- 1Department of Operating Room, The Second Affiliated Hospital of Nanchang University, Nanchang, Jiangxi, China
- 2Department of Anesthesiology, The Second Affiliated Hospital of Nanchang University, Nanchang, Jiangxi, China
- 3Nanchang University Queen Mary School, Nanchang, Jiangxi, China
Ischemic stroke is a condition caused by an interruption of blood flow to the brain that can lead to neurological damage. The severe neurological damage caused by an ischemic stroke can lead to cognitive impairment and even disability. Reperfusion therapy is the mainstay of treatment for ischemic stroke. However, while restoring oxygen and blood flow to the brain tissue can reduce or prevent neuronal cell damage and death caused by cerebral ischemia, ischemia/reperfusion may trigger pathological tissue reactions leading to neuronal cell damage. Excessive autophagy in neuronal cells, disruption of cellular oxidative homeostasis leading to oxidative stress, apoptosis, glutamatergic excitatory damage, ferroptosis, and neuroinflammation are all key pathways contributing to cerebral ischemia/reperfusion injury. Electroacupuncture, as an extension of traditional Chinese acupuncture, has obvious effects on alleviating cerebral ischemia/reperfusion injury. Many experiments have observed that after electroacupuncture treatment or pretreatment in rats, cognitive impairment was reduced, brain tissue morphology was improved, and the damage pathways such as autophagy, oxidative stress, neuroinflammation, and apoptosis were significantly inhibited, and the recovery pathways such as the blood-brain barrier and angiogenesis were significantly promoted. Although the specific mechanism of electroacupuncture therapy is not known, it has great potential in the treatment of ischemic stroke and cerebral ischemia/reperfusion injury. Electroacupuncture to improve cerebral ischemia/reperfusion injury is a new target for therapeutic approaches. In the future, electroacupuncture is expected to become an effective therapy for cerebral ischemia/reperfusion by conducting more clinical trials and enriching the understanding of its mechanism for improving cerebral ischemia/reperfusion injury.
1 Introduction
Stroke, a cerebrovascular accident, results from acute focal CNS damage and presents as neurological deficits (Hilkens et al., 2024). These deficits primarily stem from cerebrovascular anomalies that disrupt cerebral circulation. Global stroke prevalence has risen steadily, recording a 70% surge in incident cases between 1990 and 2019 (Bersano and Gatti, 2023; Feigin et al., 2022). China shows particularly concerning statistics, with age-standardized stroke incidence increasing by 11.5% during 1990-2021 (Krishnamurthi et al., 2020), highlighting ongoing challenges in national stroke management. Ischemic stroke constitutes 63% of total stroke cases (Potter et al., 2022). This condition features cerebral hypoperfusion, triggering tissue necrosis and vascular territory-specific neurological dysfunction. As a primary cause of cognitive impairment and disability (Zhao et al., 2022), ischemic stroke exerts significant socioeconomic burdens through high morbidity, recurrence rates, and mortality, directly compromising patient outcomes and straining public health systems (Tang et al., 2021).
Current therapies employ thrombolysis and thrombectomy to achieve cerebral reperfusion prior to irreversible neuronal damage (Shen et al., 2021). Paradoxically, this intervention triggers pathological cascades—including neuroinflammation (Candelario-Jalil et al., 2022), autophagy dysregulation (Du et al., 2020), and oxidative stress (Zhu et al., 2023), culminating in cerebral ischemia/reperfusion injury (CIRI). The pathophysiological complexity of CIRI involves interrelated mechanisms: reactive oxygen species (ROS) overproduction, calcium dyshomeostasis, glial activation, and apoptotic pathways (Wang Z. et al., 2023). These processes induce microvascular constriction, blood cell aggregation, cerebral edema, and neuronal apoptosis, synergistically exacerbating pathological cascades (Zhang et al., 2024), and impeding clinical management with compromised prognostic accuracy. Electroacupuncture (EA), an advanced acupuncture technique utilizing standardized electrical parameters, delivers consistent therapeutic stimulation with demonstrated safety (Franke et al., 2021). Preclinical studies validate EA’s neuroprotective effects against CIRI-induced neurological damage (Qi et al., 2022; Wang M. et al., 2020), though its mechanisms demand further elucidation. Current findings suggest EA modulates critical pathological processes including neuroinflammatory responses, oxidative balance, and apoptotic regulation. This review synthesizes evidence on these multifactorial mechanisms to optimize EA-based therapeutic strategies for CIRI.
2 Electroacupuncture
Acupuncture therapy functions via targeted stimulation of meridian pathways to trigger systemic physiological responses (Liu et al., 2021). EA augments this process by applying controlled electrical stimulation to acupoints, amplifying therapeutic effects. Preclinical studies validate its multifactorial efficacy: facilitating endogenous neural stem cell differentiation in post-stroke recovery (Zhang et al., 2021), modulating pain signaling in inflammatory conditions (Zhang Q. et al., 2023), and enhancing cognitive ability while improving neurological function in Alzheimer’s disease model animals (Zheng et al., 2021). These findings demonstrate EA’s unique capacity to target neuro-muscular pathologies. Clinical trials corroborate these preclinical findings, confirming EA’s translatable efficacy across neurological disorders (Mao et al., 2021; Table 1).
Contemporary ischemic stroke management integrates pharmacological thrombolysis with mechanical interventions. First-line protocols prioritize intravenous tissue plasminogen activator (Zhao et al., 2022) supplemented by dual antiplatelet therapy (aspirin/clopidogrel) for minor strokes, while mechanical thrombectomy is reserved as the gold-standard intervention for disabling cases (Mendelson and Prabhakaran, 2021). EA emerges as a complementary modality, counteracting thrombolysis-associated ischemia/reperfusion injury through neuroprotective mechanisms. Its demonstrated safety profile, synergistic enhancement of conventional therapy efficacy, and minimal adverse effects establish EA as a strategic adjunct therapy for comprehensive cerebral injury management.
Despite demonstrated preclinical efficacy, EA’s clinical translation requires systematic validation through rigorously designed trials. Key research priorities center on elucidating disease-specific mechanisms across neurological conditions, optimizing stimulation protocols through parameter standardization, and establishing multi-dimensional safety assessments encompassing long-term outcomes. Addressing these challenges will enable the precise integration of EA into evidence-driven stroke rehabilitation frameworks, bridging the gap between experimental findings and clinical implementation.
3 The improvement of cerebral ischemia/reperfusion injury through electroacupuncture mechanisms
EA ameliorates CIRI through dual mechanisms: injury reversal and neuroprotection. Post-injury, neuronal and glial cells exhibit pathological manifestations including inflammation, oxidative stress, excessive autophagy, ferroptosis, and excitotoxic injury. These processes collectively induce apoptosis and impair neurological function (Zhang et al., 2024). This review systematically elucidates the mechanisms underlying EA’s therapeutic effects on CIRI, specifically analyzing its dual regulatory pathways: pathogenic process suppression and neural repair activation (Figure 1).
EA works in a variety of ways to improve cerebral ischemia/reperfusion. It promotes the blood-brain barrier and angiogenesis against injury and prevents excessive autophagy, apoptosis, oxidative stress, inflammation, ferroptosis, and excitotoxic injury.
3.1 Inhibition effect
3.1.1 Autophagy
Autophagy, an evolutionarily conserved eukaryotic cellular process, sustains intracellular homeostasis through macromolecule and organelle degradation. This mechanism employs autophagosomes and lysosomes to achieve dual biological outcomes: recycling of degradation products or induction of non-apoptotic programed cell death. Under pathological conditions, excessive autophagy may trigger cell death (Levine and Kroemer, 2019; Liu S. et al., 2023; Vargas et al., 2023; Zhang et al., 2022). Autophagy is functionally categorized into two subtypes: non-selective (bulk) autophagy and selective autophagy. The latter specifically mediates targeted degradation of individual cellular components, exemplified by mitophagy in mitochondrial clearance (Klionsky et al., 2021).
Current studies report conflicting findings regarding the neuroprotective effects of EA-mediated autophagy regulation during cerebral ischemia-reperfusion. In a recent investigation, Qiu et al. quantified autophagy markers (LC3 and p62) in CIRI rat neurons, observing that EA decreased LC3 expression and increased p62 expression. Researchers administered EA at Baihui, Quchi, and Zusanli acupoints with parameters: 2/15 Hz dense-dispersive wave, 1 mA current. Results indicate that EA suppresses autophagy during reperfusion, alleviating CIRI (Mizushima and Levine, 2020; Qiu et al., 2025).
Conversely, emerging evidence suggests autophagy upregulation exerts neuroprotective effects. Xu et al. demonstrated that EA treatment enhances autophagic activity and mitigates CIRI. EA was administered at Du20 and Du26 acupoints at 5 min and 16 h post-injury, employing sparse (3.85 Hz for the 1.28-s duration) and dense (6.25 Hz for the 2.08-s duration) wave patterns. Stimulation parameters comprised a current intensity of 0.8–1.0 mA delivered over a 30-min treatment session (Xu et al., 2020). The precise mechanisms underlying EA-mediated autophagy regulation during injury repair require systematic investigation. These divergent outcomes may stem from intercellular variations in baseline autophagy levels across experimental models. Specifically, in cellular populations with diminished autophagic activity, EA potentially enhances autophagic flux, facilitating the clearance of compromised organelles and subsequent mitigation of injury. Conversely, in cellular systems exhibiting elevated basal autophagic activity, EA may exert inhibitory regulation on autophagic flux, thereby attenuating pathological consequences associated with excessive self-digestive processes.
Cellular autophagy is modulated through multiple signaling pathways, with extensive research elucidating key regulatory mechanisms. However, the precise pathway by which EA exerts its autophagic regulation remains undetermined, attributable to limited mechanistic evidence. Current investigations primarily focus on two pathways: mTOR-dependent and mTOR-independent signaling cascades. The mTOR-independent cascade, canonically designated as the Beclin1/B-cell lymphoma 2 (Bcl-2)/c-Jun N-terminal kinase 1 (JNK1) signaling axis, operates through coordinated regulation by autophagy-related genes (ATG) (Ahsan et al., 2021).
EA ameliorates CIRI through mTOR pathway upregulation, modulating autophagy activity (Wang M. et al., 2020). mTOR functions through two distinct complexes: mTOR complex 1 (mTORC1) and mTORC2. mTORC1 primarily suppresses autophagic activity, whereas this regulatory effect operates through the class I phosphatidylinositol 3-kinase (PI3K-I)/protein kinase B (AKT) signaling axis. Class 1 PI3K initiates this signaling cascade by phosphorylating plasma membrane phospholipids, thereby activating AKT. Activated AKT subsequently phosphorylates tuberous sclerosis complex 2 (TSC2), disrupting the TSC1-TSC2 interaction and ultimately relieving mTORC1 from inhibitory regulation (Ahsan et al., 2021; Tian et al., 2022).
EA modulates autophagy through dual regulatory mechanisms: controlling autophagosome generation and upstream ATGs. Experimental evidence indicates that EA pretreatment suppresses LC3 accumulation while elevating p62 expression, concurrent with significant structural restoration of neurons in the ischemic cerebral cortex of rat models. The regulation of autophagosomal components constitutes a critical control node in autophagy modulation (Mei et al., 2020; Figure 2). Experimental evidence reveals that silent information regulator 1 modulates autophagic activity via deacetylation mechanisms targeting both histones and non-histone proteins, including FOXO1 and p53. Under cerebral ischemia-reperfusion-induced oxidative stress, cytoplasmic acetylated FOXO1 accumulates and interacts with ATG7, a core autophagy executor, thereby activating autophagic flux. EA intervention effectively reverses this molecular interaction cascade (Mei et al., 2020; Figure 2).
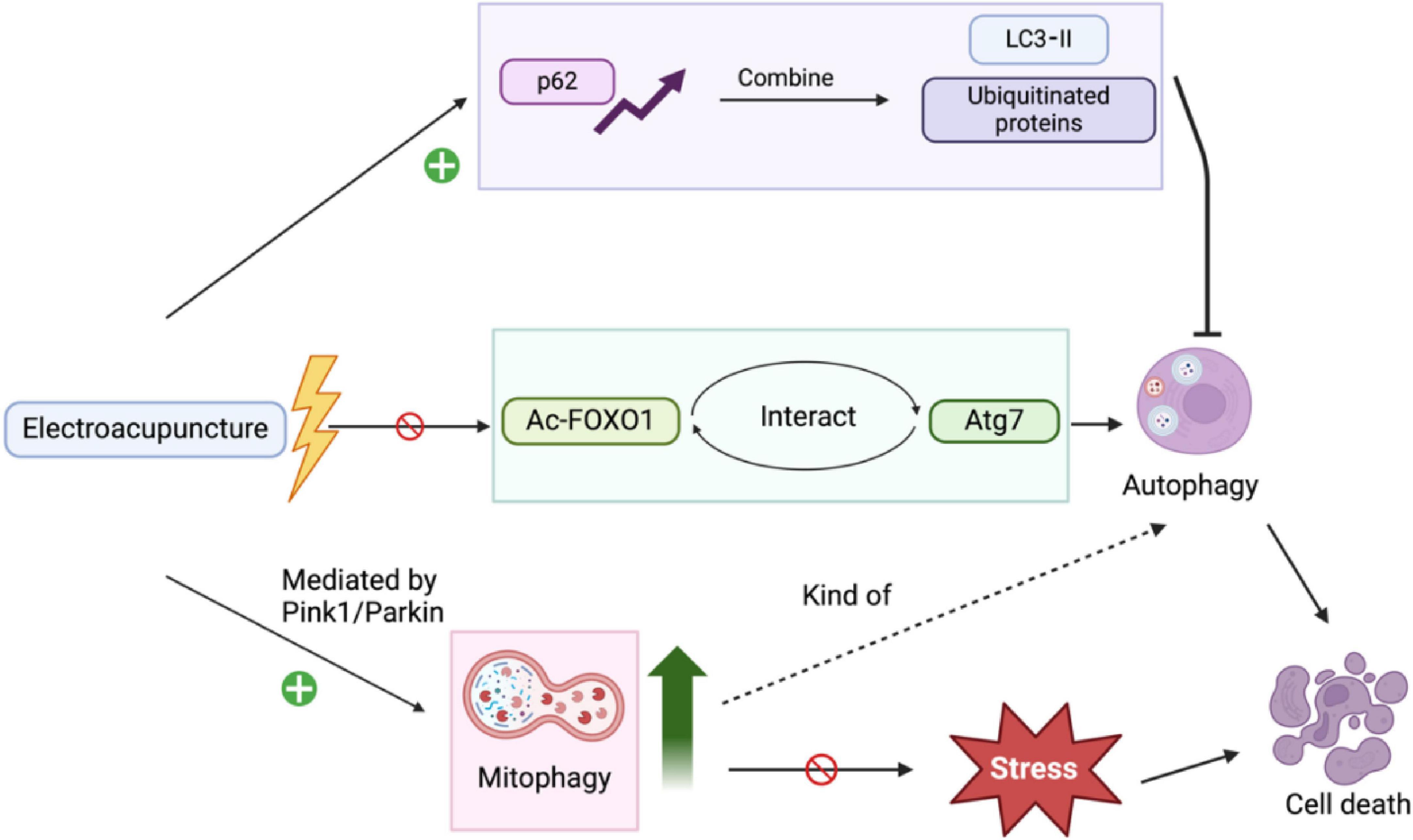
Figure 2. The pathways adjust autophagy/mitophagy to prevent cerebral ischemia/reperfusion injury. LC3-II, light chain 3-II; AC-FOXO1, acetylated FOXO1; Atg7, autophagy-related gene 7.
Mitophagy serves as a critical neuroprotective mechanism in EA-mediated mitigation of CIRI. Ischemia-hypoxia during injury, disrupts mitochondrial electron transport chain function, impairing oxygen/glucose supply and adenosine triphosphate (ATP) synthesis. This oxygen delivery-consumption mismatch compromises oxidative phosphorylation, amplifying neuronal degeneration. Prolonged hypoxia depletes ATP, disrupting energy-dependent ion channel function and triggering sequential pathological events: potassium efflux, membrane depolarization, and voltage-gated calcium channel activation. Subsequent intracellular Ca2+ overload induces mitochondrial edema, which initiates pro-apoptotic factor release and structural disintegration of mitochondria. During reperfusion, electrons escaping from the impaired respiratory chain react with molecular oxygen, generating ROS that potentiate mitochondrial damage through oxidative stress cascades. ROS mediate dual activation of AMPK and NF-κB signaling pathways, driving proinflammatory cytokine secretion while amplifying oxidative damage (Li and Xing, 2023). Autophagy can also aggravate oxidative stress injury, and the combination of these reactions worsens cellular damage. Experimental data reveal that 20 Hz compressional wave EA at Baihui (GV20) and Zusanli (ST36) acupoints for 30 min activates PTEN-induced putative kinase 1 (PINK1)/Parkin-dependent mitophagy, effectively counteracting nitro-oxidative stress-mediated mitochondrial dysfunction in CIRI (Wang et al., 2019; Figure 2). Pink1, a central regulator of mitophagy, undergoes activation in response to mitochondrial dysfunction, subsequently recruiting Parkin to orchestrate mitochondrial clearance through selective autophagy (Ji et al., 2022). The pathophysiological triad of oxidative stress, inflammatory cascades, and autophagic dysregulation exhibits reciprocal potentiation, forming a self-amplifying circuit that escalates cytopathological damage. Strategic therapeutic intervention targeting this interconnected network demonstrates enhanced potential for achieving multidimensional neuroprotection in ischemia-reperfusion pathologies. Dysfunctional mitochondria constitute primary generators of ROS in oxidative stress. Controlled mitophagy exerts cytoprotective effects by selectively eliminating compromised mitochondria and inhibiting ROS overproduction, effectively attenuating pathological oxidative stress propagation and downstream inflammatory cascades.
The precise mechanisms underlying EA dual modulation of autophagic activity and mitigation of autophagy-mediated cytopathology remain systematically uncharacterized. Current evidence suggests these regulatory processes exhibit inherent complexity and multifactorial interactions. Deciphering such mechanisms holds significant potential to enhance the efficacy of EA-based therapeutic strategies for CIRI. Targeted investigations are required to map the molecular circuitry governing autophagic regulation and its pathophysiological crosstalk in neural injury contexts.
Regulation of autophagy by EA is one of the main targets to improve CIRI, mainly by regulating the expression of autophagy-related proteins, and autophagy-related genes to achieve the prevention of damage produced by excessive autophagy in neuronal cells, but part of autophagy, such as mitophagy, is up-regulated by EA, and it can prevent the occurrence of oxidative stress.
3.1.2 Oxidative stress
Oxidative stress manifests as a pathophysiological imbalance wherein pro-oxidant forces override antioxidant defenses, disrupting cellular redox equilibrium. In biological systems, endogenous antioxidant enzymes constitute the primary molecular machinery responsible for counteracting oxidative damage and maintaining metabolic homeostasis. Antioxidant defense mechanisms encompass multiple molecular regulators and signaling pathways, notably the nuclear factor E2-related factor 2, Kelch-like ECH-associated protein 1, PI3K/AKT cascade, mitogen-activated protein kinase, NF-κB inflammatory pathway, and heme oxygenase-1 enzymatic system, which collectively maintain redox homeostasis through coordinated transcriptional regulation and stress response modulation (Forman and Zhang, 2021; Wang et al., 2022b).
Oxidative stress plays a central role in CIRI; its pathological dominance constitutes a primary therapeutic target (Huang G. et al., 2022). CIRI induces redox imbalance through two principal mechanisms: ROS overproduction and compromised antioxidant enzymatic defenses. Pathological suppression of the Wnt/β-catenin pathway exemplifies this process, as it triggers mitochondrial complex I dysfunction and ROS overgeneration. This propagates mitochondrial destruction, culminating in programed neuronal death (Zhang et al., 2024).
EA demonstrates significant efficacy in ameliorating oxidative stress injury following cerebral ischemia-reperfusion (Tang et al., 2024). Experimental studies reveal that EA preconditioning attenuates redox imbalance through coordinated suppression of transient receptor potential vanilloid 1 activation, downregulation of cytochrome C, and biomarker regulation of MDA, glutathione (GSH), and SOD levels (Figure 3; Long et al., 2019). Cheng et al. demonstrated that MAPKs serve as central regulators of intracellular oxidative stress post-cerebral ischemia. Mechanistically, p38 MAPK activation upregulates survival proteins Bcl-2 and B-cell lymphoma-extra-large (Bcl-xL) through cAMP-responsive element-binding protein-dependent transcriptional regulation, while concurrently inducing mitochondrial permeability transition pore opening - a critical determinant of mitochondrial apoptotic signaling. Mitochondrial permeability transition pore opening enables X-linked inhibitors of apoptosis protein family members to bind caspases, directly modulating apoptotic signaling pathways (Figure 3). Experimental data further indicate that frequency-specific EA elevates the Bcl-2/Bcl-2-associated X protein (Bax) and Bcl-xL/Bax ratios, stabilizing mitochondrial membrane integrity post-CIRI through anti-apoptotic protein dominance, thereby conferring neuroprotective efficacy (Cheng et al., 2015). EA exerts dual regulatory effects on astrocytes and microglia through targeted molecular interventions. Specifically, EA suppresses astrocytic S100B secretion — a critical mediator that upregulates inducible nitric oxide synthase (iNOS) expression and drives pathological nitric oxide (NO) overproduction in glial cells at elevated concentrations, ultimately triggering neurotoxic NO diffusion. This coordinated modulation attenuates nitro-oxidative stress propagation and suppresses proinflammatory cytokine release, demonstrating EA’s capacity to disrupt the S100B/iNOS/NO neurotoxic axis while preserving neural homeostasis (Cheng et al., 2014).
While the precise mechanisms underlying EA regulation of redox homeostasis require further elucidation, its capacity to mitigate CIRI through coordinated ROS suppression and enzymatic antioxidant system modulation is well-established. Current evidence reveals substantial pathway convergence among EA’s antioxidant, anti-inflammatory, and anti-apoptotic effects. Critical nodal regulators like the MAPK signaling cascade demonstrate multifunctional therapeutic potential, simultaneously addressing oxidative stress propagation, neuroinflammation amplification, and programed cell death initiation post-CIRI. This mechanistic overlap highlights this regulator as a central role in EA-mediated neural protection, positioning it as a prime translational target for developing multimodal interventions against ischemia-reperfusion pathologies.
MAPK and cytochrome C are relatively important intracellular targets in recent studies, acting by affecting the production of oxidative substances and regulating oxidase production, while in glial cells EA also reduces the production of substances that contribute to oxidative stress, such as S100B.
3.1.3 Neuroinflammation
In CIRI, inflammation is initiated through endogenous signaling, wherein damaged tissues release molecular mediators that activate innate immune responses. Plasma membrane rupture in necrotic cells triggers the extracellular release of substances, including ATP, potassium ions, uric acid, high-mobility group box 1 protein, and S100 calcium-binding proteins. The release of these substances activates multiple inflammasome complexes, including NLRP3 and NALP3, while simultaneously triggering Toll-like receptor-mediated caspase-1 activation. This signaling mechanism propagates inflammatory cascades and induces pyroptotic cell death. ROS, central to oxidative stress, further amplifies inflammation through mechanisms such as oxidized lipoprotein-induced inflammatory signaling (Jayaraj et al., 2019). Key inflammatory mediators including tumor necrosis factor-α (TNF-α), NF-κB, and interleukin-1β (IL-1β) critically regulate inflammatory cascade activation in CIRI (Cao et al., 2021).
EA significantly inhibits inflammatory responses in CIRI. Experimental studies employing focal cerebral ischemia models in male Sprague-Dawley rats demonstrated that EA suppresses NLRP3 inflammasome activation in penumbral tissues post-reperfusion, as quantified by Western blot and immunofluorescence analyses. Mechanistically, EA activates α7 nicotinic acetylcholine receptor signaling to block NLRP3 inflammasome assembly, thereby attenuating neuroinflammation (Jiang et al., 2019). EA directly inhibits the production of major inflammatory factors, including TNF-α, IL-6, IL-1β, and Ccl-2 mRNA, exerting an anti-inflammatory effect within the cell (Ren et al., 2024). It also modulates immune cells such as Tregs and γδ T-cells, which suppress other T-cells, B-cells, and pro-inflammatory factors through this pathway, thereby reducing inflammation (Wang Y. et al., 2023).
Recent studies suggest that EA is closely associated with both anti-apoptotic and anti-inflammatory mechanisms, involving pathways that simultaneously downregulate inflammatory factors and exert inflammatory-trophic effects. For example, in a brain ischemia-reperfusion model, EA treatment was evaluated using cell fluorescent dye and real-time quantitative polymerase chain reaction to assess mRNA levels. The expression of brain-derived neurotrophic factor (BDNF) was measured via western blot or enzyme-linked immunosorbent assay in vitro, revealing that EA induced delta-opioid receptor activation and improved cell morphology.
It reduced cell apoptosis and downregulated proinflammatory cytokine expression. Additionally, the delta-opioid receptor inhibited inflammation through the BDNF/tropomyosin-related kinase B pathway (Geng et al., 2020). BDNF has anti-inflammatory effects and can inhibit neuronal apoptosis (Gu W. et al., 2022). However, the specific anti-inflammatory mechanism of EA remains insufficiently studied. Future research clarifying its mechanism of action will enhance the clinical application of this traditional Chinese medicine-based treatment, enabling more targeted therapeutic effects.
3.1.4 Ferroptosis
EA can play a protective role against CIRI and ameliorate it through the inhibition of neuronal ferroptosis. Some studies (Liang et al., 2022; Yao et al., 2021) demonstrate that ferroptosis, an iron-dependent form of programed cell death caused by lipid peroxide accumulation, plays a critical role in the development of neurological diseases. This process is closely related to intracellular GSH deficiency and ROS production. Glutathione peroxidase 4 (GPX4) is a critical inhibitor of ferroptosis, and the GSH/GPX4 system reduces lipid hydroperoxides to 12/15-lipoxygenase (12/15-LOX) under oxidative stress (Liu S. et al., 2023), activating the transferrin receptor (TFRC) to enhance ferrous ion (Fe2+) uptake (Lu et al., 2021). GSH biosynthesis is critical for the functional activity of GPX4. When GSH levels are depleted, GPX4 becomes inactivated, leading to ROS accumulation and lipid peroxidation, which at a certain threshold triggers cellular ferroptosis (Ou et al., 2022). Biochemically, the Fenton reaction between excess free Fe2+ and hydrogen peroxide, a product of mitochondrial oxidative respiration, triggers a significant increase in ROS, which inhibits GSH synthesis and GPX4 activity. This leads to impaired antioxidant systems, redox imbalances, and a buildup of toxic lipid peroxides, ultimately contributing to the ferroptosis of cells (Jiang et al., 2021; Lei et al., 2019; Li et al., 2020; Xie et al., 2022). The approximate process of ferroptosis described above can be seen in Figure 4.
Li et al. demonstrated that EA has a ferroptosis-inhibitory effect in MCAO rats (Li et al., 2021). The study showed that EA enhances GPX4 and SOD activities, as well as GSH levels while decreasing MDA and iron accumulation. EA was also found to increase the levels of FTH1 and decrease the levels of Tf (transferrin) and TfR1. These results suggest that EA alleviates ICS (ischemic stroke) by inhibiting ferroptosis. Moreover, Wang G. et al. (2023) found that EA significantly reduced the expression of ACSL4 and TFR1, increased GPX4 levels, and inhibited ROS production in MCAO/R model rats. Furthermore, EA treatment improved neurological deficits and reduced infarct volume. These findings suggest that EA may alleviate CIRI by inhibiting ferroptosis. The results from these animal studies indicate that EA holds the potential for mitigating CIRI through ferroptosis inhibition.
GSH, GPX4, and ROS are keys to the occurrence of ferroptosis in neurons. EA can inhibit neuronal ferroptosis after cerebral ischemia/reperfusion and attenuate cerebral ischemia/reperfusion injury by decreasing the content of GSH and ROS and downregulating the expression of GPX4.
3.1.5 Neuronal apoptosis (apoptosis-related protein balance)
EA can exert anti-neuronal apoptosis by regulating the balance of apoptosis-related proteins, thereby demonstrating therapeutic potential in improving CIRI (Ma et al., 2016).
Firstly, EA treatment can prevent neuronal apoptosis by regulating the balance of Bcl-2 and Bax proteins. Bcl-2 and Bax are key proteins in the Bcl-2 family, closely associated with apoptosis. Bcl-2 is a membrane-stabilizing protein that is associated with organelle membranes. By inhibiting free radical production and intracellular calcium overload, this treatment prevents mitochondrial membrane permeability, maintains membrane stability, and blocks cytochrome C release and caspase activation, thereby inhibiting apoptosis. Bcl-2 overexpression reduces cellular damage, while Bax exhibits an antagonistic role, promoting apoptosis. Bcl-2 and Bax can form heterodimers. When Bcl-2 expression is increased, Bcl-2 homodimers are formed, which protect cells. Conversely, when Bax expression is increased, Bax/Bcl-2 heterodimers form, leading to apoptosis (Alam et al., 2022; Moldoveanu and Czabotar, 2020; Wolf et al., 2022). Therefore, the Bcl-2/Bax ratio plays a significant role in determining apoptosis in neurons and other cells. It has been shown that in the MCAO-induced cerebral ischemia/reperfusion rat model, EA treatment significantly enhanced the Bcl-2/Bax ratio, reduced the number of apoptotic cells, and alleviated the degree of neurological impairment. Additionally, EA improved cognitive deficits in ischemia/reperfusion-injured rats by regulating Bcl-2 and Bax expression (Liu et al., 2015). This finding is consistent with the experimental study by Ma et al. (2016), which demonstrated that EA stimulation in MCAO rats up-regulated Bcl-2 protein expression, down-regulated Bax protein expression and significantly reduced the number of apoptotic cells. These results suggest that EA may mitigate neuronal apoptosis following CIRI by modulating the balance between Bax and Bcl-2 proteins.
Secondly, EA can also reduce CIRI by regulating caspase-3 expression, an apoptotic execution factor that exists in normal cells as a zymogen. During cerebral ischemia, caspase-3 activation triggers a series of events, including nuclear factor activation, cytoskeletal protein disruption, and DNA cleavage. These processes lead to cell morphological changes, apoptotic vesicle formation, and ultimately apoptosis (Sahoo et al., 2023; Unnisa et al., 2023; Vatandoust et al., 2023). Meng et al. (2024) demonstrated that EA treatment in MCAO rats significantly upregulated the mRNA and protein expression of the Bcl-2 gene in the ischemic brain, thereby downregulating caspase-3, Bax, and cleaved caspase-3. This led to a reduction in apoptosis of the ischemic brain tissue in stroke rats and attenuation of CIRI in MCAO rats. EA treatment enhances neuroprotection by promoting the expression of protective proteins and inhibiting pro-apoptotic factors, resulting in improved outcomes for cerebral ischemia-reperfusion injury.
In addition, studies have demonstrated that EA may inhibit neuronal apoptosis by activating the PI3K/Akt pathway, thereby protecting brain tissue from CIRI (Rao et al., 2025; Wang et al., 2021). Additionally, EA enhances BDNF expression, further exerting anti-apoptotic, neuroprotective effects in CIRI (Geng et al., 2020; Li et al., 2022). These mechanisms are summarized in Figure 5.
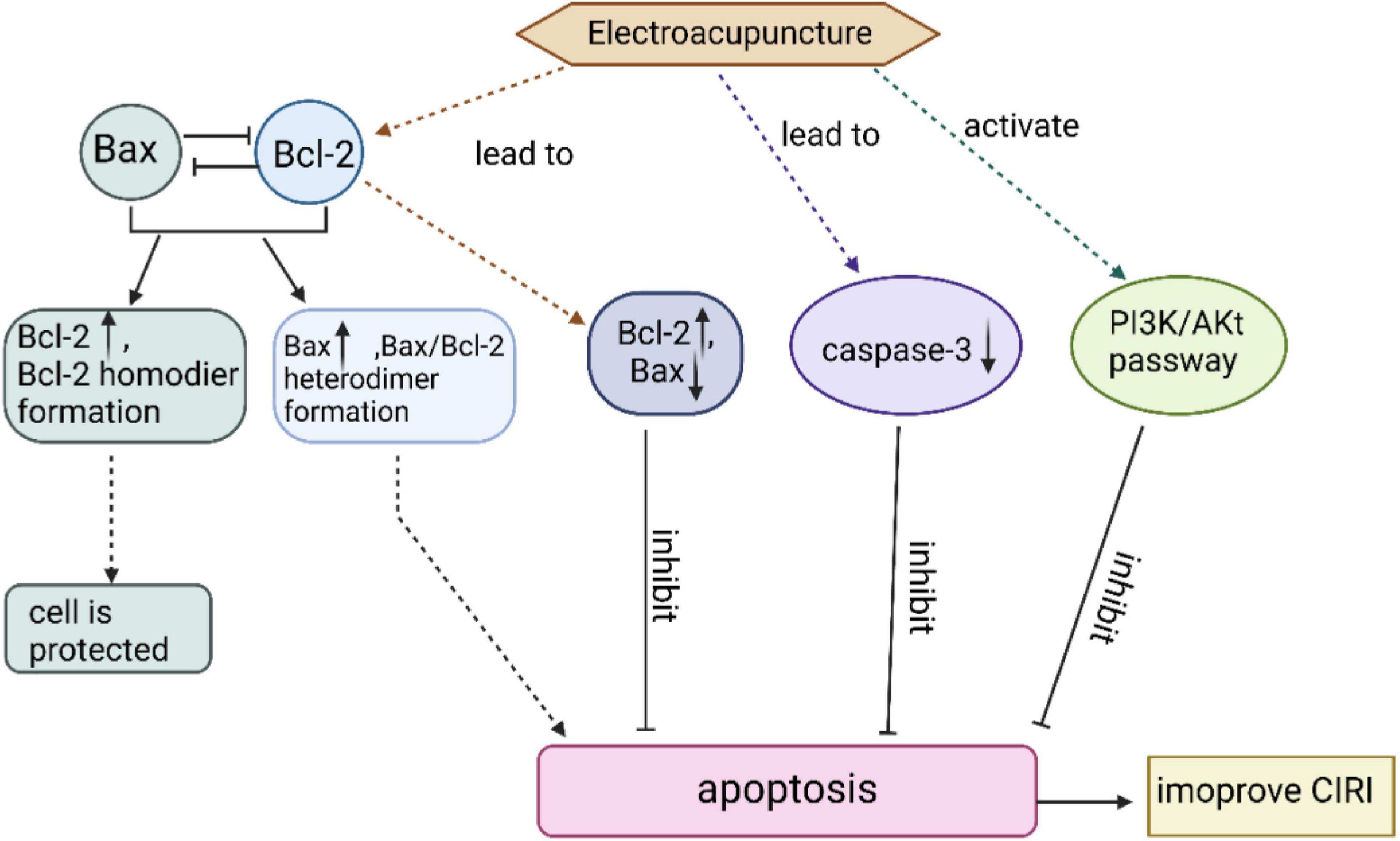
Figure 5. EA can inhibit neuronal apoptosis and ameliorate brain tissue injury after CIRI through three mechanisms.
Overexpression of Bcl-2 attenuates cell damage, whereas Bax acts in the opposite direction to Bcl-2 and has a pro-apoptotic effect. EA intervention in MCAO rats up-regulated Bcl-2 protein expression and down-regulated Bax protein expression, which significantly reduced the number of apoptotic cells and exerted neuroprotective effects. caspase-3 is an apoptotic execution factor that triggers cell apoptosis. EA can inhibit apoptosis in the ischemic brain tissue of stroke rats by reducing the expression of caspase-3. EA can also activate the PI3K/Akt pathway, causing a series of responses to inhibit neuronal apoptosis after CIRI.
3.1.6 Excitotoxic injury (high calcium permeability of NMDARs)
CIRI leads to excitotoxic injury, primarily due to the excessive release of excitatory amino acids. This process causes neuronal apoptosis and neurotoxic injury, characterized by increased glutamate release. Glutamate (Glu), an excitatory neurotransmitter, activates NMDA receptors (NMDARs). The excessive release of glutamate into the synaptic gap triggers increased calcium influx through NMDARs (NMDA receptors), which is central to the toxic damage (Liao et al., 2023).
Under pathological conditions such as cerebral ischemia, glutamate is excessively released into the synaptic gap, which then activates the NR2B-containing NMDAR (GluN2B), leading to intracellular calcium overload. The overloaded calcium activates cytoplasmic m-calpain, which triggers the enzymatic activity of STEP61, further inhibits p38 MAPK dephosphorylation, and ultimately leads to apoptosis through signal transduction (Huang W. et al., 2022; Jang et al., 2014). This process is illustrated in Figure 6. Therefore, inhibition of glutamate over-release is very important for mitigating CIRI. It has been reported in the literature (Zhang et al., 2018) that EA administration in MCAO rat models increased NMDAR2A protein expression in the hippocampus compared to the MCAO group. It also reduced Glu and NMDAR2B protein levels and decreased intracellular Ca2+ levels in the MCAO rats. This helped protect the hippocampus and attenuate the CIRI caused by MCAO. The mechanism behind this effect includes the reduction of NMDAR2B expression and the decrease of Ca2+-related neurotoxicity by inhibiting Glu release. Zhang et al. (2020) demonstrated that EA pretreatment down-regulated the GluN2B expression, reduced m-calpain activity, and inhibited p38 MAPK phosphorylation in hippocampal CA1 neurons. These findings suggest that EA suppresses the GluN2B/m-calpain/p38 MAPK pathway, thereby reducing brain damage following CIRI. The proapoptotic pathway of GluN2B/m-calpain/p38 MAPK effectively reduced the volume of cerebral infarction and the level of neuronal apoptosis after CIRI.
Following CIRI, EA reduced excitotoxicity brought on by calcium overload in brain tissues and prevented glutamate release. Pretreatment with EA may be useful in lowering the amount of cerebral infarction and the degree of neuronal death during CIRI by blocking the GluN2B/m-calpain/p38 MAPK pro-apoptotic pathway.
In addition, NMDARs exhibit two subtypes, NMDAR2A and NMDAR2B, which have opposing roles. NMDAR2A activation promotes neuronal regeneration and protects neuronal function, while NMDAR2B mediates the free radical chain reaction, inducing neurotoxicity and apoptosis (Goldsmith, 2019; Skowrońska et al., 2019). Zhang et al. showed that EA enhanced NMDAR2A expression and down-regulated NMDAR2B expression, thereby alleviating CIRI. This modulation significantly influenced cognitive recovery post-stroke in a rat model (Zhang et al., 2018).
Previous studies have shown that while reducing glutamate release or enhancing glutamate reuptake are effective strategies to counter ischemic injury, most drugs in this category are inefficient in reducing morbidity or mortality and exhibit neurological side effects in stroke clinical trials. Targeted therapies and interventions based on the “NMDAR” subtype hypothesis have also shown limited efficacy and significant side effects in preclinical studies (Mao et al., 2022). Therefore, the current study on EA for CIRI highlights a potential mechanism to address excitotoxic injury, which could address the limitations of existing ischemic stroke therapies. Moreover, EA intervention is free of drug toxicity and has minimal neurological side effects, offering promise for broader clinical application in the foreseeable future.
3.2 Promotion effect
3.2.1 Blood-brain barrier
EA intervention may also ameliorate CIRI by protecting the blood-brain barrier. Cerebral ischemia/reperfusion can cause and aggravate cerebral edema by increasing the expression of Aquaporin-4 (AQP4), a water channel protein closely associated with cerebral edema formation (Vandebroek and Yasui, 2020). Taniguchi et al. (2000) found that after focal cerebral ischemia in rats, cerebral edema worsened over time and the expression of AQP4 protein and mRNA was in line with the time of aggravation of cerebral edema. The changes in AQP4 mRNA expression in MCAO were closely related to the changes in edema formation and regression. Xu et al. (2014) demonstrated that EA significantly attenuated the expression of water channel protein AQP4 in the ischemic brain, effectively alleviating cerebral edema symptoms in rats following cerebral ischemia and reperfusion. Studies have also shown that AQP4 is closely related to the BBB (Mueller et al., 2023). Neural astrocyte peduncles contribute to the BBB’s structure by forming a glial border membrane around capillaries, acting as the BBB’s second barrier. This structure aids in regulating water transport, with AQP4 concentrated in the peduncles of glial cells. Under cerebral ischemia, AQP4 expression on astrocyte membranes is enhanced. AQP4 channels open abundantly, leading to cerebral edema formation and progression. Therefore, EA downregulates AQP4 expression, thereby reducing astrocyte peduncle swelling and restricting AQP4 activity. This leads to BBB protection, cerebral edema alleviation, and improved outcomes in CIRI.
Additionally, EA may improve CIRI by down-regulating Matrix metalloproteinase 2 (MMP2)/AQP4/APQ9 expression. MMPs belong to the family of Zn2+-dependent enzymes associated with BBB permeability and disruption (Lu and Wen, 2025; Sun et al., 2023), and MMP2 plays an important role in brain water transport homeostasis, as do AQP4 and AQP9. All three are associated with the permeability of the BBB in CIRI. BBB disruption, tissue inflammation, and MMP/AQP up-regulation together trigger cerebral edema/swelling after CIRI. EA mitigates this condition by regulating MMP2/AQP4/AQP9 activity, thereby exerting a neuroprotective effect (Xu et al., 2014).
MMP-9, a collagenase in the MMP family, degrades the extracellular matrix and contributes to BBB disruption during CIRI (Gu Y. et al., 2022). In contrast, some studies have shown that MMP-9 expression in the brain of MCAO rats pretreated with EA was significantly reduced, suggesting that EA pretreatment may maintain the integrity of the blood-brain barrier after injury by inhibiting MMP-9 expression, reduce the brain tissue damage, and improve the cerebral function (Lin et al., 2016).
3.2.2 Angiogenesis
Following CIRI, the microvasculature of the cerebral cortex is damaged, resulting in neuronal death in the ischemic central zone. Neurons in the ischemic hemiparetic zone are subjected to ischemia and hypoxia, leading to neuronal damage and functional impairments (Wang et al., 2018). In this case, angiogenesis in ischemic areas is crucial for reducing the damage and improving prognosis. Angiogenesis not only increases collateral circulation to reduce ischemia-induced brain damage but also plays a key role in restoring neurological function after ischemic stroke (Shi et al., 2017). Angiogenesis is regulated by several vascular growth factors, including vascular endothelial growth factor (VEGF) and erythropoietin (EPO). Previous studies have shown that VEGF is a key cytokine associated with the differentiation, proliferation, and migration of endothelial cells. By binding to its receptor (VEGFR), VEGF activates downstream signaling pathways and transmits angiogenic signals (Wang H. et al., 2020). Therefore, promoting the expression of VEGF and VEGFR is important in angiogenesis after brain injury. In this regard, studies have shown that EA can promote neovascularization after focal cerebral ischemia/reperfusion by up-regulating VEGF expression, reducing the damage of the vascular wall induced by ischemia/reperfusion, and exerting a protective effect against ischemia/reperfusion-induced brain injury (Zhang W. et al., 2023). EPO exhibits multiple functions, including erythropoiesis promotion, anti-inflammatory, antioxidant, pro-angiogenic, and neurotrophic effects (Alural et al., 2014; Si et al., 2019). EPO activates its function by binding to the EPO receptor (EPOR). Importantly, both EPO and EPOR are expressed in red lineage cells, neurons, astrocytes, and cerebrovascular endothelial cells (Tsiftsoglou, 2021). The non-receptor tyrosine kinase Src plays an important role in angiogenesis, and increased levels of Src phosphorylation have a protective effect against ischemia-induced brain damage (Sun et al., 2021). Wang et al. (2022a) showed that EA increased the expression of EPO, VEGF and P-Src proteins. This effect may involve EPO-mediated activation of the Src signaling pathway and VEGF signaling pathway, thereby promoting angiogenesis. Notably, EPO activates the Src pathway, which further increases VEGF expression (Figure 7), amplifying pro-angiogenic effects. This process facilitates vascular neogenesis, enhancing blood flow and oxygen supply in ischemic brain areas, and alleviates CIRI.
In CIRI rat brain tissue, EA stimulates the VEGF and Src signaling pathways, which are mediated by EPO, to encourage angiogenesis. Moreover, activation of the Src signaling pathway by EPO contributes to the increase in VEGF expression, which further enhances the pro-angiogenic effect and facilitates neovascularization, thereby attenuating CIRI.
Moreover, EA may also promote the mobilization of endothelial progenitor cells (EPCs) to ischemic areas and promote vascular neogenesis. EPCs are a group of naïve endothelial cells with wandering characteristics and capable of further proliferation and directional differentiation. Their main function is to participate in vascular endothelial repair and neointimal formation in ischemic organs (Kong et al., 2018; Shi et al., 2023). After the ischemic injury, EPCs in bone marrow may mobilize, enter the peripheral circulation, and migrate to the ischemic site to participate in vascular endothelialisation and angiogenesis. EA promotes the mobilization of EPCs in the bone marrow and their migration in the peripheral blood, which facilitates angiogenesis after cerebral ischemia/reperfusion and improves injury symptoms (Lu et al., 2013; Xie et al., 2016).
EA’s key advantage in CIRI treatment lies in its ability to stimulate cerebral angiogenesis at injury sites. Unlike existing ischemic stroke treatments, fewer drugs target cerebral angiogenesis. EA offers a promising new therapeutic approach for the clinical treatment of ischemic stroke.
4 Conclusion
After thrombolysis or thrombectomy for cerebral ischemic stroke, the infarcted blood vessel is reopened, and the blood supply is restored. However, this process triggers a series of cellular responses that exacerbate the damage to the ischemic tissue, known as CIRI. EA is an enhanced traditional Chinese medicine technique that provides protective mechanisms for the brain. These mechanisms include but are not limited to, modulating neuroinflammatory responses, regulating cellular autophagy, and reducing oxidative stress.
The beneficial effects of EA on CIRI are evident across multiple dimensions, particularly in its antioxidative stress and anti-inflammatory responses. These two effects are interdependent and synergistic, collectively reducing the production of inflammatory factors and mitigating the inflammatory response. Concurrently, EA alleviates the accumulation of ROS and the occurrence of oxidative stress. Although the exact mechanism by which EA regulates autophagy remains unclear and some experimental results appear contradictory, with controlled conditions, a consistent mechanism and pattern can be discerned. Regulation of autophagy in neuronal cells, glial cells, and intracellular organelles has emerged as a significant target for EA therapy. EA can reverse cell death and apoptosis caused by excessive autophagy in neuronal cells after injury while up-regulating autophagy in intracellular mitochondria helps clear cytotoxic substances. Despite the current uncertainty regarding the regulation of autophagy and the selection of autophagic targets, the phenomenon observed in EA therapy is promising and suggests substantial therapeutic potential. Investigating the detailed mechanisms of autophagy regulation by EA is crucial. Autophagy is intricately linked to both antioxidative and anti-inflammatory mechanisms. For instance, autophagy-related pathways can generate essential ROS for the inflammatory response and oxidative stress. These mechanisms are interconnected rather than isolated. By leveraging these amplifying effects, we can identify central targets and substances, such as MAPK and ROS, which interact and regulate related pathways to alleviate CIRI. This integrated approach holds significant promise for enhancing the therapeutic efficacy of EA in treating CIRI.
The mechanisms by which EA improves CIRI are interconnected and multifaceted. Oxidative stress induced by CIRI contributes to cellular ferroptosis, and inhibiting neuronal ferroptosis can mitigate oxidative stress damage. EA promotes angiogenesis, which enhances blood and oxygen supply to brain tissues and helps protect the blood-brain barrier. Therefore, the mechanisms of EA should not be viewed in isolation. However, current studies often focus narrowly on specific pathways or proteins, and most use MCAO rat models, limiting the direct applicability to human treatment. In the design of research experiments, there are issues related to inadequate sample sizes and inconsistencies in EA points and frequencies. In studies examining EA for stroke treatment, animal experiment sample sizes typically range from 30 to 100, which is generally considered small. This limited sample size may not accurately represent the overall effectiveness of EA therapy, leading to restricted applicability of the findings. Additionally, the lack of standardized EA parameters, such as points, frequency, and intensity, complicates comparisons across different studies, thereby impacting the reliability and reproducibility of the results. Future experimental designs should focus on varying acupoints, stimulation durations, and treatment lengths to identify the most effective treatment protocols, thereby controlling EA-related variables and enhancing the study’s quality. Furthermore, future research could increase sample sizes and consider using primate models to improve the experimental animal models. Nonetheless, EA treatment and pretreatment have both demonstrated significant improvement in CIRI in experimental animals, highlighting the potential importance of this research for stroke and related brain diseases. These findings offer new avenues for stroke intervention and treatment, providing hope for improving cognitive and memory functions post-CIRI in humans. Future research should aim to elucidate the precise molecular mechanisms through which EA exerts its protective effects on CIRI. This includes detailed studies on how EA regulates autophagy and its impact on cellular pathways involved in oxidative stress, inflammation, and ferroptosis. Integrating advanced techniques such as RNA sequencing and proteomics can help identify novel targets and pathways influenced by EA.
Additionally, clinical trials are essential to validate EA’s efficacy and safety in human patients. Combining traditional Chinese medicine with modern biomedical research could lead to innovative treatments for stroke and other ischemic brain injuries. Integrating EA into standard clinical practice could revolutionize condition management, significantly improving global patient outcomes. As a traditional Chinese medicine therapeutic method, EA has many unknown mechanisms. Investigating its molecular pathways could uncover new therapeutic mechanisms. EA has advantages, including minimal harm and side effects, a holistic effect on ischemic stroke improvement, and use as a complement to existing therapies for neuroprotection. It is also effective as a post-stroke treatment for neurological injuries and has broad clinical applications. Neuroprotective agents play a crucial role in the current therapeutic approach to ischemic stroke. The current generation of drugs targets inflammatory, excitotoxic, and oxidative stress signaling pathways, including human urinary kallidinogenase and ginkgolides (Paul and Candelario-Jalil, 2021). These mechanisms are analogous to those induced by EA interventions. Consequently, EA can serve as a supplementary enhancement or even an alternative to neuroprotective agents, thereby achieving multi-targeted and multi-effective effects that are challenging to attain with individual neuroprotective agents. It can be used as an enhanced complementary or even alternative treatment to neuroprotective agents, exerting multi-target and multi-effective effects that are difficult to realize by a single neuroprotective agent. This approach reduces the use of chemical drugs and improves the prognosis of stroke patients. There is optimism that EA will be formally introduced into clinical practice, offering new hope to patients worldwide.
Author contributions
JZ: Writing – original draft. XG: Writing – original draft. YC: Writing – original draft. RX: Writing – review & editing. XD: Writing – review & editing.
Funding
The author(s) declare that financial support was received for the research and/or publication of this article. This study was supported by The Science and Technology Plan of Jiangxi Provincial Health Commission (20204369).
Acknowledgments
We sincerely appreciated the guidance from our tutors and the work of every member of our team. The graphical abstracts were created with BioRender software (http://BioRender.com).
Conflict of interest
The authors declare that the research was conducted in the absence of any commercial or financial relationships that could be construed as a potential conflict of interest.
Publisher’s note
All claims expressed in this article are solely those of the authors and do not necessarily represent those of their affiliated organizations, or those of the publisher, the editors and the reviewers. Any product that may be evaluated in this article, or claim that may be made by its manufacturer, is not guaranteed or endorsed by the publisher.
References
Ahsan, A., Liu, M., Zheng, Y., Yan, W., Pan, L., Li, Y., et al. (2021). Natural compounds modulate the autophagy with potential implication of stroke. Acta Pharm. Sin. B 11, 1708–1720. doi: 10.1016/j.apsb.2020.10.018
Alam, M., Alam, S., Shamsi, A., Adnan, M., Elasbali, A., Al-Soud, W., et al. (2022). Bax/Bcl-2 cascade is regulated by the EGFR pathway: Therapeutic targeting of non-small cell lung cancer. Front. Oncol. 12:869672. doi: 10.3389/fonc.2022.869672
Alural, B., Duran, G., Tufekci, K., Allmer, J., Onkal, Z., Tunali, D., et al. (2014). EPO mediates neurotrophic, neuroprotective, anti-oxidant, and anti-apoptotic effects via downregulation of miR-451 and miR-885-5p in SH-SY5Y neuron-like cells. Front. Immunol. 5:475. doi: 10.3389/fimmu.2014.00475
Bersano, A., and Gatti, L. (2023). Pathophysiology and treatment of stroke: Present status and future perspectives. Int. J. Mol. Sci. 24:14848. doi: 10.3390/ijms241914848
Candelario-Jalil, E., Dijkhuizen, R., and Magnus, T. (2022). Neuroinflammation, Stroke, blood-brain barrier dysfunction, and imaging modalities. Stroke 53, 1473–1486. doi: 10.1161/STROKEAHA.122.036946
Cao, Y., Wang, L., Lin, L., Wang, X., Ma, S., Yang, N., et al. (2021). Acupuncture attenuates cognitive deficits through α7nAChR mediated anti-inflammatory pathway in chronic cerebral hypoperfusion rats. Life Sci. 266:118732. doi: 10.1016/j.lfs.2020.118732
Cheng, C., Lin, J., Tang, N., Kao, S., and Hsieh, C. (2014). Electroacupuncture-like stimulation at the Baihui (GV20) and Dazhui (GV14) acupoints protects rats against subacute-phase cerebral ischemia-reperfusion injuries by reducing S100B-mediated neurotoxicity. PLoS One 9:e91426. doi: 10.1371/journal.pone.0091426
Cheng, C., Lin, J., Tang, N., Kao, S., and Hsieh, C. (2015). Electroacupuncture at different frequencies (5Hz and 25Hz) ameliorates cerebral ischemia-reperfusion injury in rats: Possible involvement of p38 MAPK-mediated anti-apoptotic signaling pathways. BMC Complement. Altern. Med. 15:241. doi: 10.1186/s12906-015-0752-y
Du, J., Yin, G., Hu, Y., Shi, S., Jiang, J., Song, X., et al. (2020). Coicis semen protects against focal cerebral ischemia-reperfusion injury by inhibiting oxidative stress and promoting angiogenesis via the TGFβ/ALK1/Smad1/5 signaling pathway. Aging 13, 877–893. doi: 10.18632/aging.202194
Feigin, V., Brainin, M., Norrving, B., Martins, S., Sacco, R., Hacke, W., et al. (2022). World stroke organization (WSO): Global stroke fact sheet 2022. Int. J. Stroke 17, 18–29. doi: 10.1177/17474930211065917
Forman, H., and Zhang, H. (2021). Targeting oxidative stress in disease: Promise and limitations of antioxidant therapy. Nat. Rev. Drug Discov. 20, 689–709. doi: 10.1038/s41573-021-00233-1
Franke, M., Bieber, M., Kraft, P., Weber, A., Stoll, G., and Schuhmann, M. (2021). The NLRP3 inflammasome drives inflammation in ischemia/reperfusion injury after transient middle cerebral artery occlusion in mice. Brain Behav. Immun. 92, 223–233. doi: 10.1016/j.bbi.2020.12.009
Geng, Y., Chen, Y., Sun, W., Gu, Y., Zhang, Y., Li, M., et al. (2020). Electroacupuncture ameliorates cerebral I/R-induced inflammation through DOR-BDNF/TrkB pathway. Evid. Based Complement. Alternat. Med. 2020:3495836. doi: 10.1155/2020/3495836
Goldsmith, P. J. (2019). NMDAR PAMs: Multiple chemotypes for multiple binding sites. Curr. Top. Med. Chem. 19, 2239–2253. doi: 10.2174/1568026619666191011095341
Gu, W., Bao, C., Xie, H., Chen, B., Lin, W., Wu, Y., et al. (2022). [Effect of electroacupuncture invention on activities of PGC-1α/Irisin (FNDC5)/BDNF signaling in cerebral cortex, hippocampus and muscles in focal cerebral ischemic/reperfusion injury rats]. Zhen Ci Yan Jiu. 47, 428–434. doi: 10.13702/j.1000-0607.20210495
Gu, Y., Zhou, C., Piao, Z., Yuan, H., Jiang, H., Wei, H., et al. (2022). Cerebral edema after ischemic stroke: Pathophysiology and underlying mechanisms. Front. Neurosci. 16:988283. doi: 10.3389/fnins.2022.988283
Hilkens, N., Casolla, B., Leung, T., and de Leeuw, F. (2024). Stroke. Lancet 403, 2820–2836. doi: 10.1016/S0140-6736(24)00642-1
Huang, G., Zang, J., He, L., Zhu, H., Huang, J., Yuan, Z., et al. (2022). Bioactive nanoenzyme reverses oxidative damage and endoplasmic reticulum stress in neurons under ischemic stroke. ACS Nano 16, 431–452. doi: 10.1021/acsnano.1c07205
Huang, W., Wang, Z., Wang, G., Li, K., Jin, Y., and Zhao, F. (2022). Disturbance of glutamate metabolism and inhibition of CaM-CaMKII-CREB signaling pathway in the hippocampus of mice induced by 1,2-dichloroethane exposure. Environ. Pollut. 310:119813. doi: 10.1016/j.envpol.2022.119813
Jang, J., Choi, Y., Kim, H., Kim, Y., Hong, J., Bae, D., et al. (2014). Neuroprotective effects of a novel single compound 1-methoxyoctadecan-1-ol isolated from Uncaria sinensis in primary cortical neurons and a photothrombotic ischemia model. PLoS One 9:e85322. doi: 10.1371/journal.pone.0085322
Jayaraj, R., Azimullah, S., Beiram, R., Jalal, F., and Rosenberg, G. (2019). Neuroinflammation: Friend and foe for ischemic stroke. J. Neuroinflammation 16, 142. doi: 10.1186/s12974-019-1516-2
Ji, Z., Shi, Y., Li, X., Hou, R., Yang, Y., Liu, Z., et al. (2022). Neuroprotective effect of taohong siwu decoction on cerebral ischemia/reperfusion injury via mitophagy-NLRP3 inflammasome pathway. Front. Pharmacol. 13:910217. doi: 10.3389/fphar.2022.910217
Jiang, T., Wu, M., Zhang, Z., Yan, C., Ma, Z., He, S., et al. (2019). Electroacupuncture attenuated cerebral ischemic injury and neuroinflammation through α7nAChR-mediated inhibition of NLRP3 inflammasome in stroke rats. Mol. Med. 25:22. doi: 10.1186/s10020-019-0091-4
Jiang, X., Stockwell, B., and Conrad, M. (2021). Ferroptosis: Mechanisms, biology and role in disease. Nat. Rev. Mol. Cell. Biol. 22, 266–282. doi: 10.1038/s41580-020-00324-8
Kang, B., Zhao, C., Ma, J., Wang, H., Gu, X., Xu, H., et al. (2023). Electroacupuncture alleviates pain after total knee arthroplasty through regulating neuroplasticity: A resting-state functional magnetic resonance imaging study. Brain Behav. 13:e2913. doi: 10.1002/brb3.2913
Klionsky, D., Petroni, G., Amaravadi, R., Baehrecke, E., Ballabio, A., Boya, P., et al. (2021). Autophagy in major human diseases. EMBO J. 40:e108863. doi: 10.15252/embj.2021108863
Kong, J., Puetz, C., Tian, L., Haynes, I., Lee, E., Stafford, R., et al. (2020). Effect of electroacupuncture vs sham treatment on change in pain severity among adults with chronic low back pain: A randomized clinical trial. JAMA Netw. Open 3:e2022787. doi: 10.1001/jamanetworkopen.2020.22787
Kong, Z., Hong, Y., Zhu, J., Cheng, X., and Liu, Y. (2018). Endothelial progenitor cells improve functional recovery in focal cerebral ischemia of rat by promoting angiogenesis via VEGF. J. Clin. Neurosci. 55, 116–121. doi: 10.1016/j.jocn.2018.07.011
Krishnamurthi, R., Ikeda, T., and Feigin, V. (2020). Global, regional and country-specific burden of ischaemic stroke, intracerebral haemorrhage and subarachnoid haemorrhage: A systematic analysis of the global burden of disease study 2017. Neuroepidemiology 54, 171–179. doi: 10.1159/000506396
Lei, P., Bai, T., and Sun, Y. (2019). Mechanisms of ferroptosis and relations with regulated cell death: A review. Front. Physiol. 10:139. doi: 10.3389/fphys.2019.00139
Levine, B., and Kroemer, G. (2019). Biological functions of autophagy genes: A disease perspective. Cell 176, 11–42. doi: 10.1016/j.cell.2018.09.048
Li, G., Li, X., Dong, J., and Han, Y. (2021). Electroacupuncture ameliorates cerebral ischemic injury by inhibiting ferroptosis. Front. Neurol. 12:619043. doi: 10.3389/fneur.2021.619043
Li, J., Cao, F., Yin, H., Huang, Z., Lin, Z., Mao, N., et al. (2020). Ferroptosis: Past, present and future. Cell Death Dis. 11:88. doi: 10.1038/s41419-020-2298-2
Li, S., Hua, X., Zheng, M., Wu, J., Ma, Z., Xing, X., et al. (2022). Electroacupuncture treatment improves motor function and neurological outcomes after cerebral ischemia/reperfusion injury. Neural Regen. Res. 17, 1545–1555. doi: 10.4103/1673-5374.330617
Li, Z., and Xing, J. (2023). Contribution and therapeutic value of mitophagy in cerebral ischemia-reperfusion injury after cardiac arrest. Biomed. Pharmacother. 167:115492. doi: 10.1016/j.biopha.2023.115492
Liang, D., Minikes, A., and Jiang, X. (2022). Ferroptosis at the intersection of lipid metabolism and cellular signaling. Mol. Cell 82, 2215–2227. doi: 10.1016/j.molcel.2022.03.022
Liao, W., Wen, Y., Yang, S., Duan, Y., and Liu, Z. (2023). Research progress and perspectives of N-methyl-D-aspartate receptor in myocardial and cerebral ischemia-reperfusion injury: A review. Medicine 102:e35490. doi: 10.1097/MD.0000000000035490
Lin, R., Yu, K., Li, X., Tao, J., Lin, Y., Zhao, C., et al. (2016). Electroacupuncture ameliorates post-stroke learning and memory through minimizing ultrastructural brain damage and inhibiting the expression of MMP-2 and MMP-9 in cerebral ischemia-reperfusion injured rats. Mol. Med. Rep. 14, 225–233. doi: 10.3892/mmr.2016.5227
Liu, F., Jiang, Y., Zhao, H., Yao, L., and Chen, L. (2015). Electroacupuncture ameliorates cognitive impairment and regulates the expression of apoptosis-related genes Bcl-2 and Bax in rats with cerebral ischaemia-reperfusion injury. Acupunct. Med. 33, 478–484. doi: 10.1136/acupmed-2014-010728
Liu, S., Wang, Z., Su, Y., Qi, L., Yang, W., Fu, M., et al. (2021). A neuroanatomical basis for electroacupuncture to drive the vagal-adrenal axis. Nature 598, 641–645. doi: 10.1038/s41586-021-04001-4
Liu, S., Yao, S., Yang, H., Liu, S., and Wang, Y. (2023). Autophagy: Regulator of cell death. Cell Death Dis. 14:648. doi: 10.1038/s41419-023-06154-8
Liu, Y., Wan, Y., Jiang, Y., Zhang, L., and Cheng, W. (2023). GPX4: The hub of lipid oxidation, ferroptosis, disease and treatment. Biochim. Biophys. Acta Rev. Cancer 1878:188890. doi: 10.1016/j.bbcan.2023.188890
Long, M., Wang, Z., Zheng, D., Chen, J., Tao, W., Wang, L., et al. (2019). Electroacupuncture pretreatment elicits neuroprotection against cerebral ischemia-reperfusion injury in rats associated with transient receptor potential Vanilloid 1-mediated anti-oxidant stress and Anti-Inflammation. Inflammation 42, 1777–1787. doi: 10.1007/s10753-019-01040-y
Lu, T., Luo, Y., Sun, H., Qin, W., and Li, Y. (2013). Electroacupuncture improves behavioral recovery and increases SCF/c-kit expression in a rat model of focal cerebral ischemia/reperfusion. Neurol. Sci. 34, 487–495. doi: 10.1007/s10072-012-1081-2
Lu, W., and Wen, J. (2025). The relationship among H2S, neuroinflammation and MMP-9 in BBB injury following ischemic stroke. Int. Immunopharmacol. 146:113902. doi: 10.1016/j.intimp.2024.113902
Lu, Y., Yang, Q., Su, Y., Ji, Y., Li, G., Yang, X., et al. (2021). MYCN mediates TFRC-dependent ferroptosis and reveals vulnerabilities in neuroblastoma. Cell Death Dis. 12:511. doi: 10.1038/s41419-021-03790-w
Ma, R., Yuan, B., Du, J., Wang, L., Ma, L., Liu, S., et al. (2016). Electroacupuncture alleviates nerve injury after cerebra ischemia in rats through inhibiting cell apoptosis and changing the balance of MMP-9/TIMP-1 expression. Neurosci. Lett. 633, 158–164. doi: 10.1016/j.neulet.2016.09.033
Mao, J., Liou, K., Baser, R., Bao, T., Panageas, K., Romero, S., et al. (2021). Effectiveness of electroacupuncture or auricular acupuncture vs usual care for chronic musculoskeletal pain among cancer survivors: The PEACE randomized clinical trial. JAMA Oncol. 7, 720–727. doi: 10.1001/jamaoncol.2021.0310
Mao, R., Zong, N., Hu, Y., Chen, Y., and Xu, Y. (2022). Neuronal death mechanisms and therapeutic strategy in ischemic stroke. Neurosci. Bull. 38, 1229–1247. doi: 10.1007/s12264-022-00859-0
Mei, Z., Huang, Y., Feng, Z., Luo, Y., Yang, S., Du, L., et al. (2020). Electroacupuncture ameliorates cerebral ischemia/reperfusion injury by suppressing autophagy via the SIRT1-FOXO1 signaling pathway. Aging 12, 13187–13205. doi: 10.18632/aging.103420
Mendelson, S., and Prabhakaran, S. (2021). Diagnosis and management of transient ischemic attack and acute ischemic stroke: A review. JAMA 325, 1088–1098. doi: 10.1001/jama.2020.26867
Meng, L., Wu, B., OuYang, L., Peng, R., Chen, Y., Tang, Z., et al. (2024). Electroacupuncture regulates histone acetylation of Bcl-2 and Caspase-3 genes to improve ischemic stroke injury. Heliyon 10:e27045. doi: 10.1016/j.heliyon.2024.e27045
Mizushima, N., and Levine, B. (2020). Autophagy in human diseases. N. Engl. J. Med. 383, 1564–1576. doi: 10.1056/NEJMra2022774
Moldoveanu, T., and Czabotar, P. E. (2020). BAX, BAK, and BOK: A coming of age for the BCL-2 family effector proteins. Cold Spring Harb. Perspect. Biol. 12:a036319. doi: 10.1101/cshperspect.a036319
Mueller, S., McFarland White, K., Fass, S., Chen, S., Shi, Z., Ge, X., et al. (2023). Evaluation of gliovascular functions of AQP4 readthrough isoforms. Front. Cell. Neurosci. 17:1272391. doi: 10.3389/fncel.2023.1272391
Ou, M., Jiang, Y., Ji, Y., Zhou, Q., Du, Z., Zhu, H., et al. (2022). Role and mechanism of ferroptosis in neurological diseases. Mol. Metab. 61:101502. doi: 10.1016/j.molmet.2022.101502
Paul, S., and Candelario-Jalil, E. (2021). Emerging neuroprotective strategies for the treatment of ischemic stroke: An overview of clinical and preclinical studies. Exp. Neurol. 335:113518. doi: 10.1016/j.expneurol.2020.113518
Penza, P., Bricchi, M., Scola, A., Campanella, A., and Lauria, G. (2011). Electroacupuncture is not effective in chronic painful neuropathies. Pain Med. 12, 1819–1823. doi: 10.1111/j.1526-4637.2011.01230.x
Potter, T., Tannous, J., and Vahidy, F. S. (2022). A contemporary review of epidemiology, risk factors, etiology, and outcomes of premature stroke. Curr. Atheroscler. Rep. 24, 939–948. doi: 10.1007/s11883-022-01067-x
Qi, R., Xue, Z., and Liu, Y. (2022). Visualization and analysis of the mapping knowledge domain of acupuncture and central nervous system cell apoptosis. Evid. Based Complement. Alternat. Med. 2022:1751702. doi: 10.1155/2022/1751702
Qiu, Z., Ma, J., Zhang, X., Jiao, M., and Zhi, L. (2025). Electroacupuncture combined with trigonelline inhibits pyroptosis in cerebral ischemia-reperfusion by suppressing autophagy via the PI3K/AKT/mTOR signaling pathway. Brain Res. Bull. 221:111200. doi: 10.1016/j.brainresbull.2025.111200
Rao, R., Gan, L., Zhao, R., and Han, Y. (2025). Electroacupuncture alleviates cerebral ischemia injury by regulating PI3K/AKT/NF-κB signaling in microglia of ischemic stroke rats. Neuroreport 36, 22–30. doi: 10.1097/WNR.0000000000002115
Ren, X., Gao, X., Li, Z., Ding, Y., Xu, A., Du, L., et al. (2024). Electroacupuncture ameliorates neuroinflammation by inhibiting TRPV4 channel in ischemic stroke. CNS Neurosci. Ther. 30:e14618. doi: 10.1111/cns.14618
Sahoo, G., Samal, D., Khandayataray, P., and Murthy, M. K. (2023). A review on caspases: Key regulators of biological activities and apoptosis. Mol. Neurobiol. 60, 5805–5837. doi: 10.1007/s12035-023-03433-5
Shaosong, W., Jingqing, S., Qingyin, F., Bin, L., Xin, W., Fan, Y., et al. (2024). Effectivenss of electroacupuncture for skeletal muscle pain in Parkinson’s disease: A Clinical randomized controlled trial. J. Tradit. Chin. Med. 44, 388–395. doi: 10.19852/j.cnki.jtcm.20240203.004
Shen, L., Gan, Q., Yang, Y., Reis, C., Zhang, Z., Xu, S., et al. (2021). Mitophagy in cerebral ischemia and ischemia/reperfusion injury. Front. Aging Neurosci. 13:687246. doi: 10.3389/fnagi.2021.687246
Shi, L., Cao, H., Li, Y., Xu, S., Zhang, Y., Zhang, Y., et al. (2017). Electroacupuncture improves neurovascular unit reconstruction by promoting collateral circulation and angiogenesis. Neural Regen. Res. 12, 2000–2006. doi: 10.4103/1673-5374.221156
Shi, X., Seidle, K., Simms, K., Dong, F., Chilian, W., and Zhang, P. (2023). Endothelial progenitor cells in the host defense response. Pharmacol. Ther. 241:108315. doi: 10.1016/j.pharmthera.2022.108315
Si, W., Wang, J., Li, M., Qu, H., Gu, R., Liu, R., et al. (2019). Erythropoietin protects neurons from apoptosis via activating PI3K/AKT and inhibiting Erk1/2 signaling pathway. 3 Biotech 9:131. doi: 10.1007/s13205-019-1667-y
Skowrońska, K., Obara-Michlewska, M., Zielińska, M., and Albrecht, J. (2019). NMDA receptors in astrocytes: In search for roles in neurotransmission and astrocytic homeostasis. Int. J. Mol. Sci. 20:309. doi: 10.3390/ijms20020309
Sun, P., Ma, F., Xu, Y., Zhou, C., Stetler, R., and Yin, K. (2021). Genetic deletion of endothelial microRNA-15a/16-1 promotes cerebral angiogenesis and neurological recovery in ischemic stroke through Src signaling pathway. J. Cereb. Blood Flow Metab. 41, 2725–2742. doi: 10.1177/0271678X211010351
Sun, X., Liu, Z., Zhou, L., Ma, R., Zhang, X., Wang, T., et al. (2023). Escin avoids hemorrhagic transformation in ischemic stroke by protecting BBB through the AMPK/Cav-1/MMP-9 pathway. Phytomedicine 120:155071. doi: 10.1016/j.phymed.2023.155071
Tang, B., Li, Y., Xu, X., Du, G., and Wang, H. (2024). Electroacupuncture ameliorates neuronal injury by NLRP3/ASC/Caspase-1 mediated pyroptosis in cerebral ischemia-reperfusion. Mol. Neurobiol. 61, 2357–2366. doi: 10.1007/s12035-023-03712-1
Tang, X., Jiang, L., Luo, Y., Fan, H., Song, L., Liu, P., et al. (2021). Leukoaraiosis and acute ischemic stroke. Eur. J. Neurosci. 54, 6202–6213. doi: 10.1111/ejn.15406
Taniguchi, M., Yamashita, T., Kumura, E., Tamatani, M., Kobayashi, A., Yokawa, T., et al. (2000). Induction of aquaporin-4 water channel mRNA after focal cerebral ischemia in rat. Brain Res. Mol. Brain Res. 78, 131–137. doi: 10.1016/s0169-328x(00)00084-x
Tian, W., Zhu, M., Zhou, Y., Mao, C., Zou, R., Cui, Y., et al. (2022). Electroacupuncture pretreatment alleviates cerebral ischemia-reperfusion injury by regulating mitophagy via mTOR-ULK1/FUNDC1 axis in rats. J. Stroke Cerebrovasc. Dis. 31:106202. doi: 10.1016/j.jstrokecerebrovasdis.2021.106202
Tsiftsoglou, A. (2021). Erythropoietin (EPO) as a key regulator of erythropoiesis, bone remodeling and endothelial transdifferentiation of multipotent mesenchymal stem cells (MSCs): Implications in regenerative medicine. Cells 10:2140. doi: 10.3390/cells10082140
Unnisa, A., Greig, N., and Kamal, M. (2023). Inhibition of caspase 3 and caspase 9 mediated apoptosis: A multimodal therapeutic target in traumatic brain injury. Curr. Neuropharmacol. 21, 1001–1012. doi: 10.2174/1570159X20666220327222921
Vandebroek, A., and Yasui, M. (2020). Regulation of AQP4 in the central nervous system. Int. J. Mol. Sci. 21:1603. doi: 10.3390/ijms21051603
Vargas, J., Hamasaki, M., Kawabata, T., Youle, R., and Yoshimori, T. (2023). The mechanisms and roles of selective autophagy in mammals. Nat. Rev. Mol. Cell. Biol. 24, 167–185. doi: 10.1038/s41580-022-00542-2
Vatandoust, S., Mahmoudi, J., Oryan, S., Farajdokht, F., Sadigh-Eteghad, S., Shotorbani, S., et al. (2023). Sericin improves memory and sociability impairments evoked by transient global cerebral ischemia through suppression of hippocampal oxidative stress, inflammation, and apoptosis. Chin. J. Physiol. 66, 209–219. doi: 10.4103/cjop.CJOP-D-23-00006
Wang, G., Xu, S., Lv, H., Zhang, C., and Peng, Y. (2023). Electroacupuncture inhibits ferroptosis induced by cerebral ischemiareperfusion. Curr. Neurovasc. Res. 20, 346–353. doi: 10.2174/1567202620666230623153728
Wang, H., Chen, S., Zhang, Y., Xu, H., and Sun, H. (2019). Electroacupuncture ameliorates neuronal injury by Pink1/Parkin-mediated mitophagy clearance in cerebral ischemia-reperfusion. Nitric Oxide 91, 23–34. doi: 10.1016/j.niox.2019.07.004
Wang, H., Liu, F., Li, R., Wan, M., Li, J., Shi, J., et al. (2021). Electroacupuncture improves learning and memory functions in a rat cerebral ischemia/reperfusion injury model through PI3K/Akt signaling pathway activation. Neural Regen. Res. 16, 1011–1016. doi: 10.4103/1673-5374.300454
Wang, H., Xu, X., Yin, Y., Yu, S., Ren, H., Xue, Q., et al. (2020). Catalpol protects vascular structure and promotes angiogenesis in cerebral ischemic rats by targeting HIF-1α/VEGF. Phytomedicine 78:153300. doi: 10.1016/j.phymed.2020.153300
Wang, L., Sheng, G., Cui, J., Yao, Y., Bai, X., Chen, F., et al. (2022a). Electroacupuncture attenuates ischemic injury after stroke and promotes angiogenesis via activation of EPO mediated Src and VEGF signaling pathways. PLoS One 17:e0274620. doi: 10.1371/journal.pone.0274620
Wang, L., Zhang, X., Xiong, X., Zhu, H., Chen, R., Zhang, S., et al. (2022b). Nrf2 regulates oxidative stress and its role in cerebral ischemic stroke. Antioxidants 11:2377. doi: 10.3390/antiox11122377
Wang, M., Zhang, M., Feng, Y., Xing, Y., Tan, Z., Li, W., et al. (2020). Electroacupuncture inhibits neuronal autophagy and apoptosis via the PI3K/AKT pathway following ischemic stroke. Front. Cell. Neurosci. 14:134. doi: 10.3389/fncel.2020.00134
Wang, W., Wang, Q., Yu, W., Chen, L., and Li, Z. (2018). Efficacy of phosphocreatine pre-administration on XIAP and Smac in ischemic penumbra of rats with focal cerebral ischemia reperfusion injury. Acta Cir. Bras. 33, 117–124. doi: 10.1590/s0102-865020180020000003
Wang, Y., Chen, Y., Meng, L., Wu, B., Ouyang, L., Peng, R., et al. (2023). Electro-acupuncture treatment inhibits the inflammatory response by regulating γδ T and Treg cells in ischemic stroke. Exp. Neurol. 362:114324. doi: 10.1016/j.expneurol.2023.114324
Wang, Z., Xia, Y., Wang, Y., Zhu, R., Li, H., Liu, Y., et al. (2023). The E3 ligase TRIM26 suppresses ferroptosis through catalyzing K63-linked ubiquitination of GPX4 in glioma. Cell Death Dis. 14:695. doi: 10.1038/s41419-023-06222-z
Wolf, P., Schoeniger, A., and Edlich, F. (2022). Pro-apoptotic complexes of BAX and BAK on the outer mitochondrial membrane. Biochim. Biophys. Acta Mol. Cell. Res. 1869:119317. doi: 10.1016/j.bbamcr.2022.119317
Xie, C., Gao, X., Luo, Y., Pang, Y., and Li, M. (2016). Electroacupuncture modulates stromal cell-derived factor-1α expression and mobilization of bone marrow endothelial progenitor cells in focal cerebral ischemia/reperfusion model rats. Brain Res. 1648, 119–126. doi: 10.1016/j.brainres.2016.07.038
Xie, L., Fefelova, N., Pamarthi, S., and Gwathmey, J. (2022). Molecular mechanisms of ferroptosis and relevance to cardiovascular disease. Cells 11:2726. doi: 10.3390/cells11172726
Xu, H., Zhang, Y., Sun, H., Chen, S., and Wang, F. (2014). Effects of acupuncture at GV20 and ST36 on the expression of matrix metalloproteinase 2, aquaporin 4, and aquaporin 9 in rats subjected to cerebral ischemia/reperfusion injury. PLoS One 9:e97488. doi: 10.1371/journal.pone.0097488
Xu, S., Lv, H., Li, W., Hong, H., Peng, Y., and Zhu, B. (2020). Electroacupuncture alleviates cerebral ischemia/reperfusion injury in rats by histone H4 lysine 16 acetylation-mediated autophagy. Front. Psychiatry 11:576539. doi: 10.3389/fpsyt.2020.576539
Yao, M., Liu, T., Zhang, L., Wang, M., Yang, Y., and Gao, J. (2021). Role of ferroptosis in neurological diseases. Neurosci. Lett. 747:135614. doi: 10.1016/j.neulet.2020.135614
Yin, X., Li, W., Liang, T., Lu, B., Yue, H., Li, S., et al. (2022). Effect of electroacupuncture on insomnia in patients with depression: A randomized clinical trial. JAMA Netw. Open 5:e2220563. doi: 10.1001/jamanetworkopen.2022.20563
Zhang, B., Wang, G., Ning, W., Liu, J., Yang, S., Shen, Y., et al. (2020). Electroacupuncture pretreatment elicits tolerance to cerebral ischemia/reperfusion through inhibition of the GluN2B/m-Calpain/p38 MAPK proapoptotic pathway. Neural Plast. 2020:8840675. doi: 10.1155/2020/8840675
Zhang, M., Liu, Q., Meng, H., Duan, H., Liu, X., Wu, J., et al. (2024). Ischemia-reperfusion injury: Molecular mechanisms and therapeutic targets. Signal Transduct. Target Ther. 9:12. doi: 10.1038/s41392-023-01688-x
Zhang, Q., Jia, M., Wang, Y., Wang, Q., and Wu, J. (2022). Cell death mechanisms in cerebral ischemia-reperfusion injury. Neurochem. Res. 47, 3525–3542. doi: 10.1007/s11064-022-03697-8
Zhang, Q., Zhou, M., Huo, M., Si, Y., Zhang, Y., Fang, Y., et al. (2023). Mechanisms of acupuncture-electroacupuncture on inflammatory pain. Mol. Pain 19:17448069231202882. doi: 10.1177/17448069231202882
Zhang, S., Jin, T., Wang, L., Liu, W., Zhang, Y., Zheng, Y., et al. (2021). Electro-acupuncture promotes the differentiation of endogenous neural stem cells via exosomal microRNA 146b after ischemic stroke. Front. Cell. Neurosci. 14:223. doi: 10.3389/fncel.2020.00223
Zhang, W., Han, L., Wen, Y., Su, L., Li, Y., and Luo, X. (2023). Electroacupuncture reverses endothelial cell death and promotes angiogenesis through the VEGF/Notch signaling pathway after focal cerebral ischemia-reperfusion injury. Brain Behav. 13:e2912. doi: 10.1002/brb3.2912
Zhang, Y., Mao, X., Lin, R., Li, Z., and Lin, J. (2018). Electroacupuncture ameliorates cognitive impairment through inhibition of Ca2+-mediated neurotoxicity in a rat model of cerebral ischaemia-reperfusion injury. Acupunct. Med. 36, 401–407. doi: 10.1136/acupmed-2016-011353
Zhao, Y., Zhang, X., Chen, X., and Wei, Y. (2022). Neuronal injuries in cerebral infarction and ischemic stroke: From mechanisms to treatment (Review). Int. J. Mol. Med. 49:15. doi: 10.3892/ijmm.2021.5070
Zheng, X., Lin, W., Jiang, Y., Lu, K., Wei, W., Huo, Q., et al. (2021). Electroacupuncture ameliorates beta-amyloid pathology and cognitive impairment in Alzheimer disease via a novel mechanism involving activation of TFEB (transcription factor EB). Autophagy 17, 3833–3847. doi: 10.1080/15548627.2021.1886720
Keywords: ischemic stroke, cerebral ischemia/reperfusion injury, electroacupuncture, neural protection, autophagy, oxidative stress
Citation: Zhu J, Ge X, Cao Y, Xiao R and Deng X (2025) Neuroprotective effects of electroacupuncture in ischemic stroke: from mechanisms to clinical implications. Front. Aging Neurosci. 17:1562925. doi: 10.3389/fnagi.2025.1562925
Received: 18 January 2025; Accepted: 07 April 2025;
Published: 24 April 2025.
Edited by:
R. M. Damian Holsinger, The University of Sydney, AustraliaReviewed by:
Dongwei Sun, University of California, Riverside, United StatesTianlong Liu, The 940th Hospital of Joint Logistic Support Force of PLA, China
Copyright © 2025 Zhu, Ge, Cao, Xiao and Deng. This is an open-access article distributed under the terms of the Creative Commons Attribution License (CC BY). The use, distribution or reproduction in other forums is permitted, provided the original author(s) and the copyright owner(s) are credited and that the original publication in this journal is cited, in accordance with accepted academic practice. No use, distribution or reproduction is permitted which does not comply with these terms.
*Correspondence: Renjie Xiao, c2hlbnRhbnhAMTI2LmNvbQ==; Xiao Deng, ZGVuZ3hpYW8xMTA0MDlAMTI2LmNvbQ==
†These authors have contributed equally to this work and share first authorship