- 1Department of Neurosurgery, Tongji Hospital, Tongji Medical College, Huazhong University of Science and Technology, Wuhan, Hubei, China
- 2Department of Neurology, Tongji Hospital, Tongji Medical College, Huazhong University of Science and Technology, Wuhan, Hubei, China
As a crucial component of the cerebral cholinergic system and the Papez circuit in the basal forebrain, dysfunction of the nucleus basalis of Meynert (NBM) is associated with various neurodegenerative disorders. However, no drugs, including existing cholinesterase inhibitors, have been shown to reverse this dysfunction. Due to advancements in neuromodulation technology, researchers are exploring the use of deep brain stimulation (DBS) therapy targeting the NBM (NBM-DBS) to treat mental and neurological disorders as well as the related mechanisms. Herein, we provided an update on the research progress on cognition-related neural network oscillations and complex anatomical and projective relationships between the NBM and other cognitive structures and circuits. Furthermore, we reviewed previous animal studies of NBM lesions, NBM-DBS models, and clinical case studies to summarize the important functions of the NBM in neuromodulation. In addition to elucidating the mechanism of the NBM neural network, future research should focus on to other types of neurons in the NBM, despite the fact that cholinergic neurons are still the key target for cell type-specific activation by DBS.
1 Introduction
As the largest group of cholinergic neurons in the basal forebrain, the nucleus basalis of Meynert (NBM) is a crucial source of cholinergic efferents to the neocortex and plays an indispensable role in supporting vital brain functions, such as arousal, attention, multimodal encoding, visual processing, and experience-dependent cortical plasticity (Goard and Dan, 2009; Sanchez-Alavez et al., 2014; Liu et al., 2015; Ljubojevic et al., 2018; Martinez-Rubio et al., 2018; Meir et al., 2018; Wan et al., 2019). NBM dysfunction is implicated in multiple neuropsychiatric and neurological disorders, including Alzheimer’s disease (AD), schizophrenia, Parkinson’s disease (PD), Lewy body dementia (LBD), and Down syndrome (Williams et al., 2013; González et al., 2021; Mehraram et al., 2022; Schumacher et al., 2022). The involvement of the NBM may be a critical prodrome or an early molecular cascade event. For instance, research has demonstrated that in AD, the earliest accumulation of neurofibrillary tangles (NFTs) occurs in the NBM, preceding the accumulation in the entorhinal cortex and locus coeruleus (Braak et al., 2011; Arendt et al., 2015; Chung et al., 2016; Hanna et al., 2020). Drugs that regulate the activity of cholinergic neurons in the NBM and its cortical efferent have been proposed as promising therapeutic approaches for various neurodegenerative diseases. However, current drugs targeting this pathway, including existing cholinesterase inhibitors, have limited efficacy and cannot halt or reverse neurodegenerative diseases (Yu et al., 2021). Based on advancements in neuromodulation technology, deep brain stimulation (DBS) has been proven to be the most effective strategy for treating neurodegenerative diseases due to its precise circuit-targeted neuromodulation (Krauss et al., 2021). Therefore, DBS has been approved by the Food and Drug Administration (FDA) for treating PD, essential tremor, dystonia and other movement disorders, and it has yielded satisfactory clinical outcomes and evidence (Krauss et al., 2021). In addition, DBS has been used to treat pain syndromes, such as neuropathic pain and cluster headache, as well as epilepsy (Pereira and Aziz, 2014; Lee et al., 2019). Based on the key role of the NBM in cognition, many published studies have focused on the effect of cognitive neuromodulation via DBS targeting the NBM (NBM-DBS) in various diseases (Kuhn et al., 2015; Gratwicke et al., 2018, 2020; Cappon et al., 2022; Sasikumar et al., 2022). A phase I clinical trial conducted by Kuhn et al. (2015) investigated the effects of NBM-DBS on the treatment of AD, revealing a mere 3-point deceleration in the rate of progression according to the Alzheimer’s Disease Assessment Scale-Cognitive Subscale (ADAS-Cog) and an increase in cerebral cortical glucose metabolism revealed by [18F]-fluoro-desoxyglucose-PET at a 12-month follow-up. Davide Cappon et al. explored the effects of NBM-DBS in five Parkinson’s disease dementia (PDD) patients, two of whom experienced slower cognitive decline; however, the Mini-Mental State Examination (MMSE) score still exhibited an annual mean decrease of 0.3 points at the 36-month follow-up (Cappon et al., 2022). However, studies from Sasikumar S and Gratwicke J found that NBM-DBS did not improve cognitive function in PDD patients (Gratwicke et al., 2018; Sasikumar et al., 2022). In addition, Gratwicke J found that although no consistent improvements were observed in exploratory clinical outcome measures for NBM-DBS, but the severity of neuropsychiatric symptoms reduced with NBM-DBS in 3 of 5 dementia with Lewy bodies (DLB) patients (Gratwicke et al., 2018).
The mechanisms of NBM-DBS have also been investigated recently in animal models of AD, and a variety of hypotheses have been proposed, such as regulating the cholinergic system (Hotta et al., 2009), modulating regional glucose metabolism (Wang et al., 2018), increasing regional cerebral blood flow (Adachi et al., 1990a,b), providing neuroprotective effects (Temel et al., 2006; Wallace et al., 2007) and regulating neural circuits (Singh et al., 2022). However, the significant treatment effects of NBM-DBS on cognitive dysfunction that have been observed in animal studies have not been replicated in clinical trials. Therefore, NBM-DBS is still in the preliminary stage of exploration.
The current review aims to provide a comprehensive update and summary of progress on cognition-related neural networks or circuits as well as the neuroanatomical and neurophysiological properties of the NBM. Additionally, this study aims to provide evidence from animal and clinical studies regarding the effect of NBM-DBS on cognitive dysfunction in various neurological diseases. The findings of this study will provide a basis for obtaining a precise understanding of the important and promising role of the NBM in neuromodulation.
2 Neural network oscillations related to cognition
Rhythmic neural electrical activity, also known as oscillations, has attracted research attention for nearly a century. Behavior-dependent oscillations are the result of dynamic interactions between intrinsic cellular and circuit properties within the brain and are ubiquitous in mammals (Berger, 1929; Berger, 1969; Llinas, 1988; Steriade, 2001; Laurent, 2002; Destexhe and Sejnowski, 2003). The neural network contains multiple frequency bands of oscillations, ranging from 0.05 Hz to 500 Hz, which are categorized as slow oscillations (<1.5 Hz), δ oscillations (1.5–4 Hz), θ oscillations (4–8 Hz), α oscillations (8–13 Hz), β oscillations (13–30 Hz), γ oscillations (30–80 Hz) and fast oscillations (>100 Hz) based on frequency (Jasper and Penfield, 1949; Nokia and Penttonen, 2022). Oscillations are currently thought to be shaped by the summations of thousands of neurons in a particular brain region (Engel et al., 2001; Varela et al., 2001). In normal cognitive processes, each oscillatory frequency band is associated with a specific behavioral or executive function. Studies have shown that the δ oscillation phase correlates with the reaction time of behavior and plays a role in neural group synchronization in multiple brain regions (Stefanics et al., 2010). In addition, these oscillations are often associated with resting states, and slow oscillations (<1 Hz) can trigger thalamically generated spindles (Amzica and Steriade, 1998). θ oscillations are believed to play a crucial role in almost all cognitive functions. Previous studies have revealed the key roles of these proteins in learning, memory and synaptic plasticity (Herweg et al., 2020; Senoussi et al., 2022). Additionally, it is common for pyramidal neurons to fire phase locking with θ oscillations, indicating their key roles in interconnecting various brain regions (Pare and Gaudreau, 1996; Buzsaki, 2002; Jacobs et al., 2007; Rutishauser et al., 2010). θ oscillations are also the fundamental bands for cross-frequency coupling (CFC), as they synchronizing with the gamma band in a phenomenon known as θ–γ cross-frequency coupling; the role of θ–γ cross-frequency coupling in working memory has been extensively studied (Lisman and Jensen, 2013). α oscillations are also the most widely understood frequency bands, mostly due to their well-defined roles in attention needs and visual perception (Başar et al., 1997; Klimesch, 2012). β oscillations are often associated with motor-induced events (Pittman-Polletta et al., 2018). Synchronization of β oscillations has always been an indicator of normal motor system function and has also been shown to play a role in working memory (Schmidt et al., 2019). Finally, γ oscillations also play a crucial role in memory, as observed in the θ-γ coupled oscillations throughout the hippocampus and cortex (Lisman and Buzsaki, 2008).
Although each frequency band appears to be separated into a variety of behavioral or cognitive functions, synchronization between different bands through CFC analysis has provided new insights into the complexity of neural networks (Palva and Palva, 2007). CFCs are thought to be integral to the spatiotemporal activation of specific cortical circuits (Canolty and Knight et al., 2010; Yakubov et al., 2022). For example, phenomena such as the θ-γ phase-amplitude (p-a) CFC – which refers to the γ amplitude modulated by the θ phase (Bragin et al., 1995; Montgomery et al., 2008; Colgin et al., 2009; Sakalar et al., 2022) and is known for its crucial role in working memory and memory formation in the hippocampal CA1 region – are among the many modes of CFCs (Lisman and Jensen, 2013). By optogenetic selectively targeting fast-spiking GABAergic interneurons in the barrel cortex in vivo, Cardin JA et al. tested whether the synchronous activity of fast-spiking GABAergic interneurons generates γ oscillations, which produce rhythmic inhibitory postsynaptic potentials (IPSPs) to distal dendrites of local pyramidal neurons; their findings showed that the rhythmic inhibition produced excitatory neural ensemble synchrony to process information (Cardin et al., 2009). Sakalar E et al. found that neurogliaform cells (NGFCs), which form synaptic connections with excitatory neuronal ensembles, lead to delayed synchronous firing; moreover, this delay leads to GABAergic inhibition to excitatory neural ensembles when information processing is complete, thereby resulting in actively disengaged excitatory neural ensemble synchronization that enables the formation of new patterns of information processing (Sakalar et al., 2022). These findings represent compelling examples of the significance of cell type-specific activation in rhythmic neural electrical activity and provide a reference for research on the mechanism underlying neural networks. Based on these studies, we constructed a schematic diagram of how θ-γ p-a CFC regulates neuronal firing patterns and cognitive processes (Figure 1).
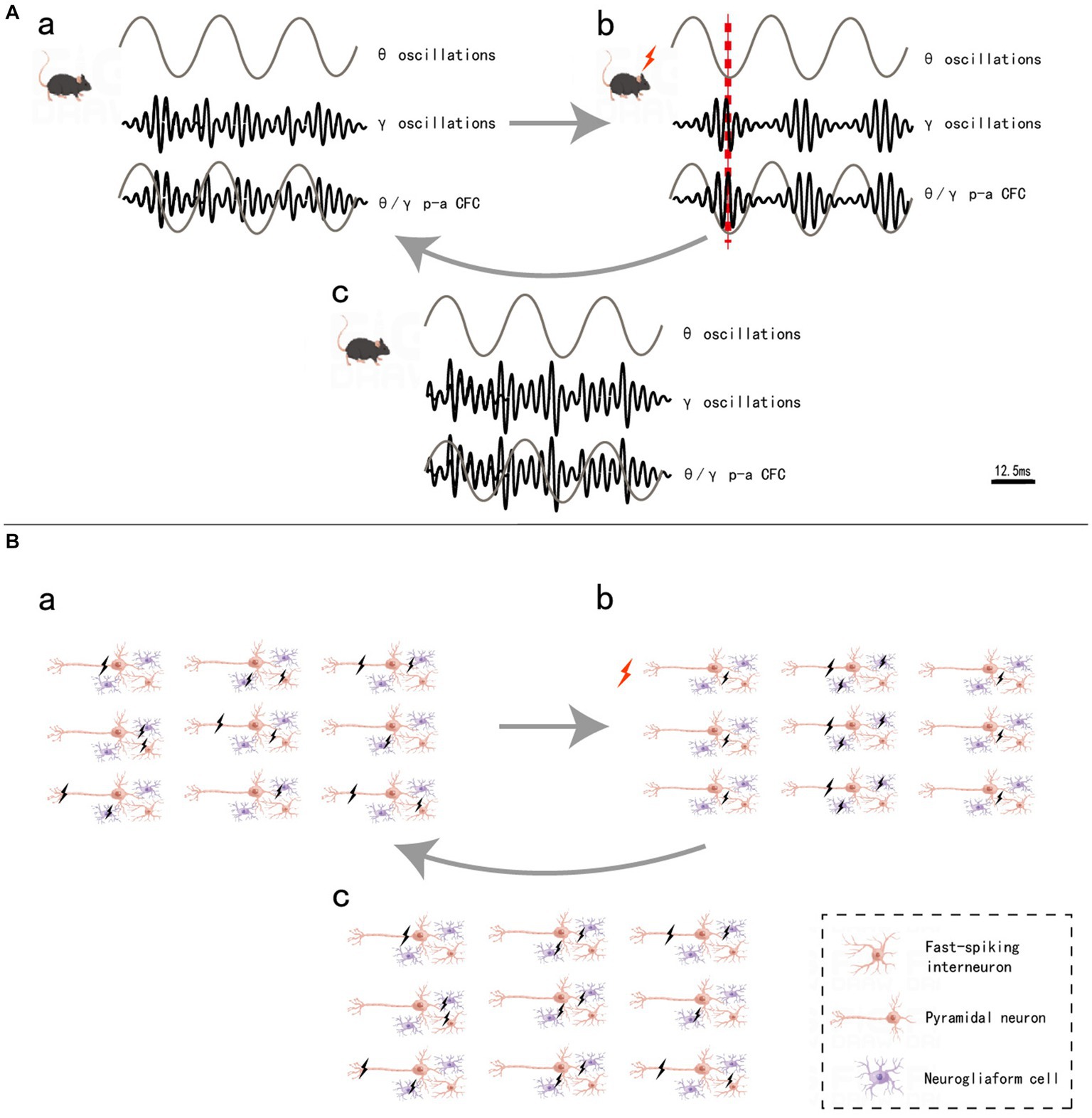
Figure 1. θ/γ p-a CFC regulation (A) and neuronal firing pattern (B). a, in mice without stimulation, θ oscillations did not form coupling with γ oscillations (A), and the firing of the three types of neurons was irregular (B). b, when mice are stimulated, the fast-spiking interneurons synchronous firing (IPSP) causes γ oscillations, θ oscillations and γ oscillations, thus leading to θ-γ p-a CFC (A). The pyramidal neurons, which are inhibited by GABA released by interneurons, do not fire, while the uninhibited pyramidal neurons form an ensemble and fire synchronously, completing the information encoding. Additionally, neurogliaform cells that form synaptic connections with this pyramidal neuronal ensemble also form delayed synchronous firing (B). c, after a very short interval when the information processing is done, the delayed synchronous firing from neurogliaform cells cause the release of GABA from the synaptic, which inhibits the pyramidal neuronal ensemble and stops fire (B), and decouples of the θ-γ p-a CFC and return to state awaiting to receive new information (A). Black lightning bolt, neuronal firing, red lightning bolt and red dashed line, the moment when mice are stimulated.
The cholinergic system plays a key role in controlling neural oscillations throughout the brain and cortical structures (Semba and Fibiger, 1989). Cholinergic neurons suppressed specific low-frequency oscillations, including δ, θ, and α oscillations, while high-frequency oscillations, such as β and γ oscillations, were accompanied by increased acetylcholine release in the thalamus and cortex (Steriade, 2004). Furthermore, cholinergic neurons appear to play a crucial role in influencing θ oscillations in the hippocampus. Acetylcholine (ACh) levels are directly related to θ oscillations in hippocampal neurons and have a direct effect on the amplitude of θ waves (Danışman et al., 2022). θ-γ p-a CFC also appears to rely heavily on ACh modulation, with detected cues evoking phasic ACh release as well as neuronal synchrony across several frequency bands and the emergence of θ-γ coupling (Howe et al., 2017). It is reasonable to assume that the NBM, as the largest group of cholinergic neurons in the basal forebrain, plays an independent role in the regulation of nerve concussion. Although the NBM acetylcholine system has an important influence on neural network oscillations, as shown by Rodriguez R et al., the cholinergic system may also regulate neural network oscillations through muscarinic receptors in addition to nicotinic receptors (Rodriguez et al., 2004), indicating that other types of NBM neurons or receptor components also play an important role in the generation and regulation of neural network oscillations. There are few relevant studies, which need to be explored in the future.
3 Anatomy and electrophysiology properties of the NBM
3.1 Anatomy of the NBM
The basal forebrain is one of the four regions containing cholinergic neurons in the mammalian brain (the others being the brainstem, striatum, and limbic system). This region is anatomically situated above the optic nerve, below the anterior commissure and medially adjacent to the lateral ventricular wall, and it includes the medial septal nucleus (MS), the diagonal band of the Broca nucleus (DBB) (with vertical and horizontal branches), the preoptic nucleus, the basal ganglia (NB) and the anonymous substance (SI) (Woolf, 1991). Mesulam et al. investigated acetyl cholinergic neurons in nonhuman primate brain tissue and introduced the Ch1-Ch4 nomenclature to classify four distinct cholinergic neuron groups located in the basal forebrain. Ch1 corresponds to the MS; many of its neurons are embedded among the fibers of the precommissural fornix, and approximately 10% of its neurons are cholinergic, thus providing a substantial projection to the hippocampus (Figure 2). Ch2 corresponds to the vertical limb of DBB; at least 70% of its neurons are cholinergic, and they merge with the Ch1 cell group dorsally and with Ch3 and Ch4 ventrally. Furthermore, Ch2 is the major source of innervation that the hippocampus and hypothalamus receive from the basal forebrain (Figure 3). The Ch3 group most closely corresponds to the horizontal limb of the DBB. It extends from the septal-preoptic region medially to the amygdaloid region laterally. Only 1% of its neurons can definitely be proven to be cholinergic; furthermore, this group is the major source of basal forebrain projections to the olfactory bulb (Figure 4) (Mesulam et al., 1983, 1984).
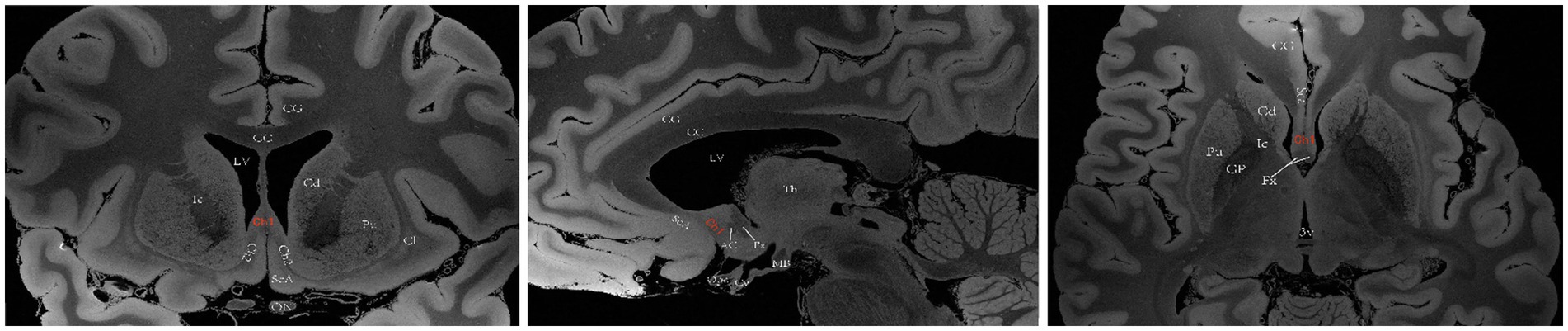
Figure 2. A diagram depicting the location of the human Ch1 on MRI. Images sourced from https://openneuro.org/datasets/ds002179/versions/1.1.0 (Marceglia et al., 2021). Ch1 represents the cholinergic component of MS. Coronal (left), sagittal (middle), axial (right). LV lateral ventricle, CG cingulate gyrus, 3 V third ventricle, Fx Fornix, Th thalamus, Cd caudatum, Cl claustrum, Gp globus pallidus, Pu putamen, Ic Internal capsule, ON optic nerve, Opc optic chiasma, CC corpus callosum, ScA subcallosal area, AC anterior commissure, MB mamillary body, Cy cyathus.
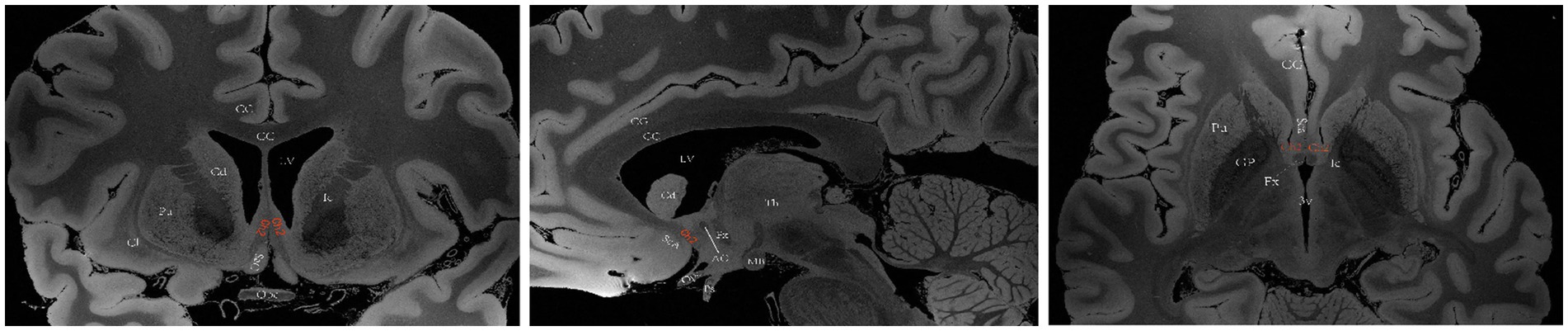
Figure 3. A diagram depicting the location of the human Ch2 on MRI. Images sourced from https://openneuro.org/datasets/ds002179/versions/1.1.0. (Marceglia et al., 2021) Ch2 corresponds to the cholinergic component of the vertical branch of DBB. Coronal (left), sagittal (middle), axial (right). LV lateral ventricle, CG cingulate gyrus, 3 V third ventricle, Fx Fornix, Th thalamus, Cd caudatum, Cl claustrum, Gp globus pallidus, Pu putamen, Ic Internal capsule, Opc optic chiasma, CC corpus callosum, ScA subcallosal area, AC anterior commissure, MB mamillary body, Ps pituitary stalk.
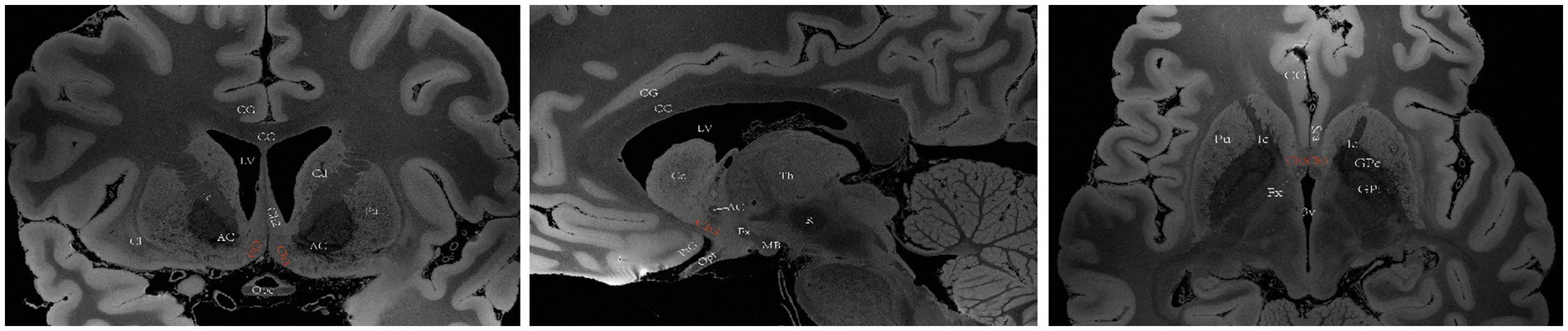
Figure 4. A diagram depicting the location of the human Ch3 on MRI. Images sourced from https://openneuro.org/datasets/ds002179/versions/1.1.0.33 (Marceglia et al., 2021). Ch3 refers to the cholinergic component of the horizontal branch of DBB. Coronal (left), sagittal (middle), axial (right). LV lateral ventricle, CG cingulate gyrus, 3 V third ventricle, Fx Fornix, Th thalamus, Cd caudatum, Cl claustrum, GPi internal globus pallidus, GPe external globus pallidus, Pu putamen, Ic Internal capsule, Opt optic tract, Opc optic chiasma, PtG paraterminal gyrus, CC corpus callosum, ScA subcallosal area, AC anterior commissure, MB mamillary body, R red nucleus.
Ch4 encompasses a complex network of basal nuclear giant cells, including the substantia innominate (SI), NBM, preoptic nucleus, and ventral pallidum. Ch4 is further divided into five subregions: the anterior portion (Ch4a), which is subdivided into anteromedial (Ch4am) and anterolateral (Ch4al) regions; the middle portion (Ch4i), which is subdivided into dorsal middle (Ch4id) and ventral middle (Ch4iv) regions; and the posterior portion (Ch4p). (Mesulam et al., 1983) In humans, the Ch4 nucleus is situated in the posterior region of the basal forebrain and measures approximately 13–14 mm in length and 16–18 mm in width. However, a distinct subregion known as Ch4ai exists between Ch4a and Ch4i within the human brain; this subregion may have emerged due to an increased demand for cholinergic neurons and projection fibers to accommodate the expanded lateral surface area of the neocortex during the evolution of primates into Homo sapiens (Mesulam and Geula, 1988). Liu et al. (2015) proposed a conceptual definition for the anterior, intermediate and posterior subsectors of the human Ch4 to simplify its original classification. The anterior division of Ch4 (Ch4a) is situated laterally to the supraoptic nucleus and ventrally to the anterior commissure. The intermediate division (Ch4i) is located in the inferior part of the globus pallidus, medially adjacent to the lateral end of the anterior commissure. At this level, the intermedullary lamina divides the globus pallidus into internal and external components, with the lateral end of the anterior commissure located ventral to the putamen and sometimes the infundibulum visible. The posterior division (Ch4p) is anatomically connected to the globus pallidus medially and superiorly, the putamen laterally and superiorly, and the amygdala inferiorly; furthermore, Ch4p passes through the optic tract medially (Figure 5) (Liu et al., 2015).
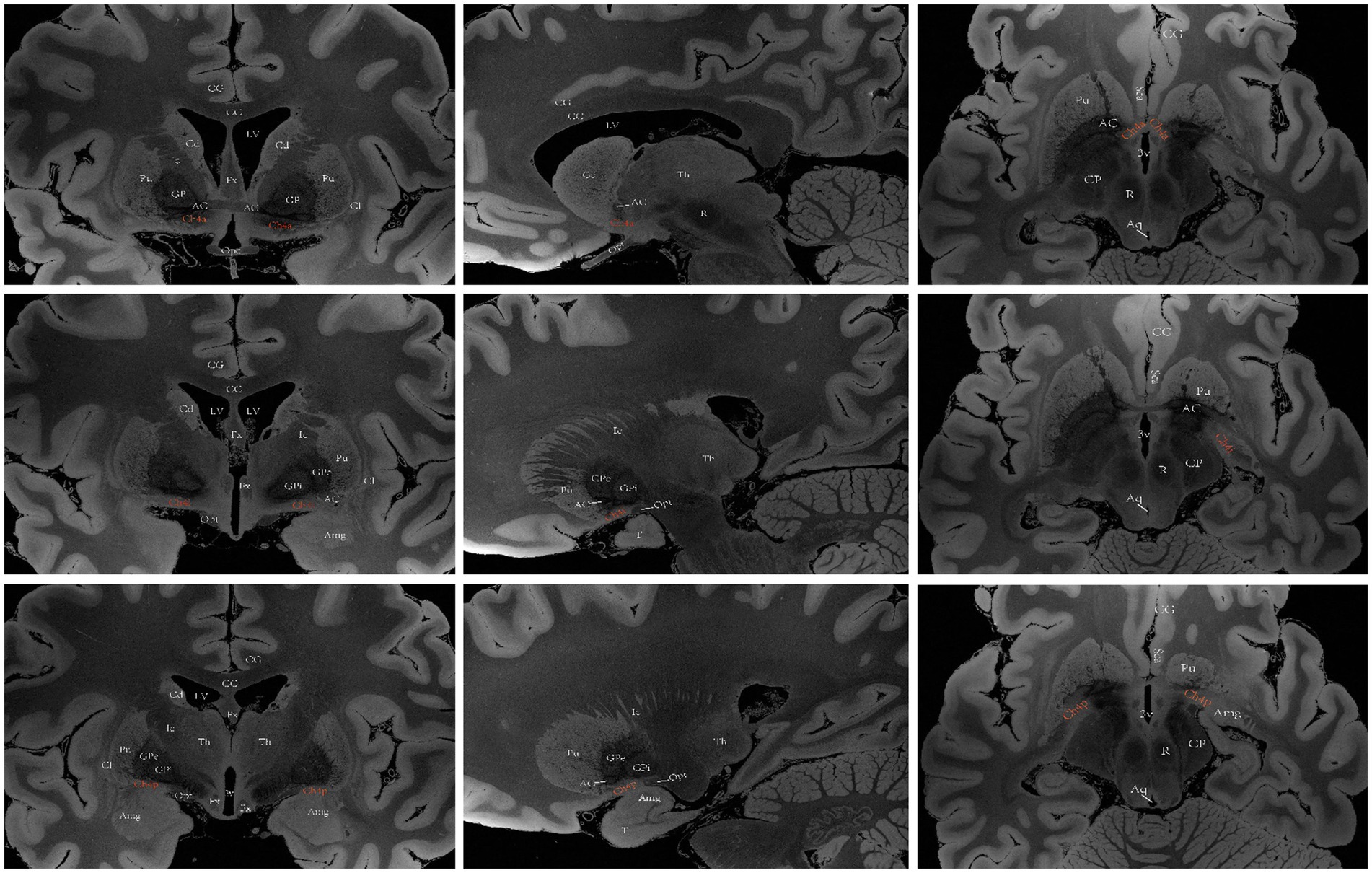
Figure 5. A diagram depicting the location of the human Ch4 on MRI. Images sourced from https://openneuro.org/datasets/ds002179/versions/1.1.0.33 (Marceglia et al., 2021). Coronal (left), sagittal (middle), axial (right). Ch4a (top), Ch4i (middle), Ch4p (bottom). LV lateral ventricle, CG cingulate gyrus, 3 V third ventricle, Fx Fornix, T temporal lobe, Th thalamus, Cd caudatum, Cl claustrum, Gp globus pallidus, GPi internal globus pallidus, GPe external globus pallidus, Pu putamen, Ic Internal capsule, Opt optic tract, Opc optic chiasma, CC corpus callosum, ScA subcallosal area, AC anterior commissure, Amg Amygdala, CP cerebral peduncle, Aq aqueduct of midbrain, R red nucleus.
Mesulam MM et al. utilized HRP retrograde tracer and acetylcholinesterase (AChE) colocalization technology to effectively demonstrate that cortical topographical innervations originate from distinct subregions of CH4 in the macaque brain. In summary, Ch4a cells innervate limbic regions. Specifically, Ch4am projects to medial cortical regions such as the cingulate cortex, while Ch4al targets fronto-parietal opercular regions and the amygdala. Additionally, Ch4p is responsible for superior temporal and temporal polar regions, while Ch4i covers all remaining cortical areas (Mesulam et al., 1983). By examining human cadaveric brain tissue sections and performing diffusion tensor imaging (DTI) studies, it was discovered that cholinergic efferent fibers originating from the NBM converged into medial and lateral bundles before diffusing throughout the target cortex. The medial bundle fibers converge toward the rostrum of the corpus callosum, penetrate into the cingulate gyrus, and extend posteriorly to reach the splenium and retrosplenial white matter. These axons emanate from the bundle to innervate various regions, including the medial orbitofrontal cortex, subcallosal cortex, cingulate cortex, pericingulate cortex and retrosplenial cortex. The lateral bundle comprises two additional divisions. The capsule division traverses the external capsule and projects fibers to the amygdala and temporal cortex, while the perisylvian division initially enters white matter surrounding the claustrum before radiating laterally to innervate the frontoparietal cortex, superior temporal gyrus, and insula (Selden et al., 1998; Hong and Jang, 2010).
Notably, not all magnocellular neurons in the basal forebrain are cholinergic, and basal forebrain cholinergic neurons are interspersed with noncholinergic neurons and distributed across these nuclei. Therefore, the terms NBM and Ch4 cannot be used interchangeably. Additionally, NBM afferent fibers are primarily derived from the central amygdala, with additional nonsensory and nonmotor cortical input originating from the orbitofrontal cortex, anterior insular lobe, temporal pole, entorhinal cortex, and medial temporal lobe. Subcortical input is received from the diencephalon (midthalamic nucleus and lateral hypothalamus) as well as brainstem structures such as the tegmental area of the midbrain, pontine reticular formation, and nucleus tractus solitarius. These synaptic inputs to the NBM include cholinergic, catecholaminergic, and γ-aminobutyric acid (GABA) axons (Jones et al., 1976; Woolf and Butcher, 1982; Russchen et al., 1985; Kasa, 1986; Mesulam and Geula, 1988; Jones and Cuello, 1989; Smiley and Mesulam, 1999; Zheng et al., 2018). From an anatomical perspective, in addition to emitting cholinergic projection fibers to the neocortex, the NBM also forms bidirectional projections with numerous structures within the limbic system and Papez loops.
3.2 Electrophysiology properties of the NBM
Cholinergic neurons in the NBM possess a resting membrane potential of approximately −45 mV and are equipped with voltage-gated ion channels, including fast-activated and rapidly inactivated Na + channels as well as fast-activated and delayed rectification K+ channels (Song et al., 2013; Morelli et al., 2017). Additionally, these neurons are equipped with various ligand-gated ion channels, such as ACh, nicotinic receptors (Morelli et al., 2017), muscarinic receptors (Khateb et al., 1993), epinephrine α1 and β receptors (Fort et al., 1995), histamine H1 and H2 receptors (Khateb et al., 1995; Knoche et al., 2003; Luo and Leung, 2009) and GABA receptors (Khateb et al., 1998; Manfridi et al., 2001). These properties enable the NBM to respond excitably or inhibitably to various neuromodulators, including ACh, nerve growth factor (NGF), estrogen, GABA and dopamine. Cholinergic neurons in the NBM account for approximately 5% of all neurons in this region and exhibit high-frequency pulse bursts that have been shown to be correlated with cortical activation, including cortical γ and θ oscillations; these bursts occur during both awake (AW) and paradoxical sleep (PS) states but are almost completely absent during slow-wave sleep (SWS) (Lee et al., 2005). Spencer JP et al. examined the induction of γoscillations in rat hippocampal slices by AChE inhibitors in vitro (Spencer et al., 2010). Chernyshev BV et al. discovered a significant negative correlation between cortical δ oscillation power and the spontaneous firing rate of cholinergic neurons in the NBM by simultaneously recording basal forebrain cholinergic cell firing rates and frontal lobe EEG in spontaneous behavior rabbits; the results indicated that cortical δ oscillation power can serve as an indicator of the activity of cholinergic neurons in the NBM (Chernyshev et al., 2004). These studies demonstrated the indispensable role of ACh pathways in maintaining normal neural network function.
4 The role of the NBM in neural network activation and modulation
4.1 Evidence from NBM injury animal models
An increasing number of researchers have focused on alterations in neural network oscillations in numerous animal models of NBM injury (Table 1). These findings indicated that NBM lesions caused some consistent changes in neural network oscillations: (1) an increase in the total power of electroencephalography (EEG), particularly in low-frequency bands (δ and θ); (2) a significant decrease in high-frequency oscillations, especially in the γ band; and (3) a reduction in event-related oscillation (ERO) phase-locking index (PLI) (Berntson et al., 2002; Rispoli et al., 2004, 2008, 2013; Sanchez-Alavez et al., 2014; Sanchez-Alavez and Ehlers, 2016). Despite these consistent changes, Rispoli et al. (2013) performed a quantitative EEG (qEEG) analysis of θ oscillations in the hippocampus and the entire EEG spectrum in the cerebral cortexes of rats with bilateral NBM lesions induced by 2-amino-3-(3 hydroxy-5-methylisoxazol-4-yl)-propionic acid (AMPA); the results showed increased power of θ oscillations in the frontal parietal cortex but not in the hippocampus, indicating that the EEG effect of NBM lesions might depend on brain regions. Ljubojevic et al. (2018) reported that the accuracy of visual and olfactory target detection was lower in rats with NBM lesions induced by the selective cholinergic immunotoxin 192 IgG-saporin than in healthy controls; furthermore, the detection accuracy was correlated with decreased local field potential (LFP) coherence in the β range between the prefrontal and posterior parietal cortex and with decreased β power in the posterior parietal cortex before the target’s appearance. Ciric et al. (2016) performed sleep EEG monitoring and found that δ-amplitude attenuation in the sensorimotor cortex during rapid eye movement sleep (REMS) was the earliest indication following bilateral NBM lesions induced by ibotenic acid (IBO) (Ciric et al., 2016). Similarly, Chernyshev et al. (2004) reported a significant negative correlation between cortical δ power and the spontaneous firing rate of cholinergic neurons in the NBM through simultaneous recordings of basal forebrain cholinergic cell firing rates and frontal lobe EEG signals in spontaneous behavior rabbits (Chernyshev et al., 2004). These two studies above indicating that cortical δ oscillation power can serve as an indicator of the activity of cholinergic neurons in the NBM, and future targeted studies are needed to verify this association and explain the underlying mechanisms. Rispoli et al. (2013) examined the use of Huperzine A, a natural selective AChE inhibitor; after this inhibitor was intraperitoneally injected into rats with NBM lesions, the EEG architecture was partially reversed, and desynchronization and reduced θ-power were observed in the frontal and parietal cortexes. Spencer et al. (2010) revealed that the use of AChE inhibitors induced γ oscillations in rat hippocampal slices in vitro. These two studies provide further evidence regarding the indispensable role of ACh pathways in the maintenance of normal neural network functioning within the hippocampus.
4.2 Neuromodulation effects of NBM-DBS in animal models
Due to the challenges of current drug therapy and the confirmed mechanism of neural network dysfunction in neurodegenerative diseases, there is an urgent need for research on neuromodulation strategies, especially NBM-DBS. Previous animal studies have provided evidence that NBM-DBS could improve cognitive function in AD models, for example, Liu et al. (2018) showed that NBM-DBS could improve performance in monkeys on a continuous performance task, Lee et al. (2016), Nagasaka et al. (2017), and Huang et al. (2019) demonstrated that NBM-DBS could improve spatial memory performance in AD mice, as assessed by Morris water maze. While these animal studies have focused on behavioral outcomes, cellular and molecular mechanisms, and neural network effects. Relatively consistent findings indicate that NBM-DBS leads to decreased power in θ and α oscillations but increased power in γ oscillations in the visual and auditory cortexes and prefrontal cortex (Table 2) (McLin et al., 2002, 2003; Weinberger et al., 2006; Goard and Dan, 2009; Singh et al., 2022). In addition, Dezawa et al. (2021) reported that NBM-DBS facilitated the release of ACh in the primary somatosensory cortex (S1) of rats with NBM lesions and partially reversed the increase in the peak LFP amplitude in S1 after forepaw stimulation. Goard M et al. performed NBM-DBS on healthy rats and recorded the LFP and neuron spike rate in the primary sensory cortex (V1); the findings revealed that (1) NBM-DBS induces notable changes in the power spectrum of the V1 LFP, including increased γ band power and decreased power at low frequencies (<10 Hz), and (2) NBM-DBS leads to prominent decorrelation among neurons and marked improvement in the reliability of neuronal responses to natural scenes by analyzing each single-neuron response to 30 repeats of a natural movie (Goard and Dan, 2009).
4.3 Evidence of the role of the NBM in neuromodulation from clinical cases
In recent years, many studies have performed qEEG analysis on clinical patients with diseases related to NBM lesions (Table 3). Wan et al. (2019) examined the role of NBM-visual cortex functional connectivity in α reactivity by analyzing simultaneous EEG-multimodal MRI data from cohorts of young and older adults; the results showed that the decrease in α reactivity in older adults was associated with a reduced volume of the fiber bundles connecting the NBM to the visual cortex quantified by leukoaraiosis volume. Similarly, patients with AD (Schumacher et al., 2020) and DLB (Rea et al., 2021) also exhibited decreased α reactivity, which was correlated with a reduced volume of NBM. Furthermore, Schumacher et al. (2021) found that, compared to healthy controls and MCI-AD patients, MCI-DLB patients generally displayed a slower EEG background due to shifts in power from the β and α bands to the preα and θ bands, which was also linked to decreased NBM volume. The evidence above suggests that general sluggishness of EEG signals, an increase in energy at slow frequencies, and a decrease in α reactivity are common characteristics in the process of both natural aging and neurodegenerative diseases and may be associated with lesions of the NBM and its projected fibers. However, the observed decrease in γ oscillation power in animal models with NBM lesions has not been replicated in humans. This may be due to the poor spatial resolution of EEG analysis in humans, which reduces the accuracy of local cortical function represented by γ oscillations compared to LFPs generally applied in animal models.
With the development of advanced techniques such as fMRI and LFP recording by microelectrodes in NBM-DBS, the NBM neural network can now be explored in greater depth in patients with neurodegenerative diseases. Oswal et al. analyzed the LFP of NBMs with DBS electrodes recorded from PDD (6 patients) and DLB (5 patients) patients treated with NBM-DBS, along with MEG and MRI tractography, to explore the intersection between the NBM neural network and fiber junction (Oswal et al., 2021). First, a β band network strongly related to the projection fiber from the supplementary motor area to the NBM was identified, indicating that the supplementary motor region might be the driver of NBM activity. Then, a δ/θ band network was further identified to be strongly related to the projection fiber from the NBM to the parahippocampal gyrus and lingual gyrus, which might play important roles in memory and visual function. Lee DJ et al. observed distinct neuronal discharge rates in internal globus pallidus (GPi) cells (92.6 ± 46.1 Hz), border cells (34 ± 21 Hz), and NBM cells (13 ± 10 Hz) by performing microelectrode recordings through the GPi and NBM in five PDD patients who received bilateral GPi/NBM-DBS. In addition, the oddball task significantly reduced the discharge rate of NBM cells (from 2.9 ± 0.9 to 2.0 ± 1.1 Hz, p < 0.05) (Lee et al., 2019). Nazmuddin M et al. recorded LFP in two PDD patients who underwent GPi-DBS with microelectrodes in the GPi and one or more distal contacts close to or inside the NBM and found that δ (1–4 Hz) oscillations were more prominently present in the NBM region than in its vicinity (Nazmuddin et al., 2018). Considering the negative correlation between δ oscillations and spontaneous discharge of cholinergic neurons in the NBM in an NBM lesion animal model (Chernyshev et al., 2004), the often-ignored δ oscillations can serve as a potential indicator of the specific effects and mechanisms of the NBM on neural network oscillations. In the phase I clinical trial of NBM-DBS for AD patients completed by Kuhn J et al., a positive effect was observed in slowing the decline in ADAS-Cog and increasing cortical glucose metabolism on FDG-PET. However, regarding neural network changes, only one patient exhibited significantly decreased power of α oscillations, and the expected increase in high-frequency oscillation power was not observed. (Kuhn et al., 2015) The possible reason is bias due to the small clinical sample size, while another possible reason that cannot be ignored is the complex relationship between neural network oscillations and different types of cholinergic receptors in the cortex. As shown in the study by Rodriguez R et al., cortical muscarinic receptors can also regulate neural networks (Rodriguez et al., 2004), so the effect of NBM-DBS must be affected simultaneously by the muscarinic and nicotine receptors, and the changes of cortical cholinergic receptor types are different in different neurodegenerative diseases (Graham et al., 2002).
4.4 Effects of the NBM in arousal, sleep, and epilepsy
Luo T et al. reversed the frontal EEG inhibition effect of isoflurane by stereotactically injecting histamine into the NBM of anesthetized rats under isoflurane, resulting in a shift from burst inhibition to δ activity, which can be blocked by NBM premicroinjection with an H1 receptor antagonist but not by an H2 receptor antagonist (Luo and Leung, 2009). Similarly, Marino J et al. discovered that cortical nitric oxide (NO) was elevated after basal forebrain electrical stimulation but could be inhibited by systemic nitric oxide synthase (NOS). Additionally, blocking the activity of NOS in the sensory cortex of anesthetized cats significantly weakened the ability of basal forebrain electrical stimulation to induce cortical activation, as evidenced by decreased low-frequency high-amplitude oscillatory activity and increased high-frequency low-amplitude activity (Marino and Cudeiro, 2003). Manfridi’s study on sleep EEG in rats revealed that the activation of GABAA and GABAB receptors in NBM neurons led to increased SWS time and decreased wake time and that only the activation of GABAA receptors led to decreased REMS time (Manfridi et al., 2001). Berdiev et al. (2007) discovered that in the WAG/Rij rat (a model of absence epilepsy), NBM lesions (induced by the selective cholinergic immunotoxin 192 IgG-saporin) increased frontal cortical synchronous activity characterized by the number of spike–wave discharges (SWDs), which were successfully inhibited by the NBM/reticular nucleus of the thalamus (RT)-DBS. Combined with the findings of above studies, NBM neurons are involved in modulating arousal, sleep and epilepsy -related neural networks (Table 4).
5 Future research prospects of the NBM for neuromodulation
Neuromodulation, represented by DBS and brain-computer interfaces (BCIs), is undoubtedly a promising therapeutic method that meets the urgent need for more effective therapies for a range of debilitating diseases and conditions, including inflammatory and autoimmune disorders, obesity, diabetes, cardiovascular disease, cancer, neurodegenerative and neuromuscular disorders and paralysis (Fetz, 2007; Jackson and Fetz, 2011; Orsborn and Carmena, 2013). The aforementioned findings collectively underscore the pivotal role of the NBM in the neural network, thereby emphasizing the need to investigate its function within the neural network by using advanced techniques such as optogenetics, multielectrode recording, and computer modeling to unravel the underlying mechanisms. Such research will serve as a fundamental basis for precise modulation via DBS and BCIs in the future (Wang, 2010; Bensmaia and Miller, 2014; Shenoy and Carmena, 2014; Guidetti et al., 2021; Marceglia et al., 2021). Moreover, with regard to cholinergic neurons in the NBM that have diffuse projections and diverse functions, it is crucial to avoid treating them as a homogeneous population. Instead, distinguishing them based on histological differences is imperative. Furthermore, cholinergic neurons account for only 5% of the neurons in the NBM; the other cellular components of the NBM have received little attention (Buzsaki et al., 1988; King et al., 1998; Lee et al., 2005). The involvement of other cell types, including glial cells, interneurons, glutamatergic neurons, etc., in each anatomical subdivision unit of the NBM poses an inevitable challenge for investigating the underlying neural network mechanism.
Considering the complexity of neural networks and the multi-site damage of brain tissue in neurodegenerative diseases, it is necessary to break the boundary of single nucleus, and future neural network research needs to be integrated from the perspective of the whole brain. On the one hand, in addition to cholinergic neurons, other neurons or receptor components, such as serotonergic (Viana et al., 2021), GABAergic (Iaccarino et al., 2016), glutamatergic neurons (Xu et al., 2015) and even muscarinic receptor (Velazquez-Moctezuma et al., 1991) also play important roles in the generation and modulation of neural network oscillations. On the other hand, in addition to NBM, other sites, such as the dorsal raphe nucleus (Qamhawi et al., 2015), entorhinal cortex (Leng et al., 2021), and hippocampus (Moodley and Chan, 2014) also play an important role in the development of neurodegenerative diseases, this may explain why so far evidence for NBM-DBS of improving cognitive function in clinical AD patients is rather scant, so dual-target NBM-DBS in the treatment of neurodegenerative diseases is a promising strategy and worth exploring in the future.
6 Conclusion
The NBM plays an important and complex role in the neural network dysfunction of various neurodegenerative diseases. The above studies demonstrated that lesions in the NBM can induce alterations in neuronal firing patterns within the prefrontal cortex, auditory cortex, and visual cortex, leading to a reduction in γ oscillation power and an increase in θ and α oscillation power. The underlying mechanism for these changes primarily involves the degradation of cholinergic neurons within the NBM and their cortical projection fibers; however, the contribution of other cellular components within the NBM, such as interneurons, should not be disregarded. Animal experiments utilizing NBM-DBS have shown that NBM-DBS partially reverses neural network abnormalities following NBM lesions, as indicated by decreased cortical θ and α oscillation power, increased γ oscillation power, and improved animal memory function. Although clinical trials investigating the effectiveness of NBM-DBS may not demonstrate outcomes as significant as studies in animal models, delayed cognitive decline associated with AD has been observed. With further advancements in our understanding of neural networks and the development of neuromodulation technologies, neuromodulation based on the NBM will play a transformative role in treating neurodegenerative disorders.
Author contributions
LJ: Data curation, Software, Writing – original draft. HK: Conceptualization, Data curation, Writing – review & editing. YG: Investigation, Software, Writing – review & editing. XL: Data curation, Formal analysis, Writing – review & editing. MW: Software, Writing – review & editing. KS: Project administration, Resources, Supervision, Writing – review & editing.
Glossary
Funding
The author(s) declare that financial support was received for the research, authorship, and/or publication of this article. All sources of fundings received for the research have been submitted. National Natural Science Foundation of China [81974279], which supported the information retrieval; National Key Project of Common and Frequently Occurring Diseases [2022YFC2503800], which supported the analysis and summary of related literatures; Natural Science Foundation of Hubei Province [2022CFB279], which supported language polishing and Health Commission of Hubei Province [WJ2021M131], which supported the publication fee.
Conflict of interest
The authors declare that the research was conducted in the absence of any commercial or financial relationships that could be construed as a potential conflict of interest.
Publisher’s note
All claims expressed in this article are solely those of the authors and do not necessarily represent those of their affiliated organizations, or those of the publisher, the editors and the reviewers. Any product that may be evaluated in this article, or claim that may be made by its manufacturer, is not guaranteed or endorsed by the publisher.
References
Adachi, T., Biesold, D., Inanami, O., and Sato, A. (1990a). Stimulation of the nucleus basalis of Meynert and substantia innominata produces widespread increases in cerebral blood flow in the frontal, parietal and occipital cortices. Brain Res. 514, 163–166. doi: 10.1016/0006-8993(90)90452-H
Adachi, T., Inanami, O., Ohno, K., and Sato, A. (1990b). Responses of regional cerebral blood flow following focal electrical stimulation of the nucleus basalis of Meynert and the medial septum using the [14C]iodoantipyrine method in rats. Neurosci. Lett. 112, 263–268. doi: 10.1016/0304-3940(90)90214-T
Amzica, F., and Steriade, M. (1998). Electrophysiological correlates of sleep delta waves. Electroencephalogr. Clin. Neurophysiol. 107, 69–83. doi: 10.1016/S0013-4694(98)00051-0
Arendt, T., Brückner, M. K., Morawski, M., Jäger, C., and Gertz, H. J. (2015). Early neurone loss in Alzheimer's disease: cortical or subcortical? Acta Neuropathol. Commun. 3:10. doi: 10.1186/s40478-015-0187-1
Başar, E., Schürmann, M., Başar-Eroglu, C., and Karakaş, S. (1997). Alpha oscillations in brain functioning: an integrative theory. Int. J. Psychophysiol. 26, 5–29. doi: 10.1016/S0167-8760(97)00753-8
Bensmaia, S. J., and Miller, L. E. (2014). Restoring sensorimotor function through intracortical interfaces: progress and looming challenges. Nat. Rev. Neurosci. 15, 313–325. doi: 10.1038/nrn3724
Berdiev, R. K., Chepurnov, S. A., Veening, J. G., Chepurnova, N. E., and van Luijtelaar, G. (2007). The role of the nucleus basalis of Meynert and reticular thalamic nucleus in pathogenesis of genetically determined absence epilepsy in rats: a lesion study. Brain Res. 1185, 266–274. doi: 10.1016/j.brainres.2007.09.010
Berdiev, R. K., and van Luijtelaar, G. (2009). Cholinergic stimulation of the nucleus basalis of Meynert and reticular thalamic nucleus affects spike-and-wave discharges in WAG/Rij rats. Neurosci. Lett. 463, 249–253. doi: 10.1016/j.neulet.2009.07.068
Berger, H. (1929). Über das Elektrenkephalogramm des Menschen. Arch. Psychiatr. Nervenkr. 87, 527–570. doi: 10.1007/BF01797193
Berntson, G. G., Shafi, R., and Sarter, M. (2002). Specific contributions of the basal forebrain corticopetal cholinergic system to electroencephalographic activity and sleep/waking behaviour. Eur. J. Neurosci. 16, 2453–2461. doi: 10.1046/j.1460-9568.2002.02310.x
Braak, H., Thal, D. R., Ghebremedhin, E., and del Tredici, K. (2011). Stages of the pathologic process in Alzheimer disease: age categories from 1 to 100 years. J. Neuropathol. Exp. Neurol. 70, 960–969. doi: 10.1097/NEN.0b013e318232a379
Bragin, A., Jando, G., Nadasdy, Z., Hetke, J., Wise, K., and Buzsaki, G. (1995). Gamma (40-100 Hz) oscillation in the hippocampus of the behaving rat. J. Neurosci. 15, 47–60. doi: 10.1523/JNEUROSCI.15-01-00047.1995
Buzsaki, G. (2002). Theta oscillations in the hippocampus. Neuron 33, 325–340. doi: 10.1016/S0896-6273(02)00586-X
Buzsaki, G., Bickford, R. G., Ponomareff, G., Thal, L. J., Mandel, R., and Gage, F. H. (1988). Nucleus basalis and thalamic control of neocortical activity in the freely moving rat. J. Neurosci. 8, 4007–4026. doi: 10.1523/JNEUROSCI.08-11-04007.1988
Canolty, R. T., and Knight, R. T. (2010). The functional role of cross-frequency coupling. Trends Cogn. Sci. 14, 506–515. doi: 10.1016/j.tics.2010.09.001
Cappon, D., Gratwicke, J., Zrinzo, L., Akram, H., Hyam, J., Hariz, M., et al. (2022). Deep brain stimulation of the nucleus basalis of Meynert for Parkinson's disease dementia: a 36 months follow up study. Movement Disord. Clin. Pract. 9, 765–774. doi: 10.1002/mdc3.13510
Cardin, J. A., Carlén, M., Meletis, K., Knoblich, U., Zhang, F., Deisseroth, K., et al. (2009). Driving fast-spiking cells induces gamma rhythm and controls sensory responses. Nature 459, 663–667. doi: 10.1038/nature08002
Chernyshev, B. V., Panasyuk, Y. A., Semikopnaya, I. I., and Timofeeva, N. O. (2004). Activity of neurons in the basal magnocellular nucleus during performance of an operant task. Neurosci. Behav. Physiol. 34, 907–918. doi: 10.1023/B:NEAB.0000042575.79517.b6
Chung, J. K., et al. (2016). Alzheimer's disease neuroimaging initiative. Cortical amyloid beta deposition and current depressive symptoms in Alzheimer disease and mild cognitive impairment (vol 29, pg 149, 2015). J. Geriatr. Psychiatry Neurol. 29, 237–241. doi: 10.1177/0891988715606230
Ciric, J., Lazic, K., Petrovic, J., Kalauzi, A., and Saponjic, J. (2016). Age-related disorders of sleep and motor control in the rat models of functionally distinct cholinergic neuropathology. Behav. Brain Res. 301, 273–286. doi: 10.1016/j.bbr.2015.12.046
Colgin, L. L., Denninger, T., Fyhn, M., Hafting, T., Bonnevie, T., Jensen, O., et al. (2009). Frequency of gamma oscillations routes flow of information in the hippocampus. Nature 462, 353–357. doi: 10.1038/nature08573
Danışman, B., Akçay, G., Gökçek-Saraç, Ç., Kantar, D., Aslan, M., and Derin, N. (2022). The role of acetylcholine on the effects of different doses of sulfite in learning and memory. Neurochem. Res. 47, 3331–3343. doi: 10.1007/s11064-022-03684-z
Destexhe, A., and Sejnowski, T. J. (2003). Interactions between membrane conductances underlying thalamocortical slow-wave oscillations. Physiol. Rev. 83, 1401–1453. doi: 10.1152/physrev.00012.2003
Dezawa, S., Nagasaka, K., Watanabe, Y., and Takashima, I. (2021). Lesions of the nucleus basalis magnocellularis (Meynert) induce enhanced somatosensory responses and tactile hypersensitivity in rats. Exp. Neurol. 335:113493. doi: 10.1016/j.expneurol.2020.113493
Engel, A. K., Fries, P., and Singer, W. (2001). Dynamic predictions: oscillations and synchrony in top-down processing. Nat. Rev. Neurosci. 2, 704–716. doi: 10.1038/35094565
Fetz, E. E. (2007). Volitional control of neural activity: implications for brain-computer interfaces. J. Physiol. 579, 571–579. doi: 10.1113/jphysiol.2006.127142
Fort, P., Khateb, A., Pegna, A., Mühlethaler, M., and Jones, B. E. (1995). Noradrenergic modulation of cholinergic nucleus basalis neurons demonstrated by in vitro pharmacological and immunohistochemical evidence in the guinea-pig brain. Eur. J. Neurosci. 7, 1502–1511. doi: 10.1111/j.1460-9568.1995.tb01145.x
Goard, M., and Dan, Y. (2009). Basal forebrain activation enhances cortical coding of natural scenes. Nat. Neurosci. 12, 1444–1449. doi: 10.1038/nn.2402
González, H. F. J., Narasimhan, S., Johnson, G. W., Wills, K. E., Haas, K. F., Konrad, P. E., et al. (2021). Role of the nucleus basalis as a key network node in temporal lobe epilepsy. Neurology 96, E1334–E1346. doi: 10.1212/WNL.0000000000011523
Graham, A. J., Martin-Ruiz, C., Teaktong, T., Ray, M., and Court, J. (2002). Human brain nicotinic receptors, their distribution and participation in neuropsychiatric disorders. Curr. Drug Targets CNS Neurol. Disord. 1, 387–397. doi: 10.2174/1568007023339283
Gratwicke, J., Oswal, A., Akram, H., Jahanshahi, M., Hariz, M., Zrinzo, L., et al. (2020). Resting state activity and connectivity of the nucleus basalis of Meynert and globus pallidus in Lewy body dementia and Parkinson's disease dementia. NeuroImage 221:117184. doi: 10.1016/j.neuroimage.2020.117184
Gratwicke, J., Zrinzo, L., Kahan, J., Peters, A., Beigi, M., Akram, H., et al. (2018). Bilateral deep brain stimulation of the nucleus basalis of Meynert for Parkinson disease dementia: a randomized clinical trial. JAMA Neurol. 75, 169–178. doi: 10.1001/jamaneurol.2017.3762
Guidetti, M., Marceglia, S., Loh, A., Harmsen, I. E., Meoni, S., Foffani, G., et al. (2021). Clinical perspectives of adaptive deep brain stimulation. Brain Stimul. 14, 1238–1247. doi: 10.1016/j.brs.2021.07.063
Hanna, A. S. F. S., Duara, R., and Crook, J. E. (2020). Selective vulnerability of the nucleus basalis of Meynert among Neuropathologic subtypes of Alzheimer disease. JAMA Neurol. 77:265. doi: 10.1001/jamaneurol.2019.3606
Herweg, N. A., Solomon, E. A., and Kahana, M. J. (2020). Theta oscillations in human memory. Trends Cogn. Sci. 24, 208–227. doi: 10.1016/j.tics.2019.12.006
Hong, J. H., and Jang, S. H. (2010). Neural pathway from nucleus basalis of Meynert passing through the cingulum in the human brain. Brain Res. 1346, 190–194. doi: 10.1016/j.brainres.2010.05.088
Hotta, H., Kagitani, F., Kondo, M., and Uchida, S. (2009). Basal forebrain stimulation induces NGF secretion in ipsilateral parietal cortex via nicotinic receptor activation in adult, but not aged rats. Neurosci. Res. 63, 122–128. doi: 10.1016/j.neures.2008.11.004
Howe, W. M., Gritton, H. J., Lusk, N. A., Roberts, E. A., Hetrick, V. L., Berke, J. D., et al. (2017). Acetylcholine release in prefrontal cortex promotes gamma oscillations and Theta-gamma coupling during Cue detection. J. Neurosci. 37, 3215–3230. doi: 10.1523/JNEUROSCI.2737-16.2017
Huang, C., Chu, H., Ma, Y., Zhou, Z., Dai, C., Huang, X., et al. (2019). The neuroprotective effect of deep brain stimulation at nucleus basalis of Meynert in transgenic mice with Alzheimer's disease. Brain Stimul. 12, 161–174. doi: 10.1016/j.brs.2018.08.015
Iaccarino, H. F., Singer, A. C., Martorell, A. J., Rudenko, A., Gao, F., Gillingham, T. Z., et al. (2016). Gamma frequency entrainment attenuates amyloid load and modifies microglia. Nature 540, 230–235. doi: 10.1038/nature20587
Jackson, A., and Fetz, E. E. (2011). Interfacing with the computational brain. IEEE Trans. Neural Syst. Rehabil. Eng. 19, 534–541. doi: 10.1109/TNSRE.2011.2158586
Jacobs, J., Kahana, M. J., Ekstrom, A. D., and Fried, I. (2007). Brain oscillations control timing of single-neuron activity in humans. J. Neurosci. 27, 3839–3844. doi: 10.1523/JNEUROSCI.4636-06.2007
Jasper, H., and Penfield, W. (1949). Electrocorticograms in man: effect of voluntary movement upon the electrical activity of the precentral gyrus. Eur. Arch. Psychiatry Clin. Neurosci. 183, 163–174. doi: 10.1007/BF01062488
Jones, E. G., Burton, H., Saper, C. B., and Swanson, L. W. (1976). Midbrain, diencephalic and cortical relationships of the basal nucleus of Meynert and associated structures in primates. J. Comp. Neurol. 167, 385–419. doi: 10.1002/cne.901670402
Jones, B. E., and Cuello, A. C. (1989). Afferents to the basal forebrain cholinergic cell area from pontomesencephalic--catecholamine, serotonin, and acetylcholine--neurons. Neuroscience 31, 37–61. doi: 10.1016/0306-4522(89)90029-8
Kasa, P. (1986). The cholinergic systems in brain and spinal cord. Prog. Neurobiol. 26, 211–272. doi: 10.1016/0301-0082(86)90016-X
Khateb, A., Fort, P., Alonso, A., Jones, B. E., and Mülethaler, M. (1993). Pharmacological and immunohistochemical evidence for serotonergic modulation of cholinergic nucleus basalis neurons. Eur. J. Neurosci. 5, 541–547. doi: 10.1111/j.1460-9568.1993.tb00519.x
Khateb, A., Fort, P., Pegna, A., Jones, B. E., and Mühlethaler, M. (1995). Cholinergic nucleus basalis neurons are excited by histamine in vitro. Neuroscience 69, 495–506. doi: 10.1016/0306-4522(95)00264-J
Khateb, A., Fort, P., Williams, S., Serafin, M., Mühlethaler, M., and Jones, B. E. (1998). GABAergic input to cholinergic nucleus basalis neurons. Neuroscience 86, 937–947. doi: 10.1016/S0306-4522(98)00094-3
King, C., Recce, M., and O'Keefe, J. (1998). The rhythmicity of cells of the medial septum/diagonal band of Broca in the awake freely moving rat: relationships with behaviour and hippocampal theta. Eur. J. Neurosci. 10, 464–477. doi: 10.1046/j.1460-9568.1998.00026.x
Klimesch, W. (2012). Alpha-band oscillations, attention, and controlled access to stored information. Trends Cogn. Sci. 16, 606–617. doi: 10.1016/j.tics.2012.10.007
Knoche, A., Yokoyama, H., Ponomarenko, A., Frisch, C., Huston, J., and Haas, H. L. (2003). High-frequency oscillation in the hippocampus of the behaving rat and its modulation by the histaminergic system. Hippocampus 13, 273–280. doi: 10.1002/hipo.10057
Knox, D., and Berntson, G. G. (2008). Cortical modulation by nucleus basalis magnocellularis corticopetal cholinergic neurons during anxiety-like states is reflected by decreases in delta. Brain Res. 1227, 142–152. doi: 10.1016/j.brainres.2008.06.060
Krauss, J. K., Lipsman, N., Aziz, T., Boutet, A., Brown, P., Chang, J. W., et al. (2021). Technology of deep brain stimulation: current status and future directions. Nat. Rev. Neurol. 17, 75–87. doi: 10.1038/s41582-020-00426-z
Kuhn, J., Hardenacke, K., Lenartz, D., Gruendler, T., Ullsperger, M., Bartsch, C., et al. (2015). Deep brain stimulation of the nucleus basalis of Meynert in Alzheimer's dementia. Mol. Psychiatry 20, 353–360. doi: 10.1038/mp.2014.32
Laurent, G. (2002). Olfactory network dynamics and the coding of multidimensional signals. Nat. Rev. Neurosci. 3, 884–895. doi: 10.1038/nrn964
Lee, M. G., Hassani, O. K., Alonso, A., and Jones, B. E. (2005). Cholinergic basal forebrain neurons burst with theta during waking and paradoxical sleep. J. Neurosci. 25, 4365–4369. doi: 10.1523/JNEUROSCI.0178-05.2005
Lee, J. E., Jeong, D. U., Lee, J., Chang, W. S., and Chang, J. W. (2016). The effect of nucleus basalis magnocellularis deep brain stimulation on memory function in a rat model of dementia. BMC Neurol. 16:6. doi: 10.1186/s12883-016-0529-z
Lee, D. J., Lozano, C. S., Dallapiazza, R. F., and Lozano, A. M. (2019). Current and future directions of deep brain stimulation for neurological and psychiatric disorders. J. Neurosurg. 131, 333–342. doi: 10.3171/2019.4.JNS181761
Lee, D. J., Milosevic, L., Gramer, R., Sasikumar, S., al-Ozzi, T. M., de Vloo, P., et al. (2019). Nucleus basalis of Meynert neuronal activity in Parkinson's disease. J. Neurosurg. 132, 574–582. doi: 10.3171/2018.11.JNS182386
Leng, K., Li, E., Eser, R., Piergies, A., Sit, R., Tan, M., et al. (2021). Molecular characterization of selectively vulnerable neurons in Alzheimer's disease. Nat. Neurosci. 24, 276–287. doi: 10.1038/s41593-020-00764-7
Lisman, J., and Buzsaki, G. (2008). A neural coding scheme formed by the combined function of gamma and theta oscillations. Schizophr. Bull. 34, 974–980. doi: 10.1093/schbul/sbn060
Lisman, J. E., and Jensen, O. (2013). The theta-gamma neural code. Neuron 77, 1002–1016. doi: 10.1016/j.neuron.2013.03.007
Liu, A. K., Chang, R. C. C., Pearce, R. K. B., and Gentleman, S. M. (2015). Nucleus basalis of Meynert revisited: anatomy, history and differential involvement in Alzheimer's and Parkinson's disease. Acta Neuropathol. 129, 527–540. doi: 10.1007/s00401-015-1392-5
Liu, R., Crawford, J., Callahan, P. M., Terry, A. V., Constantinidis, C., and Blake, D. T. (2018). Intermittent stimulation in the nucleus basalis of meynert improves sustained attention in rhesus monkeys. Neuropharmacology 137, 202–210. doi: 10.1016/j.neuropharm.2018.04.026
Ljubojevic, V., Luu, P., Gill, P. R., Beckett, L. A., Takehara-Nishiuchi, K., and de Rosa, E. (2018). Cholinergic modulation of Frontoparietal cortical network dynamics supporting Supramodal attention. J. Neurosci. 38, 3988–4005. doi: 10.1523/JNEUROSCI.2350-17.2018
Llinas, R. R. (1988). The intrinsic electrophysiological properties of mammalian neurons: insights into central nervous system function. Science 242, 1654–1664. doi: 10.1126/science.3059497
Luo, T., and Leung, L. S. (2009). Basal forebrain histaminergic transmission modulates electroencephalographic activity and emergence from isoflurane anesthesia. Anesthesiology 111, 725–733. doi: 10.1097/ALN.0b013e3181b061a0
Manfridi, A., Brambilla, D., and Mancia, M. (2001). Sleep is differently modulated by basal forebrain GABA(a) and GABA(B) receptors. Am. J. Phys. Regul. Integr. Comp. Phys. 281, R170–R175. doi: 10.1152/ajpregu.2001.281.1.R170
Marceglia, S., Guidetti, M., Harmsen, I. E., Loh, A., Meoni, S., Foffani, G., et al. (2021). Deep brain stimulation: is it time to change gears by closing the loop? J. Neural Eng. 18:061001. doi: 10.1088/1741-2552/ac3267
Marino, J., and Cudeiro, J. (2003). Nitric oxide-mediated cortical activation: a diffuse wake-up system. J. Neurosci. 23, 4299–4307. doi: 10.1523/JNEUROSCI.23-10-04299.2003
Martinez-Rubio, C., Paulk, A. C., McDonald, E. J., Widge, A. S., and Eskandar, E. N. (2018). Multimodal encoding of novelty, reward, and learning in the primate nucleus basalis of Meynert. J. Neurosci. 38, 1942–1958. doi: 10.1523/JNEUROSCI.2021-17.2017
McLin, D. E. 3rd, Miasnikov, A. A., and Weinberger, N. M. (2002). The effects of electrical stimulation of the nucleus basalis on the electroencephalogram, heart rate, and respiration. Behav. Neurosci. 116, 795–806. doi: 10.1037/0735-7044.116.5.795
McLin, D. E. 3rd, Miasnikov, A. A., and Weinberger, N. M. (2003). CS-specific gamma, theta, and alpha EEG activity detected in stimulus generalization following induction of behavioral memory by stimulation of the nucleus basalis. Neurobiol. Learn. Mem. 79, 152–176. doi: 10.1016/S1074-7427(02)00009-6
Mehraram, R., Peraza, L. R., Murphy, N. R. E., Cromarty, R. A., Graziadio, S., O’Brien, J. T., et al. (2022). Functional and structural brain network correlates of visual hallucinations in Lewy body dementia. Brain 145, 2190–2205. doi: 10.1093/brain/awac094
Meir, I., Katz, Y., and Lampl, I. (2018). Membrane potential correlates of network decorrelation and improved SNR by cholinergic activation in the somatosensory cortex. J. Neurosci. 38, 10692–10708. doi: 10.1523/JNEUROSCI.1159-18.2018
Mesulam, M. M., and Geula, C. (1988). Nucleus basalis (Ch4) and cortical cholinergic innervation in the human brain: observations based on the distribution of acetylcholinesterase and choline acetyltransferase. J. Comp. Neurol. 275, 216–240. doi: 10.1002/cne.902750205
Mesulam, M. M., Mufson, E. J., Levey, A. I., and Wainer, B. H. (1983). Cholinergic innervation of cortex by the basal forebrain: cytochemistry and cortical connections of the septal area, diagonal band nuclei, nucleus basalis (substantia innominata), and hypothalamus in the rhesus monkey. J. Comp. Neurol. 214, 170–197. doi: 10.1002/cne.902140206
Mesulam, M. M., Mufson, E. J., Levey, A. I., and Wainer, B. H. (1984). Atlas of cholinergic neurons in the forebrain and upper brainstem of the macaque based on monoclonal choline acetyltransferase immunohistochemistry and acetylcholinesterase histochemistry. Neuroscience 12, 669–686. doi: 10.1016/0306-4522(84)90163-5
Montgomery, S. M., Sirota, A., and Buzsaki, G. (2008). Theta and gamma coordination of hippocampal networks during waking and rapid eye movement sleep. J. Neurosci. 28, 6731–6741. doi: 10.1523/JNEUROSCI.1227-08.2008
Moodley, K. K., and Chan, D. (2014). The hippocampus in neurodegenerative disease. Front. Neurol. Neurosci. 34, 95–108. doi: 10.1159/000356430
Morelli, A., Sarchielli, E., Guarnieri, G., Coppi, E., Pantano, D., Comeglio, P., et al. (2017). Young human cholinergic neurons respond to physiological regulators and improve cognitive symptoms in an animal model of Alzheimer's disease. Front. Cell. Neurosci. 11:339. doi: 10.3389/fncel.2017.00339
Nagasaka, K., Watanabe, Y., and Takashima, I. (2017). Topographical projections from the nucleus basalis magnocellularis (Meynert) to the frontal cortex: a voltage-sensitive dye imaging study in rats. Brain Stimul. 10, 977–980. doi: 10.1016/j.brs.2017.06.008
Nazmuddin, M., Oterdoom, D. L. M., van Dijk, J. M. C., van Zijl, J. C., Kampman, A. K., Drost, G., et al. (2018). Oscillatory activity and cortical coherence of the nucleus basalis of Meynert in Parkinson's disease dementia. Parkinsonism Relat. Disord. 52, 102–106. doi: 10.1016/j.parkreldis.2018.03.024
Nokia, M. S., and Penttonen, M. (2022). Rhythmic memory consolidation in the hippocampus. Front. Neurol. Circuits. 16:885684. doi: 10.3389/fncir.2022.885684
Orsborn, A. L., and Carmena, J. M. (2013). Creating new functional circuits for action via brain-machine interfaces. Front. Comput. Neurosci. 7:157.
Oswal, A., Gratwicke, J., Akram, H., Jahanshahi, M., Zaborszky, L., Brown, P., et al. (2021). Cortical connectivity of the nucleus basalis of Meynert in Parkinson's disease and Lewy body dementias. Brain 144, 781–788. doi: 10.1093/brain/awaa411
Palva, S., and Palva, J. M. (2007). New vistas for alpha-frequency band oscillations. Trends Neurosci. 30, 150–158. doi: 10.1016/j.tins.2007.02.001
Pare, D., and Gaudreau, H. (1996). Projection cells and interneurons of the lateral and basolateral amygdala: distinct firing patterns and differential relation to theta and delta rhythms in conscious cats. J. Neurosci. 16, 3334–3350. doi: 10.1523/JNEUROSCI.16-10-03334.1996
Pereira, E. A., and Aziz, T. Z. (2014). Neuropathic pain and deep brain stimulation. Neurotherapeutics 11, 496–507. doi: 10.1007/s13311-014-0278-x
Pittman-Polletta, B. R., Quach, A., Mohammed, A. I., Romano, M., Kondabolu, K., Kopell, N. J., et al. (2018). Striatal cholinergic receptor activation causes a rapid, selective and state-dependent rise in cortico-striatal beta activity. Eur. J. Neurosci. 48, 2857–2868. doi: 10.1111/ejn.13906
Qamhawi, Z., Towey, D., Shah, B., Pagano, G., Seibyl, J., Marek, K., et al. (2015). Clinical correlates of raphe serotonergic dysfunction in early Parkinson's disease. Brain 138, 2964–2973. doi: 10.1093/brain/awv215
Rea, R. C., Berlot, R., Martin, S. L., Craig, C. E., Holmes, P. S., Wright, D. J., et al. (2021). Quantitative EEG and cholinergic basal forebrain atrophy in Parkinson's disease and mild cognitive impairment. Neurobiol. Aging 106, 37–44. doi: 10.1016/j.neurobiolaging.2021.05.023
Rispoli, V., Marra, R., Costa, N., Rotiroti, D., Tirassa, P., Scipione, L., et al. (2008). Choline pivaloyl ester enhances brain expression of both nerve growth factor and high-affinity receptor TrkA, and reverses memory and cognitive deficits, in rats with excitotoxic lesion of nucleus basalis magnocellularis. Behav. Brain Res. 190, 22–32. doi: 10.1016/j.bbr.2008.02.002
Rispoli, V., Ragusa, S., Nisticò, R., Marra, R., Russo, E., Leo, A., et al. (2013). Huperzine a restores cortico-hippocampal functional connectivity after bilateral AMPA lesion of the nucleus basalis of meynert. J. Alzheimers Dis. 35, 833–846. doi: 10.3233/JAD-130278
Rispoli, V., Rotiroti, D., Carelli, V., Liberatore, F., Scipione, L., Marra, R., et al. (2004). Electroencephalographic effects induced by choline pivaloyl esters in scopolamine-treated or nucleus basalis magnocellularis lesioned rats. Pharmacol. Biochem. Behav. 78, 667–673. doi: 10.1016/j.pbb.2004.04.031
Rodriguez, R., Kallenbach, U., Singer, W., and Munk, M. H. J. (2004). Short- and long-term effects of cholinergic modulation on gamma oscillations and response synchronization in the visual cortex. J. Neurosci. 24, 10369–10378. doi: 10.1523/JNEUROSCI.1839-04.2004
Russchen, F. T., Amaral, D. G., and Price, J. L. (1985). The afferent connections of the substantia innominata in the monkey, Macaca fascicularis. J. Comp. Neurol. 242, 1–27. doi: 10.1002/cne.902420102
Rutishauser, U., Ross, I. B., Mamelak, A. N., and Schuman, E. M. (2010). Human memory strength is predicted by theta-frequency phase-locking of single neurons. Nature 464, 903–907. doi: 10.1038/nature08860
Sakalar, E., Klausberger, T., and Lasztoczi, B. (2022). Neurogliaform cells dynamically decouple neuronal synchrony between brain areas. Science 377:324. doi: 10.1126/science.abo3355
Sanchez-Alavez, M., and Ehlers, C. L. (2016). Event-related oscillations (ERO) during an active discrimination task: effects of lesions of the nucleus basalis magnocellularis. Int. J. Psychophysiol. 103, 53–61. doi: 10.1016/j.ijpsycho.2015.02.010
Sanchez-Alavez, M., Robledo, P., Wills, D. N., Havstad, J., and Ehlers, C. L. (2014). Cholinergic modulation of event-related oscillations (ERO). Brain Res. 1559, 11–25. doi: 10.1016/j.brainres.2014.02.043
Sasikumar, S., Cohn, M., Harmsen, I. E., Loh, A., Cho, S. S., Sáenz-Farret, M., et al. (2022). Single-trajectory multiple-target deep brain stimulation for parkinsonian mobility and cognition. Mov. Disord. 37, 635–640. doi: 10.1002/mds.28870
Schmidt, R., Herrojo Ruiz, M., Kilavik, B. E., Lundqvist, M., Starr, P. A., and Aron, A. R. (2019). Beta oscillations in working memory, executive control of movement and thought, and sensorimotor function. J. Neurosci. 39, 8231–8238. doi: 10.1523/JNEUROSCI.1163-19.2019
Schumacher, J., Ray, N. J., Hamilton, C. A., Donaghy, P. C., Firbank, M., Roberts, G., et al. (2022). Cholinergic white matter pathways in dementia with Lewy bodies and Alzheimer's disease. Brain 145, 1773–1784. doi: 10.1093/brain/awab372
Schumacher, J., Taylor, J. P., Hamilton, C. A., Firbank, M., Cromarty, R. A., Donaghy, P. C., et al. (2021). In vivo nucleus basalis of Meynert degeneration in mild cognitive impairment with Lewy bodies. Neuroimage Clin 30:102604. doi: 10.1016/j.nicl.2021.102604
Schumacher, J., Thomas, A. J., Peraza, L. R., Firbank, M., Cromarty, R., Hamilton, C. A., et al. (2020). EEG alpha reactivity and cholinergic system integrity in Lewy body dementia and Alzheimer's disease. Alzheimers Res. Ther. 12:46. doi: 10.1186/s13195-020-00613-6
Selden, N. R., Gitelman, D. R., Salamon-Murayama, N., Parrish, T. B., and Mesulam, M. M. (1998). Trajectories of cholinergic pathways within the cerebral hemispheres of the human brain. Brain 121, 2249–2257. doi: 10.1093/brain/121.12.2249
Semba, K., and Fibiger, H. C. (1989). Organization of central cholinergic systems. Prog. Brain Res. 79, 37–63. doi: 10.1016/S0079-6123(08)62464-4
Senoussi, M., Verbeke, P., Desender, K., de Loof, E., Talsma, D., and Verguts, T. (2022). Theta oscillations shift towards optimal frequency for cognitive control. Nat. Hum. Behav. 6, 1000–1013. doi: 10.1038/s41562-022-01335-5
Shenoy, K. V., and Carmena, J. M. (2014). Combining decoder design and neural adaptation in brain-machine interfaces. Neuron 84, 665–680. doi: 10.1016/j.neuron.2014.08.038
Singh, B., Qi, X. L., Blake, D. T., and Constantinidis, C. (2022). Rhythmicity of prefrontal local field potentials after nucleus basalis stimulation. eNeuro 9, ENEURO.0380–ENEU21.2022. doi: 10.1523/ENEURO.0380-21.2022
Smiley, J. F., and Mesulam, M. M. (1999). Cholinergic neurons of the nucleus basalis of meynert receive cholinergic, catecholaminergic and gabaergic synapses: an electron microscopic investigation in the monkey. Neuroscience 88, 241–255. doi: 10.1016/S0306-4522(98)00202-4
Song, M., Mohamad, O., Chen, D., and Yu, S. P. (2013). Coordinated development of voltage-gated Na+ and K+ currents regulates functional maturation of forebrain neurons derived from human induced pluripotent stem cells. Stem Cells Dev. 22, 1551–1563. doi: 10.1089/scd.2012.0556
Spencer, J. P., Middleton, L. J., and Davies, C. H. (2010). Investigation into the efficacy of the acetylcholinesterase inhibitor, donepezil, and novel procognitive agents to induce gamma oscillations in rat hippocampal slices. Neuropharmacology 59, 437–443. doi: 10.1016/j.neuropharm.2010.06.005
Stefanics, G., Hangya, B., Hernádi, I., Winkler, I., Lakatos, P., and Ulbert, I. (2010). Phase entrainment of human delta oscillations can mediate the effects of expectation on reaction speed. J. Neurosci. 30, 13578–13585. doi: 10.1523/JNEUROSCI.0703-10.2010
Steriade, M. (2001). Impact of network activities on neuronal properties in corticothalamic systems. J. Neurophysiol. 86, 1–39. doi: 10.1152/jn.2001.86.1.1
Steriade, M. (2004). Acetylcholine systems and rhythmic activities during the waking--sleep cycle. Prog. Brain Res. 145, 179–196. doi: 10.1016/S0079-6123(03)45013-9
Temel, Y., Visser-Vandewalle, V., Kaplan, S., Kozan, R., Daemen, M. A. R. C., Blokland, A., et al. (2006). Protection of nigral cell death by bilateral subthalamic nucleus stimulation. Brain Res. 1120, 100–105. doi: 10.1016/j.brainres.2006.08.082
Varela, F., Lachaux, J. P., Rodriguez, E., and Martinerie, J. (2001). The brainweb: phase synchronization and large-scale integration. Nat. Rev. Neurosci. 2, 229–239. doi: 10.1038/35067550
Velazquez-Moctezuma, J., Shalauta, M., Christian Gillin, J., and Shiromani, P. J. (1991). Cholinergic antagonists and REM sleep generation. Brain Res. 543, 175–179. doi: 10.1016/0006-8993(91)91064-8
Viana, M. B., Martins, R. S., Silva, M. S. C. F., Xapelli, S., Vaz, S. H., and Sebastião, A. M. (2021). Deep brain stimulation of the dorsal raphe abolishes serotonin 1A facilitation of AMPA receptor-mediated synaptic currents in the ventral hippocampus. Behav. Brain Res. 403:113134. doi: 10.1016/j.bbr.2021.113134
Wallace, B. A., Ashkan, K., Heise, C. E., Foote, K. D., Torres, N., Mitrofanis, J., et al. (2007). Survival of midbrain dopaminergic cells after lesion or deep brain stimulation of the subthalamic nucleus in MPTP-treated monkeys. Brain 130, 2129–2145. doi: 10.1093/brain/awm137
Wan, L., Huang, H., Schwab, N., Tanner, J., Rajan, A., Lam, N. B., et al. (2019). From eyes-closed to eyes-open: role of cholinergic projections in EC-to-EO alpha reactivity revealed by combining EEG and MRI. Hum. Brain Mapp. 40, 566–577. doi: 10.1002/hbm.24395
Wang, X. J. (2010). Neurophysiological and computational principles of cortical rhythms in cognition. Physiol. Rev. 90, 1195–1268. doi: 10.1152/physrev.00035.2008
Wang, X., Hu, W. H., Zhang, K., Zhou, J. J., Liu, D. F., Zhang, M. Y., et al. (2018). Acute fornix deep brain stimulation improves hippocampal glucose metabolism in aged mice. Chin. Med. J. 131, 594–599. doi: 10.4103/0366-6999.226067
Weinberger, N. M., Miasnikov, A. A., and Chen, J. C. (2006). The level of cholinergic nucleus basalis activation controls the specificity of auditory associative memory. Neurobiol. Learn. Mem. 86, 270–285. doi: 10.1016/j.nlm.2006.04.004
Williams, M. R., Marsh, R., Macdonald, C. D., Jain, J., Pearce, R. K. B., Hirsch, S. R., et al. (2013). Neuropathological changes in the nucleus basalis in schizophrenia. Eur. Arch. Psychiatry Clin. Neurosci. 263, 485–495. doi: 10.1007/s00406-012-0387-7
Woolf, N. J. (1991). Cholinergic systems in mammalian brain and spinal cord. Prog. Neurobiol. 37, 475–524. doi: 10.1016/0301-0082(91)90006-M
Woolf, N. J., and Butcher, L. L. (1982). Cholinergic projections to the basolateral amygdala: a combined Evans blue and acetylcholinesterase analysis. Brain Res. Bull. 8, 751–763. doi: 10.1016/0361-9230(82)90102-2
Xu, M., Chung, S., Zhang, S., Zhong, P., Ma, C., Chang, W. C., et al. (2015). Basal forebrain circuit for sleep-wake control. Nat. Neurosci. 18, 1641–1647. doi: 10.1038/nn.4143
Yakubov, B., das, S., Zomorrodi, R., Blumberger, D. M., Enticott, P. G., Kirkovski, M., et al. (2022). Cross-frequency coupling in psychiatric disorders: a systematic review. Neurosci. Biobehav. Rev. 138:104690. doi: 10.1016/j.neubiorev.2022.104690
Yu, T. W., Lane, H. Y., and Lin, C. E. H. (2021). Novel therapeutic approaches for Alzheimer's disease: an updated review. Int. J. Mol. Sci. 22:8208. doi: 10.3390/ijms22158208
Zheng, Y., Feng, S., Zhu, X., Jiang, W., Wen, P., Ye, F., et al. (2018). Different subgroups of cholinergic neurons in the basal forebrain are distinctly innervated by the olfactory regions and activated differentially in olfactory memory retrieval. Front Neural Circuits 12:99. doi: 10.3389/fncir.2018.00099
Keywords: nucleus basalis of Meynert, neurophysiology, Alzheimer’s disease, deep brain stimulation, neural network, oscillations, cross-frequency coupling
Citation: Jiao L, Kang H, Geng Y, Liu X, Wang M and Shu K (2024) The role of the nucleus basalis of Meynert in neuromodulation therapy: a systematic review from the perspective of neural network oscillations. Front. Aging Neurosci. 16:1376764. doi: 10.3389/fnagi.2024.1376764
Edited by:
Allison B. Reiss, Long Island School of Medicine, New York University, United StatesReviewed by:
Heling Chu, Shanghai Sixth People's Hospital, Shanghai Jiao Tong University, ChinaCosta Vakalopoulos, Richmond Hill Medical Centre, Australia
Copyright © 2024 Jiao, Kang, Geng, Liu, Wang and Shu. This is an open-access article distributed under the terms of the Creative Commons Attribution License (CC BY). The use, distribution or reproduction in other forums is permitted, provided the original author(s) and the copyright owner(s) are credited and that the original publication in this journal is cited, in accordance with accepted academic practice. No use, distribution or reproduction is permitted which does not comply with these terms.
*Correspondence: Kai Shu, a3NodUB0amgudGptdS5lZHUuY24=
†These authors have contributed equally to this work and share first authorship