- 1Graduate Program of Hydrological Sciences, University of Nevada, Reno, NV, United States
- 2Division of Atmospheric Sciences, Desert Research Institute, Reno, NV, United States
- 3Department of Geography, University of Nevada, Reno, NV, United States
Mountain snowpacks provide 53–78% of water used for irrigation, municipalities, and industrial consumption in the western United States. Snowpacks serve as natural reservoirs during the winter months and play an essential role in water storage for human consumption and ecosystem functions. However, wildfires across the West are increasing in severity, size, and frequency, progressively putting snowpacks at risk as they burn further into the seasonal snow zone. Following a fire, snow disappears 4–23 days earlier and melt rates increase by up to 57%. In a high burn severity fire in the Oregon Cascades, the black carbon and charred woody debris shed from burned trees onto the snowpack decreased the snow albedo by 40%. Canopy cover loss causes a 60% increase in solar radiation reaching the snow surface. Together, these effects produce a 200% increase in net shortwave radiation absorbed by the snowpack. This mini-review synthesizes the implications of wildfire for snow hydrology in mountainous watersheds with the primary aim to characterize wildfires' varied influences on the volume and timing of water resources across time scales (daily to decadal), space (plot to watershed) and burn severity (low to high). The increase in the geographical overlap between fire and snow poses unique challenges for managing snow-dominated watersheds and highlights deficiencies in research and operational snow hydrologic modeling, emphasizing the need for additional field and remote-sensing observations and model experiments.
Introduction
In the western U.S., snow is the primary source of water and streamflow (Li et al., 2017). Mountain snowpacks accumulate during the winter and release meltwater in the spring and summer when water demands are the greatest (Church, 1935; Garen, 1992). Snowpack observations provide information to make streamflow forecasts and inform water allocations, reservoir operations, and irrigation scheduling decisions (Pagano et al., 2004; Anghileri et al., 2016). Snowpacks are vital to recharge downstream reservoirs that capture and store winter precipitation for release during the dry summer months (Mote et al., 2005, 2018). In snow-dominated watersheds, snowmelt-derived runoff contributes up to 80% of the total annual flow (Stewart et al., 2004). Ongoing population growth, urbanization, and aridification simultaneously increase water demand (Boretti and Rosa, 2019). With climate change, snowpacks are predicted to continue to decline by 40–75% by 2,100 (Mote et al., 2018; Rhoades et al., 2021; Siirila-Woodburn et al., 2021). Understanding the quantity of accumulation and timing of snowmelt is integral for managing water resources to meet both consumptive and ecosystem demands.
Concurrent with declining snowpacks, wildfire characteristics are changing. From 1984 to 2020, area burned across the western U.S. increased 1,150%, and in 2020 alone, nearly 6% of California's forested area burned (Williams et al., 2022). From 1984 to 2011, the top 10% of fires by size in California increased by 2 km2yr−1 without a significant increase in the number of fires (Dennison et al., 2014). Especially in years with earlier snowmelt, wildfires burn higher in the snow zone (Alizadeh et al., 2021), which alters western water supply (Kinoshita and Hogue, 2015; Stevens, 2017; Hallema et al., 2018).
Climatic conditions conducive to wildfire including vapor pressure deficit, fuel aridity (Seager et al., 2015), and climatic water deficit (Dobrowski et al., 2013) coincide with increasing fire activity (Abatzoglou and Kolden, 2013; Dennison et al., 2014; Williams and Abatzoglou, 2016). Climate warming and atmospheric drying potential increase fuel loading, which increases the likelihood of extreme fire behavior (Alizadeh et al., 2021). This warming and drying trend is projected to increase across the western U.S., enhanced by more frequent dry days (Polade et al., 2015) and earlier snow disappearance (Moritz et al., 2012; Semmens and Ramage, 2012; Westerling, 2016; Abatzoglou et al., 2021).
The geographical overlap between fire and snow is accelerating. In the western U.S., between 1984 and 2017, Gleason et al. (2019) found a 9% per year increase in area burned within the seasonal snow zone (areas with a persistent winter snowpack). Since 1984, the greatest increase in burned areas has occurred above 2500 m (Alizadeh et al., 2021), well into the seasonal snow zone (Hatchett, 2021). Wildfires in the seasonal snow zone threaten the potential of mountain snowpacks to store water and require a comprehensive bedrock-through-atmosphere perspective to quantify their impacts in a warming world (Siirila-Woodburn et al., 2021).
This mini-review synthesizes peer-reviewed literature examining the impacts of wildfires on mountain snowpacks and water resource availability in the western U.S. It provides an overview of the post-fire impacts on snow including: (1) the impacts of burn severity; (2) snow albedo changes that alter the snowpack energy balance; (3) changing forest canopy that alters accumulation and shading patterns; and (4) altered timing of snow disappearance and peak snow-water equivalent (SWE). Finally, it summarizes current knowledge gaps and concludes with recommendations for future measurements and model improvements.
Burn severity
Fires do not burn evenly across a landscape due to variations in fuels, weather, topography, and suppression tactics. As a result, fire impacts vary widely, creating a mosaic of burn severities. Burn severity is typically lumped into classifications of unburned, low, medium, and high severity (Figure 1A). In high-severity burns, wildfires remove the forest canopy, which increases light transmission through the canopy and decreases longwave radiation emission from once-healthy photosynthesizing trees. These processes increase net shortwave radiation, altering the snowpack energy balance (Pomeroy and Dion, 1996; Burles and Boon, 2011). In a moderately burned forest, some canopy remains and many trees remain alive while in low burn severity, the entire forest canopy remains despite combustion of surface fuels. Each classification has varied effects on post-fire snowpack (Figure 1A).
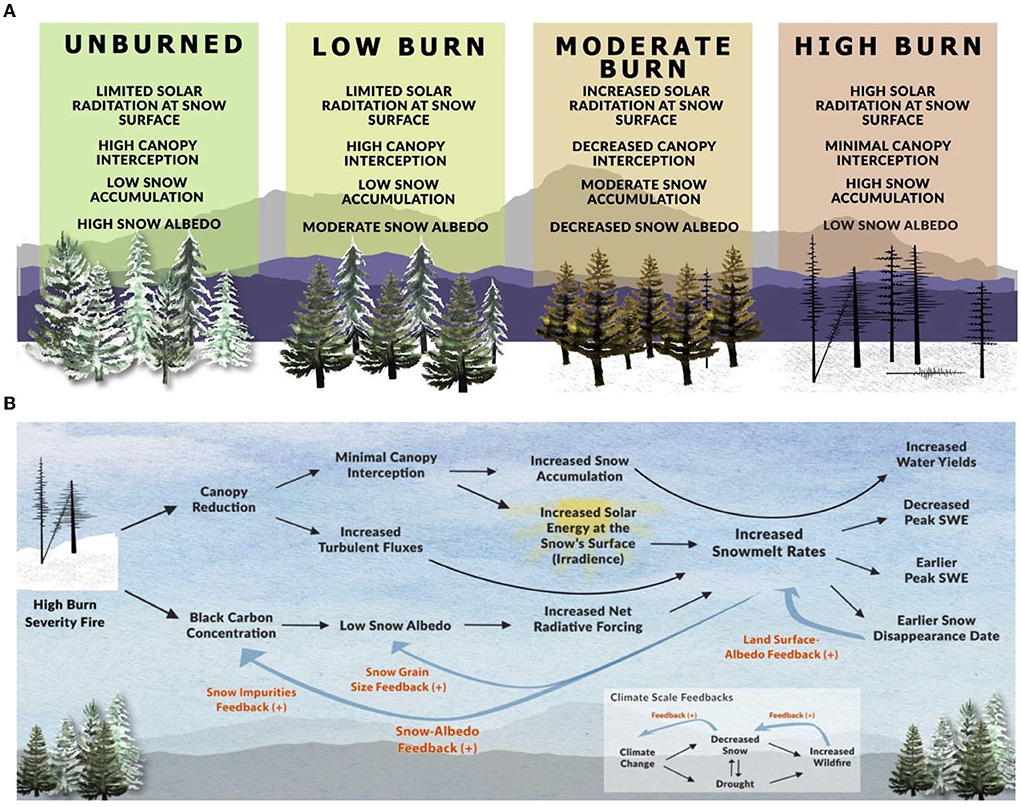
Figure 1. (A) Schematic drawing of changes in snow processes during melt season after a fire for each burn severity. Changes in solar radiation, canopy interception, snow accumulation and snow albedo are noted above. (B) Diagram of post-wildfire effects on snow processes. Existing feedbacks are noted by blue arrows. Climate scale feedbacks are noted in the smaller box on the right.
Burn severity is commonly evaluated by the Difference Normalized Burned Ratio (dNBR) using Landsat satellite images from pre-and post-fire. The dNBR normalizes the reflectance in the near-infrared (NIR) (0.85–0.88 μm) and shortwave-infrared (SWIR) (2.11–2.29 μm) wavelengths to differentiate between burned and unburned vegetation. Burned vegetation and bare earth have high reflectance in the SWIR compared with the NIR, while healthy vegetation has high reflectance in the NIR and is lower in the SWIR.
where RNIR is near-infrared reflectance, RSWIR is shortwave-infrared reflectance and dNBR is the change in NBR from pre- to post-fire (Key and Benson, 2006; Eidenshink et al., 2007; Miller and Thode, 2007; Cardil et al., 2019). Using the dNBR, the Monitoring Trends in Burn Severity (MTBS) program maintains a database of vegetation burn severities published by the U.S. Forest Service (USFS) 2 years after the fire occurs (Eidenshink et al., 2007). Immediately after a fire, the USFS performs on-the-ground assessments for large fires and compares them with remote sensing products to produce the Burned Area Emergency Rehabilitation (BAER) soil burn severity maps. MTBS relies solely on remote sensing to assess post-fire damage to vegetation and is produced for all large fires across the West, while BAER combines ground-truth and remote sensing to evaluate soil burn severity in a subset of large fires. Despite being different metrics and difficult to reproduce, these maps are the most consistent products to-date. Consistency in burn severity maps is challenging (Kolden et al., 2015). For example, the remote sensing image acquisition date affects estimates of vegetation regrowth. The higher vegetation severity (loss of canopy), the more bare soil is exposed. Burn severity classification is a complex, not well-agreed upon, and difficult to replicate process, however the MTBS/BAER datasets provide temporal continuity for research use.
To fully understand the watershed scale impacts of wildfires, we must understand how burn severity variability alters snowpack characteristics and snowmelt rates (Figure 1A). For every 20% increase in overstory mortality, used as a proxy for burn severity, Maxwell and St. Clair (2019) found accumulated snow depth increased by 17% across burn severity gradients in south-central Utah. However, this relationship is weaker at higher elevations where storm intensity is greater and forest density is thinner, overcoming the impacts of canopy structure changes (D'Eon, 2004). The discrepancies in burn severity can partially be explained by the two orders of magnitude difference in black carbon (BC) concentration between high and low burn severity (Uecker et al., 2020). The effects of reduced canopy density also change the energy balance of the snowpack. Most studies focus on specific fire case studies. More research is needed to understand the nuance between burn severities across different forest types and snowpack climates. Most studies compare high burn severity with unburned areas and do not account for low and moderate burn severities. In California, only 18% of fires that occurred from 2000 to 2019 in the snow zone were classified as high burn severity (Koshkin, 2022). Since moderate and low burn severities account for over 65% of the burned area (Koshkin, 2022), additional research is warranted for lower severity fires, especially as prescribed burns are scaled-up. Conceptualizing the effects of fire on snow by burn severity could help understand wildfire's influence on snow-water storage at watershed- to mountain range-scales.
Snow albedo and snowpack energy balance
The high albedo (0.9–0.95) of clean, new snow (Warren, 1982) influences snowpack energy balance from the snow's local surface to global climate scales across varied timescales (hours to millennia) (Skiles et al., 2018). Shortwave albedo is tightly coupled with the energy and mass balance of the snowpack (Dozier et al., 1981; Skiles et al., 2012). As snow albedo decreases, the snowpack absorbs more solar energy and the rates of warming and melt accelerate. This shifts the date of snow disappearance earlier (Warren and Wiscombe, 1980; Gleason et al., 2013). Since snow is highly reflective in the visible wavelengths (400–700 nm), small changes in visible albedo due to light-absorbing particles (LAPs), such as dust, soot, and charred woody debris, increase net shortwave radiation and accelerate snowmelt (Warren and Wiscombe, 1980; Dozier et al., 2009; Skiles et al., 2012; Gleason and Nolin, 2016; Uecker et al., 2020). Burned forests decrease snow albedo by shedding BC and burned woody debris onto the snowpack (Gleason et al., 2013; Gleason and Nolin, 2016; Aubry-Wake et al., 2022). In January, when the snow cover extent is the greatest, Gleason et al. (2019) calculated the radiative forcing from albedo changes to be 32 and 101 Wm−2 1 year after a severe fire using the Snow, Ice, and Aerosol Radiation (SNICAR) model and measured albedo values, respectively. The radiative forcing declined to 23 and 44 Wm−2 15 years post-fire (Gleason et al., 2019). SNICAR uses Mie Theory and cannot capture scattering and absorption from larger LAPs such as woody debris (Flanner and Zender, 2006), implying it provides a minimum estimate of radiative forcing.
LAPs in the snow increase radiative heating and accelerate snowmelt rates (Painter et al., 2007; Flanner et al., 2009; Skiles et al., 2012, 2018; Aubry-Wake et al., 2022). However, BC is an order of magnitude more effective at absorbing solar energy in the visible spectrum compared to mineral dust (Warren and Wiscombe, 1980; Thomas et al., 2017; Gleason et al., 2019). In burned forests, BC can decrease snow albedo by 40%, an effect that is most significant during periods of high insolation (Gleason et al., 2013; Uecker et al., 2020). In high severity burns, forest canopy removal increases incoming solar radiation by 60% (Gleason et al., 2013). Together, the albedo decrease combined with canopy removal increased snowpack net shortwave radiation by 200% in the Oregon Cascades (Gleason et al., 2013). Decreased snow albedo and increased insolation can reduce maximum snow water storage in burned forests because of mid-winter melt events (Musselman et al., 2018). As winter snowpacks continue to diminish, lower snow albedo and increased net shortwave radiation will amplify declines in SWE (Burles and Boon, 2011; Skiles et al., 2012; Thackeray et al., 2014). Together, these processes amplify several feedbacks that occur across climate and local processes-based scales. Most notably, fire intensifies the positive feedback effects of the snow-albedo and land-surface albedo feedbacks (Figure 1B).
Forest-snow-fire interactions
Snowpack mass balance in burned areas is affected by tradeoffs between increased net shortwave and decreased canopy interception (Varhola et al., 2010). A healthy forest canopy intercepts up to 60% of snowfall (Storck et al., 2002; Roth and Nolin, 2019), which reduces snow accumulation by up to 40% (Varhola et al., 2010; Lundquist et al., 2013; Harpold et al., 2014). Post-fire canopy removal increases the snow accumulation in burned areas (Gleason et al., 2013, 2019). On the plot scale, burned areas undergo increases in snow depth during the accumulation season compared to nearby control plots after a fire (Burles and Boon, 2011; Harpold et al., 2014). On a watershed scale, Micheletty et al. (2014) showed a significant increase in basin-average snow cover fraction (fSCA) and the total number of high snow-covered days after a fire due to the canopy reduction.
In an unburned forest, the canopy shades the snow and is a source of longwave radiation, influencing the timing of snowmelt in the forest (Lundberg et al., 2004; Roth and Nolin, 2019). Consequently, canopy removal increases solar radiation and alters turbulent heat fluxes of the snowpack (Faria et al., 2000; Varhola et al., 2010). Without tree cover, the combination of solar forcing and sublimation can result in vapor loss of up to 50% (Molotch et al., 2007; Reba et al., 2012; Musselman et al., 2018). Eddy-covariance measurements demonstrate that sublimation rates in open areas are 3–10 times higher than in forested areas (Reba et al., 2012). For example, Harpold et al. (2014) found a 50% reduction in snow depth in burned forests during winter ablation in New Mexico. A decrease in net longwave radiation (Burles and Boon, 2011) and an increase in sublimation can partially offset and balance the increase in net shortwave radiation (Harpold et al., 2014). Increased solar radiation combined with decreased albedo amplifies the effects of post-fire changes on water retention in snowpacks (Figure 1B).
Timing of snowmelt
Decreased albedo and increased solar radiation accelerate snowmelt after a fire (Figures 1A, 2). In burned forests, snow disappears 4–23 days earlier, and snowmelt rates increase as much as 57% during ablation (Burles and Boon, 2011; Winkler, 2011; Gleason et al., 2013; Uecker et al., 2020). Smoot and Gleason (2021) assessed 78 burned western U.S. SNOTEL sites and found that maximum snow-water storage decreased on average by 30 mm and snowmelt rates increased by 3 mm/day in high severity burned areas compared to unburned areas. In the Washington Cascades, Uecker et al. (2020) showed that in burned areas, 84% of seasonal snow melted out before 1 May compared to only 56% prior to the fire. The effects of reduced water storage and earlier snowmelt can be observed for at least 10 years following a fire (Gleason et al., 2019; Uecker et al., 2020; Smoot and Gleason, 2021; Williams et al., 2022).
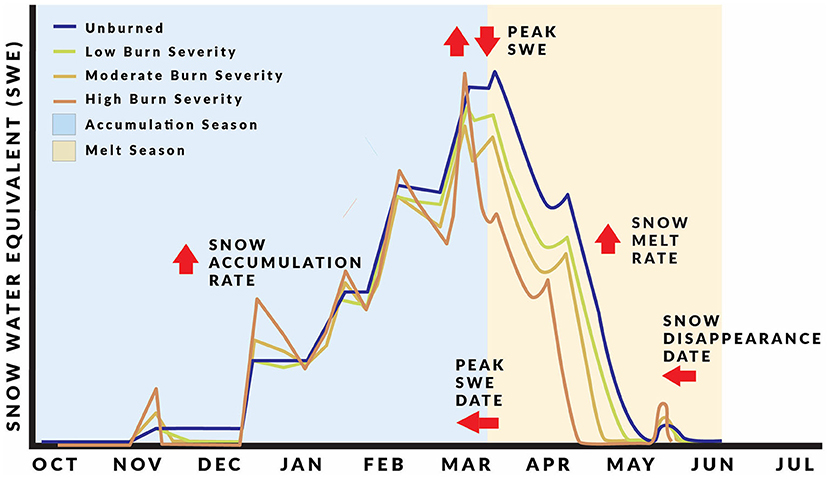
Figure 2. Synthesis of five snow metrics after a wildfire delineated by burn severity including peak snow water equivalent (peak SWE), peak SWE date, snow disappearance date (SDD), snow accumulation rate, and snowmelt rate. Red arrows denote changes after a wildfire.
Lower latitude and warmer maritime climate snowpacks undergo larger post-fire shifts in earlier snow disappearance date, earlier peak SWE, and decreased volume of snow-water storage (Serreze et al., 1999; Sun et al., 2019) (Figure 2). Already more vulnerable to climate warming, warmer snowpacks are persistently near 0°C, therefore a slight change in the energy balance due to increased solar forcing from wildfires imply large-scale impacts on the persistence of the snowpack. Colder and drier continental climates yield shallower snowpacks with higher cold content (Sturm et al., 1995; Sturm and Liston, 2021), but remain susceptible to wildfire and therefore vulnerable to changes in the timing of peak SWE and snow disappearance. The shift in the timing of snowmelt, snow disappearance date, and peak SWE, especially in high burn severity areas, decreases late season runoff (Smoot and Gleason, 2021). Changes in peak SWE appear to be site specific. Smoot and Gleason (2021) found a general trend of decrease in peak SWE post-fire; however, Maxwell and St. Clair (2019) demonstrated a 15% increase in peak SWE in Utah. The region, topography, and inter- and intra- annual climate and weather variability all contribute to variation in post-fire peak SWE outcomes. As a significant contributor to mountain runoff, shifts in snowmelt may have serious consequences on the patterns and magnitudes of late-season stream temperatures and discharge.
Land surface and snowpack models
Investigations of post-fire impacts on snow are primarily remote sensing- or field-based. Small-scale wildfire modeling studies typically focus on infiltration (Ebel, 2013) or run-off and erosion (Rulli and Rosso, 2007; Martin et al., 2011). Larger-scale modeling focuses on evapotranspiration (Bond-Lamberty et al., 2009; Roche et al., 2018) or overland flow (Beeson et al., 2001; McMichael and Hope, 2007). No study has directly simulated landscape changes with the sole focus of snow processes. Maina and Siirila-Woodburn (2020) use a physically-based model to assess post-fire watershed hydrology incorporating snow dynamics in the Northern Sierra Nevada. They found that SWE increases after a wildfire, increasing water yields and following snowmelt, post-fire changes in surface roughness increased surface flow (Maina and Siirila-Woodburn, 2020). Beyond this study, most runoff models (e.g., iSNOBAL, Alpine3D, and SnowModel) and land surface models (e.g., NOAH, CLM, ISBA) do not explicitly incorporate post-fire landscape changes. Neither type of model accurately parameterizes post-fire snow albedo nor effectively characterizes burned landscapes (Marks et al., 1999; Lehning et al., 2006; Liston and Elder, 2006). Therefore, these models may not adequately account for increasing net shortwave radiation or turbulent heat fluxes. Gridded snow reanalyses also do not explicitly account for post-wildfire landscape changes (Broxton et al., 2016; Margulis et al., 2016). Therefore, time-series analyses based on these data products may not adequately represent post-fire changes in snow hydrology and canopy structure.
The lack of albedo parameterization and incorporation of LAPs in climate and energy balance models increases uncertainty in snowmelt quantity and timing (Hedrick et al., 2018; Skiles et al., 2018). Earth system models have shown notable sensitivity to changes in snow-albedo (Letcher and Minder, 2015). However, radiative transfer models still miss the mark on incorporating LAP in snow radiative forcing (Hao et al., 2022). Gleason and Nolin (2016) developed an albedo decay function that accounts for BC. Although implemented into SnowModel, this parameterization has not yet been incorporated into distributed watershed models. Incorporating observational daily updates to albedo and seasonal updates to canopy structure could help constrain changes to canopy structure and the snowpack energy balance. Still, the problem of scales remains. Ultimately, we need to bring together multi-scale models to incorporate these changes into models spanning the atmosphere to bedrock to fully address this issue (Siirila-Woodburn et al., 2021). If net shortwave and topography are the biggest drivers of changes in SWE (Maina and Siirila-Woodburn, 2020), then incorporating canopy changes and snow albedo is imperative to reduce uncertainty in land surface (Letcher and Minder, 2015; Hao et al., 2022). Additional physically-based model studies examining the direct impacts of wildfire-induced landscape changes on snowpack dynamics are needed to supplement growing observational studies.
Conclusion
Wildfires yield persistent and widespread effects on snow hydrology. As fires burn larger, more frequently, and higher in elevation, snowpacks are increasingly vulnerable. It is critical to understand the sensitivity of snow-dominated watersheds to wildfire's influences to evaluate direct and indirect impacts and feedbacks on snow-derived water resources. Researchers and managers alike can leverage models and observations to gain a more complete understanding of impacts, however improvements are needed. Explicitly incorporating fire impacts into SWE reanalysis products and parameterizing their effects in land surface models represents a recommended first step toward improving our understanding of post-fire outcomes. The increasing spatial overlap between fire and snow creates a need to continue observing the physical processes accompanying these landscape-altering events. To better assess wildfire impacts on snow, we recommend prioritizing more albedo and canopy density multi-scale observations to improve parameterizations in research and operational models. Evaluating the changes in net shortwave radiation in post-fire environments is critical to skillfully simulate runoff magnitude and timing. Further research is needed to understand the uncertainties associated with multi-scale (meter to watershed) and regional variability in wildfire impacts on snowmelt timing and snow-water storage. This predictive skill hinges on representative data to improve hydrologic and climate models' forecasting capabilities at daily to decadal scales.
Author contributions
AK and BH designed the framework of the review and designed the figures. AK prepared the manuscript. BH and AN contributed to the scope of the review and provided manuscript comments and revisions. All authors contributed to the article and approved the submitted version.
Funding
Financial support for this study was provided by the National Integrated Drought Information System under agreement 1332KP21DNEEN0006 and Nolin research start-up funding from the University of Nevada, Reno. Any use of trade, product, or firm names is for descriptive purposes only and does not imply endorsement by the U.S. government.
Acknowledgments
Authors would like to thank Jessi LeMay for her graphical expertise in helping design both Figures 1, 2. Authors would also like to thank Dr. Nicoleta Cristea and the reviewer of the manuscript for timely, insightful feedback which helped improve the overall paper.
Conflict of interest
The authors declare that the research was conducted in the absence of any commercial or financial relationships that could be construed as a potential conflict of interest.
Publisher's note
All claims expressed in this article are solely those of the authors and do not necessarily represent those of their affiliated organizations, or those of the publisher, the editors and the reviewers. Any product that may be evaluated in this article, or claim that may be made by its manufacturer, is not guaranteed or endorsed by the publisher.
References
Abatzoglou, J. T., Juang, C. S., Williams, A. P., Kolden, C. A., and Westerling, A. L. (2021). Increasing synchronous fire danger in forests of the western United States. Geophys. Res. Lett. 48. doi: 10.1029/2020GL091377
Abatzoglou, J. T., and Kolden, C. A. (2013). Relationships between climate and macroscale area burned in the western United States. Int. J. Wildland Fire 22, 1003. doi: 10.1071/WF13019
Alizadeh, M. R., Abatzoglou, J. T., Luce, C. H., Adamowski, J. F., Farid, A., and Sadegh, M. (2021). Warming enabled upslope advance in western US forest fires. Proc. Natl. Acad. Sci USA. 118, e2009717118. doi: 10.1073/pnas.2009717118
Anghileri, D., Voisin, N., Castelletti, A., Pianosi, F., Nijssen, B., and Lettenmaier, D. P. (2016). Value of long-term streamflow forecasts to reservoir operations for water supply in snow-dominated river catchments. Water Res. Res. 52, 4209–4225. doi: 10.1002/2015WR017864
Aubry-Wake, C., Bertoncini, A., and Pomeroy, J. W. (2022). Fire and ice: the impact of wildfire-affected albedo and irradiance on glacier melt. Earth's Fut. 10. doi: 10.1029/2022EF002685
Beeson, P. C., Martens, S. N., and Breshears, D. D. (2001). Simulating overland flow following wildfire: mapping vulnerability to landscape disturbance. Hydrol. Proc. 15, 2917–2930. doi: 10.1002/hyp.382
Bond-Lamberty, B., Peckham, S. D., Gower, S. T., and Ewers, B. E. (2009). Effects of fire on regional evapotranspiration in the central Canadian boreal forest. Global Change Biol. 15, 1242–1254. doi: 10.1111/j.1365-2486.2008.01776.x
Boretti, A., and Rosa, L. (2019). Reassessing the projections of the World Water Development Report. Npj Clean Water 2, 15. doi: 10.1038/s41545-019-0039-9
Broxton, P. D., Dawson, N., and Zeng, X. (2016). Linking snowfall and snow accumulation to generate spatial maps of SWE and snow depth. Earth Space Sci. 3, 246–256. doi: 10.1002/2016EA000174
Burles, K., and Boon, S. (2011). Snowmelt energy balance in a burned forest plot, crowsnest Pass, Alberta, Canada. Hydrol. Proc. 25, 3012–3029. doi: 10.1002/hyp.8067
Cardil, A., Mola-Yudego, B., Blázquez-Casado, Á., and González-Olabarria, J. R. (2019). Fire and burn severity assessment: calibration of relative differenced normalized burn ratio (RdNBR) with field data. J. Environ. Manag. 235, 342–349. doi: 10.1016/j.jenvman.2019.01.077
Church, J. (1935). Principles of snow surveying as applied to forecasting stream flow. J. Agric. Res. 51, 97–130.
Dennison, P. E., Brewer, S. C., Arnold, J. D., and Moritz, M. A. (2014). Large wildfire trends in the western United States, 1984-2011. Geophys. Res. Lett. 41, 2928–2933. doi: 10.1002/2014GL059576
D'Eon, R. G. (2004). Snow Depth as a Function of Canopy Cover (No. 2). JC J. Ecosyst. Manag. 3, 1–9. Available online at: https://citeseerx.ist.psu.edu/viewdoc/download?doi=10.1.1.556.1657&rep=rep1&type=pdf
Dobrowski, S. Z., Abatzoglou, J., Swanson, A. K., Greenberg, J. A., Mynsberge, A. R., Holden, Z. A., et al. (2013). The climate velocity of the contiguous United States during the 20th century. Global Change Biol. 19, 241–251. doi: 10.1111/gcb.12026
Dozier, J., Green, R. O., Nolin, A. W., and Painter, T. H. (2009). Interpretation of snow properties from imaging spectrometry. Remote Sens. Environ. 113(Suppl. 1), S25–S37. doi: 10.1016/j.rse.2007.07.029
Dozier, J., Schneider, S. R., and McGinnis, D. F. (1981). Effect of grain size and snowpack water equivalence on visible and near-infrared satellite observations of snow. Water Res. Res. 17, 1213–1221. doi: 10.1029/WR017i004p01213
Ebel, B. A. (2013). Simulated unsaturated flow processes after wildfire and interactions with slope aspect. Water Res. Res. 49, 8090–8107. doi: 10.1002/2013WR014129
Eidenshink, J., Schwind, B., Brewer, K., Zhu, Z.-L., Quayle, B., and Howard, S. (2007). A project for monitoring trends in burn severity. Fire Ecol. 3, 3–21. doi: 10.4996/fireecology.0301003
Faria, D. A., Pomeroy, J. W., and Essery, R. L. H. (2000). Effect of covariance between ablation and snow water equivalent on depletion of snow-covered area in a forest. Hydrol. Proc. 14, 2683–2695. doi: 10.1002/1099-1085(20001030)14:15<2683::AID-HYP86>3.0.CO;2-N
Flanner, M. G., and Zender, C. S. (2006). Linking snowpack microphysics and albedo evolution. J. Geophys. Res. 111, D12208. doi: 10.1029/2005JD006834
Flanner, M. G., Zender, C. S., Hess, P. G., Mahowald, N. M., Painter, T. H., Ramanathan, V., et al. (2009). Springtime warming and reduced snow cover from carbonaceous particles. Atmosp. Chem. Phys. 9, 2481–2497. doi: 10.5194/acp-9-2481-2009
Garen, D. (1992). Garen_JWRPM_1992.pdf. J. Water Res. Plan. Manag. 118, 654–670. doi: 10.1061/(ASCE)0733-9496(1992)118:6(654)
Gleason, K. E., McConnell, J. R., Arienzo, M. M., Chellman, N., and Calvin, W. M. (2019). Four-fold increase in solar forcing on snow in western U.S. burned forests since 1999. Nat. Commun. 10, 2026. doi: 10.1038/s41467-019-09935-y
Gleason, K. E., and Nolin, A. W. (2016a). Charred forests accelerate snow albedo decay: parameterizing the post-fire radiative forcing on snow for three years following fire: charred forests accelerate snow albedo decay. Hydrol. Proc. 30, 3855–3870. doi: 10.1002/hyp.10897
Gleason, K. E., Nolin, A. W., and Roth, T. R. (2013). Charred forests increase snowmelt: effects of burned woody debris and incoming solar radiation on snow ablation. Geophys. Res. Lett. 40, 4654–4661. doi: 10.1002/grl.50896
Hallema, D. W., Sun, G., Caldwell, P. V., Norman, S. P., Cohen, E. C., Liu, Y., et al. (2018). Burned forests impact water supplies. Nat. Commun. 9, 1307. doi: 10.1038/s41467-018-03735-6
Hao, D., Bisht, G., He, C., Bair, E., Huang, H., Dang, C., et al. (2022). Improving Snow Albedo Modeling in E3SM Land Model (version 2.0) and Assessing Its Impacts on Snow and Surface Fluxes Over the Tibetan Plateau. Munich: European Geophysical Union-Geoscientific Model Development.
Harpold, A. A., Biederman, J. A., Condon, K., Merino, M., Korgaonkar, Y., Nan, T., et al. (2014). Changes in snow accumulation and ablation following the Las Conchas Forest Fire, New Mexico, USA. Ecohydrology 7, 440–452. doi: 10.1002/eco.1363
Hatchett, B. J. (2021). Seasonal and ephemeral snowpacks of the conterminous United States. Hydrology 8, 32. doi: 10.3390/hydrology8010032
Hedrick, A. R., Marks, D., Havens, S., Robertson, M., Johnson, M., Sandusky, M., et al. (2018). Direct insertion of NASA airborne snow observatory-derived snow depth time series into the isnobal energy balance snow model. Water Reso. Res. 54, 8045–8063. doi: 10.1029/2018WR023190
Key, C. H., and Benson, N. C. (2006). “Landscape assessment (LA),” in FIREMON: Fire Effects Monitoring and Inventory System (Ogden, UT: USDA Forest Service, Rocky Mountain Research Station), 1–51.
Kinoshita, A. M., and Hogue, T. S. (2015). Increased dry season water yield in burned watersheds in Southern California. Environ. Res. Lett. 10, 014003. doi: 10.1088/1748-9326/10/1/014003
Kolden, C. A., Smith, A. M. S., and Abatzoglou, J. T. (2015). Limitations and utilization of monitoring trends in burn severity products for assessing wildfire severity in the USA. Int. J. Wildland Fire. 24, 1023–1028. doi: 10.1071/WF15082
Koshkin, A. L. (2022). Changes in snow hydrology after a severe wildfire (Masters Thesis). University of Nevada, Reno, NV, United States.
Lehning, M., Völksch, I., Gustafsson, D., Nguyen, T. A., Stähli, M., and Zappa, M. (2006). ALPINE3D: a detailed model of mountain surface processes and its application to snow hydrology. Hydrol. Proc. 20, 2111–2128. doi: 10.1002/hyp.6204
Letcher, T. W., and Minder, J. R. (2015). Characterization of the simulated regional snow albedo feedback using a regional climate model over complex terrain. J. Climate 28, 7576–7595. doi: 10.1175/JCLI-D-15-0166.1
Li, D., Wrzesien, M. L., Durand, M., Adam, J., and Lettenmaier, D. P. (2017). How much runoff originates as snow in the western United States, and how will that change in the future? Geophys. Res. Lett. 44, 6163–6172. doi: 10.1002/2017GL073551
Liston, G. E., and Elder, K. (2006). A distributed snow-evolution modeling system (SnowModel). J. Hydrometeorol. 7, 1259–1276. doi: 10.1175/JHM548.1
Lundberg, A., Nakai, Y., Thunehed, H., and Halldin, S. (2004). Snow accumulation in forests from ground and remote-sensing data. Hydrol. Proc. 18, 1941–1955. doi: 10.1002/hyp.1459
Lundquist, J. D., Dickerson-Lange, S. E., Lutz, J. A., and Cristea, N. C. (2013). Lower forest density enhances snow retention in regions with warmer winters: A global framework developed from plot-scale observations and modeling. Water Reso. Res. doi: 10.1002/wrcr.20504
Maina, F. Z., and Siirila-Woodburn, E. R. (2020). Watersheds dynamics following wildfires: Nonlinear feedbacks and implications on hydrologic responses. Hydrol. Proc. 34, 33–50. doi: 10.1002/hyp.13568
Margulis, S. A., Cortés, G., Girotto, M., Durand, M., Margulis, S. A., Cortés, G., et al. (2016). A landsat-era sierra nevada snow reanalysis (1985–2015). J. Hydrometeorol. 17, 1203–1221. doi: 10.1175/JHM-D-15-0177.1
Marks, D., Domingo, J., Susong, D., Link, T., and Garen, D. (1999). A spatially distributed energy balance snowmelt model for application in mountain basins. Hydrol. Proc. 13:1935–59. doi: 10.1002/(SICI)1099-1085(199909)13:12/13<1935::AID-HYP868>3.0.CO;2-C
Martin, Y. E., Johnson, E. A., Gallaway, J. M., and Chaikina, O. (2011). Negligible soil erosion in a burned mountain watershed, Canadian rockies: field and modelling investigations considering the role of duff. Earth Surface Proc. Landforms 36, 2097–2113. doi: 10.1002/esp.2236
Maxwell, J., and St Clair, S. B. (2019). Snowpack properties vary in response to burn severity gradients in montane forests. Environ. Res. Lett. 14, 124094. doi: 10.1088/1748-9326/ab5de8
McMichael, C. E., and Hope, A. S. (2007). Predicting streamflow response to fire-induced landcover change: implications of parameter uncertainty in the MIKE SHE model. J. Environ. Manag. 84, 245–256. doi: 10.1016/j.jenvman.2006.06.003
Micheletty, P. D., Kinoshita, A. M., and Hogue, T. S. (2014). Application of MODIS snow cover products: wildfire impacts on snow and melt in the Sierra Nevada. Hydrol. Earth Syst. Sci. 18, 4601–4615. doi: 10.5194/hess-18-4601-2014
Miller, J. D., and Thode, A. E. (2007). Quantifying burn severity in a heterogeneous landscape with a relative version of the delta Normalized Burn Ratio (dNBR). Remote Sens. Environ. 109, 66–80. doi: 10.1016/j.rse.2006.12.006
Molotch, N., Blanken, P., Williams, M., Turnipseed, A., Monson, R., and Margulis, S. (2007). Estimating sublimation of intercepted and sub-canopy snow using eddy covariance system. Hydrol. Proc. 21, 1567–1575. doi: 10.1002/hyp.6719
Moritz, M. A., Parisien, M.-A., Batllori, E., Krawchuk, M. A., Van Dorn, J., Ganz, D. J., et al. (2012). Climate change and disruptions to global fire activity. Ecosphere 3:art49. doi: 10.1890/ES11-00345.1
Mote, P. W., Hamlet, A. F., Clark, M. P., and Lettenmaier, D. P. (2005). Declining mountain snowpack in western north America. Bulletin Am. Meteorol. Soc. 86, 39–49. doi: 10.1175/BAMS-86-1-39
Mote, P. W., Li, S., Lettenmaier, D. P., Xiao, M., and Engel, R. (2018). Dramatic declines in snowpack in the western US. Npj Clim. Atmospheric Sci. 1, 2. doi: 10.1038/s41612-018-0012-1
Musselman, K. N., Lehner, F., Ikeda, K., Clark, M. P., Prein, A. F., Liu, C., et al. (2018). Projected increases and shifts in rain-on-snow flood risk over western North America. Nat. Climate Change 8, 808–812. doi: 10.1038/s41558-018-0236-4
Pagano, T., Garen, D., and Sorooshian, S. (2004). Evaluation of official western U.S. seasonal water supply outlooks, 1922–2002. J. Hydrometeorol. 5, 896–909. doi: 10.1175/1525-7541(2004)005<0896:EOOWUS>2.0.CO;2
Painter, T. H., Barrett, A. P., Landry, C. C., Neff, J. C., Cassidy, M. P., Lawrence, C. R., et al. (2007). Impact of disturbed desert soils on duration of mountain snow cover. Geophys. Res. Lett. 34, L12502. doi: 10.1029/2007GL030284
Polade, S. D., Pierce, D. W., Cayan, D. R., Gershunov, A., and Dettinger, M. D. (2015). The key role of dry days in changing regional climate and precipitation regimes. Sci. Rep. 4, 4364. doi: 10.1038/srep04364
Pomeroy, J. W., and Dion, K. (1996). Winter radiation extinction and reflection in a boreal pine canopy: measurements and modelling. Hydrol. Proc. 10, 1591–1608. doi: 10.1002/(SICI)1099-1085(199612)10:12<1591::AID-HYP503>3.0.CO;2-8
Reba, M. L., Pomeroy, J., Marks, D., and Link, T. E. (2012). Estimating surface sublimation losses from snowpacks in a mountain catchment using eddy covariance and turbulent transfer calculations. Hydrol. Proc. 26, 3699–3711. doi: 10.1002/hyp.8372
Rhoades, A., Hatchett, B., Risser, M., Collins, W., Bambach, N., Huning, L., et al. (2021). Asymmetric Emergence of Low-to-No Snow in the American Cordillera. Berlin: Nature Portfolio.
Roche, J. W., Bales, R. C., Rice, R., and Marks, D. G. (2018). Management implications of snowpack sensitivity to temperature and atmospheric moisture changes in yosemite national Park, CA. J. Am. Water Res. Assoc. 54, 724–741. doi: 10.1111/1752-1688.12647
Roth, T. R., and Nolin, A. W. (2019). Characterizing maritime snow canopy interception in forested mountains. Water Res. Res. 55, 4564–4581. doi: 10.1029/2018WR024089
Rulli, M. C., and Rosso, R. (2007). Hydrologic response of upland catchments to wildfires. Adv. Water Res. 30, 2072–2086. doi: 10.1016/j.advwatres.2006.10.012
Seager, R., Hooks, A., Williams, A. P., Cook, B., Nakamura, J., and Henderson, N. (2015). Climatology, variability, and trends in the U.S. Vapor pressure deficit, an important fire-related meteorological quantity. J. Appl. Meteorol. Climatol. 54, 1121–1141. doi: 10.1175/JAMC-D-14-0321.1
Semmens, K. A., and Ramage, J. (2012). Investigating correlations between snowmelt and forest fires in a high latitude snowmelt dominated drainage basin. Hydrol. Proc. 26, 2608–2617. doi: 10.1002/hyp.9327
Serreze, M. C., Clark, M. P., Armstrong, R. L., McGinnis, D. A., and Pulwarty, R. S. (1999). Characteristics of the western United States snowpack from snowpack telemetry (SNOTEL) data. Water Reso. Res. 35, 2145–2160. doi: 10.1029/1999WR900090
Siirila-Woodburn, E. R., Rhoades, A. M., Hatchett, B. J., Huning, L. S., Szinai, J., Tague, C., et al. (2021). A low-to-no snow future and its impacts on water resources in the western United States. Nat. Rev. Earth Environ. 2, 800–819. doi: 10.1038/s43017-021-00219-y
Skiles, S. M., Flanner, M., Cook, J. M., Dumont, M., and Painter, T. H. (2018). Radiative forcing by light-absorbing particles in snow. Nat. Clim. Change 8, 964–971. doi: 10.1038/s41558-018-0296-5
Skiles, S. M., Painter, T. H., Deems, J. S., Bryant, A. C., and Landry, C. C. (2012). Dust radiative forcing in snow of the upper colorado river basin: 2. Interannual variability in radiative forcing and snowmelt rates. Water Reso. Res. 48, 1–11. doi: 10.1029/2012WR011986
Smoot, E. E., and Gleason, K. E. (2021). Forest fires reduce snow-water storage and advance the timing of snowmelt across the western U.S. Water 13, 3533. doi: 10.3390/w13243533
Stevens, J. T. (2017). Scale-dependent effects of post-fire canopy cover on snowpack depth in montane coniferous forests. Ecol. Appl. 27, 1888–1900. doi: 10.1002/eap.1575
Stewart, I., Cayan, D., and Dettinger, M. (2004). Changes in snowmelt runoff timing in western North America under abusiness as usual'. Clim. Change 1, 8–9. doi: 10.1023/B:CLIM.0000013702.22656.e8
Storck, P., Lettenmaier, D. P., and Bolton, S. M. (2002). Measurement of snow interception and canopy effects on snow accumulation and melt in a mountainous maritime climate, Oregon, United States. Water Reso. Res. 38, 5-1-5–16. doi: 10.1029/2002WR001281
Sturm, M., Holmgren, J., and Liston, G. (1995). A seasonal snow cover classification system for local to global applications. J. Clim. 8, 1261–1283. doi: 10.1175/1520-0442(1995)008[1261:ASSCCS]2.0.CO;2
Sturm, M., and Liston, G. E. (2021). Revisiting the global seasonal snow classification: an updated dataset for earth system applications. J. Hydrometeorol. 22, 2917–2938. doi: 10.1175/JHM-D-21-0070.1
Sun, N., Yan, H., Wigmosta, M. S., Leung, L. R., Skaggs, R., and Hou, Z. (2019). Regional snow parameters estimation for large-domain hydrological applications in the Western United States. J. Geophys. Res. Atmosp. 124, 5296–5313. doi: 10.1029/2018JD030140
Thackeray, C. W., Fletcher, C. G., and Derksen, C. (2014). The influence of canopy snow parameterizations on snow albedo feedback in boreal forest regions: Boreal forest snow albedo feedback. J. Geophys. Res. Atmosp. 119, 9810–9821. doi: 10.1002/2014JD021858
Thomas, J. L., Polashenski, C. M., Soja, A. J., Marelle, L., Casey, K. A., Choi, H. D., et al. (2017). Quantifying black carbon deposition over the Greenland ice sheet from forest fires in Canada. Geophys. Res. Lett. 44, 7965–7974. doi: 10.1002/2017GL073701
Uecker, T. M., Kaspari, S. D., Musselman, K. N., and McKenzie Skiles, S. (2020). The post-wildfire impact of burn severity and age on black carbon snow deposition and implications for snow water resources, cascade range, Washington. J. Hydrometeorol. 21, 1777–1792. doi: 10.1175/JHM-D-20-0010.1
Varhola, A., Coops, N. C., Weiler, M., and Moore, R. D. (2010). Forest canopy effects on snow accumulation and ablation: an integrative review of empirical results. J. Hydrol. 392, 219–233. doi: 10.1016/j.jhydrol.2010.08.009
Warren, S. G. (1982). Optical properties of snow. Rev. Geophys. 20, 67. doi: 10.1029/RG020i001p00067
Warren, S. G., and Wiscombe, W. J. (1980). A model for the spectral albedo of snow. ii: snow containing atmospheric aerosols. J. Atmosp. Sci. 37, 2734–2745. doi: 10.1175/1520-0469(1980)037<2734:AMFTSA>2.0.CO;2
Westerling, A. L. R. (2016). Increasing western US forest wildfire activity: sensitivity to changes in the timing of spring. Philosoph Trans. Royal Soc. B Biol. Sci. 371, 1–10. doi: 10.1098/rstb.2015.0178
Williams, A. P., and Abatzoglou, J. T. (2016). Recent advances and remaining uncertainties in resolving past and future climate effects on global fire activity. Curr. Clim. Change Rep. 2, 1–14. doi: 10.1007/s40641-016-0031-0
Williams, A. P., Livneh, B., McKinnon, K. A., Hansen, W. D., Mankin, J. S., Cook, B. I., et al. (2022). Growing impact of wildfire on western US water supply. Proc. Natl. Acad. Sci. 119, e2114069119. doi: 10.1073/pnas.2114069119
Keywords: snow hydrology, forest-snow interactions, wildfires, snowmelt acceleration, snow-water storage, snow albedo, burn severity
Citation: Koshkin AL, Hatchett BJ and Nolin AW (2022) Wildfire impacts on western United States snowpacks. Front. Water 4:971271. doi: 10.3389/frwa.2022.971271
Received: 16 June 2022; Accepted: 05 August 2022;
Published: 26 August 2022.
Edited by:
Nicoleta Cristea, University of Washington, United StatesReviewed by:
Erkan Istanbulluoglu, University of Washington, United StatesCopyright © 2022 Koshkin, Hatchett and Nolin. This is an open-access article distributed under the terms of the Creative Commons Attribution License (CC BY). The use, distribution or reproduction in other forums is permitted, provided the original author(s) and the copyright owner(s) are credited and that the original publication in this journal is cited, in accordance with accepted academic practice. No use, distribution or reproduction is permitted which does not comply with these terms.
*Correspondence: Arielle L. Koshkin, YXJpZWxsZS5rb3Noa2luQGdtYWlsLmNvbQ==