- 1National Nanotechnology (NANOTEC), National Science and Technology Development Agency, Pathum Thani, Thailand
- 2School of Cosmetic Science, Mae Fah Luang University, Chiang Rai, Thailand
- 3Phytocosmetics and Cosmeceuticals Research Group, School of Cosmetic Science, Mae Fah Luang University, Chiang Rai, Thailand
- 4Department of Pharmacy, Faculty of Pharmacy, Mahidol University, Bangkok, Thailand
The field of anti-aging research has made remarkable strides with the identification of geroprotectors—compounds capable of extending healthspan and lifespan in animal models—presenting promising implications for human longevity. Building on these advances, we propose a novel product category: longevity cosmeceutical actives and products. Unlike conventional anti-aging products that primarily target superficial signs of aging, longevity cosmeceuticals address the molecular hallmarks of aging, fundamentally enhancing skin health and longevity. To clearly distinguish these scientifically validated products from marketing-driven claims, we define, for the first time, longevity cosmeceutical actives and products based on stringent criteria: (1) they must directly target and modulate established hallmarks of skin aging; (2) they must demonstrably extend “skinspan” over time, reflected by improved skin viability, structure, and functional integrity; and (3) their efficacy must be validated through clinical trials, preferably with post-trial skin biopsies to evaluate aging hallmark biomarkers, along with comprehensive safety assessments. This review explores molecular hallmarks of skin aging, highlights geroprotective compounds with potential cosmeceutical applications, and recommends essential biomarkers for assessing prevention of rapid biological aging. Additionally, we propose methodologies for skinspan assessment and emphasize the importance of robust clinical trial designs. By establishing these scientifically rigorous standards, we aim to drive innovation, substantiate longevity claims, and transform the cosmetic industry toward meaningful biological improvements in skin health.
1 Introduction
As the aging population grows, consumer demand is shifting toward skincare solutions that go beyond traditional cosmetics, seeking products that target the biological mechanisms of aging rather than merely alleviating its signs. Although the term “longevity cosmeceuticals” has begun to appear in industry and marketing narratives, it remains undefined and unsupported by peer-reviewed scientific literature. In this manuscript, we define longevity cosmeceutical actives and products as skincare innovations grounded in advances in aging hallmarks and geroscience, offering a transformative approach to promoting and preserving skin health. This shift is reflected in the 2024 Cosmetic 360 exhibition, which highlights longevity-driven innovations in regenerative skincare, cellular anti-senescence, and biomolecular longevity strategies, emphasizing proteomics, genomics, epigenetics, and sustainability to enhance both cutaneous health and product durability (COSMETIC360, 2024). Unlike conventional products driven by marketing claims, longevity cosmeceuticals require scientific validation, bridging dermatology and longevity science. This review examines the hallmarks of skin aging, geroprotectors, biomarkers, skinspan, and clinical testing, establishing a rigorous scientific framework for this rapidly evolving category. To provide an integrated overview of these concepts, the conceptual framework for longevity cosmeceuticals is presented in Figure 1.
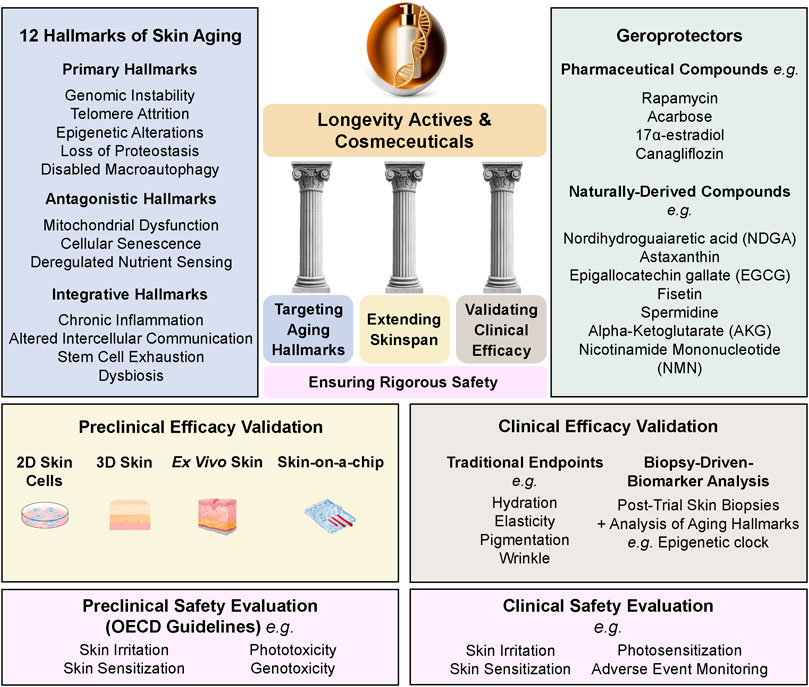
Figure 1. Conceptual Framework for Longevity Cosmeceuticals. The framework outlines four essential components: Targeting Aging Hallmarks, Extending Skinspan, Validating Clinical Efficacy, and Ensuring Rigorous Safety. It integrates the 12 Hallmarks of Skin Aging, representative geroprotective compounds (naturally derived and pharmaceutical), advanced preclinical evaluation models (2D/3D cultures, ex vivo skin, skin-on-a-chip), and clinical validation approaches, including traditional endpoints, aging biomarker analyses, and safety assessments conducted through both preclinical (OECD guidelines) and clinical evaluations.
1.1 Hallmarks of aging and their relevance to skin aging
Aging is a progressive process characterized by cellular decline—manifested as reduced cell functionality, accumulation of senescent cells, depletion of regenerative stem cell pools, and molecular damage—alongside tissue and organ deterioration, dysfunction, and an increased risk of chronic diseases, including cardiovascular disease, diabetes, arthritis, cancer, neurodegenerative disorders, and other metabolic conditions (Guralnik et al., 2020a; Guo et al., 2022). Skin aging is a multifaceted process influenced by both intrinsic and extrinsic factors, leading to structural and physiological changes in the skin. Intrinsic aging is primarily driven by endogenous oxidative stress and accumulated cellular damage, whereas extrinsic aging is induced by environmental factors, such as ultraviolet (UV) radiation and pollution, which accelerate skin deterioration (Shin et al., 2023). In addition to intrinsic and extrinsic aging mechanisms, cumulative psychosocial and environmental stress across the life course has been associated with accelerated biological aging (Suglia et al., 2024).
Skin aging is most visibly recognized through wrinkles, loss of elasticity, and pigmentation; however, these external changes are merely surface reflections of deeper biological dysfunctions. The iceberg model of skin aging (Figure 2) illustrates this relationship, where clinical signs (visible aging) are driven by underlying, non-visible mechanisms occurring from the molecular to tissue levels. At its core, aging is governed by twelve interconnected biological processes known as the twelve hallmarks of aging, which primarily originate at the molecular and cellular levels before manifesting structurally in the skin (López-Otín et al., 2023).
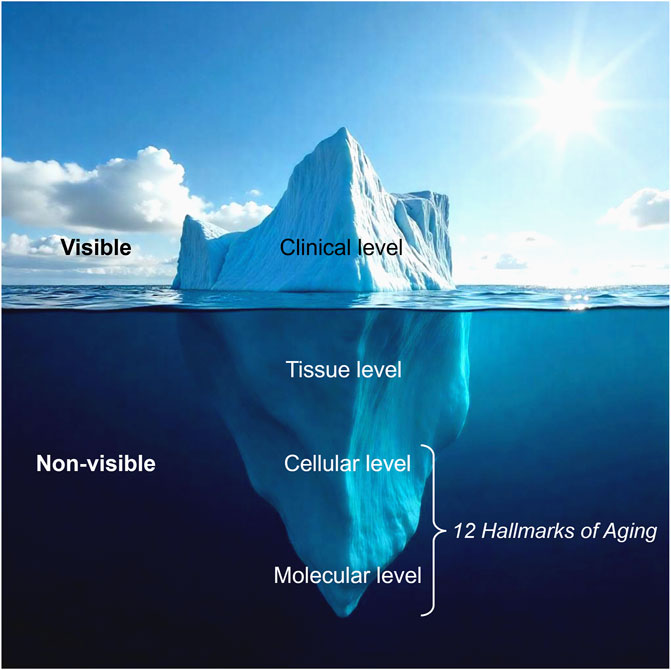
Figure 2. The iceberg model of skin aging. Visible signs of aging represent only the surface level, while deeper, non-visible biological processes at the tissue, cellular, and molecular levels drive skin deterioration. The twelve hallmarks of aging primarily affect the cellular and molecular levels, underlying the fundamental mechanisms of skin aging (Modified from (QIMA-LifeSciences, 2025)).
The twelve hallmarks of aging, classified as primary (damage drivers), antagonistic (initially protective but later harmful), and integrative (systemic frailty contributors), provide a framework for understanding molecular aging and developing intervention strategies (López-Otín et al., 2023; Tartiere et al., 2024). Given skin’s continuous renewal demands and exposure to environmental stressors, the skin is particularly vulnerable to aging processes, leading to barrier dysfunction, extracellular matrix degradation, and impaired regeneration (Wong and Chew, 2021; Jin et al., 2023). Understanding these hallmarks is critical for developing longevity-focused interventions that do more than mask aging signs—they aim to modulate the biological pathways driving skin aging, ultimately preserving skin health and extending skin longevity.
1.1.1 Primary hallmarks: Initiating aging-related damage
Genomic instability from UV exposure, pollution, and oxidative stress leads to mutations, chromosomal aberrations, and impaired repair mechanisms, driving premature senescence and increased skin cancer risk (Vijg and Dong, 2020; Usategui et al., 2022; Jin et al., 2023). Telomere attrition limits stem cell proliferation and tissue regeneration, resulting in thinning epidermis, delayed wound healing, and reduced elasticity (Martinez et al., 2014; Srinivas et al., 2020; Jacczak et al., 2021). Epigenetic alterations, including DNA methylation and histone modifications, disrupt gene regulation, barrier function, and pigmentation, impairing skin renewal (Frye et al., 2007; Driskell et al., 2012; Li et al., 2017). Loss of proteostasis leads to misfolded protein accumulation, weakening extracellular matrix (ECM) integrity, collagen structure, and stress resistance, promoting wrinkles and barrier dysfunction (Bulteau et al., 2007). Disabled macroautophagy, essential for cellular waste removal, worsens oxidative stress and inflammation, accelerating ECM degradation and loss of elasticity (Kohl et al., 2011; Kim et al., 2018).
1.1.2 Antagonistic hallmarks: initially protective, later detrimental
Mitochondrial dysfunction impairs ATP production, increasing ROS damage, which weakens fibroblast function, collagen integrity, and barrier resilience, accelerating photoaging (Hamanaka et al., 2013; Quan et al., 2015; Nakashima et al., 2017; Lan et al., 2019; Oblong et al., 2020). Cellular senescence, driven by stress-induced growth arrest, results in senescence-associated secretory phenotype (SASP) secretion, promoting chronic inflammation, ECM disruption, and delayed healing, leading to wrinkle formation and skin thinning (Blume-Peytavi et al., 2016; Ho and Dreesen, 2021; Danczak-Pazdrowska et al., 2023). Deregulated nutrient sensing, involving mTOR, AMPK, and IGF-1, disrupts metabolic homeostasis and repair mechanisms, where mTOR hyperactivation accelerates collagen degradation, and reduced AMPK/SIRT1 impairs skin resilience (Zouboulis and Makrantonaki, 2011; Choi et al., 2016; Golubtsova et al., 2017; Chung et al., 2019; Bielach-Bazyluk et al., 2021).
1.1.3 Integrative hallmarks: driving systemic frailty
Chronic inflammation (“inflammaging”), fueled by senescent cells and immune dysregulation, leads to barrier dysfunction, prolonged wound healing, and increased sensitivity to environmental damage (Quan et al., 2011; Meyer et al., 2017). Altered intercellular communication, marked by Notch, Wnt, and IGF-1 signaling decline, weakens epidermal differentiation, collagen production, and tissue homeostasis, accelerating structural degradation (López-Otín et al., 2023). Stem cell exhaustion reduces keratinocyte and fibroblast renewal, causing thinner, fragile skin with diminished self-repair capacity (Liu et al., 2019). Dysbiosis, or skin microbiome imbalance, disrupts immune homeostasis and barrier integrity, increasing susceptibility to inflammation, infections, and premature aging (Howard et al., 2022; Ratanapokasatit et al., 2022).
1.2 Geroprotectors: targeting aging mechanisms
Geroprotectors extend lifespan by delaying aging-related decline and improving biomarkers with minimal side effects (Moskalev et al., 2017; Moskalev, 2023). The Interventions Testing Program (ITP), initiated by the National Institute on Aging (NIA), has identified key pharmaceutical geroprotectors with significant lifespan extension in mice. Rapamycin, an mTOR inhibitor, extends lifespan by 14%–26% in females and 9%–23% in males through autophagy enhancement and metabolic regulation (Nadon et al., 2017). Acarbose, an α-glucosidase inhibitor, increases male lifespan by 22% and female lifespan by 5% by slowing glucose absorption and reducing metabolic stress (Harrison et al., 2014). 17α-estradiol, with anti-inflammatory and antioxidant properties, extends male lifespan by 12% but has little effect on females (Harrison et al., 2014). Canagliflozin, an SGLT2 inhibitor that mimics caloric restriction by inhibiting renal glucose reabsorption, increases male lifespan by 14%. (Miller et al., 2020). While effective in aging models, their pharmaceutical nature limits direct cosmeceutical applications.
Naturally derived geroprotectors hold greater potential for longevity-focused cosmeceuticals compared to clinical drugs due to their broader applicability and ability to modulate aging pathways. Nordihydroguaiaretic acid (NDGA), an autophagy activator and p300 inhibitor, extends male lifespan by 8%–10% and enhances skin barrier function in atopic dermatitis models (Kim et al., 2012; Harrison et al., 2014; Tezil et al., 2019). Astaxanthin, a Nrf2 activator and mitochondrial protectant, extends male lifespan by 12%, while improving elasticity, hydration, and wrinkle reduction by mitigating oxidative damage (Davinelli et al., 2018; Harrison et al., 2024). Epigallocatechin gallate (EGCG), a green tea polyphenol, increases lifespan by 7% in females and 10% in males via AMPK activation and fibroblast protection, enhancing skin hydration, elasticity, and pigmentation balance, particularly in photoaged skin (Jiang et al., 2024; Sun et al., 2024).
Several natural geroprotectors, though not validated by the Interventions Testing Program (ITP), have demonstrated significant lifespan extension and skin benefits in independent studies. Fisetin, a senolytic flavonoid, extends median and maximum lifespan by eliminating senescent cells, reducing inflammation, and strengthening the skin barrier (Yousefzadeh et al., 2018; Takaya et al., 2024). Topically, it reduces UVB-induced wrinkles, enhances collagen levels, and inhibits MMPs and COX-2 (Wu et al., 2017). Spermidine, an autophagy-boosting polyamine, extends lifespan by 15%–30%, improves skin hydration, elasticity, wound healing, and preserves collagen integrity (Eisenberg et al., 2009; Kim et al., 2021). GlyNAC, a combination of Glycine (Gly) and N-acetylcysteine (NAC), extends lifespan by 24%, enhances mitochondrial function, and supports collagen synthesis and antioxidant defense (Sekhar, 2021; Kumar et al., 2022). Calcium alpha-ketoglutarate (CaAKG), with anti-inflammatory and metabolic benefits, extends female lifespan by 10.5%–16.6%, improving skin hydration and barrier function (Asadi Shahmirzadi et al., 2020; Yang et al., 2022). Nicotinamide mononucleotide (NMN), an NAD+ precursor, extends median lifespan by 8.5%, delays frailty, protects against mitochondrial dysfunction, and supports keratinocyte defense against oxidative damage and inflammation (Brito et al., 2022; Kane et al., 2024; Zhang et al., 2024).
These natural geroprotectors target key aging pathways, extending lifespan and supporting longevity-focused cosmeceuticals. Validating their effects requires biomarker analysis, skinspan extension studies, and clinical trials. It should be noted that while most of these compounds are naturally derived, NAC is a synthetic molecule, and for industrial applications, naturally occurring compounds such as NMN and CaAKG are often produced through synthetic methods to ensure purity, stability, and scalability.
1.3 Testing reversal of aging hallmarks
To assess the reversal of skin aging hallmarks, reconstructed 3D skin and ex vivo human skin models offer superior structural and functional replication compared to traditional 2D cultures. These models better predict clinical outcomes, including inflammatory responses, cytokine production, and epidermal lipid profiles (Netzlaff et al., 2005; Eberlin et al., 2020; Klinngam et al., 2022; Wang E. H. C. et al., 2023; Klinngam et al., 2024). Unlike 2D cultures, which lack complexity and are highly sensitive to elevated ingredient concentrations and cosmetic formulations (Badr-Eldin et al., 2022), 3D and ex vivo models provide a reliable platform for evaluating longevity interventions (Liu et al., 2022; Ansaf et al., 2023; Lombardi et al., 2024). To measure aging hallmark reversal, we recommend evaluating key biomarkers alongside complementary markers for supporting evidence. Protein-level detection is prioritized due to its direct functional relevance over mRNA levels.
Genomic instability is assessed using DNA damage markers, oxidative DNA damage indicators, and DNA damage response factors. Telomere attrition is evaluated through telomere length measurements, telomerase activity, and telomere maintenance factors. Epigenetic alterations are characterized by DNA methylation clocks, histone modifications, and chromatin regulators. Loss of proteostasis is examined through protein degradation markers, molecular chaperones, misfolded protein aggregates, and proteasome activity, while disabled macroautophagy is assessed separately through autophagy-related proteins and lysosomal activity markers (López-Otín et al., 2023).
Deregulated nutrient sensing is monitored via metabolic signaling regulators and growth factor signaling activity, while mitochondrial dysfunction is identified through mitochondrial membrane potential assays, oxidative stress markers, mitochondrial DNA copy number, and mitophagy indicators. Cellular senescence is detected using the senescence-associated β-galactosidase (SA-β-gal) assay, cell cycle regulators, nuclear integrity factors, and senolytic indicators. SASP components, which reflect the secretory phenotype of senescent cells, may be classified under cellular senescence or, for clarity, preferably categorized within chronic inflammation, where pro-inflammatory cytokines and SASP-related mediators are used for assessment. Altered intercellular communication is evaluated by examining cell signaling pathways and junction proteins, and stem cell exhaustion is assessed through epidermal and follicular stem cell markers, along with proliferation indicators. Lastly, dysbiosis is characterized by microbiome composition shifts, microbial diversity indices, and bacterial population imbalances (López-Otín et al., 2023). A detailed list of biomarkers, detection methods, and references is provided in Table 1.
1.4 Testing “skinspan”: Extending skin viability, structure, and function over time
The term “skinspan” is relatively new and has not yet been widely adopted in peer-reviewed dermatology literature. It was first introduced in industry and aesthetic medicine communications, such as the PMFA Journal article by Pearlman (2024), which highlights the importance of maintaining skin integrity over time (Pearlman, 2024). In this context, we define “skinspan” as the period of sustained skin viability, structure, and function—measurable through relevant biological and clinical markers. To evaluate the ability of longevity cosmeceuticals to extend “skinspan”—the period of optimal skin health and longevity—we propose assessing skin viability, structure, and function over time using 3D reconstructed or ex vivo human skin models.
1.4.1 Assessing skin viability as a reflection of skin lifespan
Skin viability is one of the key determinants of skin lifespan. Measuring skin viability over time provides a functional readout of skin lifespan extension, helping to determine whether longevity cosmeceuticals support sustained skin health and delay overall deterioration.
Viability can be measured through destructive or non-destructive methods. Destructive methods, which require sample processing and prevent further testing, include the MTT assay for mitochondrial activity (Castagnoli et al., 2003; Eberlin et al., 2020), caspase-3/7 activation for detecting apoptosis (Eberlin et al., 2020; Wurbs et al., 2024), Ki-67 staining for proliferation assessment (Wurbs et al., 2024), and live/dead staining, which uses calcein-AM (viable) and propidium iodide (dead) for visual viability analysis (Jin et al., 2022). Additionally, acridine orange staining assesses cellular integrity by fluorescing green in viable cells (double-stranded DNA) and red-orange in single-stranded DNA or RNA, making it a reliable tool for skin viability evaluation (Mohamadali et al., 2023).
In contrast, non-destructive methods allow real-time viability monitoring without tissue termination. The LDH release assay evaluates cytotoxicity based on membrane integrity (Halprin and Ohkawara, 1966; Messager et al., 2003; Bauhammer et al., 2019), the resazurin (Alamar Blue) assay tracks metabolic activity (Garre et al., 2018; Petiti et al., 2024), and the oxygen consumption assay measures mitochondrial activity (Messager et al., 2003; Hong et al., 2020). These methods assess whether active ingredients and formulated products enhance skin viability. For example, a serum containing L-ascorbic acid, ergothioneine, hyaluronic acid, and proteoglycans improved viability in irradiated ex vivo skin, with the resazurin assay indicating higher metabolic activity than controls. This suggests protection against solar-induced damage and prolonged skin function (Garre et al., 2018). A summary of methods for assessing skin viability, including both destructive and non-destructive approaches, is provided in Table 2.
1.4.2 Evaluating structural integrity and functional biomarkers
Assessing skin structure and function over time is crucial for evaluating their impact on skin healthspan and lifespan, determining whether interventions can delay structural and functional deterioration. Key aspects include epidermal barrier integrity, dermal-epidermal junction (DEJ) stability, and ECM composition (Eberlin et al., 2020). Key methods for evaluating skin structural integrity and functional biomarkers essential to skin longevity are summarized in Table 3.
Epidermal barrier integrity protects against water loss, environmental stressors, and microbes, crucial for maintaining skin longevity (Barthe et al., 2024). It consists of keratinocytes, structural proteins (filaggrin, loricrin, involucrin), tight junctions (claudin-1, occludin, ZO-1), and a lipid matrix (ceramides, cholesterol, fatty acids) (Rinnerthaler et al., 2013; Zhang et al., 2014; Brandner et al., 2015; Eberlin et al., 2020; Wang et al., 2020; Yang et al., 2024). Aging depletes these components, accelerating moisture loss and skin aging (Rogers et al., 1996; Feingold, 2009). Restoration of epidermal barrier integrity can be evaluated using IHC/IF, lipid chromatography, and TEWL measurements. (Rosenthal et al., 1992; Brandner et al., 2015; Kocsis et al., 2022).
The DEJ links the epidermis and dermis, facilitating cell adhesion, communication, and renewal (Nishiyama et al., 2000; Roig-Rosello and Rousselle, 2020). Aging and stressors degrade DEJ proteins (collagen IV, VII, XVII, integrin β4, laminin-5), weakening structure, elasticity, and healing (Vázquez et al., 1996; Langton et al., 2016; Khalid et al., 2022). Maintaining DEJ integrity supports skin firmness and regeneration, with IHC and IF used for evaluation (Roig-Rosello and Rousselle, 2020; Jeong et al., 2021; Lim et al., 2022).
ECM composition provides structural support and regulates skin homeostasis (Pfisterer et al., 2021). Key components include collagen I and III for strength (Zorina et al., 2022), elastin for elasticity (Pasquali-Ronchetti and Baccarani-Contri, 1997), and hyaluronic acid for moisture retention (Lee et al., 2016). ECM remodeling, regulated by MMPs, TIMPs, and TGF-β, influences collagen turnover and skin aging (Quan and Fisher, 2015). MMPs serve a dual role: increased expression indicates chronic inflammation and tissue degradation, aligning with the aging hallmark of chronic inflammation, while improved MMP/TIMP balance indicates enhanced ECM remodeling, reflecting improved structural integrity and extended skinspan. To assess ECM integrity, various methods are employed, including IHC, IF, and ELISA to detect collagen type I and III, elastin, hyaluronic acid, MMPs, TIMPs, TGF-β (Vaalamo et al., 1999; Hou et al., 2016; Šínová et al., 2021; Bhardwaj et al., 2022; Sato et al., 2023). Histological stains such as Masson’s trichrome staining for collagen I, reticulin staining for collagen III, and Picrosirius red staining for total collagens I and III provide structural visualization (Calvi et al., 2012). Biochemical assays, including ELISA, allow quantification of collagen I and III, elastin, hyaluronic acid, MMPs, TIMPs, and TGF-β, while gelatin zymography measures MMP activity, and reverse zymography evaluates TIMPs (Lee et al., 1997; Snoek-van Beurden and Von den Hoff, 2005; Lamoral-Theys et al., 2009; Klinngam et al., 2022; Yokota et al., 2022; Ivarsson et al., 2023). These techniques offer comprehensive insights into ECM composition, remodeling, and its role in skin aging.
1.4.3 Advances in long-term skin culture systems
Aging biomarkers, such as telomere length and epigenetic clocks, provide critical insights into skin longevity and rejuvenation but require extended treatment periods to observe meaningful changes (Bär and Blasco, 2016; Galow and Peleg, 2022). Traditional ex vivo and 3D skin models degrade within 1–2 weeks due to nutrient depletion and metabolic decline, limiting their use in long-term studies (Abaci et al., 2015; Mohamadali et al., 2023; Teertam et al., 2025). To address this, advanced culture systems have been developed, such as Phenion® Full-thickness LONG-LIFE 3D Skin, which extends viability to 50 days (Rimal et al., 2024). As shown in Figures 3A, B (a representative image from our laboratory), this model closely mimics native human skin, featuring epidermal stratification and a well-developed dermal matrix. Its prolonged viability makes it a valuable platform for evaluating skin viability, structure, and function in longevity research. Additionally, microfluidic skin-on-a-chip platforms integrate continuous perfusion, oxygenation, and mechanical stimulation, preserving epidermal and dermal structure for several weeks (Ataç et al., 2013; Abaci et al., 2015; Kim et al., 2019; Jeong et al., 2021; Zoio et al., 2021). As shown in Figures 3C, D (images from our laboratory), prolonged culture in a skin-on-a-chip device gradually thins the epidermis, mirroring features of chronological aging. This highlights its potential as a valuable model for studying long-term skin aging. While further research is needed to optimize these models for assessing key aging markers, particularly epigenetic aging clocks, long-term 3D and ex vivo skin cultures will be essential for evaluating longevity cosmeceuticals.
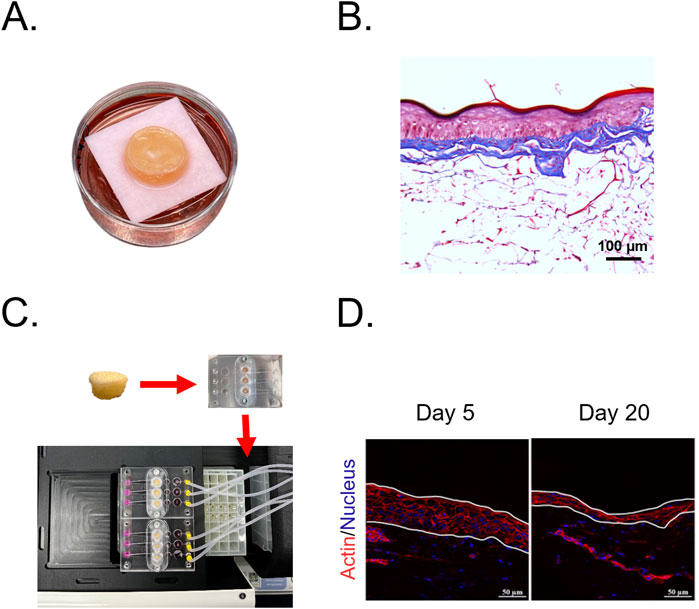
Figure 3. Advanced skin models for long-term culture and aging studies. (A) Full-thickness LONG-LIFE 3D Skin, a bioengineered skin model designed for extended viability in culture. (B) Histological structure of the full-thickness 3D skin model stained with Masson’s Trichrome, showing a well-developed epidermis and organized dermal matrix. (C) Skin-on-a-chip culture system integrating human skin biopsies into a microfluidic platform, enabling long-term perfusion and mechanical stimulation. (D) Epidermal thinning in ex vivo skin-on-a-chip cultures, visualized through actin (red) and nuclear (blue) staining. Images show a progressive reduction in epidermal thickness from Day 5 to Day 20, a characteristic feature of chronological aging.
1.5 Testing the reversal of skin aging phenotypes through clinical trials
To rigorously confirm the efficacy of longevity cosmeceuticals in reversing skin aging phenotypes, clinical trials should integrate both standard efficacy assessments and biomarker-driven longevity evaluations. While traditional cosmetic clinical tests—such as measurements of skin hydration, elasticity, density, pigmentation, and wrinkle reduction—remain important for evaluating visible improvements, they do not capture the underlying biological mechanisms of skin aging. To substantiate true longevity effects, we propose a longevity-specific assessment framework that complements traditional endpoints with molecular and cellular evaluations. This includes post-treatment skin biopsies and biomarker analyses targeting the hallmarks of aging to determine whether these products genuinely reverse biological skin aging.
Clinical trials evaluating anti-aging cosmeceuticals typically enroll 30 or more subjects, with sample sizes determined by statistical power analysis, expected effect size, and study objectives. Given that visible aging signs (e.g., wrinkles, elasticity loss, uneven pigmentation) become more pronounced after 40, this population is ideal for efficacy assessments (Watson et al., 2009; Sadowski and Sadowski, 2020). Randomized, double-blind, placebo-controlled trials remain the gold standard for minimizing bias and ensuring reliability.
Trials may adopt either a split-face (within-person) or split-group (between-subject) design, each with distinct advantages. Split-face trials minimize inter-individual variability and require smaller sample sizes, making them ideal for topical treatments. However, their validity can be compromised if active treatments induce noticeable side effects (e.g., irritation), potentially biasing subjective assessments. In contrast, split-group trials are necessary for systemic treatments, as they eliminate cross-treatment contamination but require larger sample sizes due to inter-individual differences (Leducq et al., 2023). Careful study design is essential to ensure valid, reproducible outcomes, strengthening the scientific rigor of longevity cosmeceutical evaluations.
1.5.1 Traditional cosmetic clinical tests
Traditional skin assessments are fundamental in dermatological research, enabling the validation of longevity cosmeceutical efficacy. Examples of key parameters include skin hydration, barrier function, surface topography, and mechanical properties (Fischer et al., 2001).
1.5.1.1 Skin hydration and barrier function
Aging leads to progressive hydration loss and weakened barrier integrity, increasing susceptibility to dryness, irritation, and environmental stressors. The stratum corneum, which plays a crucial role in moisture retention and barrier protection, undergoes structural deterioration with age, resulting in reduced hydration levels and increased transepidermal water loss (TEWL) (Wang et al., 2020).
Skin hydration is evaluated using conductance- and capacitance-based methods, which assess water content within the stratum corneum (Wilson et al., 1988). Devices such as the DermaLab Combo, SkiCon, Corneometer, and MoistureMeter SC measure hydration through different principles, including conductance (DermaLab Combo and SkiCon), capacitance (Corneometer), and impedance (MoistureMeter SC) (Kanlayavattanakul and Lourith, 2015a; Akdeniz et al., 2018).
TEWL, a key marker of barrier integrity, reflects water loss from the skin (Wilson et al., 1988). Open-chamber devices (e.g., DermaLab Combo, and Tewameter) provide real-time TEWL measurements but require strict environmental controls (Cohen et al., 2009; Kanlayavattanakul and Lourith, 2015a; Alexander et al., 2018). Closed-chamber devices (e.g., VapoMeter) monitor humidity changes in a sealed environment, allowing for rapid readings, though occlusion effects may impact results (Fluhr and Darlenski, 2014). New ventilated closed-chamber models improve precision by preventing water vapor saturation (Uehara et al., 2023). Given TEWL’s sensitivity to environmental conditions, controlled settings (18°C–21°C, 40%–60% relative humidity) are recommended for consistent measurements (Akdeniz et al., 2018; Alexander et al., 2018).
By providing objective hydration and barrier function data, these assessments are crucial for longevity cosmeceuticals, ensuring formulations enhance moisture retention and skin resilience while supporting long-term skin health.
1.5.1.2 Skin elasticity and firmness
As skin ages, collagen and elastin production decline, leading to reduced elasticity and firmness, which manifests as sagging and wrinkle formation (Muzumdar and Ferenczi, 2021). To objectively assess these changes, instruments such as the Cutometer (Ohshima et al., 2013; Evans et al., 2021) and DermaLab Combo with the skin elasticity probes (Peperkamp et al., 2019; Cheng et al., 2022) are commonly used. These devices utilize negative pressure (suction) to measure skin deformation and recovery, providing insights into viscoelastic properties and collagen-elastin functionality.
Additional tools offer complementary biomechanical assessments: the Reviscometer® RVM 600 analyzes skin viscoelasticity through acoustic wave resonance (Paye et al., 2007). Twistometers, including the Dermal Torque Meter®, evaluate torsional resistance to assess elasticity and mechanical stiffness (Kanlayavattanakul and Lourith, 2015b). These biomechanical tools enable quantifiable evaluations of skin elasticity, firmness, and extracellular matrix integrity, making them essential for tracking treatment efficacy in longevity cosmeceuticals.
1.5.1.3 Skin density
Chronological aging leads to reduced skin density, thickness, and collagen organization, affecting tissue stiffness and resilience (Russell-Goldman and Murphy, 2020). High-frequency ultrasound (HFUS) imaging (20–100 MHz) is a widely used non-invasive technique that provides detailed cross-sectional images of the epidermis and dermis, enabling precise thickness and density measurements (Levy et al., 2021; Wang S. et al., 2023). Devices such as the DermaScan C USB and DermaLab Combo (20 MHz probe) visualize dermal density patterns and track collagen organization changes (Peperkamp et al., 2019; Phillips et al., 2020).
Advanced 3D imaging technologies, including ImagePro Opera 3D, Antera 3D, and LifeViz Micro, enable volumetric assessments of specific skin regions, further enhancing dermal structural evaluations (Hurley et al., 2020; Ramirez et al., 2024). These advanced imaging techniques are essential for assessing the efficacy of longevity cosmeceuticals in enhancing skin density, increasing thickness, and restoring ECM integrity.
1.5.1.4 Skin pigmentation and tone
Skin aging is often linked to hyperpigmentation, partly driven by cellular senescence (Kim et al., 2022). Various advanced imaging tools assess age-related pigmentation and treatment efficacy. Narrow-band reflectance meters, such as the Mexameter® MX18, measure skin color by calculating erythema and melanin indices, utilizing wavelengths of 568 nm (melanin absorption), 660 nm (oxyhemoglobin absorption), and 880 nm (hemoglobin absorption) (Clarys et al., 2000; Matias et al., 2015). Chromameters, based on the CIE Lab color space*, quantify skin tone, with increases in lightness (L) and the Individual Typological Angle (ITA°) indicating improvements in skin brightness (Ly et al., 2020).
Additionally, high-resolution imaging systems, including Canfield VISIA and Antera 3D, provide multispectral and topographical analyses, enabling precise tracking of pigmentation changes, melanin distribution, and hemoglobin patterns (Linming et al., 2018). These analytical tools are essential for evaluating longevity cosmeceuticals’ ability to regulate melanogenesis and mitigate aging-driven hyperpigmentation.
Beyond its cosmetic significance, emerging evidence suggests that skin pigmentation serves as a key marker of skin aging, reflecting underlying genetic and cellular aging processes. Notably, pigmentation-associated genes such as MC1R, IRF4, and BNC2 exhibit pleiotropic effects, influencing melanin synthesis as well as age-related traits such as solar lentigines, wrinkles, and perceived age (Ng and Chew, 2022). This highlights the need to consider skin tone changes as a fundamental aspect of longevity-focused skincare interventions.
1.5.1.5 Wrinkle depth, surface roughness, and fine-line reduction
Skin aging leads to decreased collagen production, altered elastin network assembly, and impaired dermal repair, ultimately contributing to wrinkle formation (Veroutis et al., 2021). Advanced imaging techniques allow precise assessment of wrinkle patterns and skin texture.
The VISIA system captures multi-spectral images for detailed wrinkle analysis, while PRIMOS utilizes digital fringe projection to generate high-resolution 3D surface maps for quantitative wrinkle measurement (Nguyen et al., 2021). The Antera 3D system enables multi-layer wrinkle analysis with micrometer precision, while the Visioscan VC 98 evaluates surface roughness and smoothness using image analysis algorithms (Dabrowska et al., 2018; Messaraa et al., 2018).
These advanced imaging techniques are essential for quantifying wrinkle depth, tracking fine-line reduction, and objectively evaluating the efficacy of longevity cosmeceuticals in improving skin texture and minimizing visible signs of aging.
1.5.1.6 Expanding clinical endpoints for skin aging assessment
Emerging research highlights that clinical trial endpoints—such as hydration, elasticity, and pigmentation—capture only a limited portion of the broader skin aging process. In fact, commonly studied features like wrinkles, pigmentation, and photo-aging explain only one-fourth of the total variance in skin aging phenotypes (Ng et al., 2025). Additional underrepresented markers, including telangiectasia, poor lip fullness, ephelides (freckles), ptosis (droopy eyelids), eyebags, and low eyebrow positioning, contribute another quarter of the variance yet are often overlooked in standard cosmetic evaluations (Ng et al., 2025). Integrating these less commonly measured but significant phenotypic markers into clinical methodologies could improve the accuracy and relevance of longevity cosmeceutical assessments, offering a more comprehensive understanding of aging-related changes and enhancing treatment efficacy evaluation.
1.5.2 Biopsy-driven biomarker analysis for validating longevity effects
Standard clinical assessments track visible signs of skin aging and structural changes, such as skin density, but fail to capture the deeper biological processes at the molecular and cellular levels (Figure 2). To address this limitation, post-trial skin biopsies enable the analysis of aging hallmark biomarkers, particularly epigenetic aging clocks, such as Horvath’s Skin & Blood Clock, offering an objective measure of biological age reduction. By revealing underlying rejuvenation mechanisms, rather than just surface-level improvements, this approach strengthens the scientific validation of longevity cosmeceuticals, distinguishing them from conventional skincare products.
Recent studies highlight the value of biopsy-driven biomarker analysis for evaluating longevity interventions. Naughton et al. (2023) initially demonstrated reduced cellular senescence (H2A.J), improved ECM integrity, and favorable gene expression changes related to loss of proteostasis, stem cell exhaustion, and altered intercellular communication using preclinical models. Subsequently, a 24-week placebo-controlled clinical trial confirmed significant improvements in facial sagging, wrinkles, photodamage, and hyperpigmentation. Post-treatment biopsies validated these biological improvements in cellular senescence, ECM integrity, and epidermal barrier function (Naughton et al., 2023).
Similarly, Falckenhayn et al. evaluated dihydromyricetin (DHM) in a clinical trial of 19 women (aged 50–65) over 8 weeks. Participants applied a DHM-containing formulation, and biopsy analysis revealed upregulation of 34 age-related genes previously silenced by DNA methylation, including 23 linked to wrinkle formation. While epigenetic aging clocks were not assessed in biopsies, the study demonstrated in human keratinocytes that DHM significantly reduced DNA methylation age in human keratinocytes, supporting its potential for epigenetic age reversal (Falckenhayn et al., 2023).
By integrating molecular biomarkers into clinical assessments, biopsy-driven analysis establishes a crucial link between visible improvements and the modulation of aging hallmarks, reinforcing the classification of these products as longevity cosmeceuticals.
1.6 Safety evaluation of longevity active ingredients and cosmeceutical products
Ensuring the safety of longevity cosmeceuticals requires a comprehensive approach to meet regulatory standards and protect consumers. For new active ingredients with limited safety data, in vitro assays are essential in preclinical evaluations. Skin irritation is assessed using the Reconstructed Human Epidermis (RhE) Test (OECD TG 439), which measures cell viability in epidermal models (OECD, 2010). Phototoxicity tests (e.g., 3T3 NRU test, OECD TG 432) evaluate the risk of a substance causing adverse skin reactions in the presence of UV light (OECD, 2019), while skin sensitization assays (e.g., ARE-Nrf2 luciferase test, OECD TG 442D; h-CLAT, OECD TG 442E) detect potential sensitizers through immune activation markers (OECD, 2024a; OECD, 2024b). Genotoxicity assessments (e.g., Ames test, OECD TG 471; micronucleus test, OECD TG 487) determine a substance’s potential to cause DNA damage or mutations (Azqueta and Dusinska, 2015; OECD, 2020; OECD, 2023).
To assess long-term safety, carcinogenicity studies have traditionally relied on long-term animal testing to detect cancer risks (OECD, 2014). However, modern alternatives, such as Cell Transformation Assays (CTAs), offer a more ethical and efficient option by modeling key stages of carcinogenesis without animal use. These assays, including BALB/c 3T3 and Bhas 42, detect early-stage cellular transformations, providing insights into tumor initiation and promotion, closely mimicking multi-stage carcinogenesis despite being in vitro models (Steinberg, 2017).
Clinical safety testing of longevity cosmeceutical actives and products should include at least two assessments for skin irritation and skin sensitization, ensuring thorough evaluation of local and allergic reactions. Single patch tests (24–48 h) are commonly used for skin irritation assessments, evaluating erythema and edema. Additionally, the Cumulative Irritation Test (CIT) is recommended to assess whether repeated application leads to progressive irritation, which is critical for daily-use products (Walters et al., 2015). For skin sensitization testing, the human repeated insult patch test (HRIPT) remains the gold standard, consisting of an induction phase (repeated exposure) followed by a challenge phase to assess allergic responses (Politano and Api, 2008). Beyond these core assessments, additional clinical safety tests should be tailored to the active ingredient and intended product application. Photosensitization and phototoxicity tests are recommended for sun-exposed products to ensure they do not trigger UV-induced reactions (Kerr et al., 2010; Lozzi et al., 2020) Similarly, ocular irritation testing is necessary for eye-area formulations to assess potential irritation risks (Gao and Kanengiser, 2004). Additionally, adverse event monitoring should be systematically conducted throughout clinical trials to document and assess any unexpected reactions or side effects, ensuring comprehensive evaluation of product safety.
Although longevity cosmeceuticals currently lack a specific regulatory category and clear safety guidelines, implementing rigorous safety assessments at both the active ingredient and final formulation levels ensures consumer safety, supports regulatory compliance, and advances the scientific validation of effective longevity interventions.
2 Discussion
2.1 Proving longevity cosmeceutical actives and products
Longevity cosmeceuticals must demonstrate true biological aging reversal, beginning with evaluations of their effects on biomarkers associated with aging hallmarks through laboratory testing. Unlike systemic aging research, which relies on lifespan assays in animal models, standardized ‘skinspan’ biomarkers for quantifying long-term improvements in skin viability, structure, and function have yet to be clearly established. In this review, we propose a skinspan assessment strategy that utilizes laboratory tests focused on extending skin viability and delaying structural and functional deterioration. Epigenetic aging clocks represent a promising biomarker for quantifying biological age reversal; however, further validation is required to establish their reliability and correlation with functional skin outcomes.
Current clinical tests primarily measure visible or structural skin improvements, inadequately capturing the deeper biological mechanisms required to substantiate longevity benefits. To address this limitation, post-trial skin biopsies can directly demonstrate rejuvenation at molecular, cellular, and tissue levels by analyzing aging hallmark biomarkers, consistent with laboratory-based evaluations (Falckenhayn et al., 2023; Naughton et al., 2023). Additionally, rigorous safety evaluations conducted through both in vitro assays and clinical trials remain essential for active ingredients and cosmeceutical products (Council, 2009; Barthe et al., 2021). Ultimately, employing these advanced methodologies will clearly differentiate scientifically validated longevity cosmeceuticals from conventional cosmetic skincare.
2.2 Challenges in formulation and delivery
Many active ingredients frequently encounter formulation challenges, including instability from oxidation, UV exposure, pH variations, and poor water solubility. Achieving sufficient skin penetration and maintaining bioavailability further complicate their effective use (Oliveira et al., 2022). Advanced encapsulation technologies, such as liposomes, nanoemulsions, and polymeric carriers, help stabilize these ingredients and ensure targeted delivery into deeper skin layers (Salvioni et al., 2021). Innovative delivery methods, including microneedles and penetration enhancers, can additionally enhance absorption through the skin barrier (Badran et al., 2009; Hmingthansanga et al., 2022). Furthermore, the predictive assessments and testing of dermal penetration should be performed to confirm that active ingredients reliably reach their cellular targets and exert meaningful biological effects beyond superficial skin layers (Liang et al., 2024).
2.3 Navigating regulatory challenges in longevity-focused skincare
Increasing consumer demand for longevity products now extends beyond pharmaceuticals and nutraceuticals, prominently encompassing skincare (Wyles et al., 2024). As skincare transitions from superficial enhancement toward products capable of modulating aging hallmarks and achieving true rejuvenation, clear regulatory guidelines become increasingly essential. Currently, the term “cosmeceutical” lacks official recognition by most regulatory authorities, with products typically classified strictly as either cosmetics or drug (Pandey et al., 2025). Nevertheless, several countries have established intermediate regulatory categories bridging cosmetics and pharmaceuticals, such as “quasi-drugs” in Japan, “functional cosmetics” in South Korea, and “herbal cosmeceuticals” in Thailand (Cho et al., 2017; Ferreira et al., 2022; Food and Drug Administration, 2023). These categories permit mild therapeutic claims beyond conventional cosmetics but require additional regulatory approval supported by demonstrated clinical efficacy and safety data (Pandey et al., 2025). Given these existing frameworks, the introduction of a new regulatory category specifically for longevity cosmeceuticals appears unlikely in the near future. However, if scientifically validated through biomarker-driven clinical studies, longevity skincare products could potentially qualify for expanded claims beyond traditional cosmetic standards without being as restrictive as pharmaceuticals. Without clearly defined regulatory criteria, innovation risks being stifled, while increasing consumer demand could result in misleading claims and compromised market credibility. Establishing explicit regulatory guidelines would promote scientific rigor, responsible innovation, and enhance consumer confidence in longevity skincare products. Nonetheless, implementing rigorous scientific protocols may incur substantial costs, potentially limiting access for smaller enterprises. Balancing scientific rigor with feasibility is essential for broad adoption and sustained innovation.
2.4 Future directions and industry impact
This manuscript represents the first comprehensive effort to propose detailed scientific criteria and regulatory guidance specifically for longevity cosmeceutical active ingredients and products. Rapid advancements in aging biology and geroscience have significantly expanded our understanding of aging mechanisms, enabling the development of innovative skincare interventions, including natural geroprotectors already suitable for cosmetic use. Given the rising consumer interest in scientifically validated longevity skincare, clearly defining and validating this emerging product category is increasingly critical.
Unlike traditional cosmetics, longevity cosmeceuticals aim to modulate fundamental biological processes underlying skin aging, delivering measurable, sustained improvements rather than immediate superficial effects. To credibly support these advanced claims, comprehensive evaluation using validated biomarkers of aging hallmarks in laboratory studies and clinical trials will be essential (Wyles et al., 2024). Transparent consumer communication strategies must also be adopted to prevent false or misleading longevity claims, thereby protecting market credibility and consumer trust. Additionally, achieving global harmonization in product definitions and regulatory guidelines will ensure consistent quality standards internationally. Success in this endeavor will depend on active interdisciplinary collaboration among geroscientists, dermatologists, cosmetic formulators, and regulatory experts, facilitating the translation of cutting-edge research into effective, consumer-accessible skincare innovations.
While acknowledging that regulators do not yet formally recognize longevity cosmeceuticals as a distinct product category, the foundational scientific framework provided by this manuscript will serve as a valuable resource for researchers, innovators, industry stakeholders, and policymakers. Ultimately, the proposed guidelines represent an essential blueprint, establishing longevity cosmeceuticals as a scientifically rigorous skincare category that promotes sustained skin health and drives economic growth within the beauty and health industries.
Author contributions
WK: Conceptualization, Funding acquisition, Writing – original draft, Writing – review and editing. AC: Writing – review and editing, Writing – original draft. SO: Writing – review and editing, Writing – original draft. UR: Writing – review and editing. MK: Writing – review and editing. NL: Writing – review and editing. AW: Writing – original draft, Writing – review and editing. VT: Writing – original draft, Writing – review and editing. TI: Conceptualization, Funding acquisition, Supervision, Writing – original draft, Writing – review and editing.
Funding
The author(s) declare that financial support was received for the research and/or publication of this article. Our work is funded by the Budget Bureau under the National Budget Allocation for Fiscal Year 2024: Strategic Budget for Sustainable Growth–Service Platform for Food & Functional Ingredients under Project P2450586.
Acknowledgments
We would like to thank the scientific community in geroscience, whose pioneering research has laid the foundation upon which this review is built. The pursuit of extending beauty, healthspan, and lifespan holds profound implications, not only for the future of the longevity industry but also for the wellbeing of humanity as a whole.
Conflict of interest
The authors declare that the research was conducted in the absence of any commercial or financial relationships that could be construed as a potential conflict of interest.
Generative AI statement
The authors declare that Gen AI was used in the creation of this manuscript. The authors verify and take full responsibility for the use of generative AI in the preparation of this manuscript. Generative AI, specifically ChatGPT, was used solely for English language grammar editing and refinement.
Publisher’s note
All claims expressed in this article are solely those of the authors and do not necessarily represent those of their affiliated organizations, or those of the publisher, the editors and the reviewers. Any product that may be evaluated in this article, or claim that may be made by its manufacturer, is not guaranteed or endorsed by the publisher.
References
Abaci, H. E., Gledhill, K., Guo, Z., Christiano, A. M., and Shuler, M. L. (2015). Pumpless microfluidic platform for drug testing on human skin equivalents. Lab. Chip 15 (3), 882–888. doi:10.1039/c4lc00999a
Adamus, J., Aho, S., Meldrum, H., Bosko, C., and Lee, J.-M. (2013). p16INK4A influences the aging phenotype in the living skin equivalent. J. Invest Dermatol 134 (4), 1131–1133. doi:10.1038/jid.2013.468
Akdeniz, M., Gabriel, S., Lichterfeld-Kottner, A., Blume-Peytavi, U., and Kottner, J. (2018). Transepidermal water loss in healthy adults: a systematic review and meta-analysis update. Br. J. Dermatol. 179 (5), 1049–1055. doi:10.1111/bjd.17025
Alexander, H., Brown, S., Danby, S., and Flohr, C. (2018). Research techniques made simple: transepidermal water loss measurement as a research tool. J. Invest Dermatol. 138 (11), 2295–2300.e1. doi:10.1016/j.jid.2018.09.001
Ansaf, R. B., Ziebart, R., Gudapati, H., Simoes Torigoe, R. M., Victorelli, S., Passos, J., et al. (2023). 3D bioprinting-a model for skin aging. Regen. Biomater. 10, rbad060. doi:10.1093/rb/rbad060
Arani, R. H., Mohammadpour, H., Moosavi, M. A., Muhammadnejad, A., Abdollahi, A., and Rahmati, M. (2021). The role of autophagy-related proteins of beclin-1/BECN1, LC3II, and p62/SQSTM1 in melanoma tumors. APJCB 6 (4), 263–272. doi:10.31557/APJCB.2021.6.4.263
Asadi Shahmirzadi, A., Edgar, D., Liao, C. Y., Hsu, Y. M., Lucanic, M., Asadi Shahmirzadi, A., et al. (2020). Alpha-ketoglutarate, an endogenous metabolite, extends lifespan and compresses morbidity in aging mice. Cell Metab. 32 (3), 447–456.e6. doi:10.1016/j.cmet.2020.08.004
Ataç, B., Wagner, I., Horland, R., Lauster, R., Marx, U., Tonevitsky, A. G., et al. (2013). Skin and hair on-a-chip: in vitro skin models versus ex vivo tissue maintenance with dynamic perfusion. Lab. Chip 13 (18), 3555–3561. doi:10.1039/c3lc50227a
Azqueta, A., and Dusinska, M. (2015). The use of the comet assay for the evaluation of the genotoxicity of nanomaterials. Front. Genet. 6, 239. doi:10.3389/fgene.2015.00239
Badran, M. M., Kuntsche, J., and Fahr, A. (2009). Skin penetration enhancement by a microneedle device (Dermaroller) in vitro: dependency on needle size and applied formulation. Eur. J. Pharm. Sci. 36 (4-5), 511–523. doi:10.1016/j.ejps.2008.12.008
Badr-Eldin, S. M., Aldawsari, H. M., Kotta, S., Deb, P. K., and Venugopala, K. N. (2022). Three-dimensional in vitro cell culture models for efficient drug discovery: progress so far and future prospects. Pharm. (Basel) 15 (8), 926. doi:10.3390/ph15080926
Bakkenist, C. J., and Kastan, M. B. (2003). DNA damage activates ATM through intermolecular autophosphorylation and dimer dissociation. Nature 421 (6922), 499–506. doi:10.1038/nature01368
Bär, C., and Blasco, M. A. (2016). Telomeres and telomerase as therapeutic targets to prevent and treat age-related diseases. F1000Res 5, 89. doi:10.12688/f1000research.7020.1
Barthe, M., Bavoux, C., Finot, F., Mouche, I., Cuceu-Petrenci, C., Forreryd, A., et al. (2021). Safety testing of cosmetic products: overview of established methods and new approach methodologies (NAMs). Cosmetics 8 (2), 50. doi:10.3390/cosmetics8020050
Barthe, M., Clerbaux, L. A., Thénot, J. P., Braud, V. M., and Osman-Ponchet, H. (2024). Systematic characterization of the barrier function of diverse ex vivo models of damaged human skin. Front. Med. (Lausanne) 11, 1481645. doi:10.3389/fmed.2024.1481645
Bauhammer, I., Sacha, M., and Haltner, E. (2019). Establishment of an in vitro model of cultured viable human, porcine and canine skin and comparison of different media supplements. PeerJ 7, e7811. doi:10.7717/peerj.7811
Bhardwaj, P., Tripathi, P., Pandey, S., Gupta, R., and Ramchandra Patil, P. (2022). Cyclosporine and Pentoxifylline laden tailored niosomes for the effective management of psoriasis: in-vitro optimization, Ex-vivo and animal study. Int. J. Pharm. 626, 122143. doi:10.1016/j.ijpharm.2022.122143
Bielach-Bazyluk, A., Zbroch, E., Mysliwiec, H., Rydzewska-Rosolowska, A., Kakareko, K., Flisiak, I., et al. (2021). Sirtuin 1 and skin: implications in intrinsic and extrinsic aging-A systematic review. Cells 10 (4), 813. doi:10.3390/cells10040813
Blume-Peytavi, U., Kottner, J., Sterry, W., Hodin, M. W., Griffiths, T. W., Watson, R. E., et al. (2016). Age-associated skin conditions and diseases: current perspectives and future options. Gerontologist 56 (Suppl. 2), S230–S242. doi:10.1093/geront/gnw003
Bormann, F., Rodriguez-Paredes, M., Hagemann, S., Manchanda, H., Kristof, B., Gutekunst, J., et al. (2016). Reduced DNA methylation patterning and transcriptional connectivity define human skin aging. Aging Cell 15 (3), 563–571. doi:10.1111/acel.12470
Brandner, J. M., Zorn-Kruppa, M., Yoshida, T., Moll, I., Beck, L. A., and De Benedetto, A. (2015). Epidermal tight junctions in health and disease. Tissue Barriers 3 (1-2), e974451. doi:10.4161/21688370.2014.974451
Brito, S., Baek, J. M., Cha, B., Heo, H., Lee, S. H., Lei, L., et al. (2022). Nicotinamide mononucleotide reduces melanin production in aged melanocytes by inhibiting cAMP/Wnt signaling. J. Dermatol Sci. 106 (3), 159–169. doi:10.1016/j.jdermsci.2022.05.002
Bulteau, A. L., Moreau, M., Nizard, C., and Friguet, B. (2007). Proteasome and photoaging: the effects of UV irradiation. Ann. N. Y. Acad. Sci. 1100, 280–290. doi:10.1196/annals.1395.029
Calvi, E. N., Nahas, F. X., Barbosa, M. V., Calil, J. A., Ihara, S. S., Silva Mde, S., et al. (2012). An experimental model for the study of collagen fibers in skeletal muscle. Acta Cir. Bras. 27 (10), 681–686. doi:10.1590/s0102-86502012001000003
Carvalho, M. J. S., Oliveira, A. L., Santos Pedrosa, S., Pintado, M., Pinto-Ribeiro, I., and Madureira, A. R. (2023). Skin microbiota and the cosmetic industry. Microb. Ecol. 86 (1), 86–96. doi:10.1007/s00248-022-02070-0
Castagnoli, C., Alotto, D., Cambieri, I., Casimiri, R., Aluffi, M., Stella, M., et al. (2003). Evaluation of donor skin viability: fresh and cryopreserved skin using tetrazolioum salt assay. Burns 29 (8), 759–767. doi:10.1016/j.burns.2003.01.001
Chang, K. V., Chen, Y. C., Wu, W. T., Shen, H. J., Huang, K. C., Chu, H. P., et al. (2020). Expression of telomeric repeat-containing RNA decreases in sarcopenia and increases after exercise and nutrition intervention. Nutrients 12 (12), 3766. doi:10.3390/nu12123766
Chen, M., Liang, S., Shahid, A., Andresen, B. T., and Huang, Y. (2020). The β-blocker carvedilol prevented ultraviolet-mediated damage of murine epidermal cells and 3D human reconstructed skin. Int. J. Mol. Sci. 21 (3), 798. doi:10.3390/ijms21030798
Cheng, H., Zhang, R., and Zhuo, F. (2022). Synergistic effect of microneedle-delivered extracellular matrix compound and radiofrequency on rejuvenation of periorbital wrinkles. Front. Med. (Lausanne) 9, 900784. doi:10.3389/fmed.2022.900784
Cho, S., Oh, S., Kim, N. I., Ro, Y. S., Kim, J. S., Park, Y. M., et al. (2017). Knowledge and behavior regarding cosmetics in Koreans visiting dermatology clinics. Ann. Dermatol. 29 (2), 180–186. doi:10.5021/ad.2017.29.2.180
Choi, Y. J., Moon, K. M., Chung, K. W., Jeong, J. W., Park, D., Kim, D. H., et al. (2016). The underlying mechanism of proinflammatory NF-κB activation by the mTORC2/Akt/IKKα pathway during skin aging. Oncotarget 7 (33), 52685–52694. doi:10.18632/oncotarget.10943
Chung, C. L., Lawrence, I., Hoffman, M., Elgindi, D., Nadhan, K., Potnis, M., et al. (2019). Topical rapamycin reduces markers of senescence and aging in human skin: an exploratory, prospective, randomized trial. Geroscience 41 (6), 861–869. doi:10.1007/s11357-019-00113-y
Clarys, P., Alewaeters, K., Lambrecht, R., and Barel, A. O. (2000). Skin color measurements: comparison between three instruments: the Chromameter(R), the DermaSpectrometer(R) and the Mexameter(R). Skin. Res. Technol. 6 (4), 230–238. doi:10.1034/j.1600-0846.2000.006004230.x
Cohen, J. C., Hartman, D. G., Garofalo, M. J., Basehoar, A., Raynor, B., Ashbrenner, E., et al. (2009). Comparison of closed chamber and open chamber evaporimetry. Skin. Res. Technol. 15 (1), 51–54. doi:10.1111/j.1600-0846.2008.00334.x
COSMETIC360 (2024). Theme 2024 - longevity-durability. COSMETIC 360. Available online at: https://www.cosmetic-360.com/en/une-thematique-annuelle (Accessed February 18, 2025).
Council, t.E. P. a.o.t. (2009). Regulation (EC) No 1223/2009 of the European parliament and of the council of 30 november 2009 on cosmetic products. Official J. Eur. Union. Available online at: https://health.ec.europa.eu/system/files/2016-11/cosmetic_1223_2009_regulation_en_0.pdf (Accessed February 24, 2025).
Crowley, L. C., Christensen, M. E., and Waterhouse, N. J. (2016). Measuring mitochondrial transmembrane potential by TMRE staining. Cold Spring Harb. Protoc. 2016 (12), pdb.prot087361. doi:10.1101/pdb.prot087361
Cuanalo-Contreras, K., Schulz, J., Mukherjee, A., Park, K.-W., Armijo, E., and Soto, C. (2023). Extensive accumulation of misfolded protein aggregates during natural aging and senescence. Front. Aging Neurosci. 14, 1090109. doi:10.3389/fnagi.2022.1090109
Dabrowska, M., Mielcarek, A., and Nowak, I. (2018). Evaluation of sex-related changes in skin topography and structure using innovative skin testing equipment. Skin. Res. Technol. 24 (4), 614–620. doi:10.1111/srt.12473
Danczak-Pazdrowska, A., Gornowicz-Porowska, J., Polanska, A., Krajka-Kuzniak, V., Stawny, M., Gostynska, A., et al. (2023). Cellular senescence in skin-related research: targeted signaling pathways and naturally occurring therapeutic agents. Aging Cell 22 (6), e13845. doi:10.1111/acel.13845
Davinelli, S., Nielsen, M. E., and Scapagnini, G. (2018). Astaxanthin in skin health, repair, and disease: a comprehensive review. Nutrients 10 (4), 522. doi:10.3390/nu10040522
Dorf, N., and Maciejczyk, M. (2024). Skin senescence—from basic research to clinical practice. Front. Med. (Lausanne) 11, 1484345. doi:10.3389/fmed.2024.1484345
Dos Santos, M., Metral, E., Boher, A., Rousselle, P., Thepot, A., and Damour, O. (2015). In vitro 3-D model based on extending time of culture for studying chronological epidermis aging. Matrix Biol. 47, 85–97. doi:10.1016/j.matbio.2015.03.009
Dos Santos, M., Michopoulou, A., André-Frei, V., Boulesteix, S., Guicher, C., Dayan, G., et al. (2016). Perlecan expression influences the keratin 15-positive cell population fate in the epidermis of aging skin. Aging (Albany NY) 8 (4), 751–768. doi:10.18632/aging.100928
Driskell, I., Oda, H., Blanco, S., Nascimento, E., Humphreys, P., and Frye, M. (2012). The histone methyltransferase Setd8 acts in concert with c-Myc and is required to maintain skin. EMBO J. 31 (3), 616–629. doi:10.1038/emboj.2011.421
Eberlin, S., Silva, M. S. D., Facchini, G., Silva, G. H. D., Pinheiro, A., Eberlin, S., et al. (2020). The ex vivo skin model as an alternative tool for the efficacy and safety evaluation of topical products. Altern. Lab. Anim. 48 (1), 10–22. doi:10.1177/0261192920914193
Eisenberg, T., Knauer, H., Schauer, A., Buttner, S., Ruckenstuhl, C., Carmona-Gutierrez, D., et al. (2009). Induction of autophagy by spermidine promotes longevity. Nat. Cell Biol. 11 (11), 1305–1314. doi:10.1038/ncb1975
Evans, M., Lewis, E. D., Zakaria, N., Pelipyagina, T., and Guthrie, N. (2021). A randomized, triple-blind, placebo-controlled, parallel study to evaluate the efficacy of a freshwater marine collagen on skin wrinkles and elasticity. J. Cosmet. Dermatol. 20 (3), 825–834. doi:10.1111/jocd.13676
Falckenhayn, C., Bienkowska, A., Sohle, J., Wegner, K., Raddatz, G., Kristof, B., et al. (2023). Identification of dihydromyricetin as a natural DNA methylation inhibitor with rejuvenating activity in human skin. Front. Aging 4, 1258184. doi:10.3389/fragi.2023.1258184
Feingold, K. R. (2009). The outer frontier: the importance of lipid metabolism in the skin. J. Lipid Res. 50 (Suppl. l), S417–S422. doi:10.1194/jlr.R800039-JLR200
Ferreira, M., Matos, A., Couras, A., Marto, J., and Ribeiro, H. (2022). Overview of cosmetic regulatory frameworks around the world. Cosmetics 9, 72. doi:10.3390/cosmetics9040072
Fischer, T. W., Wigger-Alberti, W., and Elsner, P. (2001). Assessment of 'dry skin': current bioengineering methods and test designs. Skin. Pharmacol. Appl. Skin. Physiol. 14 (4), 183–195. doi:10.1159/000056346
Fluhr, J. W., and Darlenski, R. (2014). “Transepidermal water loss (TEWL),” in Non invasive diagnostic techniques in clinical dermatology (Berlin, Heidelberg: Springer Berlin Heidelberg), 353–356.
Food and Drug Administration, T (2023). Type of herbal products. Food Drug Adm. Thail. Available online at: https://en.fda.moph.go.th/entrepreneurs-herbal-products/types-of-herbal-products/(Accessed February 24, 2025).
Forraz, N., Bize, C., Desroches, A.-L., Milet, C., Payen, P., Chanut, P., et al. (2023). The world’s first acne dysbiosis-like model of human 3D ex vivo sebaceous gland colonized with cutibacterium acnes and Staphylococcus epidermidis. Microorganisms 11 (9), 2183. doi:10.3390/microorganisms11092183
Frye, M., Fisher, A. G., and Watt, F. M. (2007). Epidermal stem cells are defined by global histone modifications that are altered by Myc-induced differentiation. PLoS One 2 (8), e763. doi:10.1371/journal.pone.0000763
Galow, A. M., and Peleg, S. (2022). How to slow down the ticking clock: age-associated epigenetic alterations and related interventions to extend life span. Cells 11 (3), 468. doi:10.3390/cells11030468
Gao, Y., and Kanengiser, B. E. (2004). Categorical evaluation of the ocular irritancy of cosmetic and consumer products by human ocular instillation procedures. J. Cosmet. Sci. 55 (4), 317–325.
Garre, A., Narda, M., Valderas-Martinez, P., Piquero, J., and Granger, C. (2018). Antiaging effects of a novel facial serum containing L-Ascorbic acid, proteoglycans, and proteoglycan-stimulating tripeptide: ex vivo skin explant studies and in vivo clinical studies in women. Clin. Cosmet. Investig. Dermatol 11, 253–263. doi:10.2147/ccid.s161352
Golubtsova, N. N., Filippov, F. N., and Gunin, A. G. (2017). Age-related changes in the content of sirtuin 1 in fibroblasts of human dermis. Adv. Gerontol. 30 (3), 375–380. doi:10.1134/S207905701704004X
Guo, J., Huang, X., Dou, L., Yan, M., Shen, T., Tang, W., et al. (2022). Aging and aging-related diseases: from molecular mechanisms to interventions and treatments. Signal Transduct. Target Ther. 7 (1), 391. doi:10.1038/s41392-022-01251-0
Guralnik, J., Cesari, M., Beresniak, A., Rodriguez-Manas, L., and Cherubini, A. (2020a). Early detection of age-associated cellular decline: report of an expert consensus. Innov. Aging. 4 (Suppl. 1), 764. doi:10.1093/geroni/igaa057.2759
Halprin, K. M., and Ohkawara, A. (1966). Lactate production and lactate dehydrogenase in the human epidermis. J. Invest Dermatol. 47 (3), 222–229. doi:10.1038/jid.1966.133
Hamanaka, R. B., Glasauer, A., Hoover, P., Yang, S., Blatt, H., Mullen, A. R., et al. (2013). Mitochondrial reactive oxygen species promote epidermal differentiation and hair follicle development. Sci. Signal 6 (261), ra8. doi:10.1126/scisignal.2003638
Harrison, D. E., Strong, R., Allison, D. B., Ames, B. N., Astle, C. M., Atamna, H., et al. (2014). Acarbose, 17-α-estradiol, and nordihydroguaiaretic acid extend mouse lifespan preferentially in males. Aging cell 13 (2), 273–282. doi:10.1111/acel.12170
Harrison, D. E., Strong, R., Reifsnyder, P., Rosenthal, N., Korstanje, R., Fernandez, E., et al. (2024). Astaxanthin and meclizine extend lifespan in UM-HET3 male mice; fisetin, SG1002 (hydrogen sulfide donor), dimethyl fumarate, mycophenolic acid, and 4-phenylbutyrate do not significantly affect lifespan in either sex at the doses and schedules used. Geroscience 46 (1), 795–816. doi:10.1007/s11357-023-01011-0
Heard, C. M. (2020). An ex vivo skin model to probe modulation of local cutaneous arachidonic acid inflammation pathway. J. Biol. Methods 7 (4), e138. doi:10.14440/jbm.2020.319
Hmingthansanga, V., Singh, N., Banerjee, S., Manickam, S., Velayutham, R., and Natesan, S. (2022). Improved topical drug delivery: role of permeation enhancers and advanced approaches. Pharmaceutics 14 (12), 2818. doi:10.3390/pharmaceutics14122818
Ho, C. Y., and Dreesen, O. (2021). Faces of cellular senescence in skin aging. Mech. Ageing Dev. 198, 111525. doi:10.1016/j.mad.2021.111525
Hong, J. H., Kim, D. H., Rhyu, I. J., Kye, Y. C., and Ahn, H. H. (2020). A simple morphometric analysis method for dermal microstructure using color thresholding and moments. Skin. Res. Technol. 26 (1), 132–136. doi:10.1111/srt.12776
Horvath, S., Oshima, J., Martin, G. M., Lu, A. T., Quach, A., Cohen, H., et al. (2018). Epigenetic clock for skin and blood cells applied to Hutchinson Gilford Progeria Syndrome and ex vivo studies. Aging (Albany NY) 10 (7), 1758–1775. doi:10.18632/aging.101508
Hou, C., Miao, Y., Wang, X., Chen, C., Lin, B., and Hu, Z. (2016). Expression of matrix metalloproteinases and tissue inhibitor of matrix metalloproteinases in the hair cycle. Exp. Ther. Med. 12 (1), 231–237. doi:10.3892/etm.2016.3319
Howard, B., Bascom, C. C., Hu, P., Binder, R. L., Fadayel, G., Huggins, T. G., et al. (2022). Aging-associated changes in the adult human skin microbiome and the host factors that affect skin microbiome composition. J. Invest Dermatol. 142 (7), 1934–1946.e21. doi:10.1016/j.jid.2021.11.029
Hughes, D. C., Baehr, L. M., Waddell, D. S., Sharples, A. P., and Bodine, S. C. (2022). Ubiquitin ligases in longevity and aging skeletal muscle. Int. J. Mol. Sci. 23 (14), 7602. doi:10.3390/ijms23147602
Hurley, S., Messaraa, C., O'Connor, C., Metois, A., Walsh, M., Mc Namee, D., et al. (2020). DermaTOP Blue and Antera 3D as methods to assess cosmetic solutions targeting eyelid sagging. Skin. Res. Technol. 26 (2), 209–214. doi:10.1111/srt.12781
Ido, Y., Duranton, A., Lan, F., Weikel, K. A., Breton, L., and Ruderman, N. B. (2015). Resveratrol prevents oxidative stress-induced senescence and proliferative dysfunction by activating the AMPK-FOXO3 cascade in cultured primary human keratinocytes. PloS one 10 (2), e0115341. doi:10.1371/journal.pone.0115341
Imbert, I., Gondran, C., Oberto, G., Cucumel, K., Dal Farra, C., and Domloge, N. (2010). Maintenance of the ubiquitin–proteasome system activity correlates with visible skin benefits. Int. J. Cosmet. Sci. 32 (6), 446–457. doi:10.1111/j.1468-2494.2010.00575.x
Ivarsson, J., Ferrara, F., Vallese, A., Guiotto, A., Colella, S., Pecorelli, A., et al. (2023). Comparison of pollutant effects on cutaneous inflammasomes activation. Int. J. Mol. Sci. 24 (23), 16674. doi:10.3390/ijms242316674
Jacczak, B., Rubis, B., and Toton, E. (2021). Potential of naturally derived compounds in telomerase and telomere modulation in skin senescence and aging. Int. J. Mol. Sci. 22 (12), 6381. doi:10.3390/ijms22126381
Jeong, S., Kim, J., Jeon, H. M., Kim, K., and Sung, G. Y. (2021). Development of an aged full-thickness skin model using flexible skin-on-a-chip subjected to mechanical stimulus reflecting the circadian rhythm. Int. J. Mol. Sci. 22 (23), 12788. doi:10.3390/ijms222312788
Jiang, N., Gelfond, J., Liu, Q., Strong, R., and Nelson, J. F. (2024). The Gehan test identifies life-extending compounds overlooked by the log-rank test in the NIA Interventions Testing Program: metformin, Enalapril, caffeic acid phenethyl ester, green tea extract, and 17-dimethylaminoethylamino-17-demethoxygeldanamycin hydrochloride. Geroscience 46 (5), 4533–4541. doi:10.1007/s11357-024-01161-9
Jin, S., Li, K., Zong, X., Eun, S., Morimoto, N., and Guo, S. (2023). Hallmarks of skin aging: update. Aging Dis. 14 (6), 2167–2176. doi:10.14336/AD.2023.0321
Jin, S., Oh, Y. N., Son, Y. R., Kwon, B., Park, J. H., Gang, M. J., et al. (2022). Three-dimensional skin tissue printing with human skin cell lines and mouse skin-derived epidermal and dermal cells. J. Microbiol. Biotechnol. 32 (2), 238–247. doi:10.4014/jmb.2111.11042
Kalfalah, F., Sobek, S., Bornholz, B., Gotz-Rosch, C., Tigges, J., Fritsche, E., et al. (2014). Inadequate mito-biogenesis in primary dermal fibroblasts from old humans is associated with impairment of PGC1A-independent stimulation. Exp. Gerontol. 56, 59–68. doi:10.1016/j.exger.2014.03.017
Kane, A. E., Chellappa, K., Schultz, M. B., Arnold, M., Li, J., Amorim, J., et al. (2024). Long-term NMN treatment increases lifespan and healthspan in mice in a sex dependent manner. bioRxiv, 599604. doi:10.1101/2024.06.21.599604
Kanlayavattanakul, M., and Lourith, N. (2015a). Biopolysaccharides for skin hydrating cosmetics. 1867–1892. doi:10.1007/978-3-319-16298-0_29
Kanlayavattanakul, M., and Lourith, N. (2015b). An update on cutaneous aging treatment using herbs. J. Cosmet. Laser Ther. 17 (6), 343–352. doi:10.3109/14764172.2015.1039036
Kelly, G., Kataura, T., Panek, J., Ma, G., Salmonowicz, H., Davis, A., et al. (2024). Suppressed basal mitophagy drives cellular aging phenotypes that can be reversed by a p62-targeting small molecule. Dev. Cell 59 (15), 1924–1939.e7. doi:10.1016/j.devcel.2024.04.020
Kerr, A., Shareef, M., Dawe, R., and Ferguson, J. (2010). Photopatch testing negative in systemic quinine phototoxicity. Photodermatol. Photoimmunol. & Photomed. 26 (3), 151–152. doi:10.1111/j.1600-0781.2010.00503.x
Khalid, K. A., Nawi, A. F. M., Zulkifli, N., Barkat, M. A., and Hadi, H. (2022). Aging and wound healing of the skin: a review of clinical and pathophysiological hallmarks. Life (Basel) 12 (12), 2142. doi:10.3390/life12122142
Kim, G., Kim, M., Kim, M., Park, C., Yoon, Y., Lim, D. H., et al. (2021). Spermidine-induced recovery of human dermal structure and barrier function by skin microbiome. Commun. Biol. 4 (1), 231. doi:10.1038/s42003-020-01619-4
Kim, H. S., Park, S. Y., Moon, S. H., Lee, J. D., and Kim, S. (2018). Autophagy in human skin fibroblasts: impact of age. Int. J. Mol. Sci. 19 (8), 2254. doi:10.3390/ijms19082254
Kim, H. Y., Goo, J. H., Joo, Y. A., Lee, H. Y., Lee, S. M., Oh, C. T., et al. (2012). Impact on inflammation and recovery of skin barrier by nordihydroguaiaretic Acid as a protease-activated receptor 2 antagonist. Biomol. Ther. Seoul. 20 (5), 463–469. doi:10.4062/biomolther.2012.20.5.463
Kim, J. C., Park, T. J., and Kang, H. Y. (2022). Skin-aging pigmentation: who is the real enemy? Cells 11 (16), 2541. doi:10.3390/cells11162541
Kim, J. J., Ellett, F., Thomas, C. N., Jalali, F., Anderson, R. R., Irimia, D., et al. (2019). A microscale, full-thickness, human skin on a chip assay simulating neutrophil responses to skin infection and antibiotic treatments. Lab. Chip 19 (18), 3094–3103. doi:10.1039/c9lc00399a
Kleszczyński, K., Zwicker, S., Tukaj, S., Kasperkiewicz, M., Zillikens, D., Wolf, R., et al. (2015). Melatonin compensates silencing of heat shock protein 70 and suppresses ultraviolet radiation-induced inflammation in human skin ex vivo and cultured keratinocytes. J. Pineal Res. 58 (1), 117–126. doi:10.1111/jpi.12197
Klinngam, W., Rungkamoltip, P., Thongin, S., Joothamongkhon, J., Khumkhrong, P., Khongkow, M., et al. (2022). Polymethoxyflavones from Kaempferia parviflora ameliorate skin aging in primary human dermal fibroblasts and ex vivo human skin. Biomed. Pharmacother. 145, 112461. doi:10.1016/j.biopha.2021.112461
Klinngam, W., Rungkamoltip, P., Wongwanakul, R., Joothamongkhon, J., Du, A. M. S., Khongkow, M., et al. (2024). Skin rejuvenation efficacy and safety evaluation of kaempferia parviflora standardized extract (BG100) in human 3D skin models and clinical trial. Biomolecules 14 (7), 776. doi:10.3390/biom14070776
Kocsis, D., Klang, V., Schweiger, E. M., Varga-Medveczky, Z., Mihály, A., Pongor, C., et al. (2022). Characterization and ex vivo evaluation of excised skin samples as substitutes for human dermal barrier in pharmaceutical and dermatological studies. Skin. Res. Technol. 28 (5), 664–676. doi:10.1111/srt.13165
Kohl, E., Steinbauer, J., Landthaler, M., and Szeimies, R. M. (2011). Skin ageing. J. Eur. Acad. Dermatol Venereol. 25 (8), 873–884. doi:10.1111/j.1468-3083.2010.03963.x
Kolodziej-Wojnar, P., Borkowska, J., Domaszewska-Szostek, A., Bujanowska, O., Noszczyk, B., Krzesniak, N., et al. (2023). Ten-eleven translocation 1 and 2 enzymes affect human skin fibroblasts in an age-related manner. Biomedicines 11 (6), 1659. doi:10.3390/biomedicines11061659
Kumar, P., Osahon, O. W., and Sekhar, R. V. (2022). GlyNAC (Glycine and N-acetylcysteine) supplementation in mice increases length of life by correcting glutathione deficiency, oxidative stress, mitochondrial dysfunction, abnormalities in mitophagy and nutrient sensing, and genomic damage. Nutrients 14 (5), 1114. doi:10.3390/nu14051114
Lammermann, I., Terlecki-Zaniewicz, L., Weinmullner, R., Schosserer, M., Berlin, I., Morizot, F., et al. (2017). Blocking negative effects of senescence in human skin fibroblasts with a plant extract. NPJ Aging Mech. Dis. 137 (10), 4–S311. doi:10.1038/s41514-018-0023-5
Lamoral-Theys, D., Andolfi, A., Van Goietsenoven, G., Cimmino, A., Le Calve, B., Wauthoz, N., et al. (2009). Lycorine, the main phenanthridine Amaryllidaceae alkaloid, exhibits significant antitumor activity in cancer cells that display resistance to proapoptotic stimuli: an investigation of structure-activity relationship and mechanistic insight. J. Med. Chem. 52 (20), 6244–6256. doi:10.1021/jm901031h
Lan, C. E., Hung, Y. T., Fang, A. H., and Ching-Shuang, W. (2019). Effects of irradiance on UVA-induced skin aging. J. Dermatol. Sci. 94 (1), 220–228. doi:10.1016/j.jdermsci.2019.03.005
Langton, A. K., Halai, P., Griffiths, C. E., Sherratt, M. J., and Watson, R. E. (2016). The impact of intrinsic ageing on the protein composition of the dermal-epidermal junction. Mech. Ageing Dev. 156, 14–16. doi:10.1016/j.mad.2016.03.006
Leducq, S., Dugard, A., Allemang-Trivalle, A., Giraudeau, B., and Maruani, A. (2023). Design and methodological issues of within-person (Split-Body) randomized controlled trials evaluating a topical treatment: a systematic review. Dermatology 239 (5), 720–731. doi:10.1159/000530149
Lee, D. H., Oh, J. H., and Chung, J. H. (2016). Glycosaminoglycan and proteoglycan in skin aging. J. Dermatol Sci. 83 (3), 174–181. doi:10.1016/j.jdermsci.2016.05.016
Lee, H. S., Kooshesh, F., Sauder, D. N., and Kondo, S. (1997). Modulation of TGF-beta 1 production from human keratinocytes by UVB. Exp. Dermatol 6 (2), 105–110. doi:10.1111/j.1600-0625.1997.tb00155.x
Levy, J., Barrett, D. L., Harris, N., Jeong, J. J., Yang, X., and Chen, S. C. (2021). High-frequency ultrasound in clinical dermatology: a review. Ultrasound J. 13 (1), 24. doi:10.1186/s13089-021-00222-w
Li, G., Ye, Z., Shi, C., Sun, L., Han, M., Zhuang, Y., et al. (2017). The histone methyltransferase Ash1l is required for epidermal homeostasis in mice. Sci. Rep. 7, 45401. doi:10.1038/srep45401
Liang, B., Zhou, L., Wu, C., Fang, L., Wang, L., Peng, L., et al. (2024). Expert consensus on the technical specifications of the in vitro skin penetration test of cosmetic product by Franz diffusion cell. J. Dermatologic Sci. Cosmet. Technol. 1 (3), 100040. doi:10.1016/j.jdsct.2024.100040
Lim, J. H., Bae, J. S., Lee, S. K., and Lee, D. H. (2022). Palmitoyl-RGD promotes the expression of dermal-epidermal junction components in HaCaT cells. Mol. Med. Rep. 26 (4), 320. doi:10.3892/mmr.2022.12836
Lin, J., Smith, D. L., Esteves, K., and Drury, S. (2019). Telomere length measurement by qPCR - summary of critical factors and recommendations for assay design. Psychoneuroendocrinology 99, 271–278. doi:10.1016/j.psyneuen.2018.10.005
Linming, F., Wei, H., Anqi, L., Yuanyu, C., Heng, X., Sushmita, P., et al. (2018). Comparison of two skin imaging analysis instruments: the VISIA® from Canfield vs the ANTERA 3D® CS from Miravex. Skin. Res. Technol. 24 (1), 3–8. doi:10.1111/srt.12381
Liu, N., Matsumura, H., Kato, T., Ichinose, S., Takada, A., Namiki, T., et al. (2019). Stem cell competition orchestrates skin homeostasis and ageing. Nature 568 (7752), 344–350. doi:10.1038/s41586-019-1085-7
Liu, X., Barresi, R., Kaminer, M., Qian, K., Thillou, F., Bataillon, M., et al. (2022). Utilization of ex vivo tissue model to study skin regeneration following microneedle stimuli. Sci. Rep. 12 (1), 18115. doi:10.1038/s41598-022-22481-w
Lombardi, F., Augello, F. R., Ciafarone, A., Ciummo, V., Altamura, S., Cinque, B., et al. (2024). 3D models currently proposed to investigate human skin aging and explore preventive and reparative approaches: a descriptive review. Biomolecules 14 (9), 1066. doi:10.3390/biom14091066
López-Otín, C., Blasco, M. A., Partridge, L., Serrano, M., and Kroemer, G. (2023). Hallmarks of aging: an expanding universe. Cell 186 (2), 243–278. doi:10.1016/j.cell.2022.11.001
Lozzi, F., Di Raimondo, C., Lanna, C., Diluvio, L., Mazzilli, S., Garofalo, V., et al. (2020). Latest evidence regarding the effects of photosensitive drugs on the skin: pathogenetic mechanisms and clinical manifestations. Pharmaceutics 12 (11), 1104. doi:10.3390/pharmaceutics12111104
Ly, B. C. K., Dyer, E. B., Feig, J. L., Chien, A. L., and Del Bino, S. (2020). Research techniques made simple: cutaneous colorimetry: a reliable technique for objective skin color measurement. J. Invest Dermatol 140 (1), 3–12.e1. doi:10.1016/j.jid.2019.11.003
Martinez, P., Ferrara-Romeo, I., Flores, J. M., and Blasco, M. A. (2014). Essential role for the TRF2 telomere protein in adult skin homeostasis. Aging Cell 13 (4), 656–668. doi:10.1111/acel.12221
Martínez-Martínez, E., Atzei, P., Vionnet, C., Roubaty, C., Kaeser-Pebernard, S., Naef, R., et al. (2022). A dual-acting nitric oxide donor and phosphodiesterase 5 inhibitor activates autophagy in primary skin fibroblasts. Int. J. Mol. Sci. 23 (12), 6860. doi:10.3390/ijms23126860
Matias, A. R., Ferreira, M., Costa, P., and Neto, P. (2015). Skin colour, skin redness and melanin biometric measurements: comparison study between Antera(®) 3D, Mexameter(®) and Colorimeter(®). Skin. Res. Technol. 21 (3), 346–362. doi:10.1111/srt.12199
Messager, S., Hann, A. C., Goddard, P. A., Dettmar, P. W., and Maillard, J. Y. (2003). Assessment of skin viability: is it necessary to use different methodologies? Skin. Res. Technol. 9 (4), 321–330. doi:10.1034/j.1600-0846.2003.00039.x
Messaraa, C., Metois, A., Walsh, M., Hurley, S., Doyle, L., Mansfield, A., et al. (2018). Wrinkle and roughness measurement by the Antera 3D and its application for evaluation of cosmetic products. Skin. Res. Technol. 24 (3), 359–366. doi:10.1111/srt.12436
Meyer, P., Maity, P., Burkovski, A., Schwab, J., Mussel, C., Singh, K., et al. (2017). A model of the onset of the senescence associated secretory phenotype after DNA damage induced senescence. PLoS Comput. Biol. 13 (12), e1005741. doi:10.1371/journal.pcbi.1005741
Miller, R. A., Harrison, D. E., Allison, D. B., Bogue, M., Debarba, L., Diaz, V., et al. (2020). Canagliflozin extends life span in genetically heterogeneous male but not female mice. JCI Insight 5 (21), e140019. doi:10.1172/jci.insight.140019
Mohamadali, M., Ghiaseddin, A., Irani, S., Amirkhani, M. A., and Dahmardehei, M. (2023). Design and evaluation of a skin-on-a-chip pumpless microfluidic device. Sci. Rep. 13 (1), 8861. doi:10.1038/s41598-023-34796-3
Moskalev, A., Chernyagina, E., Kudryavtseva, A., and Shaposhnikov, M. (2017). Geroprotectors: a unified concept and screening approaches. Aging Dis. 8 (3), 354–363. doi:10.14336/AD.2016.1022
Moskalev, A. A. (2023). Potential geroprotectors–from bench to clinic. Biochem. Mosc. 88 (11), 1732–1738. doi:10.1134/S0006297923110056
Murase, D., Kusaka-Kikushima, A., Hachiya, A., Fullenkamp, R., Stepp, A., Imai, A., et al. (2020). Autophagy declines with premature skin aging resulting in dynamic alterations in skin pigmentation and epidermal differentiation. Int. J. Mol. Sci. 21 (16), 5708. doi:10.3390/ijms21165708
Muzumdar, S., and Ferenczi, K. (2021). Nutrition and youthful skin. Clin. Dermatol 39 (5), 796–808. doi:10.1016/j.clindermatol.2021.05.007
Nadon, N. L., Strong, R., Miller, R. A., and Harrison, D. E. (2017). NIA interventions testing Program: investigating putative aging intervention agents in a genetically heterogeneous mouse model. EBioMedicine 21, 3–4. doi:10.1016/j.ebiom.2016.11.038
Nakashima, Y., Ohta, S., and Wolf, A. M. (2017). Blue light-induced oxidative stress in live skin. Free Radic. Biol. Med. 108, 300–310. doi:10.1016/j.freeradbiomed.2017.03.010
Naughton, G. K., Jiang, L. I., Makino, E. T., Chung, R., Nguyen, A., Cheng, T., et al. (2023). Targeting multiple hallmarks of skin aging: preclinical and clinical efficacy of a novel growth factor-based skin care serum. Dermatol Ther. (Heidelb) 13 (1), 169–186. doi:10.1007/s13555-022-00839-2
Netzlaff, F., Lehr, C. M., Wertz, P. W., and Schaefer, U. F. (2005). The human epidermis models EpiSkin, SkinEthic and EpiDerm: an evaluation of morphology and their suitability for testing phototoxicity, irritancy, corrosivity, and substance transport. Eur. J. Pharm. Biopharm. 60 (2), 167–178. doi:10.1016/j.ejpb.2005.03.004
Ng, J. Y., and Chew, F. T. (2022). A systematic review of skin ageing genes: gene pleiotropy and genes on the chromosomal band 16q24.3 may drive skin ageing. Sci. Rep. 12 (1), 13099. doi:10.1038/s41598-022-17443-1
Ng, J. Y., Wong, Q. Y. A., Lim, J. J., Cen, D., Wong, J. Y. K., Lim, Y. Y. E., et al. (2025). A broad assessment of forty-one skin phenotypes reveals complex dimensions of skin ageing. J. Physiol. Anthropol. 44 (1), 3. doi:10.1186/s40101-024-00383-2
Nguyen, T. Q., Zahr, A. S., Kononov, T., and Ablon, G. (2021). A randomized, double-blind, placebo-controlled clinical study investigating the efficacy and tolerability of a peptide serum targeting expression lines. J. Clin. Aesthet. Dermatol 14 (5), 14–21.
Nishiyama, T., Amano, S., Tsunenaga, M., Kadoya, K., Takeda, A., Adachi, E., et al. (2000). The importance of laminin 5 in the dermal-epidermal basement membrane. J. Dermatol Sci. 24 (Suppl. 1), S51–S59. doi:10.1016/s0923-1811(00)00142-0
Noordam, R., Gunn, D., Tomlin, C., Maier, A., Griffiths, T., Catt, S., et al. (2013). Serum insulin-like growth factor 1 and facial ageing: high levels associate with reduced skin wrinkling in a cross-sectional study. Br. J. Dermatol. 168 (3), 533–538. doi:10.1111/bjd.12131
Oblong, J. E., Bowman, A., Rovito, H. A., Jarrold, B. B., Sherrill, J. D., Black, M. R., et al. (2020). Metabolic dysfunction in human skin: restoration of mitochondrial integrity and metabolic output by nicotinamide (niacinamide) in primary dermal fibroblasts from older aged donors. Aging Cell 19 (10), e13248. doi:10.1111/acel.13248
OECD (2010). “Test No. 439: in vitro skin irritation: reconstructed human epidermis test method,”. Paris: OECD Publishing. doi:10.1787/9789264090958-en
OECD (2014). “Guidance document 116 on the conduct and design of chronic toxicity and carcinogenicity studies, supporting test guidelines 451,”. Second edition. Paris: OECD Publishing, 452–453. doi:10.1787/9789264221475-en
OECD (2019). Test No. 432: in vitro 3T3 NRU phototoxicity test. OECD Guidel. Test. Chem. doi:10.1787/9789264071162-en
OECD (2020). “Test No. 471: bacterial reverse mutation test, OECD guidelines for the testing of chemicals, section 4,”. Paris: OECD Publishing. doi:10.1787/9789264071247-en
OECD (2023). “Test No. 487: in vitro mammalian cell micronucleus test,”. Paris: OECD Publishing. doi:10.1787/9789264264861-en
OECD (2024a). “Test No. 442D: in vitro skin sensitisation: assays addressing the adverse outcome pathway key event on keratinocyte activation,”. Paris: OECD Publishing. doi:10.1787/9789264229822-en
OECD (2024b). “Test No. 442E: in vitro skin sensitisation: in vitro skin sensitisation assays addressing the key event on activation of dendritic cells on the adverse outcome pathway for skin sensitisation,”. Paris: OECD Publishing. doi:10.1787/9789264264359-en
Ohshima, H., Kinoshita, S., Oyobikawa, M., Futagawa, M., Takiwaki, H., Ishiko, A., et al. (2013). Use of Cutometer area parameters in evaluating age-related changes in the skin elasticity of the cheek. Skin. Res. Technol. 19 (1), e238–e242. doi:10.1111/j.1600-0846.2012.00634.x
Oliveira, C., Coelho, C., Teixeira, J. A., Ferreira-Santos, P., and Botelho, C. M. (2022). Nanocarriers as active ingredients enhancers in the cosmetic industry-the European and north America regulation challenges. Molecules 27 (5), 1669. doi:10.3390/molecules27051669
Pandey, A., Jatana, G. K., and Sonthalia, S. (2025). “Cosmeceuticals,” in StatPearls (St. Petersburg, FL: StatPearls Publishing StatPearls Publishing LLC).
Park, S.-K., Seong, R.-K., Kim, J.-A., Son, S.-J., Kim, Y., Yokozawa, T., et al. (2016). Oligonol promotes anti-aging pathways via modulation of SIRT1-AMPK-Autophagy Pathway. Nutr. Res. Pract. 10 (1), 3–10. doi:10.4162/nrp.2016.10.1.3
Pasquali-Ronchetti, I., and Baccarani-Contri, M. (1997). Elastic fiber during development and aging. Microsc. Res. Tech. 38 (4), 428–435. doi:10.1002/(sici)1097-0029(19970815)38:4<428::aid-jemt10>3.0.co;2-l
Paye, M., Mac-Mary, S., Elkhyat, A., Tarrit, C., Mermet, P., and Humbert, P. H. (2007). Use of the Reviscometer for measuring cosmetics-induced skin surface effects. Skin. Res. Technol. 13 (4), 343–349. doi:10.1111/j.1600-0846.2007.00236.x
Peperkamp, K., Verhulst, A. C., Tielemans, H. J. P., Winters, H., van Dalen, D., and Ulrich, D. J. O. (2019). The inter-rater and test-retest reliability of skin thickness and skin elasticity measurements by the DermaLab Combo in healthy participants. Skin. Res. Technol. 25 (6), 787–792. doi:10.1111/srt.12718
Petiti, J., Revel, L., and Divieto, C. (2024). Standard operating procedure to optimize resazurin-based viability assays. Biosensors 14 (4), 156. doi:10.3390/bios14040156
Pfisterer, K., Shaw, L. E., Symmank, D., and Weninger, W. (2021). The extracellular matrix in skin inflammation and infection. Front. Cell Dev. Biol. 9, 682414. doi:10.3389/fcell.2021.682414
Phillips, J., Reynolds, K. J., and Gordon, S. J. (2020). Dermal thickness and echogenicity using DermaScan C high frequency ultrasound: methodology and reliability testing in people with and without primary lymphoedema. Skin. Res. Technol. 26 (6), 813–823. doi:10.1111/srt.12880
Piro, M. C., Pecorari, R., Smirnov, A., Cappello, A., Foffi, E., Lena, A. M., et al. (2024). p63 affects distinct metabolic pathways during keratinocyte senescence, evaluated by metabolomic profile and gene expression analysis. Cell Death Dis. 15 (11), 830. doi:10.1038/s41419-024-07159-7
Politano, V. T., and Api, A. M. (2008). The Research Institute for Fragrance Materials' human repeated insult patch test protocol. Regul. Toxicol. Pharmacol. 52 (1), 35–38. doi:10.1016/j.yrtph.2007.11.004
Potic, J., Mbefo, M., Berger, A., Nicolas, M., Wanner, D., Kostic, C., et al. (2020). An in vitro model of human retinal detachment reveals successive death pathway activations. Front. Neurosci. 14, 571293. doi:10.3389/fnins.2020.571293
Punzo, A., Perillo, M., Silla, A., Malaguti, M., Hrelia, S., Barardo, D., et al. (2024). Promising effects of novel supplement formulas in preventing skin aging in 3D human keratinocytes. Nutrients 16 (16), 2770. doi:10.3390/nu16162770
QIMA-LifeSciences (2025). Ageless beauty: the pursuit of skin longevity Available online at: https://qima-lifesciences.com/blog/blog-cosmetics/skin-longevity-aging/[Accessed 24 February 2025].
Quan, C., Cho, M. K., Perry, D., and Quan, T. (2015). Age-associated reduction of cell spreading induces mitochondrial DNA common deletion by oxidative stress in human skin dermal fibroblasts: implication for human skin connective tissue aging. J. Biomed. Sci. 22 (1), 62. doi:10.1186/s12929-015-0167-6
Quan, T., and Fisher, G. J. (2015). Role of age-associated alterations of the dermal extracellular matrix microenvironment in human skin aging: a mini-review. Gerontology 61 (5), 427–434. doi:10.1159/000371708
Quan, T., Qin, Z., Robichaud, P., Voorhees, J. J., and Fisher, G. J. (2011). CCN1 contributes to skin connective tissue aging by inducing age-associated secretory phenotype in human skin dermal fibroblasts. J. Cell Commun. Signal 5 (3), 201–207. doi:10.1007/s12079-011-0144-0
Ramirez, S., Scherz, G., and Leprince, R. (2024). Pilot study: single-depth superficial ultrasound with subdermal injections of diluted calcium hydroxylapatite for improving lower face skin quality. Plast. Reconstr. Surg. Glob. Open 12 (10), e6210. doi:10.1097/GOX.0000000000006210
Ratanapokasatit, Y., Laisuan, W., Rattananukrom, T., Petchlorlian, A., Thaipisuttikul, I., and Sompornrattanaphan, M. (2022). How microbiomes affect skin aging: the updated evidence and current perspectives. Life 12 (7), 936. doi:10.3390/life12070936
Rikken, G., Meesters, L. D., Jansen, P. A., Rodijk-Olthuis, D., van Vlijmen-Willems, I. M., Niehues, H., et al. (2023). Advanced methodology for bacterial colonization of 3D organotypic epidermal models: a gateway to long-term host-microbe interaction and intervention studies. bioRxiv 2023, 2021. doi:10.1101/2023.06.21.545853
Rimal, R., Muduli, S., Desai, P., Marquez, A. B., Möller, M., Platzman, I., et al. (2024). Vascularized 3D human skin models in the forefront of dermatological research. Adv. Healthc. Mater 13 (9), e2303351. doi:10.1002/adhm.202303351
Rinnerthaler, M., Duschl, J., Steinbacher, P., Salzmann, M., Bischof, J., Schuller, M., et al. (2013). Age-related changes in the composition of the cornified envelope in human skin. Exp. Dermatol 22 (5), 329–335. doi:10.1111/exd.12135
Rogers, J., Harding, C., Mayo, A., Banks, J., and Rawlings, A. (1996). Stratum corneum lipids: the effect of ageing and the seasons. Arch. Dermatol Res. 288 (12), 765–770. doi:10.1007/bf02505294
Roig-Rosello, E., and Rousselle, P. (2020). The human epidermal basement membrane: a shaped and cell instructive platform that aging slowly alters. Biomolecules 10 (12), 1607. doi:10.3390/biom10121607
Rosenthal, D. S., Griffiths, C. E. M., Yuspa, S. H., Roop, D. R., and Voorhees, J. J. (1992). Acute or chronic topical retinoic acid treatment of human skin in vivo alters the expression of epidermal transglutaminase, loricrin, involucrin, filaggrin, and keratins 6 and 13 but not keratins 1, 10, and 14. J. Investigative Dermatology 98 (3), 343–350. doi:10.1111/1523-1747.ep12499802
Russell-Goldman, E., and Murphy, G. F. (2020). The pathobiology of skin aging: new insights into an old dilemma. Am. J. Pathol. 190 (7), 1356–1369. doi:10.1016/j.ajpath.2020.03.007
Sadowski, G., and Sadowski, J. (2020). Safety and efficacy of a novel antiaging skin care regimen containing neutraceuticals and growth factors on the facial skin of women: a 12-week open-label study. J. Clin. Aesthet. Dermatol 13 (6), 24–34.
Salvioni, L., Morelli, L., Ochoa, E., Labra, M., Fiandra, L., Palugan, L., et al. (2021). The emerging role of nanotechnology in skincare. Adv. Colloid Interface Sci. 293, 102437. doi:10.1016/j.cis.2021.102437
Samra, T., Gomez-Gomez, T., Linowiecka, K., Akhundlu, A., Lopez de Mendoza, G., Gompels, M., et al. (2023). Melatonin exerts prominent, differential epidermal and dermal anti-aging properties in aged human eyelid skin ex vivo. Int. J. Mol. Sci. 24 (21), 15963. doi:10.3390/ijms242115963
Santiago-Rodriguez, T. M., Le François, B., Macklaim, J. M., Doukhanine, E., and Hollister, E. B. (2023). The skin microbiome: current techniques, challenges, and future directions. Microorganisms 11 (5), 1222. doi:10.3390/microorganisms11051222
Sato, T., Chiba, T., Nakahara, T., Watanabe, K., Sakai, S., Noguchi, N., et al. (2023). Eosinophil-derived galectin-10 upregulates matrix metalloproteinase expression in bullous pemphigoid blisters. J. Dermatol Sci. 112 (1), 6–14. doi:10.1016/j.jdermsci.2023.07.008
Sekhar, R. V. (2021). GlyNAC supplementation improves glutathione deficiency, oxidative stress, mitochondrial dysfunction, inflammation, aging hallmarks, metabolic defects, muscle strength, cognitive decline, and body composition: implications for healthy aging. J. Nutr. 151 (12), 3606–3616. doi:10.1093/jn/nxab309
Shen, J., Gopalakrishnan, V., Lee, J. E., Fang, S., and Zhao, H. (2015). Mitochondrial DNA copy number in peripheral blood and melanoma risk. PLoS One 10 (6), e0131649. doi:10.1371/journal.pone.0131649
Shin, S. H., Lee, Y. H., Rho, N. K., and Park, K. Y. (2023). Skin aging from mechanisms to interventions: focusing on dermal aging. Front. Physiol. 14, 1195272. doi:10.3389/fphys.2023.1195272
Šínová, R., Žádníková, P., Šafránková, B., and Nešporová, K. (2021). Anti-HA antibody does not detect hyaluronan. Glycobiology 31 (5), 520–523. doi:10.1093/glycob/cwaa118
Snoek-van Beurden, P. A., and Von den Hoff, J. W. (2005). Zymographic techniques for the analysis of matrix metalloproteinases and their inhibitors. Biotechniques 38 (1), 73–83. doi:10.2144/05381RV01
Song, X., Narzt, M. S., Nagelreiter, I. M., Hohensinner, P., Terlecki-Zaniewicz, L., Tschachler, E., et al. (2017). Autophagy deficient keratinocytes display increased DNA damage, senescence and aberrant lipid composition after oxidative stress in vitro and in vivo. Redox Biol. 11, 219–230. doi:10.1016/j.redox.2016.12.015
Srinivas, N., Rachakonda, S., and Kumar, R. (2020). Telomeres and telomere length: a general overview. Cancers (Basel) 12 (3), 558. doi:10.3390/cancers12030558
Srivastava, R. K., Muzaffar, S., Khan, J., Crossman, D. K., Agarwal, A., and Athar, M. (2024). HSP90, a common therapeutic target for suppressing skin injury caused by exposure to chemically diverse classes of blistering agents. J. Pharmacol. Exp. Ther. 388 (2), 546–559. doi:10.1124/jpet.123.001795
Steinberg, P. (2017). In vitro-in vivo carcinogenicity. Adv. Biochem. Eng. Biotechnol. 157, 81–96. doi:10.1007/10_2015_5013
Stevens, W. G., Perez, J. L., Pham, L. D., and Jimenez Lozano, J. N. (2023). Expression of HSP70 in human skin after cryolipolysis treatment. Aesthet. Surg. J. 43 (11), NP910–NP915. doi:10.1093/asj/sjad178
Suglia, S. F., Clausing, E. S., Shelton, R. C., Conneely, K., Prada-Ortega, D., DeVivo, I., et al. (2024). Cumulative stress across the life course and biological aging in adulthood. Psychosom. Med. 86 (3), 137–145. doi:10.1097/psy.0000000000001284
Sun, J., Jiang, Y., Fu, J., He, L., Guo, X., Ye, H., et al. (2024). Beneficial effects of epigallocatechin gallate in preventing skin photoaging: a review. Molecules 29 (22), 5226. doi:10.3390/molecules29225226
Takaya, K., Asou, T., and Kishi, K. (2024). Fisetin, a potential skin rejuvenation drug that eliminates senescent cells in the dermis. Biogerontology 25 (1), 161–175. doi:10.1007/s10522-023-10064-9
Tartiere, A. G., Freije, J. M. P., and López-Otín, C. (2024). The hallmarks of aging as a conceptual framework for health and longevity research. Front. Aging 5, 1334261. doi:10.3389/fragi.2024.1334261
Teertam, S. K., Setaluri, V., and Ayuso, J. M. (2025). Advances in microengineered platforms for skin research. JID Innov. 5 (1), 100315. doi:10.1016/j.xjidi.2024.100315
Tezil, T., Chamoli, M., Ng, C. P., Simon, R. P., Butler, V. J., Jung, M., et al. (2019). Lifespan-increasing drug nordihydroguaiaretic acid inhibits p300 and activates autophagy. NPJ Aging Mech. Dis. 5, 7. doi:10.1038/s41514-019-0037-7
Tsitsipatis, D., Martindale, J. L., Ubaida-Mohien, C., Lyashkov, A., Yanai, H., Kashyap, A., et al. (2022). Proteomes of primary skin fibroblasts from healthy individuals reveal altered cell responses across the life span. Aging cell 21 (5), e13609. doi:10.1111/acel.13609
Uehara, O., Kusuhara, T., and Nakamura, T. (2023). Transepidermal water loss estimation model for evaluating skin barrier function. Adv. Biomed. Eng. 12, 1–8. doi:10.14326/abe.12.1
Usategui, A., Municio, C., Arias-Salgado, E. G., Martin, M., Fernandez-Varas, B., Del Rey, M. J., et al. (2022). Evidence of telomere attrition and a potential role for DNA damage in systemic sclerosis. Immun. Ageing 19 (1), 7. doi:10.1186/s12979-022-00263-2
Vaalamo, M., Leivo, T., and Saarialho-Kere, U. (1999). Differential expression of tissue inhibitors of metalloproteinases (TIMP-1, -2, -3, and -4) in normal and aberrant wound healing. Hum. Pathol. 30 (7), 795–802. doi:10.1016/s0046-8177(99)90140-5
Vázquez, F., Palacios, S., Alemañ, N., and Guerrero, F. (1996). Changes of the basement membrane and type IV collagen in human skin during aging. Maturitas 25 (3), 209–215. doi:10.1016/s0378-5122(96)01066-3
Veroutis, D., Kouroumalis, A., Lagopati, N., Polyzou, A., Chamilos, C., Papadodima, S., et al. (2021). Evaluation of senescent cells in intervertebral discs by lipofuscin staining. Mech. Ageing Dev. 199, 111564. doi:10.1016/j.mad.2021.111564
Vijg, J., and Dong, X. (2020). Pathogenic mechanisms of somatic mutation and genome mosaicism in aging. Cell 182 (1), 12–23. doi:10.1016/j.cell.2020.06.024
Walters, R. M., Khanna, P., Hamilton, M., Mays, D. A., and Telofski, L. (2015). Human cumulative irritation tests of common preservatives used in personal care products: a retrospective analysis of over 45 000 subjects. Toxicol. Sci. 148 (1), 101–107. doi:10.1093/toxsci/kfv158
Wan, L., Jiang, D., Correa-Gallegos, D., Ramesh, P., Zhao, J., Ye, H., et al. (2021). Connexin43 gap junction drives fascia mobilization and repair of deep skin wounds. Matrix Biol. 97, 58–71. doi:10.1016/j.matbio.2021.01.005
Wang, E. H. C., Barresi-Thornton, R., Chen, L. C., Senna, M. M., Liao, I. C., Chen, Y., et al. (2023a). The development of human ex vivo models of inflammatory skin conditions. Int. J. Mol. Sci. 24 (24), 17255. doi:10.3390/ijms242417255
Wang, J., Zhang, Y., Gao, Y., Shan, S., and Li, Q. (2021). EZH2 regulates the correlation between skin regeneration and the duration of mechanical stretch. J. Invest Dermatol 141 (4), 894–902.e9. doi:10.1016/j.jid.2020.09.007
Wang, S., Yu, R. X., Fan, W., Li, C. X., Fei, W. M., Li, S., et al. (2023b). Detection of skin thickness and density in healthy Chinese people by using high-frequency ultrasound. Skin. Res. Technol. 29 (1), e13219. doi:10.1111/srt.13219
Wang, Z., Man, M. Q., Li, T., Elias, P. M., and Mauro, T. M. (2020). Aging-associated alterations in epidermal function and their clinical significance. Aging (Albany NY) 12 (6), 5551–5565. doi:10.18632/aging.102946
Watson, R. E., Ogden, S., Cotterell, L. F., Bowden, J. J., Bastrilles, J. Y., Long, S. P., et al. (2009). Effects of a cosmetic 'anti-ageing' product improves photoaged skin [corrected]. Br. J. Dermatol 161 (2), 419–426. doi:10.1111/j.1365-2133.2009.09216.x
Widgerow, A. D., Ziegler, M. E., and Shafiq, F. (2024). A Re-examination of a previous study relating to topical body formulations: validating gene expression transcription at multiple time points, and protein expression and translation in an ex vivo model. Cosmetics 11 (5), 159. doi:10.3390/cosmetics11050159
Wilson, D., Berardesca, E., and Maibach, H. I. (1988). In vivo transepidermal water loss and skin surface hydration in assessment of moisturization and soap effects. Int. J. Cosmet. Sci. 10 (5), 201–211. doi:10.1111/j.1467-2494.1988.tb00018.x
Wong, Q. Y. A., and Chew, F. T. (2021). Defining skin aging and its risk factors: a systematic review and meta-analysis. Sci. Rep. 11 (1), 22075. doi:10.1038/s41598-021-01573-z
Wu, P. Y., Lyu, J. L., Liu, Y. J., Chien, T. Y., Hsu, H. C., Wen, K. C., et al. (2017). Fisetin regulates Nrf2 expression and the inflammation-related signaling pathway to prevent UVB-induced skin damage in hairless mice. Int. J. Mol. Sci. 18 (10), 2118. doi:10.3390/ijms18102118
Wurbs, A., Karner, C., Vejzovic, D., Singer, G., Pichler, M., Liegl-Atzwanger, B., et al. (2024). A human ex vivo skin model breaking boundaries. Sci. Rep. 14 (1), 24054. doi:10.1038/s41598-024-75291-7
Wyles, S., Mehta, R., Mannick, J., and Day, D. (2024). Skin longevity: a paradigm shift in aesthetics. J. Cosmet. Dermatol 23 (9), 2814–2815. doi:10.1111/jocd.16484
Yang, F., Zhou, Z., Guo, M., and Zhou, Z. (2022). The study of skin hydration, anti-wrinkles function improvement of anti-aging cream with alpha-ketoglutarate. J. Cosmet. Dermatol 21 (4), 1736–1743. doi:10.1111/jocd.14635
Yang, S., Kang, W., Choi, D., Roh, J., and Park, T. (2024). Dihydromyrcenol modulates involucrin expression through the akt signaling pathway. Int. J. Mol. Sci. 25 (4), 2246. doi:10.3390/ijms25042246
Yasuoka, H., Larregina, A. T., Yamaguchi, Y., and Feghali-Bostwick, C. A. (2008). Human skin culture as an ex vivo model for assessing the fibrotic effects of insulin-like growth factor binding proteins. Open Rheumatol. J. 2, 17–22. doi:10.2174/1874312900802010017
Yokota, M., Kamiya, Y., Suzuki, T., Ishikawa, S., Takeda, A., Kondo, S., et al. (2022). Trehangelins ameliorate inflammation-induced skin senescence by suppressing the epidermal YAP-CCN1 axis. Sci. Rep. 12 (1), 952. doi:10.1038/s41598-022-04924-6
Yoshioka, H., Yamada, T., Hasegawa, S., Miyachi, K., Ishii, Y., Hasebe, Y., et al. (2021). Senescent cell removal via JAG1-NOTCH1 signalling in the epidermis. Exp. Dermatol 30 (9), 1268–1278. doi:10.1111/exd.14361
Yousefzadeh, M. J., Zhu, Y., McGowan, S. J., Angelini, L., Fuhrmann-Stroissnigg, H., Xu, M., et al. (2018). Fisetin is a senotherapeutic that extends health and lifespan. EBioMedicine 36, 18–28. doi:10.1016/j.ebiom.2018.09.015
Zhang, Y., Wang, B., Chen, H. C., and Wang, Y. N. (2014). Association of claudin-1 and epidermal barrier function in UV-exposed skin of different ages. J. Clin. Dermatology 43, 340–343.
Zhang, Z., Cheng, B., Du, W., Zeng, M., He, K., Yin, T., et al. (2024). The role of nicotinamide mononucleotide supplementation in psoriasis treatment. Antioxidants (Basel) 13 (2), 186. doi:10.3390/antiox13020186
Zoio, P., Ventura, S., Leite, M., and Oliva, A. (2021). Pigmented full-thickness human skin model based on a fibroblast-derived matrix for long-term studies. Tissue Eng. Part C Methods 27 (7), 433–443. doi:10.1089/ten.TEC.2021.0069
Zonari, A., Brace, L. E., Al-Katib, K., Porto, W. F., Foyt, D., Guiang, M., et al. (2023). Senotherapeutic peptide treatment reduces biological age and senescence burden in human skin models. npj Aging 9 (1), 10. doi:10.1038/s41514-023-00109-1
Zorina, A., Zorin, V., Kudlay, D., and Kopnin, P. (2022). Molecular mechanisms of changes in homeostasis of the dermal extracellular matrix: both involutional and mediated by ultraviolet radiation. Int. J. Mol. Sci. 23 (12), 6655. doi:10.3390/ijms23126655
Keywords: longevity cosmeceuticals, aging hallmarks, geroprotectors, skinspan, skin aging biomarkers
Citation: Klinngam W, Chaiwichien A, Osotprasit S, Ruktanonchai U, Kanlayavattanakul M, Lourith N, Wongrakpanich A, Teeranachaideekul V and Iempridee T (2025) Longevity cosmeceuticals as the next frontier in cosmetic innovation: a scientific framework for substantiating product claims. Front. Aging 6:1586999. doi: 10.3389/fragi.2025.1586999
Received: 03 March 2025; Accepted: 13 May 2025;
Published: 22 May 2025.
Edited by:
Dong Hun Lee, Seoul National University Hospital, Republic of KoreaReviewed by:
Marilena Gilca, Carol Davila University of Medicine and Pharmacy, RomaniaAndrey Koptyug, Mid Sweden University, Sweden
Antonello Lorenzini, University of Bologna, Italy
Copyright © 2025 Klinngam, Chaiwichien, Osotprasit, Ruktanonchai, Kanlayavattanakul, Lourith, Wongrakpanich, Teeranachaideekul and Iempridee. This is an open-access article distributed under the terms of the Creative Commons Attribution License (CC BY). The use, distribution or reproduction in other forums is permitted, provided the original author(s) and the copyright owner(s) are credited and that the original publication in this journal is cited, in accordance with accepted academic practice. No use, distribution or reproduction is permitted which does not comply with these terms.
*Correspondence: Tawin Iempridee, dGF3aW5AbmFub3RlYy5vci50aA==