- 1Exercise Physiology Laboratory, College of Physical Education and Sports, Beijing Normal University, Beijing, China
- 2Department of Exercise and Rehabilitation, Physical Education College, Hebei Normal University, Shijiazhuang, China
- 3Department of Pharmacology, University of Tennessee College of Medicine, Memphis, TN, United States
Epidemiological studies indicate that physical activity and exercise may reduce the risk of developing Parkinson's disease (PD), and clinical observations suggest that physical exercise can reduce the motor symptoms in PD patients. In experimental animals, a profound observation is that exercise of appropriate timing, duration, and intensity can reduce toxin-induced lesion of the nigrostriatal dopamine (DA) system in animal PD models, although negative results have also been reported, potentially due to inappropriate timing and intensity of the exercise regimen. Exercise may also minimize DA denervation-induced medium spiny neuron (MSN) dendritic atrophy and other abnormalities such as enlarged corticostriatal synapse and abnormal MSN excitability and spiking activity. Taken together, epidemiological studies, clinical observations, and animal research indicate that appropriately dosed physical activity and exercise may not only reduce the risk of developing PD in vulnerable populations but also benefit PD patients by potentially protecting the residual DA neurons or directly restoring the dysfunctional cortico-basal ganglia motor control circuit, and these benefits may be mediated by exercise-triggered production of endogenous neuroprotective molecules such as neurotrophic factors. Thus, exercise is a universally available, side effect-free medicine that should be prescribed to vulnerable populations as a preventive measure and to PD patients as a component of treatment. Future research needs to establish standardized exercise protocols that can reliably induce DA neuron protection, enabling the delineation of the underlying cellular and molecular mechanisms that in turn can maximize exercise-induced neuroprotection and neurorestoration in animal PD models and eventually in PD patients.
Introduction
Parkinson's disease (PD) is a common, age-dependent degenerative neurological disorder caused by a severe loss of the nigrostriatal dopaminergic projection (Kish et al., 1988; Hornykiewicz, 2001; Braak et al., 2004; Kordower et al., 2013), leading to the characteristic motor deficits and symptoms including resting tremor, a slowness and paucity of movements, muscle rigidity, and postural imbalance (Parkinson, 1817; Olanow et al., 2009). Although the dopamine (DA) replacement therapy is effective for relieving the motor deficits, the disease process (Lewy pathology) continues to progress and spread to impair multiple forebrain areas, eventually leading to severe motor function deficits, cognitive impairments, and even dementia (Braak et al., 2004; Katzenschlager et al., 2008; Hawkes et al., 2010; Coelho and Ferreira, 2012; Goedert et al., 2013; Kordower et al., 2013; Del Tredici and Braak, 2016). As the old human population grows due to increased life expectancy, the PD population is also increasing (Zhang et al., 2005; de Lau and Breteler, 2006; Pringsheim et al., 2014), causing an immense suffering to the patients and their families and also creating an enormous economical burden on the society.
There is currently no pharmacological therapy that can modify or slow the disease or protect DA neurons, despite the immense efforts and resources expended in the past five decades since the early 1960s when DA neuron degeneration was first identified as the key pathology of PD (Hornykiewicz, 2001, 2017; Kalia et al., 2015; Bartus and Johnson, 2017a,b). Current pharmacological therapies are capable of only relieving motor symptoms and can not modify or slow the disease progression. Due to the complexity of the disease and using the progress made in the past three decades as a guide, the chances are small in the next three decades for scientists to find a breakthrough pharmacotherapy that can stop, reverse or substantially slow the disease, indicating a major unmet medical need.
Evidence shows that PD has a 10–20 years or even longer presymptomatic phase (Gaig and Tolosa, 2009; Cheng et al., 2010; Hawkes et al., 2010; Savica et al., 2010; Burke and O'Malley, 2013; Noyce et al., 2016; Salat et al., 2016) (Figure 1). This presents an opportunity to modify or slow the pathological progression from presymptomatic phase to overt PD. Thus, in parallel to the continued search for pharmacotherapies, finding alternative, non-pharmacotherapies is highly necessary to delay and slow the DA neuron degeneration and hence the appearance of the PD symptoms. After the appearance of PD symptoms, non-pharmacotherapies can complement existing and future pharmacotherapies to better control the motor and non-motor (cognitive) symptoms and slow the worsening of the PD symptoms.
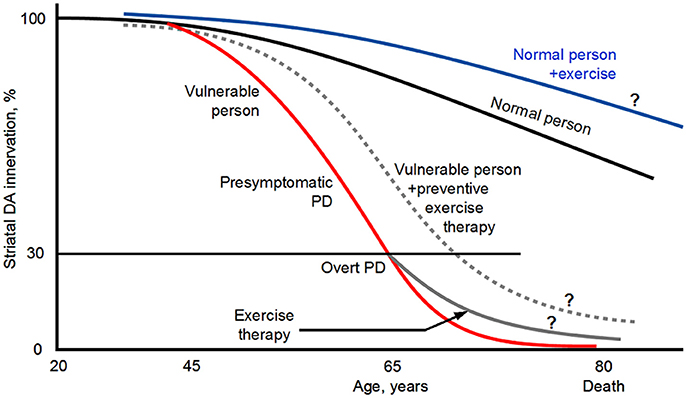
Figure 1. Diagram illustrating that PD has a long presymptomatic period and that exercise may reduce PD risk, delay the appearance of the symptoms, and slow the disease progression. ? indicates that the illustrated possibilities are suggested by indirect evidence but direct evidence is lacking. The diagram is based on Kish et al. (1988), Hornykiewicz (2001), Braak et al. (2004), Gaig and Tolosa (2009), Hawkes et al. (2010), Savica et al. (2010), Coelho and Ferreira (2012), Burke and O'Malley (2013), Goedert et al. (2013), Kordower et al. (2013), Del Tredici and Braak (2016), Noyce et al. (2016) and Salat et al. (2016). The curves and values are approximate because published data are incomplete and variable. Original illustration of Fu-Ming Zhou.
Exercise may be a non-pharmacological treatment for presymptomatic and clinical PD that is non-invasive, does not have side effects but has proven benefits for multiple organ-systems (Vina et al., 2012; Petzinger et al., 2013; Burley et al., 2016; Jackson et al., 2016; Lauzé et al., 2016). In this review, we will first briefly summarize the epidemiological and clinical evidence showing the benefits of exercise for PD patients; then we will focus on the basic science aspects of exercise's benefits in PD, particularly the striatum and nigrostriatal DA projection, as they are critically important to movement control. We will summarize, discuss, synthesize clinical, and basic science evidence that exercise may have neuroprotective effects on the nigrostriatal DA system. Equally, important, we will also discuss the critical knowledge gaps in the field where future research is needed to move the field forward and to produce clinically actionable basic science results to benefit PD patients.
Epidemiological Evidence for a Potential Exercise-Induced Protection Against Parkinson's Disease
In 1992, Sasco et al. published the results of long term longitudinal epidemiological study on a potential association between the occurrence of PD and physical exercise in 50,002 men who attended Harvard University in Cambridge, Massachusetts, or the University of Pennsylvania in Philadelphia, Pennsylvania, between 1916 and 1950 and were followed up into adulthood for disease and mortality information. This study found that having done regular physical exercise in college was associated with a lower risk for PD, providing the first scientific evidence that exercise may provide a protection against PD (Figure 1).
Since the pioneering study of Sasco et al. (1992), several follow-up large sample epidemiological studies have also examined the correlation between physical activities in early adulthood and PD occurrence in later years. The general conclusion from these epidemiological studies indicate that PD occurrence is lower in people with moderate to vigorous general physical activity and recreational activity (e.g., running, swimming, tennis, bicycling, aerobics, dancing), suggesting that physical activity in early adulthood may reduce PD risks in later years (Chen et al., 2005; Thacker et al., 2008; Xu et al., 2010; Sääksjärvi et al., 2014; Yang et al., 2015; Shih et al., 2016) (Figure 1). Since the exercise and physical activity started a long time (>2–3 decades) before the usually old onset age (~65 years), it appears that the beneficial effects probably starts to protect the DA and basal ganglia (BG) systems long before PD clinical symptoms start and when the DA neuron degeneration is just beginning such that the cellular damages may be more repairable. Certainly, these studies can not exclude the small possibility that people predisposed to PD are physically less active. We also need to note here that one low sample study found no correlation between physical activity and PD risks (Logroscino et al., 2006). Thus, taken together, the majority of the epidemiological data indicate that physical exercise in early adulthood reduces PD risk (Figure 1).
Exercise Benefits for PD Patients: Clinical Evidence
Clinical studies have examined potential therapeutic effects of exercise on PD and reported positive results. For example, 50 min treadmill exercise, three times a week for 3 months improved gait and locomotion speed in PD patients (Shulman et al., 2013). Another study reported that aerobic exercise training improved motor function and motor learning in PD patients (Duchesne et al., 2015). In a large sample study based on National Parkinson Foundation patient database, regular exercise was associated with better quality of life, mobility, and physical function, slower symptom progression, less caregiver burden and less cognitive decline (Oguh et al., 2014; Rafferty et al., 2017). It has also been reported that a 24-week Tai Chi exercise improved balance and gait function while reducing falls in PD patients (Li F. et al., 2012, 2014), although another study indicated that a 16-week Tai Chi exercise failed to produce detectable beneficial effect on the motor function in PD patients (Amano et al., 2013). Additional studies have indicated that that exercise can play an important role in slowing the physical and cognitive decline resulting from PD (Corcos et al., 2013; LaHue et al., 2016; Reynolds et al., 2016; Dipasquale et al., 2017). Physical exercise has also been reported to produce synergistic benefits with L-dopa for improving motor functions in PD patients (Kang et al., 2012).
Taken together, these clinical and epidemiological studies have provided evidence supporting the conclusion that exercise not only has a significant preventive effect on PD, but also has therapeutic value by reducing the symptoms and slowing the symptom and disease progression (Figure 1), and should be promoted to the general population, PD vulnerable population in particular (Burley et al., 2016; Jackson et al., 2016; Lauzé et al., 2016). These studies also indicate that physical exercise needs to be prescribed to PD patients and be an essential component of the treatment for PD (Ahlskog, 2011; Vina et al., 2012; Shulman et al., 2013; Bloem et al., 2015; Pedersen and Saltin, 2015; LaHue et al., 2016; Lauzé et al., 2016; Reynolds et al., 2016) (Figure 1). Indeed, the highly respected International Parkinson and Movement Disorder Society has designated exercise as an adjunct therapy for PD (Fox et al., 2011).
Although much remains to be understood, exercise's benefits for PD are likely to be mediated partly by exercise-induced improvement of the general health (e.g., increasing the cardiovascular and cerebrovascular function), the function of the skeletal musculature and also the function of the broad motor control neural systems including cerebral motor cortices, BG, the cerebellum and the thalamus (Beall et al., 2013; Petzinger et al., 2013; Singh et al., 2014; Wang et al., 2015a,b; Alberts et al., 2016; Burley et al., 2016; Jackson et al., 2016; Shah et al., 2016). Additionally, because of the known importance of the nigrostriatal DA system and the cortico-BG circuitry in motor function, a large number of studies have examined how exercise directly affects these neural systems. Below, we will summarize and synthesize these studies, starting with describing the basic anatomy and physiology of the nigrostriatal DA system and cortico-basal ganglia circuits, likely key neural targets for exercise intervention.
Anatomy of the Nigrostriatal DA System and the Basal Ganglia
Components of the Basal Ganglia and the Nigrostriatal DA System
The basal ganglia (BG) are a group of interconnected subcortical nuclei including the striatum, globus pallidus external segment (GPe) and internal segment (GPi), the subthalamic nucleus (STN), the substantia nigra pars compacta (SNc), and pars reticulata (SNr) (Gerfen and Bolam, 2017; Zhou, 2017) (Figures 2A,B). In primates, the striatum comprises the caudate nucleus and putamen. The striatum is the major input nucleus for the BG receiving glutamatergic inputs from motor and somatosensory cortices and other cortical areas (Deng et al., 2015) and the thalamus (Smith et al., 2014). The GABAergic medium spiny neurons (MSNs), comprising 90% of the striatal neurons, are the projection neurons of the striatum and critical to multiple important brain functions as indicated by the profound behavioral consequences of Huntington's disease in which MSNs are lost (Glass et al., 2000) and PD in which striatal DA innervation is lost (Kish et al., 1988; Kordower et al., 2013). One group of MSNs heavily express DA D1 receptors (D1Rs) and project to and inhibit the high frequency firing GABAergic neurons in the GPi and the SNr, the output nuclei of the basal ganglia, forming the direct pathway (Gerfen and Bolam, 2017; Zhou, 2017). The other group of MSNs heavily express D2Rs and project to and inhibit the high frequency firing GABAergic neurons in the GPe, forming the indirect pathway. The high frequency firing GPe GABAergic neurons project to and inhibit the glutamatergic STN neurons (Figure 2A). The STN comprises of a cluster of spontaneously firing glutamatergic neurons that excite GPi/SNr GABAergic neurons (Parent and Hazrati, 1995; Nambu, 2008, 2011; Kita and Kita, 2011; Sano et al., 2013).
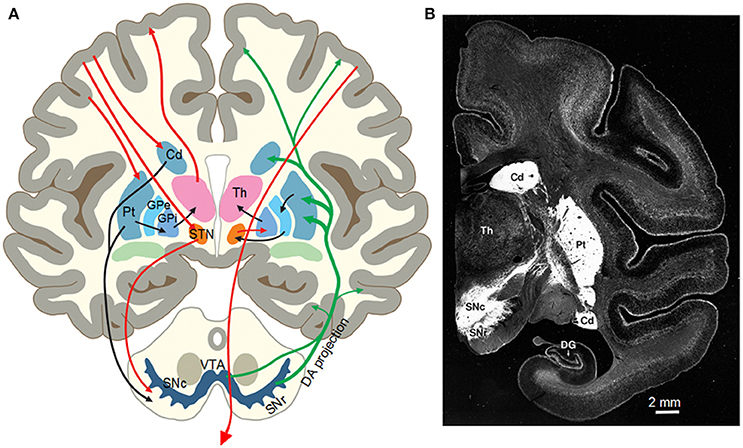
Figure 2. (A) Diagram of the nigrostriatal system and the basal ganglia circuitry of the human brain. From Zhou et al. (2003) with permission. (B) Photograph of a monkey brain coronal section showing the intense DA innervation in the striatum (the putamen and the caudate nucleus) indicated by DAT immunostain. Modified from Lewis et al. (2001) with permission.
A distinct feature of the basal ganglia is the nigrostriatal DA projection and the intense DA innervation in the striatum (Figure 2B). Though the number of cell somata is quite small (Oorschot, 1996; Hardman et al., 2002), the projection axons of the midbrain DA neurons bifurcate repeatedly in the striatum, eventually forming an extremely dense DA axon network in rodents and primates including humans (Levey et al., 1993; Ciliax et al., 1999; Prensa et al., 2000; Lewis et al., 2001; Matsuda et al., 2009; Ding et al., 2015; Morigaki and Goto, 2016). Similarly, the expression levels of D1Rs and D2Rs in the striatum are also extremely high, the highest in the brain in both rodents and primates including humans (Levey et al., 1993; Yung et al., 1995; Hurd et al., 2001; Zhou, 2017), providing the anatomical and molecular substrates for intense DA signaling in the striatum and for DA's profound behavioral effects including motor stimulation.
Motor Function of the Striatum and the Nigrostriatal DA System
The striatum and the segregated D1-MSN and D2-MSN pathways together with the intense DA regulating system process and integrate sensory, motor, cognitive, and motivational information, and then produce output signals to feedback to cortical, subcortical, and brainstem areas (Tremblay et al., 2015; Haber, 2016). Thus the striatum can affect these motor and non-motor behaviors, and loss of the DA regulation may render the striatum and hence motor and cognitive control mechanisms dysfunctional. The motor function of the striatum and the MSNs is clearly demonstrated when the striatum becomes dysfunctional and loses its normal function, e.g., the abnormal choreic movements in Huntington's disease (Walker, 2007), likely due to the loss of MSNs, especially those in the indirect pathway (Mitchell et al., 1999; Glass et al., 2000; Walker, 2007; Obeso et al., 2014). Consistent with these clinicopathological data, experimental ablation or inactivation of indirect pathway MSNs increases motor activity (Sano et al., 2003, 2013; Durieux et al., 2009, 2012; Bateup et al., 2010; Chiken et al., 2015). It is now clear that D2-MSN activity and the striatopallidal output inhibit movement (hence movement disinhibition in PD), and D1-MSN activity and the consequent striatonigral output facilitate movement (Kravitz et al., 2010; Cui et al., 2013; Sano et al., 2013; Friend and Kravitz, 2014; Jin et al., 2014).
DA activity in the striatum is absolutely required for normal motor function in both animals and humans. This is demonstrated by the fact that local drug infusion into the striatum to block striatal DA receptors induce PD-like akinesia (Franco and Turner, 2012). Local toxin infusion lesioning the striatal DA innervation also leads to motor deficits in animals (Lee et al., 1996; Kirik et al., 1998; Bagga et al., 2015; Willard et al., 2015). In humans, DA neuron degeneration leads to PD (Hornykiewicz, 2001, 2017). The accidental destruction of the nigrostriatal DA system in 1-methyl-4-phenyl-1,2,3,6-tetrahydropyridine (MPTP)-poisoned patients and their motor deficits have provided further confirmation (Ballard et al., 1985; Vingerhoets et al., 1994). These results are consistent with the fact that DA innervation and DA receptor expression are highly concentrated in the striatum (Levey et al., 1993; Yung et al., 1995; Gerfen and Bolam, 2017; Zhou, 2017) (Figures 2A,B). Thus, the DA system's main motor-promoting function is mediated by D1-MSNs and D2-MSNs, although dopaminergic activity in other brain areas may contribute additional complexity to motor control and parkinsonism. These conclusions are further supported by the facts that a total inhibition of L-dopa synthesis in brain DA neurons leads to akinesia and being to unable to feed and drink (Zhou and Palmiter, 1995). Inhibition of DA release by blocking action potential propagation of the nigrostriatal DA axons in the medial forebrain bundle also induces akinesia (Galati et al., 2009).
Exercise Effects on the Nigrostriatal DA System in Animal PD Models
Many studies have investigated the potential beneficial effects of exercise on the nigrostriatal DA system and motor function in animal models of PD, usually toxin-induced DA denervation rodent models. Large amounts of positive results have been obtained, but negative results have also been reported. The main findings from these studies are listed in Table 1 and summarized and discussed below.
Positive Results on Exercise's Neuroprotective Effects on the Nigrostriatal System
Since the key pathology causing the motor deficits in PD patients and PD animal models is a severe loss of the nigrostriatal DA projection (Kish et al., 1988; Hornykiewicz, 2001; Franco and Turner, 2012; Li et al., 2015), an obvious important question is if the exercise-induced motor benefits in PD animal models and patients is at least partially mediated by a preservation or protection of residual nigrostriatal DA neurons, in addition to exercise's beneficial effects directly on skeletal musculature. So far, there is no study that has directly investigated this question in PD patients for the obvious difficulty in obtaining postmortem tissues in appropriate human subjects. However, many studies have been performed in animal PD models to investigate this question, starting with the publication of the study on the neuroprotective effect of forced limb use on DA neurons in a unilateral 6-OHDA rat PD model in 2001 (Tillerson et al., 2001). So the field is still young.
In their pioneering studies, Schallert and his collaborators exploited the established use-promoting-recovery principle well-known in the neurorehabilitation literature (Jones and Schallert, 1994; Schallert et al., 1997, 2000; Takamatsu et al., 2010; Korol et al., 2013; Mang et al., 2013; Tamakoshi et al., 2014) and made seminal observations about the neuroprotective effects of “forced use” of the DA-lesion impaired forelimb (the limb contralateral to the DA lesion site). “Forced use,” achieved by casting the unimpaired limb 7 days before or immediately after the unilateral MFB 6-OHDA lesion, was needed because the rat does not understand human instruction. They found that forced use of the DA lesion-impaired limb drastically reduced the motor deficits of the impaired limb and also substantially reduced striatal tissue DA content loss (determined by HPLC) (Tillerson et al., 2001, 2003; Cohen et al., 2003). In contrast, forced non-use of the impaired limb immediately following the lesion worsened the motor deficits and striatal DA content loss (Tillerson et al., 2002). These results provide evidence that activity of the impaired limb promotes the recovery of the motor deficits and also the recovery of the nigrostriatal DA innervation, or protects DA neurons from 6-OHDA lesion, or both increasing neuroprotection and recovery. These studies laid a foundation for the research field.
Details of their experimental protocol are important. In their rat model with the casting/forced use protection, 6-OHDA lesion reduced the striatal DA content to ~25% of the normal level (Tillerson et al., 2001). This indicates that the lesioned striatum had significant numbers of residual DA axons; these residual DA axons may sprout upon appropriate stimulation and nourishing such as by neurotrophic factors, contributing to the observed DA recovery in the striatum. Such a non-complete DA denervation in the striatum may be necessary to produce a significant neuroprotection and recovery. If the lesion is a total or near DA denervation in a striatal subregion, usually the dorsal striatum, such as in late stage PD, it may be impossible for neuroprotection and recovery to be realized for the DA axon terminals in the dorsal striatum and associated DA neurons.
Additionally, it was shown that these behavior- and DA neuron-protecting effects were reduced when the forced use of the impaired limb was initiated 3 or 7 days after the lesion (Tillerson et al., 2001) (Table 1), indicating an effect of neuroprotection rather than enhancing recovery. Timing of neuroprotective procedures (e.g., exercise) is important. The reduced amphetamine-induced asymmetric rotation in exercised PD animals is another indication of reduced DA denervation and hence reduced DA supersensitivity in the lesioned side (Ungerstedt, 1971a; Schwarting and Huston, 1996). These results provide strong evidence that an appropriately designed exercise treatment regimen can reduce experimental toxin-induced DA neuron lesion and loss that in turn can reduce motor deficits, although exercise may also improve the motor function by improving the general health condition of the animal. These results also suggest that for exercise to have the maximal beneficial effects, it should start early, before the disease (any disease) is diagnosed, particularly when PD is diagnosed, the striatal DA loss has reached 70% and many good opportunities may have been lost. Thus, exercise should start at a young age. These studies laid a strong foundation for studying exercise's neuroprotective effects. Additionally, the forced use of the impaired limb is an excellent experimental design because the impaired limb was forced to performed natural motor activities that the limb was performing before the lesion; perhaps, more importantly, the reported behavioral improvements and DA neuron protection were robust. Although natural forelimb use is difficult to quantify, the practical importance is not diminished because natural limb use can be more easily implemented in experimental animals and humans, and has a high translational value.
The studies discussed above (Tillerson et al., 2001, 2003; Cohen et al., 2003), however, did not examine anatomical improvements of residual and potentially new DA axons in the striatum during the forced use-induced DA neuroprotection and neurorestoration; residual DA neurons and potentially repaired DA neurons (i.e., ghost DA neurons without TH) were also not examined. Future studies are needed to fill these critical knowledge gaps.
Schallert and his collaborators also expanded their forced limb use paradigm in unilateral 6-OHDA lesioned rats to the more quantifiable exercise on treadmill and running wheels in unilateral 6-OHDA rats and bilateral MPTP-lesioned mice, and replicated their earlier results of limb use's (i.e., exercise's) protection of the DA neurons or exercise's promotion of DA neuron recovery from MPTP lesion in mice and 6-OHDA lesion in rats (Tillerson et al., 2003). Since then, other laboratories have performed follow-up studies and confirmed and expanded the original findings on exercise's neuroprotective effects on DA neurons in mouse MPTP and rat 6-OHDA PD models (Tillerson et al., 2003; Garcia et al., 2017; Real et al., 2017; Shi et al., 2017). For example, using intrastriatal 6-OHDA DA lesion in rats, Yoon et al. (2007) and Tajiri et al. (2010) reported similarly strong DA neuron protection in unilateral 6-OHDA rat PD model produced by treadmill exercise that was initiated 1 day before lesion and resumed 24 h after lesion and continued for 2–4 weeks. Exercise-induced neuroprotection of DA neurons has also been reported in MPTP mouse model of PD by Richard Smeyne's group (Gerecke et al., 2010; Smeyne et al., 2015). These authors found that in a pre-lesion unrestricted wheel running exercise regimen Gerecke et al. (2010), 1 month running did not provide any protection, 2 months running provided partial protection, and 3 months running provided complete protection such that nigral DA neuron numbers and striatal tissue DA level became normal, indicating the importance of exercise duration for exercise to induce neuroprotection for DA neurons. Furthermore, Gerecke et al. (2010) also reported that the intensity of exercise is critical for the induction of neuroprotection: In the 3 months pre-lesion wheel running exercise regimen, the full daily dose 7.5 km (18,000 daily wheel revolutions) provided complete neuroprotection for DA neurons against MPTP, 2/3 of the full daily exercise dose (12,000 wheel revolutions or 4.8 km) provided partial neuroprotection, whereas 1/3 of the full daily exercise dose (6,000 wheel revolutions or 2.4 km). These data indicate the importance of exercise intensity in inducing neuroprotection.
In summary, as listed in Table 1, many studies have reported that in parallel to improved motor function, exercise induced a substantial protection of DA neurons in rodent MPTP or 6-OHDA PD models (Figure 3) (Tillerson et al., 2003; Yoon et al., 2007; Zigmond et al., 2009; Gerecke et al., 2010; Tajiri et al., 2010; Lau et al., 2011; Smeyne et al., 2015; Shi et al., 2017). Additionally, in the rat MPP+ PD model, a 4-week pre-lesion treadmill exercise regime reduced intrastriatal MPP+-induced nigrostriatal DA loss (Tsou et al., 2015).
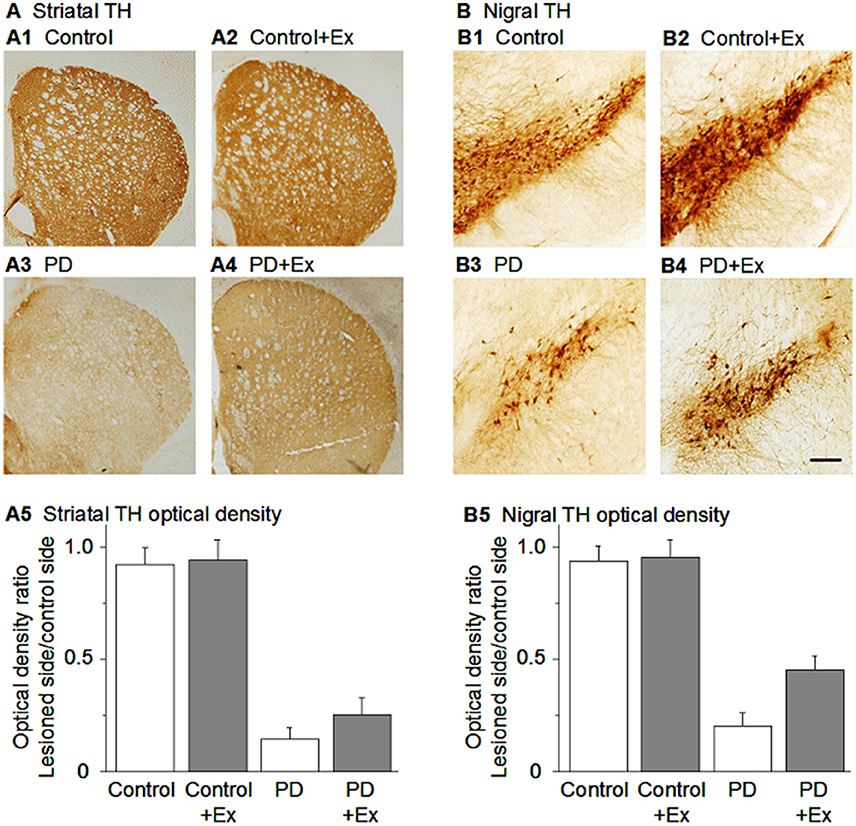
Figure 3. Exercise-induced neuroprotection of DA neurons in the SNc and DA axon terminals in the striatum. (A) TH immunostain showing DA axonal innervation under the four conditions. (B) TH immunostain showing DA neurons in the substantia nigra under the four conditions. Scale bar in (B4): 0.1 mm for (A) and 0.05 mm for (B). Modified from Shi et al. (2017) with permission.
Sources of the New or Recovered DA Axons
A critical question is where the new or recover DA axons come from. There are four possible sources. The first is the existing, lesioned DA axons. These lesioned DA axons and DA neurons may have suppressed their TH expression such that they become invisible ghost axons and cells in immunostaining examination, and certainly DA level is also reduced, and these lesioned but not destroyed DA cells and axons will recover over time, especially when the animal and hence the DA neurons are young. This recovery of ghost DA neurons and axons has been documented in 6-OHDA and MPTP-lesioned animals (Sanchez-Ramos et al., 1988; Tatton et al., 1990; Lu and Hagg, 1997; Hagg, 1998). Thus, the lesioned DA neurons and axons may transiently lose TH expression and become invisible to TH stain, but these invisible or ghost DA axons and DA cells may re-appear upon appropriate exercise intervention. Future studies need to determine this possibility. The second potential source is the sprouting of the residual DA axons in the striatum. Studies have documented striatal DA axon sprouting after DA toxin lesion in rodents and monkeys (Bezard et al., 2000; Elsworth et al., 2000; Song and Haber, 2000; Stanic et al., 2003a,b). Exercise intervention may promote the sprouting of residual DA axons, contributing to the apparent neuroprotection and recovery. To our knowledge, none of the exercise studies discussed earlier examined anatomical evidence for axonal sprouting; future studies are needed to fill this critical knowledge gap. The third potential source is the generation of local striatal DA neurons. Studies have reported that the striatum can produce local striatal TH-positive, potentially DA-secreting neurons, converted from GABA interneurons after DA denervation, as a compensatory response (Ibáñez-Sandoval et al., 2010), although recent studies indicate that these TH-positive neurons are limited in numbers, do not release DA, and are not functionally significant (Xenias et al., 2015). The fourth potential source is new DA neurons born in the SNc. However, there is currently no convincing evidence that the nigral area can produce new DA neurons in adulthood. Even if new DA neurons are born there, it is not likely that their axons can reach the striatum and repeatedly bifurcate there. So this source is unlikely.
The potential molecular mechanisms underlying exercise-induced neuroprotection and neurorestoration of the nigrostriatal DA system are discussed below in section Molecular Mechanisms Underlying Exercise-Induced Neuroprotection of the Da Neurons and Corticostriatal Circuit.
Negative Results on Exercise's Neuroprotective Effects on the Nigrostriatal System
As listed in Table 1, several studies have reported that although improving motor function, exercise intervention did not alter the striatal tissue DA or TH level, nor the numbers of nigral DA neurons and DA axon terminals in the striatum in mouse and rat DA lesion PD models (O'Dell et al., 2007; Petzinger et al., 2007; Gorton et al., 2010; VanLeeuwen et al., 2010; Kintz et al., 2013; Toy et al., 2014; Sconce et al., 2015; Hood et al., 2016; Churchill et al., 2017). For example, using the subactue MPTP C57BL/6J mouse model and a treadmill exercise regime that started 5 days after lesion and lasted for 28 days, Petzinger et al. (2007) and Gorton et al. (2010) reported that exercise did not alter the striatal tissue DA level, although the animals' balance function was improved. In a chronic, progressive MPTP young 2-month adult and also 16-month old mouse model, it has been reported that voluntary wheel running or treadmill exercise did not protect dopamine neurons in the substantia nigra and DA axon terminals in the striatum, but did improve the mouse's paw grip and gait (Sconce et al., 2015; Hood et al., 2016; Churchill et al., 2017). It has also been reported that although not altering tissue DA content, exercise may reduce DAT expression and DA reuptake and thus enhance the residual DA signal, as indicated by the prolonged DA signal monitored by fast cyclic voltammetry, providing another route for exercise to enhance the lesioned, residual nigrostriatal DA system (Petzinger et al., 2007).
These studies reporting a lack of DA neuron protection, however, used relatively low intensity treadmill exercise that was started following a 5-day waiting period after MPTP injection was completed or used voluntary wheel running. As reported in other studies, exercise-induced neuroprotection of DA neurons is critically dependent on the timing of the exercise regimen (Tillerson et al., 2001) and the duration and intensity of the exercise regimen (Gerecke et al., 2010). The use of the 5-day waiting period has probably two reasons: first, the mice are acutely systemically intoxicated in the 5 days following intraperitoneal (IP) injection of MPTP such that exercise is not practical for these mice; second, animal use regulations often impose a 5-day waiting period for MPTP-treated mice. Thus, post-lesion exercise may not be suitable for studying exercise-induced neuroprotection in the mouse MPTP model. Pre-lesion exercise may be a better alternative for mouse MPTP model, as used by Gerecke et al. (2010) and Smeyne et al. (2015). Certainly, the post-lesion exercise with the 5-day waiting period in MPTP mouse model may be used to study the potential neurorestorative effects in the DA and non-DA systems; and beneficial effects may be obtained via non-dopaminergic mechanisms, even if the DA neurons are not protected or restored (Petzinger et al., 2007; Sconce et al., 2015; Hood et al., 2016; Churchill et al., 2017).
Potential Causes of the Discrepancy
The studies discussed above indicate a major discrepancy: a large number of studies reported neuroprotective effects of exercise on the DA system, but several studies reported a lack of such a neuroprotective effect (Table 1). At this moment, we do not know the cause(s) of this discrepancy, but the following factors can contribute.
DA Lesion Severity
The more complete the DA axon denervation/destruction, the less likely a neuroprotection, restoration can be obtained. This is because at least a major source of the recovered DA axons/DA neurons are likely from ghost DA axons/neurons and/or DA axon sprouting from residual DA axons (Elsworth et al., 2000). Clinical trials of neuroprotection therapies in PD patients also suggest that neuroprotection/neurorestoration is difficult or impossible to realize when the pathology is too severe or the neurons are too sick or dead to be repaired (Bartus and Johnson, 2017a). However, DA lesion severity data were often not explicitely described such that a determination can not be made.
Exercise Initiation Timing
When initiated before the DA neuron and axons are completely destroyed, exercise may help DA neuron/axons survive and eventually recover. When exercise starts after the DA neuron and axons are completely destroyed, it is too late and no neuroprotection or repair is possible. This idea was supported by the results of Tillerson et al. (2001) that forced use of the lesioned forelimb initiated 24 h after the lesion effectively protected/recovered the striatal DA system, and delayed start of forced use decreased this neuroprotection, a 7-day delay rendered the procedure entirely ineffective. This possibility may contribute to the negative results that were obtained in studies in which the exercise intervention was initiated 5 days after DA lesion (O'Dell et al., 2007; Petzinger et al., 2007; Hood et al., 2016). As indicated by Table 1, studies reporting a neuroprotective on DA neurons commonly administered exercise before and/or immediately after DA lesion surgery, whereas studies reporting a lack of neuroprotective effect on DA neurons commonly administered exercise after a long delay.
Exercise Intensity
Low intensity exercise may not trigger the production of enough neuroprotective and neurorestorative molecules. This possibility is clearly indicated by the results of Gerecke et al. (2010) on the impact of exercise dose on the neuroprotective effect on DA neurons. It is possible that high intensity exercise may trigger the production of large amounts of neuroprotective molecules to exert neuroprotective effects.
Animal Age
Neuroprotection and repair are likely more difficult to obtain in older animals than in young animals (Fox et al., 2001). Neurodegeneration is strongly age-dependent, and DA neurons and their axon terminals in the striatum in old animals are more vulnerable to toxins such as MPTP and 6-OHD (Finnegan et al., 1995). However, both the positive and negative studies reviewed here used young adult (3–4 months of age) mice or rats. Thus, animal age is apparently not a factor contributing to the discrepancy.
Exercise Effects on MSN Anatomy, Intrinsic Physiology and Cortical Synaptic Inputs in Animal PD Models
Baseline Properties of MSNs
MSNs are a unique class of neurons in the brain. Anatomically, they have well-developed, rich dendritic spines (Kemp and Powell, 1971; Preston et al., 1980; Wilson and Groves, 1980; Bishop et al., 1981; Chang et al., 1981; McNeill et al., 1988; Kawaguchi et al., 1989, 1990; Kincaid et al., 1998; Fujiyama et al., 2011). These dendritic spines are the locations for MSNs to receive and process synaptic inputs, especially glutamatergic inputs from the cerebral cortex and the thalamus (Gerfen and Bolam, 2017). Physiologically, due to tonically active outward K currents such as the inward rectifier, MSNs have a very hyperpolarized resting membrane potential and do not fire spikes unless receiving strong or synchronized excitatory synaptic inputs from the cortex and the thalamus (Wilson and Kawaguchi, 1996; Tseng et al., 2001; Kasanetz et al., 2006; Mahon et al., 2006; Kita and Kita, 2011). The main excitatory synaptic drives to striatal MSNs receive glutamatergic synaptic inputs from the cerebral cortex and thalamus (Deng et al., 2015). MSNs rely on the cortical and thalamic glutamatergic inputs (EPSCs) to trigger spike output (Doig et al., 2010; Huerta-Ocampo et al., 2014; Smith et al., 2014). High levels of D1Rs are expressed near the glutamatergic synapses in the dendrites/spines, indicating that DA/D1 agonism may affect both cortical and thalamic inputs (Moss and Bolam, 2008; Gerfen and Bolam, 2017). D1R agonism may enhance NMDA receptor (NMDA-R)- and AMPA-R-mediated currents in D1-MSNs (André et al., 2010), and D2Rs commonly inhibit NMDA-Rs and AMPA-Rs (Tritsch and Sabatini, 2012).
MSN activity is critical to the motor function in animals including humans. Specifically, activation of the D1-MSNs in the direct pathway facilitates movements, whereas activation of D2-MSNs inhibits movements; coordinated activation of D1-MSNs and D2-MSNs confers the animal with normal motor control function (Bateup et al., 2010; Kravitz et al., 2010; Cui et al., 2013; Friend and Kravitz, 2014; Jin et al., 2014; Tecuapetla et al., 2016). Under parkinsonian condition, D2R-mediated DA inhibition of D2-MSNs is lost such that D2-MSNs become more excitable than under normal condition, thus increasing the spiking activity (Singh et al., 2016; Shi et al., 2017). Thus, MSN activity may be a key target for exercise to affect.
Exercise Effects on MSN Spine Loss in Animal PD Models
Studies in human PD brains have indicated a dendritic atrophy and a dendritic spine loss in MSNs (McNeill et al., 1988; Stephens et al., 2005; Zaja-Milatovic et al., 2005). A similar dendritic atrophy and dendritic spine loss have been observed in MPTP monkey PD models (Villalba and Smith, 2011; Villalba et al., 2015) and rodent PD models (Ingham et al., 1998; Day et al., 2006). Enlargment of synapses (number of perforated synapses) was reported to be increased in human PD patients (Muriel et al., 2001), monkey PD model (Villalba and Smith, 2011; Villalba et al., 2015), and rat PD model (Ingham et al., 1998). Given the critical role of striatal MSNs in motor control and other brain functions, an important question is: Does exercise affect MSN structure (dendrites and spines) and function? There is currently no human data on this question and only a few studies in experimental animals. Here we will summarize and discuss the data from these studies.
In mice assessed using the classic Golgi staining method supplemented by intracellular staining, Toy et al. (2014) reported that MPTP DA lesion (90% DA loss) caused a ~20% loss of dendritic spines in both D1- and D2-MSNs; a 6-week intensive treadmill exercise regimen, initiated even 5 days after MPTP lesion, reversed the dendritic spine loss in both D1- and D2-MSNs in the dorsolateral striatum (potentially by forming new spines), enhanced dendritic arborization, and increased the expression of synaptic proteins PSD-95 and synaptophysin, accompanied by a normalization of MPTP lesion-induced motor deficits; however, the striatal tissue DA content was not affected by exercise; instead, the same lab reported previously that exercise decreased DA uptake, thus increasing DA availability (Petzinger et al., 2007). Toy et al. (2014) also reported that a minimum of 5 weeks of the exercise training was needed to reverse the motor deficits with 4 weeks being insufficient. Furthermore, that study also reported that exercise increased MSN dendritic spine density in normal mice, indicating that exercise can promote new spine formation (Toy et al., 2014). Since exercise was initiated 5 days after the 1-day subacute MPTP lesion, i.e., when the toxin-induced lesion was complete, exercise's reverse of dendritic spine loss can be considered a form of neurorestoration or repair. At the Beijing Normal University Exercise Physiology Laboratory, we have also observed that in 6-OHDA-lesioned rats, a 4-week treadmill exercise regimen initiated 1 day after the 6-OHDA lesion surgery partially reversed MSN dendritic spine loss (Chen et al., 2015a) (Figure 4).
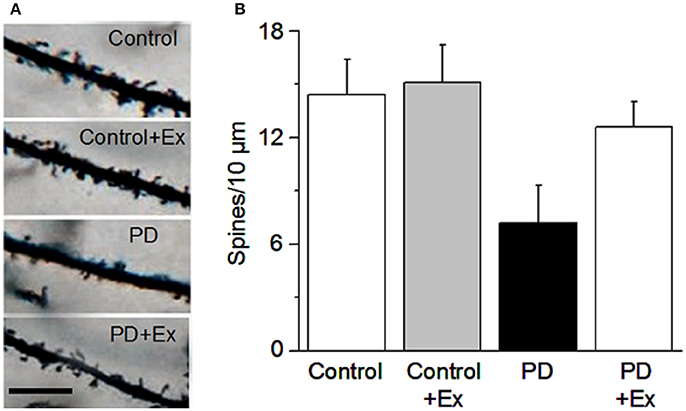
Figure 4. Exercise protects MSN dendritic spines. (A) Example dendritic spines under the four conditions. Scale bar, 5 μm. (B) Quantification of dendritic spines under the four conditions. Modified from Chen et al. (2015a) with permission.
In addition to the reported dendritic spine loss in MSN in PD patients and both D1- and D2-MSNs in monkey MPTP PD model, combined immunohistochemical and ultrastructural studies have indicated that corticostriatal and thalamostriatal axospinous synapses on MSN dendritic spines increase their volume and increase PSD size and PSD perforation (more perforated synapses), larger presynaptic glutamatergic axon terminals, providing an anatomical foundation for increased glutamatergic synaptic transmission at these remaining synapses (Villalba and Smith, 2011; Villalba et al., 2015), compensating for the loss of MSN dendritic spines. But this issue is not settled because some studies reported that 6-OHDA lesion only caused spine loss in D2-MSNs but not in D1-MSNs in mice (Day et al., 2006). In MPTP lesioned monkeys, it has been reported that dendritic spine loss are in both D1- and D2-MSNs (Villalba and Smith, 2011; Villalba et al., 2015); yet another study reported that MPTP lesion in monkeys reduced dendritic spines in D2-MSNs and increased dendritic spines in D1-MSNs (Scholz et al., 2008). In PD patient postmortem brains, Anglade et al. (1996) reported that “the size and density of dendritic spines and the size of postsynaptic density perforations were unchanged” in MSNs in the caudate nucleus. Also in PD brains, Muriel et al. (2001) reported a 50% increase in the number of perforated synapses on D1-MSN dendritic spines while seeing no change in the number of perforated synapses on non-D1R (i.e., D2-MSN) spines in striatal neurons. The reasons for these discrepancies are not known. Future studies are needed to establish the anatomical abnormalities in MSNs in PD brains and animal PD models.
At the Exercise Physiology Laboratory at Beijing Normal University, we have also investigated the potential effects of exercise on the structure and function of MSNs in the classic unilateral medial forebrain bundle 6-OHDA injection lesion rat PD model (Ungerstedt, 1971a,b). Twenty-four hours after the lesion surgery, rats in exercise group receive a 4-week exercise intervention, using a motorized treadmill at a speed of 11 m/min (30 min/day, 5 days/week), a common exercise regimen in the literature (e.g., Tajiri et al., 2010). The broad spectrum DA agonist apomorphine was subcutaneously injected to the rats on 7, 14, and 28 days to induce contralateral rotations as an indirect readout of DA lesion in the striatum. After the final behavioral tests, the rats were euthanized and the brains were harvested for anatomical and biochemical examination. Our behavioral tests showed that compared with PD rats, PD rats in the exercise group had fewer apomorphine-induced rotations, indicating lower DA sensitization and more residual DA innervation (Chen et al., 2015a). Additionally, our Golgi staining data indicate that 6-OHDA lesion induced a substantial decrease in the total spine density in the 6-OHDA-lesioned rat striatum (Chen et al., 2015a) (Figure 4); ultrastructural studies using electron microscopy indicated an increased perforated synapses in the these 6-OHDA-lesioned rats (Chen et al., 2015b). Although we did not identify D1- and D2-MSNs, the general decline in spines in our 6-OHDA rat model is consistent with a global spine loss situation reported for MPTP-lesioned monkeys (Villalba and Smith, 2011; Villalba et al., 2015). Further, our Western blotting experiments indicated that 6-OHDA lesion decreased the expression of glutamate NMDAR1 and GluR2 in the striatum. Equally important, our exercise intervention regimen partially reversed the MSN spine loss accompanied with motor behavior improvements (Chen et al., 2015a) (Figure 4).
The potential molecular mechanisms underlying exercise-induced apparent neuroprotection of the MSN spines are discussed below in section Molecular Mechanisms Underlying Exercise-Induced Neuroprotection of the Da Neurons and Corticostriatal Circuit.
Exercise Restores Corticostriatal Glutamatergic Neurotransmission
Since cortical glutamatergic inputs, together with the thalamic glutamatergic inputs, are the main force that drives MSN spiking activity (Kita and Kita, 2011) and may contribute to exercise-induced motoric benefits in PD, we have studied the effects of exercise intervention on the corticostriatal glutamatergic neurotransmission in the unilateral 6-OHDA rat model of PD, using immunohistochemical, biochemical, and ultrastructural techniques (Chen et al., 2015a,b). We found that a 4-week exercise intervention normalized both the increased glutamate level and the decreased NMDA receptor subunit 1 in the dorsal striatum in PD rats, determined by HPLC and biochemical methods, respectively (Chen et al., 2015b). Our electron microscopic ultrastructral studies showed that exercise intervention normalized the DA denervation-induced increase in perforated asymmetric, potentially glutamatergic synapses (Chen et al., 2015b). These results suggest that exercise intervention may normalize DA denervation-induced structural and functional abnormalities at the corticostriatal and/or thalamostriatal synapses, providing a neurobiological basis for exercise intervention to normalize the abnormalities at the corticostriatal synapses, although thalamostriatal synapses may also be normalized; our ongoing research is investigating the potential effects of exercise on the corticostriatal and thalamostriatal synapses in the direct and indirect pathway MSNs.
Additionally, our studies indicate that DA denervation decreased the expression of GluR2 in the striatum in 6-OHDA rat PD model, and our treadmill exercise intervention regimen described above normalized this decrease in GluR2 expression (Chen et al., 2015a), largely consistent with the results of Garcia et al. (2017). GluR2 subunit-lacking AMPA-type glutamate receptors can form Ca-permeable glutamatergic receptor ion channels, conduct inwardly rectifying EPSCs, and Ca may trigger multiple molecular and cellular mechanisms in the MSNs (Cull-Candy et al., 2006; Liu and Savtchouk, 2012; Lalanne et al., 2016; Whitehead et al., 2017). Thus, exercise-induced normalization of GluR2 expression can contribute to the ultrastructural normalization of MSNs.
Similar to our results in 6-OHDA rat model, two previous studies have reported that in a subacute MPTP PD mouse model, treadmill exercise, initiated 5 days after MPTP lesion, increased the expression of GluR2 subunit in the striatum; this exercise also reduced the size of corticostriatal EPSCs (glutamatergic input), particularly in D2-MSNs; and these exercise-induced cellular changes were accompanied by behavioral improvements (VanLeeuwen et al., 2010; Kintz et al., 2013).
In aggregate, these findings suggest that exercise may modify and normalize the corticostriatal excitatory synaptic transmission and reduce potential glutamatergic excitatoxicity in the striatum, therefore contributing to the normalization of MSN structure and function such as the dendritic spines and synaptic inputs and processing at dendritic spines, eventually leading to circuittry and behavioral restoration. Functionally, the restored/increased dendrite spines can more effectively receive and process synaptic inputs from the cortical and thalamic motor command centers, contributing to the recovery/normalization of motor functions in these PD animals.
How exercise restores and repairs dendritic spines under normal and PD conditions is not established and will be discussed in section Molecular Mechanisms Underlying Exercise-Induced Neuroprotection of the Da Neurons and Corticostriatal Circuit.
Exercise Effects on MSN Physiology in PD
MSN activity and physiology are critical to motor and other important brain functions (see section Motor Function of the Striatum and the Nigrostriatal DA System). Thus, exercise-induced motoric benefits in PD patients and animal PD models may derive at least partially from exercise's potential effects on MSN activity. There is currently no human study on this topic. To our knowledge, there is only one published study investigating the potential effects of exercise on MSN spiking activity in unilateral MFB 6-OHDA PD rats, performed in our lab (Shi et al., 2017). In our study, the 4-week exercise intervention program was initiated 24 h after the 6-OHDA lesion surgery, treadmill speed was 11 m/min, the rat was exercised 30 min/day, 5 days/week. Under these conditions, we found that in 6-OHDA PD group, MSNs had an increased average firing rate (about 3 Hz) compared with normal rats (about 0.5 Hz). This is generally consistent with the literature on DA depletion on MSN firing (Kish et al., 1999; Chen et al., 2001; Singh et al., 2016). Further, in the 6-OHDA+ exercise group, MSN firing was partially normalized (about 2 Hz), accompanied by motor function improvement. Together, these results suggest that exercise may decrease striatal neuron excitability and partially normalize the abnormal neuronal spike firing in parkinsonian striatum, potentially contributing to exercise's motor-improving effects in PD. Although how this partial normalization is achieved remains to be determined, multiple mechanisms may be involved, such as attenuated DA loss and consequent reduction in striatal neuron intrinsic excitability and modification of intrastriatal circuitry (Wei et al., 2017); exercise may also affect the cortical glutamatergic inputs, thus reducing the abnormality in striatal neuron firing (VanLeeuwen et al., 2010; Kintz et al., 2013).
Molecular Mechanisms Underlying Exercise-Induced Neuroprotection of the DA Neurons and Corticostriatal Circuit
In the preceding sections, we summarized and discussed the reported physical exercise-induced attenuation of the loss of nigral DA neurons and the loss of striatal DA axons and DA tissue level in the striatum in 6-OHDA and MPTP animal PD models. Now we discuss the possible underlying molecular mechanisms. The attenuation of DA denervation may be realized via two different though related mechanisms: neuroprotection, neurorestoration or both. In the neuroprotection scenario, the neurons are protected from toxin insults before the injury is done. In the neurorestoration scenario, the neurons are first injured and then repaired. These two mechanisms probably work together in animals, for example, IP injection of MPTP in mice often injures DA neurons and DA axon terminals in the striatum; these sick DA neurons and axons often recover after the cessation of MPTP treatment, especially in young mice.
The molecular mechanisms underlying the exercise-induced protection and restoration of the nigrostriatal neurons and corticostriatal circuit are not established, but evidence indicates that neurotrophic factors are potentially critical mediators of these beneficial effects (da Silva et al., 2016); other molecules and mechanisms also contribute, such as reducing oxidative stress, increasing energy production and mitochondrial function, and increased blood flow via vasodilatation and angionesis (Petzinger et al., 2013; Nishijima et al., 2016). Studies have reported that exercise improved mitochondrial function, reduced α-synuclein expression and reduced the production of pro-inflammatory factors in MPTP PD model, leading to attenuated DA denervation and motor function loss (Sung et al., 2012; Subramaniam and Chesselet, 2013; Goes et al., 2014; Kelly et al., 2014; Spielman et al., 2016; Jang et al., 2017; Koo et al., 2017a,b). Here, we focus on neurotrophic factors (NTFs) because exercise increases NTFs in normal animals and humans and NTFs are neuroprotective and neurorestorative (Cotman et al., 2007; Voss et al., 2013; Arnold and Salvatore, 2016).
Neurotrophic Factors (NTFs) in the Nigrostriatal DA System
Stimulation of nerve cell growth or neurotrophic effect exerted by biological agents (now referred to as neurotrophic factors) was first observed in cultured peripheral nerve cells in the early 1950s (Levi-Montalcini and Hamburger, 1951; Levi-Montalcini and Cohen, 1956). It is now established that NTFs are secreted proteins that are critical to the growth, development, maturation, maintenance, plasticity, and repair and regrowth of the peripheral and central nervous system neurons (Barde et al., 1982; Leibrock et al., 1989; Hou et al., 1996; Levi-Montalcini et al., 1996; Airaksinen and Saarma, 2002; Bespalov and Saarma, 2007; Park and Poo, 2013; Kramer and Liss, 2015; Ibáñez and Andressoo, 2017; Sasi et al., 2017). Currently, more than 20 NTFs have been identified, and these NTFs are divided into four groups according to their molecular structure and signaling mechanisms: the neurotrophine group including the extensively studied NGF and BDNF, the GDNF family ligands with GDNF being the extensively studied member, the newly found NTF group including cerebral dopamine neurotrophic factor (CDNF) and mesencephalic astrocyte-derived neurotrophic factor (MANF), and the neurokine family (Lindholm et al., 2007; Aron and Klein, 2011; Allen et al., 2013; Ibáñez and Andressoo, 2017; Lindahl et al., 2017).
NTFs and DA Neurons
In 1993, a glia cell-secreted nerve growth-stimulating factor (termed glial cell line-derived neurotrophic factor or GDNF) was purified, sequenced, and cloned (Lin et al., 1993). Further, that study reported that GDNF strongly promotes the survival of rat midbrain DA neurons in tissue culture, raising the hope that GDNF may affect the survival and degeneration of DA neurons in health and PD in humans, and hence GDNF can be used to stop DA neuron degeneration in PD. Thus, a large number of in vitro and in vivo studies were quickly performed, as reflected in the publication of four original research papers on GDNF saving DA neurons and motor neurons in the January 26th, 1995, issue of Nature (DA neurons: Beck et al., 1995; Tomac et al., 1995; motor neurons: Oppenheim et al., 1995; Yan et al., 1995). Specifically, it was reported that GDNF injected into the nigral area or striatum reduced the MPTP- or axotomy-induced DA cell loss in the SNc and DA denervation in the striatum (Beck et al., 1995; Tomac et al., 1995). Further, upon inhibition of BDNF and GDNF expression, the DA axon sprouting in the knife-lesioned striatum was reduced in mice (Batchelor et al., 2000). It has also been reported that conditional GDNF deletion performed in adult mice led to DA neuron loss in SNc and VTA and DA denervation in the striatum and a loss of motor function, indicating that the survival of DA neurons is strongly dependent on GDNF (Pascual et al., 2008); a more recent study has reported contradicting findings (Kopra et al., 2015), although Pascual and López-Barneo (2015) argued that negative results are due to technical problems such as insufficient decrease of GDNF in the new study. Additional follow-up studies investigated and established the neuroprotective effects of GDNF protein or GDNF gene vectors on DA neurons in rodent and monkey PD models (Gash et al., 1995, 1996; Choi-Lundberg et al., 1997; Kordower et al., 2000; Nakajima et al., 2001; Grondin et al., 2002; Maswood et al., 2002; Ai et al., 2003; Kirik et al., 2004; Rangasamy et al., 2010). Taken together, these studies provide strong data supporting GDNF's neurotrophic and neuroprotective effects on DA neurons.
Studies indicate that BDNF also exerts trophic effects on the development, maturation, repair and plasticityof DA neurons (Hyman et al., 1991, 1994; Levivier et al., 1995; Spenger et al., 1995; Ostergaard et al., 1996; Benisty et al., 1998; Numan and Seroogy, 1999; Baker et al., 2005; Baquet et al., 2005; Sun et al., 2005; Baydyuk et al., 2011a,b). Genetic inhibition of BDNF expression led to loss of nigral DA neurons in rats (Porritt et al., 2005). However, evidence indicates that BDNF's trophic effect on nigral DA neurons is 5–10 weaker than that of GDNF (Lu and Hagg, 1997; Sun et al., 2005). BDNF expression in the brain is very low compared with that of NGF (Hofer et al., 1990). Thus, translational research on NTFs' neuroprotective and neurorestorative effects on DA neurons in PD monkey models and clinical trials have been focused on GDNF (Gash et al., 1995, 1996; Kordower et al., 2000; Kozlowski et al., 2000; Connor et al., 2001; Grondin et al., 2002; Maswood et al., 2002; Ai et al., 2003; Gill et al., 2003; Cohen et al., 2011; Kordower and Bjorklund, 2013; Olanow et al., 2015; Bartus and Johnson, 2017a,b; Kirik et al., 2017).
NTFs and Striatal MSNs
A large number of studies have established that NTF signaling regulates somatic, dendritic and axonal local protein synthesis and gene transcripts and hence support the development, maturation, maintenance, reorganization and regeneration/repair of neuronal somata, axons, dendrites, and dendritic spines and synapses in the striatum and other brain areas (Xu et al., 2000; Horch and Katz, 2002; Gorski et al., 2003; Baquet et al., 2004; Vigers et al., 2012; Lu et al., 2013; Orefice et al., 2013, 2016; Bramham and Panja, 2014; Leal et al., 2014; Zagrebelsky and Korte, 2014). In the striatum, BDNF has been shown to be critical to the survival and maintenance of MSNs (Baydyuk and Xu, 2014).
Evidence from cortical ablation experiments and molecular genetic manipulation experiments and the fact that the cerebral cortex expresses a high level of BDNF mRNA whereas the striatum expresses a very low level of BDNF mRNA, indicating that the majority of striatal BDNF may be produced in cortical neurons and a small portion of striatal BDNF may be produced in substantia nigral neurons, and BDNF is anterogradely transported to the striatum by the corticostriatal axons (hence corticostriatal synapses) (Hofer et al., 1990; Altar et al., 1997; Conner et al., 1997; Baquet et al., 2004; Zuccato and Cattaneo, 2007; Li Y. et al., 2012). Further, conditional tissue-specific genetic deletion of bdnf gene in the cerebral cortex and the substantia nigra completely depleted BDNF protein in the striatum, confirming that striatal BDNF protein is transported to the striatum from the cerebral cortex and substantia nigra (Li Y. et al., 2012); equally important, this BDNF depletion led to smaller MSN soma size, atrophy, and loss of dendrites and dendritic spines, a lower expression of DARPP-32 (a key mediator of DA signaling) and severe motor deficits (Baquet et al., 2004; Li Y. et al., 2012). Similarly, genetic inactivation of BDNF receptor TrkB selectively in the striatal MSNs led to smaller MSN size, dendritic spine loss and a greatly reduced DARPP-32 level and also a lower level of TH expression in DA axons (Li Y. et al., 2012). Global deletion of BDNF or TrkB led to neuronal (somata, dendrite, spine) atrophy in multiple brain areas and the striatal MSNs are particularly sensitive, leading to a substantial MSN dendrite and spine atrophy (Rauskolb et al., 2010; Li Y. et al., 2012).
Taken together, the NTF mechanisms discussed above provide opportunities for exercise to affect MSN dendritic spines and corticostriatal synapses and also nigral DA neurons and their axon terminals via exercise-stimulated NTF production.
Neurotrophic Factors in PD
NTF Deficiency in PD Brains
There are only a few limited studies on this important question. These studies indicate a deficiency in NTFs in PD brains. Quantitative histochemical studies have reported that BDNF gene expression and protein are reduced in the substantia nigra in postmortem PD brains (Mogi et al., 1999; Parain et al., 1999; Howells et al., 2000). The GDNF level may also be reduced in the PD brain (Chauhan et al., 2001), although the numbers of the brains included in these studies were relatively small. Future studies are needed that will include larger samples from different stages (to compare early and late stages; early stage maybe particularly informative) to replicate, solidify and expand these results.
NTF Neuroprotective Effects in Animal PD Models
Since NTFs are intrinsically beneficial to DA neurons and MSNs and NTFs may be deficient in PD brains, an obvious idea is that increasing the production of neurotrophic factors may be neuroprotective and neurorestorative for PD brains. Indeed, since the early 1990s, it has been the focus of a large basic and clinical research effort to directly deliver NTF proteins and more recently implanting genetically engineered NTF-producing cells or gene vectors to locally produce NTFs and protect DA neurons in rodent and non-human primate PD models and patients (Olson et al., 1991; Lin et al., 1993; Kordower et al., 2000; Kozlowski et al., 2000; Gill et al., 2003; Cohen et al., 2011; Nagahara and Tuszynski, 2011; Kordower and Bjorklund, 2013; Olanow et al., 2015; Li et al., 2016; Bartus and Johnson, 2017a,b; Björklund and Lindvall, 2017).
In addition to the classical neurotrophic factors, the newly discovered conserved cerebral dopamine neurotrophic factor (CDNF) and mesencephalic astrocyte-derived neurotrophic factor (MANF) have also been reported to have trophic effects on nigral DA neurons in normal animals and neuroprotective effects on these neurons in animal PD models (Lindholm et al., 2007; Garea-Rodríguez et al., 2016; Lindahl et al., 2017).
NTF Neuroprotective Effects in Clinical Trials in PD Patients
The positive neuroprotective and neurorestoration effects of NTFs in animal PD models prompted clinical trials of NTFs in PD patients in the past three decades. Open label NTF trials produced positive results (e.g., Olson et al., 1991; Gill et al., 2003; Slevin et al., 2005). However, more rigorous double blind clinical trials failed to prove any NTF benefit in PD (Kordower and Bjorklund, 2013; Olanow et al., 2015; Bartus and Johnson, 2017a; Hegarty et al., 2017). Two reasons may have contributed to this failure. First, the exogenous NTF (mostly GDNF)–protein or gene-is supplied by a point source and hence does not provide enough NTF to the target tissue (the striatum and SNc) (Aebischer and Ridet, 2001; Gash et al., 2005; Salvatore et al., 2006). Further, NTFs can not cross the blood brain barrier, diffuses poorly and are easily degraded (Aron and Klein, 2011). Thus, the failure of these clinical trials may be a delivery problem, not because NTFs are ineffective. Second, the procedure is an invasive intracranial surgery with considerable risks and not acceptable to early-mid stage PD patients who still have considerable residual DA neurons and their striatal projection axons that can be protected by NTFs; in late stage PD patients who may accept the invasive procedure, the nigrostriatal DA system is almost completely destroyed and nothing can be done.
Therefore, an early-start, low-risk neuroprotection program is needed to protect the vulnerable DA neurons and other neuron types, slow the rate of their degeneration and hence delay the appearance of PD symptoms. Exercise is non-invasive, and risk/side effect-free stimulator of NTF production with benefits to many organ-systems. Thus, exercise may be such an early-start neuroprotective and neurorestorative treatment for PD.
Exercise Stimulates NTF Production in PD
Besides supplying exogenous NTFs via an invasive surgery-infusion means, other methods that stimulate the production of endogenous NTFs can also be neuroprotective while avoiding the risks associated with intracranial infusion surgery. Research since the 1990's has indicated that physical activity or exercise can increase NTF production in normal animals and also in humans, although human studies are few and only serum NTFs were tested due to the difficulties in obtaining human tissue samples (Neeper et al., 1995, 1996; van Praag et al., 1999, 2005; Cotman and Berchtold, 2002; Cotman et al., 2007; Pereira et al., 2007; Voss et al., 2013; Coelho et al., 2014; Arnold and Salvatore, 2016; Marston et al., 2017).
Exercise Stimulates NTF Production in PD Patients
There is no direct data on the possibility of exercise stimulating NTF production in the nigrostriatal DA system because it is currently impossible to measure NTFs in brain tissues in PD patients. There is also currently no data from postmortem brain tissues because of the difficulties in obtaining appropriate human brain tissue samples. However, studies have investigated exercise's effects on NTFs in peripheral tissues, particularly in blood. It has been reported that intensive exercise increased blood BDNF levels and increased TrkB activity in blood cells, although data on brain tissues are lacking due to the obvious difficulties in obtaining brain tissue samples (Frazzitta et al., 2014; Angelucci et al., 2016; Fontanesi et al., 2016). Independently, it has been reported that the basal serum BDNF level was lower in PD patients than in normal control (Scalzo et al., 2010), and exercise increases the production of BDNF and other NTFs in PD patients (Zoladz et al., 2014; Marusiak et al., 2015). If we extrapolate these peripheral findings, then exercise may stimulate the endogenous production of NTFs in the brain that in turn exert neuroprotective and neurorestorative effects on DA neurons, modify/slow the disease progression. Certainly, future studies need to experimentally verify this extrapolation.
Experimental Data from PD Animal Models
To circumvent the difficulties in studying PD patients, researchers have used animal PD models to investigate how exercise affects NTF production in the brain that may in turn exert neuroprotective and neurorestorative effects on DA neurons and MSNs associated with behavioral benefits in PD animals (da Silva et al., 2016). For example, in a chronic, low-moderate dose MPTP mouse model with moderate nigral DA neuron loss and striatal DA loss (Lau et al., 2011), treadmill exercise before, during and after MPTP treatment partially prevented the loss of nigral DA neurons and striatal TH and DA, compared to similarly lesioned sedentary mice, accompanied by a prevention of motor function deficits. In this mouse model, exercise also increased the tissue level of BDNF in the nigral area and the GDNF level in both the nigral area and striatum; exercise also partially normalized the mitochondrial function as indicated by increased ATP level in the striatal tissue in exercised MPTP-lesioned mice than in sedentary MPTP-lesioned mice (Lau et al., 2011). Tajiri et al. (2010) reported that exercise substantially increased striatal tissue BDNF and GDNF (by ~100%) in normal rats, increased striatal tissue BDNF and GDNF level by 50% in PD rats compared with non-exercised PD rats, measured by western blot. In both mouse and rat intrastriatal 6-OHDA lesion PD models a pre-lesion, 60-day treadmill exercise increased and hence partially normalized the striatal tissue levels of proBDNF, BDNF and its receptor TrkB, increasing striatal tissue BDNF level by 33% in PD animals compared with non-exercised PD animals, measured by western blot, accompanied by parallel behavioral improvements (Tuon et al., 2012, 2014). This pre-lesion physical exercise also partially prevented or normalized the intrastriatal 6-OHDA-induced reduction in TH (i.e., DA axon loss) in the striatum in rats (Tuon et al., 2012). Further, exercise's apparent protective effect on DA neurons was reduced when BDNF receptors were blocked (Real et al., 2013). Additionally, it has been reported that a reduced BDNF expression in haploinsufficient BDNF+/− mice eliminated exercise-induced protection of the nigrostriatal DA neurons against MPTP neurotoxicity (Gerecke et al., 2012). Besides BDNF and GDNF, exercise may also trigger the production of other trophic factors that may also be involved in exercise-induced neuroprotection in PD animals (da Silva et al., 2016). Together, these studies indicate that exercise-induced protection of DA neurons is dependent on NTF production.
Besides protecting DA neurons from toxin and other insults before the damages are done or completed, another mechanism underlying the exercise's benefits in PD is for exercise to help residual DA axons in the striatum to repair and/or regenerate-i.e., neurorestoration, potentially via the same NTF mechanisms discussed above and other mechanisms not covered in this review such as increasing blood flow and mitochondrial function and decreasing oxidative stress and inflammatory factors. Use/exercise-induced facilitation of neurorestoration is well-documented in the neurorehabilitation literature (e.g., Jones and Schallert, 1994; Schallert et al., 1997, 2000; Takamatsu et al., 2010; Korol et al., 2013; Tamakoshi et al., 2014). Further, neuroprotection and neurorestoration are likely intertwined especially when examined 1–2 months after the toxin administration, both processes lead to attenuated DA axon loss in the striatum and/or attenuated DA neuron loss in the nigral areas. The repairing/regeneration/neurorestoration idea is particularly attractive because the striatal DA axons are known to have to capacity to regenerate in 6-OHDA and MPTP rodent and non-human primate PD models. For example, it has been reported that when the 6-OHDA lesion is ~65% DA denervation in the striatum, DA axons may sprout and regenerate in rodents and non-human primates, but no sprouting/regeneration was observed when the DA denervation is more complete ≥90% (Liberatore et al., 1999; Bezard et al., 2000; Elsworth et al., 2000; Finkelstein et al., 2000; Stanic et al., 2003a,b; Petzinger et al., 2006; Mounayar et al., 2007; Lee et al., 2008). Song and Haber (2000) also reported that residual DA axons sprout in the striatum in MPTP-lesioned monkeys. These suggest that DA neuron neuroprotection and regeneration are possible before the DA neurons are completely dead/destroyed. Thus, exercise, via NTFs and other molecules, may facilitate the innate capacity of DA neurons and axons to sprout, repair and regenerate, depending on DA lesion protocol, intensity, exercise protocol/timing. When the DA lesion is too severe, it may be impossible to obtain exercise-induced neuroprotection of DA neurons and DA axons in the striatum; when the DA lesion is moderate, exercise-induced DA neuron protection is less difficult to realize.
Conclusions and Future Directions
In conclusion, epidemiological data indicate that exercise may reduce the risk of developing PD and slow PD progression (Figure 5). Clinical evidence indicates that exercise may be a convenient, non-invasive, side effect-free, cost-free treatment that is beneficial to PD patient's motor and cognitive functions. Thus, exercise should be prescribed to PD patients. Experimental data indicate, as illustrated in Figure 5, that exercise may protect and restore the nigrostriatal DA system and the corticostriatal synapse, hence restoring motor and other behavioral functions, potentially by triggering the production of trophic factors and therefore protecting and restoring DA neurons, particularly their massive and distant and hence vulnerable axonal arborization in the striatum. Exercise-stimulated trophic factors may also protect and restore the MSN-based BG-cortical circuits, improving motor, and cognitive functions.
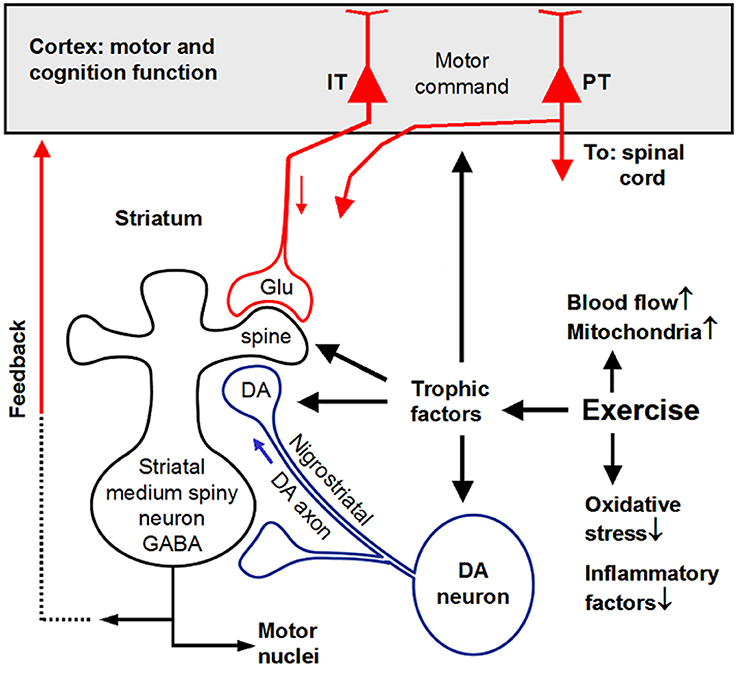
Figure 5. Summary diagram showing that exercise triggers trophic factor production that in turn induce neuroprotection and neurorestoration. IT, intratelencephalically projecting cortical neurons; PT, pyramidal tract projecting cortical neurons. Original artwork of Fu-Ming Zhou.
To advance the field of exercise-induced neuroprotection of DA neurons in PD, an important task for basic science research is to establish reliable and standardized protocols to produce exercise-induced protection of DA neurons in rodent and non-human primate PD models, thus resolving the major discrepancy that while a large number of studies saw exercise-induced neuroprotection of DA neurons in animal PD models, several studies did not. We also need to determine the anatomical changes of the DA axon terminals and somata during exercise-induced neuroprotection, elucidating the anatomical substrate for the functional recovery.
For translational and clinical research, we need to determine, in humans, which form of exercise is most effective in reducing PD risk, when exercise should start that will produce protection against PD and DA loss. In parallel experimental studies in animals, we need to determine when exercise should start to produce the maximal DA neuron protection in animal PD models. The dose (exercise intensity)-response (behavioral, anatomical, and neurochemical benefits) relation also needs to be established in both animals and humans. These mundane and incremental studies will build a solid foundation for this clinically important field to move forward.
Author Contributions
LH, WC, XL and DQ: drafting and editing. F-MZ: conceptualization, drafting, and editing.
Funding
The authors' work was supported by National Natural Science Foundation of China grants 31571221 and 31401018 and National Institutes of Health grant R01NS097671.
Conflict of Interest Statement
The authors declare that the research was conducted in the absence of any commercial or financial relationships that could be construed as a potential conflict of interest.
References
Aebischer, P., and Ridet, J. (2001). Recombinant proteins for neurodegenerative diseases: the delivery issue. Trends Neurosci. 24, 533–540. doi: 10.1016/S0166-2236(00)01899-3
Aguiar, A. S. Jr., Duzzioni, M., Remor, A. P., Tristão, F. S., Matheus, F. C., Raisman-Vozari, R., et al. (2016a). Moderate-intensity physical exercise protects against experimental 6-hydroxydopamine-induced hemiparkinsonism through Nrf2-antioxidant response element pathway. Neurochem. Res. 41, 64–72. doi: 10.1007/s11064-015-1709-8
Aguiar, A. S. Jr., Lopes, S. C., Tristão, F. S., Rial, D., de Oliveira, G., da Cunha, C., et al. (2016b). Exercise improves cognitive impairment and dopamine metabolism in MPTP-treated mice. Neurotox. Res. 29, 118–125. doi: 10.1007/s12640-015-9566-4
Aguiar, A. S. Jr., Tristão, F. S., Amar, M., Chevarin, C., Glaser, V., de Paula Martins, R., et al. (2014). Six weeks of voluntary exercise don't protect C57BL/6 mice against neurotoxicity of MPTP and MPP(+). Neurotox. Res. 25, 147–152. doi: 10.1007/s12640-013-9412-5
Ahlskog, J. E. (2011). Does vigorous exercise have a neuroprotective effect in Parkinson disease? Neurology 77, 288–294. doi: 10.1212/WNL.0b013e318225ab66
Ai, Y., Markesbery, W., Zhang, Z., Grondin, R., Elseberry, D., Gerhardt, G. A., et al. (2003). Intraputamenal infusion of GDNF in aged rhesus monkeys: distribution and dopaminergic effects. J. Comp. Neurol. 461, 250–61. doi: 10.1002/cne.10689
Airaksinen, M. S., and Saarma, M. (2002). The GDNF family: signalling, biological functions and therapeutic value. Nat. Rev. Neurosci. 3, 383–394. doi: 10.1038/nrn812
Alberts, J. L., Phillips, M., Lowe, M. J., Frankemolle, A., Thota, A., Beall, E. B., et al. (2016). Cortical and motor responses to acute forced exercise in Parkinson's disease. Parkinsonism Relat Disord. 24, 56–62. doi: 10.1016/j.parkreldis.2016.01.015
Allen, S. J., Watson, J. J., Shoemark, D. K., Barua, N. U., and Patel, N. K. (2013). GDNF, NGF and BDNF as therapeutic options for neurodegeneration. Pharmacol. Ther. 138, 155–175. doi: 10.1016/j.pharmthera.2013.01.004
Altar, C. A., Cai, N., Bliven, T., Juhasz, M., Conner, J. M., Acheson, A. L., et al. (1997). Anterograde transport of brain-derived neurotrophic factor and its role in the brain. Nature 389, 856–860. doi: 10.1038/39885
Amano, S., Nocera, J. R., Vallabhajosula, S., Juncos, J. L., Gregor, R. J., Waddell, D. E., et al. (2013). The effect of Tai Chi exercise on gait initiation and gait performance in persons with Parkinson's disease. Parkinsonism Relat. Disord. 19, 955–960. doi: 10.1016/j.parkreldis.2013.06.007
André, V. M., Cepeda, C., Cummings, D. M., Jocoy, E. L., Fisher, Y. E., William Yang, X., et al. (2010). Dopamine modulation of excitatory currents in the striatum is dictated by the expression of D1 or D2 receptors and modified by endocannabinoids. Eur. J. Neurosci. 31, 14–28. doi: 10.1111/j.1460-9568.2009.07047.x
Angelucci, F., Piermaria, J., Gelfo, F., Shofany, J., Tramontano, M., Fiore, M., et al. (2016). The effects of motor rehabilitation training on clinical symptoms and serum BDNF levels in Parkinson's disease subjects. Can. J. Physiol. Pharmacol. 94, 455–461. doi: 10.1139/cjpp-2015-0322
Anglade, P., Mouatt-Prigent, A., Agid, Y., and Hirsch, E. (1996). Synaptic plasticity in the caudate nucleus of patients with Parkinson's disease. Neurodegeneration 5, 121–18. doi: 10.1006/neur.1996.0018
Arnold, J. C., and Salvatore, M. F. (2016). Exercise-mediated increase in nigral tyrosine hydroxylase is accompanied by increased nigral GFR-α1 and EAAC1 expression in aging rats. ACS Chem. Neurosci. 7, 227–239. doi: 10.1021/acschemneuro.5b00282
Aron, L., and Klein, R. (2011). Repairing the parkinsonian brain with neurotrophic factors. Trends Neurosci. 34, 88–100. doi: 10.1016/j.tins.2010.11.001
Bagga, V., Dunnett, S. B., and Fricker, R. A. (2015). The 6-OHDA mouse model of Parkinson's disease - Terminal striatal lesions provide a superior measure of neuronal loss and replacement than median forebrain bundle lesions. Behav. Brain Res. 288, 107–117. doi: 10.1016/j.bbr.2015.03.058
Baker, S. A., Stanford, L. E., Brown, R. E., and Hagg, T. (2005). Maturation but not survival of dopaminergic nigrostriatal neurons is affected in developing and aging BDNF-deficient mice. Brain Res. 1039, 177–188. doi: 10.1016/j.brainres.2005.01.052
Ballard, P. A., Tetrud, J. W., and Langston, J. W. (1985). Permanent human parkinsonism due to 1-methyl-4-phenyl- 1,2,3,6-tetrahydropyridine (MPTP): seven cases. Neurology 35, 949–956. doi: 10.1212/WNL.35.7.949
Baquet, Z. C., Bickford, P. C., and Jones, K. R. (2005). Brain-derived neurotrophic factor is required for the establishment of the proper number of dopaminergic neurons in the substantia nigra pars compacta. J. Neurosci. 25, 6251–6259. doi: 10.1523/JNEUROSCI.4601-04.2005
Baquet, Z. C., Gorski, J. A., and Jones, K. R. (2004). Early striatal dendrite deficits followed by neuron loss with advanced age in the absence of anterograde cortical brain-derived neurotrophic factor. J. Neurosci. 24, 4250–4258. doi: 10.1523/JNEUROSCI.3920-03.2004
Barde, Y. A., Edgar, D., and Thoenen, H. (1982). Purification of a new neurotrophic factor from mammalian brain. EMBO J. 1, 549–553.
Bartus, R. T., and Johnson, E. M. Jr. (2017a). Clinical tests of neurotrophic factors for human neurodegenerative diseases, part 1: Where have we been and what have we learned? Neurobiol. Dis. 97, 156–168. doi: 10.1016/j.nbd.2016.03.027
Bartus, R. T., and Johnson, E. M. Jr. (2017b). Clinical tests of neurotrophic factors for human neurodegenerative diseases, part 2: Where do we stand and where must we go next? Neurobiol. Dis. 97, 169–178. doi: 10.1016/j.nbd.2016.03.026
Batchelor, P. E., Liberatore, G. T., Porritt, M. J., Donnan, G. A., and Howells, D. W. (2000). Inhibition of brain-derived neurotrophic factor and glial cell line-derived neurotrophic factor expression reduces dopaminergic sprouting in the injured striatum. Eur. J. Neurosci. 12, 3462–3468. doi: 10.1046/j.1460-9568.2000.00239.x
Bateup, H. S., Santini, E., Shen, W., Birnbaum, S., Valjent, E., Surmeier, D. J., et al. (2010). Distinct subclasses of medium spiny neurons differentially regulate striatal motor behaviors. Proc. Natl. Acad. Sci. U.S.A. 107, 14845–14850. doi: 10.1073/pnas.1009874107
Baydyuk, M., and Xu, B. (2014). BDNF signaling and survival of striatal neurons. Front. Cell. Neurosci. 8:254. doi: 10.3389/fncel.2014.00254
Baydyuk, M., Nguyen, M. T., and Xu, B. (2011a). Chronic deprivation of TrkB signaling leads to selective late-onset nigrostriatal dopaminergic degeneration. Exp. Neurol. 228, 118–125. doi: 10.1016/j.expneurol.2010.12.018
Baydyuk, M., Russell, T., Liao, G. Y., Zang, K., An, J. J., Reichardt, L. F., et al. (2011b). TrkB receptor controls striatal formation by regulating the number of newborn striatal neurons. Proc. Natl. Acad. Sci. U.S.A. 108, 1669–1674. doi: 10.1073/pnas.1004744108
Beall, E. B., Lowe, M. J., Alberts, J. L., Frankemolle, A. M., Thota, A. K., Shah, C., et al. (2013). The effect of forced-exercise therapy for Parkinson's disease on motor cortex functional connectivity. Brain Connect. 3, 190–198. doi: 10.1089/brain.2012.0104
Beck, K. D., Valverde, J., Alexi, T., Poulsen, K., Moffat, B., Vandlen, R. A., et al. (1995). Mesencephalic dopaminergic neurons protected by GDNF from axotomy-induced degeneration in the adult brain. Nature 373, 339–341. doi: 10.1038/373339a0
Benisty, S., Boissiere, F., Faucheux, B., Agid, Y., and Hirsch, E. C. (1998). trkB messenger RNA expression in normal human brain and in the substantia nigra of parkinsonian patients: an in situ hybridization study. Neuroscience 86, 813–826. doi: 10.1016/S0306-4522(98)00126-2
Bespalov, M. M., and Saarma, M. (2007). GDNF family receptor complexes are emerging drug targets. Trends Pharmacol. Sci. 28, 68–74. doi: 10.1016/j.tips.2006.12.005
Bezard, E., Dovero, S., Imbert, C., Boraud, T., and Gross, C. E. (2000). Spontaneous long-term compensatory dopaminergic sprouting in MPTP-treated mice. Synapse 38, 363–368. doi: 10.1002/1098-2396(20001201)38:3<363::AID-SYN16>3.0.CO;2-A
Bishop, G. A., Chang, H. T., and Kitai, S. T. (1981). Morphological and physiological properties of neostriatal neurons: an intracellular horseradish peroxidase study in the rat. Neuroscience 7, 179–191. doi: 10.1016/0306-4522(82)90159-2
Björklund, A., and Lindvall, O. (2017). Replacing dopamine neurons in parkinson's disease: how did it happen? J. Parkinsons Dis. 7, S23–S33. doi: 10.3233/JPD-179002
Bloem, B. R., de Vries, N. M., and Ebersbach, G. (2015). Nonpharmacological treatments for patients with Parkinson's disease. Mov. Disord. 30, 1504–1520. doi: 10.1002/mds.26363
Braak, H., Ghebremedhin, E., Rüb, U., Bratzke, H., and Del Tredici, K. (2004). Stages in the development of Parkinson's disease-related pathology. Cell Tissue Res. 318, 121–134. doi: 10.1007/s00441-004-0956-9
Bramham, C. R., and Panja, D. (2014). BDNF regulation of synaptic structure, function, and plasticity. Neuropharmacology 76(Pt C), 601–602. doi: 10.1016/j.neuropharm.2013.08.012
Burke, R. E., and O'Malley, K. (2013). Axon degeneration in Parkinson's disease. Exp. Neurol. 246, 72–83. doi: 10.1016/j.expneurol.2012.01.011
Burley, C. V., Bailey, D. M., Marley, C. J., and Lucas, S. J. E. (2016). Brain train to combat brain drain; focus on exercise strategies that optimise neuroprotection. Exp. Physiol. 101, 1178–1184. doi: 10.1113/EP085672
Chang, H. T., Wilson, C. J., and Kitai, S. T. (1981). Single neostriatal efferent axons in the globus pallidus: a light and electron microscopic study. Science 213, 915–918. doi: 10.1126/science.7256286
Chauhan, N. B., Siegel, G. J., and Lee, J. M. (2001). Depletion of glial cell line-derived neurotrophic factor in substantia nigra neurons of Parkinson's disease brain. J. Chem. Neuroanat. 21, 277–288. doi: 10.1016/S0891-0618(01)00115-6
Chen, W., Shi, K. X., and Liu, X. L. (2015a). Exercise intervention improves behavioral function in PD rats through modulation of striatal MSNs. Chin. J. Sports Med. 34, 228–234.
Chen, H., Zhang, S. M., Schwarzschild, M. A., Hernán, M. A., and Ascherio, A. (2005). Physical activity and the risk of Parkinson disease. Neurology 64,664–669. doi: 10.1212/01.WNL.0000151960.28687.93
Chen, M. T., Morales, M., Woodward, D. J., Hoffer, B. J., and Janak, P. H. (2001). In vivo extracellular recording of striatal neurons in the awake rat following unilateral 6-hydroxydopamine lesions. Exp. Neurol. 171, 72–83. doi: 10.1006/exnr.2001.7730
Chen, W., Wei, X., Liu, X. L., and Yan, K. L. (2015b). Effect of exercise on cortex-striatum glutamatergic neurotransmission in PD Model rats. J. Beijing Sport Univ. 38, 61–66.
Cheng, H. C., Ulane, C. M., and Burke, R. E. (2010). Clinical progression in Parkinson disease and the neurobiology of axons. Ann. Neurol. 67, 715–725. doi: 10.1002/ana.21995
Chiken, S., Sato, A., Ohta, C., Kurokawa, M., Arai, S., Maeshima, J., et al. (2015). Dopamine D1 receptor-mediated transmission maintains information flow through the cortico-striato-entopeduncular direct pathway to release movements. Cereb. Cortex 25, 4885–4897. doi: 10.1093/cercor/bhv209
Choi-Lundberg, D. L., Lin, Q., Chang, Y. N., Chiang, Y. L., Hay, C. M., Mohajeri, H., et al. (1997). Dopaminergic neurons protected from degeneration by GDNF gene therapy. Science 275, 838–841. doi: 10.1126/science.275.5301.838
Churchill, M. J., Pflibsen, L., Sconce, M. D., Moore, C., Kim, K., and Meshul, C. K. (2017). Exercise in an animal model of Parkinson's disease: motor recovery but not restoration of the nigrostriatal pathway. Neuroscience 359, 224–247. doi: 10.1016/j.neuroscience.2017.07.031
Ciliax, B. J., Drash, G. W., Staley, J. K., Haber, S., Mobley, C. J., Miller, G. W., et al. (1999). Immunocytochemical localization of the dopamine transporter in human brain. J. Comp. Neurol. 409, 38–56. doi: 10.1002/(SICI)1096-9861(19990621)409:1<38::AID-CNE4>3.0.CO;2-1
Coelho, F. G., Vital, T. M., Stein, A. M., Arantes, F. J., Rueda, A. V., Camarini, R., et al. (2014). Acute aerobic exercise increases brain-derived neurotrophic factor levels in elderly with Alzheimer's disease. J. Alzheimers Dis. 39, 401–408. doi: 10.3233/JAD-131073
Coelho, M., and Ferreira, J. J. (2012). Late-stage Parkinson disease. Nat. Rev. Neurol. 8, 435–442. doi: 10.1038/nrneurol.2012.126
Cohen, A. D., Tillerson, J. L., Smith, A. D., Schallert, T., and Zigmond, M. J. (2003). Neuroprotective effects of prior limb use in -hydroxydopamine-treated rats: possible role of GDNF. J. Neurochem. 85, 299–305. doi: 10.1046/j.1471-4159.2003.01657.x
Cohen, A. D., Zigmond, M. J., and Smith, A. D. (2011). Effects of intrastriatal GDNF on the response of dopamine neurons to 6-hydroxydopamine: time course of protection and neurorestoration. Brain Res. 1370, 80–88. doi: 10.1016/j.brainres.2010.11.006
Conner, J. M., Lauterborn, J. C., Yan, Q., Gall, C. M., and Varon, S. (1997). Distribution of brain-derived neurotrophic factor (BDNF) protein and mRNA in the normal adult rat CNS: evidence for anterograde axonal transport. J. Neurosci. 17, 2295–2313.
Connor, B., Kozlowski, D. A., Unnerstall, J. R., Elsworth, J. D., Tillerson, J. L., Schallert, T., et al. (2001). Glial cell line-derived neurotrophic factor (GDNF) gene delivery protects dopaminergic terminals from degeneration. Exp. Neurol. 169, 83–95. doi: 10.1006/exnr.2001.7638
Corcos, D. M., Robichaud, J. A., David, F. J., Leurgans, S. E., Vaillancourt, D. E., Poon, C., et al. (2013). A two-year randomized controlled trial of progressive resistance exercise for Parkinson's disease. Mov. Disord. 28, 1230–1240. doi: 10.1002/mds.25380
Cotman, C. W., and Berchtold, N. C. (2002). Exercise: a behavioral intervention to enhance brain health and plasticity. Trends Neurosci. 25, 295–301. doi: 10.1016/S0166-2236(02)02143-4
Cotman, C. W., Berchtold, N. C., and Christie, L. A. (2007). Exercise builds brain health: key roles of growth factor cascades and inflammation. Trends Neurosci. 30, 464–472. doi: 10.1016/j.tins.2007.06.011
Cui, G., Jun, S. B., Jin, X., Pham, M. D., Vogel, S. S., Lovinger, D. M., et al. (2013). Concurrent activation of striatal direct and indirect pathways during action initiation. Nature 494, 238–242. doi: 10.1038/nature11846
Cull-Candy, S., Kelly, L., and Farrant, M. (2006). Regulation of Ca2+-permeable AMPA receptors: synaptic plasticity and beyond. Curr. Opin. Neurobiol. 16, 288–297. doi: 10.1016/j.conb.2006.05.012
da Silva, P. G., Domingues, D. D., de Carvalho, L. A., Allodi, S., and Correa, C. L. (2016). Neurotrophic factors in Parkinson's disease are regulated by exercise: evidence-based practice. J. Neurol. Sci. 363, 5–15. doi: 10.1016/j.jns.2016.02.017
Day, M., Wang, Z., Ding, J., An, X., Ingham, C. A., Shering, A. F., et al. (2006). Selective elimination of glutamatergic synapses on striatopallidal neurons in Parkinson disease models. Nat. Neurosci. 9, 251–259. doi: 10.1038/nn1632
de Lau, L. M., and Breteler, M. M. (2006). Epidemiology of Parkinson's disease. Lancet Neurol. 5, 525–535. doi: 10.1016/S1474-4422(06)70471-9
Del Tredici, K., and Braak, H. (2016). Review: sporadic Parkinson's disease: development and distribution of α-synuclein pathology. Neuropathol. Appl. Neurobiol. 42, 33–50. doi: 10.1111/nan.12298
Deng, Y., Lanciego, J., Goff, L. K., Coulon, P., Salin, P., Kachidian, P., et al. (2015). Differential organization of cortical inputs to striatal projection neurons of the matrix compartment in rats. Front. Syst. Neurosci. 9:51. doi: 10.3389/fnsys.2015.00051
Ding, S., Li, L., and Zhou, F. M. (2015). Nigral dopamine loss induces a global upregulation of presynaptic dopamine D1 receptor facilitation of the striatonigral GABAergic output. J. Neurophysiol. 113, 1697–1711. doi: 10.1152/jn.00752.2014
Dipasquale, S., Meroni, R., Sasanelli, F., Messineo, I., Piscitelli, D., Perin, C., et al. (2017). Physical therapy versus a general exercise programme in patients with hoehn yahr stage II parkinson's disease: a randomized controlled trial. J. Parkinsons Dis. 7, 203–210. doi: 10.3233/JPD-161015
Doig, N. M., Moss, J., and Bolam, J. P. (2010). Cortical and thalamic innervation of direct and indirect pathway medium-sized spiny neurons in mouse striatum. J. Neurosci. 30, 14610–14618. doi: 10.1523/JNEUROSCI.1623-10.2010
Duchesne, C., Lungu, O., Nadeau, A., Robillard, M. E., Boré, A., Bobeuf, F., et al. (2015). Enhancing both motor and cognitive functioning in Parkinson's disease: aerobic exercise as a rehabilitative intervention. Brain Cogn. 99, 68–77. doi: 10.1016/j.bandc.2015.07.005
Durieux, P. F., Bearzatto, B., Guiducci, S., Buch, T., Waisman, A., Zoli, M., et al. (2009). D2R striatopallidal neurons inhibit both locomotor and drug reward processes. Nat. Neurosci. 12, 393–395. doi: 10.1038/nn.2286
Durieux, P. F., Schiffmann, S. N., and de Kerchove d'Exaerde, A. (2012). Differential regulation of motor control and response to dopaminergic drugs by D1R and D2R neurons in distinct dorsal striatum subregions. EMBO J. 31, 640–653. doi: 10.1038/emboj.2011.400
Elsworth, J. D., Taylor, J. R., Sladek, J. R. Jr., Collier, T. J., Redmond, D. E. Jr., and Roth, R. H. (2000). Striatal dopaminergic correlates of stable parkinsonism and degree of recovery in old-world primates one year after MPTP treatment. Neuroscience 95, 399–408. doi: 10.1016/S0306-4522(99)00437-6
Finkelstein, D. I., Stanic, D., Parish, C. L., Tomas, D., Dickson, K., and Horne, M. K. (2000). Axonal sprouting following lesions of the rat substantia nigra. Neuroscience 97, 99–112. doi: 10.1016/S0306-4522(00)00009-9
Finnegan, K. T., Irwin, I., Delanney, L. E., and Langston, J. W. (1995). Age-dependent effects of the 2′-methyl analog of 1-methyl-4-phenyl-1,2,3,6- tetrahydropyridine: prevention by inhibitors of monoamine oxidase B. J. Pharmacol. Exp. Ther. 273, 716–720.
Fontanesi, C., Kvint, S., Frazzitta, G., Bera, R., Ferrazzoli, D., Di Rocco, A., et al. (2016). Intensive rehabilitation enhances lymphocyte BDNF-TrkB signaling in patients with parkinson's disease. Neurorehabil. Neural Repair 30, 411–418. doi: 10.1177/1545968315600272
Fox, C. M., Gash, D. M., Smoot, M. K., and Cass, W. A. (2001). Neuroprotective effects of GDNF against 6-OHDA in young and aged rats. Brain Res. 896, 56–63. doi: 10.1016/S0006-8993(00)03270-4
Fox, S. H., Katzenschlager, R., Lim, S. Y., Ravina, B., Seppi, K., Coelho, M., et al. (2011). The movement disorder society evidence-based medicine review update: treatments for the motor symptoms of parkinson's disease. Mov. Disord. 26(Suppl. 3), S2–S41. doi: 10.1002/mds.23829
Franco, V., and Turner, R. S. (2012). Testing the contributions of striatal dopamine loss to the genesis of parkinsonian signs. Neurobiol. Dis. 47, 114–125. doi: 10.1016/j.nbd.2012.03.028
Frazzitta, G., Maestri, R., Ghilardi, M. F., Riboldazzi, G., Perini, M., Bertotti, G., et al. (2014). Intensive rehabilitation increases BDNF serum levels in parkinsonian patients: a randomized study. Neurorehabil. Neural Repair 28, 163–168. doi: 10.1177/1545968313508474
Friend, D. M., and Kravitz, A. V. (2014). Working together: basal ganglia pathways in action selection. Trends Neurosci. 37, 301–303. doi: 10.1016/j.tins.2014.04.004
Fujiyama, F., Sohn, J., Nakano, T., Furuta, T., Nakamura, K. C., Matsuda, W., et al. (2011). Exclusive and common targets of neostriatofugal projections of rat striosomes neurons: a single neuron-tracing study using a viral vector. Eur. J. Neurosci. 33, 668–677. doi: 10.1111/j.1460-9568.2010.07564.x
Gaig, C., and Tolosa, E. (2009). When does Parkinson's disease begin? Mov. Disord. 24(Suppl. 2), S656–S664. doi: 10.1002/mds.22672
Galati, S., Stanzione, P., D'Angelo, V., Fedele, E., Marzetti, F., Sancesario, G., et al. (2009). The pharmacological blockade of medial forebrain bundle induces an acute pathological synchronization of the cortico-subthalamic nucleus-globus pallidus pathway. J. Physiol. 587, 4405–4423. doi: 10.1113/jphysiol.2009.172759
Garcia, P. C., Real, C. C., and Britto, L. R. (2017). The impact of short and long-term exercise on the expression of Arc and AMPARs during evolution of the 6-hydroxy-dopamine animal model of parkinson's disease. J. Mol. Neurosci. 61, 542–552. doi: 10.1007/s12031-017-0896-y
Garea-Rodríguez, E., Eesmaa, A., Lindholm, P., Schlumbohm, C., König, J., Meller, B., et al. (2016). Comparative analysis of the effects of neurotrophic factors CDNF and GDNF in a nonhuman primate model of parkinson's disease. PLoS ONE 11:e0149776. doi: 10.1371/journal.pone.0149776
Gash, D. M., Zhang, Z., Ai, Y., Grondin, R., Coffey, R., and Gerhardt, G. A. (2005). Trophic factor distribution predicts functional recovery in parkinsonian monkeys. Ann. Neurol. 58, 224–233. doi: 10.1002/ana.20549
Gash, D. M., Zhang, Z., Cass, W. A., Ovadia, A., Simmerman, L., Martin, D., et al. (1995). Morphological and functional effects of intranigrally administered GDNF in normal rhesus monkeys. J. Comp. Neurol. 363, 345–358. doi: 10.1002/cne.903630302
Gash, D. M., Zhang, Z., Ovadia, A., Cass, W. A., Yi, A., Simmerman, L., et al. (1996). Functional recovery in parkinsonian monkeys treated with GDNF. Nature 380, 252–255. doi: 10.1038/380252a0
Gerecke, K. M., Jiao, Y., Pani, A., Pagala, V., and Smeyne, R. J. (2010). Exercise protects against MPTP-induced neurotoxicity in mice. Brain Res. 1341, 72–83. doi: 10.1016/j.brainres.2010.01.053
Gerecke, K. M., Jiao, Y., Pagala, V., and Smeyne, R. J. (2012). Exercise does not protect against MPTP-induced neurotoxicity in BDNF haploinsufficient mice. PLoS ONE 7:e43250. doi: 10.1371/journal.pone.0043250
Gerfen, C. R., and Bolam, J. P. (2017). “The neuroanatomical organization of the basal ganglia,” in Handbook of Basal Ganglia Structure and Function, eds H. Steiner and K. Y. Tseng (New York, NY: Academic Press), 3–32.
Gill, S. S., Patel, N. K., Hotton, G. R., O'Sullivan, K., McCarter, R., Bunnage, M., et al. (2003). Direct brain infusion of glial cell line-derived neurotrophic factor in Parkinson disease. Nat. Med. 9, 589–595. doi: 10.1038/nm850
Glass, M., Dragunow, M., and Faull, R. L. (2000). The pattern of neurodegeneration in Huntington's disease: a comparative study of cannabinoid, dopamine, adenosine and GABA(A) receptor alterations in the human basal ganglia in Huntington's disease. Neuroscience 97, 505–519. doi: 10.1016/S0306-4522(00)00008-7
Goedert, M., Spillantini, M. G., Del Tredici, K., and Braak, H. (2013). 100 years of Lewy pathology. Nat. Rev. Neurol. 9, 13–24. doi: 10.1038/nrneurol.2012.242
Goes, A. T., Souza, L. C., Filho, C. B., Del Fabbro, L., De Gomes, M. G., Boeira, S. P., et al. (2014). Neuroprotective effects of swimming training in a mouse model of Parkinson's disease induced by 6-hydroxydopamine. Neuroscience 256, 61–71. doi: 10.1016/j.neuroscience.2013.09.042
Gorski, J. A., Zeiler, S. R., Tamowski, S., and Jones, K. R. (2003). Brain-derived neurotrophic factor is required for the maintenance of cortical dendrites. J. Neurosci. 23, 6856–6865.
Gorton, L. M., Vuckovic, M. G., Vertelkina, N., Petzinger, G. M., Jakowec, M. W., and Wood, R. I. (2010). Exercise effects on motor and affective behavior and catecholamine neurochemistry in the MPTP-lesioned mouse. Behav. Brain Res. 213, 253–262. doi: 10.1016/j.bbr.2010.05.009
Grondin, R., Zhang, Z., Yi, A., Cass, W. A., Maswood, N., Andersen, A. H., et al. (2002). Chronic, controlled GDNF infusion promotes structural and functional recovery in advanced parkinsonian monkeys. Brain 125, 2191–2201. doi: 10.1093/brain/awf234
Hagg, T. (1998). Neurotrophins prevent death and differentially affect tyrosine hydroxylase of adult rat nigrostriatal neurons in vivo. Exp. Neurol. 149, 183–192. doi: 10.1006/exnr.1997.6684
Hardman, C. D., Henderson, J. M., Finkelstein, D. I., Horne, M. K., Paxinos, G., and Halliday, G. M. (2002). Comparison of the basal ganglia in rats, marmosets, macaques, baboons, and humans: volume and neuronal number for the output, internal relay, and striatal modulating nuclei. J. Comp. Neurol. 445, 238–255. doi: 10.1002/cne.10165
Hawkes, C. H., Del Tredici, K., and Braak, H. (2010). A timeline for Parkinson's disease. Parkinsonism Relat. Disord. 16, 79–84. doi: 10.1016/j.parkreldis.2009.08.007
Hegarty, S. V., Lee, D. J., O'Keeffe, G. W., and Sullivan, A. M. (2017). Effects of intracerebral neurotrophic factor application on motor symptoms in Parkinson's disease: a systematic review and meta-analysis. Parkinsonism Relat. Disord. 38, 19–25. doi: 10.1016/j.parkreldis.2017.02.011
Hofer, M., Pagliusi, S. R., Hohn, A., Leibrock, J., and Barde, Y. A. (1990). Regional distribution of brain-derived neurotrophic factor mRNA in the adult mouse brain. EMBO J. 9, 2459–2464.
Hood, R. L., Liguore, W. A., Moore, C., Pflibsen, L., and Meshul, C. K. (2016). Exercise intervention increases spontaneous locomotion but fails to attenuate dopaminergic system loss in a progressive MPTP model in aged mice. Brain Res. 1646, 535–542. doi: 10.1016/j.brainres.2016.06.032
Horch, H. W., and Katz, L. C. (2002). BDNF release from single cells elicits local dendritic growth in nearby neurons. Nat. Neurosci. 5, 1177–1184. doi: 10.1038/nn927
Hornykiewicz, O. (2001). Chemical neuroanatomy of the basal ganglia—normal and in Parkinson's disease. J. Chem. Neuroanat. 22, 3–12. doi: 10.1016/S0891-0618(01)00100-4
Hou, J. G., Lin, L. F., and Mytilineou, C. (1996). Glial cell line-derived neurotrophic factor exerts neurotrophic effects on dopaminergic neurons in vitro and promotes their survival and regrowth after damage by 1-methyl-4-phenylpyridinium. J. Neurochem. 66, 74–82. doi: 10.1046/j.1471-4159.1996.66010074.x
Howells, D. W., Porritt, M. J., Wong, J. Y., Batchelor, P. E., Kalnins, R., Hughes, A. J., et al. (2000). Reduced BDNF mRNA expression in the Parkinson's disease substantia nigra. Exp. Neurol. 166, 127–135. doi: 10.1006/exnr.2000.7483
Huerta-Ocampo, I., Mena-Segovia, J., and Bolam, J. P. (2014). Convergence of cortical and thalamic input to direct and indirect pathway medium spiny neurons in the striatum. Brain Struct. Funct. 219, 1787–1800. doi: 10.1007/s00429-013-0601-z
Hurd, Y. L., Suzuki, M., and Sedvall, G. C. (2001). D1 and D2 dopamine receptor mRNA expression in whole hemisphere sections of the human brain. J. Chem. Neuroanat. 22, 127–137. doi: 10.1016/S0891-0618(01)00122-3
Hyman, C., Hofer, M., Barde, Y. A., Juhasz, M., Yancopoulos, G. D., Squinto, S. P., et al. (1991). BDNF is a neurotrophic factor for dopaminergic neurons of the substantia nigra. Nature 350, 230–232. doi: 10.1038/350230a0
Hyman, C., Juhasz, M., Jackson, C., Wright, P., Ip, N. Y., and Lindsay, R. M. (1994). Overlapping and distinct actions of the neurotrophins BDNF, NT-3, and NT-4/5 on cultured dopaminergic and GABAergic neurons of the ventral mesencephalon. J. Neurosci. 14, 335–347.
Ibáñez, C. F., and Andressoo, J. O. (2017). Biology of GDNF and its receptors - Relevance for disorders of the central nervous system. Neurobiol. Dis. 97, 80–89. doi: 10.1016/j.nbd.2016.01.021
Ibáñez-Sandoval, O., Tecuapetla, F., Unal, B., Shah, F., Koós, T., and Tepper, J. M. (2010). Electrophysiological and morphological characteristics and synaptic connectivity of tyrosine hydroxylase-expressing neurons in adult mouse striatum. J. Neurosci. 30, 6999–7016. doi: 10.1523/JNEUROSCI.5996-09.2010
Ingham, C. A., Hood, S. H., Taggart, P., and Arbuthnott, G. W. (1998). Plasticity of synapses in the rat neostriatum after unilateral lesion of the nigrostriatal dopaminergic pathway. J. Neurosci. 18, 4732–4743.
Jackson, P. A., Pialoux, V., Corbett, D., Drogos, L., Erickson, K. I., Eskes, G. A., et al. (2016). Promoting brain health through exercise and diet in older adults: a physiological perspective. J. Physiol. 594, 4485–4498. doi: 10.1113/JP271270
Jang, Y., Koo, J. H., Kwon, I., Kang, E. B., Um, H. S., Soya, H., et al. (2017). Neuroprotective effects of endurance exercise against neuroinflammation in MPTP-induced Parkinson's disease mice. Brain Res. 1655, 186–193. doi: 10.1016/j.brainres.2016.10.029
Jin, X., Tecuapetla, F., and Costa, R. M. (2014). Basal ganglia subcircuits distinctively encode the parsing and concatenation of action sequences. Nat. Neurosci. 17, 423–430. doi: 10.1038/nn.3632
Jones, T. A., and Schallert, T. (1994). Use-dependent growth of pyramidal neurons after neocortical damage. J. Neurosci. 14, 2140–2152.
Kalia, L. V., Kalia, S. K., and Lang, A. E. (2015). Disease-modifying strategies for Parkinson's disease. Mov. Disord. 30, 1442–1450. doi: 10.1002/mds.26354
Kang, U. J., and Auinger, P., and Parkinson Study Group ELLDOPA Investigators (2012). Activity enhances dopaminergic long-duration response in Parkinson disease. Neurology 78, 1146–1149. doi: 10.1212/WNL.0b013e31824f8056
Kasanetz, F., Riquelme, L. A., O'Donnell, P., and Murer, M. G. (2006). Turning off cortical ensembles stops striatal Up states and elicits phase perturbations in cortical and striatal slow oscillations in rat in vivo. J. Physiol. 577, 97–113. doi: 10.1113/jphysiol.2006.113050
Katzenschlager, R., Head, J., Schrag, A., Ben-Shlomo, Y., Evans, A., and Lees, A. J. (2008). Parkinson's Disease Research Group of the United Kingdom. Fourteen-year final report of the randomized PDRG-UK trial comparing three initial treatments in PD. Neurology 71, 474–480. doi: 10.1212/01.wnl.0000310812.43352.66
Kawaguchi, Y., Wilson, C. J., and Emson, P. C. (1989). Intracellular recording of identified neostriatal patch and matrix spiny cells in a slice preparation preserving cortical inputs. J. Neurophysiol. 62, 1052–1068.
Kawaguchi, Y., Wilson, C. J., and Emson, P. C. (1990). Projection subtypes of rat neostriatal matrix cells revealed by intracellular injection of biocytin. J. Neurosci. 10, 3421–3438.
Kelly, N. A., Ford, M. P., Standaert, D. G., Watts, R. L., Bickel, C. S., Moellering, D. R., et al. (2014). Novel, high-intensity exercise prescription improves muscle mass, mitochondrial function, and physical capacity in individuals with Parkinson's disease. J. Appl. Physiol. (1985). 116, 582–592. doi: 10.1152/japplphysiol.01277.2013
Kemp, J. M., and Powell, T. P. S. (1971). The structure of the caudate nucleus of the cat: light and electron microscopy. Philos. Trans. R. Soc. Lond. B 262, 383–401. doi: 10.1098/rstb.1971.0102
Kincaid, A. E., Zheng, T., and Wilson, C. J. (1998). Connectivity and convergence of single corticostriatal axons. J. Neurosci. 18, 4722–4731.
Kintz, N., Petzinger, G. M., Akopian, G., Ptasnik, S., Williams, C., Jakowec, M. W., et al. (2013). Exercise modifies α-amino- 3-hydroxy−5-methyl-4- isoxazolepropionic acid receptor expression in striatopallidal neurons in the 1-methyl-4-phenyl-1,2,3,6-tetrahydropyridine-lesioned mouse. J. Neurosci. Res. 91, 1492–1507. doi: 10.1002/jnr.23260
Kirik, D., Cederfjäll, E., Halliday, G., and Petersén, Å. (2017). Gene therapy for Parkinson's disease: disease modification by GDNF family of ligands. Neurobiol. Dis. 97, 179–188. doi: 10.1016/j.nbd.2016.09.008
Kirik, D., Georgievska, B., and Björklund, A. (2004). Localized striatal delivery of GDNF as a treatment for Parkinson disease. Nat. Neurosci. 7, 105–110. doi: 10.1038/nn1175
Kirik, D., Rosenblad, C., and Björklund, A. (1998). Characterization of behavioral and neurodegenerative changes following partial lesions of the nigrostriatal dopamine system induced by intrastriatal 6-hydroxydopamine in the rat. Exp. Neurol. 152, 259–277. doi: 10.1006/exnr.1998.6848
Kish, L. J., Palmer, M. R., and Gerhardt, G. A. (1999). Multiple single-unit recordings in the striatum of freely moving animals: effects of apomorphine and D-amphetamine in normal and unilateral 6-hydroxydopamine-lesioned rats. Brain Res. 833, 58–70. doi: 10.1016/S0006-8993(99)01496-1
Kish, S. J., Shannak, K., and Hornykiewicz, O. (1988). Uneven pattern of dopamine loss in the striatum of patients with idiopathic Parkinson's disease. Pathophysiologic and clinical implications. N. Engl. J. Med. 318, 876–880. doi: 10.1056/NEJM198804073181402
Kita, H., and Kita, T. (2011). Cortical stimulation evokes abnormal responses in the dopamine-depleted rat basal ganglia. J. Neurosci. 31, 10311–10322. doi: 10.1523/JNEUROSCI.0915-11.2011
Koo, J. H., Cho, J. Y., and Lee, U. B. (2017a). Treadmill exercise alleviates motor deficits and improves mitochondrial import machinery in an MPTP-induced mouse model of Parkinson's disease. Exp. Gerontol. 89, 20–29. doi: 10.1016/j.exger.2017.01.001
Koo, J. H., Jang, Y. C., Hwang, D. J., Um, H. S., Lee, N. H., Jung, J. H., et al. (2017b). Treadmill exercise produces neuroprotective effects in a murine model of Parkinson's disease by regulating the TLR2/MyD88/NF-κB signaling pathway. Neuroscience 356, 102–113. doi: 10.1016/j.neuroscience.2017.05.016
Kopra, J., Vilenius, C., Grealish, S., Härma, M. A., Varendi, K., Lindholm, J., et al. (2015). GDNF is not required for catecholaminergic neuron survival in vivo. Nat. Neurosci. 18, 319–322. doi: 10.1038/nn.3941
Kordower, J. H., and Bjorklund, A. (2013). Trophic factor gene therapy for Parkinson's disease. Mov. Disord. 28, 96–109. doi: 10.1002/mds.25344
Kordower, J. H., Emborg, M. E., Bloch, J., Ma, S. Y., Chu, Y., Leventhal, L., et al. (2000). Neurodegeneration prevented by lentiviral vector delivery of GDNF in primate models of Parkinson's disease. Science 290, 767–773. doi: 10.1126/science.290.5492.767
Kordower, J. H., Olanow, C. W., Dodiya, H. B., Chu, Y., Beach, T. G., Adler, C. H., et al. (2013). Disease duration and the integrity of the nigrostriatal system in Parkinson's disease. Brain 136, 2419–2431. doi: 10.1093/brain/awt192
Korol, D. L., Gold, P. E., and Scavuzzo, C. J. (2013). Use it and boost it with physical and mental activity. Hippocampus 23, 1125–1135. doi: 10.1002/hipo.22197
Kozlowski, D. A., Connor, B., Tillerson, J. L., Schallert, T., and Bohn, M. C. (2000). Delivery of a GDNF gene into the substantia nigra after a progressive 6-OHDA lesion maintains functional nigrostriatal connections. Exp. Neurol. 166, 1–15. doi: 10.1006/exnr.2000.7463
Kramer, E. R., and Liss, B. (2015). GDNF-Ret signaling in midbrain dopaminergic neurons and its implication for Parkinson disease. FEBS Lett. 589, 3760–3772. doi: 10.1016/j.febslet.2015.11.006
Kravitz, A. V., Freeze, B. S., Parker, P. R., Kay, K., Thwin, M. T., Deisseroth, K., et al. (2010). Regulation of parkinsonian motor behaviours by optogenetic control of basal ganglia circuitry. Nature 466, 622–626. doi: 10.1038/nature09159
LaHue, S. C., Comella, C. L., and Tanner, C. M. (2016). The best medicine? The influence of physical activity and inactivity on Parkinson's disease. Mov. Disord. 31, 1444–1454. doi: 10.1002/mds.26728
Lalanne, T., Oyrer, J., Mancino, A., Gregor, E., Chung, A., Huynh, L., et al. (2016). Synapse-specific expression of calcium-permeable AMPA receptors in neocortical layer 5. J. Physiol. 594, 837–861. doi: 10.1113/JP271394
Lau, Y. S., Patki, G., Das-Panja, K., Le, W. D., and Ahmad, S. O. (2011). Neuroprotective effects and mechanisms of exercise in a chronic mouse model of Parkinson's disease with moderate neurodegeneration. Eur. J. Neurosci. 33, 1264–1274. doi: 10.1111/j.1460-9568.2011.07626.x
Lauzé, M., Daneault, J. F., and Duval, C. (2016). The effects of physical activity in parkinson's disease: a review. J. Parkinsons Dis. 6, 685–698. doi: 10.3233/JPD-160790
Leal, G., Comprido, D., and Duarte, C. B. (2014). BDNF-induced local protein synthesis and synaptic plasticity. Neuropharmacology 76(Pt C), 639–656. doi: 10.1016/j.neuropharm.2013.04.005
Lee, C. S., Sauer, H., and Bjorklund, A. (1996). Dopaminergic neuronal degeneration and motor impairments following axon terminal lesion by instrastriatal 6-hydroxydopamine in the rat. Neuroscience 72, 641–653. doi: 10.1016/0306-4522(95)00571-4
Lee, J., Zhu, W. M., Stanic, D., Finkelstein, D. I., Horne, M. H., Henderson, J., et al. (2008). Sprouting of dopamine terminals and altered dopamine release and uptake in Parkinsonian dyskinaesia. Brain 131, 1574–1587. doi: 10.1093/brain/awn085
Leibrock, J., Lottspeich, F., Hohn, A., Hofer, M., Hengerer, B., Masiakowski, P., et al. (1989). Molecular cloning and expression of brain-derived neurotrophic factor. Nature 341, 149–152. doi: 10.1038/341149a0
Levey, A. I., Hersch, S. M., Rye, D. B., Sunahara, R. K., Niznik, H. B., Kitt, C. A., et al. (1993). Localization of D1 and D2 dopamine receptors in brain with subtype-specific antibodies. Proc. Natl. Acad. Sci. U.S.A. 90, 8861–8865. doi: 10.1073/pnas.90.19.8861
Levi-Montalcini, R., and Cohen, S. (1956). In vitro and in vivo effects of a nerve-stimulating agent isolated from snake venom. Proc. Natl. Acad. Sci. U.S.A. 42, 695–699. doi: 10.1073/pnas.42.9.695
Levi-Montalcini, R., and Hamburger, V. (1951). Selective growth stimulating effects of mouse sarcoma on the sensory and sympathetic nervous system of the chick embryo. J. Exp. Zool. 116, 321–361. doi: 10.1002/jez.1401160206
Levi-Montalcini, R., Skaper, S. D., Dal Toso, R., Petrelli, L., and Leon, A. (1996). Nerve growth factor: from neurotrophin to neurokine. Trends Neurosci. 19, 514–520. doi: 10.1016/S0166-2236(96)10058-8
Levivier, M., Przedborski, S., Bencsics, C., and Kang, U. J. (1995). Intrastriatal implantation of fibroblasts genetically engineered to produce brain-derived neurotrophic factor prevents degeneration of dopaminergic neurons in a rat model of Parkinson's disease. J. Neurosci. 15, 7810–7820.
Lewis, D. A., Melchitzky, D. S., Sesack, S. R., Whitehead, R. E., Auh, S., and Sampson, A. (2001). Dopamine transporter immunoreactivity in monkey cerebral cortex: regional, laminar, and ultrastructural localization. J. Comp. Neurol. 432, 119–136. doi: 10.1002/cne.1092
Li, F., Harmer, P., Fitzgerald, K., Eckstrom, E., Stock, R., Galver, J., et al. (2012). Tai chi and postural stability in patients with Parkinson's disease. N. Engl. J. Med. 366, 511–519. doi: 10.1056/NEJMoa1107911
Li, F., Harmer, P., Liu, Y., Eckstrom, E., Fitzgerald, K., Stock, R., et al. (2014). A randomized controlled trial of patient-reported outcomes with tai chi exercise in Parkinson's disease. Mov. Disord. 29, 539–545. doi: 10.1002/mds.25787
Li, L., Sagot, B., and Zhou, F.-M. (2015). Similar L-dopa-stimulated motor activity in mice with adult-onset 6-hydroxydopamine-induced symmetric dopamine denervation and in transcription factor Pitx3 null mice with perinatal-onset symmetric dopamine denervation. Brain Res. 1615, 12–21. doi: 10.1016/j.brainres.2015.04.011
Li, W., Englund, E., Widner, H., Mattsson, B., van Westen, D., Lätt, J., et al. (2016). Extensive graft-derived dopaminergic innervation is maintained 24 years after transplantation in the degenerating parkinsonian brain. Proc. Natl. Acad. Sci. U.S.A. 113, 6544–6549. doi: 10.1073/pnas.1605245113
Li, Y., Yui, D., Luikart, B. W., McKay, R. M., Li, Y., Rubenstein, J. L., et al. (2012). Conditional ablation of brain-derived neurotrophic factor-TrkB signaling impairs striatal neuron development. Proc. Natl. Acad. Sci. U. S.A. 109, 15491–15496. doi: 10.1073/pnas.1212899109
Liberatore, G. T., Finkelstein, D. I., Wong, J. Y., Horne, M. K., Porritt, M. J., Donnan, G. A., et al. (1999). Sprouting of dopaminergic axons after striatal injury: confirmation by markers not dependent on dopamine metabolism. Exp. Neurol. 159, 565–573. doi: 10.1006/exnr.1999.7152
Lin, L. F., Doherty, D. H., Lile, J. D., Bektesh, S., and Collins, F. (1993). GDNF: a glial cell line-derived neurotrophic factor for midbrain dopaminergic neurons. Science 260, 1130–1132. doi: 10.1126/science.8493557
Lindahl, M., Saarma, M., and Lindholm, P. (2017). Unconventional neurotrophic factors CDNF and MANF: structure, physiological functions and therapeutic potential. Neurobiol. Dis. 97, 90–102. doi: 10.1016/j.nbd.2016.07.009
Lindholm, P., Voutilainen, M. H., Laurén, J., Peränen, J., Leppänen, V. M., Andressoo, J. O., et al. (2007). Novel neurotrophic factor CDNF protects and rescues midbrain dopamine neurons in vivo. Nature 448, 73–77. doi: 10.1038/nature05957
Liu, S. J., and Savtchouk, I. (2012). Ca2+ permeable AMPA receptors switch allegiances: mechanisms and consequences. J. Physiol. 590, 13–20. doi: 10.1113/jphysiol.2011.213926
Logroscino, G., Sesso, H. D., Paffenbarger, R. S. Jr., and Lee, I. M. (2006). Physical activity and risk of Parkinson's disease: a prospective cohort study. J. Neurol. Neurosurg. Psychiatry 77, 1318–1322. doi: 10.1136/jnnp.2006.097170
Lu, B., Nagappan, G., Guan, X., Nathan, P. J., and Wren, P. (2013). BDNF-based synaptic repair as a disease-modifying strategy for neurodegenerative diseases. Nat. Rev. Neurosci. 14, 401–416. doi: 10.1038/nrn3505
Lu, X., and Hagg, T. (1997). Glial cell line-derived neurotrophic factor prevents death, but not reductions in tyrosine hydroxylase, of injured nigrostriatal neurons in adult rats. J. Comp. Neurol. 388, 484–494. doi: 10.1002/(SICI)1096-9861(19971124)388:3<484::AID-CNE10>3.0.CO;2-M
Mahon, S., Vautrelle, N., Pezard, L., Slaght, S. J., Deniau, J. M., Chouvet, G., et al. (2006). Distinct patterns of striatal medium spiny neuron activity during the natural sleep-wake cycle. J. Neurosci. 26, 12587–12595. doi: 10.1523/JNEUROSCI.3987-06.2006
Mang, C. S., Campbell, K. L., Ross, C. J., and Boyd, L. A. (2013). Promoting neuroplasticity for motor rehabilitation after stroke: considering the effects of aerobic exercise and genetic variation on brain-derived neurotrophic factor. Phys. Ther. 93, 1707–1716. doi: 10.2522/ptj.20130053
Marston, K. J., Newton, M. J., Brown, B. M., Rainey-Smith, S. R., Bird, S., Martins, R. N., et al. (2017). Intense resistance exercise increases peripheral brain-derived neurotrophic factor. J. Sci. Med. Sport 20, 899–903. doi: 10.1016/j.jsams.2017.03.015
Marusiak, J., Zeligowska, E., Mencel, J., Kisiel-Sajewicz, K., Majerczak, J., Zoladz, J. A., et al. (2015). Interval training-induced alleviation of rigidity and hypertonia in patients with Parkinson's disease is accompanied by increased basal serum brain-derived neurotrophic factor. J. Rehabil. Med. 47, 372–375. doi: 10.2340/16501977-1931
Maswood, N., Grondin, R., Zhang, Z., Stanford, J. A., Surgener, S. P., Gash, D. M., et al. (2002). Effects of chronic intraputamenal infusion of glial cell line-derived neurotrophic factor (GDNF) in aged Rhesus monkeys. Neurobiol. Aging 23, 881–889. doi: 10.1016/S0197-4580(02)00022-2
Matsuda, W., Furuta, T., Nakamura, K. C., Hioki, H., Fujiyama, F., Arai, R., et al. (2009). Single nigrostriatal dopaminergic neurons form widely spread and highly dense axonal arborizations in the neostriatum. J. Neurosci. 29, 444–453. doi: 10.1523/JNEUROSCI.4029-08.2009
McNeill, T. H., Brown, S. A., Rafols, J. A., and Shoulson, I. (1988). Atrophy of medium spiny I striatal dendrites in advanced Parkinson's disease. Brain Res. 455, 148–152. doi: 10.1016/0006-8993(88)90124-2
Mitchell, I. J., Cooper, A. J., and Griffiths, M. R. (1999). The selective vulnerability of striatopallidal neurons. Prog. Neurobiol. 59, 691–719. doi: 10.1016/S0301-0082(99)00019-2
Mogi, M., Togari, A., Kondo, T., Mizuno, Y., Komure, O., Kuno, S., et al. (1999). Brain-derived growth factor and nerve growth factor concentrations are decreased in the substantia nigra in Parkinson's disease. Neurosci. Lett. 270, 45–48. doi: 10.1016/S0304-3940(99)00463-2
Morigaki, R., and Goto, S. (2016). Putaminal Mosaic Visualized by Tyrosine Hydroxylase Immunohistochemistry in the Human Neostriatum. Front. Neuroanat. 10:34. doi: 10.3389/fnana.2016.00034
Moss, J., and Bolam, J. P. (2008). A dopaminergic axon lattice in the striatum and its relationship with cortical and thalamic terminals. J. Neurosci. 28, 11221–11230. doi: 10.1523/JNEUROSCI.2780-08.2008
Mounayar, S., Boulet, S., Tandé, D., Jan, C., Pessiglione, M., Hirsch, E. C., et al. (2007). A new model to study compensatory mechanisms in MPTP-treated monkeys exhibiting recovery. Brain 130, 2898–2914. doi: 10.1093/brain/awm208
Muriel, M. P., Agid, Y., and Hirsch, E. (2001). Plasticity of afferent fibers to striatal neurons bearing D1 dopamine receptors in Parkinson's disease. Mov. Disord. 16, 435–441. doi: 10.1002/mds.1103
Nagahara, A. H., and Tuszynski, M. H. (2011). Potential therapeutic uses of BDNF in neurological and psychiatric disorders. Nat. Rev. Drug Discov. 10, 209–219. doi: 10.1038/nrd3366
Nakajima, K., Hida, H., Shimano, Y., Fujimoto, I., Hashitani, T., Kumazaki, M., et al. (2001). GDNF is a major component of trophic activity in DA-depleted striatum for survival and neurite extension of DAergic neurons. Brain Res. 916, 76–84. doi: 10.1016/S0006-8993(01)02866-9
Nambu, A. (2008). Seven problems on the basal ganglia. Curr. Opin. Neurobiol. 18, 595–604. doi: 10.1016/j.conb.2008.11.001
Nambu, A. (2011). Somatotopic organization of the primate Basal Ganglia. Front. Neuroanat. 5:26. doi: 10.3389/fnana.2011.00026
Neeper, S. A., Gómez-Pinilla, F., Choi, J., and Cotman, C. (1995). Exercise and brain neurotrophins. Nature 373:109. doi: 10.1038/373109a0
Neeper, S. A., Gómez-Pinilla, F., Choi, J., and Cotman, C. W. (1996). Physical activity increases mRNA for brain-derived neurotrophic factor and nerve growth factor in rat brain. Brain Res. 726, 49–56. doi: 10.1016/0006-8993(96)00273-9
Nishijima, T., Torres-Aleman, I., and Soya, H. (2016). Exercise and cerebrovascular plasticity. Prog. Brain Res. 225, 243–268. doi: 10.1016/bs.pbr.2016.03.010
Noyce, A. J., Lees, A. J., and Schrag, A. E. (2016). The prediagnostic phase of Parkinson's disease. J. Neurol. Neurosurg. Psychiatry. 87, 871–878. doi: 10.1136/jnnp-2015-311890
Numan, S., and Seroogy, K. B. (1999). Expression of trkB and trkC mRNAs by adult midbrain dopamine neurons: a double-label in situ hybridization study. J. Comp. Neurol. 403, 295–308.
Obeso, J. A., Rodriguez-Oroz, M. C., Stamelou, M., Bhatia, K. P., and Burn, D. J. (2014). The expanding universe of disorders of the basal ganglia. Lancet 384, 523–531. doi: 10.1016/S0140-6736(13)62418-6
O'Dell, S. J., Gross, N. B., Fricks, A. N., Casiano, B. D., Nguyen, T. B., and Marshall, J. F. (2007). Running wheel exercise enhances recovery from nigrostriatal dopamine injury without inducing neuroprotection. Neuroscience 144, 1141–1151. doi: 10.1016/j.neuroscience.2006.10.042
Oguh, O., Eisenstein, A., Kwasny, M., and Simuni, T. (2014). Back to the basics: regular exercise matters in parkinson's disease: results from the National Parkinson Foundation QII registry study. Parkinsonism Relat. Disord. 20, 1221–1225. doi: 10.1016/j.parkreldis.2014.09.008
Olanow, C. W., Bartus, R. T., Volpicelli-Daley, L. A., and Kordower, J. H. (2015). Trophic factors for Parkinson's disease: to live or let die. Mov. Disord. 30, 1715–1724. doi: 10.1002/mds.26426
Olanow, C. W., Stern, M. B., and Sethi, K. (2009). The scientific and clinical basis for the treatment of Parkinson disease (2009). Neurology 72(21Suppl. 4), S1–S136. doi: 10.1212/WNL.0b013e3181a1d44c
Olson, L., Backlund, E. O., Ebendal, T., Freedman, R., Hamberger, B., Hansson, P., et al. (1991). Intraputaminal infusion of nerve growth factor to support adrenal medullary autografts in Parkinson's disease: one-year follow-up of first clinical trial. Arch. Neurol. 48, 373–381. doi: 10.1001/archneur.1991.00530160037011
Oorschot, D. E. (1996). Total number of neurons in the neostriatal, pallidal, subthalamic, and substantia nigral nuclei of the rat basal ganglia: a stereological study using the cavalieri and optical disector methods. J. Comp. Neurol. 366, 580–599.
Oppenheim, R. W., Houenou, L. J., Johnson, J. E., Lin, L. F., Li, L., Lo, A. C., et al. (1995). Developing motor neurons rescued from programmed and axotomy-induced cell death by GDNF. Nature 373, 344–346. doi: 10.1038/373344a0
Orefice, L. L., Shih, C. C., Xu, H., Waterhouse, E. G., and Xu, B. (2016). Control of spine maturation and pruning through proBDNF synthesized and released in dendrites. Mol. Cell. Neurosci. 71, 66–79. doi: 10.1016/j.mcn.2015.12.010
Orefice, L. L., Waterhouse, E. G., Partridge, J. G., Lalchandani, R. R., Vicini, S., and Xu, B. (2013). Distinct roles for somatically and dendritically synthesized brain-derived neurotrophic factor in morphogenesis of dendritic spines. J. Neurosci. 33, 11618–11632. doi: 10.1523/JNEUROSCI.0012-13.2013
Ostergaard, K., Jones, S. A., Hyman, C., and Zimmer, J. (1996). Effects of donor age and brain-derived neurotrophic factor on the survival of dopaminergic neurons and axonal growth in postnatal rat nigrostriatal cocultures. Exp. Neurol. 142, 340–350. doi: 10.1006/exnr.1996.0203
Parain, K., Murer, M. G., Yan, Q., Faucheux, B., Agid, Y., Hirsch, E., et al. (1999). Reduced expression of brain-derived neurotrophic factor protein in Parkinson's disease substantia nigra. Neuroreport 10, 557–561. doi: 10.1097/00001756-199902250-00021
Parent, A., and Hazrati, L. N. (1995). Functional anatomy of the basal ganglia. II. The place of subthalamic nucleus and external pallidum in basal ganglia circuitry. Brain Res. Brain Res. Rev. 20, 128–154. doi: 10.1016/0165-0173(94)00008-D
Park, H., and Poo, M. M. (2013). Neurotrophin regulation of neural circuit development and function. Nat. Rev. Neurosci. 14, 7–23. doi: 10.1038/nrn3379
Pascual, A., and López-Barneo, J. (2015). Reply to “GDNF is not required for catecholaminergic neuron survival in vivo”. Nat. Neurosci. 18, 322–323. doi: 10.1038/nn.3942
Pascual, A., Hidalgo-Figueroa, M., Piruat, J. I., Pintado, C. O., Gómez-Díaz, R., and López-Barneo, J. (2008). Absolute requirement of GDNF for adult catecholaminergic neuron survival. Nat. Neurosci. 11, 755–761. doi: 10.1038/nn.2136
Pedersen, B. K., and Saltin, B. (2015). Exercise as medicine - evidence for prescribing exercise as therapy in 26 different chronic diseases. Scand. J. Med. Sci. Sports 25(Suppl. 3), 1–72. doi: 10.1111/sms.12581
Pereira, A. C., Huddleston, D. E., Brickman, A. M., Sosunov, A. A., Hen, R., McKhann, G. M., et al. (2007). An in vivo correlate of exercise-induced neurogenesis in the adult dentate gyrus. Proc. Natl. Acad. Sci. U.S.A. 104, 5638–5643. doi: 10.1073/pnas.0611721104
Petzinger, G. M., Fisher, B. E., McEwen, S., Beeler, J. A., Walsh, J. P., and Jakowec, M. W. (2013). Exercise-enhanced neuroplasticity targeting motor and cognitive circuitry in Parkinson's disease. Lancet Neurol. 12, 716–726. doi: 10.1016/S1474-4422(13)70123-6
Petzinger, G. M., Fisher, B., Hogg, E., Abernathy, A., Arevalo, P., Nixon, K., et al. (2006). Behavioral motor recovery in the 1-methyl-4-phenyl-1,2,3,6-tetrahydropyridine-lesioned squirrel monkey (Saimiri sciureus): changes in striatal dopamine and expression of tyrosine hydroxylase and dopamine transporter proteins. J. Neurosci. Res. 83, 332–347. doi: 10.1002/jnr.20730
Petzinger, G. M., Walsh, J. P., Akopian, G., Hogg, E., Abernathy, A., Arevalo, P., et al. (2007). Effects of treadmill exercise on dopaminergic transmission in the 1-methyl-4-phenyl-1,2,3,6- tetrahydropyridine-lesioned mouse model of basal ganglia injury. J. Neurosci. 27, 5291–5300. doi: 10.1523/JNEUROSCI.1069-07.2007
Porritt, M. J., Batchelor, P. E., and Howells, D. W. (2005). Inhibiting BDNF expression by antisense oligonucleotide infusion causes loss of nigral dopaminergic neurons. Exp. Neurol. 192, 226–234. doi: 10.1016/j.expneurol.2004.11.030
Prensa, L., Cossette, M., and Parent, A. (2000). Dopaminergic innervation of human basal ganglia. J. Chem. Neuroanat. 20, 207–213. doi: 10.1016/S0891-0618(00)00099-5
Preston, R. J., Bishop, G. A., and Kitai, S. T. (1980). Medium spiny neuron projection from the rat striatum: an intracellular horseradish peroxidase study. Brain Res. 183, 253–263. doi: 10.1016/0006-8993(80)90462-X
Pringsheim, T., Jette, N., Frolkis, A., and Steeves, T. D. (2014). The prevalence of Parkinson's disease: a systematic review and meta-analysis. Mov. Disord. 29, 1583–1590. doi: 10.1002/mds.25945
Rafferty, M. R., Schmidt, P. N., Luo, S. T., Li, K., Marras, C., Davis, T. L. Investigators, et al. (2017). Regular exercise, quality of life, and mobility in parkinson's disease: a longitudinal analysis of national parkinson foundation quality improvement initiative data. J. Parkinsons Dis. 7, 193–202. doi: 10.3233/JPD-160912
Rangasamy, S. B., Soderstrom, K., Bakay, R. A., and Kordower, J. H. (2010). Neurotrophic factor therapy for Parkinson's disease. Prog. Brain Res. 184, 237–264. doi: 10.1016/S0079-6123(10)84013-0
Rauskolb, S., Zagrebelsky, M., Dreznjak, A., Deogracias, R., Matsumoto, T., Wiese, S., et al. (2010). Global deprivation of brain-derived neurotrophic factor in the CNS reveals an area-specific requirement for dendritic growth. J. Neurosci. 30, 1739–1749. doi: 10.1523/JNEUROSCI.5100-09.2010
Real, C. C., Ferreira, A. F., Chaves-Kirsten, G. P., Torrão, A. S., Pires, R. S., and Britto, L. R. (2013). BDNF receptor blockade hinders the beneficial effects of exercise in a rat model of Parkinson's disease. Neuroscience 237, 118–129. doi: 10.1016/j.neuroscience.2013.01.060
Real, C. C., Garcia, P. C., and Britto, L. R. G. (2017). Treadmill exercise prevents increase of neuroinflammation markers involved in the dopaminergic damage of the 6-OHDA parkinson's disease model. J. Mol. Neurosci. 63, 36–49. doi: 10.1007/s12031-017-0955-4
Reynolds, G. O., Otto, M. W., Ellis, T. D., and Cronin-Golomb, A. (2016). The therapeutic potential of exercise to improve mood, cognition, and sleep in parkinson's disease. Mov. Disord. 31, 23–38. doi: 10.1002/mds.26484
Sääksjärvi, K., Knekt, P., Männistö, S., Lyytinen, J., Jääskeläinen, T., Kanerva, N., et al. (2014). Reduced risk of Parkinson's disease associated with lower body mass index and heavy leisure-time physical activity. Eur. J. Epidemiol. 29, 285–292. doi: 10.1007/s10654-014-9887-2
Salat, D., Noyce, A. J., Schrag, A., and Tolosa, E. (2016). Challenges of modifying disease progression in prediagnostic Parkinson's disease. Lancet Neurol. 15, 637–648. doi: 10.1016/S1474-4422(16)00060-0
Salvatore, M. F., Ai, Y., Fischer, B., Zhang, A. M., Grondin, R. C., Zhang, Z., et al. (2006). Point source concentration of GDNF may explain failure of phase II clinical trial. Exp. Neurol. 202, 497–505. doi: 10.1016/j.expneurol.2006.07.015
Sanchez-Ramos, J. R., Michel, P., Weiner, W. J., and Hefti, F. (1988). Selective destruction of cultured dopaminergic neurons from fetal rat mesencephalon by 1-methyl-4-phenylpyridinium: cytochemical and morphological evidence. J. Neurochem. 50, 1934–1944. doi: 10.1111/j.1471-4159.1988.tb02500.x
Sano, H., Chiken, S., Hikida, T., Kobayashi, K., and Nambu, A. (2013). Signals through the striatopallidal indirect pathway stop movements by phasic excitation in the substantia nigra. J. Neurosci. 33, 7583–7594. doi: 10.1523/JNEUROSCI.4932-12.2013
Sano, H., Yasoshima, Y., Matsushita, N., Kaneko, T., Kohno, K., Pastan, I., et al. (2003). Conditional ablation of striatal neuronal types containing dopamine D2 receptor disturbs coordination of basal ganglia function. J. Neurosci. 23, 9078–9088.
Sasco, A. J., Paffenbarger, R. S. Jr., Gendre, I., and Wing, A. L. (1992). The role of physical exercise in the occurrence of Parkinson's disease. Arch. Neurol. 49, 360–365. doi: 10.1001/archneur.1992.00530280040020
Sasi, M., Vignoli, B., Canossa, M., and Blum, R. (2017). Neurobiology of local and intercellular BDNF signaling. Pflugers Arch. 469, 593–610. doi: 10.1007/s00424-017-1964-4
Savica, R., Rocca, W. A., and Ahlskog, J. E. (2010). When does Parkinson disease start? Arch Neurol. 67, 798–801. doi: 10.1001/archneurol.2010.135
Scalzo, P., Kümmer, A., Bretas, T. L., Cardoso, F., and Teixeira, A. L. (2010). Serum levels of brain-derived neurotrophic factor correlate with motor impairment in Parkinson's disease. J. Neurol. 257, 540–545. doi: 10.1007/s00415-009-5357-2
Schallert, T., Fleming, S. M., Leasure, J. L., Tillerson, J. L., and Bland, S. T. (2000). CNS plasticity and assessment of forelimb sensorimotor outcome in unilateral rat models of stroke, cortical ablation, parkinsonism and spinal cord injury. Neuropharmacology 39, 777–787. doi: 10.1016/S0028-3908(00)00005-8
Schallert, T., Kozlowski, D. A., Humm, J. L., and Cocke, R. R. (1997). Use-dependent structural events in recovery of function. Adv. Neurol. 73, 229–238.
Scholz, B., Svensson, M., Alm, H., Sköld, K., Fälth, M., Kultima, K., et al. (2008). Striatal proteomic analysis suggests that first L-dopa dose equates to chronic exposure. PLoS ONE 3:e1589. doi: 10.1371/journal.pone.0001589
Schwarting, R. K., and Huston, J. P. (1996). The unilateral 6-hydroxydopamine lesion model in behavioral brain research. Analysis of functional deficits, recovery and treatments. Prog. Neurobiol. 50, 275–331. doi: 10.1016/S0301-0082(96)00040-8
Sconce, M. D., Churchill, M. J., Greene, R. E., and Meshul, C. K. (2015). Intervention with exercise restores motor deficits but not nigrostriatal loss in a progressive MPTP mouse model of Parkinson's disease. Neuroscience 299, 156–174. doi: 10.1016/j.neuroscience.2015.04.069
Shah, C., Beall, E. B., Frankemolle, A. M., Penko, A., Phillips, M. D., Lowe, M. J., et al. (2016). Exercise Therapy for parkinson's disease: pedaling rate is related to changes in motor connectivity. Brain Connect. 6, 25–36. doi: 10.1089/brain.2014.0328
Shi, K., Liu, X., Qiao, D., and Hou, L. (2017). Effects of Treadmill Exercise on Spontaneous Firing Activities of Striatal Neurons in a Rat Model of Parkinson's Disease. Motor Control. 21, 58–71. doi: 10.1123/mc.2015-0065
Shih, I. F., Liew, Z., Krause, N., and Ritz, B. (2016). Lifetime occupational and leisure time physical activity and risk of Parkinson's disease. Parkinsonism Relat. Disord. 28, 112–117. doi: 10.1016/j.parkreldis.2016.05.007
Shulman, L. M., Katzel, L. I., Ivey, F. M., Sorkin, J. D., Favors, K., Anderson, K. E., et al. (2013). Randomized clinical trial of 3 types of physical exercise for patients with Parkinson disease. JAMA Neurol. 70, 183–190. doi: 10.1001/jamaneurol.2013.646
Singh, A. M., Neva, J. L., and Staines, W. R. (2014). Acute exercise enhances the response to paired associative stimulation-induced plasticity in the primary motor cortex. Exp. Brain Res. 232, 3675–3685. doi: 10.1007/s00221-014-4049-z
Singh, A., Mewes, K., Gross, R. E., DeLong, M. R., Obeso, J. A., and Papa, S. M. (2016). Human striatal recordings reveal abnormal discharge of projection neurons in Parkinson's disease. Proc. Natl. Acad. Sci. U. S. A. 113, 9629–9634. doi: 10.1073/pnas.1606792113
Slevin, J. T., Gerhardt, G. A., Smith, C. D., Gash, D. M., Kryscio, R., and Young, B. (2005). Improvement of bilateral motor functions in patients with Parkinson disease through the unilateral intraputaminal infusion of glial cell line-derived neurotrophic factor. J. Neurosurg. 102, 216–222. doi: 10.3171/jns.2005.102.2.0216
Smeyne, M., Sladen, P., Jiao, Y., Dragatsis, I., and Smeyne, R. J. (2015). HIF1α is necessary for exercise-induced neuroprotection while HIF2α is needed for dopaminergic neuron survival in the substantia nigra pars compacta. Neuroscience 295, 23–38. doi: 10.1016/j.neuroscience.2015.03.015
Smith, Y., Galvan, A., Ellender, T. J., Doig, N., Villalba, R. M., Huerta-Ocampo, I., et al. (2014). The thalamostriatal system in normal and diseased states. Front. Syst. Neurosci. 8:5. doi: 10.3389/fnsys.2014.00005
Song, D. D., and Haber, S. N. (2000). Striatal responses to partial dopaminergic lesion: evidence for compensatory sprouting. J. Neurosci. 20, 5102–5114.
Spenger, C., Hyman, C., Studer, L., Egli, M., Evtouchenko, L., Jackson, C., et al. (1995). Effects of BDNF on dopaminergic, serotonergic, and GABAergic neurons in cultures of human fetal ventral mesencephalon. Exp. Neurol. 133, 50–63. doi: 10.1006/exnr.1995.1007
Spielman, L. J., Little, J. P., and Klegeris, A. (2016). Physical activity and exercise attenuate neuroinflammation in neurological diseases. Brain Res Bull. 125, 19–29. doi: 10.1016/j.brainresbull.2016.03.012
Stanic, D., Finkelstein, D. I., Bourke, D. W., Drago, J., and Horne, M. K. (2003a). Timecourse of striatal re-innervation following lesions of dopaminergic SNpc neurons of the rat. Eur. J. Neurosci. 18, 1175–1188. doi: 10.1046/j.1460-9568.2003.02800.x
Stanic, D., Parish, C. L., Zhu, W. M., Krstew, E. V., Lawrence, A. J., Drago, J., et al. (2003b). Changes in function and ultrastructure of striatal dopaminergic terminals that regenerate following partial lesions of the SNpc. J. Neurochem. 86, 329–343. doi: 10.1046/j.1471-4159.2003.01843.x
Stephens, B., Mueller, A. J., Shering, A. F., Hood, S. H., Taggart, P., Arbuthnott, G. W., et al. (2005). Evidence of a breakdown of corticostriatal connections in Parkinson's disease. Neuroscience 132, 741–754. doi: 10.1016/j.neuroscience.2005.01.007
Subramaniam, S. R., and Chesselet, M. F. (2013). Mitochondrial dysfunction and oxidative stress in Parkinson's disease. Prog. Neurobiol. 106–107, 17–32. doi: 10.1016/j.pneurobio.2013.04.004
Sun, M., Kong, L., Wang, X., Lu, X. G., Gao, Q., and Geller, A. I. (2005). Comparison of the capability of GDNF, BDNF, or both, to protect nigrostriatal neurons in a rat model of Parkinson's disease. Brain Res. 1052, 119–129. doi: 10.1016/j.brainres.2005.05.072
Sung, Y. H., Kim, S. C., Hong, H. P., Park, C. Y., Shin, M. S., Kim, C. J., et al. (2012). Treadmill exercise ameliorates dopaminergic neuronal loss through suppressing microglial activation in Parkinson's disease mice. Life Sci. 91, 1309–1316. doi: 10.1016/j.lfs.2012.10.003
Tajiri, N., Yasuhara, T., Shingo, T., Kondo, A., Yuan, W., Kadota, T., et al. (2010). Exercise exerts neuroprotective effects on Parkinson's disease model of rats. Brain Res. 1310, 200–207. doi: 10.1016/j.brainres.2009.10.075
Takamatsu, Y., Ishida, A., Hamakawa, M., Tamakoshi, K., Jung, C. G., and Ishida, K. (2010). Treadmill running improves motor function and alters dendritic morphology in the striatum after collagenase-induced intracerebral hemorrhage in rats. Brain Res. 1355, 165–173. doi: 10.1016/j.brainres.2010.07.070
Tamakoshi, K., Ishida, A., Takamatsu, Y., Hamakawa, M., Nakashima, H., Shimada, H., et al. (2014). Motor skills training promotes motor functional recovery and induces synaptogenesis in the motor cortex and striatum after intracerebral hemorrhage in rats. Behav. Brain Res. 260, 34–43. doi: 10.1016/j.bbr.2013.11.034
Tatton, W. G., Kwan, M. M., Verrier, M. C., Seniuk, N. A., and Theriault, E. (1990). MPTP produces reversible disappearance of tyrosine hydroxylase-containing retinal amacrine cells. Brain Res. 527, 21–31. doi: 10.1016/0006-8993(90)91056-M
Tecuapetla, F., Jin, X., Lima, S. Q., and Costa, R. M. (2016). Complementary contributions of striatal projection pathways to action initiation and execution. Cell 166, 703–715. doi: 10.1016/j.cell.2016.06.032
Thacker, E. L., Chen, H., Patel, A. V., McCullough, M. L., Calle, E. E., Thun, M. J., et al. (2008). Recreational physical activity and risk of Parkinson's disease. Mov. Disord. 23, 69–74. doi: 10.1002/mds.21772
Tillerson, J. L., Caudle, W. M., Reverón, M. E., and Miller, G. W. (2003). Exercise induces behavioral recovery and attenuates neurochemical deficits in rodent models of Parkinson's disease. Neuroscience 119, 899–911. doi: 10.1016/S0306-4522(03)00096-4
Tillerson, J. L., Cohen, A. D., Caudle, W. M., Zigmond, M. J., Schallert, T., and Miller, G. W. (2002). Forced nonuse in unilateral parkinsonian rats exacerbates injury. J. Neurosci. 22, 6790–6799.
Tillerson, J. L., Cohen, A. D., Philhower, J., Miller, G. W., Zigmond, M. J., and Schallert, T. (2001). Forced limb-use effects on the behavioral and neurochemical effects of 6-hydroxydopamine. J. Neurosci. 21, 4427–4435.
Tomac, A., Lindqvist, E., Lin, L. F., Ogren, S. O., Young, D., Hoffer, B. J., et al. (1995). Protection and repair of the nigrostriatal dopaminergic system by GDNF in vivo. Nature 373, 335–339. doi: 10.1038/373335a0
Toy, W. A., Petzinger, G. M., Leyshon, B. J., Akopian, G. K., Walsh, J. P., Hoffman, M. V., et al. (2014). Treadmill exercise reverses dendritic spine loss in direct and indirect striatal medium spiny neurons in the 1-methyl-4-phenyl-1,2,3,6- tetrahydropyridine (MPTP) mouse model of Parkinson's disease. Neurobiol. Dis. 63, 201–209. doi: 10.1016/j.nbd.2013.11.017
Tremblay, L., Worbe, Y., Thobois, S., Sgambato-Faure, V., and Féger, J. (2015). Selective dysfunction of basal ganglia subterritories: from movement to behavioral disorders. Mov. Disord. 30, 1155–1170. doi: 10.1002/mds.26199
Tritsch, N. X., and Sabatini, B. L. (2012). Dopaminergic modulation of synaptic transmission in cortex and striatum. Neuron 76, 33–50. doi: 10.1016/j.neuron.2012.09.023
Tseng, K. Y., Kasanetz, F., Kargieman, L., Riquelme, L. A., and Murer, M. G. (2001). Cortical slow oscillatory activity is reflected in the membrane potential and spike trains of striatal neurons in rats with chronic nigrostriatal lesions. J. Neurosci. 21, 6430–6439.
Tsou, Y. H., Shih, C. T., Ching, C. H., Huang, J. Y., Jen, C. J., Yu, L., et al. (2015). Treadmill exercise activates Nrf2 antioxidant system to protect the nigrostriatal dopaminergic neurons from MPP+ toxicity. Exp. Neurol. 263, 50–62. doi: 10.1016/j.expneurol.2014.09.021
Tuon, T., Valvassori, S. S., Dal Pont, G. C., Paganini, C. S., Pozzi, B. G., Luciano, T. F., et al. (2014). Physical training prevents depressive symptoms and a decrease in brain-derived neurotrophic factor in Parkinson's disease. Brain Res Bull. 108, 106–112 doi: 10.1016/j.brainresbull.2014.09.006
Tuon, T., Valvassori, S. S., Lopes-Borges, J., Luciano, T., Trom, C. B., Silva, L. A., et al. (2012). Physical training exerts neuroprotective effects in the regulation of neurochemical factors in an animal model of Parkinson's disease. Neuroscience 227, 305–312. doi: 10.1016/j.neuroscience.2012.09.063
Ungerstedt, U. (1971a). Postsynaptic supersensitivity after 6-hydroxy-dopamine induced degeneration of the nigro-striatal dopamine system. Acta Physiol. Scand. Suppl. 367, 69–93. doi: 10.1111/j.1365-201X.1971.tb11000.x
Ungerstedt, U. (1971b). Adipsia and aphagia after 6-hydroxydopamine induced degeneration of the nigro-striatal dopamine system. Acta Physiol. Scand. Suppl. 367, 95–122. doi: 10.1111/j.1365-201X.1971.tb11001.x
van Praag, H., Christie, B. R., Sejnowski, T. J., and Gage, F. H. (1999). Running enhances neurogenesis, learning, and long-term potentiation in mice. Proc. Natl. Acad. Sci. U.S.A. 96, 13427–13431. doi: 10.1073/pnas.96.23.13427
van Praag, H., Shubert, T., Zhao, C., and Gage, F. H. (2005). Exercise enhances learning and hippocampal neurogenesis in aged mice. J. Neurosci. 25, 8680–8685. doi: 10.1523/JNEUROSCI.1731-05.2005
VanLeeuwen, J.-E., Petzinger, G. M., Walsh, J. P., Akopian, G. K., Vuckovic, M., and Jakowec, M. W. (2010). Altered AMPA receptor expression with treadmill exercise in the 1-methyl-4-phenyl-1,2,3,6-tetrahydropyridine-lesioned mouse model of basal ganglia injury. J. Neurosci. Res. 88, 650–668. doi: 10.1002/jnr.22216
Vigers, A. J., Amin, D. S., Talley-Farnham, T., Gorski, J. A., Xu, B., and Jones, K. R. (2012). Sustained expression of brain-derived neurotrophic factor is required for maintenance of dendritic spines and normal behavior. Neuroscience 212, 1–18. doi: 10.1016/j.neuroscience.2012.03.031
Villalba, R. M., and Smith, Y. (2011). Differential structural plasticity of corticostriatal and thalamostriatal axo-spinous synapses in MPTP-treated Parkinsonian monkeys. J. Comp. Neurol. 519, 989–1005. doi: 10.1002/cne.22563
Villalba, R. M., Mathai, A., and Smith, Y. (2015). Morphological changes of glutamatergic synapses in animal models of Parkinson's disease. Front. Neuroanat. 9:117. doi: 10.3389/fnana.2015.00117
Vina, J., Sanchis-Gomar, F., Martinez-Bello, V., and Gomez-Cabrera, M. C. (2012). Exercise acts as a drug; the pharmacological benefits of exercise. Br. J. Pharmacol. 167, 1–12. doi: 10.1111/j.1476-5381.2012.01970.x
Vingerhoets, F. J., Snow, B. J., Tetrud, J. W., Langston, J. W., Schulzer, M., and Calne, D. B. (1994). Positron emission tomographic evidence for progression of human MPTP-induced dopaminergic lesions. Ann. Neurol. 36, 765–770. doi: 10.1002/ana.410360513
Voss, M. W., Vivar, C., Kramer, A. F., and van Praag, H. (2013). Bridging animal and human models of exercise-induced brain plasticity. Trends Cogn. Sci. 17, 525–544. doi: 10.1016/j.tics.2013.08.001
Wang, Z., Guo, Y., Myers, K. G., Heintz, R., and Holschneider, D. P. (2015a). Recruitment of the prefrontal cortex and cerebellum in Parkinsonian rats following skilled aerobic exercise. Neurobiol. Dis. 77, 71–87. doi: 10.1016/j.nbd.2015.02.020
Wang, Z., Guo, Y., Myers, K. G., Heintz, R., Peng, Y. H., Maarek, J. M., et al. (2015b). Exercise alters resting-state functional connectivity of motor circuits in parkinsonian rats. Neurobiol. Aging 36, 536–544. doi: 10.1016/j.neurobiolaging.2014.08.016
Wei, W., Ding, S., and Zhou, F. M. (2017). Dopaminergic treatment weakens medium spiny neuron collateral inhibition in the parkinsonian striatum. J. Neurophysiol. 117, 987–999. doi: 10.1152/jn.00683.2016
Whitehead, G., Regan, P., Whitcomb, D. J., and Cho, K. (2017). Ca2+-permeable AMPA receptor: a new perspective on amyloid-beta mediated pathophysiology of Alzheimer's disease. Neuropharmacology 112, 221–227. doi: 10.1016/j.neuropharm.2016.08.022
Willard, A. M., Bouchard, R. S., and Gittis, A. H. (2015). Differential degradation of motor deficits during gradual dopamine depletion with 6-hydroxydopamine in mice. Neuroscience 301, 254–267. doi: 10.1016/j.neuroscience.2015.05.068
Wilson, C. J., and Groves, P. M. (1980). Fine structure and synaptic connections of the common spiny neuron of the rat neostriatum: a study employing intracellular inject of horseradish peroxidase. J. Comp. Neurol. 194, 599–615. doi: 10.1002/cne.901940308
Wilson, C. J., and Kawaguchi, Y. (1996). The origins of two-state spontaneous membrane potential fluctuations of neostriatal spiny neurons. J. Neurosci. 16, 2397–2410.
Xenias, H. S., Ibáñez-Sandoval, O., Koós, T., and Tepper, J. M. (2015). Are striatal tyrosine hydroxylase interneurons dopaminergic? J. Neurosci. 35, 6584–6599. doi: 10.1523/JNEUROSCI.0195-15.2015
Xu, B., Zang, K., Ruff, N. L., Zhang, Y. A., McConnell, S. K., Stryker, M. P., et al. (2000). Cortical degeneration in the absence of neurotrophin signaling: dendritic retraction and neuronal loss after removal of the receptor TrkB. Neuron 26, 233–245. doi: 10.1016/S0896-6273(00)81153-8
Xu, Q., Park, Y., Huang, X., Hollenbeck, A., Blair, A., Schatzkin, A., et al. (2010). Physical activities and future risk of Parkinson disease. Neurology 75, 341–348. doi: 10.1212/WNL.0b013e3181ea1597
Yan, Q., Matheson, C., and Lopez, O. T. (1995). In vivo neurotrophic effects of GDNF on neonatal and adult facial motor neurons. Nature 373, 341–344. doi: 10.1038/373341a0
Yang, F., Trolle Lagerros, Y., Bellocco, R., Adami, H. O., Fang, F., Pedersen, N. L., et al. (2015). Physical activity and risk of Parkinson's disease in the Swedish National March Cohort. Brain 138, 269–275. doi: 10.1093/brain/awu323
Yoon, M. C., Shin, M. S., Kim, T. S., Kim, B. K., Ko, I. G., Sung, Y. H., et al. (2007). Treadmill exercise suppresses nigrostriatal dopaminergic neuronal loss in 6-hydroxydopamine-induced Parkinson's rats. Neurosci. Lett. 423, 12–17. doi: 10.1016/j.neulet.2007.06.031
Yung, K. K., Bolam, J. P., Smith, A. D., Hersch, S. M., Ciliax, B. J., and Levey, A. I. (1995). Immunocytochemical localization of D1 and D2 dopamine receptors in the basal ganglia of the rat: light and electron microscopy. Neuroscience 65, 709–730. doi: 10.1016/0306-4522(94)00536-E
Zagrebelsky, M., and Korte, M. (2014). Form follows function: BDNF and its involvement in sculpting the function and structure of synapses. Neuropharmacology 76(Pt C), 628–638. doi: 10.1016/j.neuropharm.2013.05.029
Zaja-Milatovic, S., Milatovic, D., Schantz, A. M., Zhang, J., Montine, K. S., Samii, A., et al. (2005). Dendritic degeneration in neostriatal medium spiny neurons in Parkinson disease. Neurology 64, 545–547. doi: 10.1212/01.WNL.0000150591.33787.A4
Zhang, Z. X., Roman, G. C., Hong, Z., Wu, C. B., Qu, Q. M., Huang, J. B., et al. (2005). Parkinson's disease in China: prevalence in Beijing, Xian, and Shanghai. Lancet 365, 595–597. doi: 10.1016/S0140-6736(05)70801-1
Zhou, F. M. (2017). “The substantia nigra pars reticulata,” in Handbook of Basal Ganglia Structure and Function, 2nd Edn., eds H. Steiner and K. Tseng (New York, NY: Elsevier), 293–316.
Zhou, F. M., Wilson, C., and Dani, J. A. (2003). Muscarinic and nicotinic cholinergic mechanisms in the mesostriatal dopamine systems. Neuroscientist 9, 23–36. doi: 10.1177/1073858402239588
Zhou, Q. Y., and Palmiter, R. D. (1995). Dopamine-deficient mice are severely hypoactive, adipsic, and aphagic. Cell 83, 1197–1209. doi: 10.1016/0092-8674(95)90145-0
Zigmond, M. J., Cameron, J. L., Leak, R. K., Mirnics, K., Russell, V. A., Smeyne, R. J., et al. (2009). Triggering endogenous neuroprotective processes through exercise in models of dopamine deficiency. Parkinsonism Relat. Disord. 15(Suppl. 3), S42–S45. doi: 10.1016/S1353-8020(09)70778-3
Zoladz, J. A., Majerczak, J., Zeligowska, E., Mencel, J., Jaskolski, A., Jaskolska, A., et al. (2014). Moderate-intensity interval training increases serum brain-derived neurotrophic factor level and decreases inflammation in Parkinson's disease patients. J. Physiol. Pharmacol. 65, 441–448.
Keywords: basal ganglia, dendritic spine, dopamine, glutamate, medium spiny neuron, neuroprotection, neurotrophic factor, physical activity
Citation: Hou L, Chen W, Liu X, Qiao D and Zhou F-M (2017) Exercise-Induced Neuroprotection of the Nigrostriatal Dopamine System in Parkinson's Disease. Front. Aging Neurosci. 9:358. doi: 10.3389/fnagi.2017.00358
Received: 25 July 2017; Accepted: 19 October 2017;
Published: 03 November 2017.
Edited by:
Hanting Zhang, West Virginia University, United StatesReviewed by:
Martin Darvas, University of Washington, United StatesJavier Blesa, Centro Integral en Neurociencias A.C. HM CINAC, Spain
Copyright © 2017 Hou, Chen, Liu, Qiao and Zhou. This is an open-access article distributed under the terms of the Creative Commons Attribution License (CC BY). The use, distribution or reproduction in other forums is permitted, provided the original author(s) or licensor are credited and that the original publication in this journal is cited, in accordance with accepted academic practice. No use, distribution or reproduction is permitted which does not comply with these terms.
*Correspondence: Decai Qiao, ZGVjYWlxQGJudS5lZHUuY24=
Fu-Ming Zhou, Znpob3UzQHV0aHNjLmVkdQ==
†These authors have contributed equally to this work.