- 1Institute for Molecular Bioscience, Australian Research Council Centre of Excellence for Innovations in Peptide and Protein Science, The University of Queensland, Brisbane, QLD, Australia
- 2Department of Chemistry, Iran University of Science and Technology, Tehran, Iran
- 3Faculty of Chemistry, Khajeh Nasir Toosi University of Technology, Tehran, Iran
- 4School of Chemical Engineering, The University of Queensland, Brisbane, QLD, Australia
The development of safe and efficient delivery systems for targeted and controlled release of therapeutic agents has become a major focus in pharmacotherapy. The colon is one organ that serves as an optimal site for the absorption of protein and peptide drugs, offering significant potential for both localized and systemic therapies. However, effective drug delivery is challenged by the need to protect these drugs from premature absorption and maintain their stability across the varying pH levels of the gastrointestinal tract. In this study, we introduce, for the first time, a fully natural hydrogel system composed of N-acetylated chitosan and alginate, crosslinked using a phenylalanine-phenylalanine dipeptide. The hydrogel demonstrates a unique swelling behavior, allowing for a solvent-free drug-loading method and pH-sensitive release properties. In a colon-simulated pH environment, the hydrogel achieved a high drug release efficiency, with 77.6% of the tested drug sulfasalazine and 51% of hydrocortisone over 5 hours. These findings underscore hydrogel’s potential as a promising drug delivery carrier for targeted gastrointestinal treatments, with the capacity to enhance the efficacy of current therapeutic options.
1 Introduction
In controlled drug delivery, targeting the colon is highly desirable for treating a range of localized diseases, including colorectal cancer, inflammatory bowel diseases (IBD), Crohn’s disease, and ulcerative colitis (Koev et al., 2022). In addition to treating localized diseases, this approach also enables the treatment of systemic conditions and diseases affecting distal organs (McCoubrey et al., 2023). The selective release of bioactive molecules in the colon not only reduces the required dosage for therapeutics but also minimizes side effects associated with drug release and absorption in the upper gastrointestinal (GI) tract (Casadei et al., 2008). Given the colon’s distal location in the GI tract, an ideal design for a colon-specific drug delivery system should prevent drug release in the stomach and small intestine, while ensuring a rapid onset of release upon reaching the colon (Yang et al., 2002).
Oral administration is the preferred route for drug delivery, offering several advantages, such as ease of consumption, non-invasive nature, and convenience of drug administration (Alqahtani et al., 2021). Additionally, the rectal route serves as an effective alternative for the local delivery of pharmaceutical compounds, particularly for drugs with poor oral absorption, due to the rectum’s stable environment and low enzymatic activity (Rathi et al., 2022). Designing an effective colon-targeted drug delivery system necessitates careful consideration of various factors, including the physiological, anatomical, and pathophysiological conditions in the GI tract across individuals (Hua et al., 2015). One of the key challenges in achieving colon-targeted drug delivery is the variability in pH levels across different segments of the GI tract. The stomach maintains a highly acidic environment (pH 1–3), while the small intestine ranges from slightly acidic to neutral (pH 5.9–7.8), and the colon’s pH varies between 5 and 8. As pH serves as a common biological cue, it is widely utilized to trigger responsiveness in nanomedicine (Li and Kataoka, 2020). Therefore, developing systems that can specifically respond to the unique pH conditions of the colon is crucial for facilitating targeted drug release (Van Den Abeele et al., 2017). Common strategies to achieve this include the use of polymeric materials (Soppimath et al., 2001), nanoparticles (He and Shi, 2011), and pH-responsive carriers (Liu et al., 2017), all aimed at creating effective and controlled drug delivery systems.
Polymeric hydrogels represent a promising class of biomaterials for targeted drug delivery, owing to their biocompatibility and favorable physicochemical properties (Kesharwani et al., 2021). The swellable, porous polymeric structure allows hydrogels to absorb significant amounts of water or biofluids, making them highly desirable for controlled and sustained drug delivery (Caccavo et al., 2016; Tian and Liu, 2023). Additionally, the ability to modify the physical and chemical properties of the hydrogel network, along with optimizing hydrogel–drug interactions, enables precise control over the release of therapeutic agents (Li and Mooney, 2016).
Natural polymers, such as polysaccharides and proteins derived from plants, microorganisms, and animals, offer excellent properties for hydrogel design and fabrication due to their biodegradability, biocompatibility, and non-toxicity (Karoyo and Wilson, 2021; Varghese et al., 2020). Among these, natural polymers, chitosan has garnered significant attention as a scaffold material in drug delivery systems (Tian and Liu, 2023; Saeedi et al., 2022; Yuan et al., 2022) because of its biodegradability, biocompatibility, and availability of functional moieties (N-H, O-H), which allow for chemical modification and the tailoring of specific physicochemical properties (Bhattarai et al., 2010). This attractive polysaccharide serves as an exceptional excipient, being non-toxic, stable, and can be sterilized, while, unlike other naturally derived materials, it does not induce an immune response (Cheung et al., 2015). Similarly, alginic acid and its sodium and calcium salts, collectively known as Alginate, are among the most versatile naturally derived polysaccharide polymers used in pharmaceutical applications (Motasadizadeh et al., 2022; Li et al., 2022; Maxwell et al., 2022). In addition to its non-toxicity and biocompatibility, alginate’s ability to form two distinct types of gels, one pH-dependent, and the other ionotropic endows the biopolymer with unique physicochemical properties compared to neutral macromolecules (Szekalska et al., 2016). The pH sensitivity of both alginate and chitosan, attributed to their carboxyl and amino groups, respectively, makes them particularly well-suited for drug carrier applications. Similar to chitosan, the highly porous three-dimensional structure of alginate hydrogels offers advantageous swelling properties due to the presence of hydrophilic functional groups (Lee and Mooney, 2012). However, a significant limitation of ionically cross-linked alginate gels is their reduced long-term stability under physiological conditions. In such environments, divalent ions may be released into the surrounding microenvironment through exchange reactions with monovalent cations, ultimately leading to gel dissolution (Kong et al., 2003). Despite this, alginate gels are easily modified and chemically cross-linked and can be administrated orally or injected in a minimally invasive manner, allowing for a wide range of pharmaceutical applications (Nandini et al., 2008).
The combination of chitosan and alginate has been extensively used to develop hydrating and gel-forming matrix systems for homogeneous solid dispersion and the prolonged and controlled release of various active substances at specific local sites (Ching et al., 2008; Miyazaki et al., 1995; Murata et al., 1996; Mi et al., 2002; Ribeiro et al., 2005). The electrostatic interactions that form the chitosan-alginate polyelectrolyte complex not only address the limitations of alginate hydrogels but also enhance the drug release performance. This interaction strengthens the structural integrity and functional efficiency of the hydrogel, making it a more effective platform for controlled drug delivery (Anal and Stevens, 2005). Moreover, the hydrophilic nature of chitosan and alginate results in limited swelling in acidic pH, while promoting substantial swelling in the neutral pH of the colon, enabling controlled drug release. This property makes them an ideal choice for polymeric matrix complexes designed for controlled, colon-specific drug delivery (Kaur et al., 2014). Studies by Xu et al. (2007) and Wang et al. (2016) demonstrated that chitosan-alginate hybrid matrix systems could achieve drug release rates of 97.84% and 65.6% for icariin and BSA model protein drug, respectively, triggered in the colonic environment, with minimal release of 2.35% and 10% in simulated gastric fluid (Xu et al., 2007; Wang et al., 2016).
Various cross-linking methods have been developed to form hybrid polymer networks of chitosan-alginate, enhancing their physicochemical properties and biological activity (Chen et al., 2004; Lin et al., 2005; Baysal et al., 2013). Naturally occurring cross-linking agents not only provide a non-cytotoxic option but have been shown to reinforce drug release from the chitosan-alginate matrix system (Nandini et al., 2008). In this work, we present a novel hydrogel system incorporating a naturally occurring phenylalanine-phenylalanine dipeptide cross-linker, covalently integrated into the chitosan and alginate polymeric network backbone (Figure 1). This fully natural hydrogel exhibited solvent-free drug loading properties, achieving 100% loading efficiency of model drugs. Additionally, the release behavior of sulfasalazine and hydrocortisone was evaluated under colonic pH conditions, showing rapid release in this environment and underscoring the potential of this system for targeted drug delivery to the colon.
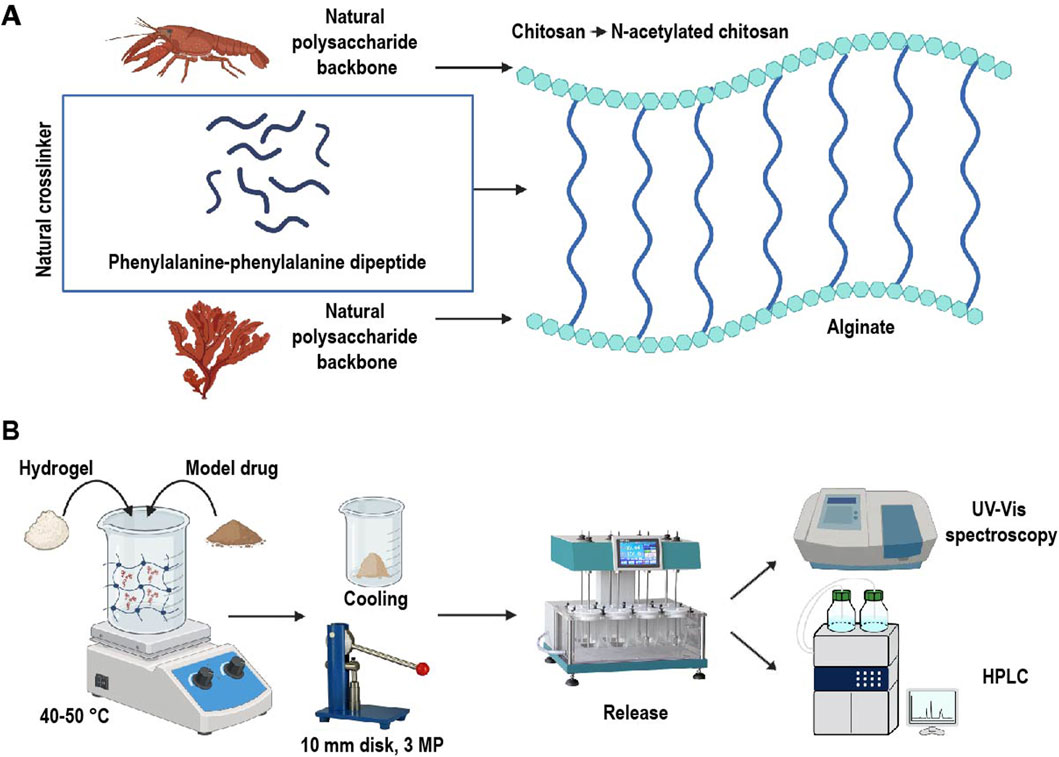
Figure 1. (A) Schematic structure of the proposed hydrogel and (B) drug loading and release process. Chitosan, derived from crustaceans, and alginate, sourced from brown algae, were used as the polymeric backbone of the hydrogel. These polymers were crosslinked with naturally occurring phenylalanine-phenylalanine dipeptide, forming a hybrid hydrogel designed as a pH-sensitive drug delivery system. This system was employed for the controlled release of model drugs hydrocortisone and sulfasalazine.
2 Experimental
2.1 Material
Commercially available materials were utilized without further purification or any modification. The phenylalanine amino acid was purchased from Iris Biotech, and DIEA (N,N-diisopropylethylamine) was obtained from Sigma–Aldrich. Organic solvents, including DMF (dimethylformamide), CH2Cl2 (dichloromethane), MeOH (methanol), and CH3CN (acetonitrile) were purchased from Merck. Alginate and medium molecular weight Chitosan (300–500 kDa, Deacetylation ≥75%) were supplied by Sigma-Aldrich.
Melting points were determined on an Electrothermal 9100 apparatus. Infrared (IR) spectra were obtained on an ABB FT-IR FTLA 2000 spectrometer. Proton nuclear magnetic resonance (1H-NMR) spectra were recorded using Bruker DRX-300 AVANCE spectrometers at 300 MHz, with Dimethyl sulfoxide (DMSO) as the solvent. The release test was performed using the Distek dissolution system 2,500. Drug release data was obtained using the Shimadzu uv-3600 spectrophotometer and a KNAUER Smartline high-performance liquid chromatography (HPLC) system.
2.2 Preparation of phenylalanine-phenylalanine dipeptide (4) crosslinker
The phenylalanine-phenylalanine dipeptide was synthesized through a three-step process, as illustrated in Figure 2. Initially, functional groups on two equal batches of L-phenylalanine (1) were selectively protected to prevent polymerization. In the first batch, the carboxylic acid functional group was protected, yielding L-phenylalanine methyl ester (2), while in the second batch, the amine group was protected, producing (Z)-OSu-Phenylalanine (3). The protected amino acids were then subjected to a solid-phase coupling reaction to form the dipeptide (4). Detailed procedures for each step are outlined in the following sections.
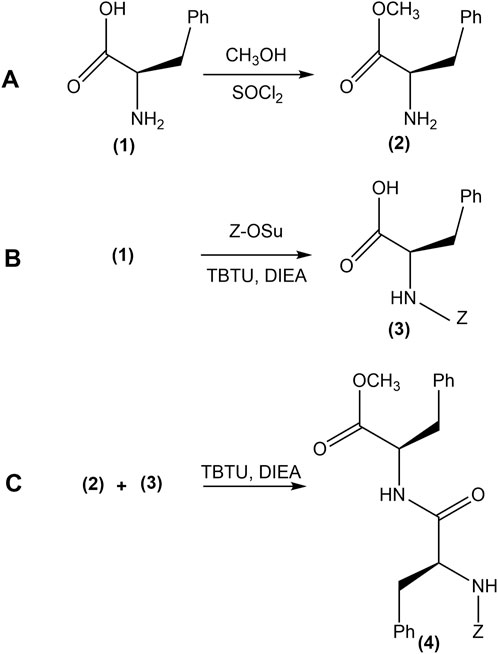
Figure 2. The synthesis process of phenylalanine-phenylalanine dipeptide (4). (A) Protection of the carboxylic acid group of L-phenylalanine (1) by conversion to its methyl ester form, yielding Phe-OMe (2). (B) (Z)-OSu protection of the amino group, forming (Z)-OSu-Phe (3). (C) Solid-phase coupling of the protected amino acids, resulting in the synthesis of (Z)-OSu-Phe-PheOMe (4).
2.2.1 Preparation of L-phenylalanine methyl ester (2)
To synthesize L-phenylalanine methyl ester (Phe-OMe) (2), L-phenylalanine (1) (10 mmol) was dissolved in 30 mL of methanol. Thionyl chloride (3 mmol) was then added dropwise to the reaction mixture while maintaining constant stirring. Due to the exothermic nature of the reaction, the flask was placed in an ice bath to control the temperature. The reaction was allowed to proceed at room temperature for 24 h. Upon completion, the reaction mixture was treated with 50 mL of diethyl ether to precipitate the product. The resulting precipitate was collected via vacuum filtration, washed with cold diethyl ether, and dried under vacuum to yield L-phenylalanine methyl ester (Phe-OMe) (2).
2.2.2 Preparation of (Z)-OSu protected L-phenylalanine (3)
N-(Benzyloxycarbonyloxy)succinimide ((Z)-OSu, (3) (Figure 3) was used as the protecting agent for the amine group in the second batch of L-phenylalanine (1). To initiate the reaction, (Z)-OSu (3) (12 mmol) was dissolved in 20 mL of ethyl acetate, while L-phenylalanine (1) (10 mmol) was dissolved in a 0.25 M sodium bicarbonate solution. The two solutions were combined and stirred at room temperature for 24 h.
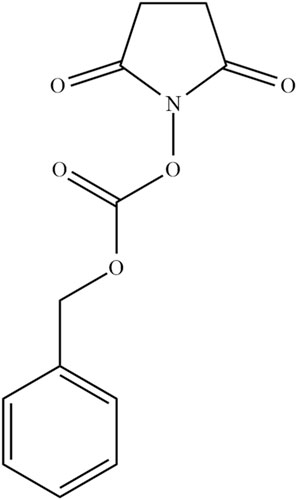
Figure 3. The structure of N-(Benzyloxycarbonyloxy)succinimide ((Z)-OSu) a protecting agent used for the amine functional group of L-phenylalanine amino acid (1).
After the reaction, the pH was adjusted to 3 by adding solid citric acid. The organic phase was then extracted with 30 mL of ethyl acetate and washed with saturated sodium chloride solution to raise the pH to 5, facilitating product precipitation. Finally, the organic phase was evaporated and the resulting (Z)-OSu-protected L-phenylalanine (Z)-OSu-Phe, (3) was dried in a vacuum oven at 40°C.
2.2.3 (Z)-OSu-Phe-Phe-OMe (4) preparation
To synthesize the phenylalanine-phenylalanine dipeptide (4), (Z)-OSu-Phe (3) (10 mmol) was dissolved in 30 mL of ethyl acetate, followed by the addition of N,N-diisopropylethylamine (DIEA) (3.9 g, 30 mmol). The peptide synthesis was carried out by the coupling reagent 2-(1H-Benzotriazole-1-yl)-1,1,3,3-tetramethylaminium tetrafluoroborate (TBTU) (5). TBTU (5) and phenylalanine methyl ester (Phe-OMe) (2) were simultaneously added to the reaction flask and the mixture was stirred at room temperature for 24 h.
Upon completion of the reaction, the mixture was washed successively with 10% w/w sodium carbonate and 10% w/w citric acid solutions to remove unreacted materials. The organic phase was then extracted and washed with a saturated NaCl solution to adjust the pH to 5. The solvent was removed under vacuum, resulting in the production of the (Z)-OSu-Phe-Phe-OMe dipeptide (4) with an 80% yield. The product was dried in a vacuum oven at 40°C overnight. Structural determination of (Z)-OSu-Phe-Phe-OMe dipeptide (4) was performed using 1H-NMR at 300 MHz with DMSO as the solvent.
2.3 Preparation of alginate-phenylalanine-phenylalanine-chitosan (Alg-Phe-Phe-Chit) (10) hydrogel
The hydrogel synthesis followed a four-step process. First, the carboxylic acid functional group of the dipeptide was deprotected. Next, the deprotected dipeptide (5) was coupled with N-acetylated chitosan (6). In the next step, the (Z)-OSu group was removed from the amine functional group of (Z)-OSu-Phe-Phe-Chit (7). Finally, sodium alginate (9) was coupled with NH₂-Phe-Phe-Chit (8), resulting in the formation of the Alg-Phe-Phe-Chit (10) hydrogel. A complete chemical overview of this synthesis is shown in Figure 4, with detailed procedures for each step described in the following sections.
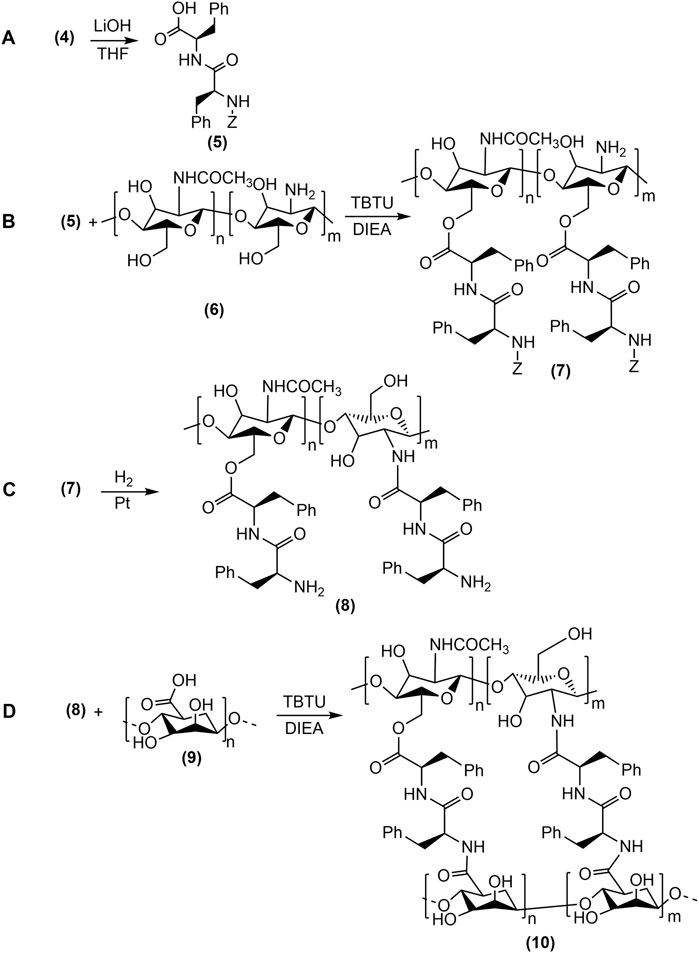
Figure 4. The synthesis of Alg-Phe-Phe-Chit hydrogel (10) was carried out in four steps: (A) Methyl ester deprotection of dipeptide and the formation of (Z)-OSu-Phe-PheOH (5), (B) Coupling of N-acetylated chitosan (6) with (Z)-OSu-Phe-PheOH (5), (C) Deprotection of (Z)-OSu (3) from (Z)-OSu-Phe-Phe-Chit (7), resulting in NH2-Phe-Phe-Chit (8) formation, (D) Coupling reaction of sodium alginate (9) with NH2-Phe-Phe-Chit (8) to form thefinal Alg-Phe-Phe-Chit (10) hydrogel.
2.3.1 Preparation of soluble chitosan by N-acetylation
Following the protocol by Lu et al. (2004) for preparing N-acetylated chitosan (6), a chitosan solution was prepared by dissolving medium molecular weight Chitosan (300–500 kDa, deacetylation ≥75%) (1 g) in 2.8% w/w acetic acid. Ethanol (25 mL) was then added to the reaction, followed by the dropwise addition of pyridine (8 mL). Acetic anhydride (0.5 g) was introduced into the reaction mixture, which was stirred at room temperature for 1 h to facilitate gel formation. The N-acetylated chitosan (6) was precipitated by adding ethanol to the reaction mixture, collected by vacuum filtration, and dried at 50°C for several hours.
2.3.1.1 Deacetylation degree (DD) of N-acetylated chitosan (6)
Deacetylation (DD) degree of N-acetylated chitosan (6) was determined by quantifying its free amino groups through acid-base titration. For this procedure, N-acetylated chitosan (6) (0.3 g) was dissolved in 0.1 M hydrochloric acid (30 mL), with methyl blue as the indicator. The titration was carried out using a standard sodium hydroxide solution, following the method described in the reference (Lin et al., 2005). The degree of deacetylation of N-acetylated chitosan (6) was calculated using the following formulas:
Where C1, V1, C2, and V2 represent the concentrations and volumes of standard solutions.
2.3.1.2 Methyl ester deprotection of dipeptide (4)
For the deprotection of the methyl ester group of the dipeptide, (Z)-OSu-Phe-Phe-OMe dipeptide (4) (10 mmol) was dissolved in tetrahydrofuran (107 mL) at a concentration of 0.14 mmol dipeptide per 1.5 mL tetrahydrofuran. Lithium hydroxide (3.5 M, 0.3 mL) was added, and the reaction mixture was stirred at 60°C for 1 h. Upon completion, the pH was adjusted to 5 using a 5% w/w hydrochloric acid solution. The deprotected dipeptide was isolated by vacuum filtration, followed by extraction with deionized water and dichloromethane. The deprotection was confirmed by comparing the melting point of the resulting (Z)-OSu-Phe-PheOH (5) to existing literature values (157°C–160°C).
2.3.1.3 Coupling of N-acetylated chitosan (6) and (Z)-OSu-Phe-PheOH (5)
In this step, the coupling method outlined in section 2.2.3 was employed to synthesize (Z)-OSu-Phe-Phe-Chit (7).
2.3.1.4 Coupling of sodium alginate (9) and (Z)-OSu-Phe-Phe-Chit (7)
Initially, (Z)-OSu-Phe-Phe-Chit (7) was dissolved in methanol, and pure palladium (1 g) was added as a catalyst. The reaction mixture was stirred at room temperature for 24 h under a hydrogen atmosphere. Upon completion, the palladium catalyst was removed by filtration through Celite, and the solvent was evaporated under vacuum. The resulting NH2-Phe-Phe-Chit (8) was dried overnight in a vacuum oven at 40°C. Subsequently, the coupling method described in section 2.2.3 was used to couple sodium alginate (9) with NH2-Phe-Phe-Chit (8), and the resulting Alg-Phe-Phe-Chit (10) was extracted using water and ethanol.
2.3.2 FT-IR
Fourier transform infrared spectroscopy (FT-IR) was used to confirm the coupling reactions and verify the synthesis of the desired products at each stage. The FT-IR analysis was conducted using potassium bromide (KBr) tablets, with a resolution of 1 cm−1, spanning the wavelength range of 400–4,000 cm−1.
2.4 Alg-Phe-Phe-Chit (10) hydrogel: swelling, drug loading, and release
2.4.1 Swelling of Alg-Phe-Phe-Chit (12) hydrogel
The swelling behavior of the Alg-Phe-Phe-Chit (10) hydrogel was evaluated using gravimetric analysis. A compressed disk of Alg-Phe-Phe-Chit (10) (10 mg) with a diameter of 10 mm was prepared under the pressure of 3 megapascal (MPa) and immersed in phosphate buffer (pH 7.5) to simulate colonic pH condition. The disk remained in the buffer for 24 h, and its weight change was monitored over time. The water uptake of the hydrogel was calculated using the following formula, based on the observed weight changes during the experiment:
Where W0 represents the initial weight of the Alg-Phe-Phe-Chit hydrogel (10) and Wt is the weight of the hydrogel through time.
2.4.2 Hydrogel drug loading
In this study, hydrocortisone and sulfasalazine were selected as model drugs. To prepare the drug-loaded hydrogel, 50 mg of Alg-Phe-Phe-Chit (10) was mixed with 10 mg of each model drug. The mixture was heated to 40°C–50°C for 2–3 min to facilitate drug incorporation into the hydrogel matrix. After the drugs were fully absorbed into the swollen hydrogel, the sample was cooled for several minutes. The drug-loaded hydrogel was then compacted into 10 mm disks under a pressure of 3 MPa, in preparation for the drug release test.
2.4.3 Drug release evaluation using dissolution testing and HPLC analysis
The drug release test was conducted using a dissolution method in a simulated colonic pH environment with phosphate buffer (900 mL, pH 7.5). Hydrogel disks were placed in dissolution cells maintained at 37°C, with agitation set at 100 rpm. Drug release was monitored by measuring the absorbance of samples at specific time intervals using UV-Vis spectroscopy, with readings at predetermined reference wavelengths (254 nm for hydrocortisone and 385 nm for sulfasalazine). A standard solution of the model drugs served as the reference for quantifying the drug release. Absorbance measurements were recorded every hour for up to 5 h.
To confirm drug release over the 5 h, HPLC was employed. A standard solution of each model drug served as a reference for calculating the drug release. Chromatographic separation was performed using an octadecylsilyl groups (ODS) (C18) column (5 μm, 4.6 × 150 mm), with a mobile phase consisting of methanol, water, and acetic acid in a 60:30:10 (v/v/v) ratio. The mobile phase was delivered at a flow rate of 1.0 mL/min. Drug release was calculated using the following equation:
Where Asample represents the absorption (UV-Vis spectroscopy) and peak area (HPLC) of the sample solution, and ASTD represents the absorption and peak area of the standard solution, respectively.
3 Results
3.1 (Z)-OSu-Phe-Phe-OMe dipeptide (4) synthesis
The chemical structure of the dipeptide (Z)-OSu-Phe-Phe-OMe (4) was confirmed by 1H-NMR analysis in DMSO at 300 MHz. The 1H-NMR spectrum (Figure 5) exhibited several characteristic peaks. The diastereotopic hydrogens of the methylene groups appeared as multiple peaks in the range of 2.8–3.10 ppm (Figure 5, peak 1). A distinct singlet corresponding to the methoxy group was observed at 3.67 ppm (Figure 5, peak 2). The methylene group of the (Z)-OSu protecting agent produced a singlet peak at 5.1 ppm (Figure 5, peak 3). Additionally, two pairs of doublets in the regions of 4.5–4.8 ppm and 4.9–5.3 ppm were attributed to the protons at the chiral centers of the dipeptide (Figure 5, peak 4), which could couple with two diastereotopic hydrogens of methylene groups, confirming the successful synthesis of the desired compound.
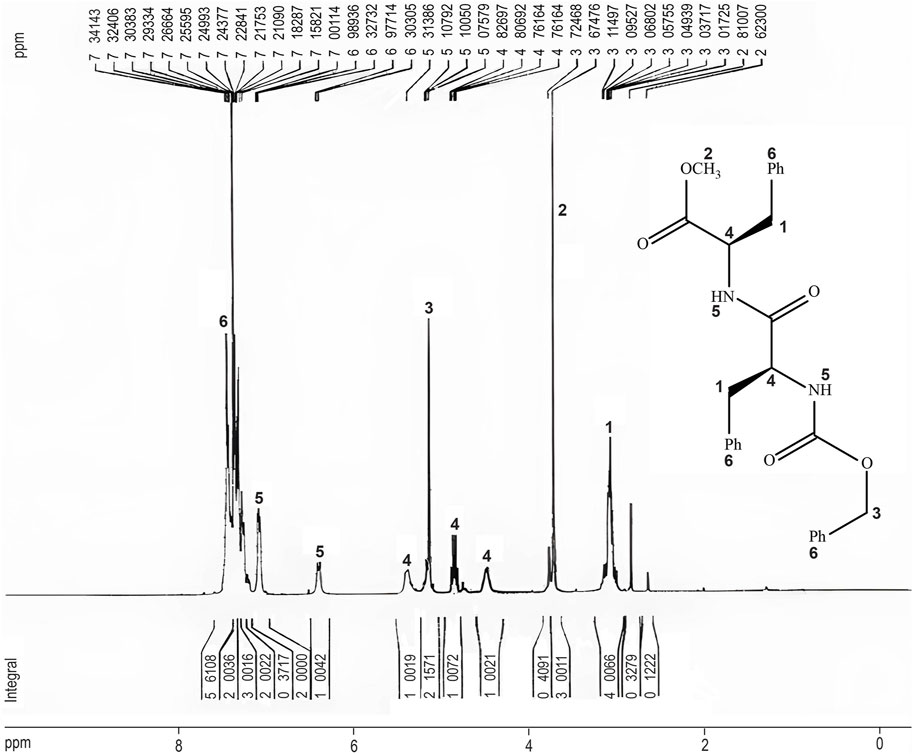
Figure 5. The 1H-NMR spectrum of (Z)-OSu-Phe-Phe-OMe (4). The structure of the dipeptide is illustrated, with corresponding peaks in the spectrum indicated in numbers. The 1H-NMR analysis confirmed the synthesis of the (Z)-OSu-Phe-Phe-OMe (4), with characteristic chemical shifts matching the expected functional groups.
3.2 Soluble chitosan preparation and characterization
The synthesis of soluble chitosan was investigated through three different methods. The first method involved ether bond hydrolysis using hydrogen peroxide (Xia et al., 2013), producing soluble low molecular weight chitosan. However, this approach was limited by low yields, harsh reaction conditions, and substantial residue formation. In a second method, ((Z)-OSu) (3) was used to partially protect the amine groups of chitosan, but the final product was obtained in low yields. The most successful method involved the synthesizing partially N-acetylated chitosan (6) by protecting the amino groups with acetic anhydride, known to enhance solubility in organic solvents. Despite a reaction time of 24 h, this method resulted in the desired chitosan with a reasonable yield. A comparative solubility test in ethyl acetate for 24 h demonstrated that N-acetylated chitosan (6) exhibited significantly improved solubility. The degree of deacetylation (DD) was determined to be 58% using acid-base titration. Additionally, the formation of amide bonds was confirmed by a characteristic peak at 1,668 cm−1 in the FT-IR spectrum.
3.3 Alg-Phe-Phe-Chit hydrogel (10) synthesis and characterization
For the synthesis of Alg-Phe-Phe-Chit hydrogel (10), the methyl ester deprotection of (Z)-OSu-Phe-Phe-OMe dipeptide (4) was successfully achieved. The preparation of (Z)-OSu-Phe-PheOH (5) was confirmed by its melting point, which matched the reference values (157°C–160°C). FT-IR analysis (KBr pellet) of (Z)-OSu-Phe-PheOH (5) revealed characteristic peaks at 3,443 cm−1, 1714 cm−1, and 1,658 cm−1, corresponding to O-H stretching in the carboxylic acid, C=O stretching in the carboxylic acid, and the amide group of the dipeptide, respectively. The subsequent coupling of N-acetylated chitosan (6) with (Z)-OSu-Phe-Phe-OH (5) was monitored by FT-IR, which showed the formation of an ester bond through the appearance of a new carbonyl peak at 1724 cm⁻1 (Figure 6).
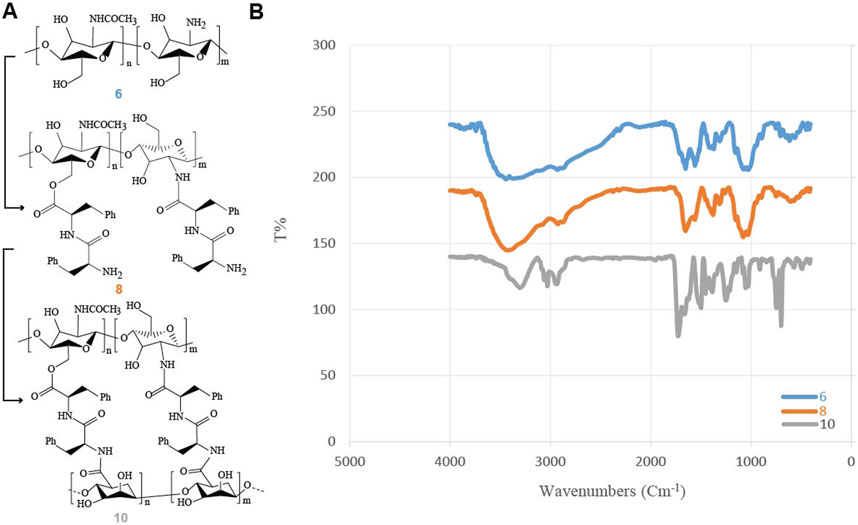
Figure 6. The FT-IR analysis of Alg-Phe-Phe-Chit hydrogel (10) synthesis. (A) Overview of the synthetic process for Alg-Phe-Phe-Chit hydrogel (10). (B) Comparison of IR spectra for N-acetylated chitosan (6), NH2-OSu-Phe-Phe-Chit (8) and Alg-Phe-Phe-Chit hydrogel (10), highlighting spectral changes during the coupling reactions that confirms successful hydrogel formation.
Following the deprotection of (Z)-OSu-Phe-Phe-Chit (7) to its amine form, NH2-Phe-Phe-Chit (8) was successfully coupled with sodium alginate (9). The FT-IR spectrum of NH2-Phe-Phe-Chit (8) displayed bands at 3,299 cm−1 and 2,929 cm−1, corresponding to O-H and N-H stretching, respectively. Additional peaks at 1726 cm−1, 1,654 cm−1, and 1,620 cm−1 were attributed to C=O stretching in ester and amide functional groups. The formation of the final Alg-Phe-Phe-Chit hydrogel (10) was confirmed by FT-IR, with peaks at 1718 cm−1 and 1,625 cm−1, indicating C=O stretching in the ester and amide groups. Bands at 3,276 cm−1 and 2,929 cm−1 were observed, corresponding to hydroxyl and amine groups, respectively. Additionally, fingerprint peaks at 698 cm−1 and 752 cm−1 confirmed the presence of C-C and C-N bond stretches. Figure 6 illustrates the significant changes in the FT-IR spectra throughout the formation of the Alg-Phe-Phe-Chit hydrogel (10).
3.4 Alg-Phe-Phe-Chit hydrogel (12) swelling
The swelling behavior of the hydrogel in a medium that mimicked colonic pH was also assessed. After 24 h, the hydrogel exhibited a 30% increase in swelling, with its weight increasing from 50 to 65 mg in phosphate buffer (pH 7.5) (Table 1).
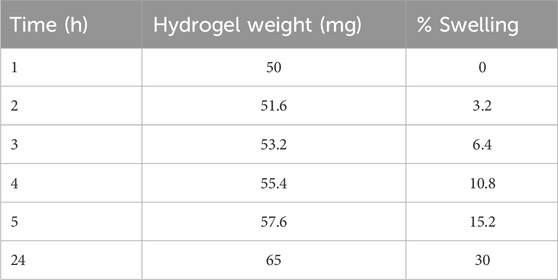
Table 1. Swelling behavior of Alg-Phe-Phe-Chit (10) hydrogel in phosphate buffer (pH 7.5). The hydrogel showed a 30% increase in swelling up to 24 h.
3.5 Drug loading in Alg-Phe-Phe-Chit hydrogel (12)
In chitosan-based hydrogels cross-linked with polymers like alginate, the conventional method for drug loading typically involves immersing the fully formed hydrogel in a drug-saturated medium (Bhattarai et al., 2010). However, the Alg-Phe-Phe-Chit hydrogel (10) demonstrated limited drug loading capacity using this method. Notably, this hydrogel exhibited unique swelling properties compared to previously reported cross-linked chitosan-alginate hydrogels. Specifically, the Alg-Phe-Phe-Chit hydrogel (10) demonstrated swelled at temperatures between 40°C–50°C, followed by precipitation upon cooling. This behavior was evident during hydrogel synthesis, during the solvent evaporation under vacuum at 40°C resulted in swelling and subsequent precipitation upon cooling. These unique swelling properties were leveraged in the drug loading procedure described in section 2.3.2, enabling a solvent-free drug loading method with a remarkable 100% drug loading capacity, while minimizing drug waste by eliminating the need for solvents during the loading process.
3.6 Hydrocortisone and sulfasalazine release from Alg-Phe-Phe-Chit hydrogel (12)
A dissolution test was performed to evaluate the release of hydrocortisone and sulfasalazine in a colonic pH medium. The experimental parameters, including the mobile phase, chromatography column, and release formula were adapted from the United States Pharmacopeia (USP) and British Pharmacopeia (BP). A summary of the drug release results is provided in Table 2. UV-Vis spectroscopy indicated that 35% of hydrocortisone was released within the first hour, increasing to 49% after 5 h (Figure 7). In contrast, sulfasalazine, showed a faster release pattern, with over 65% of the drug released in the first hour and reaching 75% by the 5-h mark (Figure 8). HPLC analysis confirmed the UV-Vis findings, revealing release rates of 51% for hydrocortisone and 77.6% for sulfasalazine over the same period. In the HPLC analysis, hydrocortisone was eluted at approximately 8 min and sulfasalazine at 18 and 19 min, with the phosphate buffer eluting between 0 and 5 min.
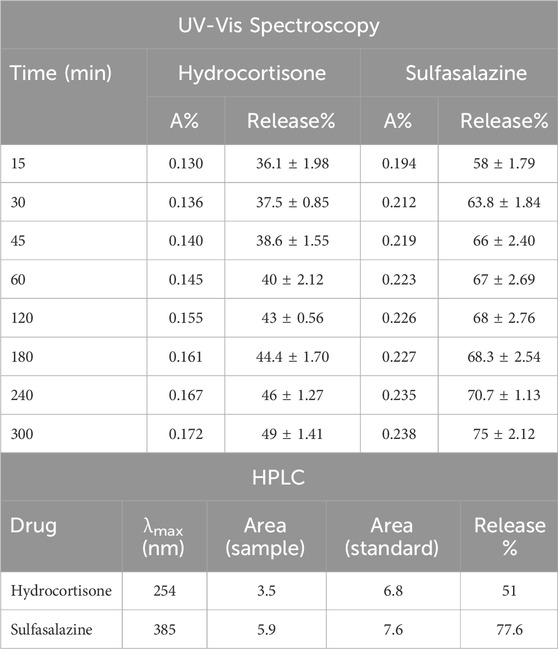
Table 2. Drug release data for hydrocortisone and sulfasalazine, obtained from UV-Vis spectroscopy (top panel) and HPLC (bottom panel) over 5 h. The release data from UV-Vis at 5 h was consistent with HPLC results. Hydrocortisone exhibited a release of 49% via UV-Vis and 51% via HPLC. Sulfasalazine showed a release of 75% via UV-Vis and 77.6% via HPLC. Data are presented as Mean ± standard deviation (SD), with results generated from two experimental replicates.
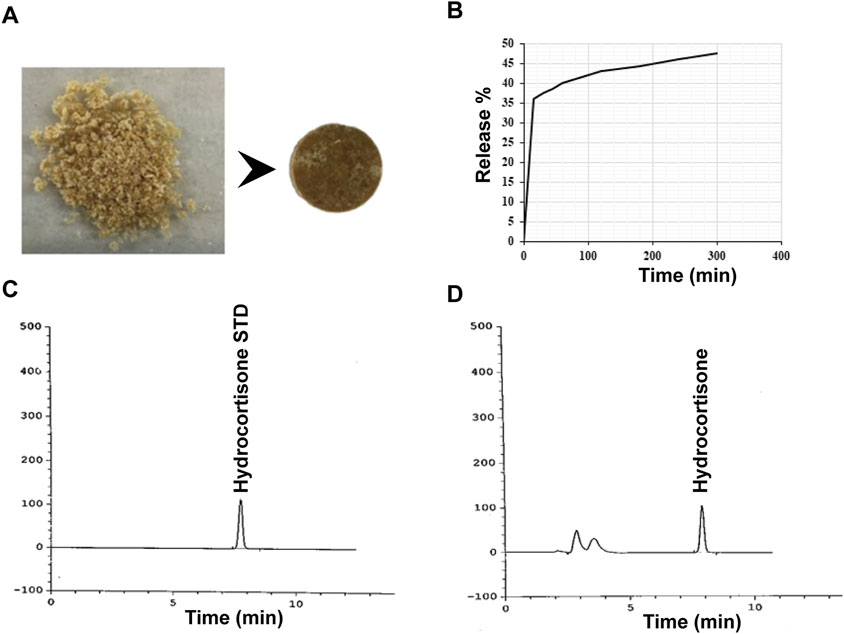
Figure 7. Release profile of hydrocortisone. (A) Image of hydrocortisone loaded Alg-Phe-Phe-Chit disk, (B) UV-Vis release pattern of hydrocortisone showing an initial release of 35% within the first hour, increasing to 49% after 5 h. HPLC profile for standard (C) and sample (D) solutions of hydrocortisone. The release data indicates a consistent finding of 51% hydrocortisone release, confirming the UV-Vis analysis results.
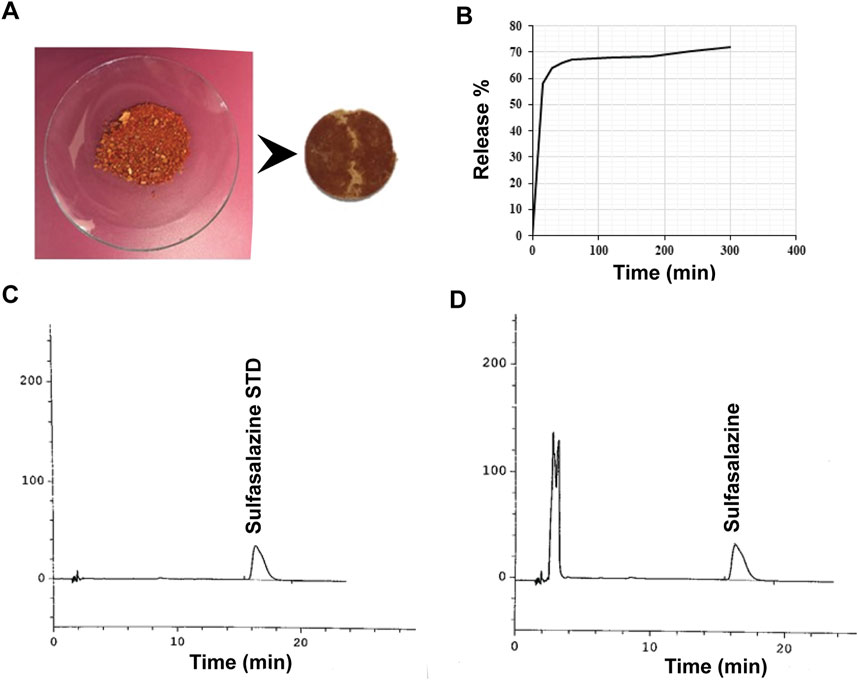
Figure 8. Sulfasalazine release profile. (A) Image of sulfasalazine-loaded Alg-Phe-Phe-Chit disk. (B) UV-Vis analysis indicated a release of 58% within the first hour, increasing to 75% after 5 h. HPLC profiles for the standard (C) and sample (D) solutions of sulfasalazine. HPLC analysis of released sulfasalazine from the hydrogel confirmed a release of 77.6% after 5 h, consistent with the UV-Vis analysis results.
4 Discussion
The structural analysis of the phenylalanine-phenylalanine dipeptide crosslinker provided valuable insights into the functional groups and stereochemistry. Peaks observed between 2.8 and 3.10 ppm confirmed the presence of diastereotopic hydrogens in the methylene groups, while a single peak at 3.67 ppm indicated uniform methoxy groups. A distinct peak at 5.1 ppm for the (Z)-OSu protecting agent confirmed its successful attachment to the dipeptide, a critical step for controlling reactivity during synthesis. The presence of doublet peaks around 4.5–4.8 ppm and 4.9–5.3 ppm, corresponding to chiral centers, showed the stereospecificity of the molecule. Additionally, peaks at 6.8 ppm and 7.1 ppm in the aromatic region were attributed to the hydrogens of the amide groups (Figure 5, peak 5), while peaks above 7 ppm were associated with the aromatic hydrogens of the phenyl groups (Figure 5, peak 6), confirming the presence of the phenyl groups in the structure (Pavia et al., 2015).
A significant challenge in synthesizing the Alg-Phe-Phe-Chit hydrogel (10) was obtaining a soluble chitosan derivative for effective coupling reactions. Among the various approaches tested, the partial N-acetylation of chitosan (Lu et al., 2004) using acetic anhydride yielded the most favourable results. In contrast to the hydrolysis method (Xia et al., 2013) which resulted in low yield and involved challenging reaction conditions, N-acetylation produced chitosan with enhanced solubility, particularly in organic solvents like ethyl acetate. This improved solubility facilitates efficient coupling reactions. The degree of deacetylation was determined to be 58% through acid-base titration, further demonstrating the effectiveness of acetic anhydride in achieving the desired degree of acetylation. Additionally, the presence of a peak at 1,668 cm−1 in the FT-IR spectrum confirmed the successful formation of amide bonds, validating the structural integrity of the synthesized N-acetylated chitosan (6).
The synthesis of the Alg-Phe-Phe-Chit hydrogel (10) involved critical reaction stages, as confirmed by FT-IR spectroscopy (Pavia et al., 2015) and melting point analysis. The successful deprotection of (Z)-OSu-Phe-Phe-OMe dipeptide (4) and its conversion to (Z)-OSu-Phe-PheOH (5) was validated by its melting point and characteristic FT-IR peaks. Notably, the appearance of the carbonyl peak at 1724 cm−1 confirmed the formation of ester bonds during the coupling reaction between N-acetylated chitosan (6) and the dipeptide, highlighting the efficiency of the synthetic pathway. FT-IR analysis of the final hydrogel revealed the characteristic peaks for O-H, N-H, and C=O stretching, confirming the presence of ester and amide groups in the cross-linked structure. This comprehensive characterization of chemical changes throughout the synthesis process (Figure 6) underscores the robustness of the methodology in forming a well-defined hydrogel structure, which directly impacts its efficacy in controlling drug release and enhancing its stability in physiological conditions (Thang et al., 2023).
The unique swelling behavior observed in the Alg-Phe-Phe-Chit hydrogel (10) presents an innovative approach to drug loading, setting it apart from conventional chitosan-alginate hydrogels (Baysal et al., 2013). The hydrogel’s ability to swell at temperatures between 40°C–50°C, followed by precipitation upon cooling, enables a solvent-free drug-loading process that achieves a 100% drug-loading capacity. This method not only improves drug loading efficiency but also significantly reduces solvent use and drug waste. This in turn enhances patient safety by avoiding potential toxicity or side effects that may arise from residual solvents while decreasing the overall cost of manufacturing (Kumar et al., 2024).
The temperature-dependent swelling behavior is likely influenced by the thermal properties of the phenylalanine-phenylalanine dipeptide cross-linker, which undergoes structural changes that enhance drug entrapment. Previous studies have shown that simple dipeptides, such as L-phenylalanine, can readily self-assemble and form β-amyloid proteins (Sedman et al., 2006). Thermal stability assessments have demonstrated that as the temperature increases, the structural integrity of phenylalanine-phenylalanine blocks diminishes, leading to a loss of free phenylalanine. This degradation was observed over a temperature range of 25°C–200°C (Sedman et al., 2006). These findings suggest that the drug-loading process conducted at 50°C may be facilitated by a reduction in the structural integrity of the phenylalanine-phenylalanine cross-linker, allowing for the effective drug entrapment (Sedman et al., 2006). This temperature-dependent structural alteration highlights the efficiency of the newly developed drug loading method for the Alg-Phe-Phe-Chit hydrogel (10), positioning it as a promising platform for targeted drug delivery applications.
The dissolution study demonstrated the hydrogel’s effectiveness as a carrier for hydrocortisone and sulfasalazine under colonic pH conditions. The burst release of hydrocortisone (35% in the first hour) followed by a slower, sustained release pattern (49% in 5 h), suggests a dual-phase release mechanism (Kasiński et al., 2023) 02. This behavior is likely due to the initial release of surface-bound drugs, followed by the gradual diffusion of drug molecules through the hydrogel matrix. The consistency between UV-Vis spectroscopy and HPLC data reinforces the reliability of these findings, with HPLC confirming 51% release in 5 h. Sulfasalazine exhibited a faster release profile, with over 65% of the drug released in the first hour and up to 77.6% after 5 h, as confirmed by both UV-Vis and HPLC analyses. The rapid initial release may be attributed to sulfasalazine’s hydrophilic nature (Ol’khovich et al., 2017), allowing it to readily diffuse through the hydrogel matrix in aqueous environments. The close agreement between UV-Vis and HPLC results for both drugs and the consistent retention times observed during HPLC analysis, further validate the accuracy of the release data.
In summary, the Alg-Phe-Phe-Chit hydrogel (10) exhibited an optimal balance of swelling properties, drug-loading capacity, and controlled release profile under colonic pH conditions, making it a promising candidate for pH-responsive drug delivery systems. Its ability to effectively encapsulate and release drug models in simulated colonic environments underscores its potential for targeted colon-specific drug delivery. Further refinement and optimization of the hydrogel synthesis parameters could significantly enhance its performance, paving the way for broader applications in both localized and systemic drug delivery strategies.
5 Conclusion
In this study, the synthesis, characterization, and drug delivery potential of the Alg-Phe-Phe-Chit hydrogel were thoroughly investigated. The hydrogel’s unique swelling properties at elevated temperatures enabled the development of a solvent-free drug-loading process, achieving 100% drug-loading capacity. This innovative approach highlights the hydrogel’s efficiency in incorporating therapeutic agents without waste. The thermal behavior of the phenylalanine-phenylalanine dipeptide cross-linker played a crucial role in facilitating this high drug-loading efficiency. The results underscore the hydrogel’s potential as a carrier for pH-responsive drug delivery, offering both immediate and sustained release profiles. The solvent-free loading process and high drug loading capacity reinforce the hydrogel’s suitability for targeted drug delivery and controlled release. Future studies should focus on optimizing the hydrogel composition and evaluating its efficacy across a broader range of drugs to maximize its potential for specific drug delivery applications.
Data availability statement
The original contributions presented in the study are included in the article/supplementary material, further inquiries can be directed to the corresponding authors.
Author contributions
NK: Writing–original draft. MN-J: Writing–review and editing, Supervision. SB: Writing–review and editing, Supervision. AS: Writing–review and editing, Supervision.
Funding
The author(s) declare that financial support was received for the research, authorship, and/or publication of this article. This study is funded by the Iran University of Science and Technology.
Acknowledgments
We thank Aburaihan Pharmaceutical Company for providing us with the equipment including dissolution test and HPLC.
Conflict of interest
The authors declare that the research was conducted in the absence of any commercial or financial relationships that could be construed as a potential conflict of interest.
Publisher’s note
All claims expressed in this article are solely those of the authors and do not necessarily represent those of their affiliated organizations, or those of the publisher, the editors and the reviewers. Any product that may be evaluated in this article, or claim that may be made by its manufacturer, is not guaranteed or endorsed by the publisher.
References
Alqahtani, M. S., Kazi, M., Alsenaidy, M. A., and Ahmad, M. Z. (2021). Advances in oral drug delivery. Front. Pharmacol. 12, 618411. doi:10.3389/fphar.2021.618411
Anal, A. K., and Stevens, W. F. (2005). Chitosan–alginate multilayer beads for controlled release of ampicillin. Int. J. Pharm. 290 (1-2), 45–54. doi:10.1016/j.ijpharm.2004.11.015
Baysal, K., Aroguz, A. Z., Adiguzel, Z., and Baysal, B. M. (2013). Chitosan/alginate crosslinked hydrogels: preparation, characterization and application for cell growth purposes. Int. J. Biol. Macromol. 59, 342–348. doi:10.1016/j.ijbiomac.2013.04.073
Bhattarai, N., Gunn, J., and Zhang, M. (2010). Chitosan-based hydrogels for controlled, localized drug delivery. Adv. drug Deliv. Rev. 62 (1), 83–99. doi:10.1016/j.addr.2009.07.019
Caccavo, D., Cascone, S., Lamberti, G., Barba, A. A., and Larsson, A. (2016). “Swellable hydrogel-based systems for controlled drug delivery,” in Smart drug delivery system (IntechOpen), 237–303.
Casadei, M. A., Pitarresi, G., Calabrese, R., Paolicelli, P., and Giammona, G. (2008). Biodegradable and pH-sensitive hydrogels for potential colon-specific drug delivery: characterization and in vitro release studies. Biomacromolecules 9 (1), 43–49. doi:10.1021/bm700716c
Chen, S.-C., Wu, Y.-C., Mi, F.-L., Lin, Y.-H., Yu, L.-C., and Sung, H.-W. (2004). A novel pH-sensitive hydrogel composed of N, O-carboxymethyl chitosan and alginate cross-linked by genipin for protein drug delivery. J. Control. Release 96 (2), 285–300. doi:10.1016/j.jconrel.2004.02.002
Cheung, R. C. F., Ng, T. B., Wong, J. H., and Chan, W. Y. (2015). Chitosan: an update on potential biomedical and pharmaceutical applications. Mar. drugs 13 (8), 5156–5186. doi:10.3390/md13085156
Ching, A. L., Liew, C. V., Chan, L. W., and Heng, P. W. S. (2008). Modifying matrix micro-environmental pH to achieve sustained drug release from highly laminating alginate matrices. Eur. J. Pharm. Sci. 33 (4-5), 361–370. doi:10.1016/j.ejps.2008.01.007
He, Q., and Shi, J. (2011). Mesoporous silica nanoparticle based nano drug delivery systems: synthesis, controlled drug release and delivery, pharmacokinetics and biocompatibility. J. Mater. Chem. 21 (16), 5845–5855. doi:10.1039/c0jm03851b
Hua, S., Marks, E., Schneider, J. J., and Keely, S. (2015). Advances in oral nano-delivery systems for colon targeted drug delivery in inflammatory bowel disease: selective targeting to diseased versus healthy tissue. Nanomedicine Nanotechnol. Biol. Med. 11 (5), 1117–1132. doi:10.1016/j.nano.2015.02.018
Karoyo, A. H., and Wilson, L. D. (2021). A review on the design and hydration properties of natural polymer-based hydrogels. Materials 14 (5), 1095. doi:10.3390/ma14051095
Kasiński, A., Świerczek, A., Zielińska-Pisklak, M., Kowalczyk, S., Plichta, A., Zgadzaj, A., et al. (2023). Dual-Stimuli-sensitive smart hydrogels containing magnetic nanoparticles as antitumor local drug delivery systems—synthesis and characterization. Int. J. Mol. Sci. 24 (8), 6906. doi:10.3390/ijms24086906
Kaur, A., Kaur, A., P Kaur, V., Kaur, M., and Murthy, R. (2014). Polymeric drug delivery approaches for colon targeting: a review. Drug Deliv. Lett. 4 (1), 38–48. doi:10.2174/22103031113036660017
Kesharwani, P., Bisht, A., Alexander, A., Dave, V., and Sharma, S. (2021). Biomedical applications of hydrogels in drug delivery system: an update. J. Drug Deliv. Sci. Technol. 66, 102914. doi:10.1016/j.jddst.2021.102914
Koev, T. T., Harris, H. C., Kiamehr, S., Khimyak, Y. Z., and Warren, F. J. (2022). Starch hydrogels as targeted colonic drug delivery vehicles. Carbohydr. Polym. 289, 119413. doi:10.1016/j.carbpol.2022.119413
Kong, H. J., Smith, M. K., and Mooney, D. J. (2003). Designing alginate hydrogels to maintain viability of immobilized cells. Biomaterials 24 (22), 4023–4029. doi:10.1016/s0142-9612(03)00295-3
Kumar, A., Saha, M., Vishwakarma, R., Behera, K., and Trivedi, S. (2024). Green solvents tailored nanostructures of block copolymers and their potential applications in drug delivery. J. Mol. Liq. 410, 125642. doi:10.1016/j.molliq.2024.125642
Lee, K. Y., and Mooney, D. J. (2012). Alginate: properties and biomedical applications. Prog. Polym. Sci. 37 (1), 106–126. doi:10.1016/j.progpolymsci.2011.06.003
Li, J., and Kataoka, K. (2020). Chemo-physical strategies to advance the in vivo functionality of targeted nanomedicine: the next generation. J. Am. Chem. Soc. 143 (2), 538–559. doi:10.1021/jacs.0c09029
Li, J., and Mooney, D. J. (2016). Designing hydrogels for controlled drug delivery. Nat. Rev. Mater. 1 (12), 16071. doi:10.1038/natrevmats.2016.71
Li, L., Lei, D., Zhang, J., Xu, L., Li, J., Jin, L., et al. (2022). Dual-responsive alginate hydrogel constructed by sulfhdryl dendrimer as an intelligent system for drug delivery. Molecules 27 (1), 281. doi:10.3390/molecules27010281
Lin, Y.-H., Liang, H.-F., Chung, C.-K., Chen, M.-C., and Sung, H.-W. (2005). Physically crosslinked alginate/N, O-carboxymethyl chitosan hydrogels with calcium for oral delivery of protein drugs. Biomaterials 26 (14), 2105–2113. doi:10.1016/j.biomaterials.2004.06.011
Liu, L., Yao, W., Rao, Y., Lu, X., and Gao, J. (2017). pH-Responsive carriers for oral drug delivery: challenges and opportunities of current platforms. Drug Deliv. 24 (1), 569–581. doi:10.1080/10717544.2017.1279238
Lu, S., Song, X., Cao, D., Chen, Y., and Yao, K. (2004). Preparation of water-soluble chitosan. J. Appl. Polym. Sci. 91 (6), 3497–3503. doi:10.1002/app.13537
Maxwell, C. J., Soltisz, A. M., Rich, W. W., Choi, A., Reilly, M. A., and Swindle-Reilly, K. E. (2022). Tunable alginate hydrogels as injectable drug delivery vehicles for optic neuropathy. J. Biomed. Mater. Res. Part A 110 (10), 1621–1635. doi:10.1002/jbm.a.37412
McCoubrey, L. E., Favaron, A., Awad, A., Orlu, M., Gaisford, S., and Basit, A. W. (2023). Colonic drug delivery: formulating the next generation of colon-targeted therapeutics. J. Control. Release 353, 1107–1126. doi:10.1016/j.jconrel.2022.12.029
Mi, F.-L., Sung, H.-W., and Shyu, S.-S. (2002). Drug release from chitosan–alginate complex beads reinforced by a naturally occurring cross-linking agent. Carbohydr. Polym. 48 (1), 61–72. doi:10.1016/s0144-8617(01)00212-0
Miyazaki, S., Nakayama, A., Oda, M., Takada, M., and Attwood, D. (1995). Drug release from oral mucosal adhesive tablets of chitosan and sodium alginate. Int. J. Pharm. 118 (2), 257–263. doi:10.1016/0378-5173(94)00396-m
Motasadizadeh, H., Tavakoli, M., Damoogh, S., Mottaghitalab, F., Gholami, M., Atyabi, F., et al. (2022). Dual drug delivery system of teicoplanin and phenamil based on pH-sensitive silk fibroin/sodium alginate hydrogel scaffold for treating chronic bone infection. Biomater. Adv. 139, 213032. doi:10.1016/j.bioadv.2022.213032
Murata, Y., Miyamoto, E., and Kawashima, S. (1996). Additive effect of chondroitin sulfate and chitosan on drug release from calcium-induced alginate gel beads. J. Control. Release 38 (2-3), 101–108. doi:10.1016/0168-3659(95)00098-4
Nandini, V. V., Venkatesh, K. V., and Nair, K. C. (2008). Alginate impressions: a practical perspective. J. Conservative Dent. 11 (1), 37–41. doi:10.4103/0972-0707.43416
Ol’khovich, M. V., Sharapova, A. V., Blokhina, S. V., and Perlovich, G. L. (2017). Sulfasalazine: dissolution and distribution in pharmaceutically relevant mediums. J. Chem. Eng. Data 62 (1), 123–128. doi:10.1021/acs.jced.6b00497
Rathi, R., Sanshita, S., Kumar, A., Vishvakarma, V., Huanbutta, K., Singh, I., et al. (2022). Advancements in rectal drug delivery systems: clinical trials, and patents perspective. Pharmaceutics 14 (10), 2210. doi:10.3390/pharmaceutics14102210
Ribeiro, A. J., Silva, C., Ferreira, D., and Veiga, F. (2005). Chitosan-reinforced alginate microspheres obtained through the emulsification/internal gelation technique. Eur. J. Pharm. Sci. 25 (1), 31–40. doi:10.1016/j.ejps.2005.01.016
Saeedi, M., Vahidi, O., Moghbeli, M. R., Ahmadi, S., Asadnia, M., Akhavan, O., et al. (2022). Customizing nano-chitosan for sustainable drug delivery. J. Control. Release 350, 175–192. doi:10.1016/j.jconrel.2022.07.038
Sedman, V. L., Adler-Abramovich, L., Allen, S., Gazit, E., and Tendler, S. J. (2006). Direct observation of the release of phenylalanine from diphenylalanine nanotubes. J. Am. Chem. Soc. 128 (21), 6903–6908. doi:10.1021/ja060358g
Soppimath, K. S., Aminabhavi, T. M., Kulkarni, A. R., and Rudzinski, W. E. (2001). Biodegradable polymeric nanoparticles as drug delivery devices. J. Control. release 70 (1-2), 1–20. doi:10.1016/s0168-3659(00)00339-4
Szekalska, M., Puciłowska, A., Szymańska, E., Ciosek, P., and Winnicka, K. (2016). Alginate: current use and future perspectives in pharmaceutical and biomedical applications. Int. J. Polym. Sci. 2016 (1), 7697031. doi:10.1155/2016/7697031
Thang, N. H., Chien, T. B., and Cuong, D. X. (2023). Polymer-based hydrogels applied in drug delivery: an overview. Gels 9 (7), 523. doi:10.3390/gels9070523
Tian, B., and Liu, J. (2023). Smart stimuli-responsive chitosan hydrogel for drug delivery: a review. Int. J. Biol. Macromol. 235, 123902. doi:10.1016/j.ijbiomac.2023.123902
Van Den Abeele, J., Rubbens, J., Brouwers, J., and Augustijns, P. (2017). The dynamic gastric environment and its impact on drug and formulation behaviour. Eur. J. Pharm. Sci. 96, 207–231. doi:10.1016/j.ejps.2016.08.060
Varghese, S. A., Rangappa, S. M., Siengchin, S., and Parameswaranpillai, J. (2020). “Natural polymers and the hydrogels prepared from them,” in Hydrogels based on natural polymers (Elsevier), 17–47.
Wang, Q.-S., Wang, G.-F., Zhou, J., Gao, L.-N., and Cui, Y.-L. (2016). Colon targeted oral drug delivery system based on alginate-chitosan microspheres loaded with icariin in the treatment of ulcerative colitis. Int. J. Pharm. 515 (1-2), 176–185. doi:10.1016/j.ijpharm.2016.10.002
Xia, Z., Wu, S., and Chen, J. (2013). Preparation of water soluble chitosan by hydrolysis using hydrogen peroxide. Int. J. Biol. Macromol. 59, 242–245. doi:10.1016/j.ijbiomac.2013.04.034
Xu, Y., Zhan, C., Fan, L., Wang, L., and Zheng, H. (2007). Preparation of dual crosslinked alginate–chitosan blend gel beads and in vitro controlled release in oral site-specific drug delivery system. Int. J. Pharm. 336 (2), 329–337. doi:10.1016/j.ijpharm.2006.12.019
Yang, L., Chu, J. S., and Fix, J. A. (2002). Colon-specific drug delivery: new approaches and in vitro/in vivo evaluation. Int. J. Pharm. 235 (1-2), 1–15. doi:10.1016/s0378-5173(02)00004-2
Keywords: natural hydrogel, chitosan, alginate, phenylalanine-phenylalanine dipeptide, phsensitive, drug delivery
Citation: Khatibi N, Naimi-Jamal MR, Balalaie S and Shokoohmand A (2024) Development and evaluation of a pH-sensitive, naturally crosslinked alginate-chitosan hydrogel for drug delivery applications. Front. Biomater. Sci. 3:1457540. doi: 10.3389/fbiom.2024.1457540
Received: 30 June 2024; Accepted: 23 October 2024;
Published: 01 November 2024.
Edited by:
Silvia Fare’, Polytechnic University of Milan, ItalyReviewed by:
Junjie Li, Kyushu University, JapanMatteo Pitton, Polytechnic University of Milan, Italy
Copyright © 2024 Khatibi, Naimi-Jamal, Balalaie and Shokoohmand. This is an open-access article distributed under the terms of the Creative Commons Attribution License (CC BY). The use, distribution or reproduction in other forums is permitted, provided the original author(s) and the copyright owner(s) are credited and that the original publication in this journal is cited, in accordance with accepted academic practice. No use, distribution or reproduction is permitted which does not comply with these terms.
*Correspondence: Ali Shokoohmand, YS5zaG9rb29obWFuZEB1cS5lZHUuYXU=; M. Reza Naimi-Jamal, bmFpbWlAaXVzdC5hYy5pcg==