- 1Department of Natural Resources and the Environment, University of New Hampshire, Durham, NH, United States
- 2Department of Biological and Ecological Engineering, Oregon State University, Corvallis, OR, United States
- 3Department of Geosciences, Colorado State University, Fort Collins, CO, United States
- 4U.S. Geological Survey, Earth System Processes Division, Reston, VA, United States
River corridors integrate the active channels, geomorphic floodplain and riparian areas, and hyporheic zone while receiving inputs from the uplands and groundwater and exchanging mass and energy with the atmosphere. Here, we trace the development of the contemporary understanding of river corridors from the perspectives of geomorphology, hydrology, ecology, and biogeochemistry. We then summarize contemporary models of the river corridor along multiple axes including dimensions of space and time, disturbance regimes, connectivity, hydrochemical exchange flows, and legacy effects of humans. We explore how river corridor science can be advanced with a critical zone framework by moving beyond a primary focus on discharge-based controls toward multi-factor models that identify dominant processes and thresholds that make predictions that serve society. We then identify opportunities to investigate relationships between large-scale spatial gradients and local-scale processes, embrace that riverine processes are temporally variable and interacting, acknowledge that river corridor processes and services do not respect disciplinary boundaries and increasingly need integrated multidisciplinary investigations, and explicitly integrate humans and their management actions as part of the river corridor. We intend our review to stimulate cross-disciplinary research while recognizing that river corridors occupy a unique position on the Earth's surface.
1. Introduction
1.1. Motivations and overview
The critical zone (CZ) is an open system of coupled chemical, biological, and physical processes operating together to support life at Earth's surface. It is defined as the volume of the near-surface from the weathering front to the top of the vegetative canopy (National Research Council, 2001; Brantley et al., 2007; Rasmussen et al., 2011). The CZ perspective has been powerful in advancing multidisciplinary research that crosses scales to integrate processes that regulate fluxes of energy, material, and water. River corridors exist as unique landforms within the CZ that span the terrestrial-aquatic interface, actively exchanging mass and energy with the contemporary river channel and reflecting the history and evolution of the drainage network. The river corridor integrates the active channel(s), geomorphic floodplain, riparian areas, and the underlying hyporheic zone. The river corridor also receives inputs from the surrounding hillslopes and exchanges water and solutes with groundwater systems, while also interacting with the atmosphere (National Research Council, 2001; Harvey and Gooseff, 2015; Figure 1).
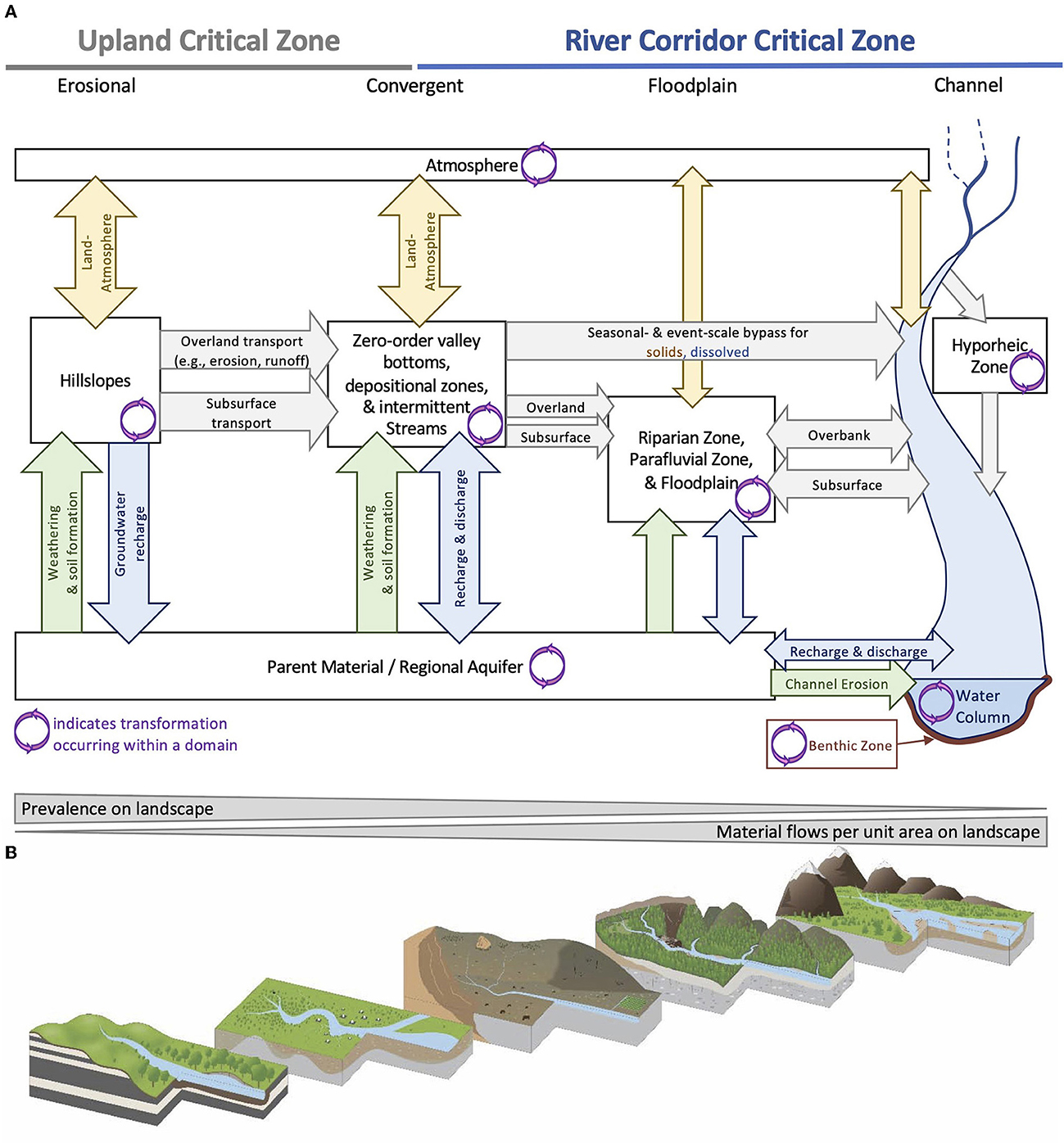
Figure 1. Conceptualization of the river corridor as part of the critical zone, as a landscape element that integrates, stores, transforms, and modulates the downstream export of dissolved and particulate constituents. Existing critical zone science has predominantly focused on upland, erosional environments, which is reasonable given their prevalence on the landscape. However, we contend the river corridor is substantially under-studied given its role in connecting upland with lowland terrestrial systems as well as exporting materials and energy to lacustrine, estuarine, and marine receiving waters. The river corridor also has the highest material fluxes per unit area on the landscape among all terrestrial and aquatic environments, making it an essential connector of landscape elements in Earth's critical zone. The process conceptualizations in (A) determine which process(es) or landscape element(s) are dominant for the river corridor functions in (B) that illustrate a range of geologic and climatic settings in the U.S. including (left-to-right) Konza Prairie, KS; Ipswich River, MA; Reynolds Creek, ID; H.J. Andrews, OR; Middle Fork Flathead River, MT.
Our overarching objectives in this paper are to trace how we have come to a contemporary understanding of river corridors and how this understanding can be advanced to meet emerging environmental grand challenges. We embrace the CZ framework as a basis to inform the sustainable management of river corridors as part of Earth's livable near surface. This includes using the physical definition of the CZ (bedrock to canopy) as well as recognition of the multiple spatial and temporal scales implicit in the study of the CZ (Brantley et al., 2007). To accomplish these goals, we provide a history of the development of river corridor science and assess the state of river corridor integration into the CZ framework to answer the following questions: How has the river corridor already been studied as an integrated system? Why is the CZ approach appropriate for study of river corridors? What elements of the CZ approach are lacking in the current study of river corridors? What opportunities would the CZ approach bring to advance our predictive understanding of river corridors?
The CZ perspective focuses on Earth's living and thin skin, requiring multi-scale and cross-disciplinary approaches to explain and predict earth surface processes that sustain life (Amundson et al., 2007; Brantley et al., 2007; Chorover et al., 2007). These features are a hallmark of modern river corridor science (Ward and Packman, 2019; Krause et al., 2022). While occupying only a small fraction of Earth's surface (e.g., 1.8% in the conterminous U.S. including lakes and ponds but not floodplains; Harvey and Schmadel, 2021), river corridors play a disproportionate role in landscape evolution and material transport, connecting uplands to the broader Earth system. River corridors facilitate exchanges of water and reactivity of biogenic elements between the atmosphere and geosphere and between channels and their surrounding hillslopes and floodplains, as well as longitudinal exchanges linking mountain tops to the ocean (Butman et al., 2018; Harvey et al., 2019; Wohl et al., 2019b). River corridors are far from being one-way pipes or “gutters” (sensu Leopold et al., 1964; Cole et al., 2007). Rather, river corridors can control the fluxes, forms, and ages of terrestrially derived materials (Fisher and Likens, 1973; Seitzinger et al., 2010; Blair and Aller, 2012), existing as both transformers and transporters (Kumar et al., 2018; Fazekas et al., 2021). This balance between long-distance connectivity and internal transformations yields a distinct environment that integrates inputs from spatially disparate locations over multiple timescales.
As part of the CZ, the primary controls on river corridor structure and function are geologic setting, climate, and human alteration and management (Amundson et al., 2007). Collectively, these forcings influence flow conditions and rates of material exchange and transformation in channels, floodplains, and riparian and hyporheic areas and interactions with adjacent landforms and groundwater systems (Bouwman et al., 2013). Depending on climate and geologic setting, river corridors may receive water discharging from groundwater systems or they may recharge groundwater (Fan et al., 2013; Famiglietti, 2014). In humid areas with shallow groundwater, the discharge of groundwater is a dominant source of streamflow (Jasechko et al., 2016) and a source of chemical constituents that mix with and react with surface water constituents in hyporheic and riparian flow paths (Gomez-Velez et al., 2015). In arid areas the surface flow through river corridors is often intermittent or ephemeral. When flowing, arid-zone rivers are often important in recharging groundwater (Famiglietti, 2014; Jasechko et al., 2021). Groundwater pumping for water supply has had major influences of groundwater-surface water interactions (Thaw et al., 2022; Van Loon et al., 2022), including groundwater interactions with rivers that draw modern groundwater deeper into aquifers.
River corridors differ fundamentally from upland environments, in the bidirectional linkages of terrestrial and aquatic processes that affect weathering and geomorphic change, including developing unique soils and sediments, hosting high rates of primary and secondary productivity and enhancing associated biogeochemical reactions (Aufdenkampe et al., 2011; Bernhardt et al., 2022). For example, soil and sediment in river corridors are a mixture of mineral and organic substrates derived from upland and upstream sources that are transported, mixed, stored, and transformed within the river corridor (Gooseff et al., 2002; Battin et al., 2009). Residence times are highly variable but can be long across broad alluvial plains, while biogeochemical pathways can oscillate between fully oxic and anoxic conditions as a function of flow, atmospheric gas exchange, and degree of soil saturation. These spatially and temporally dynamic environmental conditions generate a multi-scale and complex system that requires integrative science to achieve a predictive understanding (Hedin et al., 1998; Baker et al., 2000; Blaszczak et al., 2022). Through these processes, river corridors provide services and functions that benefit society (Banwart, 2011; Field et al., 2015) and many of the desirable functions associated with rivers depend on the presence of an integrated, fully functional river corridor (Table 1).
Here, we review past and present river corridor science, and reflect on opportunities to advance our predictive understanding of river corridors by embracing the CZ approach. First, we trace the evolution of river corridor science from a collection of seemingly disparate disciplinary topics to an integrated topic of study with clear parallels to the modern CZ perspective. We review how different communities of river, watershed, ecosystem, and ecological scientists have conceptualized river corridors during the past century and how these lines of thinking converge for a multidimensional view of river corridors (Sections 1.2, 1.3). Next, we contend that modern river corridor science is inherently integrative of processes within the entire CZ, and that a CZ perspective is necessary to advance our predictive understanding of river corridors. We review modern conceptual models of river corridors to assess their parallels with the CZ approach (Section 2) and identify both strengths and limitations of our existing understanding of the river corridor (Section 3). We contend that the river corridor is sufficiently important to be recognized as a landscape feature that contributes disproportionately to CZ structure and function and should be elevated as a scientific focal point for CZ science due to its integrative multi-scale nature. Put plainly, we propose a future scientific focus on the river corridor as part of CZ research. Finally, we assert that river corridors can be most effectively understood if research embraces integration across traditional disciplines and across diverse spatial and temporal scales. Critical Zone science has helped to redefine boundaries at the near-earth surface demonstrating how different compartments (e.g., the weathering zone, the biosphere) interact in both amplifying and suppressing various processes rates. River corridor science should maintain a framework that investigates interactions across boundaries opening the door for new discoveries. We identify four opportunities to advance our predictive understanding of river corridors (Section 4), specifically by embracing and integrating tenets of the CZ approach with our current understanding of these unique, ubiquitous landforms. We conclude with recommendations for developing a unified, multi-disciplinary framework that we believe can advance our predictive understanding of river corridors, enabling forecasting and proactive stewardship of Earth's natural resources.
1.2. A brief history of thinking about rivers
1.2.1. Earth science perspectives
People have recognized the fundamental process of downstream gravity-driven movement of rivers for millennia. Scattered historical writings attest to the understanding that flowing water carries sediment and shapes channels, floodplains, and deltas (Orme, 2013; Sack and Orme, 2013). Herodotus used the famous phrase “Egypt is the gift of the Nile” when writing of the Nile River delta and floodplain in the 4th century BC (Griffiths, 1966). Chinese philosopher Lao Tzu wrote of the erosion of rock by running water during the 5th or 6th century BC in the Tao Te Ching. By the 8th century AD, the Japanese were installing erosion control measures to limit sediment yield from hillslopes. Central Asian scholar al-Biruni related current strength to particle size in the Ganges River in writings circa 1000 AD. Leonardo da Vinci's notebooks from the late 15th century include measurements of velocity distribution in rivers and observations of fluvial sediment transport and deposition (Mays, 1996). Upstream movement by fish and other organisms was also recognized early on. Indigenous communities who relied on anadromous fisheries were aware of annual migrations and Izaak Walton describes the migrations of salmon in his 1,653 edition of The Compleat Angler.
The earliest conceptualizations of rivers included several fundamental components: downstream movement of material; lateral exchange between channels and floodplains; transported material deposited and stored in floodplains and deltas for variable lengths of time; up and downstream movement of organisms; and movement of materials from uplands into the river network and to the near coastal environment. While these might all seem like obvious points, they encapsulate nearly all of the physical exchanges (save surface-subsurface interactions) conceptualized in contemporary river science demonstrating a long history of recognizing the unique properties and functional attributes of river corridors.
These early descriptions of rivers corridors were not part of the systematic investigation of the natural world that began in earnest during the 18th century. James Hutton (1726–1797) was among the first to think about rivers in the context of geologic history. Hutton is credited with formulating the concept of uniformitarianism as a reaction to the catastrophist theories describing the formation and topography of Earth resulting from natural event such as Noah's flood or cometary bombardment early in the planet's history (Orme, 2013). Hutton inferred that more gradual processes, such as annual floods, acting over very long timespans, could shape river valleys (Playfair, 1802). His ideas continue to influence geological thinking.
Emphasis on reproducible experiments and quantitative description in natural sciences such as chemistry and physics influenced geologists and biologists working on rivers, late in the 19th century. Early physical conceptualizations crossed spatial and temporal scales focusing on adjustments among landscape features such as hillslopes and river channels that produce the relative stability described as equilibrium over timescales of thousands to millions of years (Gilbert, 1876, 1877), downstream decrease in the average size of sediment in the streambed (Sternberg, 1875), adjustments between base level and the river longitudinal profile (Powell, 1875), and progressive evolution of drainage basins over geological timespans (Davis, 1899). The dynamic stability embodied in Gilbert's ideas conflicted with the progressive change of Davis' conceptualization. Davis' ideas dominated geological thinking about rivers and drainage basins until the mid-20th century, but Gilbert's ideas underpin contemporary thinking about landscapes and rivers.
For the first half of the 20th century, river physical science focused on the mechanics of water and sediment movement, developing mathematical descriptions of hydrology, hydraulics, and sediment entrainment and transport (e.g., Shields, 1936). This mechanistic understanding formed the foundation for broader conceptualizations developed during the latter half of the 20th century that included:
• Conceptual (e.g., Fisk, 1947) and, subsequently, numerical (e.g., Allen, 1978; Bridge and Leeder, 1979) models of alluvial sediment architecture,
• The role of water (Leopold and Maddock, 1953) and of discharges of differing magnitude and frequency in transporting sediment (Wolman and Miller, 1960) and shaping channels (Wolman and Gerson, 1978),
• How adjustments among multiple variables create dynamically stable channel morphology, expressed as a balance (Lane, 1955), minimum variance (Langbein, 1964) and quasi-equilibrium (Langbein and Leopold, 1964),
• Developing state diagrams for channel planform based on variables such as discharge, channel gradient, and bank material sediment texture (Leopold and Wolman, 1957; Schumm, 1981; Montgomery and Buffington, 1997),
• Sediment (Hansen, 1970; Dietrich and Dunne, 1978) and water (Meade et al., 1990) budgets that identified and quantified inputs, outputs, and storage of material at spatial scales from a limited length of river corridor to the entire drainage basin,
• Storage time is inherent in the concept of a budget and led to quantification of floodplain residence time, storage time, and turnover time (Dietrich and Dunne, 1978),
• River metamorphosis—complete alteration of river morphology—in response to perturbations such as climate change or changes in land cover (Schumm, 1969),
• Internal and external thresholds that create nonlinear change and complex response in river corridors (Schumm, 1973),
• Landscape sensitivity, which is described as the transience or persistence of individual landforms relative to the magnitude and recurrence interval of disturbances that alter these landforms (Brunsden and Thornes, 1979),
• Catastrophe theory (Graf, 1979) as a mechanism for explaining rapid change in river morphology, and
• Spatial differentiation of dominant geomorphic processes and disturbance regimes within a river network (Schumm, 1977) and along the length of a river corridor (Montgomery, 1999).
Although physical scientists began to recognize the influence of woody vegetation on physical processes (e.g., Hack and Goodlett, 1960; Zimmermann et al., 1967), 19th and 20th century geomorphic and engineering conceptualizations of river corridors focused primarily on water and sediment fluxes interacting with the boundaries of the river corridor (channel banks, valley margins, base level). It was not until the very end of the 20th century that river conceptualizations developed by physical scientists began to explicitly include ideas from stream ecology including relationships between geomorphology and ecosystem services (Montgomery, 1999). The integration of concepts from stream ecology accelerated dramatically as physical scientists adapted hypotheses regarding connectivity (Bracken et al., 2013, 2015; Wohl et al., 2019a), resilience (Thoms et al., 2018), alternative states (Collins et al., 2012; Livers et al., 2018), and natural regimes (Wohl et al., 2015, 2019b). This interdisciplinary perspective resulted from the recognition that biotic influences on river corridor process and form equaled the influences of water and sediment (e.g., Castro and Thorne, 2019).
1.2.2. Ecological science perspectives
The conceptualization of the river corridor in the ecological sciences first emerged in the ideas of ecotones (Clements, 1905; Odum, 1953) later maturing in theories of functional interactions across terrestrial-aquatic interfaces (Forbes, 1887; Hynes, 1975; Swanson et al., 1982; Gregory et al., 1991; Naiman and Décamps, 1997). Ecotone (from the Greek words meaning home and tension) describes areas of sharp transition between ecosystems, typically with high species richness and that exist along steep environmental gradients. River corridor thinking from an ecological perspective was pioneered by Orghidan (1959) who described the hyporheos (from the Greek words beneath the flow) where stream water flows through the sediments and where aquatic insects shelter, reproduce, and take refuge, and where biogeochemical activity is high. Similarly, riparian is from the Latin word riparius meaning belonging to the bank of the river serving as a transition between the river channel and the drier uplands of a watershed. “Riparian” has been in use in legal and policy papers referring to water rights for a century and in the scientific literature for ~60 years (National Research Council, 2001).
Much of the formative thinking by aquatic ecologists came from working with, or drawing inspiration from, the science of other disciplines. The river continuum concept (Vannote et al., 1980) substantially influenced ecological thinking about river corridors by hypothesizing geomorphic controls on riparian processes and detrital-based food webs. For example, the river continuum concept leverages the downstream hydraulic geometric scaling of river channels (Leopold and Maddock, 1953) to posit that biotic communities and ecological processes vary predictably with river size. The flood pulse hypothesis (Junk et al., 1989) theorized biological subsidies to rivers arise from river flooding and interactions with floodplain vegetation, sediments, and biological communities. Ward and Stanford (1983) delineated interactions between serially arranged lotic and lentic waterbodies that structured aquatic communities in ways that would not be predicted by limnologists or river ecologists alone. From Ward's serial discontinuity concept came Stanford and Ward's (1988), Stanford and Ward (1993) four-dimensional concept of hydroecology, where longitudinal transport through the river network interacted with lateral floodplains and subsurface waters. Findlay (1995) and Tockner et al. (2000) focused on outcomes for river biogeochemical functions of hyporheic zones and floodplains, respectively. These examples highlight interactions between geomorphic and ecological processes that are central to the processes occurring throughout the river corridor.
The river corridor is now a unified concept of interactions among water, sediment, and living organisms including living riparian vegetation (e.g., Gurnell et al., 2016), diverse forms and functions of biofilm-forming microbial communities (e.g., Besemer, 2015; Battin et al., 2016; Hayer et al., 2021), as well as fallen large wood (e.g., Collins et al., 2012; Wohl et al., 2019b). Numerous examples of eco-hydraulic feedback interactions are found within the river corridor including: (i) flow drag around vegetation (Nepf, 1999) and feedbacks on flow depth, geomorphic change resulting from vegetation growth and organic sediment accumulation (Larsen et al., 2007); (ii) loss of transport capacity and accumulation of large wood on in-channel bars that promote vegetation establishment, wood deposition, and bar stabilization (e.g., Gurnell et al., 2005); (iii) construction of salmon redds that alter hydraulic conductivity and hyporheic exchange flows (Tonina and Buffington, 2009); and (iv) beaver dams that promote overbank flow and formation of a multithread channel planform, with associated reductions in hydraulic force during peak flows (John and Klein, 2004; Polvi and Wohl, 2013). Life history strategies of aquatic organisms have evolved with needs for micro-habitats in river corridors for feeding, nesting, and refugia (e.g., salmonids, Hassan et al., 2008; crayfish, Johnson et al., 2011). Terrestrial animals such as beavers (e.g., Burchsted et al., 2010; Polvi and Wohl, 2012) and aquatic animals such as salmon (Moore, 2006) are now recognized as ecosystem engineers of the physical and chemical processes in both aquatic and terrestrial environments.
The early advances described above tended to be conceptually rich but generally less quantitative, which limited applications. A critical advance was that of natural flow regimes (Poff et al., 1997), which provided evidence for how aquatic communities were adapted to flow frequency and that the integrity of stream and river ecosystem was intimately linked to the natural dynamics and heterogeneity of the system. This framework provided a quantitative structure to understand ecosystem-scale perturbation from dam construction, urbanization, and other disturbances. Dam construction, in particular, to expand water supply, control floods, improve navigation, and increase recreation has disrupted natural flow regimes threatening native river organism dispersal and biodiversity worldwide (Collier et al., 1996; Magilligan and Nislow, 2005; Graf, 2006; Poff et al., 2007, 2015). So influential was the natural flow regime concept that case studies sprung up worldwide and public-private partnerships put it into use in frameworks known as Indicators of Hydrologic Alteration (IHA; Richter et al., 1996) and Ecological Limits of Hydrologic Alteration (ELOHA; Arthington et al., 2006; Kendy et al., 2012). Doyle et al. (2005) proposed an effective ecological discharge concept that linked flow frequency with biologically relevant attributes to quantify the discharge at which specific ecological and biogeochemical processes functions are maximized including organic matter transport and nutrient retention.
1.2.3. Biogeochemical perspectives
River corridors provide spatial and temporal heterogeneity which support a myriad of biogeochemical reactions, many of which have consequences for atmospheric pollution and the elemental stoichiometry of ground and surface water chemistry. River corridor biogeochemistry provides a framework to integrate terrestrial and aquatic biogeochemical perspectives (Grimm et al., 2003; Bianchi, 2021), which are often siloed. Although not necessarily formalized by name, there is a rich history of research that aims to understand biogeochemical processes within the river corridor. It is important to recognize that river corridor biogeochemistry is similar yet unique from the whole-ecosystem approach as articulated by Likens and Bormann (1974) developed through the paired-watershed approach at Hubbard Brook Experimental Forest. Here terrestrial and aquatic processes are integrated through a budgetary approach and the study of surface water chemistry to understand watershed-scale vectors of material flux (i.e., atmospheric, biologic, geologic). What may be more akin to river corridor biogeochemistry is research that emphasizes links between the riparian zone (Naiman and Décamps, 1997), the stream channel (Likens and Buso, 2006), and the hyporheic zone (Hynes, 1983). Collectively, these areas are often referred to as the terrestrial-aquatic interface (McClain et al., 2003). Much of the associated work has focused on the biogeochemical controls of inorganic nitrogen and rates of denitrification due to the prepotency of anoxia in riparian zones and the implications for water quality and nitrous oxide production (e.g., Hynes, 1975; Peterjohn and Correll, 1984; Vidon and Hill, 2004; Hille et al., 2014).
One early unifying biogeochemical concept was that of hot spots and hot-moments (HSHM; McClain et al., 2003). The HSHM conceptual model attempts to account for patches within the landscape and relatively short periods of time that have high biogeochemical reaction rates and thus disproportionate influence on material flux. HSHMs are typically enhanced at terrestrial-aquatic interfaces such as riparian zones where redox conditions often fluctuate due to cycles of wetting and drying. This model was expanded upon with the “ecosystem control points” framework (Bernhardt et al., 2017), which focuses on gradients of biogeochemical activity by explicitly incorporating hydrological connectivity across timescales. The ecosystem control point framework identifies four unique mechanisms (permanent control points, activated control points, export control points, and transport control points) that are operating within river corridors. Permanent control points, for example, are defined as a point on the landscape where the continuous delivery of reactants and appropriate environmental conditions come together to support sustained high rates of biogeochemical reactions. Riparian and hyporheic zones are two examples within river corridors that commonly meet the criteria of permanent control points (Peterjohn and Correll, 1984; Triska et al., 1993; Hedin et al., 1998). These interfaces across the landscape where flow paths (and their dissolved solutes) converge, create opportunities for key biogeochemical reactions (Wymore et al., 2021a).
A significant body of research has also explored biogeochemical reactions within the stream channel, demonstrating the biogeochemical power of streams (Peterson et al., 2001; Mulholland et al., 2008)—especially smaller order streams that are tightly linked to the landscape (Bernhardt et al., 2022). The concept of nutrient spiraling (Webster, 1975; Newbold et al., 1982; Stream Solute Workshop, 1990; Ensign and Doyle, 2006) offered a unifying concept for the unique biogeochemical processes occurring in flowing environments, supporting paradigm shifts away from streams and rivers as pipes for water and sediment (Leopold et al., 1964) to biologically active ecosystems (Meyer and Likens, 1979). Biogeochemical processes occurring within the stream channel are now recognized to have global biogeochemical consequences (Cole et al., 2007; Hotchkiss et al., 2015; Yao et al., 2020) contributing to the overall functional significance of the river corridor.
Biogeochemical reaction rates are also modulated by microbial communities which can be highly responsive to changing environmental conditions. While no explicit framework exists to place microbes in the river corridor, considerable work has characterized microbial communities within the different compartments of the landscape connecting their composition and diversity to biogeochemical cycles with the river corridor. Microbial communities with the stream channel reflect interactions across the terrestrial-aquatic interface suggesting that the terrestrial landscape provides microbial subsidies to the aquatic environment (URycki et al., 2020) which are able to actively grow and replicate. For example, terrestrial phyllosphere fungal communities actively incorporate heavy oxygen isotopes (18O) during leaf litter decomposition (Hayer et al., 2021). The composition, diversity, and activities of these microbial communities is directly related to both climate and geomorphology and variation in land use and land cover with stronger relationships found in headwater streams relative to downstream sites (Read et al., 2015; Savio et al., 2015; URycki et al., 2020) likely reflecting the stronger connectivity between smaller-order streams and their surrounding watershed. Building correlative relationships between microbial community composition and biogeochemical reactions and ecosystem services remains a challenge due to the sheer diversity of operational taxonomic units (OTUs) that has been revealed by advances in DNA sequencing. Many environmental samples include non-actively growing taxa (Jones and Lennon, 2010) which contribute to low signal to noise ratios. As such, methods that can identify the actively growing community (e.g., stable isotope probing; Hayer et al., 2021) will help reveal the various ways in which microbial communities drive key biogeochemical reactions and respond to environmental gradients. Such data are highly valuable for hydrologic models including reactive transport simulations which can incorporate microbiological, geochemical, and CZ structural data to predict hydro-biogeochemical responses (e.g., Li et al., 2017, 2021; Rubinstein et al., 2022) within the river corridor.
1.2.4. Anthropogenic perspectives
Although conceptual models of river corridors are beginning to explicitly include human effects, we argue that most river corridor conceptual models have not effectively incorporated human influences on physical, biological, and biogeochemical aspects of rivers, despite the ubiquity of the human footprint on ecosystems (Vitousek et al., 1997; Galloway et al., 2004). Nor do economic valuations of rivers and water bodies fully account for their valued function. Here, we briefly review how human activities have affected contemporary river corridors.
Human influences on river corridors include upland activities such as changes in land cover and topography (e.g., Syvitski et al., 2005; Walling, 2008), as well as warming climate (Palmer et al., 2008). In most regions of the world, human influences on river corridors start with deforestation in connection with agriculture, an alteration in land cover that began thousands of years ago (e.g., Van Andel et al., 1990; Mei-e and Xianmo, 1994; Macklin, 1999). Some indigenous societies modified land cover and a smaller number actively modified river corridors for food production (crops or fishing, e.g., Doolittle, 1990; Tagseth, 2008), but the alteration of upland environments and river corridors typically accelerated following either the development of agriculture capable of supporting population concentrations in large cities or colonization by Europeans in the Americas, Australia and New Zealand, and elsewhere. Land cover was commonly altered earlier than river corridors (e.g., Wohl et al., 2017), but rivers have been altered for more than two centuries in the temperate latitudes to facilitate navigation, flood control, and waste disposal (e.g., James and Marcus, 2006; Brown et al., 2018). More contemporary human activities within river corridors include placer mining (James, 1991), gravel mining (Koehnken et al., 2020), riparian deforestation, beaver trapping (Brazier et al., 2021; Harvey and Schmadel, 2021; Wohl, 2021b), log floating (Tornlund and Ostlund, 2002), removal of large wood (Montgomery et al., 2003), flow regulation via dams and diversions (Grill et al., 2015), construction of artificial levees (Knox et al., 2022), channelization and bank stabilization (Mattingly et al., 1993), deliberate or accidental introduction of exotic species (Birken and Cooper, 2006), and floodplain drainage (Roley et al., 2012).
The net effect of human activities within the river corridor has been the simplification and homogenization of river corridor morphology, downstream fluxes of material, and biotic communities. Channelization in response to enhanced sediment yields (Knox, 2001) and extirpation of beavers during colonization of the U.S. are useful examples (Wohl, 2021b). Similar trends have been documented globally to regionally for morphology (Wohl, 2014; Peipoch et al., 2015; Wohl et al., 2021), flow regimes (Poff et al., 2007), sediment regimes (Wohl et al., 2015), nutrient stoichiometry (Wymore et al., 2021b), and riverine biota (Rahel, 2002; Petsch, 2016). The phrase “legacy effects” now typically refers to historical human activities that may have ceased more than a century earlier, but continue to influence channel morphology, sediment dynamics, and associated transport and fate of contaminants and thus river corridor morphology (e.g., Walter and Merritts, 2008; James, 2013; Wohl, 2015, 2019; Harvey et al., 2019). The long history of river alteration in much of the world may not equate to contemporary recognition of this history if the human activities are no longer occurring and river form and function have been altered for decades to centuries (e.g., Wohl and Merritts, 2007; Walter and Merritts, 2008). This lack of recognition can lead to contemporary underestimation of the influences of humans on any particular river corridor or on rivers around the world (Wohl, 2013).
2. Contemporary river corridor concepts and models
From a rich history of discipline-specific concepts and models, river corridor science has evolved toward more interdisciplinary approaches and increasingly direct engagement with humans and our management of river corridors (Figure 2). Here, we briefly summarize some of the integrative and widely applied concepts in recent river corridor research.
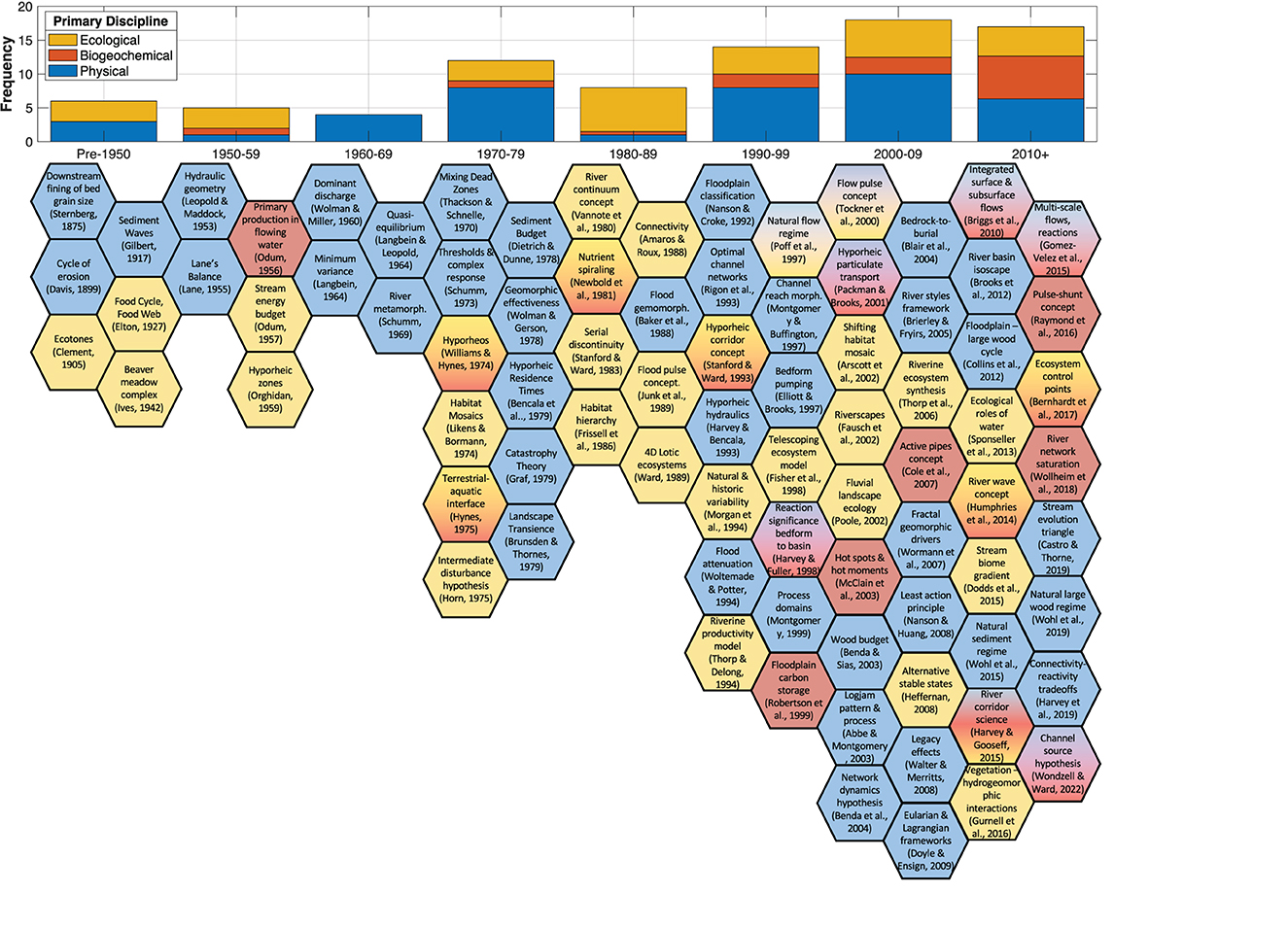
Figure 2. Key conceptual advances in river corridor science through time from Ward et al. (2023). Colors represent the predominance of physical (blue), biogeochemical (red), and/or ecological (yellow) motivations and applications in each study. Papers represented in this figure include: Sternberg, 1875; Davis, 1899; Clements, 1905; Gilbert, 1917; Elton, 1927; Ives, 1942; Leopold and Maddock, 1953; Lane, 1955; Odum, 1956, 1957; Orghidan, 1959; Wolman and Miller, 1960; Langbein, 1964; Langbein and Leopold, 1964; Schumm, 1969, 1973; Thackston and Schnelle, 1970; Likens and Bormann, 1974; Williams and Hynes, 1974; Horn, 1975; Hynes, 1975; Dietrich and Dunne, 1978; Wolman and Gerson, 1978; Brunsden and Thornes, 1979; Graf, 1979; Vannote et al., 1980; Newbold et al., 1982; Ward and Stanford, 1983; Bencala et al., 1984; Frissell et al., 1986; Amoros and Roux, 1988; Baker et al., 1988; Junk et al., 1989; Ward, 1989; Nanson and Croke, 1992; Harvey and Bencala, 1993; Rigon et al., 1993; Morgan et al., 1994; Thorp and Delong, 1994; Woltemade and Potter, 1994; Elliott and Brooks, 1997; Montgomery and Buffington, 1997; Poff et al., 1997; Fisher et al., 1998; Harvey and Fuller, 1998; Montgomery, 1999; Robertson et al., 1999; Tockner et al., 2000; Packman et al., 2001; Arscott et al., 2002; Fausch et al., 2002; Poole, 2002; Abbe and Montgomery, 2003; Benda and Sias, 2003; McClain et al., 2003; Benda et al., 2004; Blair et al., 2004; Brierley and Fryirs, 2005; Thorp et al., 2006; Cole et al., 2007; Worman and Wachniew, 2007; Heffernan, 2008; Nanson and Huang, 2008; Walter and Merritts, 2008; Doyle and Ensign, 2009; Brooks et al., 2012; Collins et al., 2012; Sponseller et al., 2013; Humphries et al., 2014; Briggs et al., 2015; Dodds et al., 2015; Gomez-Velez et al., 2015; Harvey and Gooseff, 2015; Wohl et al., 2015, 2019a,b; Gurnell et al., 2016; Raymond et al., 2016; Bernhardt et al., 2017; Wollheim et al., 2018; Castro and Thorne, 2019; Wondzell and Ward, 2022.
2.1. Disturbance regimes and stability
River corridors experience a myriad of spatially and temporally variable disturbance regimes. With the above section describing human alterations of river corridors, we focus this section on “natural” disturbance regimes while acknowledging the far reach of human-made global changes in modulating many forms of disturbance (e.g., extreme event frequency). Floods and runoff events have been recognized for decades as a form of disturbance with geomorphic consequences for the river corridor (Wolman and Gerson, 1978). Runoff events reshape the landscape (e.g., channel widening) with recurrence intervals and recovery times that vary spatially as a function of regional state factors (sensu stricto Jenny, 1941). Many of these early flood-based analyses focused on sediment mobilization (e.g., Wolman and Miller, 1960), erosion, and floodplain evolution (e.g., Knox, 1972). Interactions, however, between flood-based disturbances and other processes operating at various spatial and temporal scales (e.g., large wood and riparian vegetation) ultimately determine the functioning and resilience of the river corridor as a holistic system.
Much of the conceptualization of disturbance that extends beyond precipitation-runoff events has origins in the ecological literature (Brookhaven Symposium on Biology, 1969; Connell, 1978; White and Pickett, 1985) including work specific to lotic ecosystems (Resh et al., 1988; Lake, 2000). The ecological literature has contributed significantly to the closely related concepts of resilience (Lewontin, 1969; Holling, 1973) and alternative stable states (Holling, 1973; May, 1977) to characterize the uncertainty and nonlinear dynamics of complex systems. Emerging from this literature are quantitative approaches to assess resilience and tipping points (Scheffer et al., 2001; Carpenter et al., 2006; Hillebrand et al., 2017; Hillebrand and Kunze, 2020). Many of these concepts apply to the investigation of the river corridor. A unique challenge, however, to characterizing disturbance within the river corridor is integrating across the spatial and temporal timescales of disturbance processes in river corridors. For example, Brunsden and Thornes (1979) examined landscape sensitivity in the context of persistent landforms, which remain present for longer than the recurrence interval of the disturbance that created them (e.g., secondary channels created by a large flood), and transient landforms that are significantly modified by subsequent smaller flows at shorter time spans than the recurrence interval of the flood that originally created them.
Concepts of resilience, sensitivity, and resistance are common to physical and ecological understanding of river corridors (e.g., Fryirs, 2017; Parsons and Thoms, 2018). While definitions can vary across subdisciplines, each of these concepts addresses the amount of change a system can undergo and remain within the same regime or at a certain equilibrium (Walker and Salt, 2012). These concepts also include the response and recovery time of the system to disturbance.
The quantification of a disturbance event is challenging within the river corridor. For example, following storm events, solute concentrations can remain elevated even though flows have returned to baseline (e.g., Baker and Showers, 2019). This separation of the hydrograph vs. the chemograph in response to disturbance occurs with both smaller storms (Scholl et al., 2015; Baker and Showers, 2019) as well as major disturbance events including hurricanes (McDowell et al., 2013) and challenges our notion of how we determine the start and end of disturbance events due to the integrative nature of the river corridor reflecting multiple time scales and processes. And while some forms of disturbance return to baseline at some scale, other forms of disturbance result in more permanent change to the geomorphology of the river corridor (e.g., avulsions; Jones and Schumm, 1999). Regardless of outcome (e.g., ephemeral changes in discharge or longer-term changes in channel location), both forms of disturbance have implications for the mobilization and distribution of solutes and sediments throughout the river corridor.
Disturbances can push river corridors across stability thresholds and into novel alternative steady states, as for example has been argued for in vast Everglades floodplain of south Florida, USA (Zweig and Kitchens, 2010). Disturbances such as dramatic changes in runoff interact with other physical, chemical, and ecological processes across hierarchical levels of biological organization. For example, changes in discharge influence organic matter retention (Snaddon et al., 1992) and the fragmentation of leaf litter (Gessner and Chauvet, 1994) with implications for heterotrophic metabolism in light-limited corridors. Variation in runoff impacts algal productivity, predation, and food chain length (Marks et al., 2000), the stoichiometry of energy (carbon) and nutrients (nitrogen) delivered to aquatic ecosystems (Wymore et al., 2021a), the spatial and temporal partitioning of biogeochemical reaction rates and metabolism (i.e., pulse-shunt concept; Raymond et al., 2016) and the maintenance of biodiversity (Junk and Wantzen, 2006) by influencing landscape heterogeneity (Tockner et al., 2000). The spatial and temporal variability captured by these various physical, chemical and biological processes points to the multiple ways in which different conceptualizations of the river corridor interact and the dynamics of river corridor disturbance and resilience.
The natural range of variability, sometimes described as the historical range of variability (Morgan et al., 1994), can be defined as the ecological conditions experienced by the system at various spatial and temporal scales. This range of variability is key to defining an envelope of stability and when systems may be approaching tipping points to alternative and novel equilibria. The natural range of variability recognizes that (i) natural systems such as river corridors vary through time and space in response to varying inputs and boundary conditions, but (ii) human influences can change these variations beyond this range. For example, hurricanes can lead to elevated concentrations of nitrate due to organic matter decomposition and reduced plant uptake and these pulses can be sustained over consecutive years before returning to baseline (Schaefer et al., 2000). Clearing native land cover in a watershed commonly causes significant increases in sediment yield to a river corridor (Walling, 2008), for example, that can exceed the variability in the natural sediment regime (Wohl et al., 2015), or commercial agriculture can result in greater nitrate inputs to a river network than would occur under natural “background” conditions (Raymond et al., 2012).
Variability in physical and biological drivers can alter the ecological state of river ecosystems (e.g., Wolf et al., 2007; Collins et al., 2012; Livers et al., 2018; Dutton et al., 2021; Zipper et al., 2022). Often there are alternative geomorphic and biological states that are possible with unique biotic and abiotic conditions that are non-transitory over ecologically relevant timescales (Holling, 1973). An example are the narrow, anastomosing, and fast-flowing channels of semi-arid rivers that may be present if floods regularly remove aquatic vegetation that can transition to wider slower flowing channels if emergent and flood resistant vegetation takes hold (Heffernan, 2008). This definition overlaps with the concept of river metamorphosis or the change in river corridor morphology (Schumm, 1969). Sometimes, novel or hybrid ecosystems emerge from historical states by unprecedented abiotic drivers or change in species composition, or both (Francis, 2014).
One increasingly relevant form of disturbance is river corridor drought, including examples in relatively humid northern temperate and tropical latitudes (Clark et al., 2017; Gómez-Gener et al., 2020; Gutiérrez-Fonseca et al., 2020). In these systems which receive consistent precipitation through the year, drought is an emerging and relatively novel form of disturbance compared to drought in more xeric systems. In general, the effects of more intense precipitation and increased runoff have been better studied than the effects of drought. Part of this disparity comes from the fact that drought conditions are harder to identify and often only recognized long after they begin (Lake, 2003, 2011; Boulton and Lake, 2008). Drought influences on multiple river processes are receiving more attention (e.g., on anadromous fisheries; Woelfle-Erskine et al., 2017), demonstrating that longitudinal and lateral connectivity throughout the river corridor is affected.
Another increasingly relevant form of disturbance is wildfire (Jolly et al., 2015). Wildfire creates substantial changes in upland and river corridor fluxes of water, solutes, sediment, and organic matter, and associated changes in river corridor form and function (e.g., Hatchett et al., 2022; Roebuck et al., 2022; Shuman et al., 2022). Changes in the spatial extent, frequency, and severity of wildfire may push river corridors across thresholds into alternative states.
Stationarity is often used as a unifying concept to understand stability in river corridor science. Stationarity encapsulates natural fluctuations within an unchanging envelope of variability. For example, a variable such as annual flood peak has a time-invariant (or 1-year-periodic) probability density function whose properties can be estimated from the systematic record of stream flow (Milly et al., 2008). Commonly applied to river corridors in the context of hydrology, stationarity can be equated to the natural range of variability. Human alterations of river corridors, watersheds, and the global climate system have significantly increased variability in natural systems, creating nonstationarity that limits our ability to predict future conditions and river corridor response to disturbances (e.g., Habersack et al., 2014). Investigations of spatial and temporal variations in inputs, boundary conditions, and the resilience and stability of river corridor structure and function remain central to nearly all aspects of river corridor science.
2.2. Connectivity
Connectivity describes the degree to which matter and organisms can move among spatially defined components in a system. Components could include a (sub)-watershed, with uplands and river corridors as components (e.g., Fryirs et al., 2007; Bracken et al., 2013) or floodplains, active channels, hyporheic zones, and groundwater aquifers (Pringle, 2003; Rice, 2017). Descriptions of connectivity can also focus on different forms of matter (e.g., water, solutes, sediment, organic matter, microbes, fish, insects) emphasizing the connectivity across scales. Explicit characterization of connectivity, which occurs along a continuum from fully connected to disconnected, provides a useful conceptual framing for investigating the spatial and temporal variability of fluxes by directing attention to (i) interactions among components that may appear to be isolated in time and space, (ii) the response of diverse systems to varying inputs, (iii) the specific features of systems that govern connectivity, and (iv) how human alterations of natural systems influence system behavior (Wohl et al., 2019a). Consequently, physical, biological, and biogeochemical investigations of river corridors commonly employ connectivity as a conceptual framework.
Downstream flow of water and the apparent downstream changes in channel characteristics and associated riparian and aquatic communities emphasize a continuum of longitudinal changes. Examples include downstream decrease in grain size (Sternberg, 1875), changes in channel width and depth (Leopold and Maddock, 1953), and the shape of river longitudinal profiles (Yatsu, 1955), as well as changes in structural and functional attributes of lotic communities (Vannote et al., 1980). Conceptualization of a longitudinal continuum of change fosters attention to patterns at spatial scales of a river network or whole river, but noise (scatter about a trend) typically exists in these patterns.
Foundational conceptualizations of connectivity in river corridors focus on the three-dimensional nature of connectivity (longitudinal, lateral, vertical; Ward, 1989) and these conceptualizations underlie models such as the flow-pulse (Tockner et al., 2000), flood-pulse (Junk et al., 1989) or pulse-shunt (Raymond et al., 2016). This leads to an alternative conceptualization that focuses on spatial and temporal discontinuities in patterns of physical characteristics, disturbance regimes, and biotic communities within river corridors (e.g., Ward and Stanford, 1995; Montgomery, 1999; Poole, 2002). Conceptualizing a river corridor as exhibiting either continuous longitudinal trends or discontinuities (or both, simultaneously) is useful and both frameworks remain widely used (e.g., Fullerton et al., 2015; Tomscha et al., 2017; Wohl et al., 2018; Harvey et al., 2019). Longitudinal discontinuities are often associated with the lateral exchanges with off-channel areas (Ward, 1989; Stanford and Ward, 1993) that provide for efficient processing of carbon and nutrients as well as habitat where aquatic organisms feed, or are reared (Poole et al., 2006; Thorp et al., 2006). Lateral connectivity provides areas where organisms take refuge during floods, as well as microbial and macroinvertebrate habitat that influences biogeochemical processes and water quality (Junk et al., 1989; Tockner et al., 2000). The spatial and temporal dynamics of the river corridor are fundamentally a result of connectivity and discontinuities along longitudinal, lateral, and vertical dimensions and provide a framework for how anthropogenic forcing impacts the functioning of the river corridor (Ward and Stanford, 1983, 1995).
Both longitudinal and lateral aspects of connectivity can be quantified by weighing their relative strengths in a dimensionless metric that accounts for their influence on biogeochemical reactions (Harvey et al., 2019). For multi-thread channels through wetlands and floodplains, Larsen et al. (2012) quantified connectivity by drawing from graph theory and hydrogeology that quantified effective permeability through river corridors with complex anabranching channels interacting with patchy ridges of floodplain at higher elevation and with denser vegetation (Larsen et al., 2012). Brooks et al. (2022) used naturally occurring geochemical and microbial tracers to measure hydrologic connectivity in river-floodplain systems. From all of these efforts emerged broadly applicable model frameworks for hydrologic exchange flows and material exchanges between physical, chemical, and biological compartments in river corridors (Covino, 2017). Connectivity has played an important role in building scientific support for changes in U.S. regulatory policy protecting headwaters (e.g., Alexander et al., 2018).
2.3. Hydrochemical exchange flows
Hydrologic exchange flows (HEFs) are the lateral and vertical flows of water that exchange main channel water and constituents with off-channel areas and subsurface waters where chemical reactivity and biological uptake are enhanced (Harvey, 2016). HEF studies aim to understand water storage, water purification, and ecological functions of river corridor units ranging from individual bedforms to whole river basins. Stanford and Ward (1988, 1993) offered conceptual models of the exchange pathways and their associated functions. Quantification of flows and associated fluxes is often completed with tracer releases to track water and chemical mass balances and timescales, which also provides an empirical basis for quantifying river “dead zones” (Thackston and Schnelle, 1970) and “subsurface exchange” processes (Bencala et al., 1984). The storage processes not only involve physical exchanges of water but also biotic uptake and release of nutrients and other biogeochemical transformations measurable by tracer additions of reactive compounds to rivers, e.g., nutrients, metals, fine particulate matter analogs (Ensign and Doyle, 2006). Those tracer tests were modeled with generalized forms of transport and storage-reaction models referred to as “spiraling” equations (Newbold et al., 1982; Stream Solute Workshop, 1990; Runkel, 2007).
Although powerful, spiraling concepts and modeling offer an inherently empirical description of storage and reaction that lacks a basis in fundamental process models and are therefore difficult to transfer between rivers. To address that need, physical models of HEFs were developed for components of the river spectrum, including sides eddies and cavities (Jackson et al., 2013), bank storage (Gerecht et al., 2011; Welch et al., 2014), overbank floodplain flows (Bates et al., 1997; Scott et al., 2019), hyporheic flow interacting with hillslope groundwater (Harvey et al., 1996; Wondzell et al., 2009), and river bedform pumping (Savant et al., 1987; Cardenas and Wilson, 2007). Those models reduced the dependence on empirical, tracer-based approaches with its spatial and temporal constraints (e.g., Becker et al., 2023), and opened the door to coupling between fluvial geomorphic, hydraulic, hydrogeologic, and biogeochemical models at multiple scales (see review by Boano et al., 2014).
Modeling HEFs has helped focus questions about the dominant geomorphic and hydraulic controls on biogeochemical reactions. The early tests have moved toward more fully realized basin-scale modeling of HEF impacts on broadly important reactions such as nutrient cycling, progressing from reach-scale work (Harvey and Fuller, 1998; Wondzell and Swanson, 1999; Conant et al., 2004) to the basin to regional modeling (Chen et al., 2020; Schmadel et al., 2020, 2021; Dwivedi et al., 2022). Contemporary models of HEFs combine hydraulic and biogeochemical variables in models that are driven by increasingly available measurements of discharge, riverbed slope, sinuosity, grain size distribution, and sizes and shapes of river bars, banks, and bedforms. A new emphasis is being placed on integrating spatially and temporarily dynamic HEFs across a broad spectrum of variability to assess dominant processes. Multi-scale models combine surface and subsurface contributions of pools and side cavities (Stewart et al., 2011), with exchanges with stream bedforms, bars, and banks (Gomez-Velez and Harvey, 2014), along with overbank exchanges with riparian and floodplain areas (Scott et al., 2019). This multi-scale multi-compartment perspective facilitates an understanding of dominant drivers, cumulative effects, and downstream consequences of biogeochemical processing (Gomez-Velez et al., 2015; Bernhardt et al., 2017; Fang et al., 2020). HEFs concepts and models are also providing approaches to evaluate the effectiveness of management strategies (e.g., National Research Council, 2001; Hester and Gooseff, 2010). For example, new styles of “design with nature” engineering of HEFs are poised to improve water purification functions in urban streams (Herzog et al., 2015, 2019). From those and similar efforts are emerging scientifically based strategies to anticipate how rivers functions are changing and how to adapt to protect and restore valuable river corridor functions in a changing climate (Jones and Stanley, 2016; Ward et al., 2018, 2020).
2.4. Legacy effects of humans
The phrase “legacy effects” is now widely used to describe the continuing influence of past human activities. Investigators have tended to assume that a watershed or river corridor with no obvious or contemporary human alterations (e.g., native land cover or no contemporary dams) reflects a lack of human influence. Multiple studies, however, indicate that even human activities that ceased more than a century prior to the study continue to influence river corridor morphology and stratigraphy, dynamics of water and sediment within the river corridor, three-dimensional connectivity, biotic community composition, and resilience (e.g., Harding et al., 1998; Walter and Merritts, 2008; Wang et al., 2013; Ruffing et al., 2015; Wohl, 2019). Given the ubiquity and long history of human alteration in many of the world's watersheds, as well as the tendency of river corridors to integrate and record fluxes from across the entire watershed and the atmosphere (Wohl, 2020), riverine scientists are coming to understand that the existence of human alteration should be the default assumption for most of the world's river systems (Wohl, 2013; Burgi et al., 2017).
Constructed ponds and reservoirs in river corridors may shorten the frequency of low flows and intermittent flow in streams, although low-flow responses are variable and depend on hydroclimatic condition and management style (Eng et al., 2013). To understand legacies associated with constructed ponds and reservoirs, Harvey and Schmadel (2021) compared river corridor surface area before and after beaver extirpation and with present-day with construction of urban stormwater retention and agricultural impoundments. The result has been large swings in river corridor surface area and residence times resulting from lentic area changes associated with biological extirpations and anthropogenic modifications of river corridors. In addition to lengthening transit times (Harvey and Schmadel, 2021), lentic waters moderate downstream flow variability in river corridors by lowering the flow peaks exacerbated by impervious surfaces and piped and tiled drainage (Graf, 2006). There have been concomitant increases in the retention of sediment and contaminants (Villarreal et al., 2004; Syvitski et al., 2005; Liu et al., 2014).
Contemporary river management, including restoration, now commonly emphasizes returning river corridors to a more functional condition. Restoration activities span a broad range of temporal and spatial scales and techniques, from managing land cover in the watershed (Qi et al., 2019) and using controlled burns in an attempt to mitigate problems caused by wildfire regimes altered by decades of fire suppression (Eisenberg et al., 2019), to directly reconfiguring the active channel at the reach scale (e.g., Tullos et al., 2009). Many restoration projects focus on physical form with the expectation that processes and functions such as improved water quality or biomass and biodiversity will follow (Sudduth et al., 2011). This assumption is not always fulfilled, in part because other constraints such as limited connectivity or persistent contaminants limit chemical and biological responses (Lepori et al., 2005; Palmer et al., 2010). We contend that more explicit integration of river corridor conceptual models in the context of human-perturbations will improve our ability to understand and predict river corridor responses to management.
3. Existing conceptual models fail to represent all tenets of the critical zone perspective
CZ science advances approaches that are multi-scale, interdisciplinary, and allow for coupling physical, chemical, and biological dynamics in response to forcings or perturbations (Anderson et al., 2007; Brantley et al., 2007; White et al., 2015; Sullivan et al., 2019). For example, the application of multiple isotopes led to the development of complementary hypotheses that explain interactions among a diversity of CZ processes, representing multiple timescales, that drive landscape evolution and pathways of elemental mass loss (Sullivan et al., 2016). To gain a predictive understanding of river corridor function, approaches are needed that can represent key processes and couplings that control functions of interest. Model frameworks must be flexible (e.g., scalable and transferable) to inform management decisions on functions that result from the unique combinations of forcings, historical trajectories, and coupled process dynamics that characterize river corridors (Ward et al., 2022). To assess the degree to which existing conceptualizations of river corridors are aligned with the tenets of CZ science, we evaluated 38 modern conceptualizations of river corridors (Figure 3; see Ward et al., 2023). From this review, we found common themes missed by river corridor models but found more frequently in models of the CZ including an absence of nested processes at the microscale and the lack of provisioning services. Below we highlight some of the major take-away messages from our analysis of river corridor models.
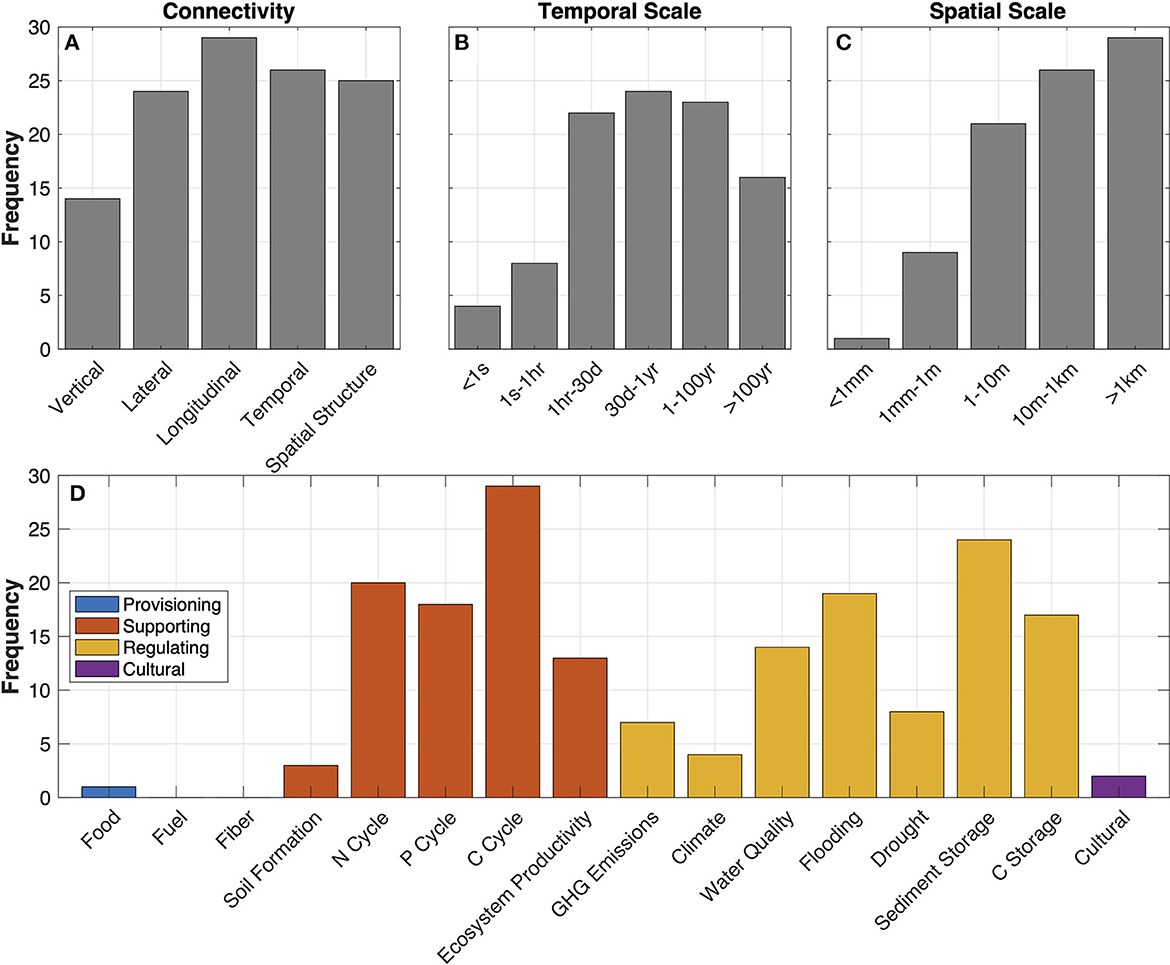
Figure 3. Summary of 38 modern conceptual models of the river corridor, including their dimensions of connectivity (A), spatial scale(s) (B), and temporal scale(s) (C). The critical zone services and functions in each study (after Field et al., 2015) were also tabulated for each conceptual model (D).
3.1. River corridor analysis of network-scale patterns has seldom included nested processes at smaller spatial and shorter temporal scales
Representing the dynamic connectivity of the lateral and vertical exchange flows that are nested within river corridor longitudinal transport is essential to understanding CZ functions and services (Harvey, 2016). Overall, we found the 16% of conceptual models (six of 38) include all four dimensions of connectivity (lateral, vertical, longitudinal, temporal) and spatial structure, while another 8% (three of 38) include all three spatial dimensions and temporal variation (Figure 3A). Put another way, more than 76% of conceptual models lack at least one key dimension of connectivity. Of the dimensions of connectivity that have been studied, more than 68% include temporal variation and more than 66% include broader spatial structure, indicating that much attention has been paid to larger-scale patterns along river corridors. In contrast, <37% of conceptual models consider vertical connectivity, likely owing to its inherently smaller spatial scale relative to apparent patterns at the scale of entire networks.
A key element to achieving predictive understanding of the river corridor is the integration of the temporal (Figure 3B) and spatial scales (Figure 3C) required to reflect the river corridor (Boano et al., 2014; Gomez-Velez and Harvey, 2014; Ward and Packman, 2019; Krause et al., 2022). Representing multiple dimensions of space and time is a defining characteristic of the CZ science approach (Brantley et al., 2017). Spatial scales included in conceptual models are heavily biased toward the larger scales of study (10s to 1,000s of kilometers), with few examples that are “telescoping” (Fisher et al., 1998) and include underlying, smaller-scale processes at 10s to 100s of meters. Only one conceptual model from our review includes sub-mm scales despite a clear recognition of the role of microbial processes in a host of biogeochemical functions of river corridors (Briggs et al., 2015).
Multi-scale hydrologic processes operate across scales through connections between drivers and outcomes at different scales (Clark et al., 2015). In river corridors, multi-scale processes connect process drivers at the scale of geomorphic units with outcomes for the larger river network. Feedbacks to the local scale are driven by larger-scale processes such as wildfire or sediment depletion or oversupply responding to climate variability, land use change, or dam building or removal. There is a relative dearth of studies that link multiple spatial scales, despite a recognition that smaller-scale processes aggregate to make network-scale predictions (Heffernan et al., 2014). At present, the available multi-scale models typically focus on time-averaged behaviors (Gomez-Velez et al., 2015; Schmadel et al., 2019), and therefore lack the resolution to explain the larger-scale influences of temporally dynamic processes (Harvey et al., 2019; Chen et al., 2020). This is despite a clear understanding that storm events can have disproportionate influence on the transformation and transportation of solutes in the river corridor (Hilton et al., 2008; Raymond and Saiers, 2010). As a result, our understanding of river corridor function has been overwhelmingly dominated by longer time-averaged dynamics, although progress toward temporally dynamic, multi-scale models is being made (Fang et al., 2020; Schmadel et al., 2021).
3.2. River corridor models do not integrate all the needed critical zone services and functions
Critical zone services broadly include provisioning services (food, fuel, fiber), supporting and habitat services (soil formation, N/P/C cycles, primary productivity, flow and depth variability), regulating services (greenhouse gas emissions, climate, water quality, flooding, drought, sediment storage, carbon storage), and cultural services (water supply, recreation, viewsheds, land values) (Field et al., 2015; Richardson and Kumar, 2017; Lee et al., 2023). Considered broader than ecosystem services in both space and time dimensions, CZ services are susceptible to changes in CZ structure and function and disturbance (Lee et al., 2023). Provisioning services are mostly absent from existing conceptual models (Figure 3D), with only one model explicitly addressing food provisioning (Kumar et al., 2018) and none with fuel or fiber provisioning. Similarly, only two studies include any mention of cultural services (Poff et al., 1997; Brierley and Fryirs, 2005). This disparity is perhaps surprising given the rich history of river management, but the core scientific advances in river corridors have evolved distinctly from the nature of the scientific questions and the needs of management and operational experts. We contend it is not a lack of awareness that has yielded the absence of cultural services in existing conceptual models, but a divergence between the scientific community who are generating conceptual models to understand the scientific phenomena occurring within river corridors and those who are managing rivers for cultural benefit.
In contrast, existing conceptual models are deeply engaged with both supporting and regulating services (Figure 3D). Carbon cycling was the most-studied CZ service in our inventory, with mention in over 76% of conceptual models. Additional provisioning services were common, including studies of the nitrogen cycle (52%), phosphorus cycle (47%), and whole ecosystem respiration or primary production (18%). Only three conceptual models address soil formation in the river corridor (Bechtold and Naiman, 2006; Wohl et al., 2015; Kumar et al., 2018), though the role of the river corridor as a depositional environment that stores and combines sediment sourced from the landscape is a more common theme (a regulating service; 63% of conceptual models). For additional regulating services, flooding was addressed by 50% of models, followed in frequency by carbon storage (45%) and water quality (37%). Drought, greenhouse gas emissions, and climate were relatively less frequent, included in only 11%, 18%, and 21% of existing conceptual models, respectively.
While coverage of exactly which CZ services were included was highly varied, human alteration or management of the river corridor appeared in more than 57% of conceptual models reviewed. Deeply integrated with existing conceptual models of the river corridor is the recognition of two-way feedbacks between humans and natural systems that are co-operating and co-evolving. This perspective is well-aligned with the CZ approach, even if some services and functions have yet to be explicitly integrated into our body of conceptual frameworks.
4. Challenges and opportunities in advancing predictive understanding of the river corridor as part of Earth's critical zone
Decades of research on the functionality of river corridors point to considerable diversity of conceptual models (Wohl, 2017) with fewer quantitative models (Harvey, 2016). A host of conceptual models from various disciplines has attempted to describe the structure and function of river corridors (Sections 1–3). These models, however, tend to be limited in the range of spatial and temporal scales considered, often focused on a single location or process. Consequently, few models offer interdisciplinary perspectives or the range of CZ services. Our ability to predict the structure and function of river corridors based on their geologic setting, climate, and human alteration remains limited (Ward et al., 2022). One recent literature review identified conflicting results reported for a majority of known controls on HEFs in the river corridor (Ward and Packman, 2019).
4.1. Opportunity 1: investigate local-scale processes in the context of larger-scale spatial gradients
Multi-scale and multi-factor studies are challenging to design and complete, but they are essential to predicting functions and services of river corridors. River corridors provide an ideal environment to test evolving concepts of macrosystems ecological theory, which emphasizes interactions among phenomena across spatial and temporal scales (Heffernan et al., 2014; McCluney et al., 2014; Thorp et al., 2021). The ideas of hotspots and hot moments (McClain et al., 2003) and ecosystem control points (Bernhardt et al., 2017) reflect the recognition that local-scale processes can be disproportionately important at larger spatial and temporal scales. At the same time, understanding larger and more slowly varying trends such as longitudinal changes in flow and sediment regimes, river corridor morphology, disturbance regime, and nutrient dynamics remain integral to our predictive and modeling capabilities. Reactive transport models provide one such opportunity to integrate processes, including those of hydrology and biogeochemistry, across spatial and temporal (Section 4.2) scales including scales beyond those of measurement (Li et al., 2017). In a statistical framework we can leverage information gleaned from both central tendencies and variability to address both local-scale processes as well as trends across scales. For example, the best-fit line in an empirical dataset can reveal consistent trends, but the noise—the distribution of data points about that line—can reveal the importance of site-specific controls. Hydrologic scaling analysis to extrapolate information on river networks is often based on bivariate and linear relationships between variables and discharge or drainage area, but that approach often hides key information about local-scale variability and their controls on the residuals.
4.2. Opportunity 2: embrace that river corridor processes are temporally variable and interacting
Useful insights have resulted from a focus on steady-state dynamics of river corridors, in which processes are investigated for baseflow or mean annual flow as though the processes were separable and had static effects across changing discharge. Existing knowledge, however, clearly indicates that processes in river corridors are temporally variable and interacting and may be nonlinear with changing discharge. Watersheds are recognized as complex non-linear filters (Kirchner et al., 2001; Kirchner, 2009) and adapting analyses that are not bound by linear assumptions is an important step forward to reduce uncertainty in models. Individual processes occur at periodic diel and seasonal frequencies, as well as irregular frequencies associated with individual storms, circulation patterns (e.g., El Nino/La Nina), and disturbances. We have relatively little understanding of these dynamic periods beyond individual floods, despite the clear indications that these can be “mission-critical” for predictions of river corridor behavior. The emerging focus on concentration-discharge (C–Q) dynamics provides an example of how the river science community is starting to explore temporal dynamics, using hysteresis loops of concentration vs. discharge to develop insight into the effects of watershed size and river network structure on C–Q relationships (Marinos et al., 2020) and weathering dynamics (Bouchez et al., 2017; Ibarra et al., 2017). The use of high-frequency sensor data which directly matches the chemograph to the hydrograph demonstrates that the scale of analysis can lead to misclassification of river corridor function (Fazekas et al., 2020).
4.3. Opportunity 3: river corridor functions do not respect disciplinary boundaries. Our science can reflect this reality by emphasizing integrated, multidisciplinary investigations
For centuries, formal scientific methods have emphasized measuring only what is needed for a specific hypothesis test. This approach is rigorous and can be useful, yet it also represents a narrow focus amenable to controlled settings that may allow only slow progress in understanding complex systems. We argue that some of the most productive advances in understanding river corridors have resulted from investigators who looked beyond their own discipline to think about river corridors holistically (e.g., Hynes, 1975; Naiman et al., 2002). We see the opportunity for substantial advances by employing systematic, organized studies that intentionally include many perspectives and measurements that cross spatial and temporal scales. In other words, we argue that a CZ lens should be brought to the river corridor. This is further enhanced by research observatories that leverage both CZ and ecological concepts and measurements. Investigations of river corridors during recent decades have primarily focused on ad hoc studies of a process, place, or timespan. By intentionally focusing on integrative studies we can move beyond the limited transferability of understanding that results from site-, scale-, and process-specific studies. Beven and Chappell (2021) discuss how perceptual model hypotheses can be tested at scales of interest and used to impose constraints on conditioning predictive models.
4.4. Opportunity 4: explicit integration of humans and their management actions beyond just the alteration of discharge
Humans have directly or indirectly (via changing climate) influenced every river corridor on Earth (Wohl, 2013). River scientists have embraced this realization in the context of natural flow regimes (Poff et al., 1997) and associated hydrological manipulations (Richter et al., 1996; King et al., 2003; Poff et al., 2010). And while discharge may be considered a master variable it is only one of many processes that can alter river corridor structure and function. Recognizing the ubiquity of other variables that create spatial and temporal variation in river corridors (e.g., channelization, sediment dynamics, stormwater retention and floodplain management, beaver and large wood removal; e.g., Peipoch et al., 2015) has lagged behind. To the best of our knowledge there are few widely used frameworks analogous to the flow-focused indicators of hydrologic alteration to the river corridor (but see Richter et al., 1996; Doyle et al., 2005). The difficulty in quantifying changes beyond flow regime arises from the lack of systematic records analogous to discharge and that are collected at similar time intervals. High-frequency in situ sensors offer the opportunity to directly match measures of solutes and turbidity to discharge (e.g., Wymore et al., 2019; Fazekas et al., 2021) without introducing error associated with interpolation methods. There has also been little progress estimating depth and overbank flooding or flow permanence in ungaged reaches compared to estimation of discharge. River travel time, which can be argued to be a master variable for water quality, is similarly poorly estimated across river basins (Harvey and Schmadel, 2021). Prediction of multi-factor events such as harmful algal blooms are only in their nascent stage (Community Coordinating Group on Integrated Hydro-Terrestrial Modeling, 2020). With these data needs in mind, we present three opportunities for river scientists that explicitly focus interactions between humans and river corridors.
The first strategy involves developing and populating frameworks that quantitatively characterize human alterations of river corridors, analogous to the existing quantifications of flow regime. Examples have been proposed for altered sediment regimes, largely in the context of dam and reservoir operations (Soar and Thorne, 2001; Grant et al., 2003; Schmidt and Wilcock, 2008). In arid areas, the flow through river corridors is often intermittent or ephemeral and needs better site-specific quantification (Jasechko et al., 2021). Rivers in arid zones are thought to be important in recharging groundwater (Famiglietti, 2014) but quantification in specific areas is elusive. Groundwater pumping is known locally as a source of streamflow depletion but quantifying the site-specific influence on rivers is difficult (Zipper et al., 2022), including groundwater interactions with rivers that draw modern groundwater deeper into aquifers (Van Loon et al., 2022).
Analogous frameworks that integrate human-induced changes in downstream river travel time, depth variability, and nutrient inputs and nutrient dynamics within river corridors (e.g., resulting from channelization and riparian deforestation) are needed, along with frameworks that quantitatively include changes in aquatic and riparian vegetation and fauna, trophic cascades, and large wood and organic matter dynamics, to name a few.
A second strategy to explicitly include humans in river corridor models involves relating human alterations to changes in river ecosystem services. As noted earlier, CZ services include provisioning, supporting and habitat, regulating, and cultural services (Field et al., 2015). River corridors excel at provisioning (commercial fisheries, clean water, fuel wood), supporting and habitat (soil formation, nutrient dynamics, primary productivity, aquatic and riparian habitat, biomass and biodiversity), regulating (water quality, contaminant dynamics, water storage and fluxes, sediment, large wood, carbon storage, greenhouse gas emissions), and cultural (recreation, spiritual, esthetic). Some investigators have explicitly quantified loss of river ecosystem services as a result of human alterations (e.g., Hanberry et al., 2015; Vaughn et al., 2015; Ekka et al., 2020), but there remain abundant opportunities to demonstrate the importance of fully functional river corridor ecosystems by quantifying the effects of their alteration.
A third strategy to incorporate humans in river corridors includes working with social scientists to integrate human perceptions and values into river management decisions. This latter point has become particularly noteworthy in the context of river restoration projects in which significant gaps can become apparent between what river scientists and other stakeholders consider to be desirable states of a river corridor (Piegay et al., 2005; Kondolf, 2006; Le Lay et al., 2013). Consequently, river restoration projects may focus on an unsustainable or ecologically inappropriate configuration of the river corridor because of conflicting restoration objectives (e.g., Kondolf et al., 2001). A long history of public resistance to scientific insights, from the links between lead in paint and gasoline and human health (Needleman, 1998) to cigarette smoking and cancer (Doll, 2016) through human-induced global warming (Shao, 2017) and the effectiveness of masks in limiting disease transmission (Zagury-Orly, 2020), clearly indicates that many stakeholders will not simply accept scientific knowledge. Integrating humans into river corridor science requires recognizing that there are diverse and “non-traditional” ways of communicating scientific information that can be much more effective than simple statements of scientific results (e.g., head, heart, hands model; Geiger et al., 2017). Still, representatives from seven U.S. federal agency recently met with academics to identify water-related priorities for improved collaboration in predictive modeling and forecasting. Selected priorities had strong elements of river corridor science, including river flooding, western U.S. water availability, and hypoxia and harmful aquatic blooms (HABs) in lakes, rivers, and estuaries (Community Coordinating Group on Integrated Hydro-Terrestrial Modeling, 2020).
Critical Zone science has also developed useful approaches to understanding the role of humans as agents of change for the functioning of the near-earth surface. For example, “earthcasting” is an approach used to predict future CZ and the chemical, physical, and biological interactions that define the CZ. Unique to earthcasting is the specific targeting of CZ processes such as weathering rates under future climate scenarios and how the lysocline will change (Goddéris and Brantley, 2013). This type of modeling can reveal non-linearities in response curves due to the incorporation of longer-time scales which may be especially key for slower processes (Sullivan et al., 2019) and the coupling of processes over short and long-time scales (McIntosh et al., 2017). Critical Zone science has also directly incorporated human dimensions into the study of the CZ by explicitly building scientific observatories in highly human-impacted environments. This includes, for example, the Intensively Managed Landscape Critical Zone Observatory (CZO) in the Midwestern United States and the Christina River Basin CZO in Piedmont/Mid-Atlantic region of the United States which incorporates a mosaic of land uses and human impact (White et al., 2015).
The river science community has developed useful methods of addressing human integration with natural systems that can add substantially to the CZ community. Integrated water resources management promotes the development and management of water, land, and related resources. Such coordination can maximize economic and social welfare in an equitable manner without compromising the sustainability of vital ecosystems (Global Water Partnership, 2000). How this coordination operates in practical scientific and management outcomes with respect to the river corridor remains vague and difficult to demonstrate (Biswas, 2008). These difficulties reflect the need to recognize cultural, political, and socioeconomic heterogeneity of human societies, the ecological and physical heterogeneity of river corridors, and the need to develop objective and impartial assessments of integrated water management applicable at diverse spatial and temporal scales (Biswas, 2008).
4.5. Organizing our understanding of the river corridor
How can river corridor scientists fully adopt an integrative CZ perspective? We proposed a framework for organizing the stores and fluxes of material in the river corridors inspired by the CZ framework (Figure 1) which incorporates gradients of lithology and climate (White et al., 2015; Pujari et al., 2020). And like the observatory approach popularized by the international CZ (Brantley et al., 2017) and ecological communities (Mirtl et al., 2018), the next generation of earth observatories could be designed to target river corridor processes specifically. While we acknowledge additional detail is needed to represent spatial and temporal heterogeneity, and that underlying processes may mediate transformation or transport dynamics, we pose Figure 1 as a unit and framework of study. We envision this schematic as a “building block” from which understanding, and predictions can be organized (Figure 4). For example, river corridors in different geologic settings, climates, or management regimes could be compared (i.e., the two river corridors depicted in Figure 4 are simply two archetypes of many that can exist; see Figure 1C for examples of additional river corridors in different geologic and climatic settings). The smaller conceptual models in Figure 4C represent different locations on the landform that are critical for a given function or process, network position, alternative state (e.g., management regime or climate), or discharge condition of interest. We provide this framework as a forward-looking example that integrates key tenets of the strategies described above. Specifically, it enables for spatial gradients and local variation (Opportunity 1), to be integrated with temporal dynamics (Opportunity 2) from across disciplines (Opportunity 3), while explicitly accommodating a variety of human impacts and management regimes (Opportunity 4).
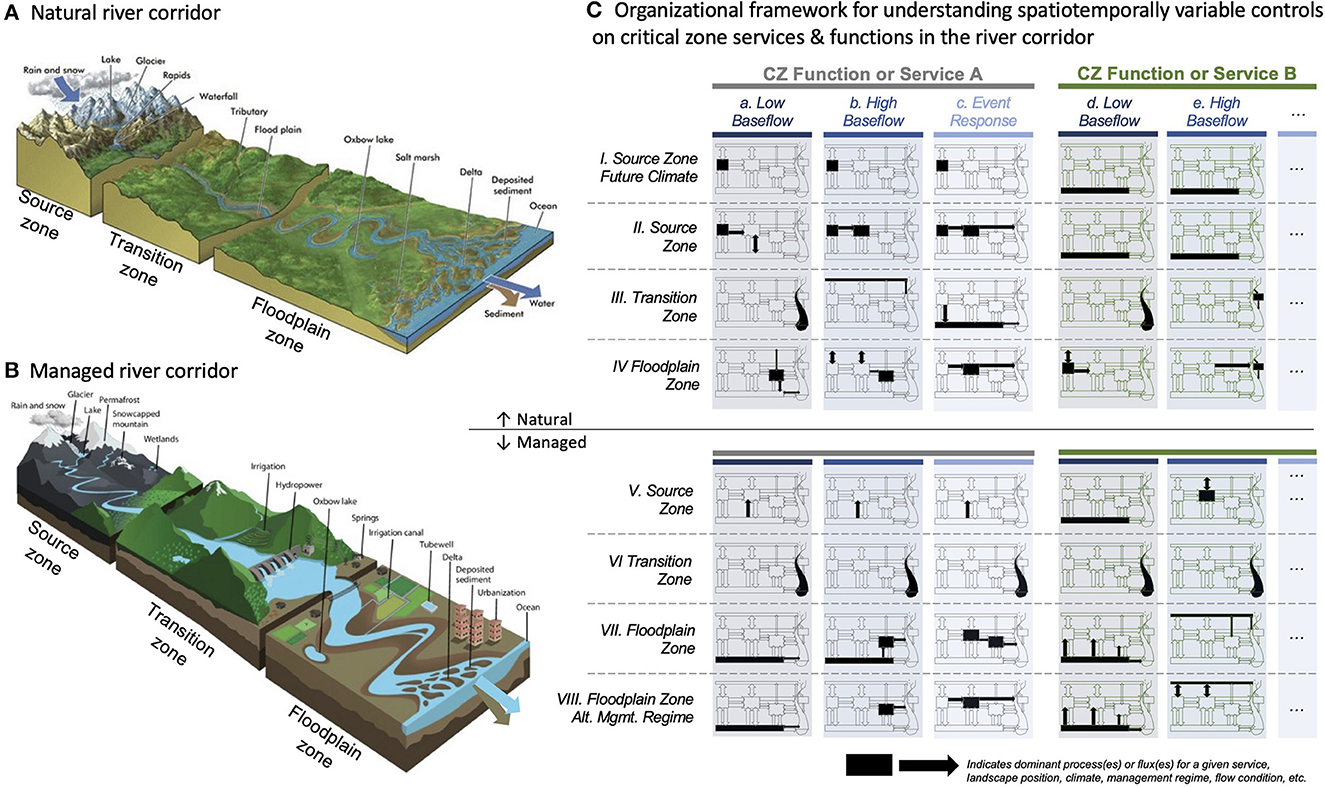
Figure 4. Proposed organization for studying the river corridor as part of the Critical Zone. Panels on the left display two different river corridors, highlighting in this case (A) a natural and (B) managed river corridor. These examples would logically be extended to include an array of river corridors spanning different geologic settings, climates, and management regimes. Panel (C) illustrates organization of spatial gradients along each river corridor (top-to-bottom representing a headwater-to-ocean transition) and the need to consider different discharge conditions (columns a vs. b vs. c) and CZ functions or services (groupings for “service A” vs. “service B”). Isometrics in (A) and (B) are modified from ICIMOD (2023) and Miller and Spoolman (2021), respectively.
5. Conclusions
The overarching objective of this review and perspective is to assert that river corridors are unique landforms within Earth's critical zone with important boundaries and dynamics worthy of integrative and multidisciplinary study. While the evolution of river science has embraced some tenets of the CZ approach (e.g., multi-scale, function/service oriented), gaps clearly remain. We emphasize that river corridor science IS critical zone science, and arguably has reflected dimensions of the CZ before the term “critical zone” was coined. The contemporary understanding of river corridors positions the field to make substantive advances in our predictive understanding by more effectively recognizing that all of the spatial and temporal dimension of the CZ are represented within the river corridor.
River corridor scientists must embrace the tenets of critical zone science, specifically, that the geologic template and climate interact to determine structural and functional aspects of the CZ while incorporating time scales from seconds to millennia (Brantley et al., 2007). The river corridor uniquely integrates these elements of the CZ, allowing for testing of fundamental CZ concepts including the transporter-transformer hypothesis (Kumar et al., 2018; Fazekas et al., 2021; Arora et al., 2022; Wymore et al., 2022), the feed-through reactor concept (Anderson et al., 2007), and characterizing the CZ as on open system of energy and mass fluxes that vary over space and time (Rasmussen et al., 2011). Furthermore, groundwater and surface water in river corridors are a single resource (Winter et al., 1998), providing services that should be economically valued and managed accordingly (Buchs et al., 2021).
We have outlined four opportunities to study river corridors that will advance our predictive understanding, to the benefit of scientists and society. We have posed a conceptual framework to build a comprehensive, transferable understanding of the river corridor that organizes our understanding with an emphasis on the dominant-process approach to modeling.
Finally, we recognize that building from this synthesis, and a rich history of integrative, multi-scale research will advance our predictive understanding of the river corridor. Specifically, we recognize river corridors as key functional elements within the CZ, serve as integrators of upland exports, and as connectors along the summit-to-sea continuum allowing for the effective integration of systems across the CZ. Employing holistic, integrative approaches will also enhance conceptualization of river corridors as dynamic hydraulic-geomorphic-biological systems within the greater CZ. The modeling community can adapt scalable and transferable model frameworks for integrated prediction in river corridors that address pressing societal problems, such as growing intermittency of stream flows in dry climates and harmful algal blooms in rivers. The observational science community can test and quality assure a greater array of sensors from ground, aerial, and remote platforms to drive model predictions across multiple spatial and temporal scales. The data science community may develop optimal network sampling designs for river corridors, as well as improve methods of hydrologic scaling using machine learning and related approaches can help refine predictive models. The call for integrated prediction capabilities informed by multidisciplinary perspectives is advancing on numerous fronts.
Author contributions
AWy, AWa, EW, and JH each contributed to the conceptualization, drafting, editing, and revisions of this manuscript. AWy and AWa initially conceptualized the work and led early efforts to review and analyze conceptual models and led the final editing of the manuscript. All authors contributed to the article and approved the submitted version.
Funding
AWy was supported by the National Science Foundation and EPSCoR project Canary in the Watershed (NSF EPS-1929148), the NSF-funded Belmont Forum ABRESO project (NSF-2129383), the New Hampshire Agricultural Experiment Station, and the USDA National Institute of Food and Agriculture Hatch Multi-State Project 1022291. This is scientific contribution number 2977. AWa was supported by Department of Energy awards DE-SC000022 and DE-SC0019377, NSF Award EAR 1652293, the Burnell and Barbara Fischer fellowship from Indiana University, and the Fulbright—University of Birmingham Scholar program. JH was supported by the U.S. Geological Survey Water Availability and Use and Water Quality Science Programs.
Acknowledgments
Early discussions about river corridors as critical zones were held with a host of colleagues providing input, including: J. Bales, A. Berhe, N. Blain, A. Burgin, M. Cohen, T. Covino, D. Edmonds, S. Godsey, G. Grant, N. Grimm, R. Hall, C. Harman, S. Herzog, D. Karwan, C. Luce, A. Packman, C. Phillips, T. Royer, J. Stegen, A. Visser, A. Wilcox, W. Wollheim, S. Wondzell, B. Yanites, L. Yoder, and J. Zarnetske. This early ideation was foundational in our thinking and informed the perspectives we set forth in this manuscript. The authors are also grateful for the effort of two reviewers and Paige Becker all of whom provided valuable feedback that improved the manuscript.
Conflict of interest
The authors declare that the research was conducted in the absence of any commercial or financial relationships that could be construed as a potential conflict of interest.
Publisher's note
All claims expressed in this article are solely those of the authors and do not necessarily represent those of their affiliated organizations, or those of the publisher, the editors and the reviewers. Any product that may be evaluated in this article, or claim that may be made by its manufacturer, is not guaranteed or endorsed by the publisher.
References
Abbe, T. B., and Montgomery, D. R. (2003). Patterns and processes of wood debris accumulation in the Queets River basin, Washington. Geomorphology 51, 81–107. doi: 10.1016/S0169-555X(02)00326-4
Alexander, L. C., Fritz, K. M., Schofield, K. A., Autrey, B. C., DeMeester, J. E., Golden, H. E., et al. (2018). Featured collection introduction: connectivity of streams and wetlands to downstream waters. J. Am. Water Resour. Assoc. 54, 287–297. doi: 10.1111/1752-1688.12630
Allen, J. R. L. (1978). Studies in fluviatile sedimentation: an exploratory quantitative model for the architecture of avulsion-controlled alluvial suites. Sediment. Geol. 21, 129–147. doi: 10.1016/0037-0738(78)90002-7
Amoros, C., and Bornette, G. (2002). Connectivity and biocomplexity in waterbodies of riverine floodplains. Freshw. Biol. 47, 761–776. doi: 10.1046/j.1365-2427.2002.00905.x
Amoros, C., and Roux, A. L. (1988). Interaction between water bodies within the floodplains of large rivers: function and development of connectivity. M?nstersche Geographische Arbeiten. 29, 125–130.
Amundson, R., Richter, D. D., Humphreys, G. S., Jobbágy, E. G., and Gaillardet, J. (2007). Coupling between biota and earth materials in the critical zone. Elements 3, 327–332. doi: 10.2113/gselements.3.5.327
Anderson, S. P., von Blanckenburg, F., and White, A. F. (2007). Physical and chemical controls on the critical zone. Elements 3, 315–319. doi: 10.2113/gselements.3.5.315
Appling, A. P., Bernhardt, E. S., and Stanford, J. A. (2014). Floodplain biogeochemical mosaics: a multidimensional view of alluvial soils. J. Geophys. Res. Biogeosci. 119, 1538–1553. doi: 10.1002/2013JG002543
Arnberger, A., Eder, R., Preiner, S., Hein, T., and Nopp-Mayr, U. (2021). Landscape preferences of visitors to the Danube Floodplains National Park, Vienna. Water 13, 2178. doi: 10.3390/w13162178
Arora, B., Briggs, M. A., Zarnetske, J. P., Stegen, J., Gomez-Velez, J. D., Dwivedi, D., et al. (2022). “Hot spots and hot moments in the critical zone: identification of and incorporation into reactive transport models,” in Biogeochemistry of the Critical Zone, eds A. S. Wymore, W. H. Yang, W. L. Silver, W. H. McDowell, and J. Chorover (Cham: Springer), 9–47. doi: 10.1007/978-3-030-95921-0_2
Arrigoni, A. S., Poole, G. C., Mertes, L. A. K., O'Daniel, S. J., Woessner, W. W., Thomas, S. A., et al. (2008). Buffered, lagged, or cooled? Disentangling hyporheic influences on temperature cycles in stream channels. Water Resour. Res. 44, W09418. doi: 10.1029/2007WR006480
Arscott, D. B., Tockner, K., van der Nat, D., and Ward, J. V. (2002). Aquatic habitat dynamics along a braided alpine river ecosystem (Tagliamento River, northeast Italy). Ecosystems 5, 802–814. doi: 10.1007/s10021-002-0192-7
Arthington, A. H., Bunn, S. E., Poff, N. L., and Naiman, R. J. (2006). The challenge of providing environmental flow rules to sustain river ecosystems. Ecol. Appl. 16, 1311–1318. doi: 10.1890/1051-0761(2006)016[1311:TCOPEF]2.0.CO;2
Aufdenkampe, A. K., Mayorga, E., Raymond, P. A., Melack, J. M., Doney, S. C., Alin, S. R., et al. (2011). Riverine coupling of biogeochemical cycles between land, oceans, and atmosphere. Front. Ecol. Environ. 9, 53–60. doi: 10.1890/100014
Baker, E. B., and Showers, W. J. (2019). Hysteresis analysis of nitrate dynamics in the Neuse River, NC. Sci. Total Environ. 652, 889–899. doi: 10.1016/j.scitotenv.2018.10.254
Baker, M. A., Dahm, C. N., and Valett, H. M. (2000). “Anoxia, anaerobic metabolism biogeochemistry of the stream water- ground water interface,” in Streams and Ground Waters, eds J. B. Jones, Jr., and P. J. Mulholland (San Diego, CA: Academic Press), 259–284. doi: 10.1016/B978-012389845-6/50012-0
Baker, V. R., Kochel, R. C., and Patton, P. C., (eds). (1988). Flood Geomorphology. New York, NY: Wiley and Sons.
Bates, P. D., Horritt, M. S., Smith, C. N., and Mason, D. (1997). Integrating remote sensing observations of flood hydrology and hydraulic modelling. Hydrol. Process. 11, 1777–1795. doi: 10.1002/(SICI)1099-1085(199711)11:14<1777::AID-HYP543>3.0.CO;2-E
Battin, T. J., Besemer, K., Bengtsson, M. M., Romani, A. M., and Packmann, A. I. (2016). The ecology and biogeochemistry of stream biofilms. Nat. Rev. Microbiol. 14, 251–263. doi: 10.1038/nrmicro.2016.15
Battin, T. J., Luyssaert, S., Kaplan, L. A., Aufdenkampe, A. K., Richter, A., Tranvik, L. J., et al. (2009). The boundless carbon cycle. Nat. Geosci. 2, 598–600. doi: 10.1038/ngeo618
Bechtold, J. S., and Naiman, R. J. (2006). Soil texture and nitrogen mineralization potential across a riparian toposequence in a semi-arid savanna. Soil Biol. Biochem. 38, 1325–1333. doi: 10.1016/j.soilbio.2005.09.028
Becker, P. S., Ward, A. S., Herzog, S. P., and Wondzell, S. M. (2023). Testing hidden assumptions of representativeness in reach-scale studies of hyporheic exchange. Water Resour. Res. 59, e2022WR032718. doi: 10.1029/2022WR032718
Bellmore, J. R., and Baxter, C. V. (2014). Effects of geomorphic process domains on river ecosystems: a comparison of floodplain and confined valley segments. River Res. App. 30, 617–630. doi: 10.1002/rra.2672
Bencala, K. E., Kennedy, V. C., Zellweger, G. W., Jackman, A. P., and Avanzino, R. J. (1984). Interactions of solutes and streambed sediment: 1. An experimental analysis of cation and anion transport in a mountain stream, Water Resour. Res. 20, 1797–1803. doi: 10.1029/WR020i012p01797
Benda, L., Poff, N. L., Miller, D., Dunne, T., Reeves, G., Pess, G., et al. (2004). The network dynamics hypothesis: how channel networks structure riverine habitats. Bioscience 54, 413–427. doi: 10.1641/0006-3568(2004)054(0413:TNDHHC)2.0.CO;2
Benda, L. E., and Sias, J. C. (2003). A quantitative framework for evaluating the mass balance of in-stream organic debris. Forest Ecol. Manag. 172, 1–16. doi: 10.1016/S0378-1127(01)00576-X
Bernhardt, E. S., Blaszczak, J. R., Ficken, C. D., Fork, M. L., Kaiser, K. E., Seybold, E. C., et al. (2017). Control points in ecosystems: moving beyond the hot spot hot moment concept. Ecosystems 20, 665–682. doi: 10.1007/s10021-016-0103-y
Bernhardt, E. S., Savoy, P., Vlah, M. J., Appling, A. P., Koenig, L. E., Hall, R. O., et al. (2022). Light and flow regimes regulate the metabolism of rivers. Proc. Natl. Acad. Sci. U.S.A 119, e2121976119. doi: 10.1073/pnas.2121976119
Besemer, K. (2015). Biodiversity, community structure and function of biofilms in stream ecosystems. Res. Microbiol. 166, 774–781. doi: 10.1016/j.resmic.2015.05.006
Beven, K. J., and Chappell, N. A. (2021). Perceptual perplexity and parameter parsimony. WIREs Water 8, e1530. doi: 10.1002/wat2.1530
Bianchi, T. S. (2021). The evolution of biogeochemistry: revisited. Biogeochemistry 154, 141–81. doi: 10.1007/s10533-020-00708-0
Birken, A. S., and Cooper, D. J. (2006). Processes of tamarisk invasion and floodplain development along the lower Green River, Utah. Ecol. Appl. 16, 1103–1120. doi: 10.1890/1051-0761(2006)016[1103:POTIAF]2.0.CO;2
Biswas, A. K. (2008). Integrated water resources management: is it working? Int. J. Water Resour. Dev. 24, 5–22. doi: 10.1080/07900620701871718
Blair, N. E., and Aller, R. C. (2012). The fate of terrestrial organic carbon in the marine environment. Ann. Rev. Mar. Sci. 4, 401–423. doi: 10.1146/annurev-marine-120709-142717
Blair, N. E., Leithold, E. L., and Aller, R. C. (2004). From bedrock to burial: the evolution of particulate organic carbon across coupled watershed-continental margin systems. Marine Chem. 92, 141–156. doi: 10.1016/j.marchem.2004.06.023
Blaszczak, J. R., Koenig, L. E., Mejia, F. H., Gomez-Gener, L., Dutton, C. L., Carter, A. M., et al. (2022). Extent, patterns, and drivers of hypoxia in the world's streams and rivers. Limnol. Oceanog. Lett. doi: 10.1002/lol2.10297
Boano, F., Harvey, J. W., Marion, A., Packman, A. I., Revelli, R., Ridolfi, L., et al. (2014). Hyporheic flow and transport processes: mechanisms, models, and biogeochemical implications. Rev. Geophys. 52, 603–679. doi: 10.1002/2012RG000417
Bouchez, J., Moquet, J. S., Espinoza, J. C., Martinez, J. M., Guyot, J. L., Lagane, C., et al. (2017). River mixing in the Amazon as a driver of concentration-discharge relationships. Water Resour. Res. 53, 8660–8685. doi: 10.1002/2017WR020591
Boulton, A. J., and Lake, P. S. (2008). “Effects of drought on stream insects and its ecological consequences,” in Aquatic Insects: Challenges to Populations, eds J. Lancaster, and R. A. Briers (Wallingford UK: CABI), 81–102. doi: 10.1079/9781845933968.0081
Bouwman, A. F., Bierkens, M. F. P., Griffioen, J., Hefting, M. M., Middelburg, J. J., Middelkoop, H., et al. (2013). Nutrient dynamics, transfer and retention along the aquatic continuum from land to ocean: towards integration of ecological and biogeochemical models. Biogeosciences 10, 1–22. doi: 10.5194/bg-10-1-2013
Bracken, L. J., Turnbull, L., Wainwright, J., and Bogaart, P. (2015). Sediment connectivity: a framework for understanding sediment transfer at multiple scales. Earth Surf. Process. Landf. 40, 177–188. doi: 10.1002/esp.3635
Bracken, L. J., Wainwright, J., Ali, G. A., Tetzlaff, D., Smith, M. W., Reaney, S. M., et al. (2013). Concepts of hydrological connectivity: research approaches, pathways and future agendas. Earth-Sci. Rev. 119, 17–34. doi: 10.1016/j.earscirev.2013.02.001
Brantley, S. L., Goldhaber, M. B., and Ragnarsdottir, K. V. (2007). Crossing disciplines and scales to understand the critical zone. Elements 3, 307–314. doi: 10.2113/gselements.3.5.307
Brantley, S. L., McDowell, W. H., Dietrich, W. E., White, T. S., Kumar, P., Anderson, S. P., et al. (2017). Designing a network of critical zone observatories to explore the living skin of the terrestrial Earth. Earth Surf. Dyn. 5, 841–860. doi: 10.5194/esurf-5-841-2017
Brazier, R. E., Puttock, A., Graham, H. A., Auster, R. E., Davies, K. H., Brown, C. M. L., et al. (2021). Beaver: nature's ecosystem engineers. WIREs Water 8, e1494. doi: 10.1002/wat2.1494
Bridge, J. S., and Leeder, M. R. (1979). A simulation model of alluvial stratigraphy. Sedimentology 26, 617–644. doi: 10.1111/j.1365-3091.1979.tb00935.x
Brierley, G. J., and Fryirs, K. A. (2005). Geomorphology and River Management: Applications of the River Styles Framework. Carlton, VIC: Blackwell Publishing. doi: 10.1002/9780470751367
Briggs, M. A., Day-Lewis, F. D., Zarnetske, J. P., and Harvey, J. W. (2015). A mechanistic explanation for the development of hyporheic redox microzones. Geophys. Resear. Lett. 42, 4402–4410. doi: 10.1002/2015GL064200
Brooks, A. C., Covino, T., and Hall, E. K. (2022). Evaluating spatial and temporal dynamics of river-floodplain surface water connectivity using hydrometric, geochemical and microbial indicators. Water Resour. Res. 58, e2021WR030336. doi: 10.1029/2021WR030336
Brooks, J. R., Wigington, P. J., Phillips, D. L., Comeleo, R., and Coulombe, R. (2012). Willamette River Basin surface water isoscape (∂18O and ∂2H): temporal changes of source water within the river. Ecosphere 3, 1–21. doi: 10.1890/ES11-00338.1
Brown, A. G., Lespez, L., Sear, D. A., Macaire, J. J., Houben, P., Klimek, K., et al. (2018). Natural vs anthropogenic streams in Europe: history, ecology and implications for restoration, river-rewilding and riverine ecosystem services. Earth-Sci. Rev. 180, 185–205. doi: 10.1016/j.earscirev.2018.02.001
Brunsden, D., and Thornes, J. B. (1979). Landscape sensitivity and change. Trans. Inst. Br. Geogr. 4, 463–484. doi: 10.2307/622210
Buchs, A., Calvo-Mendieta, I., Petit, O., and Roman, P. (2021). Challenging the ecological economics of water: social and political perspectives. Ecol. Econ. 190, 107176. doi: 10.1016/j.ecolecon.2021.107176
Burchsted, D., Daniels, M., Thorson, R., and Vokoun, J. (2010). The river discontinuum: applying beaver modifications to baseline conditions for restoration of forested headwaters. Bioscience 60, 908–922. doi: 10.1525/bio.2010.60.11.7
Burgi, M., Ostlund, L., and Mladenoff, D. J. (2017). Legacy effects of human land use: ecosystems as time-lagged systems. Ecosystems 20, 94–103. doi: 10.1007/s10021-016-0051-6
Butman, D., Strigl, R., Stackpoole, S., del Giorgio, P., Prairie, Y., Pilcher, D., et al. (2018). “Chapter 14: Inland waters,” in Second State of the Carbon Cycle Report (SOCCR2): A Sustained Assessment Report, eds N. Cavallaro, G. Shrestha, R. Birdsey, M. A. Mayes, R. G. Najjar, S. C. Reed, et al. (Washington, DC: U.S. Global Change Research Program), 568–595. doi: 10.7930/SOCCR2.2018.Ch14
Cardenas, M. B., and Wilson, J. L. (2007). Dunes, turbulent eddies, and interfacial exchange with permeable sediments. Water Resour. Res. 43, W08412. doi: 10.1029/2006WR005787
Carpenter, S. R., DeFries, R., Mooney, H. A., Polasky, S., Reid, W., Scholes, R., et al. (2006). Millennium ecosystem assessment: research needs. Science 313, 257–258. doi: 10.1126/science.1131946
Cartwright, I., Werner, A. D., and Woods, J. A. (2019). Using geochemistry to discern the patterns and timescales of groundwater recharge and mixing on floodplains in semi-arid regions. J. Hydrol. 570, 612–622. doi: 10.1016/j.jhydrol.2019.01.023
Castro, J. M., and Thorne, C. R. (2019). The stream evolution triangle: integrating geology, hydrology, and biology. River Resear. Appl. 35, 315–326. doi: 10.1002/rra.3421
Chen, X., Zachara, J. M., Vermuel, V. R., Hammond, G., Freshley, M., and Fang, Y. (2020). Understanding contaminant migration within a dynamic river corridor through field experiments and reactive transport modeling. Front. Water. 2, 533796. doi: 10.3389/frwa.2020.533796
Chorover, J., Kretzschmar, R., Garcia-Pichel, F., and Sparks, D. L. (2007). Soil biogeochemical processes within the critical zone. Elements 3, 321–326. doi: 10.2113/gselements.3.5.321
Clark, K. E., Shanley, J. B., Scholl, M. A., Perdrial, N., Perdrial, J. N., Plante, A. F., et al. (2017). Tropical river suspended sediment and solute dynamics in storms during an extreme drought. Water Res. Res. 53, 3695–712. doi: 10.1002/2016WR019737
Clark, M. P., Fan, Y., Lawrence, D. M., Adam, J. C., Bolster, D., Gochis, D. J., et al. (2015). Improving the representation of hydrologic processes in Earth System Models. Water Resour. Res. 51, 5929–5956. doi: 10.1002/2015WR017096
Clements, F. E. (1905). Research Methods in Ecology. Lincoln, NE: University Publishing Company. doi: 10.5962/bhl.title.54858
Cole, J. J., Prairie, Y. T., Caraco, N. F., McDowell, W. H., Tranvik, L. J., Striegl, R. G., et al. (2007). Plumbing the global carbon cycle: integrating inland waters into the terrestrial carbon budget. Ecosystems 10, 172–185. doi: 10.1007/s10021-006-9013-8
Collier, M., Webb, R. H., and Schmidt, J. C. (1996). Dams and Rivers: A Primer on the Downstream Effects of Dams. Tucson, AZ: U.S. Geological Survey Circular 1126. doi: 10.3133/cir1126
Collins, B. L., Montgomery, D. R., Fetherston, K. L., and Abbe, T. B. (2012). The floodplain large-wood cycle hypothesis: a mechanism for the physical and biotic structuring of temperate forested alluvial valleys in the North Pacific coastal ecoregion. Geomorphology 139–140, 460–470. doi: 10.1016/j.geomorph.2011.11.011
Community Coordinating Group on Integrated Hydro-Terrestrial Modeling. (2020). Integrated Hydro-Terrestrial Modeling: Development of a National Capability. Report of an Interagency Workshop held September 4-6, 2019 with Support from the National Science Foundation, the U.S. Department of Energy, and the U.S. Geological Survey. doi: 10.25584/09102020/1659275
Conant, B Jr., Cherry, J. A., and Gillham, R. W. (2004). A PCE groundwater plume discharging to a river: influence of the streambed and near-river zone on contaminant distributions. J. Contam. Hydrol. 73, 249–279. doi: 10.1016/j.jconhyd.2004.04.001
Connell, J. P. (1978). Diversity in tropical rain forest and coral reefs. Science 199, 1302–1310. doi: 10.1126/science.199.4335.1302
Cordell, H. K., Bergstrom, J. C., Ashley, G. A., and Karish, J. (1990). Economic effects of river recreation on local economies. J. Am. Water Resour. Assoc. 26, 53–60. doi: 10.1111/j.1752-1688.1990.tb01350.x
Covino, T. (2017). Hydrologic connectivity as a framework for understanding biogeochemical flux through watersheds and along fluvial networks. Geomorphology 277, 133–144. doi: 10.1016/j.geomorph.2016.09.030
Dietrich, W. E., and Dunne, T. (1978). Sediment budget for a small catchment in mountainous terrain. Z. Geomorphol. Suppl. 29, 191–206.
Doble, R. C., Crosbie, R. S., Smerdon, B. D., Peeters, L., and Cook, F. J. (2012). Groundwater recharge from overbank floods. Water Resour. Res. 48, W09522. doi: 10.1029/2011WR011441
Dodds, W. K., Gido, K., Whiles, M. R., Daniels, M. D., and Grudzinski, B. P. (2015). The stream biome gradient concept: factors controlling lotic systems across broad biogeographic scales. Freshw. Sci. 34, 1–19. doi: 10.1086/679756
Doll, R. (2016). Uncovering the effects of smoking: historical perspective. Stat. Methods Med. Res. 7, 87–117. doi: 10.1191/096228098668199908
Doolittle, W. E. (1990). Canal Irrigation in Prehistoric Mexico: The Sequence of Technological Change. Austin: University of Texas Press. doi: 10.7560/715585
Doyle, M. W., and Ensign, S. H. (2009). Alternative reference frames in river system science. BioScience 59, 499–510. doi: 10.1525/bio.2009.59.6.8
Doyle, M. W., Stanley, E. H., Strayer, D. L., Jacobson, R. B., and Schmidt, J. C. (2005). Effective discharge analysis of ecological processes in streams. Water Resour. Res. 41, W11411. doi: 10.1029/2005WR004222
Dunne, T., Mertes, L. A. K., Meade, R. H., Richey, J. E., and Forsberg, B. R. (1998). Exchanges of sediment between the flood plain and channel of the Amazon River in Brazil. Geol. Soc. Am. Bull. 110, 450–467. doi: 10.1130/0016-7606(1998)110<0450:EOSBTF>2.3.CO;2
Dutton, C. L., Subalusky, A. L., Hamilton, S. K., Bayer, E. C., Njoroge, L., Rosi, E. J., et al. (2021). Alternative biogeochemical states of river pools mediated by hippo use and flow variability. Ecosystems 24, 284–300. doi: 10.1007/s10021-020-00518-3
Dwivedi, D., Steefel, C. I., Arora, B., Banfield, J., Bargar, J., Boyanov, M. I., et al. (2022). From legacy contamination to watershed systems science: a review of scientific insights and technologies developed through DOE-supported research in water and energy security. Environ. Res. Lett. 17, 043004. doi: 10.1088/1748-9326/ac59a9
Eisenberg, C., Anderson, C. L., Collingwood, A., Sissons, R., Dunn, C. J., Meigs, G. W., et al. (2019). Out of the ashes: ecological resilience to extreme wildfire, prescribed burns, and indigenous burning in ecosystems. Front. Ecol. E7, 436. doi: 10.3389/fevo.2019.00436
Ekka, A., Pande, S., Jiang, Y., and van der Zaag, P. (2020). Anthropogenic modifications and river ecosystem services: a landscape perspective. Water 12, 706. doi: 10.3390/w12102706
Elliott, A. H., and Brooks, N. H. (1997). Transfer of nonsorbing solutes to a streambed with bed forms: Theory. Water Resour. Res. 33, 123–136.
Eng, K., Wolock, D. M., and Carlisle, D. M. (2013). River flow changes related to land and water management practices across the conterminous United States. Sci. Total Environ. 463–464, 414–422. doi: 10.1016/j.scitotenv.2013.06.001
Ensign, S. H., and Doyle, M. W. (2006). Nutrient spiraling in streams and river networks. J. Geophys. Resear. Biogeosci. 111, G04009. doi: 10.1029/2005JG000114
Famiglietti, J. (2014). The global groundwater crisis. Nat. Clim. Change 4, 945–948. doi: 10.1038/nclimate2425
Fan, Y., Li, H., and Miguez-Macho, G. (2013). Global patterns of groundwater table depth. Science 339, 940–943. doi: 10.1126/science.1229881
Fang, Y., Song, X., Ren, H., Perkins, W. A., Shuai, P., Richmond, M. C., et al. (2020). High-performance simulation of dynamic hydrologic exchange and implications for surrogate flow and reactive transport modeling in a large river corridor. Front. Water. 2, 687. doi: 10.46427/gold2020.687
Fausch, K. D., Torgersen, C. E., Baxter, C. V., and Li, H. W. (2002). Landscapes to riverscapes: bridging the gap between research and conservation of stream fishes. Bioscience 52, 483–498. doi: 10.1641/0006-3568(2002)052[0483:LTRBTG]2.0.CO;2
Fazekas, H. M., McDowell, W. H., Shanley, J. B., and Wymore, A. S. (2021). Climate variability drives watersheds along a transporter-transformer continuum. Geophys. Res. Lett. 48, e2021GL094050. doi: 10.1029/2021GL094050
Fazekas, H. M., Wymore, A. S., and McDowell, W. H. (2020). Dissolved organic carbon and nitrate concentration-discharge behavior across scales: land use, excursions, and misclassification. Water Resour. Res. 56, e2019WR027028. doi: 10.1029/2019WR027028
Field, J. P., Breshears, D. D., Law, D. J., Villegas, J. C., López-Hoffman, L., Brooks, P. D., et al. (2015). Critical zone services: expanding context, constraints, and currency beyond ecosystem services. Vadose Zone J. 14, 1–7. doi: 10.2136/vzj2014.10.0142
Findlay, S. (1995). Importance of surface-subsurface exchange in stream ecosystems: the hyporheic zone. Limnol. Oceanogr. 40, 159–164. doi: 10.4319/lo.1995.40.1.0159
Fisher, S. G., Grimm, N. B., Marti, E., Holmes, R. M., and Jones, J. B. (1998). Material spiraling in stream corridors: a telescoping ecosystem model. Ecosystems 1, 19–34. doi: 10.1007/s100219900003
Fisher, S. G., and Likens, G. E. (1973). Energy flow in Bear Brook, New Hampshire: an integrative approach to stream ecosystem metabolism. Ecol. Monogr. 43, 421–39. doi: 10.2307/1942301
Fisk, H. N. (1947). Fine-grained Alluvial Deposits and Their Effects on Mississippi River Activity. Vicksburg, MS: US Army Corps of Engineers, Waterways Experiment Station.
Forbes, S. A. (1887). The Lake as a Microcosm. Peoria, IL: Bulletin of the Scientific Association of Peoria, 77–87. Reprinted in Illinois Natural History Survey Bulletin 15, 537–550. doi: 10.21900/j.inhs.v15.303
Francis, R. A. (2014). Urban rivers: novel ecosystems, new challenges. WIREs Water 1, 19–29. doi: 10.1002/wat2.1007
Frissell, C. A., Liss, W. J., Warren, C. E., and Hurley, M. D. (1986). A hierarchical framework for stream habitat classification: viewing streams in a watershed context. Environ. Manag. 10, 199–214. doi: 10.1007/BF01867358
Fryirs, K. A. (2017). River sensitivity: a lost foundation concept in fluvial geomorphology. Earth Surf. Process. Landf. 42, 55–70. doi: 10.1002/esp.3940
Fryirs, K. A., Brierley, G. J., Preston, N. J., and Kasai, M. (2007). Buffers, barriers and blankets: the (dis)connectivity of catchment-scale sediment cascades. Catena 70, 49–67. doi: 10.1016/j.catena.2006.07.007
Fuller, C. C., and Harvey, J. W. (2000). Reactive uptake of trace metals in the hyporheic zone of a mining-contaminated stream, Pinal Creek, Arizona. Environ. Sci. Technol. 34, 1150–1155. doi: 10.1021/es990714d
Fullerton, A. H., Torgersen, C. E., Lawler, J. J., Faux, R. N., Steel, E. A., Beechie, T. J., et al. (2015). Rethinking the longitudinal stream temperature paradigm: region-wide comparison of thermal infrared imagery reveals unexpected complexity of river temperatures. Hydrol. Process. 29, 4719–4737. doi: 10.1002/hyp.10506
Galloway, J. N., Dentener, F. J., Capone, D. G., Boyer, E. W., Howarth, R. W., Seitzinger, S. P., et al. (2004). Nitrogen cycle: past, present, and future. Biogeochemistry 70, 153–226. doi: 10.1007/s10533-004-0370-0
Geiger, N., Swim, J., and Fraser, J. (2017). Creating a climate for change: interventions, efficacy and public discussion about climate change. J. Environ. Psychol. 51, 104–116. doi: 10.1016/j.jenvp.2017.03.010
Gerecht, K. E., Cardenas, M. B., Guswa, A. J., Sawyer, A. H., Nowinski, J. D., Swanson, T. E., et al. (2011). Dynamics of hyporheic flow and heat transport across a bed-to-bank continuum in a large regulated river. Water Resour. Res. 47, W03524. doi: 10.1029/2010WR009794
Gessner, M. O., and Chauvet, E. (1994). Importance of stream microfungi in controlling breakdown rates of leaf litter. Ecology 75, 1807–17. doi: 10.2307/1939639
Gilbert, G. K. (1876). The Colorado Plateau Province as a field for geological study. Am. J. Sci. 12, 16–24. 85–103. doi: 10.2475/ajs.s3-12.67.16
Gilbert, G. K. (1877). Report on the Geology of the Hendry Mountains. Washington, DC: US Geographical and Geological Survey of the Rocky Mountain Region, US Government Printing Office. doi: 10.5962/bhl.title.51652
Gilbert, G. K. (1917). Hydraulic-Mining Debris in the Sierra Nevada. Washington, DC: U.S. Geological Survey. p. 188. doi: 10.3133/pp105
Global Water Partnership (2000). Integrated Water Resources Management. Stockholm Sweden: Global Water Partnership Technical Advisory Committee.
Goddéris, Y., and Brantley, S. L. (2013). Earthcasting the future Critical Zone. Elementa 1, 1. doi: 10.12952/journal.elementa.000019
Gómez-Gener, L., Lupon, A., Laudon, H., and Sponseller, R. A. (2020). Drought alters the biogeochemistry of boreal stream networks. Nat. Commun. 11, 1–1. doi: 10.1038/s41467-020-15496-2
Gomez-Velez, J. D., and Harvey, J. W. (2014). A hydrogeomorphic river network model predicts where and why hyporheic exchange is important in large basins. Geophys. Res. Lett. 41, 6403–6412. doi: 10.1002/2014GL061099
Gomez-Velez, J. D., Harvey, J. W., Cardenas, M. B., and Kiel, B. (2015). Denitrification in the Mississippi River network controlled by flow through river bedforms. Nat. Geosci. 8, 941–945. doi: 10.1038/ngeo2567
Gooseff, M. N., McKnight, D. M., Lyons, W. B., and Blum, A. E. (2002). Weathering reactions and hyporheic exchange controls on stream water chemistry in a glacial meltwater stream in the McMurdo Dry Valleys. Water Resour. Res. 38, 1279. doi: 10.1029/2001WR000834
Graf, W. L. (1979). “Catastrophe theory as a model for change in fluvial systems,” in Adjustments of the Fluvial System, eds D. D. Rhodes, and G. P. Williams (London; Allen and Unwin), 13–32. doi: 10.4324/9781003026709-1
Graf, W. L. (2006). Downstream hydrologic and geomorphic effects of large dams on American rivers. Geomorphology 79, 336–360. doi: 10.1016/j.geomorph.2006.06.022
Grant, G. E., Schmidt, J. C., Lewis, S. L., and O'Connor, J. E. (2003). “A geological framework for interpreting downstream effects of dams on rivers,” in A Peculiar River: Geology, Geomorphology, and Hydrology of the Deschutes River, Oregon, eds G. E. Grant, and J. E. O'Connor (Washington, DC: American Geophysical Union Press), 203–219. doi: 10.1029/007WS13
Gregory, S., Swanson, F., McKee, W., and Cummins, K. (1991). An ecosystem perspective of riparian zones. Bioscience 41, 10.2307/1311607. doi: 10.2307/1311607
Griffiths, J. G. (1966). Hecataeus and Herodotus on “A Gift of the River.” J Near East. Stud. 25, 57–61. doi: 10.1086/371846
Grill, G., Lehner, B., Lumsdon, A. E., MacDonald, G. K., Zarfl, C., Liermann, C. R., et al. (2015). An index-based framework for assessing patterns and trends in river fragmentation and flow regulation by global dams at multiple scales. Environ. Res. Lett. 10, 015001. doi: 10.1088/1748-9326/10/1/015001
Grimm, N. B., Gergel, S. E., McDowell, W. H., Boyer, E. W., Dent, C. L., Groffman, P., et al. (2003). Merging aquatic and terrestrial perspectives of nutrient biogeochemistry. Oecologia 137, 485–501. doi: 10.1007/s00442-003-1382-5
Gurnell, A., Tockner, K., Edwards, P., and Petts, G. (2005). Effects of deposited wood on biocomplexity of river corridors. Front. Ecol. Environ. 3, 377–382. doi: 10.1890/1540-9295(2005)003[0377:EODWOB]2.0.CO;2
Gurnell, A. M., Corenblit, D., Garcia de Jalon, D., Gonzalez del Tanago, M., Grabowski, R. C., O'Hare, M. T., et al. (2016). A conceptual model of vegetation-hydrogeomorphology interactions within river corridors. River Resear. Appl. 32, 142–163. doi: 10.1002/rra.2928
Gutiérrez-Fonseca, P. E., Ramírez, A., Pringle, C. M., Torres, P. J., McDowell, W. H., Covich, A., et al. (2020). When the rainforest dries: drought effects on a montane tropical stream ecosystem in Puerto Rico. Freshw. Sci. 39, 197–212. doi: 10.1086/708808
Habersack, H., Haspel, D., and Kondolf, M. (2014). Large rivers in the Anthropocene: insights and tools for understanding climatic, land use, and reservoir influences. Water Resour. Res. 50, 3641–3646. doi: 10.1002/2013WR014731
Hack, J. T., and Goodlett, J. C. (1960). Geomorphology and forest ecology of a mountain region in the central Appalachians. US Geological Survey Professional Paper 347. Washington, DC. doi: 10.3133/pp347
Hanberry, B. B., Kabrick, J. M., and He, H. S. (2015). Potential tree and soil carbon storage in a major historical floodplain forest with disrupted ecological function. Perspect. Plant Ecol. Evol. Syst. 17, 17–23. doi: 10.1016/j.ppees.2014.12.002
Hansen, E. E. (1970). “Sediment movement in a pool and riffle stream,” in Proc. Wellington Symp. IAHS Publ. no. 96, 541–561.
Harding, J. S., Benfield, E. F., Bolstad, P. V., and Jones, E. B. D. (1998). Stream biodiversity: the ghost of land use past. Proc. Natl. Acad. Sci. U.S.A 95, 14843–14847. doi: 10.1073/pnas.95.25.14843
Harvey, J., Gomez-Velez, J., Schmadel, N., Scott, D., Boyer, E., Alexander, R., et al. (2019). How hydrologic connectivity regulates water quality in river corridors. J. Am. Water Resour. Assoc. 55, 369–381. doi: 10.1111/1752-1688.12691
Harvey, J., and Gooseff, M. (2015). River corridor science: hydrologic exchange and ecological consequences from bedforms to basins. Water Resour. Res. 50, 6893–6922. doi: 10.1002/2015WR017617
Harvey, J. W. (2016). “Hydrologic exchange flows and their ecological consequences in river corridors,” in Streams in a Changing Environment, eds J. Jones, and E. Stanley (London: Academic Press), 1–84. doi: 10.1016/B978-0-12-405890-3.00001-4
Harvey, J. W., and Bencala, K. E. (1993). The effect of streambed topography on surface-subsurface water exchange in mountain catchments. Water Resour. Res. 29, 89–98.
Harvey, J. W., and Fuller, C. C. (1998). Effect of enhanced manganese oxidation in the hyporheic zone on basin-scale geochemical mass balance. Water Resour. Res. 34, 623–636. doi: 10.1029/97WR03606
Harvey, J. W., and Schmadel, N. M. (2021). The river corridor's evolving connectivity of lotic and lentic waters. Front. Water 2, 580727. doi: 10.3389/frwa.2020.580727
Harvey, J. W., Wagner, B. J., and Bencala, K. E. (1996). Evaluating the reliability of the stream tracer approach to characterize stream-subsurface water exchange. Water Resour. Res. 32, 2441–2451. doi: 10.1029/96WR01268
Hassan, M. A., Gottesfeld, A. S., Montgomery, D. R., Tunnicliffe, J. F., Clarke, K. C., Wynn, G., et al. (2008). Salmon-drive bed load transport and bed morphology in mountain streams. Geophys. Resear. Lett. 35, L04405. doi: 10.1029/2007GL032997
Hatchett, B. J., Koshkin, A. L., Guirguis, K., Rittger, K., Nolin, A. W., Heggli, A., et al. (2022). Midwinter dry spells amplify post-fire snowpack decline. Geophys. Resear. Lett. 50, e2022GL101235. doi: 10.1002/essoar.10512494.1
Hayer, M., Wymore, A. S., Schwartz, E., Koch, B. J., Hungate, B. A., Marks, J. C., et al. (2021). Microbes on decomposing litter in streams: entering on the leaf or colonizing in the water? ISME J. 16, 717–725. doi: 10.1038/s41396-021-01114-6
Hedin, L. O., von Fischer, J. C., Ostrom, N. E., Kennedy, B. P., Brown, M. G., Robertson, G. P., et al. (1998). Thermodynamic constraints on nitrogen transformations and other biogeochemical processes at soil-stream interfaces Ecology 79, 684–703. doi: 10.1890/0012-9658(1998)079[0684:TCONAO]2.0.CO;2
Heffernan, J. B. (2008). Wetlands as an alternative stable state in desert streams. Ecology 89, 1261–1271. doi: 10.1890/07-0915.1
Heffernan, J. B., Soranno, P. A., Angilletta, M. J., Buckley, L. B., Gruner, D. S., Keitt, T. H., et al. (2014). Macrosystems ecology: understanding ecological patterns and processes at continental scales. Front. Ecol. Environ. 12, 5–14. doi: 10.1890/130017
Herzog, S. P., Eisenstein, W. A., Halpin, B. N., Portmann, A. C., Fitzgerald, N. J. M., Ward, A. S., et al. (2019). Co-design of engineered hyporheic zones to improve in-stream stormwater treatment and facilitate regulatory approval. Water 11, 2543. doi: 10.3390/w11122543
Herzog, S. P., Higgins, C. P., and McCray, J. E. (2015). Engineered streambeds for induced hyporheic flow: enhanced removal of nutrients, pathogens, and metals from urban streams. J Environ. Eng. 142, 04015053. doi: 10.1061/(ASCE)EE.1943-7870.0001012
Hester, E. T., and Gooseff, M. N. (2010). Moving beyond the banks: hyporheic restoration is fundamental to restoring ecological services and functions of streams. Environ. Sci. Technol. 44, 1521–1525. doi: 10.1021/es902988n
Hille, S., Kristensen, E. A., Graeber, D., Riis, T., Jørgensen, N. K., Baattrup-Pedersen, A., et al. (2014). Fast reaction of macroinvertebrate communities to stagnation and drought in streams with contrasting nutrient availability. Freshw. Sci. 33, 847–859. doi: 10.1086/677554
Hillebrand, H., and Kunze, C. (2020). Meta-analysis on pulse disturbances reveals differences in functional and compositional recovery across ecosystems. Ecol. Lett. 23, 575–585. doi: 10.1111/ele.13457
Hillebrand, H., Langenheder, S., Lebret, K., Lindström, E., Östman, Ö., Striebel, M., et al. (2017). Decomposing multiple dimensions of stability in global change experiments. Ecol. Lett. 21, 21–30. doi: 10.1111/ele.12867
Hilton, R. G., Galy, A., Hovius, N., Chen, M. C., Horng, M. J., Chen, H., et al. (2008). Tropical-cyclone-driven erosion of the terrestrial biosphere from mountains. Nat. Geosci. 1, 759–762. doi: 10.1038/ngeo333
Holling, C. S. (1973). Resilience and stability of ecological systems. Ann. Rev. Ecol. Syst. 4, 1–24. doi: 10.1146/annurev.es.04.110173.000245
Horn, H. S. (1975). “Markovian processes of forest succession,” in Ecology and Evolution of Communities, eds M. L. Cody, and J. M. Diamond (McKenzie Bridge, OR: Belknap), 196–213.
Hotchkiss, E. R., Hall, R. O. Jr., Sponseller, R. A., Butman, D., Klaminder, J, Laudon, H., et al. (2015). Sources of and processes controlling CO2 emissions changes with the size of streams and rivers. Nat. Geosci. 8, 696–699. doi: 10.1038/ngeo2507
Humphries, P., Keckeis, H., and Finlayson, B. (2014). The river wave concept: integrating river ecosystem models. Bioscience 64, 870–882. doi: 10.1093/biosci/biu130
Hynes, H. B. (1983). Groundwater and stream ecology. Hydrobiologia 100, 93–99. doi: 10.1007/BF00027424
Hynes, H. B. N. (1975). The stream and its valley. Int. Ver. Theor. Angew. Limnol. 19, 1–15. doi: 10.1080/03680770.1974.11896033
Ibarra, D. E., Moon, S., Caves, J. K., Chamberlain, C. P., and Maher, K. (2017). Concentration-discharge patterns of weathering products from global rivers. Acta Geochim. 36, 405–409. doi: 10.1007/s11631-017-0177-z
ICIMOD (2023). River Corridors and the Chryosphere. Available online at: https://www.icimod.org/regional-programme/river-basins-and-cryosphere/ (accessed January 18, 2023).
Jackson, T. R., Haggerty, R., and Apte, S. V. (2013). A fluid-mechanics based classification scheme for surface transient storage in riverine environments: quantitatively separating surface from hyporheic transient storage. Hydrol. Earth Syst. Sci. 17, 2747–2779. doi: 10.5194/hess-17-2747-2013
James, L. A. (1991). Incision and morphologic evolution of an alluvial channel recovering from hydraulic mining sediment. Geol. Soc. Am. Bull. 103, 723–736. doi: 10.1130/0016-7606(1991)103<0723:IAMEOA>2.3.CO;2
James, L. A. (2013). Legacy sediment: definitions and processes of episodically produced anthropogenic sediment. Anthropocene 2, 16–26. doi: 10.1016/j.ancene.2013.04.001
James, L. A., and Marcus, W. A. (2006). The human role in changing fluvial systems: retrospect, inventory and prospect. Geomorphology 79, 152–171. doi: 10.1016/j.geomorph.2006.06.017
Jasechko, S., Kirchner, J., Welker, J., and McDonnell, J. (2016). Substantial proportion of global streamflow less than three months old. Nat. Geosci. 9, 126–129. doi: 10.1038/ngeo2636
Jasechko, S., Seybold, H., Perrone, D., Fan, Y., and Kirchner, J. (2021). Widespread potential loss of streamflow into underlying aquifers across the USA. Nature 591, 391–395. doi: 10.1038/s41586-021-03311-x
Jenny, H. (1941). Factors of Soil Formation—A System of Quantitative Pedology. New York, NY: McGraw-Hall, 281.
John, S., and Klein, A. (2004). Hydrogeomorphic effects of beaver dams on floodplain morphology: avulsion processes and sediment fluxes in upland valley floors (Spessart, Germany). Quaternaire 15, 219–231. doi: 10.3406/quate.2004.1769
Johnson, M. F., Rice, S. P., and Reid, I. (2011). Increase in coarse sediment transport associated with disturbance of gravel river beds by signal crayfish (Pacifastacus leniusculus). Earth Surf. Process. Landf. 36, 1680–1692. doi: 10.1002/esp.2192
Jolly, W. M., Cochrane, M. A., Freeborn, P. H., Holden, Z. A., Brown, T. J., Williamson, G. J., et al. (2015). Climate-induced variations in global wildfire danger from 1979 to 2013. Nat. Commun. 6, 7537. doi: 10.1038/ncomms8537
Jones, L. S., and Schumm, S. A. (1999). “Causes of avulsion: an overview,” in Fluvial Sedimentology VI, eds N. D. Smith, and J. Rogers (Oxford: International Association of Sedimentologists), 169–178. doi: 10.1002/9781444304213.ch13
Jones, S. E., and Lennon, J. T. (2010). Dormancy contributes to the maintenance of microbial diversity. Proc. Natl. Acad. Sci. U.S.A 107, 5881–5886. doi: 10.1073/pnas.0912765107
Junk, W. J., Bayley, P. B., and Sparks, R. E. (1989). The flood pulse concept in river-floodplain systems. Canadian Special Publication of Fisheries and Aquatic Sciences.
Junk, W. J., and Wantzen, K. M. (2006). “Flood pulsing and the development and maintenance of biodiversity in floodplains,” in Ecology of Freshwater and Estuarine Wetlands, eds D. P. Batzer, and R. R. Sharitz (Berkeley, CA: University of California Press), 407–435. doi: 10.1525/california/9780520247772.003.0011
Kendy, E., Apse, C., and Blann, K. (2012). A Practical Guide to Environmental Flows for Policy and Planning. The Nature Conservancy. Arlington, VA, 72.
King, J., Brown, C., and Sabet, H. (2003). A scenario-based holistic approach to environmental flow assessment for rivers. River Resear. Appl. 19, 619–639. doi: 10.1002/rra.709
Kirchner, J. W. (2009). Catchments as simple dynamical systems: catchment characterization, rainfall-runoff modeling, and doing hydrology backward. Water Resour. Res. 45. doi: 10.1029/2008WR006912
Kirchner, J. W., Feng, X., and Neal, C. (2001). Catchment-scale advection and dispersion as a mechanism for fractal scaling in stream tracer concentrations. J. Hydrol. 254, 82–101. doi: 10.1016/S0022-1694(01)00487-5
Knox, J. C. (1972). Valley alluviation in southwestern Wisconsin. Ann. Assoc. Am. Geograph. 62, 401–410. doi: 10.1111/j.1467-8306.1972.tb00872.x
Knox, J. C. (2001). Agricultural influence on landscape sensitivity in the Upper Mississippi River valley. Catena 42, 193–224. doi: 10.1016/S0341-8162(00)00138-7
Knox, R. L., Morrison, R. R., and Wohl, E. E. (2022). Identification of artificial levees in the contiguous United States. Water Resour. Res. 58, e2021WR031308. doi: 10.1029/2021WR031308
Koehnken, L., Rintoul, M. S., Goichot, M., Tickner, D., Loftus, A.-C., Acreman, M. C., et al. (2020). Impacts of riverine sand mining on freshwater ecosystems: a review of the scientific evidence and guidance for future research. River Res Appl. 36, 362–370. doi: 10.1002/rra.3586
Kondolf, G. M. (2006). River restoration and meanders. Ecol. Soc. 11, 42. doi: 10.5751/ES-01795-110242
Kondolf, G. M., Smeltzer, M. W., and Railsback, S. F. (2001). Design and performance of a channel reconstruction project in a coastal California gravel-bed stream. Environ. Manag. 28, 761–776. doi: 10.1007/s002670010260
Krause, S., Abbott, B. W., Baranov, V., Bernal, S., Blaen, P., Datry, T., et al. (2022). Organizational principles of hyporheic exchange flow and biogeochemical cycling in river networks across scales. Water Resour. Res. 58, e2021WR029771. doi: 10.1029/2021WR029771
Kumar, P., Le, P. V., Papanicolaou, A. T., Rhoads, B. L., Anders, A. M., Stumpf, A., et al. (2018). Critical transition in critical zone of intensively managed landscapes. Anthropocene 22, 10–19. doi: 10.1016/j.ancene.2018.04.002
Lake, P. S. (2000). Disturbance, patchiness, and diversity in streams. J. North Am. Benthol. Soc. 19, 573–592. doi: 10.2307/1468118
Lake, P. S. (2003). Ecological effects of perturbation by drought in flowing waters. Freshw. Biol. 48, 1161–1172. doi: 10.1046/j.1365-2427.2003.01086.x
Lake, P. S. (2011). Drought and Aquatic Ecosystems: Effects and Responses. Hoboken, NJ: John Wiley and Sons. doi: 10.1002/9781444341812
Lane, E. W. (1955). The importance of fluvial morphology in hydraulic engineering. ASCE Proc. Separate 81, 1–17.
Langbein, W. B. (1964). Geometry of river channels. ASCE J. Hydraul. Div. 90, 301–312. doi: 10.1061/JYCEAJ.0001019
Langbein, W. B., and Leopold, L. B. (1964). Quasi-equilibrium states in channel morphology. Am. J. Sci. 262, 782–794. doi: 10.2475/ajs.262.6.782
Larsen, L. G., Choi, J., Nungesser, M. K., and Harvey, J. W. (2012). Directional connectivity in hydrology and ecology. Ecol. Appl. 22, 2204–2220. doi: 10.1890/11-1948.1
Larsen, L. G., Harvey, J. W., and Crimaldi, J. P. (2007). A delicate balance: ecohydrological feedbacks governing landscape morphology in a lotic peatland. Ecol. Monogr. 77, 591–614. doi: 10.1890/06-1267.1
Le Lay, Y. F., Piegay, H., and Riviere-Honegger, A. (2013). Perception of braided river landscapes: implications for public participation and sustainable management. J. Environ. Manag. 119, 1–12. doi: 10.1016/j.jenvman.2013.01.006
Lee, R. M., Shoshitaishvili, B., Buck, R. L., Bekker, J., and Abbott, B. W. (2023). The meanings of the Critical Zone. Anthropocene 16, 100377. doi: 10.1016/j.ancene.2023.100377
Leopold, L. B., and Maddock, T. (1953). The hydraulic geometry of stream channels and some physiographic implications. U.S. Geological Survey Professional Paper 252, Washington, DC. doi: 10.3133/pp252
Leopold, L. B., and Wolman, M. G. (1957). River channel patterns: braided, meandering and straight. U.S. Geological Survey Professional Paper 282-B, Washington, DC. doi: 10.3133/pp282B
Leopold, L. B., Wolman, M. G., and Miller, J. P. (1964). Fluvial Processes in Geomorphology. San Francisco, CA: WH Freeman and Company.
Lepori, F., Palm, D., Brannas, E., and Malmqvist, B. (2005). Does restoration of structural heterogeneity in streams enhance fish and macroinvertebrate diversity? Ecol. Appl. 15, 2060–2071. doi: 10.1890/04-1372
Lewontin, R. C. (1969). The meaning of stability. In: Diversity and Stability of Ecological Systems. Brookhaven Symp. Biol. 22, 13–24.
Li, L., Maher, K., Navarre-Sitchler, A., Druhan, J., Meile, C., Lawrence, C., et al. (2017). Expanding the role of reactive transport models in critical zone processes. Earth-Sci. Rev. 165, 280–301. doi: 10.1016/j.earscirev.2016.09.001
Li, L., Sullivan, P., Benettin, P., Cirpka, O. A., Bishop, K., Brantley, S., et al. (2021). Toward catchment hydro-biogeochemical theories. WIREs Water 8, e1495. doi: 10.1002/wat2.1495
Likens, G. E., and Bormann, F. H. (1974). Linkages between terrestrial and aquatic ecosystems. Bioscience 24, 447–456. doi: 10.2307/1296852
Likens, G. E., and Buso, D. C. (2006). Variation in streamwater chemistry throughout the Hubbard Brook Valley. Biogeochemistry 78, 1–30. doi: 10.1007/s10533-005-2024-2
Liu, F., Yang, Q., Chen, S., Luo, Z., Yuan, F., Wang, R., et al. (2014). Temporal and spatial variability of sediment flux into the sea from the three largest rivers in China. J. Asian Earth Sci. 87, 102–115. doi: 10.1016/j.jseaes.2014.02.017
Livers, B., Wohl, E., Jackson, K. J., and Sutfin, N. A. (2018). Historical land use as a driver of alternative states for stream form and function in forested mountain watersheds of the Southern Rocky Mountains. Earth Surf. Process. Landf. 43, 669–684. doi: 10.1002/esp.4275
Macklin, M. G. (1999). Holocene river environments in prehistoric Britain: human interaction and impact. Quat. Proc. 7, 521–530. doi: 10.1002/(SICI)1099-1417(199910)14:6<521::AID-JQS487>3.0.CO;2-G
Magilligan, F. J., and Nislow, K. H. (2005). Changes in hydrologic regime by dams. Geomorphology 71, 61–78. doi: 10.1016/j.geomorph.2004.08.017
Magliozzi, C., Grabowski, R. C., Packman, A. I., and Krause, S. (2018). Toward a conceptual framework of hyporheic exchange across spatial scales. Hydrol. Earth Syst. Sci. 22, 6163–6185. doi: 10.5194/hess-22-6163-2018
Marinos, R. E., Van Meter, K. J., and Basu, N. B. (2020). Is the river a chemostat?: scale vs. land use controls on nitrate concentration-discharge dynamics in the Upper Mississippi River basin. Geophys. Resear. Lett. 47, e2020GL087051. doi: 10.1029/2020GL087051
Marks, J. C., Power, M. E., and Parker, M. S. (2000). Flood disturbance, algal productivity, and interannual variation in food chain length. Oikos 90, 20–27. doi: 10.1034/j.1600-0706.2000.900103.x
Mattingly, R. L., Herricks, E. E., and Johnston, D. M. (1993). Channelization and levee construction in Illinois: review and implications for management. Environ. Manag. 17, 781–795. doi: 10.1007/BF02393899
May, R. M. (1977). Thresholds and breakpoints in ecosystems with a multiplicity of stable states. Nature 269, 471–477. doi: 10.1038/269471a0
McClain, M. E., Boyer, E. W., Dent, C. L., Gergel, S. E., Grimm, N. B., Groffman, P. M., et al. (2003). Biogeochemical hot spots and hot moments at the interface of terrestrial and aquatic ecosystems. Ecosystems 6, 301–312. doi: 10.1007/s10021-003-0161-9
McCluney, K. E., Poff, N. L., Palmer, M. A., Thorp, J. H., Poole, G. C., Williams, B. S., et al. (2014). Riverine macrosystems ecology: sensitivity, resistance, and resilience of whole river basins with human alterations. Front. Ecol. Environ. 12, 48–58. doi: 10.1890/120367
McDowell, W. H., Brereton, R. L., Scatena, F. N., Shanley, J. B., Brokaw, N. V., Lugo, A. E., et al. (2013). Interactions between lithology and biology drive the long-term response of stream chemistry to major hurricanes in a tropical landscape. Biogeochemistry 116, 175–186. doi: 10.1007/s10533-013-9916-3
McIntosh, J. C., Schaumberg, C., Perdrial, J., Harpold, A., Vázquez-Ortega, A., Rasmussen, C., et al. (2017). Geochemical evolution of the Critical Zone across variable time scales informs concentration-discharge relationships: jemez River Basin Critical Zone Observatory. Water Resour. Res. 53, 4169–4196. doi: 10.1002/2016WR019712
Meade, R. H., Yuzyk, T. R., and Day, T. J. (1990). “Movement and storage of sediment in rivers of the United States and Canada,” in Surface Water Hydrology. Geology of North America Series, vol. O-1, eds M. G. Wolman, and H. C. Riggs (Boulder, CO: Geological Society of America), 255–280. doi: 10.1130/DNAG-GNA-O1.255
Mei-e, R., and Xianmo, Z. (1994). Anthropogenic influences on changes in the sediment load of the Yellow River, China, during the Holocene. Holocene 4, 314–320. doi: 10.1177/095968369400400311
Meyer, J. L., and Likens, G. E. (1979). Transport and transformation of phosphorus in a forest stream ecosystem. Ecology 60, 1255–1269. doi: 10.2307/1936971
Miller, G. T., and Spoolman, S. (2021). Living in the Environment, 20E. ? Boston, MA: Cengage Learning, 832.
Milly, P. C. D., Betancourt, J., Falkenmark, M., Hirsch, R. M., Kundzewicz, Z. W., Lettenmaier, D. P., et al. (2008). Stationarity is dead: whither water management? Science 319, 573–574. doi: 10.1126/science.1151915
Mirtl, M., Borer, E. T., Djukic, I., Forsius, M., Haubold, H., Hugo, W., et al. (2018). Genesis, goals and achievements of long-term ecological research at the global scale: a critical review of ILTER and future directions. Sci. Total Environ. 626, 1439–1462. doi: 10.1016/j.scitotenv.2017.12.001
Montgomery, D. R. (1999). Process domains and the river continuum. J. Am. Water Resour. Assoc. 35, 397–410. doi: 10.1111/j.1752-1688.1999.tb03598.x
Montgomery, D. R., and Buffington, J. M. (1997). Channel-reach morphology in mountain drainage basins. Geol. Soc. Am. Bull. 109, 596–611. doi: 10.1130/0016-7606(1997)109<0596:CRMIMD>2.3.CO;2
Montgomery, D. R., Collins, B. D., Buffington, J. M., and Abbe, T. B. (2003). “Geomorphic effects of wood in rivers,” in The Ecology and Management of Wood in World Rivers, eds S. V. Gregory, K. L. Boyer, A. M. Gurnell. American Fisheries Society Symposium 37, (Bethesda, MA: American Fisheries Society), 21–47.
Moore, J. W. (2006). Animal ecosystem engineers in streams. Bioscience 56, 237–246. doi: 10.1641/0006-3568(2006)056[0237:AEEIS]2.0.CO;2
Morgan, P., Aplet, G. H., Haufler, J. B., Humphries, H. C., Moore, M. M., Wilson, W. D., et al. (1994). Historical range of variability. J. Sustain. For. 2, 87–111. doi: 10.1300/J091v02n01_04
Mulholland, P. J., Helton, A. M., Poole, G. C., Hall, R. O., Hamilton, S. K., Peterson, B. J., et al. (2008). Stream denitrification across biomes and its response to anthropogenic nitrate loading. Nature 452, 202–205. doi: 10.1038/nature06686
Naiman, R., and Décamps, H. (1997). The ecology of interfaces: riparian zones. Ann. Rev. Ecol. Syst. 28, 621–658. doi: 10.1146/annurev.ecolsys.28.1.621
Naiman, R. J., Bilby, R. E., Schindler, D. E., and Helfield, J. M. (2002). Pacific salmon, nutrients, and the dynamics of freshwater and riparian ecosystems. Ecosystems 5, 399–417. doi: 10.1007/s10021-001-0083-3
Nanson, G. C., and Croke, J. C. (1992). A genetic classification of floodplains. Geomorphology 4, 459–486. doi: 10.1016/0169-555X(92)90039-Q
Nanson, G. C., and Huang, H. Q. (2008). Least action principle, equilibrium states, iterative adjustment and the stability of alluvial channels. Earth Surface Process. Landforms 33, 923–942. doi: 10.1002/esp.1584
National Research Council. (2001). Basic Research Opportunities in Earth Science. Washington, DC: National Academic Press.
Needleman, H. L. (1998). Clair Patterson and Robert Kehoe: two views of lead toxicity. Environ. Resear. Section A 78, 79–85. doi: 10.1006/enrs.1997.3807
Nepf, H. M. (1999). Drag, turbulence, and diffusion in flow through emergent vegetation. Water Res. Resear. 35, 479–489. doi: 10.1029/1998WR900069
Newbold, J. D., O'Neill, R. V., Elwood, J. W., and Van Winkle, W. (1982). Nutrient spiraling in streams: implications for nutrient limitation and invertebrate activity. Am. Nat. 120, 628–652. doi: 10.1086/284017
Odum, H. T. (1956). Primary production in flowing waters. Limnol. Oceanograph. 1, 102–117. doi: 10.4319/lo.1956.1.2.0102
Odum, H. T. (1957). Trophic structure and productivity of Silver Springs, Florida. Ecol. Monograph. 27, 55–112. doi: 10.2307/1948571
Orghidan, T. (1959). Ein neuer Lebensraum des unterirdischen Wassers: der hyporheische Biotop. Arch. Hydrobiol. 55, 392–414.
Orme, A. R. (2013). “The scientific roots of geomorphology before 1830,” in Treatise on Geomorphology, vol. 1, ed J. Shroder (The foundations of geomorphology, eds A. R. Orme, and D. Sack) (San Diego, CA: Academic Press), 11–36. doi: 10.1016/B978-0-12-374739-6.00002-6
Packman, I. I., Packman, A. I., and Brooks, N. H. (2001). Hyporheic exchange of solutes and colloids with moving bed forms. Water Resour. Res. 37, 2591–2605.
Palmer, M. A., Liermann, C. A. R., Nilsson, C., Florke, M., Alcamo, J., Lake, P. S., et al. (2008). Climate change and the world's river basins: anticipating management options. Front. Ecol. Environ. 6, 81–89. doi: 10.1890/060148
Palmer, M. A., Menninger, H. L., and Bernhardt, E. (2010). River restoration, habitat heterogeneity and biodiversity: a failure of theory or practice? Freshw. Biol. 55, 205–222. doi: 10.1111/j.1365-2427.2009.02372.x
Parsons, M., and Thoms, M. C. (2018). From academic to applied: operationalising resilience in river systems. Geomorphology 305, 242–251. doi: 10.1016/j.geomorph.2017.08.040
Peipoch, M., Brauns, M., Hauer, F. R., Weitere, M., and Valett, H. M. (2015). Ecological simplification: human influences on riverscape complexity. Bioscience 65, 1057–1065. doi: 10.1093/biosci/biv120
Peterjohn, W. T., and Correll, D. L. (1984). Nutrient dynamics in an agricultural watershed: observations on the role of a riparian forest. Ecology 65, 1466–1475. doi: 10.2307/1939127
Peterson, B. J., Wollheim, W. M., Mulholland, P. J., Webster, J. R., Meyer, J. L., Tank, J. L., et al. (2001). Control of nitrogen export from watersheds by headwater streams. Science 292, 86–90. doi: 10.1126/science.1056874
Petsch, D. K. (2016). Causes and consequences of biotic homogenization in freshwater aquatic ecosystems. Int. Rev. Hydrobiol. 101, 113–122. doi: 10.1002/iroh.201601850
Piegay, H., Gregory, K. J., Bondarev, V., Chin, A., Dahlstrom, N., Elosegi, A., et al. (2005). Public perception as a barrier to introducing wood in rivers for restoration purposes. Environ. Manag. 36, 665–674. doi: 10.1007/s00267-004-0092-z
Playfair, J. (1802). Illustration of the Huttonian Theory. Edinburgh: Cadell and Davies. doi: 10.5962/bhl.title.50752
Poff, N., Brown, C., Grantham, T., Mendoza, G., Dominique, K., Haasnoot, M., et al. (2015). Sustainable water management under future uncertainty with eco-engineering decision scaling. Nat. Clim. Change 6, 25–34, doi: 10.1038./NCLIMATE2765
Poff, N. L., Allan, J. D., Bain, M. B., Karr, J. R., Prestegaard, K. L., Richter, B. D., et al. (1997). The natural flow regime. Bioscience 47, 769–784. doi: 10.2307/1313099
Poff, N. L., Olden, J. D., Merritt, D. M., and Pepin, D. M. (2007). Homogenization of regional river dynamics by dams and global biodiversity implications. Proc. Natl. Acad. Sci. U.S.A. 104, 5732–5737. doi: 10.1073/pnas.0609812104
Poff, N. L., Richter, B. D., Arthington, A. H., Bunn, S. E., Naiman, R. J., et al. (2010). The ecological limits of hydrologic alteration (ELOHA): a new framework for developing regional environmental flow standards. Freshw. Biol. 55, 141–170. doi: 10.1111/j.1365-2427.2009.02204.x
Polvi, L. E., and Wohl, E. (2012). The beaver meadow complex revisited – the role of beavers in post-glacial floodplain development. Earth Surf. Process. Landf. 37, 332–346. doi: 10.1002/esp.2261
Polvi, L. E., and Wohl, E. (2013). Biotic drivers of stream planform: implications for understanding the past and restoring the future. Bioscience 63, 439–452. doi: 10.1525/bio.2013.63.6.6
Poole, G., Stanford, J., Running, S., and Frissell, C. (2006). Multiscale geomorphic drivers of groundwater flow paths: subsurface hydrologic dynamics and hyporheic habitat diversity. J. North Am. Benthol. Soc. 25, 288–303. doi: 10.1899/0887-3593(2006)25[288:MGDOGF]2.0.CO;2
Poole, G. C. (2002). Fluvial landscape ecology: addressing uniqueness within the river discontinuum. Freshw. Biol. 47, 641–660. doi: 10.1046/j.1365-2427.2002.00922.x
Powell, J. W. (1875). Exploration of the Colorado River of the West. US Government Printing Office, Washington, DC.
Pringle, C. (2003). What is hydrologic connectivity and why is it ecologically important? Hydrol. Process. 17, 2685–2689. doi: 10.1002/hyp.5145
Pujari, P. R., Jain, V., Singh, V., Sreelash, K., Dhyani, S., Nema, M., et al. (2020). Critical zone: an emerging research area for sustainability. Current Sci. 118, 1487–1488.
Qi, W., Li, H., Zhang, Q., and Zhang, K. (2019). Forest restoration efforts drive changes in land-use/land-cover and water-related ecosystem services in China's Han River basin. Ecol. Eng. 126, 64–73. doi: 10.1016/j.ecoleng.2018.11.001
Rahel, F. J. (2002). Homogenization of freshwater faunas. Ann. Rev. Ecol. Syst. 33, 291–315. doi: 10.1146/annurev.ecolsys.33.010802.150429
Rasmussen, C., Troch, P. A., Chorover, J., Brooks, P., Pelletier, J., Huxman, T. E., et al. (2011). An open system energy-based framework for predicting critical zone structure and function. Biogeochemistry 102, 15–29. doi: 10.1007/s10533-010-9476-8
Raymond, P. A., David, M. B., and Saiers, J. E. (2012). The impact of fertilization and hydrology on nitrate fluxes from Mississippi watersheds. Curr. Opin. Environ. Sust. 4, 212–218. doi: 10.1016/j.cosust.2012.04.001
Raymond, P. A., and Saiers, J. E. (2010). Event controlled DOC export from forested watersheds. Biogeochemistry 100, 197–209. doi: 10.1007/s10533-010-9416-7
Raymond, P. A., Saiers, J. E., and Sobczak, W. V. (2016). Hydrological and biogeochemical controls on watershed dissolved organic matter transport: pulse-shunt concept. Ecology 97, 5–16. doi: 10.1890/14-1684.1
Read, D. S., Gweon, H. S., Bowes, M. J., Newbold, L. K., Field, D., Bailey, M. J., et al. (2015). Catchment-scale biogeography of riverine bacterioplankton. ISME J. 9, 516–526. doi: 10.1038/ismej.2014.166
Resh, V. H., Brown, A. V., Covich, A. P., Gurtz, M. E., Li, H. W., Minshall, G. W., et al. (1988). The role of disturbance in stream ecology. J. North Am. Benthol. Soc. 7, 433–455. doi: 10.2307/1467300
Rice, S. P. (2017). Tributary connectivity, confluence aggradation and network biodiversity. Geomorphology 277, 6–16. doi: 10.1016/j.geomorph.2016.03.027
Richardson, M., and Kumar, P. (2017). Critical zone services as environmental assessment criteria in intensively managed landscapes. Earth's Future 5, 617–632. doi: 10.1002/2016EF000517
Richter, B. D., Baumgartner, J. V., Powell, J., and Braun, D. P. (1996). A method for assessing hydrologic alteration within ecosystems. Conserv. Biol. 10, 1163–1174. doi: 10.1046/j.1523-1739.1996.10041163.x
Rigon, R., Rinaldo, A., Rodriguez-Iturbe, I., Bras, R. L., and Ijjasz-Vasquez, E. (1993). Optimal channel networks: a framework for the study of river basin morphology. Water Resour. Res. 29, 1635–1646. doi: 10.1029/92WR02985
Robertson, A. I., Bunn, S. E., Boon, P. I., and Walker, K. F. (1999). Sources, sinks and transformations of organic carbon in Australian floodplain rivers. Mar. Freshw. Res. 50, 813–829. doi: 10.1071/MF99112
Roebuck, J. A., Bladon, K. D., Donahue, D., Graham, E. B., Grieger, S., Morgenstern, K., et al. (2022). Spatiotemporal controls on the delivery of dissolved organic matter to streams following a wildfire. Geophys. Res. Lett. 49, e2022GL099535. doi: 10.1029/2022GL099535
Rohde, S., Hostmann, M., Peter, A., and Ewald, K. C. (2006). Room for rivers: an integrative search strategy for floodplain restoration. Landsc. Urban Plan. 78, 50–70. doi: 10.1016/j.landurbplan.2005.05.006
Roley, S. S., Tank, J. L., Stephen, M. L., Johnson, L. T., Beaulieu, J. J., Witter, J. D., et al. (2012). Floodplain restoration enhances denitrification and reach-scale nitrogen removal in an agricultural stream. Ecol. Appl. 22, 281–297. doi: 10.1890/11-0381.1
Rubinstein, R. L., Borton, M. A., Zhou, H., Shaffer, M., Hoyt, D. W., Stegen, J., et al. (2022). ORT: a workflow linking genome-scale metabolic models with reactive transport codes. Bioinformatics 38, 778–84. doi: 10.1093/bioinformatics/btab753
Ruffing, C. M., Daniels, M. D., and Dwire, K. A. (2015). Disturbance legacies of historic tie-drives persistently alter geomorphology and large wood characteristics in headwater streams, southeast Wyoming. Geomorphology 231, 1–14. doi: 10.1016/j.geomorph.2014.10.029
Runkel, R. L. (2007). Toward a transport-based analysis of nutrient spiraling and uptake in streams. Limnol. Oceanogr. Methods 5, 50–62. doi: 10.4319/lom.2007.5.50
Sack, D., and Orme, A. R. (2013). “Introduction to the foundations of geomorphology,” in Treatise on Geomorphology, v. 1, ed J. Shroder (The Foundations of Geomorphology, eds A. R. Orme, and D. Sack) (San Diego, CA: Academic Press), 1–10. doi: 10.1016/B978-0-12-374739-6.00001-4
Savant, S. A., Reible, D. D., and Thibodeaux, L. J. (1987). Convective transport within stable river sediments. Water Resour. Res. 23, 1763–1768. doi: 10.1029/WR023i009p01763
Savio, D., Sinclair, L., Ijaz, U. Z., Parajka, J., Reischer, G. H., Stadler, P., et al. (2015). Bacterial diversity along a 2600 km river continuum. Environ. Microbiol. 17, 4994–5007. doi: 10.1111/1462-2920.12886
Schaefer, D. A., McDowell, W. H., Scatena, F. N., and Asbury, C. E. (2000). Effects of hurricane disturbance on stream water concentrations and fluxes in eight tropical forest watersheds of the Luquillo Experimental Forest, Puerto Rico. J. Trop. Ecol. 16, 189–207. doi: 10.1017/S0266467400001358
Scheffer, M., Carpenter, S. R., Foley, J. A., Folke, C., and Walker, B. (2001). Catastrophic shifts in ecosystems. Nature 413, 591–596. doi: 10.1038/35098000
Schmadel, N. M., Harvey, J. W., Alexander, R. M., Boyer, E. W., Schwarz, G. E., Gomez-Velez, J. D., et al. (2020). Low threshold for nitrogen concentration saturation in headwaters increases regional and coastal delivery. Environ. Res. Lett. 15, 044018. doi: 10.1088/1748-9326/ab751b
Schmadel, N. M., Harvey, J. W., and Schwarz, G. E. (2021). Seasonally dynamic nutrient modeling quantifies storage lags and time-varying reactivity across large river basins. Environ. Res. Lett. 16, 095004. doi: 10.1088/1748-9326/ac1af4
Schmadel, N. M., Harvey, J. W., Schwarz, G. E., Alexander, R. B., Gomez-Velez, J. D., Scott, D., et al. (2019). Small ponds in headwater catchments are a dominant influence on regional nutrient and sediment budgets. Geophy. Resear. Lett. 46, 9669–9677. doi: 10.1029/2019GL083937
Schmidt, J. C., and Wilcock, P. R. (2008). Metrics for assessing the downstream effects of dams. Water Resour. Res. 44, W04404. doi: 10.1029/2006WR005092
Scholl, M. A., Shanley, J. B., Murphy, S. F., Willenbring, J. K., Occhi, M., González, G., et al. (2015). Stable-isotope and solute-chemistry approaches to flow characterization in a forested tropical watershed, Luquillo Mountains, Puerto Rico. Appl. Geochem. 63, 484–497. doi: 10.1016/j.apgeochem.2015.03.008
Schumm, S. A. (1969). River metamorphosis. ASCE J. Hydraul. Div. 95, 255–274. doi: 10.1061/JYCEAJ.0001938
Schumm, S. A. (1973). “Geomorphic thresholds and complex response of drainage systems,” in Fluvial Geomorphology, ed M. Morisawa (Binghamton, NY: State University of New York), 299–310.
Schumm, S. A. (1981). “Evolution and response of the fluvial system, sedimentologic implications,” in Society of Economic Paleontologists and Mineralogists Special Publication 31, 19–29. doi: 10.2110/pec.81.31.0019
Scott, D. T., Gomez-Velez, J. D., Jones, C. N., and Harvey, J. W. (2019). Floodplain inundation spectrum across the United States. Nat. Commun. 10, 5194. doi: 10.1038/s41467-019-13184-4
Seitzinger, S. P., Mayorga, E., Bouwman, A. F., Kroeze, C., Beusen, A. H., Billen, G., et al. (2010). Global river nutrient export: a scenario analysis of past and future trends. Glob. Biogeochem. Cycles 24. doi: 10.1029/2009GB003587
Shao, W. (2017). Weather, climate, politics, or God? Determinants of American public opinions toward global warming. Environ. Polit. 26, 71–96. doi: 10.1080/09644016.2016.1223190
Shields, A. (1936). Application of Similarity Principles and Turbulence Research to Bed-load Movement (transl. from German). Berlin: Mitteilungen der Preussischen Versuchsanstalt fur Wasserbau, 26.
Shuman, J. K., Balch, J. K., Barnes, R. T., Higuera, P. E., Roos, C. I., Schwilk, D. W., et al. (2022). Reimagine fire science for the Anthropocene. PNAS Nexus 1, pgac115. doi: 10.1093/pnasnexus/pgac115
Snaddon, C. D., Stewart, B. A., and Davies, B. R. (1992). The effect of discharge on leaf retention in two headwater streams. Arch. Hydrobiol. 109–120. doi: 10.1127/archiv-hydrobiol/125/1992/109
Soar, P. J., and Thorne, C. R. (2001). Channel Restoration Design for Meandering Rivers. Vicksburg, MS: US Army Corps of Engineers, Engineering Research and Development Center, MSERDC/CHL CR-01-1. doi: 10.21236/ADA397049
Sponseller, R. A., Heffernan, J. B., and Fisher, S. G. (2013). On the multiple ecological roles of water in river networks. Ecosphere 4, 1–14. doi: 10.1890/ES12-00225.1
Stanford, J. A., and Ward, J. V. (1988). The hyporheic habitat of river ecosystems. Nature 335, 64–66. doi: 10.1038/335064a0
Stanford, J. A., and Ward, J. V. (1993). An ecosystem perspective of alluvial rivers: connectivity and the hyporheic corridor. J. North Am. Benthol. Soc. 12, 48–60. doi: 10.2307/1467685
Stegen, J. C., Johnso, T., Fredrickson, J. K., Wilkins, M. J., Konopka, A. E., Nelson, W. C., et al. (2018). Influences of organic carbon speciation on hyporheic corridor biogeochemistry and microbial ecology. Nat. Commun. 9, 1–11. doi: 10.1038/s41467-018-02922-9
Steiger, J., Tabacchi, E., Dufour, S., Corenblit, D., and Peiry, J. L. (2005). Hydrogeomorphic processes affecting riparian habitat within alluvial channel-floodplain river systems: a review for the temperate zone. River Resear. Appl. 21, 719–737. doi: 10.1002/rra.879
Sternberg, H. (1875). Untersuchungen über längen- und querprofil geschiebeführender flüss. Z. Bauwesen 25, 483–506.
Stewart, R. J., Wollheim, W. M., Gooseff, M. N., Briggs, M. A., Jacobs, J. M., Peterson, B. J., et al. (2011). Separation of river network–scale nitrogen removal among the main channel and two transient storage compartments. Water Resour. Res. 47, W00J10. doi: 10.1029/2010WR009896
Stream Solute Workshop (1990). Concepts and methods for assessing solute dynamics in stream ecosystems. J. North Am. Benthol. Soc. 9, 95–119. doi: 10.2307/1467445
Sudduth, E. B., Hassett, B. A., Cada, P., and Bernhardt, E. S. (2011). Testing the field of dreams hypothesis: functional responses to urbanization and restoration in stream ecosystems. Ecol. Appl. 21, 1972–1988. doi: 10.1890/10-0653.1
Sullivan, P. L., Goddéris, Y., Shi, Y., Gu, X., Schott, J., Hasenmueller, E. A., et al. (2019). Exploring the effect of aspect to inform future earthcasts of climate-driven changes in weathering of shale. J Geophys. Res.: Earth Surf. 124, 974–993. doi: 10.1029/2017JF004556
Sullivan, P. L., Ma, L., West, N., Jin, L., Karwan, D. L., Noireaux, J., et al. (2016). CZ-tope at Susquehanna Shale Hills CZO: Synthesizing multiple isotope proxies to elucidate Critical Zone processes across timescales in a temperate forested landscape. Chem. Geol. 445, 103–119.
Sutfin, N. A., Wohl, E. E., and Dwire, K. A. (2016). Banking carbon: a review of organic carbon storage and physical factors influencing retention in floodplains and riparian ecosystems. Earth Surf. Process. Landf. 41, 38–60. doi: 10.1002/esp.3857
Swanson, F. J., Janda, R. J., Dunne, T., and Swanston, D. N. (tech. eds.) (1982). Workshop on Sediment Budgets and Routing in Forested Drainage Basins: Proceedings. Gen. Tech. Rep. PNW-141. Portland, OR: U.S. Department of Agriculture, Forest Service, Pacific Northwest Forest and Range Experiment Station 165.
Syvitski, J. P. M., Vorosmarty, C. J., Kettner, A. J., and Green, P. (2005). Impact of humans on the flux of terrestrial sediment to the global coastal ocean. Science 308, 376–380. doi: 10.1126/science.1109454
Tagseth, M. (2008). Oral history and the development of indigenous irrigation: methods and examples from Kilimanjaro, Tanzania. Nor. J. Geogr. 62, 9–22. doi: 10.1080/00291950701864898
Thackston, E. L., and Schnelle, K. B. (1970). Predicting effects of dead zones on stream mixing. J. Sanit. Eng. Div. Am. Soc. Civ. Eng. 96, 319–331. doi: 10.1061/JSEDAI.0001078
Thaw, M., GebreEgziabher, M., Villafañe-Pagán, J., and Jasechko, S. (2022). Modern groundwater reaches deeper depths in heavily pumped aquifer systems. Nat. Commun. 13, 5263. doi: 10.1038/s41467-022-32954-1
Thoms, M. C., Piegay, H., and Parsons, M. (2018). What do you mean, ‘resilient geomorphic systems'? Geomorphology 305, 8–19. doi: 10.1016/j.geomorph.2017.09.003
Thorp, J. H., and Delong, M. D. (1994). The riverine productivity model: an heuristic view of carbon sources and organic processing in large river ecosystems. Oikos 70, 305–308. doi: 10.2307/3545642
Thorp, J. H., Dodds, W. K., Robbins, C. J., Maasri, A., Arsenault, E. R., Lutchen, J. A., et al. (2021). A framework for lotic macrosystem research. Ecosphere 12, e03342. doi: 10.1002/ecs2.3342
Thorp, J. H., Thoms, M. C., and Delong, M. D. (2006). The riverine ecosystem synthesis: biocomplexity in river networks across space and time. River Res. Appl. 22, 123–147. doi: 10.1002/rra.901
Tockner, K., Malard, F., and Ward, J. V. (2000). An extension of the flood pulse concept. Hydrol. Process. 14, 2861–2883. doi: 10.1002/1099-1085(200011/12)14:16/17<2861::AID-HYP124>3.0.CO;2-F
Tomscha, S. A., Gergel, S. E., and Tomlinson, M. J. (2017). The spatial organization of ecosystem services in river-floodplains. Ecosphere 8, e01728. doi: 10.1002/ecs2.1728
Tonina, D., and Buffington, J. M. (2009). A three-dimensional model for analyzing the effects of salmon redds on hyporheic exchange and egg pocket habitat. Can. J. Fish. Aquat. Sci. 66, 2157–2173. doi: 10.1139/F09-146
Tornlund, E., and Ostlund, L. (2002). Floating timber in northern Sweden: the construction of floatways and transformation of rivers. Environ. Hist. 8, 85–106. doi: 10.3197/096734002129342611
Triska, F. J., Duff, J. H., and Avanzino, R. J. (1993). The role of water exchange between a stream channel and its hyporheic zone in nitrogen cycling at the terrestrial-aquatic interface. Hydrobiologia 251, 167–184. doi: 10.1007/BF00007177
Tullos, D. D., Penrose, D. L., Jennings, G. D., and Cope, W. G. (2009). Analysis of functional traits in reconfigured channels: implications for the bioassessment and disturbance of river restoration. J. North Am. Benthol. Soc. 28, 80–92. doi: 10.1899/07-122.1
URycki, D. R., Good, S. P., Crump, B. C., Chadwick, J., and Jones, G. D. (2020). River microbiome composition reflects macroscale climatic and geomorphic differences in headwater streams. Front. Water 2, 574728. doi: 10.3389/frwa.2020.574728
Van Andel, T. H., Zangger, E., and Demitrack, A. (1990). Land use and soil erosion in prehistoric and historical Greece. J. Field Archaeol. 17, 379–396. doi: 10.1179/009346990791548628
Van Loon, A., Rangecroft, S., Coxon, G., Werner, G., Wanders, N., Di Baldassarre, G., et al. (2022). Streamflow droughts aggravated by human activities despite management. Environ. Res. Lett. 17, 044059. doi: 10.1088/1748-9326/ac5def
Vannote, R. L., Minshall, G. W., Cummins, K. W., Sedell, J. R., and Cushing, C. E. (1980). The river continuum concept. Can. J. Fish. Aquat. Sci. 37, 130–137. doi: 10.1139/f80-017
Vaughn, C. C., Atkinson, C. L., and Julian, J. P. (2015). Drought-induced changes in flow regimes lead to long-term losses in mussel-provided ecosystem services. Ecol. Evol. 5, 1291–1305. doi: 10.1002/ece3.1442
Vidon, P. G., and Hill, A. R. (2004). Landscape controls on nitrate removal in stream riparian zones. Water Resour. Res. 40, 1–14. doi: 10.1029/2003WR002473
Villarreal, E. L., Semadeni-Davies, A., and Bengtsson, L. (2004). Inner city stormwater control using a combination of best management practices. Ecol. Eng. 22, 279–298. doi: 10.1016/j.ecoleng.2004.06.007
Vitousek, P. M., Aber, J. D., Howarth, R. W., Likens, G. E., Matson, P. A., Schindler, D. W., et al. (1997). Human alteration of the global nitrogen cycle: sources and consequences. Ecol. Appl. 7, 737–750. doi: 10.1890/1051-0761(1997)007[0737:HAOTGN]2.0.CO;2
Walker, B., and Salt, D. (2012). Resilience Practice: Building Capacity to Absorb Disturbance and Maintain Function. Washington, DC: Island Press. doi: 10.5822/978-1-61091-231-0
Walling, D. E. (2008). The changing sediment loads of the world's rivers. Ann. Wars. Univ. Life Sci. 39, 3–20. doi: 10.2478/v10060-008-0001-x
Walling, D. E., Owens, P. N., Carter, J., Leeks, G. J. L., Lewis, S., Meharg, A. A., et al. (2003). Storage of sediment-associated nutrients and contaminants in river channel and floodplain systems. Appl. Geochem. 18, 195–220. doi: 10.1016/S0883-2927(02)00121-X
Walter, R. C., and Merritts, D. J. (2008). Natural streams and the legacy of water-powered mills. Science 319, 299–304. doi: 10.1126/science.1151716
Wang, L., Butcher, A. S., Stuart, M. E., Gooddy, D. C., and Bloomfield, J. P. (2013). The nitrate time bomb: a numerical way to investigate nitrate storage and lag time in the unsaturated zone. Environ. Geochem. Health 35, 667–681. doi: 10.1007/s10653-013-9550-y
Ward, A. S., Morgan, J., Royer, T., and White, J. (2018). Streambed restoration to remove fine sediment alters reach-scale transient storage in a low-gradient 5th order river, Indiana, USA. Hydrol. Process. 32, 1786–1800. doi: 10.1002/hyp.11518
Ward, A. S., Packman, A., Bernal, S., Brekenfeld, N., Drummond, J., Graham, E., et al. (2022). Advancing river corridor science beyond disciplinary boundaries with an inductive approach to catalyse hypothesis generation. Hydrol. Process. 36, e14540. doi: 10.1002/hyp.14540
Ward, A. S., and Packman, A. I. (2019). Advancing our predictive understanding of river corridor exchange. Wiley Interdiscip. Rev. Water 6, e1327. doi: 10.1002/wat2.1327
Ward, A. S., Wondzell, S. M., Schmadel, N. M., and Herzog, S. P. (2020). Climate change causes river network contraction and disconnection in the H.J. Andrews Experimental Forest, Oregon, USA. Front. Water 2, 7. doi: 10.3389/frwa.2020.00007
Ward, A. S., Wymore, A. S., Harvey, J. W., and Wohl, E. (2023). A Systematic Review of River Corridor Conceptual Models, HydroShare. Available online at: http://www.hydroshare.org/resource/bf14a1cb0c8a42c28fd2439765de04cb (accessed May 15, 2023).
Ward, J. V. (1989). The four-dimensional nature of lotic ecosystems. J. North Am. Benthol. Soc. 8, 2–8. doi: 10.2307/1467397
Ward, J. V., and Stanford, J. A. (1983). “The serial discontinuity concept of lotic ecosystems,” in Dynamics of Lotic Ecosystems, eds T. D. Fontaine and S. M. Bartell (Ann Arbor: (Ann Arbor Science), 29–42.
Ward, J. V., and Stanford, J. A. (1995). The serial discontinuity concept: extending the model to floodplain rivers. Regul. Rivers Res. Manage. 10, 159–168. doi: 10.1002/rrr.3450100211
Ward, J. V., Tockner, K., and Schiemer, F. (1999). Biodiversity of floodplain river ecosystems: ecotones and connectivity. Regul. Rivers Resear. Manag. 15, 125–139. doi: 10.1002/(SICI)1099-1646(199901/06)15:1/3<125::AID-RRR523>3.0.CO;2-E
Webster, J. R. (1975). Analysis of Potassium and Calcium Dynamics in Stream Ecosystem on Three Southern Appalachian Watersheds of Contrasting Vegetation [Dissertation]. University of Georgia, Athens, GA.
Welch, C., Harrington, G. A., and Cook, P. G. (2014). Influence of groundwater hydraulic gradient on bank storage metrics. Groundwater 53, 782–793. doi: 10.1111/gwat.12283
White, P. S., and Pickett, S. T. A. (1985) “Natural disturbance patch dynamics: an introduction,” in The Ecology of Natural Disturbance Patch Dynamics, eds S. T. A. Pickett, P. S. White (New York, NY: Academic Press), 3–16. doi: 10.1016/B978-0-08-050495-7.50006-5
White, T., Brantley, S., Banwart, S., Chorover, J., Dietrich, W., Derry, L., et al. (2015). “The role of critical zone observatories in critical zone science,” in Developments in Earth Surface Processes, Vol. 19, eds J. R. Giardino and C. Houser (Amsterdam: Elsevier), 15–78. doi: 10.1016/B978-0-444-63369-9.00002-1
Williams, D. D., and Hynes, H. (1974). The occurrence of benthos deep in the substratum of a stream. Freshwater Biol. 4, 233–256.
Winter, T. C., Harvey, J. W., Franke, O. L., and Alley, W. (1998). Ground Water and Surface Water: A Single Resource. Denver, CO: U.S. Geological Survey Circular 1139, 90. p. doi: 10.3133/cir1139
Woelfle-Erskine, C., Larsen, L. G., and Carlson, S. M. (2017). Abiotic habitat thresholds for salmonid over-summer survival in intermittent streams. Ecosphere 8, e01645. doi: 10.1002/ecs2.1645
Wohl, E. (2013). Wilderness is dead: whither critical zone studies and geomorphology in the Anthropocene? Anthropocene 2, 4–15. doi: 10.1016/j.ancene.2013.03.001
Wohl, E. (2014). A legacy of absence: wood removal in US rivers. Prog. Phys. Geogr. 38, 637–663. doi: 10.1177/0309133314548091
Wohl, E. (2015). Legacy effects on sediments in river corridors. Earth-Sci. Rev. 147, 30–53. doi: 10.1016/j.earscirev.2015.05.001
Wohl, E. (2017). Connectivity in rivers. Prog. Phy. Geogr. 41, 345–362. doi: 10.1177/0309133317714972
Wohl, E. (2019). Forgotten legacies: understanding and mitigating historical human alterations of river corridors. Water Resour. Res. 55, 5181–5201. doi: 10.1029/2018WR024433
Wohl, E. (2020). Rivers in the Landscape, 2nd ed. Oxford: Wiley-Blackwell. doi: 10.1002/9781119535409
Wohl, E. (2021a). An integrative conceptualization of floodplain storage. Rev. Geophys. 59, e2020RG000724. doi: 10.1029/2020RG000724
Wohl, E. (2021b). Legacy effects of loss of beavers in the continental United States. Environ. Res. Lett. 16, 025010. doi: 10.1088/1748-9326/abd34e
Wohl, E., Bledsoe, B. P., Jacobson, R. B., Poff, N. L., Rathburn, S. L., Walters, D. M., et al. (2015). The natural sediment regime in rivers: broadening the foundation for ecosystem management. BioScience 65, 358–371. doi: 10.1093/biosci/biv002
Wohl, E., Brierley, G., Cadol, D., Coulthard, T. J., Covino, T., Fryirs, K. A., et al. (2019a). Connectivity as an emergent property of geomorphic systems. Earth Surf. Process. Landf. 44, 4–26. doi: 10.1002/esp.4434
Wohl, E., Castro, J., Cluer, B., Merritts, D., Powers, P., Staab, B., et al. (2021). Rediscovering, reevaluating, and restoring lost river-wetland corridors. Front. Earth Sci. 9, 653623. doi: 10.3389/feart.2021.653623
Wohl, E., and Knox, R. L. (2022). A first-order approximation of floodplain soil organic carbon stocks in a river network: the South Platte River, Colorado, USA as a case study. Sci. Total Environ. 852, 158507. doi: 10.1016/j.scitotenv.2022.158507
Wohl, E., Kramer, N., Ruiz-Villanueva, V., Scott, D. N., Comiti, F., Gurnell, A. M., et al. (2019b). The natural wood regime in rivers. BioScience 69, 259–273. doi: 10.1093/biosci/biz013
Wohl, E., Lininger, K. B., and Baron, J. (2017). Land before water: the relative temporal sequence of human alteration of freshwater ecosystems in the conterminous United States. Anthropocene 18, 27–46. doi: 10.1016/j.ancene.2017.05.004
Wohl, E., Lininger, K. B., and Scott, D. N. (2018). River beads as a conceptual framework for building carbon storage and resilience to extreme climate events into river management. Biogeochemistry 141, 365–383. doi: 10.1007/s10533-017-0397-7
Wohl, E., and Merritts, D. J. (2007). What is a natural river? Geogr. Compass 1, 871–900. doi: 10.1111/j.1749-8198.2007.00049.x
Wolf, E. C., Cooper, D. J., and Hobbs, N. T. (2007). Hydrologic regime and herbivory stabilize an alternative state in Yellowstone National Park. Ecol. Appl. 17, 1572–1587. doi: 10.1890/06-2042.1
Wollheim, W. M., Bernal, S., Burns, D. A., Czuba, J. A., Driscoll, C. T., Hansen, A. T., et al. (2018). River network saturation concept: factors influencing the balance of biogeochemical supply and demand of river networks. Biogeochemistry 141, 503–521. doi: 10.1007/s10533-018-0488-0
Wolman, M. G., and Gerson, R. (1978). Relative scales of time and effectiveness of climate in watershed geomorphology. Earth Surf. Process. 3, 189–208. doi: 10.1002/esp.3290030207
Wolman, M. G., and Miller, J. P. (1960). Magnitude and frequency of forces in geomorphic processes. The J. Geol. 68, 54–74. doi: 10.1086/626637
Woltemade, C. J., and Potter, K. W. (1994). A watershed modeling analysis of fluvial geomorphic influences on flood peak attenuation. Water Resour. Res. 30, 1933–1942. doi: 10.1029/94WR00323
Wondzell, S. M., and Swanson, F. J. (1999). Floods, channel change, and the hyporheic zone. Water Resour. Res. 35, 555–567. doi: 10.1029/1998WR900047
Wondzell, S. M., and Ward, A. S. (2022). The channel-source hypothesis: Empirical evidence for in-channel sourcing of dissolved organic carbon to explain hysteresis in a headwater mountain stream. Hydrol. Process. 36, e14570. doi: 10.1002/hyp.14570
Wondzell, S. M., LaNier, J., and Haggerty, R. (2009). Evaluation of alternative groundwater flow models for simulating hyporheic exchange in a small mountain stream. J. Hydrol. 364, 142–151. doi: 10.1016/j.jhydrol.2008.10.011
Worman, A., and Wachniew, P. (2007). Reach scale and evaluation methods as limitations for transient storage properties in streams and rivers. Water Resour. Res. 43, 13–13. doi: 10.1029/2006wr005808
Wymore, A. S., Fazekas, H. M., and McDowell, W. H. (2021a). Quantifying the frequency of synchronous carbon and nitrogen export to the river network. Biogeochemistry 152, 1–12. doi: 10.1007/s10533-020-00741-z
Wymore, A. S., Johnes, P. J., Bernal, S., Brookshire, E. N. J., Fazekas, H. M., Helton, A. M., et al. (2021b). Gradients of anthropogenic nutrient enrichment alter N composition and DOM stoichiometry in freshwater ecosystems. Glob. Biogeochem. Cycles 35, e2021GB006953. doi: 10.1029/2021GB006953
Wymore, A. S., Leon, M. C., Shanley, J. B., and McDowell, W. H. (2019). Hysteretic response of solutes and turbidity at the event scale across forested tropical montane watersheds. Front. Earth Sci. 7, 126. doi: 10.3389/feart.2019.00126
Wymore, A. S., Yang, W. H., Silver, W. L., McDowell, W. H., and Chorover, J. (2022). “An Introduction to Biogeochemistry of the Critical Zone,” in Biogeochemistry of the Critical Zone, eds A. S. Wymore, W. H. Yang, W. L. Silver, W. H. McDowell, and J. Chorover (Cham: Springer), 1–7. doi: 10.1007/978-3-030-95921-0
Yao, Y., Tian, H., Shi, H., Pan, S., Xu, R., Pan, N., et al. (2020). Increased global nitrous oxide emissions from streams and rivers in the Anthropocene. Nat. Clim. Change 10, 138–142. doi: 10.1038/s41558-019-0665-8
Yatsu, E. (1955). On the longitudinal profile of graded rivers. EOS. 36, 655–663. doi: 10.1029/TR036i004p00655
Zagury-Orly, I. (2020). Unmasking reasons for face mask resistance. Glob. Biosecur. 1. doi: 10.31646/gbio.80
Zimmermann, R. C., Goodlett, J. C., and Comer, G. H. (1967). “Influence of vegetation on channel form of small streams,” in IASH Publ. 75, Symposium on River Morphology (Berne), 255–275.
Zipper, S., Farmer, W., Brookfield, A., Ajami, H., Reeves, H., Wardropper, C., et al. (2022). Quantifying streamflow depletion from groundwater pumping: a practical review of past and emerging approaches for water management. J. Am. Water Resour. Assoc. 58, 89–312. doi: 10.1111/1752-1688.12998
Keywords: river corridor, critical zone, watersheds, floodplain, channel, ecosystems, freshwater, water resources
Citation: Wymore AS, Ward AS, Wohl E and Harvey JW (2023) Viewing river corridors through the lens of critical zone science. Front. Water 5:1147561. doi: 10.3389/frwa.2023.1147561
Received: 18 January 2023; Accepted: 27 April 2023;
Published: 31 May 2023.
Edited by:
Carl I. Steefel, Berkeley Lab (DOE), United StatesReviewed by:
Tim Scheibe, Pacific Northwest National Laboratory (DOE), United StatesJosep Mas-Pla, Catalan Institute for Water Research, Spain
Copyright © 2023 Wymore, Ward, Wohl and Harvey. This is an open-access article distributed under the terms of the Creative Commons Attribution License (CC BY). The use, distribution or reproduction in other forums is permitted, provided the original author(s) and the copyright owner(s) are credited and that the original publication in this journal is cited, in accordance with accepted academic practice. No use, distribution or reproduction is permitted which does not comply with these terms.
*Correspondence: Adam S. Ward, YWRhbS53YXJkQG9yZWdvbnN0YXRlLmVkdQ==
†These authors have contributed equally to this work and share first authorship