation. The stimulus intensity was linearly modulated in response to the inner angle,
2.1.3.3 Auditory stimuli
Monaural white noise was used as the auditory stimulus. We used the same modulation method for the auditory stimuli as that for the electrostatic force stimuli, as shown in Figure 2. Specifically, we linearly modulated the stimulus intensity in response to the inner angle
2.1.4 Task and conditions
We designed a within-participant experiment to compare the effects of haptic and auditory guidance in a visual search task. The participants were instructed to find the target stimulus and indicate its direction using the joystick on the VR controller. For example, when they discovered a target stimulus “┴,” they tilted the joystick upward as quickly as possible. The trial was terminated once the joystick was manipulated. Feedback was provided between sessions, showing the success rate of the previous session, to encourage participants to complete the task. The task was conducted both with and without sensory cues, resulting in four conditions based on the combinations of cue stimuli: visual only (V), vision with auditory (A), vision with electrostatic force (E), and vision with auditory and electrostatic force (AE) cues.
2.1.5 Procedure
The experiment included 12 sessions comprising 12 visual search trials, for a total of 144 trials per participant. Therefore, each condition (V, A, E, and AE) was presented 36 times in one experiment. In three of the 12 sessions, only condition V was presented, whereas in the other sessions, conditions A, E, and AE were presented in a pseudo-random order. Before each session, we informed the participants whether the next session would be a V-only session or a session with the non-visual-cued conditions. This prevented participants from waiting for non-visual cues during condition V and inadvertently wasting search time.
Each trial comprised a rest period of variable-length (3–6 s) and a 10-s search period. In the rest period, 40 randomly generated distractors were presented, whereas in the following search period, one of the distractors was replaced with a target stimulus. The trials progressed as soon as the target stimulus was found or when the 10-s time limit was reached. Note that the participants underwent two practice sessions to understand the task and response methods prior to these sessions.
2.2 Analysis
2.2.1 Behavioral data analysis
2.2.1.1 Modeling
We recorded the participants’ responses and extents of their head movements during the search period. The trials with a correct response were labeled as successful, whereas those with an incorrect or no response were labeled as failed. The travel angle was defined as the accumulated rotational changes in the head direction during the target search. If guidance by electrostatic forces and auditory cues is effective, the travel angles should be shorter than those with no cues. Therefore, we investigated the modulation efficiency of the target discovery according to cue type.
The travel angle allowed us to model the participants’ behavior in the visual search experiment with non-overt multimodal cues appropriately. In the original visual search experiment (Treisman and Gelade, 1980; McElree and Carrasco, 1999), wherein participants had to find the target stimuli with specified visual features as quickly as possible, the performance was measured by the reaction time required for identification. These experimental paradigms have recently been extended to investigate user behavior in VR. Cue-based visual search experiments in VR involve the analysis of reaction times and/or movement angles towards a target object (Gruenefeld, Ennenga, et al., 2017a; Gruenefeld, El Ali, et al., 2017b; Danieau et al., 2017; Gruenefeld et al., 2018; 2019; Schmitz et al., 2020; Harada and Ohyama, 2022). In addition, previous studies employed overt cues that directly indicated the target location, whereas we employed non-overt cues that weakly indicated them, without interfering with the visuals. This difference could have affected the behavior of participants, depending on their individual traits. For example, some participants may have adopted a scanning strategy wherein they sequentially scanned the surrounding visual world, ignoring the cues because they considered subtle cues to be unreliable. Participants with better physical ability could have completed the task faster using this strategy. In such cases, the reaction time would not accurately reflect the effects of cueing on the visual search performance and the effects would differ significantly from those we were investigating. Because behaviors including scanning that are not based on presented cues would result in larger travel angles, the effects of cues would likely be better reflected in the travel angle than in the reaction time. Therefore, we employed travel angles instead of reaction times to evaluate the performance.
We employed Bayesian modeling to evaluate the efficacy of each cue, as follows:
where
Thus,
2.2.1.2 Poisson process model derivation
The total travel angle
By minimizing the bin width using
2.2.1.3 Statistics
We created a dataset by pooling all observations that were obtained from the participants. Thereafter, we obtained the posterior distributions of the target discovery rate,
2.3 Simulation analysis
2.3.1 Overview
To better comprehend how participants processed the multimodal inputs in the experiment, we conducted a simulation analysis assuming a perceptual model wherein a participant determined the head direction by simply averaging two vectors directed towards the target induced through auditory and haptic sensations, as shown in Figure 3, constituting the most typical explanation of the multimodal effect (Ernst and Banks, 2002; Ernst, 2006; 2007). We manipulated the noise levels
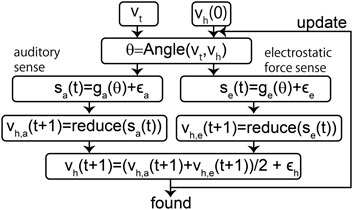
Figure 3. Perceptual model of visual search with multimodal cues. The possible head directions were estimated separately based on the synthesized auditory and electrostatic force sensations generated by
We implemented a computational model to determine the target stimulus direction based on the synthesized sensations. The head direction vector at time
The simulation was initially conducted using randomly generated
We ran the simulation using the parameter settings that were closest to those used in the real experiment; for example, the maximum amount and speed of head rotation and the number of trials were appropriately selected. The simulation was performed 468 times for each condition, corresponding to the setup in the real experiment (36 trials × 13 participants). The travel angle and target discovery rate were computed using the methods described in Section 2.2.1.1. To examine the effects of
2.3.2 Procedure
In this section, we describe the details of the simulation, as summarized in Section 2.3.1 and Figure 3.
The simulation model iteratively updated the head direction vector
where
Finally, the next head direction vector was obtained as follows:
where
where
We define a head-direction matrix
where
Therefore, by substituting
Initially,
The target search was conducted using a maximum of 1000 steps. The simulation parameter values of
3 Results
3.1 Behavioral results
We pooled all data obtained from the 13 participants. The number of successful trials
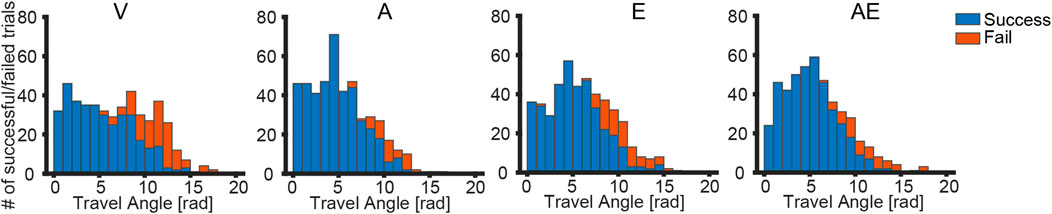
Figure 4. Relationships between the number of discoveries and travel angles. The data of 13 participants were pooled. The panels, from left to right, shows the relationships for each condition: vision only (V), vision + auditory cue (A), vision + electrostatic force cue (E), and vision + auditory and electrostatic force cues (AE).
Figure 5 shows the posterior distributions of
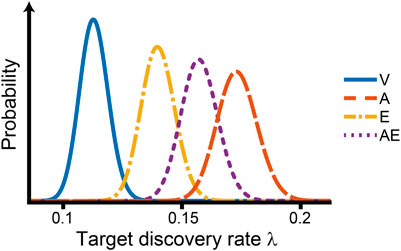
Figure 5.
We observed that the performance in condition AE was situated between those in conditions A and E, thereby rejecting the possibilities of synergy and masking effects because the search using both cues did not enhance the performance and the participants could not ignore the other cue. This result supports the medial effect, which was one of the anticipated candidates.
3.2 Simulation results
Figure 6 shows
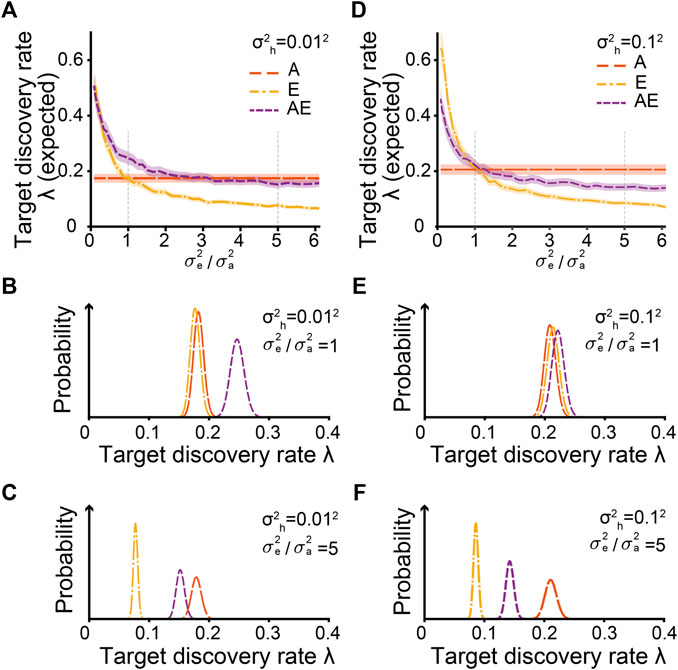
Figure 6. Comparisons of
As shown in Figures 6A, D, the expected
4 Discussion
We demonstrated the multimodal effects of AE cues on visual field guidance in 360° VR, and found that both medial and synergy effects were observable depending on the uncertainty of the cue stimuli through the psychophysical experiment and the simulation analysis. Specifically, guidance performance with multimodal cueing is modulated by balancing the perceptual uncertainty elicited by each cue stimulus. We also demonstrated that the applicability of the electrostatic force-based stimulation method in VR applications; electrostatic stimulation through the corona charging gun allowed users to make large body movements. These results suggest that multimodal cueing with electrostatic force has sufficient potential to guide user behavior in 360° VR gently, offering a highly immersive visual experience through spontaneous viewing.
We showed that electrostatic force can be used as a haptic cue to guide the visual field. However, the search performance did not reach that with the auditory cue, even though we selected cue intensities that varied equally in small ranges around the supra- and sub-thresholds, with no significant difference in the perceptual domain. In the informal post-experiment interviews, some participants reported that the sensation induced by the electrostatic force was attenuated, especially while moving. In addition, most participants reported that the auditory cue made it easier to identify the target location. This suggests that the haptic sensation was affected by body motion that inevitably accompanied the updating of the head direction. The increased uncertainty for the haptic sensation was estimated to be approximately five times greater than that for the auditory sensation, as suggested by the simulation results (Figure 6F). Thus, the perception of changes in the stimulus intensity associated with visual field updates acts as a cue for estimating the target direction, which means that increasing the electrostatic field intensity such that it is strong enough to resist the effects of body motion could mitigate this uncertainty. As suggested by the simulation results presented in Section 3.2, reducing the perceptual uncertainty improves the search performance. This finding has been overlooked in previous studies that mainly focused on visual field guidance using overt cue stimuli (Gruenefeld, Ennenga, et al., 2017a; Gruenefeld, El Ali, et al., 2017b; Danieau et al., 2017; Gruenefeld et al., 2018; 2019; Harada and Ohyama, 2022). This results in the requirement for the property of cue stimuli to improve performance in multimodal visual field guidance.
The medial effect might have been counterintuitive because participants received more information regarding the target stimulus from multimodal cues than unimodal cues. Because the cues conveyed the same information, the synergy effect was more likely if participants used the received information properly. The simulation analysis showed that both effects could be observed under specific noise settings. This can also be explained theoretically: let
where
Addressing the out-of-view problem has been a major challenge in 360° VR video viewing (Lin Y. T. et al., 2017; Schmitz et al., 2020; Wallgrun et al., 2020; Yamaguchi et al., 2021). Gentle and diegetic guidance that does not interfere with the visual content has received substantial attention from VR content providers (Nielsen et al., 2016; Sheikh et al., 2016; Rothe et al., 2017; Rothe and Hußmann, 2018; Bala et al., 2019; Tong et al., 2019). This study showed that subtle cues using artificial electrostatic force can guide the visual field, thereby demonstrating the application potential for 360° VR. Whereas previous studies using static electricity have severely limited the movements of the user (Fukushima and Kajimoto, 2012b; 2012a; Karasawa and Kajimoto, 2021), the use of the corona discharge phenomenon mitigated this limitation. The simulation analysis using the computational model helped to provide an understanding of the mechanisms of multimodal cueing. Similar to the observations in this study, previous studies using non-overt cues with perceptual uncertainty have reported both positive and negative effects of multimodal cueing in 360° VR (Sheikh et al., 2016; Rothe and Hußmann, 2018; Bala et al., 2019; Malpica, Serrano, Gutierrez, et al., 2020a). We believe that our results also provide a rational explanation for these previous findings.
However, this study had some limitations. Some participants exhibited insufficient sensitivity to the electrostatic force stimuli. Although their hair moved when they were exposed to static electricity, they reported low sensations, which may be caused by skin moisture or other factors; however, this phenomenon has not yet been investigated. Furthermore, as humans are incapable of electroreception, it is reasonable to believe that the mechanoreceptors in the skin are involved in providing the sensations (Horch et al., 1977; Johnson, 2001; Zimmerman et al., 2014); however, this must be investigated further. In addition, the wristband used to tether the participants to the ground may have restricted free body movement; this can be addressed by introducing an ionizer that remotely neutralizes the charge level (Ohsawa, 2005), thereby allowing participants to move freely. Finally, the results presented in this study were obtained under reductive conditions. While the results provide insight into stimulus design, further experiments are required to demonstrate the effectiveness in real-world VR applications such as video viewing and gaming, which will be the focus of our future study.
In future work, we will implement electrostatic stimulation in a VR application. We believe that haptic stimulation by electrostatic force could be used not only to guide the visual field, but also to enhance the user’s subjective impression. Although this has not been discussed here, we have experimentally implemented a VR game wherein a user shoots zombies charged with static electricity approaching from all sides. The electrostatic force-based stimulus can result in unpleasant sensations. Other haptic stimuli, such as vibrations, could also be used to cue the zombies. However, we believe that these stimuli are too obvious and artificial, and may detract from the subjective quality of experience to a certain extent. The use of static electricity can result in an unsettling experience for users when charged zombies approach them from behind. Thus, by comparing the effects of electrostatic force and other haptic stimuli on subjective impressions, we will be able to demonstrate the availability of electrostatic force-based stimulation to provide a highly immersive experience.
5 Conclusion
We investigated the multimodal effects of auditory and electrostatic force-based haptic cues on visual field guidance in 360° VR, demonstrating the potential for a visual field guidance method that does not interfere with the visual content. We found that modulating the degree of perceptual uncertainty for each cue improves the overall guidance performance under simultaneous multimodal cueing. Moreover, we presented a simple haptic stimulation method using only a single channel of a corona charging gun. In the future, we will increase the number of channels to present more complex stimulations in a larger area by dynamically controlling the electric fields, allowing for remote haptic stimulation under a six-degrees-of-freedom viewing condition. Finally, our results showed that multimodal stimuli have the potential to increase the richness in VR environments.
Data availability statement
The raw data supporting the conclusion of this article will be made available by the authors, upon reasonable request.
Ethics statement
The studies involving humans were approved by Japan Broadcasting Corporation. The studies were conducted in accordance with the local legislation and institutional requirements. The participants provided their written informed consent to participate in this study.
Author contributions
YS: Conceptualization, Methodology, Formal analysis, Writing–original draft, Writing–review and editing. MH: Supervision, Writing–review and editing. KK: Project administration, Writing–review and editing.
Funding
The author(s) declare that no financial support was received for the research, authorship, and/or publication of this article.
Conflict of interest
Authors YS, MH, and KK were employed by Japan Broadcasting Corporation.
Publisher’s note
All claims expressed in this article are solely those of the authors and do not necessarily represent those of their affiliated organizations, or those of the publisher, the editors and the reviewers. Any product that may be evaluated in this article, or claim that may be made by its manufacturer, is not guaranteed or endorsed by the publisher.
References
Bailey, R., McNamara, A., Sudarsanam, N., and Grimm, C. (2009). Subtle gaze direction. ACM Trans. Graph. 28 (4), 1–14. doi:10.1145/1559755.1559757
Bala, P., Masu, R., Nisi, V., and Nunes, N. (2018). “Cue control: interactive sound spatialization for 360° videos,” in Interactive storytelling. ICIDS 2018. Lecture notes in computer science, vol 11318 Editors R. Rouse, H. Koenitz, and M. Haahr (Cham: Springer), 333–337. doi:10.1007/978-3-030-04028-4_36
Bala, P., Masu, R., Nisi, V., and Nunes, N. (2019). “When the elephant trumps,” in Proceedings of the 2019 CHI Conference on Human Factors in Computing Systems, Glasgow, Scotland, UK, May 4-9, 2019, 1–13.
Chang, H.-Y., Tseng, W.-J., Tsai, C.-E., Chen, H.-Y., Peiris, R. L., and Chan, L. (2018). “FacePush: introducing normal force on face with head-mounted displays,” in Proceedings of the 31st Annual ACM Symposium on User Interface Software and Technology, 927–935.
Chao, F. Y., Ozcinar, C., Wang, C., Zerman, E., Zhang, L., Hamidouche, W., et al. (2020). “Audio-visual perception of omnidirectional video for virtual reality applications,” in 2020 IEEE International Conference on Multimedia and Expo Workshops, ICMEW 2020, 2–7.
Cooper, N., Milella, F., Pinto, C., Cant, I., White, M., and Meyer, G. (2018). The effects of substitute multisensory feedback on task performance and the sense of presence in a virtual reality environment. PLOS ONE 13 (2), e0191846. doi:10.1371/journal.pone.0191846
Dalgarno, B., and Lee, M. J. W. (2010). What are the learning affordances of 3-D virtual environments? Br. J. Educ. Technol. 41 (1), 10–32. doi:10.1111/j.1467-8535.2009.01038.x
Danieau, F., Guillo, A., and Doré, R. (2017). “Attention guidance for immersive video content in head-mounted displays,” in 2017 IEEE Virtual Reality (VR), 205–206.
Ernst, M. O. (2006). “A bayesian view on multimodal cue integration,” in Human body perception from the inside out: advances in visual cognition (Oxford University Press), 105–131.
Ernst, M. O. (2007). Learning to integrate arbitrary signals from vision and touch. J. Vis. 7 (5), 7. doi:10.1167/7.5.7
Ernst, M. O., and Banks, M. S. (2002). Humans integrate visual and haptic information in a statistically optimal fashion. Nature 415 (6870), 429–433. doi:10.1038/415429a
Flach, J. M., and Holden, J. G. (1998). The reality of experience: gibson’s way. Presence 7 (1), 90–95. doi:10.1162/105474698565550
Fukushima, S., and Kajimoto, H. (2012a). “Chilly chair: facilitating an emotional feeling with artificial piloerection,” in ACM SIGGRAPH 2012 emerging Technologies (SIGGRAPH ’12), 1, article, 5–1. doi:10.1145/2343456.2343461
Fukushima, S., and Kajimoto, H. (2012b). “Facilitating a surprised feeling by artificial control of piloerection on the forearm,” in Proceedings of the 3rd Augmented Human International Conference (AH ’12), Article 8, 1–4.
Gruenefeld, U., El Ali, A., Boll, S., and Heuten, W. (2018). “Beyond halo and wedge: visualizing out-of-view objects on head-mounted virtual and augmented reality devices,” in Proceedings of the 20th International Conference on Human-Computer Interaction with Mobile Devices and Services (MobileHCI ’18), 1–11.
Gruenefeld, U., El Ali, A., Heuten, W., and Boll, S. (2017a). “Visualizing out-of-view objects in head-mounted augmented reality,” in Proceedings of the 19th International Conference on Human-Computer Interaction with Mobile Devices and Services (MobileHCI ’17), Article 87, 1–7.
Gruenefeld, U., Ennenga, D., Ali, A.El, Heuten, W., and Boll, S. (2017b). “EyeSee360: designing a visualization technique for out-of-view objects in head-mounted augmented reality,” in Proceedings of the 5th Symposium on Spatial User Interaction (SUI ’17), 109–118.
Gruenefeld, U., Koethe, I., Lange, D., Weirb, S., and Heuten, W. (2019). “Comparing techniques for visualizing moving out-of-view objects in head-mounted virtual reality,” in 2019 IEEE Conference on Virtual Reality and 3D User Interfaces (VR), 742–746.
Harada, Y., and Ohyama, J. (2022). Quantitative evaluation of visual guidance effects for 360-degree directions. Virtual Real. 26 (2), 759–770. doi:10.1007/s10055-021-00574-7
Horch, K. W., Tuckett, R. P., and Burgess, P. R. (1977). A key to the classification of cutaneous mechanoreceptors. J. Investigative Dermatology 69 (1), 75–82. doi:10.1111/1523-1747.ep12497887
Hüttner, T., von Fersen, L., Miersch, L., and Dehnhardt, G. (2023). Passive electroreception in bottlenose dolphins (Tursiops truncatus): implication for micro- and large-scale orientation. J. Exp. Biol. 226 (22), jeb245845. doi:10.1242/jeb.245845
Johnson, K. (2001). The roles and functions of cutaneous mechanoreceptors. Curr. Opin. Neurobiol. 11 (4), 455–461. doi:10.1016/S0959-4388(00)00234-8
Karasawa, M., and Kajimoto, H. (2021). “Presentation of a feeling of presence using an electrostatic field: presence-like sensation presentation using an electrostatic field,” in Extended Abstracts of the 2021 CHI Conference on Human Factors in Computing Systems (CHI EA ’21), Article 285, 1–4.
Lin, Y.-C., Chang, Y.-J., Hu, H.-N., Cheng, H.-T., Huang, C.-W., and Sun, M. (2017). “Tell me where to look,” in Proceedings of the 2017 CHI Conference on Human Factors in Computing Systems, 2535–2545.
Lin, Y. T., Liao, Y. C., Teng, S. Y., Chung, Y. J., Chan, L., and Chen, B. Y. (2017). “Outside-in: visualizing out-of-sight regions-of-interest in a 360 video using spatial picture-in-picture previews,” in Proceedings of the 30th Annual ACM Symposium on User Interface Software and Technology (UIST ’17), 255–265.
Malpica, S., Serrano, A., Allue, M., Bedia, M. G., and Masia, B. (2020a). Crossmodal perception in virtual reality. Multimedia Tools Appl. 79 (5–6), 3311–3331. doi:10.1007/s11042-019-7331-z
Malpica, S., Serrano, A., Gutierrez, D., and Masia, B. (2020b). Auditory stimuli degrade visual performance in virtual reality. Sci. Rep. 10 (1), 12363–12369. doi:10.1038/s41598-020-69135-3
Martin, D., Malpica, S., Gutierrez, D., Masia, B., and Serrano, A. (2022). Multimodality in VR: a survey. ACM Comput. Surv. 54 (10s), 1–36. doi:10.1145/3508361
Masia, B., Camon, J., Gutierrez, D., and Serrano, A. (2021). Influence of directional sound cues on users’ exploration across 360° movie cuts. IEEE Comput. Graph. Appl. 41 (4), 64–75. doi:10.1109/MCG.2021.3064688
Matsuda, A., Nozawa, K., Takata, K., Izumihara, A., and Rekimoto, J. (2020). “HapticPointer,” in Proceedings of the Augmented Humans International Conference (AHs ’20), 1–10.
McElree, B., and Carrasco, M. (1999). The temporal dynamics of visual search: evidence for parallel processing in feature and conjunction searches. J. Exp. Psychol. Hum. Percept. Perform. 25 (6), 1517–1539. doi:10.1037/0096-1523.25.6.1517
Melo, M., Goncalves, G., Monteiro, P., Coelho, H., Vasconcelos-Raposo, J., and Bessa, M. (2022). Do multisensory stimuli benefit the virtual reality experience? A systematic review. IEEE Trans. Vis. Comput. Graph. 28 (2), 1428–1442. doi:10.1109/TVCG.2020.3010088
Mikropoulos, T. A., and Natsis, A. (2011). Educational virtual environments: a ten-year review of empirical research (1999–2009). Comput. Educ. 56 (3), 769–780. doi:10.1016/j.compedu.2010.10.020
Murray, N., Lee, B., Qiao, Y., and Muntean, G. M. (2016). Olfaction-enhanced multimedia: a survey of application domains, displays, and research challenges. ACM Comput. Surv. 48 (4), 1–34. doi:10.1145/2816454
Newton, K. C., Gill, A. B., and Kajiura, S. M. (2019). Electroreception in marine fishes: chondrichthyans. J. Fish Biol. 95 (1), 135–154. doi:10.1111/jfb.14068
Nielsen, L. T., Møller, M. B., Hartmeyer, S. D., Ljung, T. C. M., Nilsson, N. C., Nordahl, R., et al. (2016). “Missing the point,” in Proceedings of the 22nd ACM Conference on Virtual Reality Software and Technology, 229–232.
Ohsawa, A. (2005). Modeling of charge neutralization by ionizer. J. Electrost. 63 (6–10), 767–773. doi:10.1016/j.elstat.2005.03.043
Pavel, A., Hartmann, B., and Agrawala, M. (2017). “Shot orientation controls for interactive cinematography with 360° video,” in Proceedings of the 30th Annual ACM Symposium on User Interface Software and Technology (UIST ’17), 289–297.
Proske, U., Gregory, J. E., and Iggo, A. (1998). Sensory receptors in monotremes. Philosophical Trans. R. Soc. B Biol. Sci. 353 (1372), 1187–1198. doi:10.1098/rstb.1998.0275
Ranasinghe, N., Jain, P., Karwita, S., Tolley, D., and Do, E. Y.-L. (2017). “Ambiotherm,” in Proceedings of the 2017 CHI Conference on Human Factors in Computing Systems, 1731–1742.
Ranasinghe, N., Jain, P., Thi Ngoc Tram, N., Koh, K. C. R., Tolley, D., Karwita, S., et al. (2018). “Season traveller,” in Proceedings of the 2018 CHI Conference on Human Factors in Computing Systems, 1–13.
Rothe, S., Althammer, F., and Khamis, M. (2018). “GazeRecall: using gaze direction to increase recall of details in cinematic virtual reality,” in Proceedings of the 17th International Conference on Mobile and Ubiquitous Multimedia, 115–119.
Rothe, S., Buschek, D., and Hußmann, H. (2019). Guidance in cinematic virtual reality-taxonomy, research status and challenges. Multimodal Technol. Interact. 3 (1), 19. doi:10.3390/mti3010019
Rothe, S., and Hußmann, H. (2018). “Guiding the viewer in cinematic virtual reality by diegetic cues,” in Augmented reality, virtual reality, and computer graphics. AVR 2018. Lecture notes in computer science Editors L. De Paolis,, and P. Bourdot (Cham: Springer), 101–117. doi:10.1007/978-3-319-95270-3_7
Rothe, S., Hußmann, H., and Allary, M. (2017). “Diegetic cues for guiding the viewer in cinematic virtual reality,” in Proceedings of the 23rd ACM Symposium on Virtual Reality Software and Technology, 1–2.
Schmitz, A., Macquarrie, A., Julier, S., Binetti, N., and Steed, A. (2020). “Directing versus attracting attention: exploring the effectiveness of central and peripheral cues in panoramic videos,” in Proceedings - 2020 IEEE Conference on Virtual Reality and 3D User Interfaces, VR 2020, 63–72.
Sheikh, A., Brown, A., Watson, Z., and Evans, M. (2016). Directing attention in 360-degree video. IBC 2016 Conf., 1–9. doi:10.1049/ibc.2016.0029
Slater, M. (2009). Place illusion and plausibility can lead to realistic behaviour in immersive virtual environments. Philosophical Trans. R. Soc. B Biol. Sci. 364 (1535), 3549–3557. doi:10.1098/rstb.2009.0138
Slater, M., Banakou, D., Beacco, A., Gallego, J., Macia-Varela, F., and Oliva, R. (2022). A separate reality: an update on place illusion and plausibility in virtual reality. Front. Virtual Real. 3. doi:10.3389/frvir.2022.914392
Spence, C. (2011). Crossmodal correspondences: a tutorial review. Atten. Percept. Psychophys. 73 (4), 971–995. doi:10.3758/s13414-010-0073-7
Suzuki, K., Abe, K., and Sato, H. (2020). Proposal of perception method of existence of objects in 3D space using quasi-electrostatic field. Int. Conf. Human-Computer Interact., 561–571. doi:10.1007/978-3-030-49760-6_40
Tanaka, Y., Nishida, J., and Lopes, P. (2022). Electrical head actuation: enabling interactive systems to directly manipulate head orientation. Proc. 2022 CHI Conf. Hum. Factors Comput. Syst. 1, 1–15. doi:10.1145/3491102.3501910
Tong, L., Jung, S., and Lindeman, R. W. (2019). “Action units: directing user attention in 360-degree video based VR,” in 25th ACM Symposium on Virtual Reality Software and Technology, 1–2.
Treisman, A. M., and Gelade, G. (1980). A feature-integration theory of attention. Cogn. Psychol. 12 (1), 97–136. doi:10.1016/0010-0285(80)90005-5
Walker, B. N., and Lindsay, J. (2003). “Effect of beacon sounds on navigation performance in a virtual reality environment,” in Proceedings of the 9th International Conference on Auditory Display (ICAD2003), July, 204–207.
Wallgrun, J. O., Bagher, M. M., Sajjadi, P., and Klippel, A. (2020). “A comparison of visual attention guiding approaches for 360° image-based VR tours,” in 2020 IEEE Conference on Virtual Reality and 3D User Interfaces (VR), 83–91.
Wang, D., Li, T., Zhang, Y., and Hou, J. (2016). Survey on multisensory feedback virtual reality dental training systems. Eur. J. Dent. Educ. 20 (4), 248–260. doi:10.1111/eje.12173
Yamaguchi, S., Ogawa, N., and Narumi, T. (2021). “Now I’m not afraid: reducing fear of missing out in 360° videos on a head-mounted display using a panoramic thumbnail,” in 2021 IEEE International Symposium on Mixed and Augmented Reality (ISMAR), 176–183.
Keywords: out-of-view problem, visual field guidance, electrostatic force, haptics, multimodal processing, integrated perception model
Citation: Sawahata Y, Harasawa M and Komine K (2024) Synergy and medial effects of multimodal cueing with auditory and electrostatic force stimuli on visual field guidance in 360° VR. Front. Virtual Real. 5:1379351. doi: 10.3389/frvir.2024.1379351
Received: 31 January 2024; Accepted: 14 May 2024;
Published: 04 June 2024.
Edited by:
Justyna Świdrak, August Pi i Sunyer Biomedical Research Institute (IDIBAPS), SpainReviewed by:
Alejandro Beacco, University of Barcelona, SpainPierre Bourdin-Kreitz, Open University of Catalonia, Spain
Copyright © 2024 Sawahata, Harasawa and Komine. This is an open-access article distributed under the terms of the Creative Commons Attribution License (CC BY). The use, distribution or reproduction in other forums is permitted, provided the original author(s) and the copyright owner(s) are credited and that the original publication in this journal is cited, in accordance with accepted academic practice. No use, distribution or reproduction is permitted which does not comply with these terms.
*Correspondence: Yasuhito Sawahata, sawahata.y-jq@nhk.or.jp