- 1Department of Earth Sciences – Geochemistry, Faculty of Geosciences, Utrecht University, Utrecht, Netherlands
- 2PBL Netherlands Environmental Assessment Agency, The Hague, Netherlands
Nitrogen (N) and phosphorus (P) from anthropogenic sources are needed to produce food for the growing world population. As a result, these nutrients can be found in nearly every water body across the globe. Not only nutrient loading is important but also the molar ratio and its deviation from the “natural” Redfield ratio. Here we show that rivers, which have more than 50% anthropogenic sources and at the same time elevated N:P ratios (> 25) contributed 36% to the total global N export to coastal waters in 2015. The five Shared Socioeconomic Pathways (SSP) were used in combination with the Representative Concentration Pathways climate scenarios to project river nutrient loadings for 2050. Future nutrient export is projected to decline in high-income countries (with N:P ratios exceeding Redfield). In Brazil, India and China, however, a decline of N:P is only the case in a scenario oriented toward sustainable development (SSP1). The human-dominated river N and P export with elevated N:P ratios will increase in all SSPs, except in SSP1 where it stabilizes. Integrated strategies for both N and P considering all relevant trade-offs and societal sectors are urgently needed to reduce the nutrient pressure on surface waters.
Introduction
Human activities have markedly altered the earth's cycles of the plant nutrients nitrogen (N) and phosphorus (P) since pre-industrial times (Galloway et al., 1995; Smil, 2000). Increasing population and affluence have boosted energy, crop, livestock, and aquaculture production. This has led to dramatic growth of emissions from fossil fuel combustion, use of fertilizer N and P and animal manure. This has resulted in increasing nutrient loading of freshwaters through losses from agricultural land by leaching and runoff, discharge of wastewater in urbanized areas, and atmospheric N deposition. Loads and concentrations of N and P have been increasing in nearly every water body across the globe with multiple effects on ecosystem structure and functioning, such as changes in the community structure (e.g. from macrophytes to fast growing macro- and microalgae, from diatoms to flagellates), reduced water clarity, oxygen depletion and the formation of dead zones, benthic habitat loss, and the proliferation of harmful or toxic algal blooms (Duarte, 1995; Conley et al., 2009).
In the 1960s these nutrient-induced environmental problems were recognized in freshwater ecosystems, but it took another two decades before coastal eutrophication became a topic of societal, economic and scientific concern. Initial eutrophication research focussed on linking riverine nutrient loading with responses such as chlorophyll (as a proxy for algal biomass), primary production and occurrence of anoxic conditions (Cloern, 2001). In addition to nutrient loading per se, the coastal research community has become increasingly aware that the stoichiometric ratio of nutrients is essential (Billen et al., 1991). Disruption of the N:P ratio away from the Redfield molar ratio of 16:1 (Redfield, 1934) is one of the major causes of harmful algal bloom (HAB) proliferation (Glibert, 2019), even in a situation of declining nutrient loads. The Indicator for Coastal Eutrophication Potential (ICEP; Billen and Garnier, 2006; Garnier et al., 2010) was proposed as a basis for assessing the status of progress toward achieving Sustainable Development Goal (SDG) 14 (Life below water), and its target 14.1.1a (UNEP, 2021). The ICEP is based on loads and ratios of N, P and silicon (Si) exported by rivers to coastal waters. This indicator assumes that excess nitrogen or phosphorus relative to silica (ICEP > 0) will result in increased growth of potentially harmful algae.
Scenarios are essential tools for analyzing how nutrient loading and their ratio in freshwaters and coastal marine waters could develop in the future. Such scenarios would need to project developments in agriculture and sewage as the key drivers of future nutrient emissions (Seitzinger et al., 2010) and can be used to analyze future changes in N and P loading and export.
During the past four decades, models have been used to quantify past and future nutrient loading and their ratio in fresh and coastal waters at global and continental scales. The first estimate of N and P export to global coastal waters, made by Meybeck (1982), was based on classification of types of rivers and observations of some of these rivers. The next important step in global model development was made by Boyer et al. (2006) based on the Net Anthropogenic Nitrogen Input (NANI), regression approaches for N (e.g. Green et al., 2004), and more recently the Global-NEWS group (Seitzinger et al., 2005, 2010). The lumped Global-NEWS approach describes regression relations between input parameters averaged over river basins (e.g. the percentage agricultural land, use of manure and fertilizers, wastewater discharge, etc.) and the export of the river basin for different forms of N, P, carbon (C) and silicon (Si). These species-specific models were applied for individual years (1970, 2000) and were also used to project river export for future years (2030, 2050). The models where calibrated with observations available in the early 1990s (Meybeck and Ragu, 1995) which were assumed to represent the year 2000. A third step in the development of models for simulating nutrient export was the distributed and spatially explicit approach, where the focus is on better understanding of the different transport pathways in landscapes to surface water, biogeochemical processing and export of nutrients from different anthropogenic and natural sources. The latter approach provides spatially explicit information on the delivery and instream biogeochemistry and legacies which is not available in the lumped approaches. Examples of this distributed approach for the global scale are the models Integrated Model to Assess the Global Environment (IMAGE)-Global Nutrient Model (GNM) for N and P (Beusen et al., 2015, 2016, 2022) and DLEM for N (Tian et al., 2009), and at the European scale the models GREEN (Grizzetti et al., 2008, 2012), CAPRI-DNDC model (Britz and Leip, 2009; Leip et al., 2011) and INTEGRATOR (De Vries et al., 2009; Kros et al., 2011).
There are various approaches to make project future nutrient loading, in-stream biogeochemistry and export. First attempts to assess the influence of human activities on loading of total dissolved N and phosphate were presented by Meybeck (1982) and for nitrate by Caraco (1995). First projections of project future river export of DIN were made with a predecessor of Global-NEWS for a business-as-usual and a low N diet scenario (Kroeze et al., 2001). Bouwman et al. (2005) explored future total N export on the basis of FAO projections for agriculture (Bruinsma, 2003). More recently, projections of river export of different forms of N, P, C and Si from global watersheds with the Global-NEWS models (Seitzinger et al., 2010) were based on the Millenium Ecosystem Assessment (MA) scenarios; the MA scenarios form a wide spectrum of scenarios varying in population and economic growth and attitude toward the environment (Alcamo et al., 2006). The input for Global-NEWS was obtained from the IMAGE Integrated Assessment Model (version 2.4; Bouwman et al., 2006, 2009).
The goal of this paper is to analyze past and future changes in nutrient loading and export to global coastal waters, and the processes underlying these changes. We use the most recent IMAGE-GNM model (Beusen et al., 2022) which allows for consistent spatially explicit analysis of long-term changes in N and P loading, in-stream biogeochemical processing and export, and analysis of future scenarios. This enables to unravel the role of different sources, pathways (soils, groundwater, riparian zones, and the riverbed), processes and water body types. Regarding the future projections, we will follow the most recent community consensus: the five shared socioeconomic pathways (SSP; Riahi et al., 2016), scenarios used to study climate and other environmental changes.
Methods
Data and model used
Here we analyse both nutrient loading and nutrient ratios in rivers draining into global coastal waters during recent decades (1970–2015) and for the future up till 2050 with a time step of 5 years. The Global Nutrient Model (GNM; Beusen et al., 2015, 2016, 2022), which is part of the Integrated Model to Assess the Global Environment (IMAGE, version 3.2; Stehfest et al., 2014), was used to address the societal-ecological links relevant to nutrient sources and eutrophication and describe the complex biogeochemical filtering processes in terrestrial and aquatic ecosystems (Figure 1). In this study we use all results of the IMAGE-GNM model (historical and scenarios) which are available on https://dataportaal.pbl.nl/downloads/IMAGE/GNM/ and described in detail by Beusen et al. (2022). A short description of all processes and scenario assumptions is provided below.
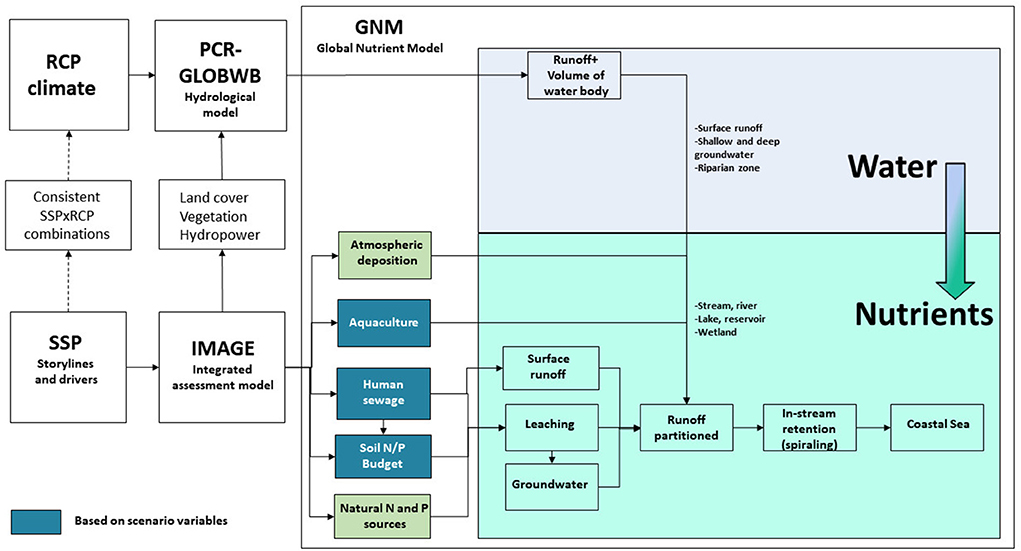
Figure 1. Scheme of the Integrated Model to Assess the Global Environment (IMAGE) – Global Nutrient Model (GNM), including the PCR-GLOBWB model to describe the hydrology. The human (sewage), aquaculture and soil N/P budget boxes indicate where scenario assumptions are used to express the N and P scenario storylines in quantitative terms.
For simulating nutrient delivery to surface waters, in-stream retention and river export to coastal waters by natural and anthropogenic sources, IMAGE–GNM with a spatial resolution of 0.5 × 0.5° (Beusen et al., 2015) was employed. IMAGE-GNM has been used to cover the full twentieth century (Beusen et al., 2016) and for the five SSP scenarios (Beusen et al., 2022). IMAGE-GNM describes nutrient delivery to surface water by diffuse and point sources via diverse pathways (Figure 1). This is achieved through a coupling with the global hydrological model PCR-GLOBWB (Van Beek et al., 2011; Sutanudjaja et al., 2018) which computes the water balance, runoff, discharge and water temperature for each year (Figure 1). For every grid cell, the water and nutrient fluxes through streams and rivers, lakes, wetlands and reservoirs (Figure 1) are based on the routing scheme of PCR-GLOBWB.
Diffuse sources include N and P delivery from agricultural and natural ecosystems, with various transport pathways (Figure 1; i.e. surface runoff and erosion, leaching through and outflow of shallow and deep groundwater, shallow groundwater transport through riparian zones to surface water; input of litter material from vegetation in flooded areas; aquaculture; weathering; atmospheric deposition).
Point sources are the discharges of N and P of rural and urban wastewater from households and industries with a sewage connection (Figure 1). Emissions from households are calculated from the protein intake and the use of P-containing detergents. Sewage water is discharged either directly or after wastewater treatment. The efficiency of nutrient removal during treatment depends on the mix of types of installations which varies from country to country. Four treatment types are distinguished, i.e., primary, secondary, tertiary and quaternary treatment, each having a typical removal efficiency (Van Puijenbroek et al., 2018).
IMAGE-GNM calculates soil nutrient budgets as the difference between inputs and outputs for estimating diffuse nutrient flows from agriculture and natural ecosystems. In the soil budgets, N and P inputs include (where relevant) fertilizer, animal manure, atmospheric deposition and biological N2 fixation. Output is the harvesting of agricultural crops or grass and forages or grass consumption by grazing animals. Natural ecosystems are assumed to be mature, which implies that annual N and P uptake by the vegetation equal the nutrients in litterfall. Since there is no net uptake, inputs from biological N2 fixation and atmospheric deposition are removed through runoff and erosion, ammonia volatilization, leaching and denitrification.
Soil nutrient budgets are calculated with 0.5 by 0.5 degree resolution on the basis of various data sources (Bouwman et al., 2017) and distributed spatially using the land cover maps that are simulated for each year. IMAGE simulates land cover using three coupled submodels (Stehfest et al., 2014). The agricultural economy model MAGNET (Woltjer and Kuiper, 2014) calculates demand, production and trade of food and crop production intensification on the basis of trends of Gross Domestic Product and population. The second model to simulate land use dynamics is IMAGE-Land Management (Doelman et al., 2017), which considers 16 food crop types including feed crops, 5 animal categories, bio-energy, forestry and built-up area. The third model is the dynamic global vegetation model LPJ-ml (Bondeau et al., 2007) which simulates potential yields of crops and grass, and the C and hydrological cycles for natural ecosystems, forestry and agriculture (Bondeau et al., 2007). IMAGE-GNM uses aggregated data from IMAGE-Land Management for upland crops, N-fixing legumes, wetland rice and grassland with specific fertilizer applications rates. Livestock, manure production and grazing land are distributed over mixed/landless and pastoral livestock production systems, and stored manure is distributed over crops and grass (see Bouwman et al., 2017). In the livestock system, inputs include feed crops, grass, crop residues and scavenging, and meat, milk and animal manure are outputs.
Two soil erosion loss routes are distinguished, i.e., losses from recent nutrient applications in the form of fertilizer, manure or organic matter, and loss of soil material with a “memory” effect related to historical changes of the topsoil composition resulting from long-term soil budget surplus or deficit. Using the soil P inputs, and after accounting for P erosion loss, IMAGE-GNM calculates available, labile and recalcitrant P pools in soils and P uptake using the dynamic soil P model DPPS (Mogollón et al., 2018; Langhans et al., 2022).
Gaseous N losses occur through ammonia volatilization and denitrification. Ammonia volatilization is computed for each grid cell with a regression model using soil, climate, crop and fertilizer properties (Bouwman et al., 2002). The soil N budget surplus excluding ammonia volatilization can be lost through denitrification and leaching. The balance between leaching and denitrification is calculated on the basis of soil moisture, temperature, and a number of physical and chemical soil properties (Van Drecht et al., 2003; Keuskamp et al., 2012; Beusen et al., 2015). Leached N percolates to shallow and subsequently to deep groundwater. Overall groundwater denitrification loss depends on both travel time and half-life. Groundwater travel time is calculated on basis of porosity of the parent material. Denitrification rates are based on the half-life of nitrate, which is a function of the lithology (Van Drecht et al., 2003). Deep groundwater discharges to large water bodies. Shallow groundwater can flow directly to streams or indirectly via the riparian zone. Groundwater travel times of years to several decades imply a temporary storage, which varies due to the geohydrological and climate conditions (Van Drecht et al., 2003). In addition, the history of fertilizer use and surplus applications are reflected in the groundwater outflow in a certain year (Bouwman et al., 2013a) and forms a legacy source from past nutrient management.
The aboveground litter from vegetation in flooded areas such as floodplains is obtained from LPJ-ml. N and P inputs are based on the proportion aboveground litter (Vogt et al., 1986) together with assumed N and P contents (Vitousek and Matson, 1984; Vitousek et al., 1988). P from weathering is based on a model that relates P release to lithology, runoff and temperature (Hartmann et al., 2013).
Nutrient release by freshwater aquaculture is calculated with the nutrient budget model for the various species groups of finfish (Bouwman et al., 2013b) and shellfish (Bouwman et al., 2011) using country-scale data from FAO (2020). The nutrient release is distributed spatially within countries using different scalars including population density, presence of surface water bodies, and mean annual air temperature (Beusen et al., 2015). The uptake of nutrients from filtered plankton by bivalve shellfish is taken into account in the calculation of the nutrient release of aquaculture. Atmospheric N deposition is scaled to the patterns for the year 2000 (Dentener et al., 2006) using grid-based emissions of ammonia from the IMAGE model.
In-stream retention in streams, rivers, lakes and reservoirs is calculated with the nutrient spiraling ecological concept (Newbold et al., 1981; Wollheim et al., 2008). IMAGE-GNM also includes groundwater N transport through submarine fresh groundwater discharge to the coastal waters (Beusen et al., 2013). At every location within a river basin, nutrient sources can be attributed by recording the pathways of delivery and in-stream retention accounting for delayed inputs from the groundwater system.
The validation of the IMAGE-GNM model was performed for the time period 1970–2010 for the Meuse and Rhine (Western Europe), Yangtze (China) and Mississipi (USA) as described in Beusen et al. (2022). The validation showed that the simulated long-term trends of these rivers (N and P concentration and discharge) were close to observations, which is a requirement for a model used for making future projections for decades ahead. Lacking uncertainty ranges and distribution functions for input parameters, an extensive sensitivity analyses was performed with IMAGE-GNM to rank input parameters for the five SSP scenarios on the basis of their importantce for the model outputs N and P delivery, retention and export.
Scenarios
Future changes in global nutrient loading may not develop as a continuation of past trends due to unpredictability of human behavior and the complexity of interactions of the economic and earth systems. Here we use the implementation of the five Shared Socio-economic Pathways (SSP; Riahi et al., 2016) with IMAGE version 3.2 (Van Vuuren et al., 2021). The SSPs are the set of community scenarios used to explore a spectrum of global environmental futures considering different developments in society and economy, the energy and agricultural systems, climate change and hydrology (Riahi et al., 2016). The sustainability scenario SSP1 strives for achieving the SDGs by reducing intensity of resources use and the dependency on fossil fuels. SSP1 contrasts the fossil fuel-based SSP5, which involves traditional development with a focus on economic growth relying strongly on fossil fuels. SSP2 is a middle-of-the-road scenario where current trends will continue in coming decades. Fragmentation is the keyword of SSP3 due to wide variation in socio-economic development between regions. Finally, inequality is strong in SSP4 with societies characterized by small elites and large, poor and vulnerable groups.
For each SSP, assumptions and projections were made for domestic production and trade of energy, food, feed and biofuel crops, meat and milk, land use and cover, greenhouse gas emissions and climate change as described earlier by Van Vuuren et al. (2016) and updated for the base year 2015 (Van Vuuren et al., 2021).
PCR-GLOBWB was run with different climate change scenarios for simulating future hydrology. These climate scenarios are the Representative Concentration Pathways (RCP) implemented with the HadCM3 Global Circulation Model (MetOffice, 2020). The RCP data were bias-corrected using historical weather data covering 1960–1999 (Hempel et al., 2013). The RCPs differ in the global average radiative forcing in the year 2100, respectively 4.5, 6.0, and 8.5 W m−2. For this paper, the combinations SSP1-RCP4.5, SSP2-SSP4 with RCP6.0, and SSP5-RCP8.5 were implemented, as they correspond to future radiative forcing simulated for the different SSPs by the IMAGE model.
In the scenario-mode, the dynamic DPPS model (Mogollón et al., 2018) takes projected crop P yield for year x and the soil P pools and soil P availability for plant uptake in the year x-1 to calculate the total P input required. Projected P input depends on the proportion of the labile soil P pool that is directly available for uptake by plant roots, which can change in future along with technology and management improvement depending on the SSP scenario. Projections for total N requirement are based on future crop production and the N use efficiency (NUE). Fertilizer N and P use are calculated as the difference between required total N or P input and N or P from non-fertilizer sources which are generated by IMAGE (animal manure N or P, biological N2 fixation and atmospheric N or P deposition). Manure availability depends on the projected livestock numbers, and the proportion of manure that is not recycled. In SSP1, all manure is recycled. In other scenarios the proportion of manure ending outside agriculture is constant after 2015.
In SSP1 and SSP5, the direct availability of P in the labile soil P pool increases at the same rate as the increase during 1990–2005, in SSP2 by half that increase, and in SSP3 by one quarter. In SSP4, they follow the income level (SSP1 values for high-income regions, SSP2 values for middle-income regions and SSP3 values for low-income regions). NUE values in SSP2 and SSP5 continue the trend of the past 3–4 decades, in SSP1 they bridge half of the gap between the current value and the target values proposed by Zhang et al. (2015). These NUE values are ambitious targets defined to achieve the planetary boundary for N while meeting the future food demand in 2050. NUEs were assumed not to change in SSP3, while in SSP4 they follow the income level (similar to the direct soil P availability).
Scenarios for wastewater N and P discharge to surface water are based on income relationships of human emissions represented by protein consumption, the proportion of the population with a connection to sewerage systems, and the presence of wastewater treatment plants with corresponding level of nutrient removal (Van Puijenbroek et al., 2018).
Freshwater aquaculture finfish and shellfish production changes along with the population, assuming a constant global mean per capita production. The efficiency of production depends on the scenario variables feed conversion ratio (FCR) and the fraction of compound feed in the feed ration for the different finfish and shellfish groups.
Threshold for N:P ratio
Many phytoplankton causing harmful algal blooms (HABs) have physiological adaptive strategies that favor them under conditions of elevated N:P conditions. A threshold value of 25 for the total N: total P molar ratio (here denoted as N:P) was suggested by Wang et al. (2021) for Chinese coastal waters in the Yellow Sea, East China Sea and South China Sea. In these ecosystems, HABs started to proliferate when the N:P ratio in river export rose above this threshold in the 1980s. Here we assume that this threshold for N:P is also valid in coastal waters outside China.
We recognize that in river fluxes to coastal waters, the molar ratio of dissolved inorganic nitrogen (DIN) and dissolved inorganic phosphorus (DIP) often exceeds than that of N:P, since a large fraction of total P consists of inactive P bound to soil particles, and the DIP buffer mechanism by the sorption/desorption of DIP by suspended particles. It is well known that silica abundance in river export also plays an important role in HAB risk. Silica in rivers mainly orginates from rock weathering. Some tropical rivers show high N:P ratios from natural sources mainly, and at the same time high Si loading. Lacking a consistent up-to-date model for silica in rivers, we use a second criterion for distinguishing HAB risk: >50% anthropogenic share in the total N or P export to coastal waters.
Results
Historical trends
Global N and P delivery to surface waters has increased from 44 to 71 Tg N yr−1 for N and 7.1 to 9.7 Tg P yr−1 for P during the period 1970–2015, which is the net result of rapid increases in Brazil, India and China (BIC), a decrease in industrialized countries in North America, Europe, the Russian Federation, Japan and Oceania (INDUS) and an increase in the rest of the world (ROW; Figure 2). In this 45 year period 1970–2015, the N and P delivery to surface waters was constant at 17 Tg N yr−1 for N and declining from 2.5 to 2.2 Tg P yr−1 for P in the INDUS countries, while the BIC countries show an increase of 10 to 25 Tg N yr−1 and 1.3 to 2.6 Tg P yr−1, while in the ROW countries there has been an increase from 18 to 30 Tg N yr−1 and 3.4 to 4.9 Tg P yr−1. The increasing nutrient delivery is driven by the increasing contribution from anthropogenic sources to inland waters, which increased globally from 60 to 74% of total N delivery between 1970 and 2015 (Figure 3A), with a strong increase in BIC and ROW countries (Figures 3C,D). The INDUS countries show a declining anthropogenic fraction between 1970 and 2015 (Figure 3B). Hence, while the relative contribution from agriculture, human and aquaculture has been increasing in time and the contribution of natural sources has been declining.
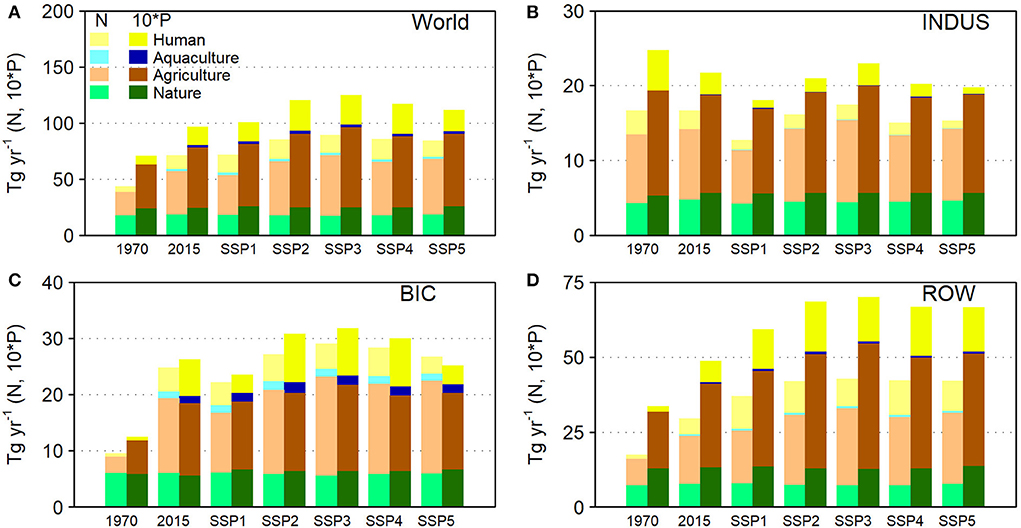
Figure 2. Annual total N and total P delivery for (A) the world; (B) industrialized countries in North America, Europe including the Russian Federation, Japan and Oceania (INDUS); (C) Brazil, India and China (BIC) and (D) the rest of the world (ROW) for 1970, 2015, and 2050 for the five SSPs.
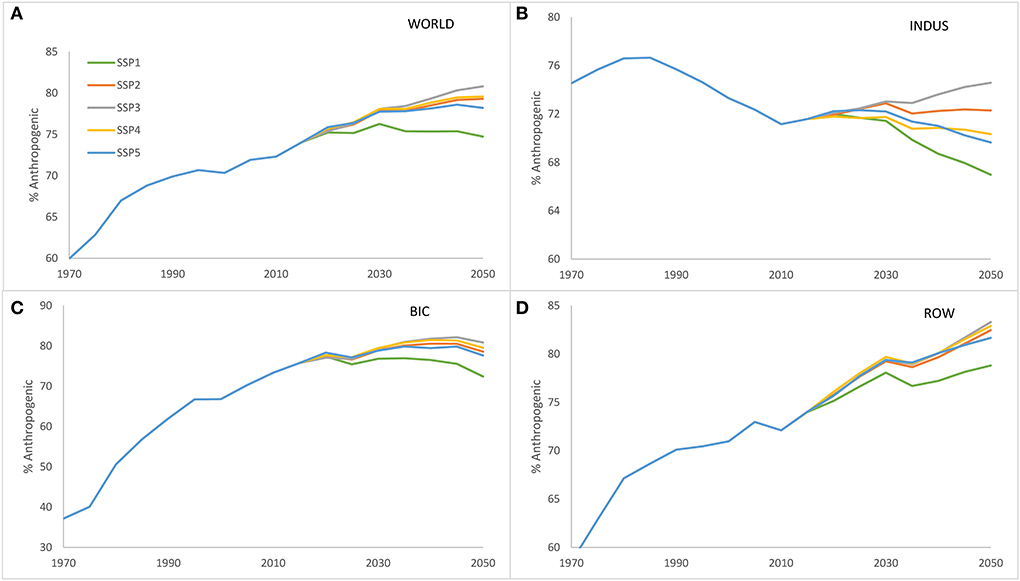
Figure 3. Percentage of N delivery which is from anthropogenic sources for (A) the world, (B) INDUS, (C) BIC, and (D) ROW for the five SSPs.
Figure 4 shows the patterns of the anthropogenic share in the total N export to global coastal waters for the year 2015. The river export is nowadays dominated by anthropogenic sources in large parts of the world. and the N export by Arctic rivers and a number of large tropical rivers like Amazon and Congo are still dominated by natural sources. The export by both rivers has a high N:P ratio, which is caused by the large N inputs from biological N2 fixation by tropical leguminous trees, and low P weathering fluxes from the prevailing strongly and deeply weathered ferralitic soils. Figure 5 presents the molar ratio of total N to total P (here denoted as N:P) of the river export at the mouth of the river basins for 2015. In 2015 Europe, South Asia and large part of Northern America, and the Amazon and Congo rivers have N:P ratios > 25.
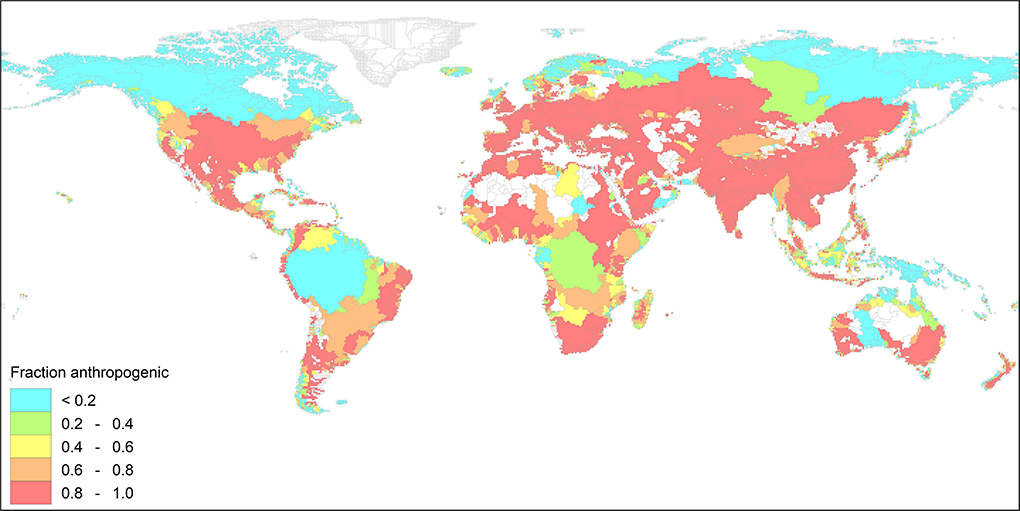
Figure 4. Fraction anthropogenic sources in the river export to global coastal waters for the year 2015. The fraction anthropogenic is calculated as the maximum of the anthropogenic N or P sources.
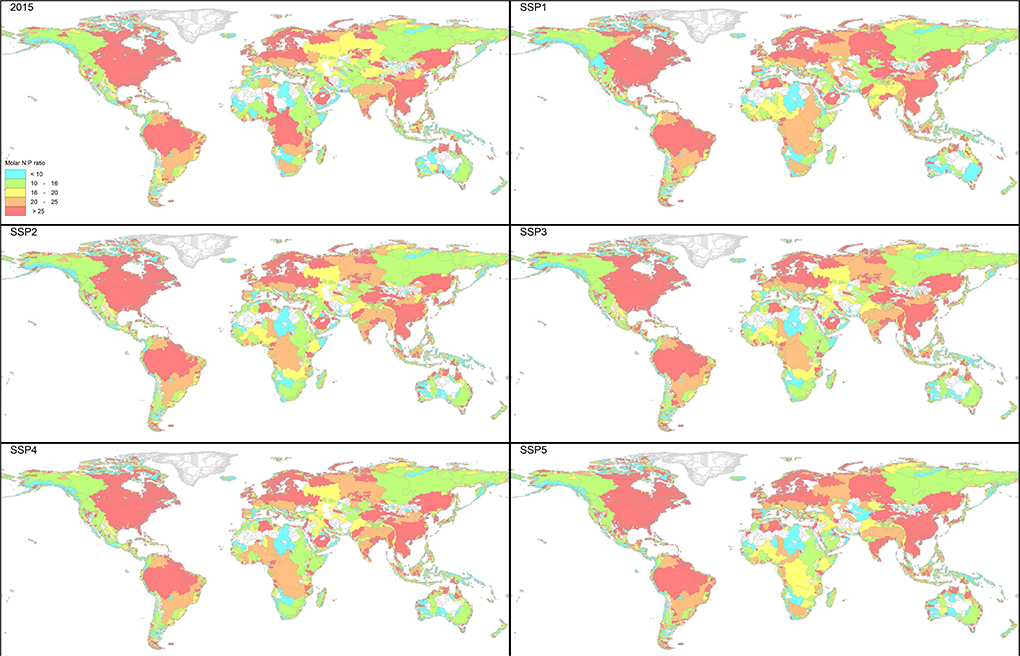
Figure 5. Molar ratio of total N: total P (here denoted as N:P) in the river export to global coastal waters for the year 2015 and 2050 for the five SSPs.
These differences in N and P ratio are reflected by the proportions in which nutrients are transported and exported to coastal waters. Table 1 shows that the N:P of the total export to the coastal waters in all world regions has been increasing in the period 1970–2015 by 24% from 17 to 21. The N:P ratios for the year 1970 and 2000 (17 and 20, respectively) exceed estimates by the Global NEWS model, which predicts a constant N:P ratio of 11 between 1970 and 2000, possibly due to the inconsistency of models for different N and P species and the absence of a dynamic model for in-stream retention and legacies. The global dissolved silica export for the year 2000, shows that the Amazon and Congo rivers export large amounts of Si (Figure 6), a product of the rapid desilication process in soils (Driessen and Dudal, 1990). Therefore, the water discharged by these rivers dominated by natural nutrient sources with low N:Si and P:Si ratios (due to high N:P, see Figure 5) is not prone to HAB development.
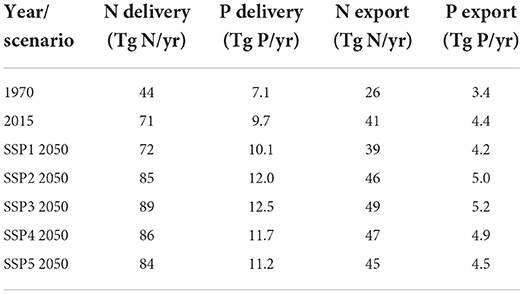
Table 1. Total global delivery and global export for N and P of the rivers for the years 1970 and 2015 and for 2050 for different SSP scenarios.
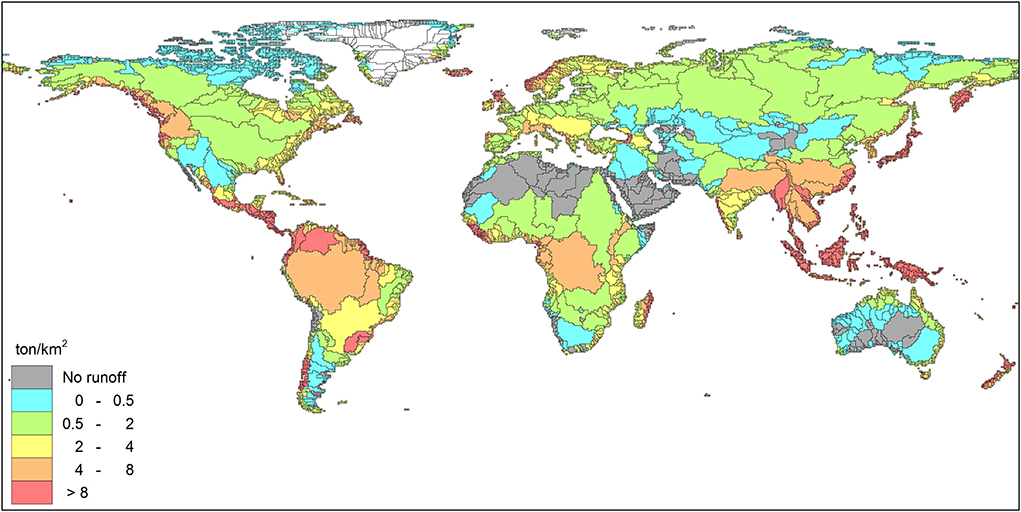
Figure 6. SiO2 yield in ton per km2 for the year 2000. Modified from Beusen et al. (2009).
The global N:P ratio has changed rapidly during the period 1970–2015 due to changes in the anthropogenic loading, which all show increasing N:P ratios (Table 2). The N:P ratio in water delivered from agriculture has been increasing from 12 to 16, that from the human sources from 15 to 16 and that from aquaculture from 17 to 20. The natural sources have an almost constant N:P ratio of 16 (15–17).
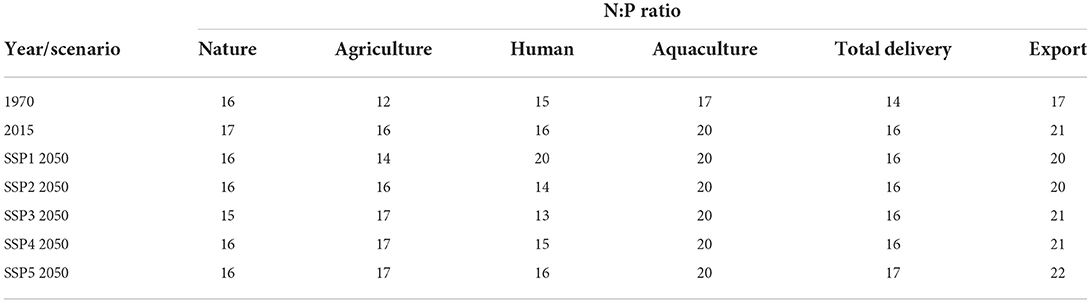
Table 2. Molar N:P ratio for the different sources of the global delivery, total global delivery and global export of the rivers for the years 1970 and 2015 and for 2050 for different SSP scenarios.
Parallel to the increasing anthropogenic share and the change in the mix of anthropogenic sources, there are also changes in the total quantities of exported N and P if we consider export fluxes with (i) dominant (> 50%) anthropogenic sources of N, P or both, and (ii) N:P ratio > 25. This selection reveals that global river N export to coastal waters increased by more than a factor of 3 between 1970 and 2015 to 16 Tg yr−1 (Figure 7A), which represents 36% of the global river N export of 41 Tg N yr−1. Here the same patterns are found for the three regions. The anthropogenic dominated export by rivers with a high N:P ratio has been declining in the INDUS countries, and increasing for ROW and BIC countries during the period 1970–2015.
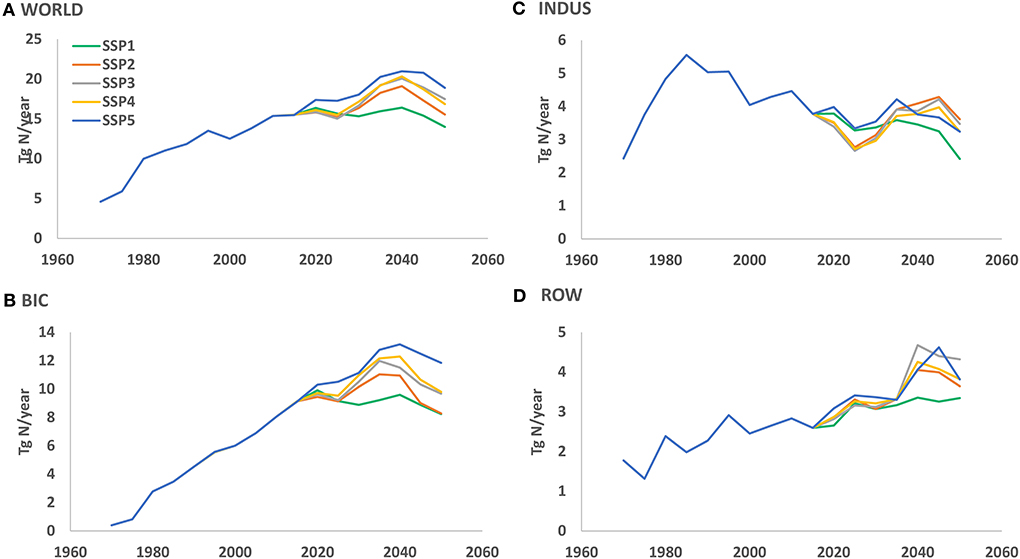
Figure 7. River N export for rivers with dominant (> 50%) anthropogenic N sources and N:P ratio > 25 for (A) the world, (B) INDUS, (C) BIC, and (D) ROW.
Future trends
The SSPs used here have all a different population assumptions. Figure 8 shows the global total population and the global N and P delivery per capita. The figure shows that the delivery per capita decreases which means that there is less N and P loss to the rivers per capita. However the population is increasing, which results in a increasing delivery of N and P to the rivers.
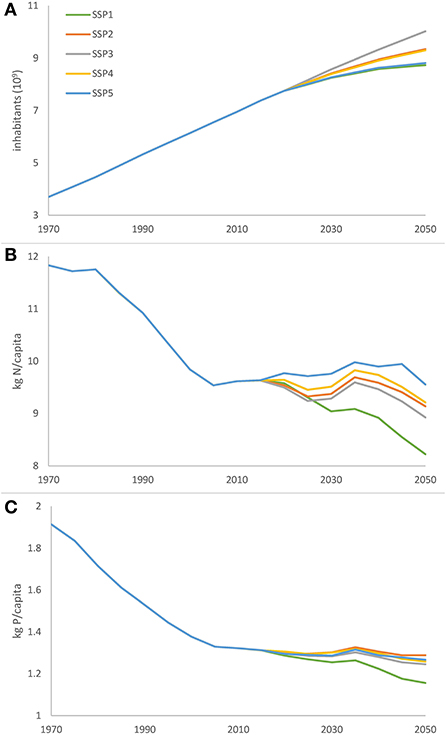
Figure 8. (A) Global total population and (B) the annual global total N delivery per capita and (C) annual global total P delivery per capita for the five SSPs for the time period 1970–2050.
The SSP-based projections show a global increase of nutrient delivery in all scenarios, which is the result of differentiated regional developments (Figure 2, Table 1). In SSP3 there is the highest increase in all world regions. SSP1 portrays a reduction of N and P delivery in BIC and INDUS countries, but in ROW there is an increase. SSP2 portrays increasing delivery in ROW and BIC, but a decrease in INDUS countries, while SSP4 shows an increase in all parts of the world. SSP5 and SSP1 show similar patterns, i.e., a reduction of N and P delivery in BIC and INDUS countries, and an increase in the ROW countries. The anthropogenic fraction is only decreasing for SSP1 in the BIC countries and for SSP1, SSP4, and SSP5 for the INDUS countries. The global anthropogenic fraction will reach values between 75 and 80% in 2050 (Figure 3).
In the scenarios, the agricultural delivery has an N:P ratio between 14 (SSP1) and 17 (SSP3) (Table 2). The N:P ratio of the human sources depends on the amount and type of treatment and ranges between 20 (SSP1) and 13 (SSP3). Especially the tertiary and quaternary treatment systems remove P more efficiently than N, which results in higher N:P ratio. The overall N:P delivery for all sources range between 16 (SSP2) and 17 (SSP5). Figure 5 presents the spatial pattern of the molar N:P ratio of the river export at the mouth of the river basins for the five SSPs for the year 2050.
Discussion
Instream biogeochemistry
The N:P ratio in the water delivered to inland waters depends on the source of the delivery (Table 2). Apart from the changes in the sources, the various biogeochemical filter systems cause further changes in the N:P ratio. The N:P ratio of water delivered to surface water bodies increases during transport because of higher retention of P vs. N in aquatic systems. Between 1970 and 2015 the global N:P ratio at the mouth of the rivers increased from 17 to 21 and future N:P ratios range between 20 (SSP1 and SSP2) and 22 (SSP5) in 2050. In particular, N:P ratios in lakes and reservoirs are much higher than those in rivers due to more efficient P removal (Maranger et al., 2018). Construction of reservoirs thus increases the N:P ratio of export. A further cause of increasing N:P ratios observed in a series of large lakes where nutrient concentrations have been reduced as a result of mitigation policies, is due to complex interactions between N and P; reduction of particularly P leads to less primary biological production, less sedimentation of organic matter, less decomposition and oxygen depletion, therefore less N removal by denitrification, and increasing N:P (Finlay et al., 2013).
The impact of fire is not taken into account in this study. Forest fire for land clearing has an impact on the amount and pathways of nutrients in soil, volatilization, mineralization, erosion, runoff and leaching (Neary et al., 1999), but it is unclear (depending on the scale of the fire area in relation to the total basin area and the frequencies of the fires) whether this results in long-term changes of nutrient exports and nutrient ratios.
Eventually, the N:P ratio of nutrients exported to coastal waters exceeds that of the delivery to surface waters (Table 2). While policies aimed at reducing nutrient pollution have been successful in reducing or stabilizing nutrient concentrations in rivers in Europe and the USA, this has also had repercussions for nutrient ratios. Sewage treatment now efficiently removes nutrients from wastewater, particularly in Europe (Van Puijenbroek et al., 2018) and P is often removed more efficiently than N. Despite the considerable improvement of the nitrogen use efficiency in agriculture, a large input of N fertilizers is required to support crop and forage production (Bouwman et al., 2017).
However, due to accumulation of P fertilizer surpluses in agricultural soils in the 1960–1980s, P availability for crops has increased and P inputs can be reduced without affecting crop productivity (Sattari et al., 2012). Consequently, P fertilizer use in INDUS countries has declined to very small amounts as reflected by small P surpluses and high N:P ratios in the overall surface budgets. Rivers in INDUS countries currently reflect the effects of excessive nutrient mobilization in the 1960–1980s, i.e., the legacy of historical nutrient use. With declining nutrient inputs, soils may be releasing nutrients by organic matter decomposition, and aquifers (particularly N) and sediments (particularly P) in lakes, reservoirs and rivers continue to deliver nutrients. The N concentration in the Mississippi has not decreased in recent decades, despite policies to reduce nutrient loading which is attributed to groundwater N legacy (Sprague et al., 2011). The simulated N:P ratio changes agree with observed increases in the N:P ratios in many European (Romero et al., 2013) and Chinese (Dai et al., 2011) rivers and elevated levels in the Mississippi (Alexander et al., 2008).
River export
Despite the wide spectrum covered by the five SSPs, with large differences in all aspects of human society ranging from population growth, human diets, energy consumption patterns, nutrient use efficiencies in food production, sewage connection and wastewater treatment, there is little difference in the magnitude and stoichiometry of global river export in human-dominated rivers. This means that even in the SSP1 scenario with a focus on a more sustainable development, water quality will not improve in large parts of the world like ROW and BIC countries but for the INDUS countries it will improve.
The anthropogenic part of all N and P sources has been rapidly increasing over the past and will exceed 65% in the future for all scenarios. The global future projections show that for the human-dominated rivers, the global nutrient export to coastal waters with N:P ratios > 25 will not decline in the coming decades in any scenario except for SSP1 (Figure 7). For the INDUS countries the anthropogenic high N:P export will decline over the time period 2015–2050 for all scenarios. The opposite is true for the ROW countries, where it will increase in all scenarios. For the BIC countries there is a decline in SSP1 and SSP2 but an increase according to the other scenarios.
Climate does influence the whole system, from leaching, groundwater transport, travel times and in-stream retention. The effect of climate change in the first part of the twenty first century is small, due to the small differences between the RCP climate scenarios, and after 2050 the climate scenarios start to diverge. The differences between agricultural practices and point sources are the main drivers of differences between the scenarios for delivery and export.
Concluding remarks
All SSPs portray a stabilization or a decline of freshwater quality. The human-dominated river N and P export with elevated N:P ratios will increase in all SSPs. Only in SSP1 for industrialized countries will there be progress toward reducing nutrient loading of coastal waters. In the ROW countries the river export of N and P will increase for all SSP scenarios. Van Meijl et al. (2020) have shown that achieving food security remains a challenge but is possible, except for South Asia and in Sub-Saharan Africa in SSP3 and SSP4. Langhans et al. (2022) showed that doubling smallholder production is possible, but the investments needed to achieve this are in general lacking in Sub-Saharan Africa and South Asia. Hence, the most vulnerable inhabitants that face multiple problems due to lack of food security and poor water quality, are especially in the low-income countries of the world. Hence, policies oriented toward improvement of food security should also consider nutrient use in agriculture and the management of human wastes.
There will be an important future role of legacies of past nutrient management. River export with high N:P ratios causing HAB risk will not decline even in environmently friendly and sustainability-oriented futures in industrialized countries due to legacies from nutrients that have accumulated in landscapes in past decades. Since nutrients are currently also rapidly accumulating in many river basins in Brazil, India, China and in many medium and low income countries, similar legacies will emerge when nutrient mitigation strategies will become effective.
Future HAB risk assessment will be improved if the impact of Si is included. Si fluxes have been rapidly changing in the past due to increasing human interference in the hydrology. Building new reservoirs for hydropower and irrigation is needed to satisfy our increasing food and energy demand. However, dams also diminish the Si export into the coastal area and may impact HAB risk. For example, the SDG target 14.1.1 uses the Indicator for Coastal Eutrophication Potential (ICEP; Billen and Garnier, 2006; Garnier et al., 2010), based on the Redfield ratios of N or P to Si (Redfield et al., 1963; Brzezinski, 1985). With increasing N or P export and decreasing Si export, the ICEP will increase, indicating an increasing HAB risk.
Our results indicate that the interplay between N and P is important in order to find well-balanced and integrated management strategies for water quality considering all societal sectors and trade-offs, to avoid high N:P ratios. Apart from nutrient loading (Table 1) and ratios, the occurrence of HABs is determined by factors such as temperature, available light and hydrodynamics. It is uncertain how nutrients and these additional factors contribute to the HAB proliferation, but the trend of the N:P ratios and the total load of N and P in surface water (Table 1) indicate that the HAB risk will probably not diminish.
Without additional policies, HAB risk will not be reduced or will even extend to more rivers in large parts of the world. There are different management strategies to improve water quality. The first option is to reduce the use of N or P in agriculture. This means that for N and P inputs (fertilizer and manure) are applied at the right rate, at the right moment and at the right place (Zhang et al., 2015). The second option is to avoid the transfer of N or P to the surface water. Reducing erosion by soil conservation is important (for example no crop fields on land with steep slopes, erosion control by terracing and other conservation measures). Treating waste water from households and industries also reduces the effluent of N and P to surface water. However, especially for the agricultural nutrient losses, it is probably difficult to manage N:P ratios at the scale of landscapes with a heterogeneous production system and multiple pathways of nutrient transport from the field to surface water.
Data availability statement
Publicly available datasets were analyzed in this study. This data can be found at: https://dataportaal.pbl.nl/downloads/IMAGE/GNM/.
Author contributions
AHWB and AFB contributed equally to model development, data analysis, and writing the manuscript. All authors contributed to the article and approved the submitted version.
Funding
PBL received funding for this work from the International Nitrogen Management System Project (INMS, http://www.inms.international/) funded by GEF and implemented by UNEP. AHWB and AFB received support from PBL Netherlands Environmental Assessment Agency through in-kind contributions to the New Delta 2014 ALW project nos. 869.15.015 and 869.15.014.
Conflict of interest
The authors declare that the research was conducted in the absence of any commercial or financial relationships that could be construed as a potential conflict of interest.
Publisher's note
All claims expressed in this article are solely those of the authors and do not necessarily represent those of their affiliated organizations, or those of the publisher, the editors and the reviewers. Any product that may be evaluated in this article, or claim that may be made by its manufacturer, is not guaranteed or endorsed by the publisher.
References
Alcamo, J., Van Vuuren, D., and Cramer, W. (2006). “Changes in ecosystem services and their drivers across the scenarios,” in Ecosystems and Human Well-Being: Scenarios, eds. S.R. Carpenter, P.L. Pingali, E.M. Bennett and M.B. Zurek. (Washington, D.C.: Island Press) 279–354.
Alexander, R. B., Smith, R. A., Schwarz, G. E., Boyer, E. W., Nolan, J. V., Brakebill, J. W., et al. (2008). Differences in phosphorus and nitrogen delivery to the Gulf of Mexico from the Mississippi River Basin. Environ. Sci. Technol. 42, 822–830. doi: 10.1021/es0716103
Beusen, A. H. W., Bouwman, A. F., Dürr, H. H., Dekkers, A. L. M., and Hartmann, J. (2009). Global patterns of dissolved silica export to the coastal zone: Results from a spatially explicit global model. Global Biogeochem. Cycles 23, GB0A. doi: 10.1029/2008GB003281
Beusen, A. H. W., Bouwman, A. F., Van Beek, L. P. H., Mogollón, J. M., and Middelburg, J. J. (2016). Global riverine N and P transport to ocean increased during the 20th century despite increased retention along the aquatic continuum. Biogeosciences 13, 2441–2451. doi: 10.5194/bg-13-2441-2016
Beusen, A. H. W., Doelman, J. C., Van Beek, L. P. H., Van Puijenbroek, P. J. T. M., Mogollón, J. M., Van Grinsven, H. J. M., et al. (2022). Exploring river nitrogen and phosphorus loading and export to global coastal waters in the Shared Socio-economic pathways. Global Environ. Change 72, 102426. doi: 10.1016/j.gloenvcha.2021.102426
Beusen, A. H. W., Slomp, C. P., and Bouwman, A. F. (2013). Global land–ocean linkage: direct inputs of nitrogen to coastal waters via submarine groundwater discharge. Environ. Res. Lett. 8, 034035. doi: 10.1088/1748-9326/8/3/034035
Beusen, A. H. W., Van Beek, L. P. H., Bouwman, A. F., Mogollón, J. M., and Middelburg, J. J. (2015). Coupling global models for hydrology and nutrient loading to simulate nitrogen and phosphorus retention in surface water. Description of IMAGE-GNM and analysis of performance. Geosci. Model Dev. 8, 4045–4067. doi: 10.5194/gmd-8-4045-2015
Billen, G., and Garnier, J. (2006). (2007). River basin nutrient delivery to the coastal sea: assessing its potential to sustain new production of non-siliceous algae. Marine Chem. 106, 148–160, 1017. doi: 10.1016/j.marchem.2006.12.017
Billen, G., Lancelot, C., and Meybeck, M. (1991). “N, P, and Si retention along the aquatic continuum from land to ocean,” in Ocean Margin Processes in Global Change, eds. R.F.C. Mantoura, J.M. Martin and R. Wollast (New York, NY: John Wiley and Sons), 19–44.
Bondeau, A., Smith, P. C., Zaehle, S. N., Schaphoff, S., and Lucht, W. (2007). Modelling the role of agriculture for the 20th century global terrestrial carbon balance. Global Change Biol. 13, 679–706. doi: 10.1111/j.1365-2486.2006.01305.x
Bouwman, A. F., Beusen, A. H. W., and Billen, G. (2009). Human alteration of the global nitrogen and phosphorus soil balances for the period 1970–2050. Global Biogeochem. Cycles 23, GB0A. doi: 10.1029/2009GB003576
Bouwman, A. F., Beusen, A. H. W., Griffioen, J., Van Groenigen, J. W., Hefting, M. M., Oenema, O., et al. (2013a). Global trends and uncertainties in terrestrial denitrification and N2O emissions. Philos. Trans. Royal Soc. B Biol. Sci. 368:1621. doi: 10.1098/rstb.2013.0112
Bouwman, A. F., Beusen, A. H. W., Lassaletta, L., Van Apeldoorn, D. F., Van Grinsven, H. J. M., Zhang, J., et al. (2017). Lessons from temporal and spatial patterns in global use of N and P fertilizer on cropland. Sci. Rep. 7, 40366. doi: 10.1038/srep40366
Bouwman, A. F., Beusen, A. H. W., Overbeek, C. C., Bureau, D. P., Pawlowski, M., Glibert, P. M., et al. (2013b). Hindcasts and future projections of global inland and coastal nitrogen and phosphorus loads due to finfish aquaculture. Rev. Fisheries Sci. 21, 112–156. doi: 10.1080/10641262.2013.790340
Bouwman, A. F., Boumans, L. J. M., and Batjes, N. H. (2002). Estimation of global NH3 volatilization loss from synthetic fertilizers and animal manure applied to arable lands and grasslands. Global Biogeochem. Cycles 16, 1024. doi: 10.1029/2000GB001389
Bouwman, A. F., Kram, T., and Klein Goldewijk, K. (2006). Integrated Modelling of Global Environmental Change. An Overview of Image 2, 4. Bilthoven: Netherlands Environmental Assessment Agency.
Bouwman, A. F., Pawłowski, M., Liu, C., Beusen, A. H. W., Shumway, S. E., Glibert, P. M., et al. (2011). Global hindcasts and future projections of coastal nitrogen and phosphorus loads due to shellfish and seaweed aquaculture. Rev. Fisheries Sci. 19, 331–357. doi: 10.1080/10641262.2011.603849
Bouwman, A. F., Van Drecht, G., Knoop, J. M., Beusen, A. H. W., and Meinardi, C. R. (2005). Exploring changes in river nitrogen export the the world's oceans. Global Biogeochem. Cycles 19, GB1002. doi: 10.1029/2004GB002314
Boyer, E. W., Howarth, R. W., Galloway, J. N., Dentener, F. J., Green, P. A., Vörösmarty, C. J., et al. (2006). Riverine nitrogen export from the continents to the coasts. Global Biogeochem. Cycles 20:GB1S91. doi: 10.1029/2005GB002537
Britz, W., and Leip, A. (2009). (2009). Development of marginal emission factors for N losses from agricultural soils with the DNDC–CAPRI meta-model. Agri. Ecosyst. Environ. 133, 267–279. doi: 10.1016/j.agee.2009.04.026
Bruinsma, J. E. (2003). World Agriculture: Towards 2015/2030. An FAO perspective. London: Earthscan.
Brzezinski, M. A. (1985). The Si:C:N ratio of marine diatoms: Interspecific variability and the effect of some environmental variables. J. Phycol. 21, 347–357. doi: 10.1111/j.0022-3646.1985.00347.x
Caraco, N. F. (1995). “Influence of human populations on P transfers to aquatic systems: A regional scale study using large rivers,” in Phosphorous in the Global Environment, ed. H. Tiessen (New York, NY: Wiley and Sons), 235–244.
Cloern, J. E. (2001). Our evolving conceptual model of the coastal eutrophication problem. Marine Ecol. Prog. Series 210, 223–253. doi: 10.3354/meps210223
Conley, D. J., Paerl, H. W., Howarth, R. W., Boesch, D. F., Seitzinger, S. P., Havens, K. E., et al. (2009). Controlling eutrophication: nitrogen and phosphorus. Science 323, 1014–1015. doi: 10.1126/science.1167755
Dai, Z., Du, J., Zhang, X., Su, N., and Li, J. (2011). Variation of riverine material loads and environmental consequences on the Changjiang (Yangtze) Estuary in recent decades (1955-2008). Environ. Sci. Technol. 45, 223–227. doi: 10.1021/es103026a
De Vries, W., Kros, J., and Reinds, G. J. E. A. (2009). “INTEGRATOR: a Modelling Tool for European-Wide Assessments of Nitrogen and Greenhouse Gas Fluxes in Response to Changes in Land Cover, Land Management and Climate – Calculation Procedures, Application Methodology and Examples of Scenario Results, Alterra Report. Alterra Wageningen UR: Wageningen.
Dentener, F., Stevenson, D., Ellingsen, K., Noije, T. V., Schultz, M., Amann, M., et al. (2006). The global atmospheric environment for the next generation. Environ. Sci. Technol. 40, 3586–3594. doi: 10.1021/es0523845
Doelman, J. C., Stehfest, E., Tabeau, A., van Meijl, H., Lassaletta, L., Gernaat, D. E. H. J., et al. (2017). (2018). Exploring SSP land-use dynamics using the IMAGE model: Regional and gridded scenarios of land-use change and land-based climate change mitigation. Global Environ. Change 48, 119–135. doi: 10.1016/j.gloenvcha.2017.11.014
Driessen, P. M., and Dudal, R. (1990). Lecture Notes on the Geography, Formation, Properties and Use of the Major Soil of the World. Leuven: Agricultural University Wageningen, Katholieke Universiteit Leuven.
Duarte, C. M. (1995). Submerged aquatic vegetation in relation to different nutrient regimes. Ophelia 41, 87–112. doi: 10.1080/00785236.1995.10422039
FAO (2020). FishStatJ - Software for Fishery and Aquaculture Statistical Time Series. Available online at: http://www.fao.org/fishery/statistics/software/fishstatj/en (Rome: Fisheries and Aquaculture Information and Statistics Service, Food and Agriculture Organization of the United Nations (accessed October 30, 2020).
Finlay, J. C., Small, G. E., and Sterner, R. W. (2013). Human influences on nitrogen removal in lakes. Science 342, 247–250. doi: 10.1126/science.1242575
Galloway, J. N., Schlesinger, W. H., Levy, H., Michaels, A., and Schnoor, J. L. (1995). Nitrogen fixation: anthropogenic enhancement-environmental response. Global Biogeochem. Cycles 9, 235–252. doi: 10.1029/95GB00158
Garnier, J., Beusen, A., Thieu, V., Billen, G., and Bouwman, L. (2010). N:P:Si nutrient export ratios and ecological consequences in coastal seas evaluated by the ICEP approach. Global Biogeochem. Cycles 24:GB0A05. doi: 10.1029/2009GB003583
Glibert, P. M. (2019). Harmful algae at the complex nexus of eutrophication and climate change. Harmful Algae 91, 101583. doi: 10.1016/j.hal.2019.03.001
Green, P., Vörösmarty, C. J., Meybeck, M., Galloway, J. N., Petersen, B. J., Boyer, E. W., et al. (2004). Pre-industrial and contemporary fluxes of nitrogen through rivers: a global assessment based on typology. Biogeochemistry 68, 71–105. doi: 10.1023/B:BIOG.0000025742.82155.92
Grizzetti, B., Bouraoui, F., and Aloe, A. (2012). Changes of nitrogen and phosphorus loads to European seas. Global Change Biol. 18, 769-782. doi: 10.1111/j.1365-2486.2011.02576.x
Grizzetti, B., Bouraoui, F., and Marsily, D. e. G. (2008). Assessing nitrogen pressures on European surface water. Global Biogeochem. Cycles 22, GB4023. doi: 10.1029/2007GB003085
Hartmann, J., Moosdorf, N., Lauerwald, R., Hinderer, M., and West, A. J. (2013). (2014). Global chemical weathering and associated P-release — The role of lithology, temperature and soil properties. Chem. Geol. 363, 145–163. doi: 10.1016/j.chemgeo.2013.10.025
Hempel, S., Frieler, K., Warszawski, L., Schewe, J., and Piontek, F. (2013). A trend-preserving bias correction andndash; the ISI-MIP approach. Earth Syst. Dynam. 4, 219–236. doi: 10.5194/esd-4-219-2013
Keuskamp, J. A., Van Drecht, G., and Bouwman, A. F. (2012). European-scale modelling of groundwater denitrification and associated N2O production. Environ. Pollut. 165, 67–76. doi: 10.1016/j.envpol.2012.02.008
Kroeze, C., Seitzinger, S. P., and Domingues, R. (2001). Future trends in worldwide river nitrogen transport and related nitrous oxide emissions: a scenario analysis. Sci. World J. 2, 328–335. doi: 10.1100/tsw.2001.279
Kros, J., Frumau, K. F. A., Hensen, A., and Vries, d. e. (2011). W. (2011). Integrated analysis of the effects of agricultural management on nitrogen fluxes at landscape scale. Environ. Pollut. 159, 3171–3182. doi: 10.1016/j.envpol.2011.01.033
Langhans, C., Beusen, A. H. W., Mogollón, J. M., and Bouwman, A. F. (2022). Phosphorus for Sustainable Development Goal target of doubling smallholder productivity. Nat. Sust. 5, 57–63. doi: 10.1038/s41893-021-00794-4
Leip, A., Britz, W., Weiss, F., and De Vries, W. (2011). Farm, land, and soil nitrogen budgets for agriculture in Europe calculated with CAPRI. Environ. Pollut. 159, 3243–3253. doi: 10.1016/j.envpol.2011.01.040
Maranger, R., Jones, S. E., and Cotner, J. B. (2018). Stoichiometry of carbon, nitrogen, and phosphorus through the freshwater pipe. Limnol. Oceanograph. Lett. 3, 89–101. doi: 10.1002/lol2.10080
MetOffice (2020). HadCM3: Met Office climate prediction model. Available online at: https://www.metoffice.gov.uk/research/approach/modelling-systems/unified-model/climate-models/hadcm3 (accessed January 16, 2020).
Meybeck, M. (1982). Carbon, nitrogen and phosphorous transport by world rivers. Am. J. Sci. 282, 401–450. doi: 10.2475/ajs.282.4.401
Meybeck, M., and Ragu, A. (1995). River Discharges to Oceans: An Assessment of Suspended Solids, Major Ions and Nutrients. Nairobi: United Nations Environment Programme (UNEP).
Mogollón, J. M., Beusen, A. H. W., van Grinsven, H. J. M., Westhoek, H., and Bouwman, A. F. (2018). Future agricultural phosphorus demand according to the shared socio-economic pathways. Global Environ. Change 50, 149–163. doi: 10.1016/j.gloenvcha.2018.03.007
Neary, D. G., Klopatek, C. C., DeBano, L. F., and Ffolliott, P. F. (1999). Fire effects on belowground sustainability: a review and synthesis. Forest Ecol. Manage. 122, 51–71. doi: 10.1016/S0378-1127(99)00032-8
Newbold, J. D., Elwood, J. W., O'Neill, R. V., and Winkle, W. V. (1981). Measuring nutrient spiralling in streams. Can. J. Fisheries Aquatic Sci. 38, 860–863. doi: 10.1139/f81-114
Redfield, A. C. (1934). On the Proportions of Organic Derivatives in Sea Water and Their Relation to the Composition of Plankton in James Johnstone Memorial Volume. Liverpool: University Press of Liverpool, 176–192.
Redfield, A. C., Ketchum, B. H., and Richards, F. A. (1963). “The influence of organisms on the composition of sea-water,” in The Sea, ed. M.N. Hills (New York, NY: Wiley and Sons), 12–37.
Riahi, K., Van Vuuren, D. P., Kriegler, E., Edmonds, J., O'Neill, B. C., Fujimori, S., et al. (2016). The shared socioeconomic pathways and their energy, land use, and greenhouse gas emissions implications: an overview. Global Environ. Change 42, 153–168. doi: 10.1016/j.gloenvcha.2016.05.009
Romero, E., Garnier, J., Lassaletta, L., Billen, G., Le Gendre, R., Riou, P., et al. (2013). Large-scale patterns of river inputs in southwestern Europe: Seasonal and interannual variations and potential eutrophication effects at the coastal zone. Biogeochemistry 113, 481–505. doi: 10.1007/s10533-012-9778-0
Sattari, S. Z., Bouwman, A. F., Giller, K. E., and van Ittersum, M. K. (2012). Residual soil phosphorus as the missing piece in the global phosphorus crisis puzzle. Proc. Nat. Acad. Sci. 109, 6348–6354. doi: 10.1073/pnas.1113675109
Seitzinger, S. P., Harrison, J. A., Dumont, E., Beusen, A. H. W., and Bouwman, A. F. (2005). Sources and delivery of carbon, nitrogen, and phosphorus to the coastal zone: an overview of Global Nutrient Export from Watersheds (NEWS) models and their application. Global Biogeochem. Cycles 19, GB4S. doi: 10.1029/2005GB002606
Seitzinger, S. P., Mayorga, E., Bouwman, A. F., Kroeze, C., Beusen, A. H. W., Billen, G., et al. (2010). Global river nutrient export: a scenario analysis of past and future trends. Global Biogeochem. Cycles 24, GB0A. doi: 10.1029/2009GB003587
Smil, V. (2000). Phosphorus in the environment: natural flows and human interferences. Ann. Rev. Energ. Environ. 25, 53–88. doi: 10.1146/annurev.energy.25.1.53
Sprague, L. A., Hirsch, R. M., and Aulenbach, B. T. (2011). Nitrate in the mississippi river and its tributaries, 1980 to 2008: are we making progress? Environ. Sci. Technol. 45, 7209–7216. doi: 10.1021/es201221s
Stehfest, E., Van Vuuren, D. P., Kram, T., and Bouwman, A. F. (2014). Integrated Assessment of Global Environmental Change with IMAGE, 0. Model Description and Policy Applications. The Hague: PBL Netherlands Environmental Assessment Agency.
Sutanudjaja, E. H., Van Beek, R., Wanders, N., Wada, Y., Bosmans, J. H. C., Drost, N., et al. (2018). PCR-GLOBWB 2: A 5 arcmin global hydrological and water resources model. Geosci. Model Dev. 11, 2429–2453. doi: 10.5194/gmd-11-2429-2018
Tian, H., Chen, G., Liu, M., Zhang, C., Sun, G., Lu, C., et al. (2009). Model estimates of net primary productivity, evapotranspiration, and water use efficiency in the terrestrial ecosystems of the southern United States during 1895–2007. Forest Ecol. Manage. 259, 1311–1327. doi: 10.1016/j.foreco.2009.10.009
UNEP (2021). Understanding the State of the Ocean: A Global Manual on Measuring SDG 14, 1.1, SDG 14.2.1 and SDG 14.5.1. United Nations Environment Programme, Nairobi.
Van Beek, L. P. H., Wada, Y., and Bierkens, M. F. P. (2011). Global monthly water stress: 1. Water BALANCE and water availability. Water Resour. Res. 47, W07517. doi: 10.1029/2010WR009791
Van Drecht, G., Bouwman, A. F., Knoop, J. M., Beusen, A. H. W., and Meinardi, C. R. (2003). Global modeling of the fate of nitrogen from point and nonpoint sources in soils, groundwater and surface water. Global Biogeochem. Cycles 17, 1115. doi: 10.1029/2003GB002060
Van Meijl, H., Tabeau, A., Stehfest, E., Doelman, J., and Lucas, P. (2020). How food secure are the green, rocky and middle roads: food security effects in different world development paths. Environ. Res. Commun. 2, 031002. doi: 10.1088/2515-7620/ab7aba
Van Puijenbroek, P. J. T. M., Beusen, A. H. W., and Bouwman, A. F. (2018). Global nitrogen and phosphorus in urban waste water based on the Shared Socio-economic pathways. J. Environ. Manage. 231, 446–456. doi: 10.1016/j.jenvman.2018.10.048
Van Vuuren, D. P., Stehfest, E., Gernaat, D., de Boer, H. S., Daioglou, V., Doelman, J. C., et al. (2021). The 2021 SSP Scenarios of the IMAGE 3.2 Model. doi: 10.31223/x5cg92
Van Vuuren, D. P., Stehfest, E., Gernaat, D. E. H. J., Doelman, J. C., Van Den Berg, M., Harmsen, M., et al. (2016). Energy, land-use and greenhouse gas emissions trajectories under a green growth paradigm. Global Environ. Change 42, 237–250. doi: 10.1016/j.gloenvcha.2016.05.008
Vitousek, P. M., Fahey, T., Johnson, D. W., and Swift, M. J. (1988). Element interactions in forest ecosystems: succession, allometry and input-output budgets. Biogeochemistry 5, 7–34. doi: 10.1007/BF02180316
Vitousek, P. M., and Matson, P. A. (1984). Mechanisms of nitrogen retention in forest ecosystems: a field experiment. Science 225, 51–52. doi: 10.1126/science.225.4657.51
Vogt, K. A., Grier, C. C., and Vogt, D. J. (1986). Production, turnover, and nutrient dynamics of above- and belowground detritus of world forests. Adv. Ecol. Res. 15, 303–377. doi: 10.1016/S0065-2504(08)60122-1
Wang, J., Bouwman, A. F., Liu, X., Beusen, A. H. W., Van Dingenen, R., Dentener, F., et al. (2021). Harmful algal blooms in chinese coastal waters will persist due to perturbed nutrient ratios. Environ. Sci. Technol. Lett. 8, 276–284. doi: 10.1021/acs.estlett.1c00012
Wollheim, W., Vörösmarty, C. J., Bouwman, A. F., Green, P., Harrison, J., Meybeck, M., et al. (2008). Global N removal by freshwater aquatic systems using a spatially distributed, within-basin approach. Global Biogeochem. Cycles 22:GB2026. doi: 10.1029/2007GB002963
Woltjer, G. B., and Kuiper, M. H. (2014). The MAGNET Model: Module Description. Wageningen: LEI Wageningen UR University and Research Centre.
Keywords: agriculture, coastal waters, nitrogen, phosphorus, ratio, river, scenario, sewage
Citation: Beusen AHW and Bouwman AF (2022) Future projections of river nutrient export to the global coastal ocean show persisting nitrogen and phosphorus distortion. Front. Water 4:893585. doi: 10.3389/frwa.2022.893585
Received: 10 March 2022; Accepted: 17 October 2022;
Published: 01 November 2022.
Edited by:
Sanjeev Kumar, Physical Research Laboratory, IndiaReviewed by:
Mengru Wang, Wageningen University and Research, NetherlandsXi Chen, University of Cincinnati, United States
Anne Ellen Giblin, Marine Biological Laboratory (MBL), United States
Copyright © 2022 Beusen and Bouwman. This is an open-access article distributed under the terms of the Creative Commons Attribution License (CC BY). The use, distribution or reproduction in other forums is permitted, provided the original author(s) and the copyright owner(s) are credited and that the original publication in this journal is cited, in accordance with accepted academic practice. No use, distribution or reproduction is permitted which does not comply with these terms.
*Correspondence: Arthur H. W. Beusen, YXJ0aHVyLmJldXNlbkBwYmwubmw=
†These authors have contributed equally to this work