Mediterranean Institute of Neurobiology, INSERM U901, Marseille, France
This review briefly discusses the main approaches for monitoring chloride (Cl−), the most abundant physiological anion. Noninvasive monitoring of intracellular Cl− ([Cl−]i) is a challenging task owing to two main difficulties: (i) the low transmembrane ratio for Cl−, approximately 10:1; and (ii) the small driving force for Cl−, as the Cl− reversal potential (ECl) is usually close to the resting potential of the cells. Thus, for reliable monitoring of intracellular Cl−, one has to use highly sensitive probes. From several methods for intracellular Cl− analysis, genetically encoded chloride indicators represent the most promising tools. Recent achievements in the development of genetically encoded chloride probes are based on the fact that yellow fluorescent protein (YFP) exhibits Cl−-sensitivity. YFP-based probes have been successfully used for quantitative analysis of Cl− transport in different cells and for high-throughput screening of modulators of Cl−-selective channels. Development of a ratiometric genetically encoded probe, Clomeleon, has provided a tool for noninvasive estimation of intracellular Cl− concentrations. While the sensitivity of this protein to Cl− is low (EC50 about 160 mM), it has been successfully used for monitoring intracellular Cl− in different cell types. Recently a CFP–YFP-based probe with a relatively high sensitivity to Cl− (EC50 about 30 mM) has been developed. This construct, termed Cl-Sensor, allows ratiometric monitoring using the fluorescence excitation ratio. Of particular interest are genetically encoded probes for monitoring of ion channel distribution and activity. A new molecular probe has been constructed by introducing into the cytoplasmic domain of the Cl−-selective glycine receptor (GlyR) channel the CFP–YFP-based Cl-Sensor. This construct, termed BioSensor-GlyR, has been successfully expressed in cell lines. The new genetically encoded chloride probes offer means of screening pharmacological agents, analysis of Cl− homeostasis and functions of Cl−-selective channels under different physiological and pathological conditions.
Fluorescent indicators designed for quantitative monitoring of intracellular ions and analysis of the distribution of various proteins have brought about a revolution in obtaining important information about the functioning, development and pathology of cells and cellular components of biological organisms.
In this review we will briefly discuss the main approaches for monitoring chloride (Cl−), the most abundant physiological anion. Cl− is present in every cell of biological organisms and participates in a variety of important cellular processes, such as neurotransmission, regulation of cell volume, pH and water–salt balance. The concentration of intracellular Cl− and its permeance is highly regulated by a variety of Cl−-selective channels and Cl− transporters (revs. Chen and Hwang, 2008
; Jentsch, 2008
). Dysfunction of these proteins results in a various diseases. For instance, the most prevalent lethal genetic disease, cystic fibrosis (Kerem et al., 1989
), arises from mutations in the specific regulator of Cl− permeability, cystic fibrosis transmembrane conductance regulator (CFTR) protein. This voltage-independent Cl− channel is found in the epithelial cells of many tissues (intestine, lung, reproductive tract, pancreatic ducts). Mutations in the gene encoding CFTR affect 1 in 2000–2500 people (Ashcroft, 2000
). Several other human diseases have been linked to dysfunction of Cl− channels or transporters: myotonia congenita (Koch et al., 1992
), congenital chloride diarrhoea (Kere et al., 1999
), inherited hypercalciuric nephrolithiasis (Lloyd et al., 1996
), Bartter’s and Gitelman’s syndromes (Simon and Lifton, 1996
), hyperekplexia/startle disease (Shiang et al., 1993
) and epilepsy (Macdonald et al., 2004
; Lerche et al., 2005
; Heron et al., 2007
; Dibbens et al., 2009
).
Direct measurement of intracellular Cl− concentration ([Cl−]i) in neurons and in other cell types is a challenging task owing to two main difficulties: (i) low transmembrane ratio for Cl−, approximately 10:1 (for Ca, for instance, 10000:1); and (ii) a small driving force for Cl−, as the Cl− reversal potential (ECl) is usually close to the resting potential of the cells. Consequently, sensitive probes with high dynamic range at physiological [Cl−]i are necessary for reliable analysis of [Cl−]i distribution and its functional variations.
For [Cl−]i monitoring several methods have been proposed. The most used are Cl−-selective microelectrodes; chloride-sensitive fluorescent dyes and genetically encoded chloride-sensitive probes. We will briefly describe these approaches with the main focus on genetically encoded chloride-sensitive probes, which are the most promising tools for effective analysis of Cl− homeostasis in various cell types.
In the 1960s, 70s and 80s the use of ion-selective electrodes was the main available technique for intracellular Cl− detection. It allowed valuable information on Cl− distribution and dynamics in a number of cell types of biological organisms to be obtained. In very early studies, AgCl electrodes were used as tools for [Cl−]i estimation (Mauro, 1954
; Keynes, 1963
; Strickholm and Wallin, 1965
; Sato et al., 1968
). With an electrode consisting of a fine AgCl wire protruding from the end of a glass capillary, [Cl−]i was measured in giant axons of squid (Mauro, 1954
; Keynes, 1963
) and crayfish (Strickholm and Wallin, 1965
). However, later observations demonstrated that all microelectrodes that use AgCl as the sensitive element develop “the same type of error in the intracellular environment and thus all give erroneously high values of [Cl−]i ” (Neild and Thomas, 1974
).
The improved method was based on the use of siliconized borosilicate glass micropipettes, the tips of which were filled with liquid chloride ion exchanger. This technique was introduced by Walker (1971) and used with some modifications in a number of studies (Walker and Brown, 1970
; Neild and Thomas, 1974
; Ascher et al., 1976
; Vaughan-Jones, 1979
). These electrodes (Figure 1
A) had a small tip (1–2 μm) and gave complete responses to changes in Cl− within 1–2 min. Preparing these electrodes is a time-consuming procedure and penetration of cells without damage is difficult.
Figure 1. Cl−-sensitive microelectrodes and intracellular chloride concentration measurements. (A) Construction of recessed-tip Cl−-sensitive microelectrode. The complete electrode (top diagram) and enlarged view of the sensitive tip (bottom diagram) are shown. (From Neild and Thomas, 1973
). (B) Diagram showing the basic experimental arrangement for chloride concentration measurements using microelectrodes. (Modified from Thomas, 1977
). (C) Example of simultaneous recording of membrane potential (Em) and [Cl−]i in smooth muscle cell of the guinea pig vas deferens using double-barrelled microelectrode. Cl−-free and normal solutions were applied. Note the very slow (min) recorded Cl− transients. (Modified from Aickin and Brading, 1982
).
Despite these complications, Cl−-sensitive microelectrodes were successfully used to measure the intracellular Cl− activity in giant neurons of molluscs Aplysia (Brown et al., 1970
; Ascher et al., 1976
) and Helix aspersa (Neild and Thomas, 1974
; Kennedy and Thomas, 1995
; Schwiening and Thomas, 1996
), in frog heart cells (Ladle and Walker, 1975
; Vaughan-Jones, 1979
) and in other preparations (Walker and Brown, 1977
; Thomas, 1978
).
An intracellular Cl−-sensitive microelectrode records the algebraic sum of the membrane potential (Em) and a voltage proportional to changes in Cl− activity. It means that Em must be separately determined using an independent electrode (Figure 1
B). To diminish the damage from insertion of two microelectrodes into a single cell, double-barrelled pipettes were proposed (Aickin, 1981
) and used for monitoring the intracellular Cl− activity in smooth muscle cells (Aickin and Brading, 1982
; Davis et al., 2000
), retinal pigment epithelium (Bialek et al., 1995
; La Cour et al., 1997
) and in other cell types (Kitano et al., 1995
; Debellis et al., 2001
; Ianowski et al., 2002
).
Early studies with Cl−-sensitive microelectrodes already demonstrated that [Cl−]i in cells differs substantially from a predicted passive distribution, suggesting that Cl− ions must be actively transported through cellular membranes. These observations were confirmed by the more recent discovery of several mechanisms of transmembrane Cl− transport (rev. Russell, 2000
; Lauf and Adragna, 2004
).
Three main obstacles limited the spread of the Cl−-sensitive microelectrode technique: (i) time-consuming procedure for microelectrode preparation; (ii) slow kinetics (Figure 1
C); and (iii) the need to use relatively large cells for reliable recording without cell damage. In addition, penetration of cells could change the native intracellular Cl− distribution. Methods based on imaging techniques are more promising as they provide an opportunity to monitor Cl− activity noninvasively and in populations of cells.
Because of the possibility of monitoring noninvasively the distribution and dynamics of ion concentration changes, fluorescent Cl−-sensitive dyes are the most popular approach for analysis of Cl− and Cl−-dependent physiological processes in different cells types.
The fluorescence of many fluorophores is decreased on application of heavy-atom anions, such as bromine and iodine. Cl− ions are less effective in this respect and relatively few fluorophores are quenched by Cl− (Geddes et al., 2001
). The background for Cl− monitoring was established by George Stokes, who described in 1852 the phenomenon of fluorescence. In 1869 Stokes observed that the “fluorescence of quinine in dilute sulfuric acid was reduced after the addition of hydrochloric acid, i.e., chloride ions” (Geddes et al., 2001
). Perhaps the fact that quinine, which is sensitive to chloride, contains a quinoline ring has stimulated the production of many quinoline analogues in the search for efficient Cl− probes.
Quinolinium Cl− indicators are based on the capability of halides to quench the fluorescence of heterocyclic organic compounds with quaternary nitrogen (Chen et al., 1988
; Verkman, 1990
; Figure 2
A). A relation between the Cl− concentration and the fluorescence intensity is described by the Stern–Volmer-equation:
Figure 2. Chemical structure and fluorescent properties of widely used Cl−-sensitive dyes. (A) Chemical structure of quinolinium Cl−-sensitive dyes. Left: Common structure, R1 and R2, radicals. Middle and right: Structural formula for SPQ and MQAE dyes respectively. (B) Examples of continuous fluorescence measurements of the MQAE-loaded cells. Note the rapid degradation in baseline fluorescence value (top and bottom traces) and dramatic decrease in the fluorescent response for the second transition from 105 to 0 mM of Cl− (top trace). (Modified from Nakamura et al., 1997
). (C) Two-photon imaging in MQAE-loaded cerebellar neurons. Changes in fluorescence (top traces) and corresponding transmembrane currents (bottom traces) caused by 150-ms-long pressure applications of GABA. Note about 50-times slower fluorescent transients in comparison with currents. (Modified from Marandi et al., 2002
).
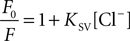
where F0 is the fluorescence in the absence of halide, F is the fluorescence in the presence of halide and KSV is the Stern-Volmer quenching constant (in M−1). From this equation the EC50, the concentration of Cl− causing a 50% decrease in fluorescence, is 1/KSV.
Table 1
shows values of the Stern-Volmer constant and corresponding EC50 values for some quinolinium indicators. The most-used quinolinium indicators are 6-methoxy-N-(3-sulfopropyl) quinolinium (SPQ), 6-methoxy-N-ethylquinolium (MEQ) and N-(6-methoxyquinolyl)-acetoethyl ester (MQAE).
The first designed quinolinium-based Cl− indicators was SPQ (Wolfbeis and Urbano, 1983
; Illsley and Verkman, 1987
; Figure 2
A, Table 1
). SPQ is excited at ultraviolet wavelengths with absorbance maxima at 318 nm and 350 nm. This fluorescent dye has a single broad emission peak centred at 450 nm with a quantum yield of 0.69 in the absence of halides. In aqueous buffers the Stern-Volmer constant for quenching of SPQ by Cl− is 118 M−1, giving EC50 ∼ 8.5 mM. The fluorescence of SPQ is not altered by cations, phosphate, nitrate or sulfate, but it is quenched weakly by other monovalent anions including citrate, acetate, gluconate and bicarbonate (Illsley and Verkman, 1987
; Krapf et al., 1988b
; Jayaraman et al., 1999
).
MQAE, the other quinolinium-based dye, which has been used in a number of studies during recent years, has high Cl− sensitivity (Figure 2
A, Table 1
) and, unlike SPQ, easily permeates through the plasma membrane (Mansoura et al., 1999
). As a result the time of incubation with this dye can be rather short. For instance, 10-min incubation of brain slices is sufficient for bright staining of neurons (Marandi et al., 2002
). However, this substance has to be used with prudence, as incubation of slices for 30–45 min caused a deterioration in the properties and even death of many neurons in neocortex and hippocampal slices (Holmgren, Zilberter, Mukhtarov, personal observations). For these reasons, results obtained after as long as 1–2 h of treatment with MQAE (e.g. Servetnyk and Roomans, 2007
) have to be interpreted with caution. The other disadvantages of this dye lie in the significant leakage rate and bleaching. The leakage rate seems to be preparation-specific, ranging from 3% per hour in liposomes (Verkman et al., 1989
) to 30% per hour in brain slices (Marandi et al., 2002
).
Quinolinium-based Cl− indicators have been used for measurements of Cl− in a variety of preparations, including isolated growth cones (Fukura et al., 1996
), neurons (Schwartz and Yu, 1995
; Inglefield and Schwartz-Bloom, 1997
; Dallwig et al., 1999
; Frech et al., 1999
; Marandi et al., 2002
), glia (Bevensee et al., 1997
), different types of epithelial cells (Krapf et al., 1988b
; Lau et al., 1994
), fibroblasts (Chao et al., 1989
; Woll et al., 1996
; Munkonge et al., 2004
), human gastric cancer cells (Miyazaki et al., 2008
) and pancreatic beta-cells (Eberhardson et al., 2000
). In general, quinolinium compounds have relatively good sensitivity and selectivity to Cl− and rapid responses to changes in Cl−. They are also insensitive to physiological changes in pH.
The major disadvantage of these probes comes from their spectral properties, i.e. excitation at ultraviolet wavelengths. As a result, they are prone to strong bleaching (Inglefield and Schwartz-Bloom, 1997
; Nakamura et al., 1997
, Figure 2
B). This restricts the duration of the measurements and allows only a very low data acquisition rate (0.2–2 frames per minute) (Inglefield and Schwartz-Bloom, 1997
; Fukuda et al., 1998
; Sah and Schwartz-Bloom, 1999
). However, the use of these dyes in combination with two-photon microscopy strongly reduces bleaching and, consequently, photochemical damage (Marandi et al., 2002
; Funk et al., 2008
).
YFP as a Tool for Intracellular Cl– Monitoring
An alternative to exogenously added indicators is the use of an endogenously expressed chromophore such as green fluorescent protein (GFP). Using appropriate targeting sequences, GFPs have been directed selectively to numerous intracellular sites. Various applications of GFP in physiological studies of living cells have been described (Tsien, 1998
; Zaccolo and Pozzan, 2000
; Bizzarri et al., 2009
). GFP derivatives with different colors have been used in FRET models to monitor Ca2+ (Miyawaki et al., 1997
, 1999
), pH (Kneen et al., 1998
; Llopis et al., 1998
; Miesenbock et al., 1998
) and protein–protein interactions (Heim, 1999
).
During the last decade, a new method of noninvasive [Cl−]i monitoring using genetically encoded optical probes has been developed. This approach is based on the halide-binding properties of yellow fluorescent protein (YFP) and it derivatives (Wachter and Remington, 1999
; Jayaraman et al., 2000
). YFP is a derivative of GFP, which contains four point mutations (T203Y/S65G/V68L/S72A). It has improved brightness and red-shifted excitation/emission spectra compared with GFP (Ormo et al., 1996
; Elsliger et al., 1999
). The halide sensitivity of YFP was conferred on this protein using a rational mutagenesis strategy based upon crystallographic data (Wachter et al., 1998
) and confirmed experimentally (Wachter and Remington, 1999
).
It was found that YFP fluorescence is sensitive to various small anions with relative potencies F− > I− > NO3− > Cl− > Br− > formate− > acetate− (Jayaraman et al., 2000
). YFP sensitivity to these small anions results from ground-state binding near the chromophore (Jayaraman and Verkman, 2000
), which apparently alters the chromophore ionization constant and hence the fluorescence emission. As with other GFP derivatives, the fluorescence of YFP is pH-dependent. The EC50 values for Cl− varied from 32.5 mM (pH = 6) to 777 mM (pH = 7.5) (Table 2
).
This analysis demonstrates that at the physiological range of intracellular pH (7.2–7.4) the sensitivity of YFP to Cl− is low, which creates difficulties and limitations in using “wild type” (WT) YFP as a Cl− indicator. Indeed, different methods of [Cl−]i estimation in various cell types gives its range of variations from 3 to 60 mM (Table 3
). Consequently, the resolution of the indicator with EC50 more then 100 mM is low and it can lead to errors in noninvasive estimation of [Cl−]i.
To further improve spectral characteristics, YFP was subjected to additional mutagenesis and the most successful variants were selected. It has been demonstrated that at pH 7.5 the EC50 for Cl− of the mutant YFP H148Q is 154 mM (Table 2
; Wachter et al., 2000
), which is still high; however, it is closer to the physiological range of [Cl−]i than WT YFP (777 mM).
To enhance sensitivity of YFP-H148Q to Cl−, libraries of mutants were generated in which pairs of residues in the vicinity of the halide binding site were randomly mutated (Galietta et al., 2001a
). Analysis of over a thousand clones revealed improved anion sensitivity with EC50s down to 40 mM for Cl− (V163S), 10 mM for NO3− (I152L) and 2 mM for I− (I152L). To check physiological applicability, the I152L mutant, which exhibited the best I− and NO3− sensitivities, was expressed in Swiss 3T3 fibroblasts carrying CFTR. Transfected cells were brightly fluorescent with a uniform cytoplasmic and nuclear staining pattern. Replacement of 20 mM Cl− with I− produced a fluorescence decrease of 53 ± 2% with YFP-I152. It was much greater than that of <10% for the same experiment with YFP-H148Q, indicating that this mutant is a good tool for monitoring I− and NO3−. The same results were obtained when the activity of CFTR was studied using a Cl−/NO3− exchange protocol (Galietta et al., 2001b
).
Some other mutants of YFP also showed greatly improved Cl− sensitivities (Table 4
), which stimulated further development of Cl− indicators. It provided the basis for using YFP mutants as genetically encoded Cl− sensors that can be targeted to specific organelles in living cells or expressed in specific cell types for monitoring [Cl−]i distribution, to study the functioning of Cl− channels and pumps.
Application of YFP in High-Throughput Screening
In a number of experimental models YFP derivatives have been used as suitable probes for high-throughput (HTP) screening. These allow the testing of tens of thousands of different compounds (Ma et al., 2002a
,b
). We present here several examples.
YFP-H148Q was transfected in Fisher rat thyroid cells (FRT) and in Swiss 3T3 fibroblasts for quantitative HTP screening of potential modulators of CFTR halide permeances (Galietta et al., 2001b
). In these assays small increases in CFTR activity by the agonists forskolin and genistein were detected. Because YFP fluorescence is sensitive to H+ a simultaneous monitoring of pH by specific pH-probe, BCECF, was also conducted (Galietta et al., 2001b
). Using HTP screening on cells expressing the YFP-H148Q mutant, new classes of CFTR modulators were identified: inhibitors (Ma et al., 2002a
), activators (Galietta et al., 2001c
; Ma et al., 2002b
; Muanprasat et al., 2004
, 2007
), potentiators (Yang et al., 2003
) and correctors (Pedemonte et al., 2005a
,b
) of wild-type CFTR, as well as of ΔF508-CFTR, the major CFTR mutation causing cystic fibrosis (see rev. Verkman and Galietta, 2009
).
To screen potential activators of CFTR-mediated Cl− flux, a YFP mutant with higher halide sensitivity (YFP-H148Q/I152L) has been co-expressed with defective ΔF508-CFTR in FRT epithelial cells (Yang et al., 2003
; Xu et al., 2008
). It has been shown that natural cumarine compounds rescue defective ΔF508-CFTR chloride channel gating (Xu et al., 2008
).
A mutant with enhanced sensitivity to halides (YFP-V163S) has been used in M1 cortical collecting-duct cells to monitor changes in Cl− mediated by CFTR or by stimulation with cAMP- and Ca2+-increasing agonists (Adam et al., 2005
).
Important observations have been obtained with YFP-H148Q/I152L, which shows a 30-fold selectivity to I− over Cl−. It was proved to be a sensitive biosensor of Na+/I− symporter-mediated I− uptake in thyroid cells and nonthyroidal cells following gene transfer (Rhoden et al., 2007
, 2008
). As defective iodide transport occurs in several inherited and acquired thyroid disorders, using this YFP mutant for detection of I− represents a useful tool for studying the pathophysiology and pharmacology of this Na+/I− symporter (Rhoden et al., 2007
).
YFP derivatives were also used for testing ligands of glycine receptors (GlyRs) and ionotropic GABA receptors. Using HEK 293 cells expressing YFP-I152L or YFP-V163S mutants with these Cl−-selective receptor-operated channels has established the optimal conditions for pharmacological screening of Cl− (Kruger et al., 2005
) and detection of functional and non-functional mutations in the GlyRs (Gilbert et al., 2009
).
These observations have demonstrated advantages of using genetically encoded YFP derivatives in HTP in comparison with other techniques.
Advantages and Disadvantages of Genetically Encoded Cl− Indicators
Of the many advantages of YFP-based Cl− indicators in comparison with fluorescent dyes, we will mention only the four most important.
First, the peak of absorbance is at a wavelength of more then 480 nm, i.e., in contrast to quinolinium-based halide indicators, YFP can be excited in the visible range, permitting more stable fluorescence signal and less cell damage. Consequently, it allows long-lasting Cl− monitoring with repetitive stable responses and bright fluorescence signals using conventional imaging equipment.
Second, genetically encoded probes can be targeted to specific cell types by cell-specific promoter, or to defined cellular compartments by fusion to short sequence tags or to specific proteins. This would allow Cl− monitoring in specific cell types or cellular compartments. For instance, transgenic mice expressing enhanced YFP (EYFP) under control of the Kv3.1 K+ channel promoter (pKv3.1) have been generated (Metzger et al., 2002
), making possible neuron-specific expression of EYFP in the hippocamus, thalamus and granule cell layer of cerebellum. This model has been used for analysis of glutamate-induced changes in intracellular Cl− and pH (Metzger et al., 2002
; Slemmer et al., 2004
). The thy1 promoter has been successfully used to drive specific neuronal expression of Clomeleon in the hippocampus and in neocortical areas as well as in the dentate gyrus, cerebellar mossy fibers and piriform cortex (Berglund et al., 2008
).
Third, the intracellular concentration of the YFP-based Cl− indicators is only a few micromolar, which is several orders of magnitude less than the [Cl−]i. This avoids buffering effects, which are a substantial problem in, for instance, fluorescence measurements of intracellular Ca2+ at using conventional fluorescent dyes.
Finally, the molecular weight of the YFP-based Cl− indicators is about 27 kDa, which prevents diffusion of the indicator from cells. In cells that are imaged without simultaneous electrophysiological recordings, indicator levels remains constant over hours.
YFP-based sensors also have several disadvantages. One of them is pH sensitivity (Table 2
). Changes in intracellular pH or in specific compartments can lead to errors in observations and interpretation of results. To overcome this problem, in some cases independent monitoring of pH is necessary.
YFP-based Cl− sensors have rather low kinetics of Cl− association/dissociation. The double mutant YFP-H148Q/V163S, which exhibits relatively high Cl− sensitivity (EC50 = 39 mM), has an association time constant τ = 1900 ms (Galietta et al., 2001a
), which would cause limitations in the time resolution when using this mutant for analysis of rapid Cl− dynamics. The other double mutant, YFP-H148Q/I152L, has much faster association/dissociation kinetics (association time constant τ = 52 ms; Galietta et al., 2001a
); however, its sensitivity to Cl− is relatively low (EC50 = 85 mM). Thus, the YFP-based probes can be used to reliably detect changes in Cl− concentrations with time course resolution in the range of hundreds of milliseconds or even seconds.
A significant limitation in the use of Cl− indicators was a lack of a Cl−-dependent change in spectral shape, which precludes ratiometric measurements. For quinolinium-based Cl−-sensitive dyes, synthesis of a series of dual-wavelength fluorescent indicators has been achieved using conjugation of Cl−-sensitive and Cl−-insensitive dye molecules with different spacers (Jayaraman et al., 1999
). Only one “chimera” (MQa4AQ) was cell-permeating and it turned out to be four times less Cl−-sensitive than MQAE. YFP-derivatives also do not have a clear isosbestic point in spectral shape at different Cl− concentrations. This precludes ratiometric measurements and, consequently, gives rise to limitations in the estimation of [Cl−]i values.
The important development of genetically encoded Cl− probes was achieved by Kuner and Augustine (2000)
, who constructed a ratiometric YFP-based Cl− indicator, termed Clomeleon.
Ratiometric Monitoring of Cl− Using Genetically Encoded Probes
Clomeleon
Clomeleon consists of two fluorescent proteins, Cyan Fluorescence Protein (CFP) and a variant of YFP, Topaz Fluorescence Protein (TFP, GFP/S65G/S72A/K79R/T203Y/H231L), connected with a polypeptide linker of 24 aminoacids (Figures 3
A,B).
Figure 3. Structure and fluorescence properties of Clomeleon. (A) Scheme of Clomeleon construct and (B) schematic reconstruction of Clomeleon structure. The fluorescent domains, CFP (cyan) and Topaz (yellow) were set in parallel orientation as N- and C-terminal ends of either domain were on the same site. The distance between CFP and Topaz chromophores was calculated to be 3.3 nm. (From Kuner and Augustine, 2000
(top) and Jose et al., 2007
(bottom). (C) Emission spectra of Clomeleon in the presence of different Cl− concentrations. In all cases, the recombinant protein was excited at 434 nm and the emission spectra were normalized to their peaks at 527 nm. (D) The relationship between fluorescence emission ratio (527 nm/485 nm) and Cl− concentrations. (Data from Kuner and Augustine, 2000
).
The work of this probe is based on the phenomenon of Fluorescence Resonance Energy Transfer (FRET) between two fluorescent proteins (see Box 1
). Binding of a Cl anion to TFP reduces its emission, leading to a decrease in FRET efficiency. This process can be visualized as a reduction in the ratio of fluorescence emission between the TFP acceptor and CFP donor fluorophores. Analysis of emission spectra of this construct revealed that the intensity of fluorescence depends on Cl− concentration. Moreover, presence of the isosbestic point in normalized spectra (Figure 3
C) allows the use of this indicator as a ratiometric probe for estimation of Cl− concentration (Figure 3
D) The construct was named Clomeleon as an allusion to the FRET-based genetically encoded Ca2+ indicator, Cameleon (Miyawaki et al., 1997
).
Box 1. FRET. Phenomenon of fluorescence resonance energy transfer (FRET) represents interaction between two fluorophores, when excitation energy from a donor (D) molecule is directly transferred to a molecule of acceptor (A).
Four main conditions have to be fulfilled for this phenomenon development:
(i) overlapping of emission spectrum of donor and excitation spectrum of acceptor;
(ii) small distance between molecules (less then 10 nm);
(iii) good orientation the dipole moments of donor emission and acceptor absorption;
(iv) high quantum yield of fluorophores.
The FRET efficiency (E) is dependent on the inverse sixth power of the distance between fluorophores (r):
E = 1/[1 + (r/R0)6]
where R0 is the distance at which the energy transfer efficiency is 50%.
This makes FRET technique a sensitive tool for analysis of protein–protein interaction and changes in intermolecular distances.
For more details see: Tsien et al., 1993 ; Pollok and Heim, 1999 ; Jares-Erijman and Jovin, 2003 ; Sekar and Periasamy, 2003 ; Wallrabe and Periasamy, 2005 .
Four main conditions have to be fulfilled for this phenomenon development:
(i) overlapping of emission spectrum of donor and excitation spectrum of acceptor;
(ii) small distance between molecules (less then 10 nm);
(iii) good orientation the dipole moments of donor emission and acceptor absorption;
(iv) high quantum yield of fluorophores.
The FRET efficiency (E) is dependent on the inverse sixth power of the distance between fluorophores (r):
E = 1/[1 + (r/R0)6]
where R0 is the distance at which the energy transfer efficiency is 50%.
This makes FRET technique a sensitive tool for analysis of protein–protein interaction and changes in intermolecular distances.
For more details see: Tsien et al., 1993 ; Pollok and Heim, 1999 ; Jares-Erijman and Jovin, 2003 ; Sekar and Periasamy, 2003 ; Wallrabe and Periasamy, 2005 .
Unlike organic probes, Clomeleon possesses several valuable features: excitation at visible wavelengths, good signal-to-noise ratio, safer loading procedures for cells, absence of leakage from cells and the possibility of targeting the probe to different cell types using specific promoters. Moreover, the construct exhibits high fluorescence stability: absence of Clomeleon bleaching during 2 h of recording has been reported (Pond et al., 2006
). Proteolitical stability of Clomeleon in transgenic mice has been observed during 9 months. It is also a low toxicity probe, which did not cause any behavioural aberration in Clomeleon-expressing mice in the course of 2 years (Berglund et al., 2006
).
The main advantage of Clomeleon is the possibility of performing ratiometric measurements of [Cl−]i. The ratiometric capabilities of Clomeleon allow optical measurements that are minimally influenced by the thickness of the specimen, intensity of the excitation light and concentration of the indicator. This, in turn, makes it possible to accurately determine Cl− values even in cells with complicated geometry, such as neurons.
Clomeleon has been used for measurements of [Cl−]i in cultured hippocampal neurons (Kuner and Augustine, 2000
), in plant cells (Lorenzen et al., 2004
) and in cells of retina and brain slices (Duebel et al., 2006
; Pond et al., 2006
). The widest field of application for genetically encoded probes comes from the possibility of targeting them to specific cell types using unique promoters, or to cellular compartments and membrane domains by fusion to respective tags or to proteins with known location. Several transgenic mouse lines have been created by insertion of Clomeleon, under control of the thy1 promoter, into their genome (Berglund et al., 2008
). Details and functional implications of these models are described in recent papers (Duebel et al., 2006
; Berglund et al., 2008
).
Potential limitations of this probe are that it, as other YFP-based Cl− indicators, is sensitive to pH and that the time course of reaction to Cl− is relatively slow. The other potential problem is that the fluorophores, CFP and TFP, may bleach at different rates, which would distort the calibration of the indicator signal.
The important disadvantage of Clomeleon is that at physiological pH it has a rather low sensitivity to Cl−. The EC50 of Clomeleon is more than 160 mM (Kuner and Augustine, 2000
; Figure 3
D, Table 5
) which is far from physiological [Cl−]i (3–60 mM). For this reason, the development of ratiometric probes with high sensitivity to Cl− is required.
Cl-Sensor
Recently a new genetically encoded indicator, termed Cl-Sensor, has been proposed (Markova et al., 2008
; Figure 4
A). Analysis of the spectral properties of this construct during simultaneous monitoring of fluorescence signals and whole-cell recordings with different Cl− concentrations in the pipette solution revealed two important features.
Figure 4. Design and fluorescence properties of Cl-Sensor. (A) Schematic representation of Cl-Sensor construct; *** indicates three mutations: YFP-H148Q, -I152L and -V163S in the YFP sequence. (B) Normalized spectra of Cl-Sensor. Whole-cell recordings from CHO cells with pipettes containing different Cl− concentrations (shown in the graph). Note that spectra have a common point at 465 nm. (C) Comparison of Cl−-sensitivities of Cl-Sensor and Clomeleon. Cl-Sensor (black squares and line): the relationship between fluorescence excitation ratio (F480/F440) and [Cl−]i obtained from whole-cell recordings with pipettes containing solutions with different Cl− concentrations (from 0 to 150 mM) (from Markova et al., 2008
). Clomeleon (blue circles and line): the relationship between fluorescence emission ratio (F527/F485) and [Cl−]i plotted from Kuner and Augustine, 2000
(see Figure 4
D). Note that main part of calibration curve for Clomeleon is out of physiological range of [Cl−]i.
First, the normalized excitation spectra obtained at different [Cl−]i have a common point near 465 nm (Figure 4
B), meaning that Cl-Sensor allows ratiometric monitoring using the fluorescence excitation ratio. This feature allows recordings using conventional setups with devices for a rapid change of excitation wavelength.
Second, due to triple YFP mutation (YFP-H148Q/I152L/V163S) this construct exhibits a relatively high sensitivity to Cl− with an estimated EC50 of about 30 mM (28 ± 5 mM). With about 5-fold higher sensitivity than Clomeleon, this indicator has a good dynamic range at physiological intracellular concentrations (Figure 4
C), providing a good basis for reliable monitoring of [Cl−]i in different cell types.
Cl-Sensor demonstrates the same advantageous features as Clomeleon, i.e. excitation at visible wavelengths, good signal-to-noise ratio, safer loading procedures, absence of leakage from cells and ability to be targeted to different cell types using specific promoters.
Similarly to other fluorescent proteins from the GFP family, Cl-Sensor exhibits a relatively high pH sensitivity with pKa ranging from 7.1 to 8.0 pH units at different Cl− concentrations.
One widespread problem with GFPs is their low transfection efficiency in neurons. To overcome this difficulty, Cl-Sensor was subcloned at two different vectors driven by mutated CMV and ubiquitine promoter. These plasmids carrying Cl-Sensor reveal higher transfection efficiency and brightness of probe in the CHO cell line, retinal cells and spinal or hippocampal neurons (Waseem et al., paper in preparation).
The Cl-Sensor was used for noninvasive estimation of [Cl−]i in CHO cells, hippocampal neurons and photoreceptor cells from retinal slices (Table 3
; Markova et al., 2008
; Mukhtarov et al., 2008
). For Cl-Sensor gene delivery to retinal cells an in vivo electroporation technique was used (Matsuda and Cepko, 2004
; Mukhtarov et al., 2008
). The efficiency of electroporation into the developing postnatal retina (at P0) was high, and transgene expression persisted for more then 1 month (Mukhtarov et al., 2008
), indicating that in vivo electroporation of Cl-Sensor cDNA is a powerful tool for monitoring [Cl−]i under different experimental conditions and through age-dependent changes in Cl− in neurons.
BioSensor-GlyR – tool for monitoring Cl−-selective channel activation
Investigation of brain functioning requires methods allowing dynamic analysis of network activity combined with determination of single-cell properties. This strategy has been developed for monitoring calcium transients using rapid two-photon microscopy (Cossart et al., 2005
). Chemically engineered proteins that are directly sensitive to light are also powerful optical methods of protein function control for modulation of signalling circuits inside cells and in cell circuits (Gorostiza and Isacoff, 2008
). However, analysis of networks formed by neuronal circuits for specific synapses (glutamatergic, GABAergic or glycinergic) is hampered by lack of adequate techniques. This problem could be solved by genetic incorporation of molecules capable of changing their fluorescence on activation of specific synapses. The best candidates for these molecules would be fluorescently modified postsynaptic receptor-operated channels. Genetic incorporation of a molecular domain which could change fluorescence upon channel activation would provide the possibility of noninvasive monitoring of ion channel activity. Development of these molecules is a highly challenging task.
One of the approaches uses the voltage-clamp fluorometry (VCF) technique, based on covalently attaching a small environmentally sensitive sulfhydryl-labeled fluorophore to a cysteine introduced into a domain of interest on the protein. This approach has been successfully used to analyze the conformational rearrangements underlying gating of voltage-gated potassium channels (Mannuzzu et al., 1996
) and ligand-gated glycine receptor (GlyR) channels (Pless and Lynch, 2008
, 2009
).
The other way consists of inserting a genetically encoded fluorescent sensor in the protein’s sequence without changing its functional properties. Recently, a new genetic probe, termed BioSensor-GlyR, has been developed (Mukhtarov et al., 2008
). This construct is a Cl−-selective GlyR channel with Cl-Sensor incorporated into the long cytoplasmic domain (Figure 5
A).
Figure 5. Structure and fluorescence properties of BioSensor-GlyR. (A) Scheme of BioSensor-GlyR construct. Two subunits are shown. (B) Whole-cell currents induced by rapid application of glycine (30 or 300 μM) and dose-response curves obtained from CHO cells transfected with either wild-type human GlyR (blue) or BioSensor-GlyR (green). Note similar kinetics and agonist sensitivity for wild-type GlyR and BioSensor-GlyR. (C) Examples of simultaneous whole-cell and fluorescence recordings from BioSensor-GlyR transfected BHK cells with pipette containing either nominally 0 (left traces) or 150 mM (right traces) Cl−. Glycine (1 mM) was pressure applied for 10 ms duration. (D) Relationship between the amplitude of glycine-induced currents and changes in fluorescence of BioSensor-GlyR at 480 nm. The amplitude of currents was regulated by the changing of Vh. (Modified from Mukhtarov et al., 2008
).
The functioning of this modified GlyR is not perturbed by the inserted Cl-Sensor. This fact was proved in whole-cell recordings of cells expressing BioSensor-GlyR, where rapid application of glycine elicited ionic currents with kinetics similar to those of wild-type GlyR (Figure 5
B). The main functional properties of BioSensor-GlyR, i.e. kinetics, agonist sensitivity and Cl− selectivity, are also similar to those of the wild-type GlyR.
Application of glycine to cells expressing BioSensor-GlyR induced changes in fluorescence (Figure 5
C). The amplitude and direction of fluorescence signals correlated with the amplitude and direction of glycine-induced currents (Figure 5
D), demonstrating that BioSensor-GlyR is a good probe for spectroscopic monitoring of GlyR activation in live cells. The sensitivity of BioSensor-GlyR was high enough to resolve changes in [Cl−]i induced by activation of postsynaptic receptors in glycinergic synapses. The decay kinetics of fluorescence responses were slow compared with those of ionic currents. This might be partially due to the kinetics of Cl− binding by YFP. However, this may also reflect slow intracellular Cl− transients, as monitoring with MQAE, the rapid quinolinium indicator, showed a similarly slow rise and decay of Cl−-dependent fluorescence in cerebellar neurons (see Figure 2
C).
In spite of these limitations, BioSensor-GlyR is a promising tool for spectroscopic monitoring of [Cl−]i changes in the local surroundings of glycine receptor ion channels. Development of transgenic animals expressing Cl-Sensor and BioSensor-GlyR will be particularly useful for studies of inhibitory neuronal networks in brain slice preparations using two-photon microscopy. For the Biosensor-GlyR it might, however, be a difficult task as expression of the GlyR, containing an additional CFP-YFP module in the long cytoplasmic loop, may modify the function of glycinergic synapses.
The development of imaging techniques and specific genetically encoded chloride-sensitive probes has opened new avenues for noninvasive monitoring of this ion in different cell types and cellular compartments in normal and pathological conditions. The main steps stimulating the development of these probes were as follows. First, the discovery of the halide sensitivity of YFP. Second, the production of YFP mutants exhibiting usefully high sensitivity to Cl− at concentrations close to the physiological range of [Cl−]i. Third, the construction of molecules (Clomeleon and Cl-Sensor) consisting of two fluorescent proteins, allowing ratiometric noninvasive estimation of [Cl−]i. Finally, the incorporation of Cl-Sensor into the sequence of the Cl−-selective glycine receptor channel (BioSensor-GlyR), which opens up the ability to monitor synaptic activation of these proteins using imaging techniques. This provides an intriguing background for the development of biosensors which make it possible to monitor the activity of ionotropic GABA receptors and other Cl−-selective channels.
The authors declare that the research was conducted in the absence of any commercial or financial relationships that could be construed as a potential conflict of interest.
This work was supported by the NEUROCYPRES grant from the European Commission Seventh Framework Programme (for Tatyana Waseem and Marat Mukhtarov) and INSERM (Piotr Bregestovski).
Galietta, L. J. V., Springsteel, M. F., Eda, M., Niedzinski, E. J., By, K., Haddadin, M. J., Kurth, M. J., Hantz, M., and Verkman, A. S. (2001c). Novel CFTR chloride channel activators identified by screening of combinatorial libraries based on flavone and benzoquinolizinium lead compounds. J. Biol. Chem. 276, 19723–19728.
Metzger, F., Repunte-Canonigo, V., Matsushita, S., Akemann, W., Diez-Garcia, J., Ho, C. S., Iwasato, T., Grandes, P., Itohara, S., Joho, R. H., and Knopfel, T. (2002). Transgenic mice expressing a pH and Cl− sensing yellow-fluorescent protein under the control of a potassium channel promoter. Eur. J. Neurosci. 15, 40–50.
Miyazaki, H., Shiozaki, A., Niisato, N., Ohsawa, R., Itoi, H., Ueda, Y., Otsuji, E., Yamagishi, H., Iwasaki, Y., Nakano, T., Nakahari, T., and Marunaka, Y. (2008). Chloride ions control the G1/S cell-cycle checkpoint by regulating the expression of p21 through a p53-independent pathway in human gastric cancer cells. Biochem. Biophys. Res. Commun. 366, 506–512.
Munkonge, F., Alton, E. W., Andersson, C., Davidson, H., Dragomir, A., Edelman, A., Farley, R., Hjelte, L., McLachlan, G., Stern, M., and Roomans, G. (2004). Measurement of halide efflux from cultured and primary airway epithelial cells using fluorescence indicators. Cyst. Fibros. 3(Suppl. 2), 171–176.
Pedemonte, N., Sonawane, N. D., Taddei, A., Hu, J., Zegarra-Moran, O., Suen, Y. F., Robins, L. I., Dicus, C. W., Willenbring, D., Nantz, M. H., Kurth, M. J., Galietta, L. J. V., and Verkman, A. S. (2005b). Phenylglycine and sulfonamide correctors of defective ΔF508 and G551D cystic fibrosis transmembrane conductance regulator chloride-channel gating. Mol. Pharmacol. 67, 1797–1807.