- 1Department of Structural Eng., University of California San Diego, La Jolla, CA, United States
- 2Department of Civil Eng., National Taiwan University, Taipei, Taiwan
This paper focuses on the abilities of the Large High-Performance Outdoor Shake Table (LHPOST6) at UC San Diego to investigate the combined effects of realistic near-field translational and rotational earthquake ground motions applied as dynamic excitation to 3-D and large- or full-scale structural, geotechnical, or soil-foundation-structural systems. The LHPOST6 supports the advancement of innovative materials, manufacturing methods, detailing, earthquake protective systems, seismic retrofit methods, and construction methods, and is a driving force towards improving seismic design codes and standards and developing transformative seismic-resistant concepts. This paper provides: (i) a brief overview of the 6-DOF capabilities of the LHPOST6 facility; (ii) an overview of the research projects conducted so far at the LHPOST6 facility focusing on the performance of the facility, and (iii) new seismic research opportunities enabled by the LHPOST6 to provide data and fragility information on structural and geotechnical systems that can support the full realization of performance- and resilient-based seismic design.
Introduction
The six-degree-of-freedom (6-DOF) large high-performance outdoor shake table (LHPOST6) at UC San Diego is a shared-use facility enabling the next-generation of seismic testing of large structural, geotechnical, and soil-foundation-structural systems due to its ability to accurately reproduce far- and near-field ground motions. The LHPOST6 was originally conceived as a 6-DOF shake table but was built as single-degree-of freedom (1-DOF) shake table and operated from 2004 to 2019 to perform thirty-four projects as a national shared use equipment facility funded by the US National Science Foundation through the Network for Earthquake Engineering Simulation (NEES) and then the Natural Hazard Engineering Research Infrastructure (NHERI, 2025) program. The details of the 34 projects and the upgrade of LHPOST6 to its 6-DOF capabilities were summarized in detail by Van Den Einde et al. (2021). Schematics of the LHPOST6 before and after the upgrade are shown in Figure 1. The LHPOST6 is the largest shake table facility in the US and possesses the largest payload capacity globally, and its 6-DOF capabilities permit investigation of important aspects of the seismic response of 3-D systems to realistic multi-directional input.
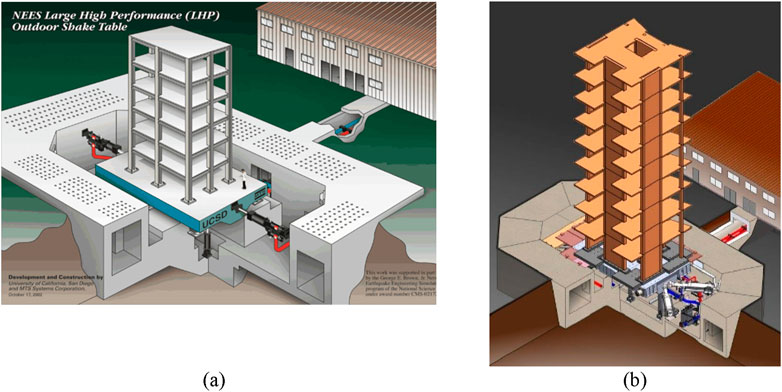
Figure 1. Schematics of LHPOST6: (a) During operation as a 1-DOF shake table (2004-2019): (b) after upgrade to a 6-DOF shake table (2022-present).
The NHERI@UC San Diego Experimental Facility, which hosts and operates the Large High-Performance Outdoor Shake Table (LHPOST6), is rooted in three critical needs for advancing the science, technology, and practice in earthquake disaster mitigation and prevention: (1) Fundamental knowledge for understanding the earthquake system-level behavior of buildings, critical infrastructure, utilities, and geo-structures, from the initiation of damage to the onset of collapse; (2) Experimental data to support the development, calibration, and validation of high-fidelity physics-based computational models that will progressively shift the reliance on physical testing to model-based simulation and address epistemic uncertainties in these models; and (3) Proof of concept testing for novel protective systems and innovative materials which can enhance community protection against earthquakes for either new or existing structures. Since completion of the upgrade in April 2022, four major projects have been performed on the facility which will be summarized in this paper. This paper will also discuss new opportunities to explore the effects of realistic ground motions on structural and geotechnical systems to advance seismic design practice and predictive capabilities for structural, geostructural, and nonstructural systems, leading to improved earthquake safety in the community overall. Indeed, the ability to test full-size structures has made it possible to physically validate the seismic performance of various systems that previously could only be studied at reduced-scale or with computer models.
Need for 6-DOF ground motion capability
The propagation of waves induced by earthquakes produces simultaneous horizontal, vertical and rotational movements of the ground surface. Therefore, the complete characterization of an earthquake ground motion at a given location consists of the three translational (ux, uy, uz) and three rotational (θx, θy, θz) degrees of freedom in a Cartesian coordinate system. Dynamic seismic response analyses of systems for either design or evaluation purposes commonly ignore the rotational components of earthquake ground motions. This has been a widely accepted practice in the earthquake engineering community due to: (1) the lack of recorded rotational ground motions during strong earthquakes and (2) a common assumption in the seismological community that rotational components are small enough to be neglected (Kalkan and Graizer, 2007). Nonetheless, Rosenblueth (1976) discussed the potentially damaging effects of these rotational components on tall buildings, which attracted research interest on the effect of the rotational ground motion components on the seismic behavior of structures (e.g., Ghafory-Ashtiany and Singh, 1986; Zembaty and Boffi, 1994; Trifunac, 2009a; Falamarz-Sheikhabadi, 2014; Falamarz-Sheikhabadi et al., 2016; Falamarz-Sheikhabadi et al., 2017). Moreover, structural failures and damage caused by past earthquakes have been linked to rotational and differential ground motions. For example, the torsional responses of tall buildings during the 1971 San Fernando earthquake were attributed to torsional ground motion (Hart et al., 1975), while rotational and differential ground motions may have caused the collapse of bridges during the 1971 San Fernando, 1978 Miyagi-ken-Oki (Bycroft, 1980), and 1994 Northridge (Trifunac et al., 1996) earthquakes. The high translational accelerations recorded during the February 2011 Christchurch earthquake suggest that rotational motions could have played a significant role in the widespread damage of the area and especially in the central business district consisting of a dense urban setting with closely spaced high-rise buildings (Guidotti, 2012). Numerical studies also indicate that rotational ground motion components can significantly affect structural response (e.g., Trifunac, 1982; Ghafory-Ashtiany and Singh, 1986; Zembaty and Boffi, 1994; Kalkan and Graizer, 2007; Trifunac, 2009a; 2009b; Jalali and Trifunac, 2009; Castellani et al., 2012; Basu et al., 2015; Falamarz-Sheikhabadi et al., 2017; Bońkowski et al., 2018; Guidotti et al., 2018; Meza Fajardo and Papageorgiou, 2018). It is hypothesized that the significance of this effect is dependent on the magnitude and frequency content of the rotational components as well as the dynamic characteristics of the structure (e.g., Figure 2).
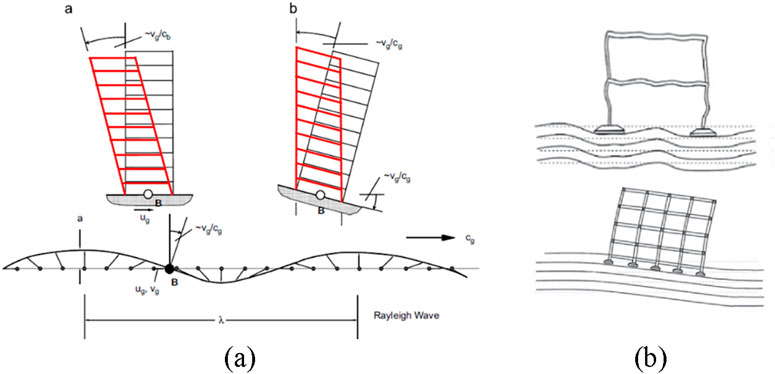
Figure 2. (a) Geometric interpretation of how horizontal translation and rocking may contribute to total drift in a simple building during the passage of a Rayleigh wave (from Trifunac, 2009b) and (b) response of a building under (top) high- and (bottom) low-frequency content rotational ground motions (from Castellani et al., 2012).
The LHPOST6 will enable the experimental investigation in full 3D and at large- or full-scale of the combined effects of realistic near-field translational and rotational ground motions applied as dynamic excitation to a structural, geotechnical, or soil-foundation-structural system, including the effects of kinematic and inertial soil-structure interaction (SSI), nonlinear soil and structural responses, and soil liquefaction. The data acquired from such landmark tests will enable calibration, validation, and improvement of (mechanics-based, physics-based) analytical and numerical models able to capture the seismic response of civil infrastructure systems, accounting for these realistic and important effects. The LHPOST6 will also be a key experimental facility to deploy, test, and validate sensors to measure rotational components of motion, which may open new frontiers for advanced system and damage identification of structures and structural health monitoring (Trifunac, 2009b).
The 6-DOF capabilities of the LHPOST6 provide new opportunities for studying these different geotechnical applications under realistic 3-D ground motions. As the stiffness and shear strength of soils depend on self-weight, vertical accelerations may lead to changes in soil properties during earthquake shaking, the effects of which are poorly understood in 2-D or 3-D site analyses (Hashash et al., 2010). At some sites, the vertical component of an earthquake motion may exceed the horizontal at short periods (e.g., Bozorgnia et al., 2000; Beresnev et al., 2002; Elgamal and He, 2004). Several element-scale studies have shown that deformations during 2-D horizontal shaking are underestimated when using methods based on unidirectional shaking (e.g., Ishihara and Nagase, 1988; Kammerer et al., 2002; Rutherford and Biscontin, 2013). Pyke et al. (1975) performed 1-g shake table tests on small samples of sand under 3-D motions and observed that while shaking in the vertical direction alone with acceleration amplitudes of less than 1 g did not affect the volumetric contraction of soils, combined vertical and horizontal shaking led to greater settlements than those from horizontal shaking alone.
Capabilities of the LHPOST6
The design of the LHPOST6 was informed using inverse simulation and was then validated using forward simulation. The MTS forward model of the LHPOST6 includes the rigid body dynamics in 6-DOFs of both the platen and a rigid specimen, servovalve and actuator dynamics (with nonlinear flow equations), accumulator banks and line accumulators, and a virtual replica of the MTS 469D controller that has been installed on the LHPOST6. In the forward model, the controller was tuned for the characteristics of the LHPOST6 design using the new 469D Auto-Tuner capability. The tuned closed-loop forward model provides the ability to perform “dry runs” of the LHPOST6 system and thus evaluate, pre-construction, its signal tracking performance capability. The forward model will also allow for offline tuning or pre-tuning based on the test specimen characteristics and will be very useful for safe offline operator training (i.e., shake table simulator). Figures 3a–c compare the target (or desired or reference) and achieved (under bare table condition) translational acceleration, velocity, and displacement time histories of the shake table, respectively, and Figure 3d compares the target and achieved five-percent damped tri-partite (displacement/pseudo-velocity/pseudo-acceleration) linear response spectra for the three translational components of the 1995 Kobe earthquake record. Similar levels of agreement between target and achieved table motions (i.e., signal tracking fidelity) were observed for the other strong tri-axial earthquake records considered for the upgrade design.
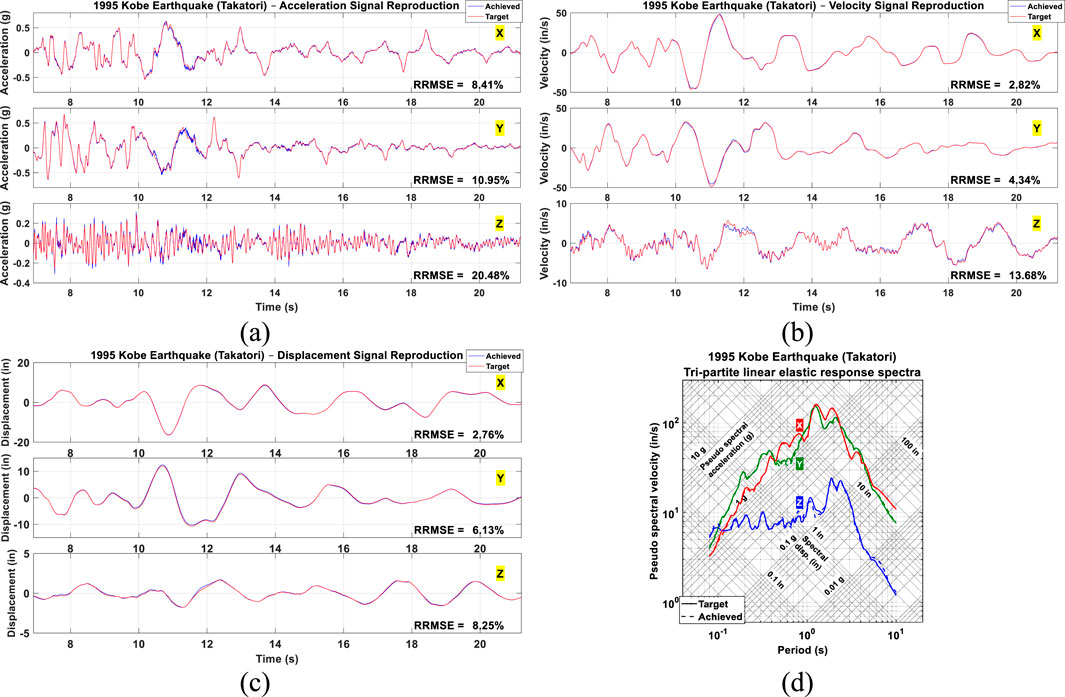
Figure 3. Comparison of target and achieved tri-axial 1995 Kobe earthquake record under bare table condition: (a) acceleration time histories, (b) velocity time-histories, (c) displacement time histories, and (d) 5% damped tri-partite spectra for linear response.
The comparisons in Figure 3 show a very good signal tracking capability of the LHPOST6. This is especially true for the vertical ground motion component, given the fact that the vertical actuators of the LHPOST6 are single-acting, i.e., these can only push (upwards) and cannot pull (downwards) the platen since they have zero retraction force. The nitrogen-filled hold-down struts pull the platen down but without closed-loop dynamic capabilities. The level of fidelity in signal reproduction for the vertical component and other motion components can also be further improved through the advanced control capabilities built in the MTS 469D controller, such as Adaptive Inverse Control (AIC), Online Iteration (OLI), and Specimen Dynamics Compensation (SDC) as well as the tools available in MTS STEX-Pro. A digital twin of the shake table and hydraulic system has been developed by Lai and Conte (2024), which can be used to simulate the interaction between a specimen on the table and the performance of the LHPOST6. A range of instrumentation including potentiometers, accelerometers, strain gages, load cells, earth pressure cells, and advanced kinemetric sensors are available for deployment on a given project.
Projects performed on the LHPOST6
Modular testbed building
The Modular Testbed Building, or MTB2, was the first specimen tested on the LHPOST6 after the upgrade. Experimental data from this program is available within the DesignSafe Data Depot (Hutchinson et al., 2024), including a detailed technical report (Morano et al., 2024a) and two journal papers (Morano et al., 2024b; 2025). The MTB2 is designed to be a reconfigurable and reusable community shared-use equipment resource. Reuse is accomplished by having a connection scheme that allows yielded members to be replaced, while leaving the rest of the structure in place. This modular design enables low-cost testing of components and subsystems at large to full-scale under simulated dynamic 3D loading. It enables seismic performance verification and acceptance testing of innovative designs, including seismic protective systems, lateral force resisting systems, stairs, nonstructural systems, among others. The MTB2 structure also provides for payload opportunities within the broader community.
The MTB2 reconfiguration allows for different structural configurations. The moment frame configuration consists of a three story (3.7 m/story), two bay (4.9 m/bay) by one bay (6.1 m) frame structure shown in Figure 4a. The moment frame configuration involves a moment frame with replaceable shear fuse plastic hinges in the longitudinal direction. A second configuration involves three levels of braced frames with buckling restrained braces (BRBs) placed at each story shown in Figure 4b. A third configuration is identical to the second except for the replacement of the BRBs at the first story with moment shear fuses to promote a softer first story. The MTB2 study also capitalized on the staging slab space at LHPOST6, using it as an area for pre-test assembly in one of the configurations to test fit and assembly efficiency. This exercise, while LHPOST6 was completing upgrade, allowed the specimen to be tested and characterized under a variety of low amplitude vibrations (Morano et al., 2024b), prior to its assembly on the shake table.
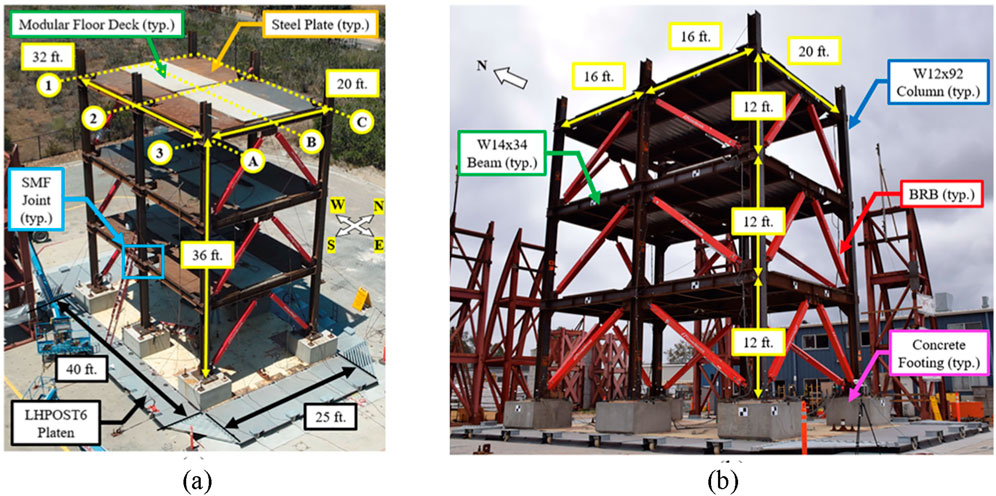
Figure 4. Photographs with annotated dimensions of the MTB2 structure (after Morano et al., 2025): (a) Moment frame configuration; (b) BRB configuration.
NHERI TallWood project
With global urbanization trends, the demands for tall residential and mixed-use buildings in the range of 8–20 stories are increasing. One new structural system in this height range is tall mass timber buildings. Granello et al. (2020) provided a review of research, testing and implementation of mass timber in buildings. The vision of the NSF-funded six-university collaborative research NHERI TallWood Project (Pei et al., 2024a) is to develop and validate a resilience-based seismic design methodology for tall wood buildings. During the second year of the NHERI TallWood project, an investigative testing program was completed at the NHERI@UC San Diego shake table facility on a full-scale two-story mass timber building with a resilient cross-laminated timber (CLT) rocking wall lateral system (Pei et al., 2017; 2019). The final capstone shake table test of a full-scale 10-story mass timber building equipped with nonstructural components was performed in 2023 as shown in Figure 5. These tests represented the tallest full-scale building structure ever tested on a shake table yielding unprecedented data which has been reported so far (Pei et al., 2024b) which will help push the boundary of resilient tall wood construction worldwide.
NHERI Converging Design project
The NHERI Converging Design project is an NSF-funded research effort led by Prof. Andre Barbosa from Oregon State University with collaborators from Colorado State University, Stanford University, and Penn State University. The vision of this project is to create a new design paradigm within structural engineering that employs multi-objective optimization to maximize functional recovery while integrating additional sustainable building design principles. This design methodology was validated through shake-table testing of a full-scale six-story mass timber structure at the NHERI@UCSD outdoor facility. This six-story specimen emerged from the previously tested ten-story shake-table specimen from the NHERI TallWood project where the top four stories were deconstructed to leave a six-story specimen to be reconfigured for the NHERI Converging Design project. The six-story structure was then subject to three distinct phases of shake-table testing that include different lateral force resisting systems with details summarized in Figure 6. The first phase of testing featured post-tensioned mass timber self-centering rocking wall lateral force-resisting systems consisting of mass timber wall panels, bounding columns, and U-shaped flexural plates (UFPs) (e.g., Chen et al., 2024) distributed over the height of the building as energy dissipators. The second phase of testing featured buckling-restrained braces (BRBs) as energy dissipators at the base of post-tensioned mass timber rocking walls to serve as the lateral force-resisting system. The third phase of testing featured yield link brace connections in exploration of hybrid steel mass timber structural system solutions. Data from this project has been archived in DesignSafe (Barbosa et al., 2025) and more information can be found at the NHERI Converging Design Project Website (2025).
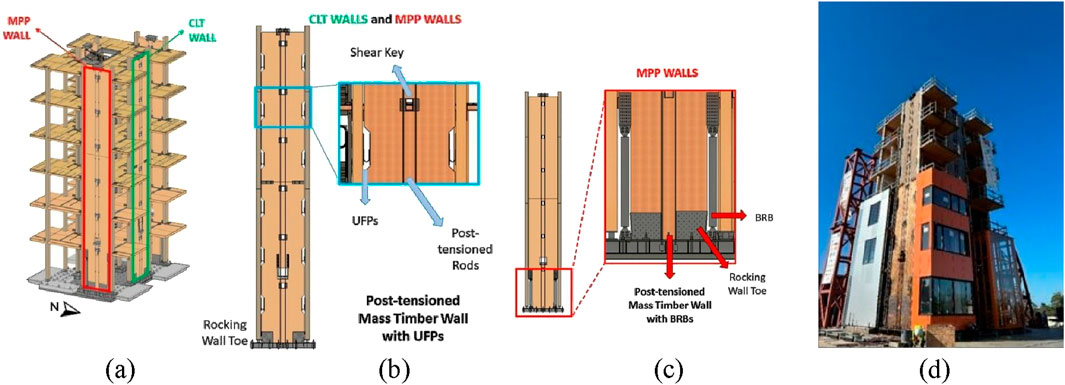
Figure 6. NHERI Converging Design 6-story specimen: (a) Three-dimensional rendering of the tested specimen with indication of location of different mass timber wall panels, including cross-laminated timber (CLT) and mass ply panels (MPP); (b) Main components of the self-centering rocking wall system tested in phase 1; (c) Schematics of different components used in the North-south direction in phase 2; (d) Picture of phase 1 tested specimen on the LHPOST6.
Seismic response of spent nuclear fuel casks
In the United States, Spent Nuclear Fuel (SNF) is currently stored onsite at 73 Nuclear Power Plants because a site for a geological repository for permanent SNF has not been identified and permitted. The SNF inventory stored on-site either in pools or dry storage was 84,500 MTU in 2020, with 46% being stored in dry storage with a rate of increase of 3,500 MTUs per year. As the SNF will be stored onsite for longer than expected, the dry storage facilities involving SNF in casks as shown in Figure 7a could experience earthquakes of a different magnitude than the one for which they were originally designed. However, there is little data on the response of SNF inside dry storage systems to seismic loads in the U.S., and the various gaps and nonlinearities between storage containers, canisters, baskets, aggregates, and fuel make it very difficult to evaluate by analytical methods. A 1468 kN generic vertical storage overpack (cask) shown in Figure 7b was tested on the LHPOST6 in summer 2024. It was equipped with a canister and a fuel basket with a thirty-two fuel assembly capacity. A mix of surrogate fuel assemblies and dummy assemblies filled all basket locations. An eight-inch-thick concrete slab was built on top of the shake table platen to act as the cask’s bearing surface. This slab had a surface finish mimicking the one found in real-world conditions. The cask, its components, and content was instrumented with a dense array of sensors consisting of accelerometers strain gauges, pressure indicating films, inclinometers, and displacement transducers. In addition, a series of high-speed, high-resolution cameras were used to monitor the local displacements of the fuel assemblies and the global displacement of the cask. The accelerometers and strain gages were fastened to fuel rods to monitor their dynamic response under generic ground motions representative of hard rock, soft rock, and soil sites in the U.S.
CFS-NHERI capstone test building
As a capstone to the multi-university-industry collaborative CFS-NHERI project, a full-scale 10-story cold-formed steel (CFS)-framed building is currently being constructed on the LHPOST6 and will be tested in Summer 2025 under increasing, multi-directional earthquake motion intensity along with subsequent live fire testing. Coined CFS10 (cfs10.ucsd.edu) the capstone effort follows successful prior system-level 2-story (CFS-NEES) and 6-story (CFS-HUD) structures previously tested on shake tables, the later program at LHPOST prior to its upgrade to 6-DOFs. The CFS10 project is being led by Tara Hutchinson from UC San Diego, Benjamin Schafer from Johns Hopkins University, and Richard Emberley from CalPoly University San Luis Obispo. In addition, collaborations with Thomas Gernay of Johns Hopkins University, Kara Peterman of the University of Massachusetts Amherst, Monica Kohler of Caltech, and Richard Allen of the University of California Berkeley enrich the program.
The CFS-NHERI capstone test building is designed with a height of 31.6 m, exceeding the height limitation of 19.8 m set by the current ASCE 7–22 design standard as shown in Figure 8 (see Singh et al., 2022; 2024; Zhang et al., 2024a; 2024b). The design also advances lateral force resisting system (LFRS) details to provide for the increases in seismic shear and overturning moment demands associated with increases in building height. Data generated will shed light on the impact of architectural exterior and interior finishes, non-designated systems, such as gravity walls or window/door framing. Higher mode effects, which can significantly influence the structural and nonstructural seismic response of tall buildings, will also be studied for the first time for repetitively framed structures. The experiments will provide vital full-scale system-level benchmark test data for a state-of-the-art CFS building under multidirectional seismic input. It will also advance knowledge of the post-earthquake fire performance of mid-rise CFS construction, by incorporating a live fire test sequence following the seismic test phase completion.
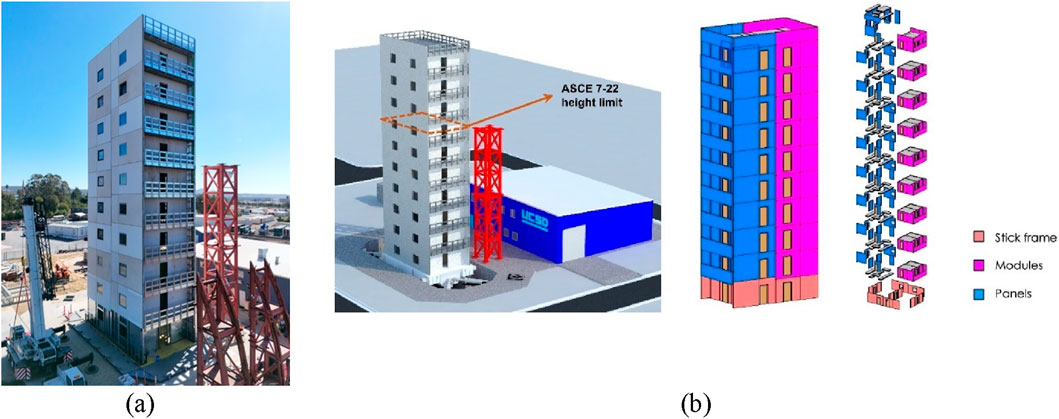
Figure 8. CFS-NHERI 10 story structure (at structural height completion, 21 Feb 2025): (a) Construction of the CFS-10 structure on the LHPOST6; (b) Schematics of the structure and construction methods (by J. Zhang and D. Rivera).
Research enabled by the LHPOST6
Importance of large-scale seismic testing of civil infrastructure
The seismic response of structures involves complex physics of heterogeneous materials with highly nonlinear constitutive properties and depends on the boundary/interface conditions, such as the interaction between the structure and the supporting/surrounding soil. There are many open issues regarding how to accurately model these phenomena at the different length and time scales over which the physical processes develop. There are significant knowledge gaps about the seismic response of structures that have been damaged or have partially collapsed and their possible failure modes as identified in the 2011 NRC report (National Research Council, 2011). State-of-the-art nonlinear structural analysis methods are still fairly limited in their ability to model the nonlinear dynamic response of structures, especially when approaching collapse (Deierlein, 2011). Failure is often triggered by localized strain concentrations resulting in stiffness and strength degradation that is sensitive to loading history. Examples of such behavior include local buckling and fracture in steel (both structural steel and reinforcing steel in concrete), shear failures in reinforced concrete (R/C) columns and walls, and connection or splice failures. While significant experimental research has been performed on individual structural components (e.g., beams, columns, slabs) and subassemblies (e.g., beam-column joints, beam-slab-column joints) at various scales (all the way to full-scale) in the US and other countries, the boundary conditions imposed on these test specimens may not be realistic as compared to their actual boundary conditions within structural systems. The scale of these physical models can also be an issue since some design details, construction materials, and damage and failure mechanisms cannot be accurately reproduced in reduced-scale models. These include the spacing of reinforcement in concrete structures, the size of aggregates in concrete, the quality and properties of welds, and the degree of plastic strain or damage localization, all which affect the ability of a structure to sustain inelastic deformations and the failure mechanisms. Hence, large- or full-scale structural system tests are essential to identifying and overcoming modeling deficiencies and validating these models and design details.
The mechanical behavior of most civil infrastructure systems under extreme earthquake loads is highly nonlinear and complex and varies significantly depending on the structural type and detailing, construction materials, and regional construction practice. Unlike aerospace structures, civil structures cannot be prototyped for mass production or designed to remain within the elastic limit during severe load events. To ensure that structures of different types, system properties and materials have a consistent level of safety and predictable performance in earthquake events, a performance-based design (PBD) approach was extensively developed in the mid-1990s. It is based on a structural reliability framework and enables engineers to design structures and facilities to meet specific performance objectives with quantifiable and acceptable risks of (i) exceeding various damage states, (ii) casualty, (iii) loss of occupancy (downtime), and (iv) economic losses in future earthquakes.
Although the PBD methodology has not been fully implemented in practice, simplified PBD methods have found their way into seismic design codes, such as AASHTO-COPRI 6 (2014), design guidelines like LATBSDC (2020) and assessment guidelines such as ASCE 41 (ASCE, 2017) for the evaluation and retrofit of existing buildings. Notably, the risk-targeted seismic hazard maps that form part of ASCE 7 (ASCE, 2022) rely not only on probabilistic seismic hazard data but also on structural fragility functions to achieve a uniform risk of structural collapse across the US. Furthermore, the recently developed and adopted FEMA 695 Methodology (FEMA, 2009) that quantifies structural performance factors for new structural systems to be designed with ASCE seven is also based on the notion of a uniform and acceptable risk of collapse. It is noteworthy that the development of reliable fragility functions, which are central to PBD, requires relevant experimental data and high-fidelity simulation models that can predict the nonlinear behavior of structural materials, components, and systems under different hazard scenarios. Experimental programs conducted at the LHPOST6 facility provide landmark experimental data at the subsystem and system levels.
It is well established that the extensive damage exhibited by code-compliant conventional buildings during strong earthquake ground motions, while avoiding collapse, has caused a push in earthquake-affected communities in the past 2 decades to use low-damage structural earthquake protective systems. Such systems can sustain significant nonlinear response, large lateral displacements, and damping with practically no damage and maintained operability throughout. Multi-axial large/full-scale shake table tests of building structures are needed to understand their response in 3-D, including the effects of rotational components of earthquake ground motions, and support the development of future design codes. Also, innovative seismic retrofit strategies that will be used and implemented for existing older non-ductile (wood, masonry, concrete, and steel) buildings should be validated through large-scale shake table system testing to better understand the force redistributions and the overall implications on the system ductility. Limited recent full-scale building shake table tests that incorporate non-structural components and systems, supported by field observations, demonstrate the importance of advancing our understanding and predictive capabilities of nonstructural components and structures (NCSs) in building systems when subjected to multi-directional seismic loading. Full-scale multi-axial shake table tests are needed to support the development of a reliable, unified design methodology for NCSs accounting for multi-directional earthquake excitation. Finally, there is a strong emphasis on the need to develop new sources of energy while preventing or reversing the degradation of the environment (i.e., renewable energy sources). Important examples of the supporting infrastructure are wind turbine farms (onshore and offshore), solar arrays, concrete dams, containment/reactor vessels of nuclear power plants and dry storage casks of spent nuclear fuel, which when built in seismic regions all require better understanding of their seismic response behavior and reliable performance-based assessment and design using experimentally validated high-fidelity computational models.
Understanding inherent damping
Inherent damping (also simply termed intrinsic or elastic damping) is introduced in time-history analyses of the inelastic seismic response of structures to account for damping before the onset of hysteretic response. The pioneering work of Carr (2005) established that the details of the inherent damping model may have significant effects on the calculated inelastic structural response. Several studies have studied inherent damping experimentally (e.g., Takayanagi and Schnobrich, 1979; Otani, 1980; Satake et al., 2003; Rodríguez et al., 2006; Petrini et al., 2008; Moaveni et al., 2010; Papagiannopoulos and Beskos, 2012; Astroza et al., 2016), but the optimal damping model has not been identified. It is also difficult to experimentally separate the inherent and hysteretic damping associated with the inelastic response or soil-structure interaction effects (e.g., Celebi, 1996).
Due to the lack of experimental data, a number of options involving Rayleigh, Caughey (Caughey, 1960; Caughey and O’Kelly, 1965), and modal (Wilson and Penzien, 1972) viscous damping matrices based on initial or tangent (degraded) stiffness properties have been proposed as models (Chrisp 1980, Shing and Mahin, 1987; Bernal, 1994; Carr, 1997; 2005; Hall, 1998; 2006; 2016a; 2017; Ryan and Polanco, 2008; Zareian and Medina, 2010; Jehel et al., 2014; Hardyniec and Charney, 2015; Chopra and McKenna, 2016a; 2016b). Warnings regarding the unintended consequences of these choices and possible remedies have been presented by several authors (Hall, 2006; Charney, 2008; Ryan and Polanco, 2008; Luco and Lanzi, 2019; Anajafi et al., 2020). In recent years, several studies have more closely investigated the problems associated with existing damping models and proposed solutions to overcome some of these problems (Carr et al., 2017; Luco and Lanzi, 2017; Lanzi and Luco, 2018; Chambreuil et al., 2022). The need to better identify the actual inherent damping mechanism(s) suggests the use of harmonic vibration tests (as opposed to seismic simulations) in the vicinity of resonance for excitations close to those leading to instability in the absence of inherent damping (Caughey, 1960; Luco, 2014). Shake table experiments with full 3-DOF or 6-DOF seismic base excitation on large- or full-scale building specimens with and without non-structural components and systems and large-scale bridge sub-structures (e.g., bridge bent) will guide the development and selection of optimum inherent damping models.
Dynamic response of geotechnical soil-foundation-structure systems
The LHPOST6 is ideally suited for experimental investigations of dynamic soil-structure and soil-foundation-structure interaction (Mylonakis and Gazetas, 2000; Gavras et al., 2020; Antonellis et al., 2015; Ebeido et al., 2019; Elsawy et al., 2019; Shahbazi et al., 2020a; Zayed et al., 2020; Zayed et al., 021). For example, the kinematic interaction between a foundation and soil (in the absence of the superstructure) under internal seismic wave excitation leads to translational and rotational components of the foundation input motion. This occurs for embedded foundations for all types of elastic wave excitation and for surface foundations subjected to non-vertically incident seismic waves and to spatially random ground motions. When a superstructure is present, the inertial interaction results in additional rocking components of motion of the foundation and additional torsional components, particularly, when the structure is not symmetrical. Thus, even when it can be assumed that the foundation is sufficiently rigid, the motion of the foundation will have at least 6 DOFs (e.g., (Roesset, 1981; Luco, 1981)). The 6-DOF capabilities of LHPOST6 will enable new types of full-scale dynamic SFSI studies needed to validate analytical or numerical simulations.
Along with the large laminar soil box and the large “rigid” soil box, the LHPOST6 is also ideally suited for experimental studies on the dynamic deformation response of geosynthetic reinforced soil (GRS) retaining walls (El-Emam and Bathurst, 2004; Ling et al., 2005; Ling et al., 2012; Sander et al., 2013; Latha and Santhanakumar 2015; Fox et al., 2015; Zheng et al., 2018a; Zheng et al., 2018b; Zheng et al., 2019a; Zheng et al., 2019b), cantilever retaining walls used in dam spillways (Kim and Elgamal, 2017a), shallow tunnels and buried water reservoirs (Kim et al., 2016; Hushmand et al., 2016a; 2016b; Durante et al., 2022), and slopes or embankments (e.g., Wartman et al., 2005; Nakamura et al., 2011; Sogabe et al., 2013; Yarahuaman and McCartney, 2022). The behavior of shallow buried structures (water reservoirs, tunnels, lifelines, utilities) has been well studied using centrifuge techniques (Hushmand et al., 2016a; 2016b) and large-scale soil-boxes (O'Rourke et al., 2008), but the soil containers on LHPOST6 can accommodate additional instrumentation and better capture system responses. The depth and flexibility of the tunnel structure and the soil compaction techniques were found to have a major effect on the seismic earth pressures in these experiments. Analytical techniques for seismic earth pressure estimation have advanced over time (Davis, 2003; Durante et al., 2022), which require detailed validation from full-scale experiments. The scale of the soil boxes permits dynamic experimental studies on the efficacy of conventional or bio-mediated soil improvement using actual construction techniques (Van Impe, 1989; DeJong et al., 2014) and experimental investigations of geotechnical issues that cannot be studied in laboratory-scale experiments like lateral spread in layered soils (Youd et al., 2002; Zayed et al., 2021), liquefaction of gravelly soils (Hubler et al., 2017), seismic or cyclic response of alternative backfill materials like tire derived aggregates with large particle sizes (Ahn and Cheng, 2014; McCartney et al., 2017) or expanded polystyrene foam (Trandafir and Bartlett, 2010; Athanasopoulos-Zekkos et al., 2012), seismic settlement of saturated soils (Tokimatsu and Seed, 1987; Bray and Olaya, 2022), and seismic compression of unsaturated soils (Stewart et al., 2004; Ghayoomi et al., 2011a; Ghayoomi et al., 2013a; Rong and McCartney, 2020; 2021).
Seismic testing of geostructures in the centrifuge permits construction of multiple specimens with different configurations to understand the impacts of different geometric variables or design features. Different from seismic testing of geostructures in the centrifuge, testing of geostructures in the large soil boxes on the LHPOST6 permits use of actual construction procedures for compacting soils, consideration of foundation installation effects, consideration of actual ground improvement techniques, and use of actual geosynthetic reinforcements. Accordingly, there are opportunities for collaboration with geotechnical centrifuge facilities to perform multiple simplified parametric-study type experiments in the centrifuge, then consider the effects of full-scale construction features like those permitted in the LHPOST6. Different from centrifuge testing on small-scale models, large-scale testing permits the incorporation of large instrumentation like earth pressure cells and settlement plates (e.g., Keykhosropour et al., 2018; Zheng et al., 2019a; 2019b; Yarahuaman and McCartney, 2022). Use of the large soil boxes available at the LHPOST6 may also help minimize near-field and boundary effects encountered when applying in-situ shear wave velocity tomography techniques that may be encountered in centrifuge-scale experiments (Ghayoomi and McCartney, 2011b). Centrifuge testing often uses transparent soil boxes (Ghayoomi et al., 2013b) or even transparent soils (Black, 2015) to visualize soil-structure interaction mechanisms. Accordingly, data obtained from centrifuge and 1-g shake table testing may be complementary.
In many cases, full-scale geostructures can be investigated on the LHPOST6 (e.g., Sander et al., 2013; Fox et al., 2015; Shahbazi et al., 2020a; 2020b). However, in other cases, it may not be possible to test a full-scale geotechnical structure due to the large size of the structure under investigation, such as cut-and-cover tunnels and dam spillways (Kim and Elgamal, 2017a; 2017b; Kim et al., 2016) or deep foundations (Ebeido et al., 2019; Zayed et al., 2020; Zayd et al., 2021). Numerical simulations are often used to extrapolate measurements from reduced-scale tests to large scale tests (e.g., Pereira and Koltuniuk, 2018; Li et al., 2020), but it is more reliable to use scaling relationships to avoid self-weight stress-dependent issues like arching that affect soil-structure interaction. Unlike centrifuge testing where a single scaling factor associated with the g-level can be used to reach geometric similitude, where stresses and strains are the same in a model and prototype, 1 g scale modeling requires a more nuanced approach. Scaling relationships like those developed by Iai (1989) permit extrapolation of measurements from reduced-scale tests to full-scale field conditions by establishing similitude between the model soil layer tested on the shake table and the prototype soil layer in the field. Iai (1989) built upon the work of Rocha (1957) to develop an approach to reach similitude between a reduced scale model and a prototype by considering a geometric scaling factor, a density scaling factor and a strain scaling factor. As the self-weight stresses are lower in a reduced scale 1 g model, the main effect of the scaling approach of Iai (1989) is to reduce the density (and thus stiffness) of the model soil layer so that it has a similar stress strain curve when scaled by the three scale factors. Zheng et al. (2019b) found that it is possible to use only a geometric scaling factor when using a strategically selected relative density that leads to a softer stress-strain response but not a major change in unit weight. Centrifuge modeling can be used to validate the scaling approach of Iai (1989) using the “modeling of models” technique (Ko, 1988). Specifically, models with different length scales (including a prototype at 1 g) can be tested under different g-levels to validate scaling relationships. When studying phenomena like liquefaction, post-liquefaction seismic settlements, seismic compression of unsaturated soils lateral spreading, or the efficacy of soil improvement techniques, scaling may not be necessary for simulating near-surface soil layers having a thickness less than the height of the laminar and rigid soil boxes.
The large soil boxes permit control of the boundary conditions necessary in modeling geostructures, which can have a major effect on the measured seismic response. The large “rigid” soil box permits control of plane strain boundary conditions representative of long geostructures (embankments, slopes, dams). It could also be used to assess soil-structure interaction behavior associated with reinforced concrete retaining wall systems (Castaldo and De Iuliis, 2014). While the rigid back wall boundary condition may not represent those for geostructures in the field, it is straightforward to consider in numerical simulations. The “rigid” soil box at UC San Diego is particularly suitable for tests with 2D shaking (vertical and horizontal shaking in one direction). The large laminar soil box is currently configured for uni-directional horizontal shaking that simulates a flexible shear soil column. While tests with shaking in the vertical and one horizontal direction can be accommodated with the laminar soil box in its current configuration, the laminar container will be upgraded using point-roller bearings for bi-directional horizontal shaking.
Three general types of experimental SSI studies are envisioned using the LHPOST6.
(1) Verification Studies under Tri-axial Excitation: Computational models of the complete soil-foundation-structure system can be used to obtain the total translational and rotational motion of the foundation which would then be applied at the base of the structure placed on the shake table. The resulting response of the structure will be compared with the predictions through numerical simulation to validate both theoretical models and computational methods. Independent of the validation justification, the response of structures to simultaneous translational and rotational base motions is of interest for research intended to represent the free-field ground motion as consisting of both translational and rotational components. This would extend the current design practice of including only translational components (in the absence of SSI) (e.g., Lee and Trifunac, 1985; 1987). Such tests will also open an avenue for blind prediction contests and discussions about the models used to augment knowledge in the community.
(2) Laminar and Rigid Soil Box Studies under Tri-axial Translational Excitation: In these studies, full-scale or scaled models of structures can be supported on soils placed in either the 1D laminar or rigid soil boxes available at the facility. The soil box can be subjected to tri-axial translational base motions to better simulate the seismic input excitation. Such tests could be used to study the nonlinear response of soils, the response of partially saturated soils, alternative backfill materials, and the nonlinear interaction between foundations, structures, and the soil (e.g., Shahbazi et al., 2020a). The contribution of radiation damping into the soil to the apparent damping in the structure (Cruz and Miranda, 2017) could also be studied using this approach. The effects of coupling through the soil on the seismic response of adjacent structures (i.e., structure-soil-structure interaction), a topic of importance in the urban environment and in farms of storage tanks and wind turbines, could also be investigated through this approach. Liquefaction, seismic-induced settlements and lateral soil spreading in urban areas have accounted for a large percentage of the damage to the built environment in cities stricken by a strong earthquake, such as in the 2010 and 2011 Christchurch earthquake swarm (Bray et al., 2017; Cubrinovski et al., 2014). Liquefaction effects on the seismic response of soil-foundation-structural systems can be studied with the LHPOST6 with one of the two large soil boxes available equipped with a flexible membrane liner to retain the water inside the soil box.
(3) Hybrid Tests: These will be ambitious tests in which the soil will be modeled in the computer and the superstructure on the shake table. The foundation input motion (i.e., the response of the foundation to seismic waves in the absence of the superstructure) and the response of the foundation to the total base inertial forces from the superstructure will be obtained numerically, in real-time. These tests will be used to study the nonlinear seismic response of structures in the presence of soil-structure interaction, including the torsional response of structures.
Advanced and/or innovative earthquake protective systems
Extensive damage in conventional buildings and bridges has caused a push in earthquake-affected communities in the past 2 decades to use low-damage structural earthquake protective systems. Such systems can sustain significant nonlinear response, large lateral displacements and damping with practically no damage and maintained operability throughout. This is a very active research area that includes base isolation, rocking foundations and systems, self-centering systems, inertial force-limiting floor anchorage systems, various types of dampers, buckling-restrained braces, and new materials (e.g., Housner, 1963; Zhang and Makris, 2001; Ozbulut et al., 2011; Clayton et al., 2012; Belleri et al., 2014; Kramer et al., 2016; Tian et al., 2016; Agalianos et al., 2017; Ganey et al., 2017; Moghadam and Konstantinidis, 2017; Silva, 2019).
Many structures have survived strong earthquakes unscathed, courtesy of rocking of the foundation (Housner, 1963). In competent soils not susceptible to liquefaction, rocking can be used as a mechanism to concentrate the nonlinear response and provide energy dissipation in some structures. This aspect has been widely demonstrated in centrifuge, field and 1-g shake table testing (e.g., Chang et al., 2007; Paolucci et al., 2008; Deng and Kutter, 2012; Gelagoti et al., 2012; Anastasopoulos et al., 2013; Liu et al., 2013; Pecker et al., 2014). Antonellis et al. (2015) carried out shake-table testing of two 1:3 scale bride piers with shallow foundations designed to rock. The test specimens were placed inside the large confinement soil box described by Fox et al. (2015), which was partially filled with poorly graded medium sand and water. Because of the uni-directional limitation of the LHPOST at the time, one of the test units was aligned with the direction of the shake table excitation, whereas the other was rotated 30°. While this provided multi-directional input to the specimens, the obvious correlation of the pair of translational input motions was present. This limitation no longer exists with the LHPOST6. The direction of shaking is also critical when studying geosynthetic-reinforced bridge abutments. Zheng et al. (2018a), Zheng et al. (2018b) and Zheng et al. (2019b) performed transverse and longitudinal shaking tests on GRS bridge abutments, respectively, and found a major difference in behavior. However, the geometries of the GRS bridge abutment specimens were different due to the limitations in the size of the UC San Diego Powell Laboratory shake table. Using the LHPOST6, the dynamic behavior of GRS bridge abutments in transverse and longitudinal directions can be evaluated using a single specimen.
To design structural systems for low-damage and reduced inertia forces, Zhang et al. (2018c) conducted shake table testing using the LHPOST on a half-scale four-story building equipped with different types of energy dissipation devices and rocking structural walls. The wall acting in the E-W direction was significantly offset from the floor center of mass to purposely create a strong coupled lateral-torsional response and subject the specimen to complex kinematics. Multi-directional input motion capability would have greatly simplified the specimen design in this research program and facilitated the acquisition of more complete information on the seismic response behavior of this innovative building archetype. Furthermore, these tests would have benefitted from the use of high-resolution piezometric strain gages recorded by the new DAQ system of the LHPOST6 to obtain principal strains in the footings of the bridge piers and rocking walls which experienced large impacts and remained within their quasi-linear range of behavior.
The LHPOST6 will make possible realistic seismic testing of prototype structures equipped with various types of earthquake protective systems at scales which are large enough so that the experimental findings are meaningful for full-scale implementation. For example, testing of a base-isolated structure on the LHPOST6 would account for the variation in axial load on isolation bearings due to multiple components of earthquake ground motion at the base of the structure. Benchmark shake table tests on full-scale rocking structures are needed because the response of such structures depends crucially on their size (e.g., Agalianos et al., 2017). The data from such tests is needed to verify and validate numerical models of rocking structures.
A wide range of promising and/or innovative passive and semi-active seismic response modification devices (SRMDs) must be tested in large- or full-scale structural systems for their ultimate validation and acceptance in real-world structural seismic design. Passive SRMDs include (Housner et al., 1997) metallic yield dampers, friction dampers, viscoelastic dampers (e.g., viscoelastic walls), viscous fluid dampers, tuned mass dampers, tuned liquid dampers, lead extrusion dampers (Parulekar et al., 2004; Soydan et al., 2012), carbon fiber reinforced isolators (Angeli et al., 2013), and shape memory alloys (DesRoches and Delemont, 2002; Ozbulut et al., 2011). A passive seismic response mitigation strategy based on extremely rapid nonlinear “scattering” of the seismic input energy from low-to high-frequency modes of a structure achieved through a system of strategically placed nonlinear vibration absorbers (termed nonlinear energy sinks) has been investigated numerically and experimentally (on a reduced-scale idealized structural model) shows promising results for practical implementation in civil infrastructure (Luo et al., 2014; Wierschem et al., 2017). Semi-active SRMDs include (Housner et al., 1997) variable-orifice fluid dampers, controllable friction devices, variable stiffness devices, semi-active impact dampers, adjustable tuned liquid dampers, controllable fluid dampers, magneto-rheological dampers (Cha et al., 2013; 2014), and electro-rheological dampers (Spencer et al., 1998).
The LHPOST6 facility will enable the performance validation of full-scale buildings protected with innovative low-cost seismic isolation technologies such as low-cost fiber-reinforced elastomeric isolators (Calabrese et al., 2019). It will also enable the evaluation of the earthquake performance of low-damage rocking-isolated structures, including bridge pier systems (Piras et al., 2022) and buildings with controlled-rocking lateral-force resisting frames subjected to multi-component earthquake base excitation (including rotational components) and validation of high-fidelity models of structural systems equipped with seismic isolation and other structural control devices (e.g., whole-building models). Base isolation for residential construction using geosynthetics or compressible fills like tire derived aggregates is another topic that has been studied with centrifuge modeling and small-scale shake table testing (Yegian and Kadakal, 2004; Tsang, 2008; Hernández et al., 2020; Yarahuaman and McCartney, 2024), but not full-scale testing. Mitigation of seismic pressures on retaining walls using alternative lightweight backfills like EPS or tire derived aggregates that have high damping are topics of interest that have not been explored in full-scale applications (Tsang, 2008; Athanasopoulos-Zekkos et al., 2012).
Retrofit systems/strategies for non-code compliant structures
To promote public welfare and safety by reducing the risk of injury and loss of life that may result from the effects of earthquakes on existing buildings, a number of cities on the west coast (e.g., Los Angeles, San Francisco) have adopted or are in the process of adopting mandatory seismic retrofit ordinances for seismically vulnerable existing buildings that have known deficiencies such as limited ductility, soft story susceptibility, and lack of proper hierarchy of strength in the members and connections. The seismic retrofit strategies that will be used and implemented must be validated through large-scale shake table testing, which serves as the final verification of acceptable performance. The LHPOST6 is well suited to support verification tests of new, innovative retrofit strategies being required by the design community.
Through ATC 78 (Holmes et al., 2017), researchers have been developing a methodology to address the vulnerability of older (pre-1980) nonductile concrete buildings, which represent a significant threat to life safety (Moehle, 2000). By evaluating the likelihood of system collapse, rather than component failures, the approach focuses on assessing existing buildings to find the critical structures without being overly conservative. Other retrofit and repair techniques to improve the seismic performance for soft-story buildings using prestressed concrete jacketing and masonry block techniques have also been tested on shake tables (Bracci et al., 1995). However, there is still a need for research to support the practical and effective application of seismic retrofitting to existing buildings both in the physical implementation of retrofit techniques and methodologies for the numerical evaluation of the performance of retrofitted systems. Other examples of retrofit and repair techniques include: (1) the rehabilitation of reinforced concrete construction using fiber reinforced polymer composites (e.g., Silva and Kanitkar, 2018; Wu and Pantelides, 2019), (2) the use of shape memory alloy on the seismic performance of concrete bridges (Johnson et al., 2008), (3) the use of rocking steel braced frames for the retrofit of seismically deficient steel buildings (Tremblay et al., 2016; Mottier et al., 2018), and (4) the use of various seismic response modification devices such as base isolation, seismic dampers, etc.
Building structures
One of the largest and most diverse areas of research is in low-rise, mid-rise, or high-rise buildings made from a variety of materials such as structural steel, cold-formed steel, reinforced/prestressed/precast concrete, high-performance concrete, wood-frame, cross-laminated (heavy) timber, unreinforced and reinforced masonry, and advanced materials such as Ultra-High Performance Concrete and non-metallic reinforcements. Research topics also include the seismic performance of total building systems, those designed with super columns or outriggers, and special issues such as floor vibration isolation.
Unreinforced Masonry (URM) buildings have suffered severe damage or collapse in past earthquakes. The failures of URM buildings in seismic events have often been characterized by the out-of-plane collapse of the walls (Felice and Giannini, 2001). In addition to other factors, such as the wall aspect ratio and the material properties, the resistance of a URM wall to in-plane seismic forces depends heavily on the gravity load carried by the wall. The gravity load can enhance the shear strength of the bed joints and provide resistance to the overturning moment imposed on the wall. In addition, the resistance of an URM wall to out-of-plane forces relies on arching action, which could be weakened by damage caused by in-plane forces. As a result, bi-axial horizontal ground motions are particularly damaging to an URM building and URM walls subjected to uniaxial in-plane forces tend to exhibit significantly better performance compared with bi-axial loading conditions. Furthermore, the vertical ground acceleration could change the axial load on a wall and thus its in-plane and out-of-plane shear resistance, again affecting the arching mechanism and stability of the wall. The LHPOST6 will enable robust assessment of the seismic safety of URM buildings, development of effective retrofit methods, and improvement of design provisions.
Current seismic design standards for reinforced concrete and masonry wall systems, such as ACI 318-25 (ACI, 2025) and TMS-402-16 (TMS, 2016) are largely based on data obtained from quasi-static testing of structural components, most of which were conducted with in-plane horizontal loading. While such data are crucial for the development of design and detailing requirements to ensure the ductile behavior of structural members, building performance in an earthquake is also highly dependent on how these components are proportioned, connected, and interact with each other as a system. Without due consideration of the system’s behavior in design, the actual seismic response and load-resisting mechanism of a building could differ significantly from what is anticipated by design standards. The uniaxial LHPOST has enabled large-scale structural system tests that have provided the much-needed data to understand the behavior of structural systems as a whole and validate analytical models that can be used to support the development of improved design standards. An important example is the tests on two reinforced masonry wall systems, one system designed according to current codes and the other with a displacement-based method (Mavros et al., 2016; Stavridis et al., 2016). Owing to the strong coupling between the walls and the slabs, both structures exhibited shear-dominated wall behavior, although the code-conforming structure had been designed to avoid this behavior. Despite this, both structures performed satisfactorily under the maximum considered earthquake (MCE). In both cases, the wall systems exhibited a much higher resistance and ductility than what had been observed from shear-dominated walls tested individually under quasi-static loading. This study underscored the importance of considering system behavior in design and providing data to develop and calibrate refined computational models.
The performance of shear-wall systems under bi-axial horizontal seismic actions is not well understood. A planar wall with a rectangular cross-section is designed to carry in-plane seismic forces. However, under multi-axial ground motions, its vertical load-carrying capacity can be significantly jeopardized when it is subjected to a large out-of-plane drift (Tomassetti et al., 2016). Flanged walls are normally designed to resist seismic forces in both horizontal directions, but the bi-axial behavior of flanged walls is not as well studied as that of reinforced concrete columns. The bi-axial behavior of a flanged wall is complicated as in-plane shear cracking or toe crushing in a wall flange could affect the flexural resistance of the wall in the other loading direction. The performance of reinforced masonry archetype buildings under biaxial ground motions have been numerically studied with refined 3D nonlinear computational models that were calibrated with experimental data from uni-directional wall component and system tests (FEMA, 2020; Koutras and Shing, 2021). The numerical results have been used to calibrate simplified simulation models to assess the collapse probability of the building archetypes using the FEMA P695 procedure (FEMA, 2009). Figure 9 also shows the numerical results obtained with the nonlinear computational model of a two-story building. It can be observed that the performance of a building with flanged reinforced masonry walls under bi-axial horizontal ground motions can be significantly worse than that under a uniaxial ground motion. Multi-axial shake table tests are needed to validate such computational models and acquire a better understanding of the behavior of shear walls under earthquake actions. This information is invaluable for developing improved code provisions and assessment methods to enhance the safety and cost-efficiency of reinforced concrete and masonry buildings.
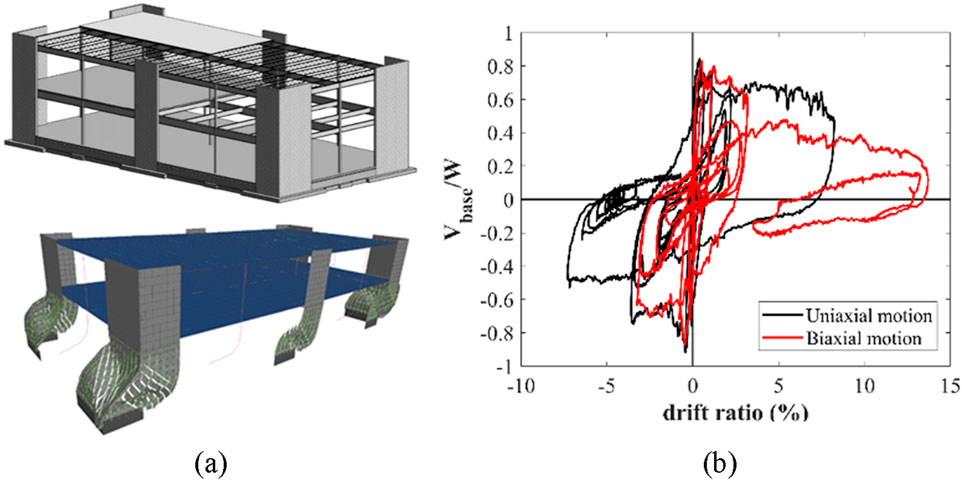
Figure 9. (a) FE model assessment of collapse potential of a reinforced masonry building under bi-axial ground motions; (b) Response of a two-story reinforced masonry building with flanged walls to uniaxial and biaxial motions (Koutras and Shing, 2021).
Structural concrete and precast systems have been a prevailing construction material for low, high-rise, and super-tall buildings. However, most research supporting seismic design with structural concrete has been limited to components (e.g., Kurama et al., 1999; Lehman et al., 2004; Naish et al., 2013; Tazarv and Saiid Saiidi, 2016) or reduced-scale models of building systems (e.g., Shahrooz and Moehle, 1987; Rodríguez et al., 1995). In the US, only three landmark building tests were performed at large- or full-scale on a shake table (Schoettler et al., 2009; Belleri et al., 2014; Chen et al., 2016; Zhang et al., 2018c) but under single-axis excitation. Therefore, research is needed on innovative, resilient, seismic-resistant concrete systems under multi-axial seismic base excitation, specifically to validate earthquake protective systems under more realistic conditions and improve modeling and analysis capabilities for component and system behavior. Of particular interest are the use of high strength (or ultra-high performance) materials (reinforcing bars and concrete) and advanced materials (e.g., fiber-reinforced concrete wrapping and non-metal reinforcement) for seismic civil applications, special concrete moment frames, and structural walls, including the combination of dual systems, precast concrete frame, and wall structures, and sustainable reinforced concrete structures utilizing recycled materials. The current building code in the US allows seismic applications of Grade 100 reinforcement only in Special Structural Walls. Insufficient test data was the leading cause for not allowing high-strength reinforcement in Special Moment Frames. Shake-table tests of Special Moment Frames and their interaction with Special Structural Walls (with and without coupling beams) are needed to support their introduction in the building code and to improve methods of analysis, linear and nonlinear, for the design office. There are also important research needs to better understand: (1) the influence of dynamic shear behavior on flexural deformation capacity in RC structural systems, (2) complex dynamic system interactions in the context of realistic multi-component earthquake base excitations to improve our current ability to model system behavior (Panagiotou et al., 2011), and (3) influence of diaphragm connections within the lateral load resisting system, especially if frames are considered.
Of great interest and somewhat neglected in research is the evaluation of the seismic performance of commercial tilt-up buildings. Many such buildings behaved poorly during the 1994 Northridge earthquake (Mitchell et al., 1995), which prompted the need to revisit various diaphragm-to-wall connection methods. Recent research indicates that some of these structures may still be vulnerable to earthquakes (Koliou et al., 2016; Henry and Ingham, 2011). To date, no shake table testing has been conducted on a partial or complete tilt-up structural system. The LHPOST6 will benefit the above research areas by providing the opportunity to conduct large-scale multi-axial shake table tests of complete buildings and structures of complex geometries.
Since the 1994 M6.7 Northridge earthquake, the precast concrete industry has been heavily involved in the research and development of new seismic systems and in developing design methods for building diaphragms (Priestley et al., 1999; Fleischman et al., 2013; Kurama et al., 2018). The speed and quality of construction, durability, and the lower carbon footprint of this industry, compared with the concrete or steel industries, make precast concrete an attractive structural solution for some building archetypes in the industry. Buckling-restrained braces (BRBs), which originated in Japan as joint venture between the steel and precast concrete industry (Wakabayashi et al., 1973), are one of the most widely used bracing methods in the US for structural steel buildings. Precast frames incorporating reinforced concrete BRBs have significant advantages as these BRBs are precast at the plant and require no special site connections (Oh et al., 2021). Moreover, the precast BRBs can be repaired and replaced at the site should these elements be severely damaged during an earthquake. Research using the LHPOST6 on building systems with precast braced frames has the potential to impact the precast concrete industry.
There has been research on the seismic performance of hot-rolled structural steel and cold-formed steel systems in the areas of structural stability and progressive collapse mitigation, connection behavior, seismic risk, and life-cycle cost quantification (Stojadinović et al., 2000; Khandelwal et al., 2008). However, research is needed to assess interactions in building systems undergoing earthquakes to improve seismic design codes for steel building like AISC 341-16 (AISC, 2016). For example, by competing inelasticity in vertical and horizontal lateral-force resisting systems, overstrength, and system effects derived from the participation of gravity and non-structural framing in lateral response (e.g., Imanpour et al., 2016; Peterman et al., 2016; Cravero et al., 2020). An important area of research that will benefit significantly from the LHPOST6 is the development of innovative low damage seismic resistant steel structures, modeling, and analysis of floor diaphragms, chords, and seismic collectors (Agarwal et al., 2018). The ability to subject a large-scale test specimen of a building or a key portion of a building to a multi-component excitation will be particularly beneficial in the study of the above topics, given their complex and extended geometries and distributed boundary conditions and sensitivity to out-of-plane and vertical excitation. Other research needs are in progressive collapse mitigation, the seismic stability of multi-tiered braced frames under bi-directional shaking, and dynamic collapse evaluation for low-ductility braced frame systems. A critical component of successful large-scale shake table testing is the precise and reliable measurement, synchronization, and storage of numerous sensor channels at a sufficiently high sampling rate. Additionally, efficient data access and organization are essential for real-time visualization and rapid evaluation during a sequence of tests. This aspect helps make decisions on proceeding or pausing the test sequence. The LHPOST6 enables this key aspect of large-scale shake table testing.
An innovative technique for enhancing the seismic performance of steel brace frame and moment frame buildings involves strategically placing seismic fuses in steel frame structures by locally changing the mechanical properties (lowering strength while increasing ductility and toughness) of steel through local exposure to high temperatures followed by slow cooling. The technique lowers seismic force demands on critical elements (promoting economic use of materials), enhances ductility and energy dissipation, and mitigates brittle failures such as connection fractures (Morrison et al., 2015) (Figure 10). With its unique capabilities, the LHPOST6 will provide validation of the benefits this approach provides and will provide knowledge on construction and design methods for applying this technique in new buildings and for retrofit of seismically venerable steel structures.
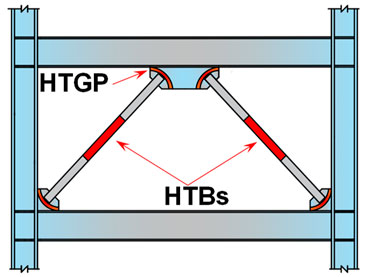
Figure 10. Schematic showing local heat-treated regions of brace (HTBs) and gusset plate (HTGPs) intended to enhance seismic performance.
Nonstructural components and systems (NCSs)
NCSs are generally categorized as elements (e.g., architectural, mechanical, electrical, plumbing, building contents) that facilitate the operation of a building. Importantly, they typically comprise 75%–85% of the construction cost of commercial buildings (Taghavi and Miranda, 2003; FEMA, 2012). NCSs have suffered significant damage, led to appreciable losses, and endangered occupants during past earthquakes (e.g., Taghavi and Miranda, 2003; FEMA, 2012; Ayres et al., 1973; Steinbrugge and Schader, 1973; Filiatrault et al., 2001; Meneses, 2010; Dhakal et al., 2016). Laudable efforts have been undertaken to develop simplified design procedures to account for the range of practical NCSs configurations (e.g., Asfura and Der Kiureghian, 1986; Burdisso and Singh, 1987a; 1987b; Villaverde, 1997; Bernal, 1999; Pozzi and Der Kiureghian, 2015). The wide range of types, varying mass distribution, and multiple connection locations in a structure have hampered the advancement of a reliable, unified design strategy. Thus, the shortcomings/limitations of modern codes are well-known (e.g., (Filiatrault and Sullivan, 2014; Lim et al., 2017)). The scarceness of full-scale building shake table tests that incorporate NCSs limits our understanding of the seismic response of these NCSs. For example, the landmark NSF-funded Building Nonstructural Components and Systems (BNCS) test program (Pantoli et al., 2016a) incorporated a complete suite of NCSs, including operable egress (stairs and elevators), facades (precast concrete and light-weight cold-formed steel), and interior equipment and architectural support contents (ceiling, HVAC, piping, etc.). This project focused on the “total building” and, in particular, the interactions between components (nonstructural-to-nonstructural and structural-to-nonstructural) and offered new insight into understanding the seismic response of a wide range of NCSs, but the tests were carried out under single-axis ground motions. This test program would have immensely benefited from the capability of the LHPOST6 (Hoehler et al., 2009; Chen et al., 2016; Pantoli et al., 2016a; 2016b). NCSs are by their nature secondary systems; their response depends upon the response of the supporting primary system, in most cases a building. The varying vibratory response of a building under multi-directional input motion will then naturally affect the input motion to the NCSs. Certain systems have been well documented to be particularly susceptible to certain components of ground motion. For example, due to their light weight and hung configurations, the presence of the vertical ground motion component has been shown to greatly affect the response of ceiling subsystems (e.g., Soroushian et al., 2012; Ryan et al., 2016; Wang et al., 2016). Similarly, systems with well delineated weak axes and abrupt bends (often a design feature of a NCS to accommodate necessary layout changes) are vulnerable to failure along these weaker axes (e.g., Ryan et al., 2016). Important to enveloping a building, the wide range of architectural facades have a high degree of variability in their connectivity to the supporting structure, and thus their response to multi-directional input requires understanding (Pantoli et al., 2016c). Limited recent tests (e.g., Pei et al., 2024a), supported by field observations, demonstrate the importance of advancing our understanding and predictive capabilities under multi-directional seismic loading of NCSs in building systems. Full-scale multi-axial shake table tests are needed to advance the development of a reliable, unified design methodology for NCSs accounting for multi-directional earthquake excitation. In this case, full-scale is required due to the difficulty/impossibility to obtain NSCs at a reduced-scale.
Energy structures
There has been a strong emphasis on the need to develop new sources of energy while preventing or reversing the degradation of the environment (i.e., renewable energy sources). Engineering solutions for wind and solar power, nuclear fusion, electrical and hydrological energy are necessary, not only to find cost effective technical solutions for harvesting the energy, but also for designing the infrastructure to support it (National Research Council, 2011).
Research on wind turbine structures has mostly focused on the structural analysis, design and/or assessment of wind turbines primarily against traditional environmental loads rather than extreme natural hazards such as earthquakes, hurricanes and tsunamis. With numerous wind farms being built in seismic regions such as China, the United States, India, Southern Europe and East Asia, more research on the seismic performance of wind structures is needed (Fitzwater and Cornell, 2002; Saranyasoontorn and Manuel, 2006; Burton et al., 2011; Guanche et al., 2013; Katsanos et al., 2016). For this reason, research utilizing the LHPOST6 will provide critical data to improve analysis tools and the seismic design of wind turbines on the dynamic response of these tall and slender structural systems. SSI effects may be considered in scaled systems or using hybrid testing. Areas of research include investigating the performance of newer, larger wind turbines and the effects of multi-directional near-field earthquake base excitations (including rotational components) to develop advanced methodologies to perform multi-hazard risk assessment of wind turbines (Katsanos et al., 2016). A series of full-scale tests of a 22 m high wind turbine with rated power of 65 kW were conducted on the LHPOST (Prowell et al., 2009). The experimentally estimated natural frequencies and mode shapes closely matched those derived from the finite element model developed and calibrated for this specific wind turbine (Prowell et al., 2009).
The performance of Electric Power Supply Systems (EPSSs) is also critical to the seismic resilience of a society (Franchin and Cavalieri, 2015; Sun et al., 2015). The EPPS includes components such as electrical substations, large power transformers, transmission poles/lines. Sun et al. (2015) developed a framework for the probabilistic assessment of the seismic resilience of an EPSS and the community it serves. Research such as this requires accurate data obtained from seismic tests on the LHPOST6 investigating system and component performance. Other researchers (Bosworth et al., 2017) are investigating using computational dynamic analysis methods for the time histories of electromagnetic reaction forces during short-circuit faults in High-Voltage substation structures.
Solar energy is a safe, clean, renewable energy resource which can replace current fossil fuels for generation of electricity. Many companies have developed solar power systems with structural frames. Testing of solar arrays subjected to wind and seismic forces is necessary to improve product development of solar array support structures and improve industry guidelines for appropriate structural design procedures and requirements for solar energy systems, considering gravity, wind, and seismic effects (Maffei et al., 2014).
The LHPOST6 will also provide a unique facility for the testing of nuclear structures, systems, and components (SSCs). Experimental seismic tests of nuclear SSCs have been performed for decades but often facing limitations on payload and/or multi-directional seismic input. These limitations result in the need for scaled models, or disregarding the vertical earthquake ground motion component, which are significant for rigid-short-period systems such as those in nuclear facilities. Furthermore, the seismic design and qualification of advanced reactors rely heavily on verified and validated numerical models that can capture the interaction between the reactor vessel, the contained fluid and the internal components (Yu et al., 2021). However, very few numerical models have been validated with experimental data. The LHPOST6 will provide critically needed experimental data for the seismic evaluation of next-generation nuclear SSCs and small modular reactors (SMRs), as well as systems and components used for nuclear waste storage and transportation; these data will assist in validating numerical models of these components and systems (Eidelpes et al., 2020; Zargar et al., 2017).
Another energy area requiring seismic testing and validation is hydroelectric dams. Research includes the selection of reasonable safety evaluation earthquakes for design, and the evaluation of structural adequacy of dams and foundations under earthquake loading (Léger, 2007; Hariri-Ardebili and Saouma, 2015). Needed areas of research are in SSI, design and analysis of foundations and abutments, material testing, and strengthening to assure foundation and abutment integrity. Furthermore, research is needed in the development of cost-effective geometry and structural detailing with minimum geometric irregularities and gradual variations in structural stiffness, and the validation of state-of-the-art numerical models of dams based on data obtained from large-scale dynamic testing. The LHPOST6 could be used to conduct scaled-up versions of the shake table experiments conducted on small scale models of concrete gravity dams at Polytechnique Montreal (Tinawi et al., 2000). Fluid-dam interaction could be accommodated on the LHPOST6 using the large soil box as a reservoir.
Bridges
The 1971 San Fernando and 1989 Loma Prieta Earthquakes were a turning point in the seismic design practice of bridges not only for California but for all seismic-prone regions in the United States. The Caltrans seismic retrofit program made large gains in designing retrofit strategies for existing bridge components with known vulnerabilities as well as developing new design strategies (Chai et al., 1991; Xiao et al., 1996; Haroun and Elsanadedy, 2005; Seible et al., 1997). In the 1990s, the feasibility of using advanced composite materials or fiber-reinforced polymers (FRPs) was investigated not only for the rehabilitation of existing structures in the form of seismic retrofit, service load strengthening, and damage repair measures but for new structural systems (Van Den Einde et al., 2003). The use of smart materials such as nitinol shape memory alloy devices for retrofitting bridges continues to be a hot topic (Johnson et al., 2008).
A great challenge in the seismic design of columns that are part of highway interchange systems which may involve complex geometries including curved bridge decks, skewness, etc., is to properly evaluate its response under the combined effects of vertical and bi-directional horizontal excitations. The capabilities of the LHPOST6 will open a new paradigm shift in properly evaluating the seismic response of slender columns and many other complex structures and validating the high-fidelity modeling of nonlinearly responding bridges (Babazadeh et al., 2016), including soil-structure-interaction and liquefaction effects in the case of soils vulnerable to liquefaction (Zhang et al., 2008; Elgamal et al., 2008).
Precast segmental construction methods can ease bridge construction costs by reducing construction time while maintaining quality control. Recent advances in new accelerated bridge construction (ABC) methodologies use precast methods for new bridges or replacing and rehabilitating existing bridges. ABC reduces traffic effects that are often impacted by onsite construction-related activities. While some applications of ABC construction exist in the U.S., regions with moderate to high seismicity require in-depth development, detailing, experimental investigation, and guidelines for suitable connections between the precast members (Mashal and Palermo, 2017; 2019). Shake table testing of prestressed and segmental bridge components and systems have been conducted (Vosooghi and Buckle, 2013; Saiidi and Kavianipour, 2018).
A variety of other bridge-related topics that will benefit from the LHPOST6 are: (1) 3D behavior of precast segmental bridge superstructures for accelerated bridge construction (Nikoukalam and Sideris, 2016), (2) bridges with hybrid sliding-rocking columns (Torres Matos and Rodríguez, 2014; Madhusudhanan and Sideris, 2018), (3) analytical models to predict the static and dynamic nonlinear response of such bridges (Li et al., 2017; Xie et al., 2019), (4) the use of smart materials in bridges (Johnson et al., 2008), (5) high-performance steel highway bridge systems, a field that is steadily growing and offers high strength, excellent fracture toughness, good weldability, and resistance to corrosion, which makes it well-suited for highway bridge applications (Abbas et al., 2015; Ma et al., 2019), and (6) multi-directional dynamic experimental evaluation of bridge superstructure-abutment-substructure interactions for configurations commonly employed in the Central United States.
Structural health monitoring
Condition assessment of structures plays a key role in supporting the decision-making process following natural or artificial hazard or aging events. These events, such as earthquakes, can potentially induce critical damage to civil structures, and subsequent decision-making related to emergency response, inspection, evacuation, and retrofit of structures is of vital importance. Damage initiation and progression cannot always be detected through visual screening and, therefore, time-consuming, costly, and invasive post-event inspection and evaluation methods are required to detect certain types of damage. Potential impacts of earthquakes as well as other natural and man-made hazards on communities can be reduced through accurate and timely risk mitigation decisions after catastrophic events, which can be supported and facilitated using structural health monitoring (SHM), diagnosis, and prognosis methods to help assess the damage in, and residual strength of, civil structures. Several approaches for SHM of civil structures, and in particular for system identification (SID) and damage identification (DID), have been proposed and studied in the literature for post-earthquake assessment of structural safety. A number of model-free and linear/nonlinear model-based approaches have been proposed in the literature for system and damage identification of civil structures (Catbas and Kijewski-Correa, 2013). Finite element (FE) model updating has emerged as a powerful methodology for structural health monitoring and damage identification of civil structures (Friswell and Mottershead, 1995). Recent years have seen significant developments in the area of nonlinear FE model updating of civil structures by using advanced Bayesian (probabilistic) estimation methods to update a high-fidelity mechanics-based nonlinear FE model of the structure of interest, which can then be interrogated to detect, localize, classify, and assess the damage in the structure at different scales (global and local) (Song et al., 2013; Astroza et al., 2017; Astroza et al., 2019; Roohi et al., 2019).
The high-quality datasets collected from future landmark experiments performed on the LHPOST6 will be invaluable for evaluating vibration-based condition/damage assessment methodologies and resolving the remaining obstacles preventing reliable real-world implementation of such methodologies. Typically, each large- or full-scale specimen tested on the LHPOST6 is subjected to a series of earthquake ground motions of increasing intensity until the brink of collapse. The SHM field will benefit from such high-quality datasets and associated metadata. An algorithm of the wave method for structural health monitoring (SHM) was tested and calibrated using shake table test data of a full-scale, seven-story, reinforced-concrete building slice tested on the LHPOST (Panagiotou et al., 2011). The method is based on monitoring changes in the velocity of waves propagating vertically through the structure, identified by least-squares fit of beam models (Ebrahimian et al., 2017). Data measured on test structures can be used to develop automated system identification and post-earthquake assessment methodologies for instrumented structures subject to complex ground motion effects as structures in the real-world do not have sufficient instrumentation to fully characterize the 6-DOF ground motion effects.
Additive manufacturing (3D printing)
In several other engineering fields, automation has been steadily replacing traditional production methods, dramatically increasing speed, quality of construction, and innovation of design while concurrently reducing cost and waste in a transformative way. Rapid prototyping of 3-dimensional parts (i.e., 3D printing) with cementitious or metallic materials allows geometrically intricate but efficient designs which are today unfeasible to construct using traditional methods (Ma et al., 2018). Recently, this technology has proven its utility at large-scale supporting the construction of elements such as connection nodes in space frame metal structures or even entire buildings and bridges. This research area is still emerging, with most efforts focused on developing the methodologies for rapid and cost-effective 3D prototyping of concrete and metal buildings and bridges at larger scales (Buswell et al., 2007; Le et al., 2012; Lim et al., 2012; Gosselin et al., 2016; Zareiyan and Khoshnevis, 2017; Camacho et al., 2018). However, the mechanical properties of 3D printed materials and the performance of 3D printed structures have not been studied in detail under seismic loading. The use of the LHPOST6 becomes crucial to support the development of large-scale additive manufacturing technologies capable of efficiently producing multifunctional structural elements with enhanced performance (Keating et al., 2017) in both low and high seismicity areas. This development includes the need for standardized testing and quality control, investigating ways to print using multiple materials, and combining additive manufacturing with other processes which rely upon traditional materials or construction techniques.
Liquid storage tanks
Liquid storage tanks (LSTs) are critical structural system elements in the industry. These tanks are used in chemical processes, water, fuel, oil and gas storage and for fermentation of alcoholic beverages, among many uses. Poor seismic performance of LSTs was observed in recent earthquakes in Chile, New Zealand, Italy, and the 2014 South Napa earthquakes (Zareian et al., 2012; Fischer, 2014; Fischer et al., 2016; Brunesi et al., 2015; Yazdanian et al., 2020; Frezzati et al., 2023) and accounted for losses of the order of $7B. The LHPOTS6 is a unique facility where much needed research can be conducted to evaluate, develop high-fidelity models that include the fluid-structure and soil-fluid-structure interaction, and improve the seismic response of storage tanks, with smaller tanks tested at full-scale and large tanks tested at reduced scales, for example, in the range of 1:10 to 1:20.
Other structures
A multitude of topics that do not fit directly into the research areas described above or perhaps span across multiple areas are classified here as “other structures”. These include wharves, ports and A-cranes (Roeder et al., 2005; Lemnitzer et al., 2010; Smith-Pardo and Ospina, 2013), water intake towers, airport control towers, deep foundations, and unique military applications such as testing the tailhook gear that fighter jets use to land on aircraft carriers, large-aircraft landing gear, embedded dynamometers and real-time hybrid testing of military hardware. The LPHOST6 will support research on the seismic behavior and design of waterfront structures under multi-directional loading which include earthen, landfill, and wharf structures including material ageing and corrosion (Andisheh et al., 2016). Research on the LHPOST6 used in combination with a large soil box can complement centrifuge experiments to investigate the seismic performance of levees on different soil conditions (Cappa et al., 2014) to validate computational models. Sideris et al. (2008) conducted shake table tests of steel pallet type tall storage rack structures equipped with advanced base isolation and rocking seismic protection systems. Shake table tests must be performed on much larger rack structures subjected to 3D ground motions which can only be accommodated at the LHPOST6 facility. The tests will require extensive measurements, including horizontal and vertical displacement and acceleration responses at multiple locations, strain measurements at critical locations in beam, column, and bracing members, and relative rotations at the beam to column joints.
Conclusions
The LHPOST6 supports the advancement of innovative materials, manufacturing methods, detailing, earthquake protective systems, seismic retrofit methods, and construction methods, and is a driver towards improving the design codes and standards and developing transformative seismic-resistant concepts. This paper provides an overview of the role of shake table testing with six-degree-of-freedom input motions using the LHPOST6 to provide data and fragility information on structural and geotechnical systems, supporting the advancement of performance-based and resilient-based seismic design.
Author contributions
JM: Writing – original draft. LV: Writing – review and editing. MM: Writing – review and editing. AP: Writing – review and editing. KL: Writing – review and editing. C-TL: Writing – review and editing. JC: Writing – review and editing.
Funding
The author(s) declare that financial support was received for the research and/or publication of this article. The authors are grateful for funding from the US National Science Foundation from award 2227407.
Acknowledgments
The authors are grateful for the support provided by the National Science Foundation (NSF), with Joy Pauschke as Program Director, and by the University of California at San Diego.
Conflict of interest
The authors declare that the research was conducted in the absence of any commercial or financial relationships that could be construed as a potential conflict of interest.
Generative AI statement
The author(s) declare that no Generative AI was used in the creation of this manuscript.
Publisher’s note
All claims expressed in this article are solely those of the authors and do not necessarily represent those of their affiliated organizations, or those of the publisher, the editors and the reviewers. Any product that may be evaluated in this article, or claim that may be made by its manufacturer, is not guaranteed or endorsed by the publisher.
References
Abbas, H. H., Kim, B. G., and Sause, R. (2015). “Unconventional high performance steel bridge girder systems,” in Advances in bridge maintenance, safety management, and life-cycle performance, set of book and CD-ROM (London: CRC Press), 813–814.
ACI (2025). “Building code requirements for structural concrete and commentary,”, 318-25. Farmington Hills, MI: ACI.
Agalianos, A., Psychari, A., Vassiliou, M. F., Stojadinovic, B., and Anastasopoulos, I. (2017). Comparative assessment of two rocking isolation techniques for a motorway overpass bridge. Front. Built Environ. 3, 47. doi:10.3389/fbuil.2017.00047
Agarwal, A., Lizarraga, D., Beedle, M., Li, C. H., Fleischman, R., Sause, R., et al. (2018). “Investigation of seismic performance of collectors in steel building structures,” in 11th national conference on earthquake engineering 2018: integrating science, engineering, and policy, NCEE 2018, 2002-2011 (Oakland, CA: Earthquake Engineering Research Institute).
Ahn, I. S., and Cheng, L. (2014). Tire derived aggregate for retaining wall backfill under earthquake loading. Constr. Build. Mater. 57, 105–116. doi:10.1016/j.conbuildmat.2014.01.091
AISC (2016). Seismic provisions for structural steel buildings (AISC 341-16) and commentary. Chicago, IL: American Institute of Steel Construction.
Anajafi, H., Medina, R. A., and Santini-Bell, E. (2020). Effects of the improper modeling of viscous damping on the first-mode and higher-mode dominated responses of base-isolated buildings. Earthq. Eng. and Struct. Dyn. 49 (1), 51–73. doi:10.1002/eqe.3223
Anastasopoulos, I., Loli, M., Georgarakos, T., and Drosos, V. (2013). Shaking table testing of rocking—isolated bridge pier on sand. J. Earthq. Eng. 17 (1), 1–32. doi:10.1080/13632469.2012.705225
Andisheh, K., Scott, A., and Palermo, A. (2016). Seismic behavior of corroded RC bridges: review and research gaps. Int. J. Corros. 2016, 1–22. doi:10.1155/2016/3075184
Angeli, P., Russo, G., and Paschini, A. (2013). Carbon fiber-reinforced rectangular isolators with compressible elastomer: analytical solution for compression and bending. Int. J. Solids Struct. 50 (22-23), 3519–3527. doi:10.1016/j.ijsolstr.2013.06.016
Antonellis, G., Gavras, A. G., Panagiotou, M., Kutter, B. L., Guerrini, G., Sander, A. C., et al. (2015). Shake table test of large-scale bridge columns supported on rocking shallow foundations. J. Geotechnical Geoenvironmental Eng. 141 (5), 04015009. doi:10.1061/(asce)gt.1943-5606.0001284
ASCE (2022). Minimum design loads and associated criteria for buildings and other structures (ASCE 7-22). Am. Soc. Civ. Eng.
Asfura, A., and Der Kiureghian, A. (1986). Floor response spectrum method for seismic analysis of multiply supported secondary systems. Earthq. Eng. and Struct. Dyn. 14 (2), 245–265. doi:10.1002/eqe.4290140206
Astroza, R., Alessandri, A., and Conte, J. P. (2019). A dual adaptive filtering approach for nonlinear finite element model updating accounting for modeling uncertainty. Mech. Syst. Signal Process. 115, 782–800. doi:10.1016/j.ymssp.2018.06.014
Astroza, R., Ebrahimian, H., Conte, J. P., Restrepo, J. I., and Hutchinson, T. C. (2016). System identification of a full-scale five-story reinforced concrete building tested on the NEES-UCSD shake table. Struct. Control Health Monit. 23 (3), 535–559. doi:10.1002/stc.1778
Astroza, R., Ebrahimian, H., Li, Y., and Conte, J. P. (2017). Bayesian nonlinear structural FE model and seismic input identification for damage assessment of civil structures. Mech. Syst. Signal Process. 93, 661–687. doi:10.1016/j.ymssp.2017.01.040
Athanasopoulos-Zekkos, A., Lamote, K., and Athanasopoulos, G. A. (2012). Use of EPS geofoam compressible inclusions for reducing the earthquake effects on yielding earth retaining structures. Soil Dyn. Earthq. Eng. 41, 59–71. doi:10.1016/j.soildyn.2012.05.004
Ayres, J. M., Sun, T. Y., and Brown, F. R. (1973). “Nonstructural damage to buildings,” in The great Alaska earthquake of 1964. Engineering division of earth sciences (Washington, DC: National Research Council), 346–456.
Babazadeh, A., Burgueño, R., and Silva, P. F. (2016). Evaluation of the critical plastic region length in slender reinforced concrete bridge columns. Eng. Struct. 125, 280–293. doi:10.1016/j.engstruct.2016.07.021
Barbosa, A., Simpson, B., van de Lindt, J., Sinha, A., Field, T., McBain, M., et al. (2025). “Shake table testing program for mass timber and hybrid resilient structures datasets for the NHERI Converging Design project,” in Shake table testing program of 6-story mass timber and hybrid resilient structures (NHERI Converging Design Project) (Austin, TX: DesignSafe-CI). doi:10.17603/ds2-rh8q-rn95
Basu, D., Whittaker, A. S., and Constantinou, M. C. (2015). Characterizing rotational components of earthquake ground motion using a surface distribution method and response of sample structures. Eng. Struct. 99, 685–707. doi:10.1016/j.engstruct.2015.05.029
Belleri, A., Schoettler, M. J., Restrepo, J. I., and Fleischman, R. B. (2014). Dynamic behavior of rocking and hybrid cantilever walls in precast concrete building. ACI Struc. J. 111 (3). doi:10.14359/51686778
Beresnev, I. A., Nightengale, A. M., and Silva, W. J. (2002). Properties of vertical ground motions. Bull. Seismol. Soc. Am. 92 (8), 3152–3164. doi:10.1785/0120020009
Bernal, D. (1994). Viscous damping in inelastic structural response. J. Struct. Eng. 120 (4), 1240–1254. doi:10.1061/(asce)0733-9445(1994)120:4(1240)
Bernal, D. (1999). A dynamic stiffness formulation for the analysis of secondary systems. Earthq. Eng. and Struct. Dyn. 28 (11), 1295–1308. doi:10.1002/(sici)1096-9845(199911)28:11<1295::aid-eqe867>3.0.co;2-2
Black, J. A. (2015). Centrifuge modelling with transparent soil and laser aided imaging. Geotechnical Test. J. 38 (5), 20140231–20140644. doi:10.1520/gtj20140231
Bońkowski, P. A., Zembaty, Z., and Minch, M. Y. (2018). Time history response analysis of a slender tower under translational-rocking seismic excitations. Eng. Struct. 155, 387–393. doi:10.1016/j.engstruct.2017.11.042
Bosworth, M., Steurer, M., Graber, L., Hodgson, I., Sause, R., Tahmasebi, E., et al. (2017). Modeling of reaction forces in high-voltage substation structures. IEEE Trans. Power Deliv. 33 (4), 1510–1517. doi:10.1109/tpwrd.2017.2754191
Bozorgnia, Y., Campbell, K. W., and Niazi, M. (2000). Observed spectral characteristics of vertical ground motion recorded during worldwide earthquakes from 1957 to 1995. Proc. 12th World Conf. Earthq. Eng. 2671 (4).
Bracci, J. M., Reinhorn, A. M., and Mander, J. B. (1995). Seismic retrofit of reinforced concrete buildings designed for gravity loads: performance of structural model. Struct. J. 92 (6), 711–723. doi:10.14359/9665
Bray, J. D., Markham, C. S., and Cubrinovski, M. (2017). Liquefaction assessments at shallow foundation building sites in the Central Business District of Christchurch, New Zealand. Soil Dyn. Earthq. Eng. 92, 153–164. doi:10.1016/j.soildyn.2016.09.049
Bray, J. D., and Olaya, F. R. (2022). “Examination of the volumetric strain potential of liquefied soil with a database of laboratory tests,” in Proc. Geo-congress 2022. Reston, VA: ASCE, 495–505.
Brunesi, E., Nascimbene, R., Pagani, M., and Beilic, D. (2015). Seismic performance of storage steel tanks during the May 2012 Emilia, Italy, earthquakes. J. Perform. Constr. Facil. 29 (5), 04014137. doi:10.1061/(asce)cf.1943-5509.0000628
Burdisso, R. A., and Singh, M. P. (1987a). Multiply supported secondary systems part I: response spectrum analysis. Earthq. Eng. and Struct. Dyn. 15 (1), 53–72. doi:10.1002/eqe.4290150105
Burdisso, R. A., and Singh, M. P. (1987b). Seismic analysis of multiply supported secondary systems with dynamic interaction effects. Earthq. Eng. and Struct. Dyn. 15 (8), 1005–1022. doi:10.1002/eqe.4290150807
Burton, T., Jenkins, N., Sharpe, D., and Bossanyi, E. (2011). “Design loads for horizontal axis wind turbines,” in Wind energy handbook. 2nd edn. Chichester, UK: John Wiley and Sons, Ltd.
Buswell, R. A., Soar, R. C., Gibb, A. G., and Thorpe, A. (2007). Freeform construction: mega-scale rapid manufacturing for construction. Automation Constr. 16 (2), 224–231. doi:10.1016/j.autcon.2006.05.002
Bycroft, G. N. (1980). Soil—foundation interaction and differential ground motions. Earthq. Eng. and Struct. Dyn. 8 (5), 397–404. doi:10.1002/eqe.4290080503
Calabrese, A., Losanno, D., Spizzuoco, M., Strano, S., and Terzo, M. (2019). Recycled Rubber Fiber Reinforced Bearings (RR-FRBs) as base isolators for residential buildings in developing countries: the demonstration building of Pasir Badak, Indonesia. Eng. Struct. 192, 126–144. doi:10.1016/j.engstruct.2019.04.076
Camacho, D. D., Clayton, P., O'Brien, W. J., Seepersad, C., Juenger, M., Ferron, R., et al. (2018). Applications of additive manufacturing in the construction industry–A forward-looking review. Automation Constr. 89, 110–119. doi:10.1016/j.autcon.2017.12.031
Cappa, R., Yniesta, S., Lemnitzer, A., Brandenberg, S. J., and Stewart, J. P. (2014). “Centrifuge experiments to evaluate the seismic performance of levees on peaty soils in the Sacramento-San Joaquin Delta,” in Paper presented at the dam safety conference. San Diego, CA.
Carr, A. J. (1997). Damping Models for inelastic analyses. Proc. Asia-Pacific Vib. Conf. 1, 42–48. doi:10.1007/978-3-031-50631-4_33
Carr, A. J. (2005). “Damping models for time-history structural analyses,” in Proceedings of Asia pacific vibration conference (Langkawi, Malaysia), 287–293.
Carr, A. J., Puthanpurayil, A. M., Lavan, O., and Dhakal, R. P. (2017). “Damping models for inelastic time history analysis: a proposed modelling approach,” in Proc. 16th world conference in earthquake engineering. Santiago, 1488.
Castaldo, P., and De Iuliis, M. (2014). Effects of deep excavation on seismic vulnerability of existing reinforced concrete framed structures. Soil Dyn. Earthq. Eng. 64, 102–112. doi:10.1016/j.soildyn.2014.05.005
Castellani, A., Stupazzini, M., and Guidotti, R. (2012). Free-field rotations during earthquakes: relevance on buildings. Earthq. Eng. and Struct. Dyn. 41 (5), 875–891. doi:10.1002/eqe.1163
Catbas, F. N., and Kijewski-Correa, T. (2013). Structural identification of constructed systems: collective effort toward an integrated approach that reduces barriers to adoption. J. Struct. Eng. 139 (10), 1648–1652. doi:10.1061/(asce)st.1943-541x.0000682
Caughey, T. K. (1960). Classical normal modes in damped linear dynamic systems. ASME J. Appl. Mech. 27, 269–271. doi:10.1115/1.3643949
Caughey, T. K., and O’Kelly, M. E. (1965). Classical normal modes in damped linear dynamic systems. ASME J. Appl. Mech. 32 (3), 583–588. doi:10.1115/1.3643949
Celebi, M. (1996). Comparison of damping in buildings under low-amplitude and strong motions. J. Wind Eng. Industrial Aerodynamics 59 (2-3), 309–323. doi:10.1016/0167-6105(96)00014-1
Cha, Y. J., Agrawal, A. K., Friedman, A., Phillips, B., Ahn, R., Dong, B., et al. (2014). Performance validations of semiactive controllers on large-scale moment-resisting frame equipped with 200-kN MR damper using real-time hybrid simulations. J. Struct. Eng. 140 (10), 04014066. doi:10.1061/(asce)st.1943-541x.0000982
Cha, Y. J., Zhang, J., Agrawal, A. K., Dong, B., Friedman, A., Dyke, S. J., et al. (2013). Comparative studies of semiactive control strategies for MR dampers: pure simulation and real-time hybrid tests. J. Struct. Eng. 139 (7), 1237–1248. doi:10.1061/(asce)st.1943-541x.0000639
Chai, Y. H., Priestley, M. N., and Seible, F. (1991). Seismic retrofit of circular bridge columns for enhanced flexural performance. Struct. J. 88 (5), 572–584. doi:10.14359/2759
Chambreuil, C., Giry, C., Ragueneau, F., and Léger, P. (2022). Seismic energy dissipation in reinforced concrete beam: investigating damping formulations. Eur. J. Environ. Civ. Eng. 26 (15), 7771–7797. doi:10.1080/19648189.2021.2009380
Chang, B. J., Raychowdhury, P., Hutchinson, T. C., Thomas, J., Gajan, S., and Kutter, B. L. (2007). Evaluation of the seismic performance of combined frame-wall-foundation structural systems through centrifuge testing. Proc. 4th Int. Conf. Earthq. Geotech. Eng.
Charney, F. A. (2008). Unintended consequences of modeling damping in structures. J. Struct. Eng. 134 (4), 581–592. doi:10.1061/(asce)0733-9445(2008)134:4(581)
Chen, M. C., Pantoli, E., Wang, X., Astroza, R., Ebrahimian, H., Hutchinson, T. C., et al. (2016). Full-scale structural and nonstructural building system performance during earthquakes: Part I–specimen description, test protocol, and structural response. Earthq. Spectra 32 (2), 737–770. doi:10.1193/012414eqs016m
Chen, Y., Palermo, A., and Mashal, M. (2024). Finite-element validation of U-shaped flexural plates integrated into a novel brace dissipator. J. Struct. Eng. 150 (9). doi:10.1061/jsendh.steng-13167
Chopra, A. K., and McKenna, F. (2016a). Modeling viscous damping in nonlinear response history analysis of buildings for earthquake excitation. Earthq. Eng. and Struct. Dyn. 45 (2), 193–211. doi:10.1002/eqe.2622
Chopra, A. K., and McKenna, F. (2016b). Response to John Hall's Discussion (EQE-16-0008) to Chopra and McKenna's paper, ‘Modeling viscous damping in nonlinear response history analysis of buildings for earthquake excitation. Earthq. Eng. and Struct. Dyn. 45 (13), 2235–2238. doi:10.1002/eqe.2762
Clayton, P. M., Winkley, T. B., Berman, J. W., and Lowes, L. N. (2012). Experimental investigation of self-centering steel plate shear walls. J. Struc. Eng 138 (7), 952–960. doi:10.1061/(asce)st.1943-541x.0000531
Cravero, J., Elkady, A., and Lignos, D. G. (2020). Experimental evaluation and numerical modeling of wide-flange steel columns subjected to constant and variable axial load coupled with lateral drift demands. J. Struct. Eng. 146 (3), 04019222. doi:10.1061/(asce)st.1943-541x.0002499
Cruz, C., and Miranda, E. (2017). Evaluation of soil-structure interaction effects on the damping ratios of buildings subjected to earthquakes. Soil Dyn. Earthq. Eng. 100, 183–195. doi:10.1016/j.soildyn.2017.05.034
Cubrinovski, M., Taylor, M., Henderson, D., Winkley, A., Haskell, J., Bradley, B., et al. (2014). “Key factors in the liquefaction-induced damage to buildings and infrastructure in Christchurch: preliminary findings,” in NZSEE conference.
Davis, C. A. (2003). “Lateral seismic pressures for design of rigid underground lifeline structures,” in Proc., 6th U.S. Conf. On lifeline earthquake engineering. Reston, VA: ASCE, 1001–1010.
Deierlein, G. G. (2011). “Earthquake engineering research needs in the planning, design, construction, and operation of buildings,” in Grand challenges in earthquake engineering research. Washington, DC: The National Academies Press.
DeJong, J. T., Soga, K., Kavazanjian, E., Burns, S., Van Paassen, L. A., Al Qabany, A., et al. (2014). Biogeochemical processes and geotechnical applications: progress, opportunities and challenges. Géotechnique 63 (4), 287–301. doi:10.1680/geot.sip13.p.017
Deng, L., and Kutter, B. L. (2012). Characterization of rocking shallow foundations using centrifuge model tests. Earthq. Eng. and Struct. Dyn. 41 (5), 1043–1060. doi:10.1002/eqe.1181
DesRoches, R., and Delemont, M. (2002). Seismic retrofit of simply supported bridges using shape memory alloys. Eng. Struct. 24 (3), 325–332. doi:10.1016/s0141-0296(01)00098-0
Dhakal, R. P., Pourali, A., Tasligedik, A. S., Yeow, T., Baird, A., MacRae, G., et al. (2016). Seismic performance of non-structural components and contents in buildings: an overview of NZ research. Earthq. Eng. Eng. Vib. 15 (1), 1–17. doi:10.1007/s11803-016-0301-9
Durante, M. G., Stewart, J. P., Brandenberg, S. J., and Mylonakis, G. (2022). Simplified solution for seismic earth pressures exerted on flexible walls. Earthq. Spectra, 87552930221083326. doi:10.1177/87552930221083326
Ebeido, A., Elgamal, A., Tokimatsu, K., and Abe, A. (2019). Pile and pile-group response to liquefaction-induced lateral spreading in four large-scale shake-table experiments. J. Geotechnical Geoenvironmental Eng. 145 (10), 04019080. doi:10.1061/(asce)gt.1943-5606.0002142
Ebrahimian, M., Todorovska, M. I., and Falborski, T. (2017). Wave method for structural health monitoring: testing using full-scale shake table experiment data. J. Struct. Eng. 143 (4), 04016217. doi:10.1061/(asce)st.1943-541x.0001712
Eidelpes, E., Ibarra, L. F., and Medina, R. A. (2020). A probabilistic assessment of PWR SNF cladding pinching failure considering potential transportation accident conditions. Nucl. Eng. Des. 358, 110423. doi:10.1016/j.nucengdes.2019.110423
El-Emam, M. M., and Bathurst, R. J. (2004). Experimental design, instrumentation and interpretation of reinforced soil wall response using a shaking table. Int. J. Phys. Model. Geotechnics 4 (4), 13–32. doi:10.1680/ijpmg.2004.040402
Elgamal, A., and He, L. (2004). Vertical earthquake ground motion records: an overview. J. Earthq. Eng. 8 (05), 663–697. doi:10.1080/13632460409350505
Elgamal, A., Yan, L., Yang, Z., and Conte, J. P. (2008). Three-dimensional seismic response of Humboldt Bay bridge-foundation-ground system. J. Struc. Eng. 134 (7), 1165–1176. doi:10.1061/(asce)0733-9445(2008)134:7(1165)
Elsawy, M. K., El Naggar, M. H., Cerato, A., and Elgamal, A. (2019). Seismic performance of helical piles in dry sand from large-scale shaking table tests. Géotechnique 69, 1071–1085. doi:10.1680/jgeot.18.p.001
Falamarz-Sheikhabadi, M. R. (2014). Simplified relations for the application of rotational components to seismic design codes. Eng. Struct. 59, 141–152. doi:10.1016/j.engstruct.2013.10.035
Falamarz-Sheikhabadi, M. R., Zerva, A., and Ghafory-Ashtiany, M. (2016). Mean absolute input energy for in-plane vibrations of multiple-support structures subjected to non-stationary horizontal and rocking components. Probabilistic Eng. Mech. 45, 87–101. doi:10.1016/j.probengmech.2016.03.001
Falamarz-Sheikhabadi, M. R., Zerva, A., and Ghafory-Ashtiany, M. (2017). Revised seismic intensity parameters for middle-field horizontal and rocking strong ground motions. J. Struct. Eng. 143 (1), 04016155. doi:10.1061/(asce)st.1943-541x.0001646
Felice, G. D., and Giannini, R. (2001). Out-of-plane seismic resistance of masonry walls. J. Earthq. Eng. 5 (02), 253–271. doi:10.1080/13632460109350394
FEMA (2009). Quantification of building seismic performance factors (FEMA P695). Washington, DC: Applied Technology Council for the Federal Emergency Management Agency.
FEMA (2012). Reducing the risks of nonstructural earthquake damage: a practical guide. Fourth Edition. Washington, DC: FEMA E-74), prepared by the Applied Technology Council for the Federal Emergency Management Agency.
FEMA (2020). Short-period building collapse performance and recommendations for improving seismic design. FEMA P-2139-3 3.
Filiatrault, A., and Sullivan, T. (2014). Performance-based seismic design of nonstructural building components: the next frontier of earthquake engineering. Earthq. Eng. Eng. Vib. 13 (1), 17–46. doi:10.1007/s11803-014-0238-9
Filiatrault, A., Uang, C. M., Folz, B., Christopoulos, C., and Gatto, K. (2001). Reconnaissance report of the february 28, 2001 nisqually (Seattle-Olympia) earthquake. San Diego, La Jolla, CA: University of California.
Fischer, E. (2014). Learning from earthquakes: 2014 Napa valley earthquake reconnaissance report. West Lafayette, IN: Lyle School of Civil Engineering, Purdue University e-Pubs. Available online at: https://docs.lib.purdue.edu/cgi/viewcontent.cgi?article=1000&context=civlgradreports.
Fischer, E. C., Liu, J., and Varma, A. H. (2016). Investigation of cylindrical steel tank damage at wineries during earthquakes: lessons learned and mitigation opportunities. Pract. Periodical Struct. Des. Constr. 21 (3), 04016004. doi:10.1061/(asce)sc.1943-5576.0000283
Fitzwater, L. M., and Cornell, C. A. (2002). Predicting the long term distribution of extreme loads from limited duration data: comparing full integration and approximate methods. J. Sol. Energy Eng. 124 (4), 378–386. doi:10.1115/1.1509768
Fleischman, R. B., Restrepo, J. I., Naito, C. J., Sause, R., Zhang, D., and Schoettler, M. (2013). Integrated analytical and experimental research to develop a new seismic design methodology for precast concrete diaphragms. J. Struct. Eng. 139 (7), 1192–1204. doi:10.1061/(asce)st.1943-541x.0000734
Fox, P. J., Sander, A. C., Elgamal, A., Greco, P., Isaacs, D., Stone, M., et al. (2015). Large soil confinement box for seismic performance testing of geo-structures. Geotechnical Test. J. 38 (1), 20140034–20140084. doi:10.1520/gtj20140034
Franchin, P., and Cavalieri, F. (2015). Probabilistic assessment of civil infrastructure resilience to earthquakes. Computer-Aided Civ. Infrastructure Eng. 30 (7), 583–600. doi:10.1111/mice.12092
Frezzati, M., Loporcaro, G., Buratti, N., Palermo, A., and Walker, A. (2023). Design code review on seismic demand and base detailing criteria: an application to wine tanks in Marlborough. Front. Built Environ. 9. doi:10.3389/fbuil.2023.1167237
Friswell, M., and Mottershead, J. E. (1995). “Finite element model updating in structural dynamics,”, 38. Springer Science and Business Media.
Ganey, R., Berman, J., Akbas, T., Loftus, S., Daniel Dolan, J., Sause, R., et al. (2017). Experimental investigation of self-centering cross-laminated timber walls. J. Struct. Eng. 143 (10), 04017135. doi:10.1061/(asce)st.1943-541x.0001877
Gavras, A. G., Kutter, B. L., Hakhamaneshi, M., Gajan, S., Tsatsis, A., Sharma, K., et al. (2020). Database of rocking shallow foundation performance: dynamic shaking. Earthq. Spectra 36 (2), 960–982. doi:10.1177/8755293019891727
Gelagoti, F., Kourkoulis, R., Anastasopoulos, I., and Gazetas, G. (2012). Rocking isolation of low-rise frame structures founded on isolated footings. Earthq. Eng. and Struct. Dyn. 41 (7), 1177–1197. doi:10.1002/eqe.1182
Ghafory-Ashtiany, M., and Singh, M. P. (1986). Structural response for six correlated earthquake components. Earthq. Eng. and Struct. Dyn. 14 (1), 103–119. doi:10.1002/eqe.4290140108
Ghayoomi, M., Dashti, S., and McCartney, J. S. (2013b). Performance of a transparent Flexible Shear Beam container for geotechnical centrifuge modeling of dynamic problems. Soil Dyn. Earthq. Eng. 53, 230–239. doi:10.1016/j.soildyn.2013.07.007
Ghayoomi, M., and McCartney, J. S. (2011b). Measurement of small-strain shear moduli of partially saturated sand during infiltration in a geotechnical centrifuge. Geotechnical Test. J. 34 (5), 503–513. doi:10.1520/gtj103608
Ghayoomi, M., McCartney, J. S., and Ko, H.-Y. (2011a). Centrifuge test to assess the seismic compression of partially saturated sand layers. Geotechnical Test. J. 34 (4), 321–331. doi:10.1520/gtj103355
Ghayoomi, M., McCartney, J. S., and Ko, H. Y. (2013a). Empirical methodology to estimate seismically induced settlement of partially saturated sand. J. Geotechnical Geoenvironmental Eng. 139 (3), 367–376. doi:10.1061/(asce)gt.1943-5606.0000774
Gosselin, C., Duballet, R., Roux, P., Gaudillière, N., Dirrenberger, J., and Morel, P. (2016). Large-scale 3D printing of ultra-high performance concrete - a new processing route for architects and builders. Mater. and Des. 100, 102–109. doi:10.1016/j.matdes.2016.03.097
Granello, G., Palermo, A., Pampanin, S., Pei, S., and van de Lindt, J. (2020). Pres-lam buildings: state-of-the-art. J. Struct. Eng. 146 (6). doi:10.1061/(asce)st.1943-541x.0002603
Guanche, Y., Guanche, R., Camus, P., Mendez, F. J., and Medina, R. (2013). A multivariate approach to estimate design loads for offshore wind turbines. Wind Energy 16, 1091–1106. doi:10.1002/we.1542
Guidotti, R. (2012). “Near-field earthquake ground motion rotations and relevance on civil engineering structures,” in Ph.D. Thesis, Politecnico di Milano, School of Structural, Seismic and Geotechnical Engineering, XXIV Cycle.
Guidotti, R., Castellani, A., and Stupazzini, M. (2018). Near-field earthquake strong ground motion rotations and their relevance on tall buildings. Bull. Seismol. Soc. Am. 108 (3A), 1171–1184. doi:10.1785/0120170140
Hall, J. F. (1998). Seismic response of steel frame buildings to near-source ground motions. Earthq. Eng. and Struct. Dyn. 27 (12), 1445–1464. doi:10.1002/(sici)1096-9845(199812)27:12<1445::aid-eqe794>3.0.co;2-c
Hall, J. F. (2006). Problems encountered from the use (or misuse) of Rayleigh damping. Earthq. Eng. and Struct. Dyn. 35 (5), 525–545. doi:10.1002/eqe.541
Hall, J. F. (2016a). Discussion of ‘Modelling viscous damping in nonlinear response history analysis of buildings for earthquake excitation’ by Anil K. Chopra and Frank McKenna. Earthq. Eng. and Struct. Dyn. 45 (13), 2229–2233. doi:10.1002/eqe.2761
Hall, J. F. (2017). Discussion on ‘an investigation into the effects of damping and nonlinear geometry models in earthquake analysis’ by Andrew Hardyniec and Finley Charney. Earthq. Eng. and Struct. Dyn. 46 (2), 341–342. doi:10.1002/eqe.2786
Hardyniec, A., and Charney, F. (2015). An investigation into the effects of damping and nonlinear geometry models in earthquake engineering analysis. Earthq. Eng. and Struct. Dyn. 44 (15), 2695–2715. doi:10.1002/eqe.2604
Hariri-Ardebili, M. A., and Saouma, V. (2015). Quantitative failure metric for gravity dams. Earthq. Eng. and Struct. Dyn. 44 (3), 461–480. doi:10.1002/eqe.2481
Haroun, M. A., and Elsanadedy, H. M. (2005). Fiber-reinforced plastic jackets for ductility enhancement of reinforced concrete bridge columns with poor lap-splice detailing. J. Bridge Eng. 10 (6), 749–757. doi:10.1061/(asce)1084-0702(2005)10:6(749)
Hart, G. C., DiJulio Jr, R. M., and Lew, M. (1975). Torsional response of high-rise buildings. J. Struct. Div. 101 (2), 397–416. doi:10.1061/jsdeag.0003999
Hashash, Y. M., Phillips, C., and Groholski, D. R. (2010). “Recent advances in non-linear site response analysis,” in 5th international conference on recent advances in geotechnical earthquake engineering and soil dynamics.
Henry, R., and Ingham, J. (2011). Behaviour of tilt-up precast concrete buildings during the 2010/2011 Christchurch earthquakes. Struct. Concr. 12 (4), 234–240. doi:10.1002/suco.201100035
Hernández, E., Palermo, A., Granello, G., Chiaro, G., and Banasiak, L. J. (2020). Eco-rubber seismic-isolation foundation systems: a sustainable solution for the New Zealand context. Struct. Eng. Int. 30 (2), 180–190. doi:10.1080/10168664.2019.1702487
Hoehler, M. S., Panagiotou, M., Restrepo, J. I., Silva, J. F., Floriani, L., Bourgund, U., et al. (2009). Performance of suspended pipes and their anchorages during shake table testing of a seven-story building. Earthq. Spectra 25 (1), 71–91. doi:10.1193/1.3046286
Holmes, W. T., Liel, A. B., Mehrain, M., Moehle, J. P., and Somers, P. (2017). Seismic evaluation of older concrete frame, frame-wall, and bearing wall buildings for collapse potential. Redwood City: Retrieved from.
Housner, G., Bergman, L. A., Caughey, T. K., Chassiakos, A. G., Claus, R. O., Masri, S. F., et al. (1997). Structural control: past, present, and future. J. Eng. Mech. 123 (9), 897–971. doi:10.1061/(asce)0733-9399(1997)123:9(897)
Housner, G. W. (1963). The behavior of inverted pendulum structures during earthquakes. Bull. Seismol. Soc. Am. 53 (2), 403–417. doi:10.1785/bssa0530020403
Hubler, J. F., Athanasopoulos-Zekkos, A., and Zekkos, D. (2017). Monotonic, cyclic, and postcyclic simple shear response of three uniform gravels in constant volume conditions. J. Geotechnical Geoenvironmental Eng. 143 (9), 04017043. doi:10.1061/(asce)gt.1943-5606.0001723
Hushmand, A., Dashti, S., Davis, C., Hushmand, B., McCartney, J. S., Hu, J., et al. (2016b). Seismic performance of underground reservoir structures: insight from centrifuge modeling on the influence of backfill soil type and geometry. J. Geotechnical Geoenvironmental Eng. 142 (11), 04016058. doi:10.1061/(asce)gt.1943-5606.0001544
Hushmand, A., Dashti, S., Davis, C., McCartney, J. S., and Hushmand, B. (2016a). A centrifuge study of the influence of site response, relative stiffness, and kinematic constraints on the seismic performance of buried reservoir structures. Soil Dyn. Earthq. Eng. 88, 427–438. doi:10.1016/j.soildyn.2016.06.011
Hutchinson, T., Pantelides, C., Morano, M., Liu, J., and Diedrich, E. (2024). “Modular testbed building shakedown test program,” in NHERI UC San Diego LHPOST6 modular testbed building (MTB2) (Austin, TX: DesignSafe-CI). doi:10.17603/ds2-ksqr-nd08
Iai, S. (1989). Similitude for shaking table tests on soil-structure-fluid model in 1g gravitational field. Soils Found. 29 (1), 105–118. doi:10.3208/sandf1972.29.105
Imanpour, A., Tremblay, R., Davaran, A., Stoakes, C., and Fahnestock, L. A. (2016). Seismic performance assessment of multitiered steel concentrically braced frames designed in accordance with the 2010 AISC seismic provisions. J. Struct. Eng. 142 (12), 04016135. doi:10.1061/(asce)st.1943-541x.0001561
Ishihara, K., and Nagase, H. (1988). Multi-directional irregular loading tests on sand. Soil Dyn. Earthq. Eng. 7 (4), 201–212. doi:10.1016/s0267-7261(88)80004-6
Jalali, R. S., and Trifunac, M. D. (2009). Response spectra for near-source, differential, and rotational strong ground motion. Bull. Seismol. Soc. Am. 99 (2B), 1404–1415. doi:10.1785/0120080067
Jehel, P., Léger, P., and Ibrahimbegovic, A. (2014). Initial versus tangent stiffness-based Rayleigh damping in inelastic time history seismic analyses. Earthq. Eng. and Struct. Dyn. 43 (3), 467–484. doi:10.1002/eqe.2357
Johnson, R., Padgett, J. E., Maragakis, M. E., DesRoches, R., and Saiidi, M. S. (2008). Large scale testing of nitinol shape memory alloy devices for retrofitting of bridges. Smart Mater. Struct. 17 (3), 035018. doi:10.1088/0964-1726/17/3/035018
Kalkan, E., and Graizer, V. (2007). Coupled tilt and translational ground motion response spectra. J. Struct. Eng. 133 (5), 609–619. doi:10.1061/(asce)0733-9445(2007)133:5(609)
Kammerer, A. M., Pestana, J. M., and Seed, R. B. (2002). “Undrained response of monterey 0/30 sand under multidirectional cyclic simple shear loading conditions,” in Geotechnical engineering report No UCB/GT/02-01.
Katsanos, E. I., Thöns, S., and Georgakis, C. Τ. (2016). Wind turbines and seismic hazard: a state-of-the-art review. Wind Energy 19 (11), 2113–2133. doi:10.1002/we.1968
Keating, S. J., Leland, J. C., Cai, L., and Oxman, N. (2017). Toward site-specific and self-sufficient robotic fabrication on architectural scales. Sci. Robotics 2 (5), eaam8986. doi:10.1126/scirobotics.aam8986
Keykhosropour, L., Lemnitzer, A., Star, L., Marinucci, A., and Keowen, S. (2018). Implementation of soil pressure sensors in large-scale soil-structure interaction studies. Geotechnical Test. J. 41 (4), 20170163–20170746. doi:10.1520/gtj20170163
Khandelwal, K., El-Tawil, S., Kunnath, S. K., and Lew, H. S. (2008). Macromodel-based simulation of progressive collapse: steel frame structures. J. Struct. Eng. 134 (7), 1070–1078. doi:10.1061/(asce)0733-9445(2008)134:7(1070)
Kim, K., and Elgamal, A. (2017a). “Cut-and-Cover tunnel shake table test program,” in Technical report to the California department of transportation, report No. SSRP-17-09, dept. Of structural engineering. San Diego, CA: Univ. of California.
Kim, K., and Elgamal, A. (2017b). Spillway retaining wall shake table test program: soil-structure interaction. San Diego, CA: Technical Report to the U.S. Bureau of Reclamation, Dept. of Structural Engineering, Univ. of California.
Kim, K., Giacalone, C., Elgamal, A., and Shing, P. B. (2016). Racking response of reinforced concrete cut and cover tunnel (No. CA15-2420; UCSD/SSRP-15-03). Calif. Dept. Transp.
Ko, H.-Y. (1988). “Summary of the state-of-the-art in centrifuge model testing,” in Centrifuges in soil mechanics (London: CRC Press), 11–18.
Koliou, M., Filiatrault, A., Kelly, D. J., and Lawson, J. (2016). Buildings with rigid walls and flexible roof diaphragms. I: evaluation of current US seismic provisions. J. Struct. Eng. 142 (3), 04015166. doi:10.1061/(asce)st.1943-541x.0001438
Koutras, A., and Shing, P. B. (2021). Finite element modeling of the seismic response of reinforced masonry wall structures. Earthq. Eng. Struct. Dyn. 50, 1125–1146. doi:10.1002/eqe.3388
Kramer, A., Barbosa, A. R., and Sinha, A. (2016). Performance of steel energy dissipators connected to cross-laminated timber wall panels subjected to tension and cyclic loading. J. Struct. Eng. 142 (4), E4015013. doi:10.1061/(asce)st.1943-541x.0001410
Kurama, Y., Pessiki, S., Sause, R., and Lu, L. W. (1999). Seismic behavior and design of unbonded post-tensioned precast concrete walls. PCI J. 44 (3), 72–89. doi:10.15554/pcij.05011999.72.89
Kurama, Y. C., Sritharan, S., Fleischman, R. B., Restrepo, J. I., Henry, R. S., Cleland, N. M., et al. (2018). Seismic-resistant precast concrete structures: state of the art. J. Struct. Eng. 144 (4), 03118001. doi:10.1061/(asce)st.1943-541x.0001972
Lai, C.-T., and Conte, J. P. (2024). Dynamic model of the UC San Diego NHERI six-degree-of-freedom large high performance outdoor shake table. Earthq. Eng. Struct. Dyn. 53, 4511–4540. doi:10.1002/eqe.4224
Lanzi, A., and Luco, J. E. (2018). Elastic velocity damping model for inelastic structures. J. Struct. Eng. 144 (6), 04018065. doi:10.1061/(asce)st.1943-541x.0002050
Latha, G. M., and Santhanakumar, P. (2015). Seismic response of reduced-scale modular block and rigid faced reinforced walls through shaking table tests. Geotext. Geomembranes 43 (4), 307–316. doi:10.1016/j.geotexmem.2015.04.008
Le, T. T., Austin, S. A., Lim, S., Buswell, R. A., Law, R., Gibb, A. G., et al. (2012). Hardened properties of high-performance printing concrete. Cem. Concr. Res. 42 (3), 558–566. doi:10.1016/j.cemconres.2011.12.003
Lee, V. W., and Trifunac, M. D. (1985). Torsional accelerograms. Int. J. Soil Dyn. Earthq. Eng. 4 (3), 132–139. doi:10.1016/0261-7277(85)90007-5
Lee, V. W., and Trifunac, M. D. (1987). Rocking strong earthquake accelerations. Soil Dyn. Earthq. Eng. 6 (2), 75–89. doi:10.1016/0267-7261(87)90017-0
Léger, P. (2007). “Reducing the earthquake induced damage and risk in monumental structures: Experience at École Polytechnique de Montréal for large concrete dams supported by Hydro-Québec and Alcan,” in Extreme man-made and natural hazards in dynamics of structures (Dordrecht: Springer), 285–309.
Lehman, D., Moehle, J., Mahin, S., Calderone, A., and Henry, L. (2004). Experimental evaluation of the seismic performance of reinforced concrete bridge columns. J. Struct. Eng. 130 (6), 869–879. doi:10.1061/(asce)0733-9445(2004)130:6(869)
Lemnitzer, A., Khalili-Tehrani, P., Ahlberg, E. R., Rha, C., Taciroglu, E., Wallace, J. W., et al. (2010). Nonlinear efficiency of bored pile group under lateral loading. J. geotechnical geoenvironmental Eng. 136 (12), 1673–1685. doi:10.1061/(asce)gt.1943-5606.0000383
Li, J., Kim, K., and Elgamal, A. (2020). Three-dimensional seismic response of a large embedded structure and induced earth pressure. J. Geotechnical Geoenvironmental Eng. 146 (5), 04020025. doi:10.1061/(asce)gt.1943-5606.0002238
Li, S., Zhang, F., Wang, J. Q., Alam, M. S., and Zhang, J. (2017). Seismic responses of super-span cable-stayed bridges induced by ground motions in different sites relative to fault rupture considering soil-structure interaction. Soil Dyn. Earthq. Eng. 101, 295–310. doi:10.1016/j.soildyn.2017.07.016
Lim, E., Jiang, L., and Chouw, N. (2017). Dynamic response of a non-structural component with three supports in multi-directional earthquakes. Eng. Struct. 150, 143–152. doi:10.1016/j.engstruct.2017.07.028
Lim, S., Buswell, R. A., Le, T. T., Austin, S. A., Gibb, A. G., and Thorpe, T. (2012). Developments in construction-scale additive manufacturing processes. Automation Constr. 21, 262–268. doi:10.1016/j.autcon.2011.06.010
Ling, H. I., Leshchinsky, D., Mohri, Y., and Wang, J. P. (2012). Earthquake response of reinforced segmental retaining walls backfilled with substantial percentage of fines. J. geotechnical geoenvironmental Eng. 138 (8), 934–944. doi:10.1061/(asce)gt.1943-5606.0000669
Ling, H. I., Mohri, Y., Leshchinsky, D., Burke, C., Matsushima, K., and Liu, H. (2005). Large-scale shaking table tests on modular-block reinforced soil retaining walls. J. Geotechnical Geoenvironmental Eng. 131 (4), 465–476. doi:10.1061/(asce)1090-0241(2005)131:4(465)
Liu, W., Hutchinson, T. C., Kutter, B. L., Hakhamaneshi, M., Aschheim, M. A., and Kunnath, S. K. (2013). Demonstration of compatible yielding between soil-foundation and superstructure components. J. Struct. Eng. 139 (8), 1408–1420. doi:10.1061/(asce)st.1943-541x.0000637
Luco, J. E. (1981). “Seismic safety margin research program (phase I), project III-Soil-Structure interaction: linear soil-structure interaction,” in Appendix to soil structure interaction: the status of current analysis methods and research, seismic safety margins research program, NUREG/CR-1780, UCRL-53011. Livermore, CA: Lawrence Livermore National Laboratory.
Luco, J. E. (2014). Effects of soil–structure interaction on seismic base isolation. Soil Dyn. Earthq. Eng. 66, 167–177. doi:10.1016/j.soildyn.2014.05.007
Luco, J. E., and Lanzi, A. (2017). Optimal Caughey series representation of classical damping matrices. Soil Dyn. Earthq. Eng. 92, 253–265. doi:10.1016/j.soildyn.2016.10.028
Luco, J. E., and Lanzi, A. (2019). Numerical artifacts associated with Rayleigh and modal damping models of inelastic structures with massless coordinates. Earthq. Eng. and Struct. Dyn. 48 (13), 1491–1507. doi:10.1002/eqe.3210
Luo, J., Wierschem, N. E., Hubbard, S. A., Fahnestock, L. A., Quinn, D. D., McFarland, D. M., et al. (2014). Large-scale experimental evaluation and numerical simulation of a system of nonlinear energy sinks for seismic mitigation. Eng. Struct. 77, 34–48. doi:10.1016/j.engstruct.2014.07.020
Ma, G., Wang, L., and Ju, Y. (2018). State-of-the-art of 3D printing technology of cementitious material—an emerging technique for construction. Sci. China Technol. Sci. 61 (4), 475–495. doi:10.1007/s11431-016-9077-7
Ma, H., Sause, R., and Dong, J. (2019). Design of horizontally curved steel bridge girders with tubular flanges. J. Bridge Eng. 24 (6), 04019040. doi:10.1061/(asce)be.1943-5592.0001403
Madhusudhanan, S., and Sideris, P. (2018). Capacity spectrum seismic design methodology for bridges with hybrid sliding-rocking columns. J. Bridge Eng. 23 (8), 04018052. doi:10.1061/(asce)be.1943-5592.0001248
Maffei, J., Fathali, S., Telleen, K., Ward, R., and Schellenberg, A. (2014). Seismic design of ballasted solar arrays on low-slope roofs. J. Struct. Eng. 140 (1), 04013020. doi:10.1061/(asce)st.1943-541x.0000865
Mashal, M., and Palermo, A. (2017). Experimental testing of emulative and post-tensioned earthquake damage resistant technologies for accelerated bridge construction. Paper# 61, 16th World Conf. Earthq. Eng. 16WCEE 2017, Santiago, Chile, January 9 – 13.
Mashal, M., and Palermo, A. (2019). Low-damage seismic design for accelerated bridge construction. J. Bridge Eng. 24 (7), 04019066. doi:10.1061/(asce)be.1943-5592.0001406
Mavros, M., Ahmadi, F., Shing, P. B., Klingner, R. E., McLean, D., and Stavridis, A. (2016). Shake-table tests of a full-scale two-story shear-dominated reinforced masonry wall structure. J. Struct. Eng. 142 (10), 04016078. doi:10.1061/(asce)st.1943-541x.0001528
McCartney, J. S., Ghaaowd, I., Fox, P. J., Sanders, M. J., Thielmann, S. S., and Sander, A. C. (2017). Shearing behavior of tire-derived aggregate with large particle size. II: cyclic simple shear. J. Geotechnical Geoenvironmental Eng. 143 (10), 04017079. doi:10.1061/(asce)gt.1943-5606.0001781
Meneses, J. (2010). in The el mayor cucapah, baja California earthquake April 4, 2010. An EERI learning from earthquakes reconnaissance report, EERI (Oakland, CA), 114.
Meza Fajardo, K. C., and Papageorgiou, A. S. (2018). Response of tall buildings to base rocking induced by Rayleigh waves. Earthq. Eng. and Struct. Dyn. 47 (8), 1755–1773. doi:10.1002/eqe.3040
Mitchell, D., DeVall, R. H., Saatcioglu, M., Simpson, R., Tinawi, R., and Tremblay, R. (1995). Damage to concrete structures due to the 1994 Northridge earthquake. Can. J. Civ. Eng. 22 (2), 361–377. doi:10.1139/l95-047
Moaveni, B., He, X., Conte, J. P., and Restrepo, J. I. (2010). Damage identification study of a seven-story full-scale building slice tested on the UCSD-NEES shake table. Struct. Saf. 32 (5), 347–356. doi:10.1016/j.strusafe.2010.03.006
Moehle, J. P. (2000). “State of research on seismic retrofit of concrete building structures in the US,” in Proceedings of US-Japan symposium on seismic rehabilitation of concrete structures-state of research and practice.
Moghadam, S. R., and Konstantinidis, D. (2017). Finite element study of the effect of support rotation on the horizontal behavior of elastomeric bearings. Compos. Struct. 163, 474–490. doi:10.1016/j.compstruct.2016.12.013
Morano, M., Hutchinson, T. C., Pantelides, C. P., Liu, J., and Williamson, E. (2024a). “Shake table testing of a full-scale three-story seismically resilient steel-framed building with reconfigurable lateral force resisting systems (modular testbed building: MTB2),” in La jolla, CA: department of structural engineering. San Diego: University of California.
Morano, M., Lin, L., Hutchinson, T., Liu, J., Williamson, E., and Pantelides, C. P. (2024b). Modal characterization of a three-story buckling-restrained braced frame steel building by nondestructive field testing. Earthq. Eng. and Struct. Dyn. 53, 1705–1726. doi:10.1002/eqe.4077
Morano, M., Liu, J., Williamson, E., Hutchinson, T. C., Pantelides, C., Saxey, B., et al. (2025). Shake table testing of a full-scale three-story seismically resilient steel-framed building with reconfigurable lateral force-resisting systems. Earthq. Spectra. doi:10.1177/87552930241301424
Morrison, M., Schweizer, D., and Hassan, T. (2015). An innovative seismic performance enhancement technique for steel building moment resisting connections. J. Constr. Steel Res. 109, 34–46. doi:10.1016/j.jcsr.2015.02.010
Mottier, P., Tremblay, R., and Rogers, C. (2018). Seismic retrofit of low-rise steel buildings in Canada using rocking steel braced frames. Earthq. Eng. and Struct. Dyn. 47 (2), 333–355. doi:10.1002/eqe.2953
Mylonakis, G., and Gazetas, G. (2000). Seismic soil-structure interaction: beneficial or detrimental? J. Earthq. Eng. 4 (3), 277–301. doi:10.1080/13632460009350372
Naish, D., Fry, A., Klemencic, R., and Wallace, J. (2013). Reinforced concrete coupling beams-Part I: testing. ACI Struct. J. 110 (6), 1057. doi:10.14359/51686160
Nakamura, T., Sekine, E., and Shirae, Y. (2011). Assessment of aseismic performance of ballasted track with large-scale shaking table tests. Q. Rep. RTRI 52 (3), 156–162. doi:10.2219/rtriqr.52.156
National Research Council (2011). Grand challenges in earthquake engineering research: a community workshop report. Washington, DC: National Academies Press.
NHERI (2025). NHERI converging design project website. Available online at: https://tallwoodinstitute.org/nheri-converging-design/(Accessed March 3, 2025)
Nikoukalam, M. T., and Sideris, P. (2016). Low-damage posttensioned segmental bridge columns with flexible end joints for seismic accelerated bridge construction. Transp. Res. Rec. 2592 (1), 151–161. doi:10.3141/2592-17
Oh, S., Kurama, Y. C., Mohle, J., and Saxey, B. W. (2021). Seismic design and analysis of precast concrete buckling-restrained braced frames. PCI J. 66 (5). doi:10.15554/pcij66.5-03
O'Rourke, T. D., Jezerski, J. M., Olson, N. A., Bonneau, A. L., Palmer, M. C., Stewart, H. E., et al. (2008). Geotechnics of pipeline system response to earthquakes. Geotechnical Earthq. Eng. Soil Dyn. IV, 1–38. doi:10.1061/40975(318)193
Otani, S. (1980). Nonlinear dynamic analysis of reinforced concrete building structures. Can. J. Civ. Eng. 7 (2), 333–344. doi:10.1139/l80-041
Ozbulut, O. E., Hurlebaus, S., and DesRoches, R. (2011). Seismic response control using shape memory alloys: a review. J. Intelligent Material Syst. Struct. 22 (14), 1531–1549. doi:10.1177/1045389x11411220
Panagiotou, M., Restrepo, J. I., and Conte, J. P. (2011). Shake-table test of a full-scale 7-story building slice. Phase I: rectangular wall. J. Struct. Eng. 137 (6), 691–704. doi:10.1061/(asce)st.1943-541x.0000332
Pantoli, E., Chen, M. C., Hutchinson, T. C., Astroza, R., Conte, J. P., Ebrahimian, H., et al. (2016b). Landmark data set from the building nonstructural components and systems (BNCS) project. Earthq. Spectra 32 (2), 1239–1259. doi:10.1193/100614eqs150
Pantoli, E., Chen, M. C., Wang, X., Astroza, R., Ebrahimian, H., Hutchinson, T. C., et al. (2016a). Full-scale structural and nonstructural building system performance during earthquakes: Part II–NCS damage states. Earthq. Spectra 32 (2), 771–794. doi:10.1193/012414eqs017m
Pantoli, E., Hutchinson, T. C., McMullin, K. M., Underwood, G. A., and Hildebrand, M. J. (2016c). Seismic-drift-compatible design of architectural precast concrete cladding: tieback connections and corner joints. PCI J. 61. doi:10.15554/pcij61.4-03
Paolucci, R., Shirato, M., and Yilmaz, M. T. (2008). Seismic behaviour of shallow foundations: shaking table experiments vs numerical modelling. Earthq. Eng. and Struct. Dyn. 37 (4), 577–595. doi:10.1002/eqe.773
Papagiannopoulos, G., and Beskos, D. (2012). Damping identification for building structures subjected to earthquakes: A. J. Serbian Soc. Comput. Mech. 6 (1), 129–147.
Parulekar, Y. M., Reddy, G. R., Vaze, K. K., and Kushwaha, H. S. (2004). Lead extrusion dampers for reducing seismic response of coolant channel assembly. Nucl. Eng. Des. 227 (2), 175–183. doi:10.1016/j.nucengdes.2003.09.006
Pecker, A., Paolucci, R., Chatzigogos, C., Correia, A. A., and Figini, R. (2014). The role of non-linear dynamic soil-foundation interaction on the seismic response of structures. Bull. Earthq. Eng. 12 (3), 1157–1176. doi:10.1007/s10518-013-9457-0
Pei, S., Ryan, K., Berman, J., van de Lindt, J., Pryor, S., Huang, D., et al. (2024b). Shake table test of a resilient full-scale ten-story mass timber building. DesignSafe-CI. doi:10.17603/ds2-sxq1-p731
Pei, S., Ryan, K. L., Berman, J. W., van de Lindt, J. W., Pryor, S., Huang, D., et al. (2024a). Shake-table testing of a full-scale 10-story resilient mass timber building. J. Struct. Eng. 150 (12), 04024183. doi:10.1061/jsendh.steng-13752
Pei, S., Van de Lindt, J., Barbosa, A., Berman, J. W., McDonnell, E., Dolan, J. D., et al. (2019). Experimental seismic response of a resilient 2-story mass timber building with post-tensioned rocking walls. J. Struct. Eng. 145 (11), 04019120. doi:10.1061/(asce)st.1943-541x.0002382
Pei, S., van de Lindt, J. W., Ricles, J., Sause, R., Berman, J., Ryan, K., et al. (2017). “Development and full-scale validation of resilience-based seismic design of tall wood buildings: the NEHRI Tallwood Project,” in Proceedings, 2017 NZSEE conference proceedings. Wellington, NZ: New Zealand society for earthquake engineering, 1–8.
Pereira, M., and Koltuniuk, R. (2018). Finite element analysis for spillway retaining wall shake table testing program. USBR Rep., 2018–2102.
Peterman, K. D., Stehman, M. J., Madsen, R. L., Buonopane, S. G., Nakata, N., and Schafer, B. W. (2016). Experimental seismic response of a full-scale cold-formed steel-framed building. I: system-level response. J. Struct. Eng. 142 (12), 04016127. doi:10.1061/(asce)st.1943-541x.0001577
Petrini, L., Maggi, C., Priestley, M. N., and Calvi, G. M. (2008). Experimental verification of viscous damping modeling for inelastic time history analyzes. J. Earthq. Eng. 12 (S1), 125–145. doi:10.1080/13632460801925822
Piras, S., Palermo, A., and Saidi, M. (2022). State-of-the-art of posttensioned rocking bridge substructure systems. J. Bridge Eng. 27 (3). doi:10.1061/(asce)be.1943-5592.0001833
Pozzi, M., and Der Kiureghian, A. (2015). Response spectrum analysis for floor acceleration. Earthq. Eng. and Struct. Dyn. 44 (12), 2111–2127. doi:10.1002/eqe.2583
Priestley, M. N., Sritharan, S., Conley, J. R., and Pampanin, S. (1999). Preliminary results and conclusions from the PRESSS five-story precast concrete test building. PCI J. 44 (6), 42–67. doi:10.15554/pcij.11011999.42.67
Prowell, I., Veletzos, M., Elgamal, A., and Restrepo, J. (2009). Experimental and numerical seismic response of a 65 kW wind turbine. J. Earthq. Eng. 13 (8), 1172–1190. doi:10.1080/13632460902898324
Pyke, R. M., Chan, C. K., and Seed, H. B. (1975). Settlement of sands under multidirectional shaking. J. Geotechnical Eng. Div. 101 (4), 379–398. doi:10.1061/ajgeb6.0000162
Rocha, M. (1957). The possibility of solving soil mechanics problems by the use of models. Proc. 4th ICSMFE. 1, 183–188.
Rodríguez, M. E., Restrepo, J. I., and Blandón, J. J. (2006). Shaking table tests of a four-story miniature steel building—model validation. Earthq. Spectra 22 (3), 755–780. doi:10.1193/1.2220575
Rodríguez, M. E., Santiago, S., and Meli, R. (1995). Seismic load tests on two-story waffle–flat-plate structure. J. Struct. Eng. 121 (9), 1287–1293. doi:10.1061/(asce)0733-9445(1995)121:9(1287)
Roeder, C. W., Graff, R., Soderstrom, J., and Yoo, J. H. (2005). Seismic performance of pile-wharf connections. J. Struct. Eng. 131 (3), 428–437. doi:10.1061/(asce)0733-9445(2005)131:3(428)
Roesset, J. M. (1981). Seismic safety margin research program (phase I), project III-Soil-Structure interaction: a review of soil-structure interaction. Appendix to soil structure interaction: the status of current analysis. Methods Res. Seismic Saf. Margins Res. Program 49.
Rong, W., and McCartney, J. S. (2020). Drained seismic compression of unsaturated sand. J. Geotechnical Geoenvironmental Eng. 146 (5), 04020029. doi:10.1061/(asce)gt.1943-5606.0002251
Rong, W., and McCartney, J. S. (2021). Undrained seismic compression of unsaturated sand. J. Geotechnical Geoenvironmental Eng. 147 (1), 04020145. doi:10.1061/(asce)gt.1943-5606.0002420
Roohi, M., Hernandez, E. M., and Rosowsky, D. (2019). Nonlinear seismic response reconstruction and performance assessment of instrumented wood-frame buildings—validation using NEESWood Capstone full-scale tests. Struct. Control Health Monit. 26 (9), e2373. doi:10.1002/stc.2373
Rosenblueth, E. (1976). Tall buildings under five-component earthquakes. J. Struct. Div. 102 (2), 455–459. doi:10.1061/jsdeag.0004289
Rutherford, C. J., and Biscontin, G. (2013). Development of a multidirectional simple shear testing device. Geotechnical Test. J. 36 (6), 858–866. doi:10.1520/gtj20120173
Ryan, K. L., and Polanco, J. (2008). Problems with Rayleigh damping in base-isolated buildings. J. Struct. Eng. 134 (11), 1780–1784. doi:10.1061/(asce)0733-9445(2008)134:11(1780)
Ryan, K. L., Soroushian, S., Maragakis, E. M., Sato, E., Sasaki, T., and Okazaki, T. (2016). Seismic simulation of an integrated ceiling-partition wall-piping system at E-Defense. I: three-dimensional structural response and base isolation. J. Struct. Eng. 142 (2), 04015130. doi:10.1061/(asce)st.1943-541x.0001384
Saiidi, M. S., and Kavianipour, F. (2018). “Shake table studies of seismic performance of a segmental bridge pier. Proceedings, special session SS08, new technologies for seismic-resistant bridge columns,” in 16th European conference on earthquake engineering.
Sander, A. C., Fox, P. J., Elgamal, A., Pradel, D. E., Isaacs, D., Stone, M., et al. (2013). Seismic testing program for large-scale MSE retaining walls at UCSD. Proc. Geo-Congress, 1188–1195. doi:10.1061/9780784412787.120
Saranyasoontorn, K., and Manuel, L. (2006). Design loads for wind turbines using the environmental contour method. ASME J. Sol. Energy Eng. 128, 554–561. doi:10.1115/1.2346700
Satake, N., Suda, K. I., Arakawa, T., Sasaki, A., and Tamura, Y. (2003). Damping evaluation using full-scale data of buildings in Japan. J. Struct. Eng. 129 (4), 470–477. doi:10.1061/(asce)0733-9445(2003)129:4(470)
Schoettler, M. J., Belleri, A., Dichuan, Z., Restrepo, J. I., and Fleischman, R. B. (2009). Preliminary results of the shake-table testing for the development of a diaphragm seismic design methodology. PCI J. 54 (1), 100–124. doi:10.15554/pcij.01012009.100.124
Seible, F., Priestley, M. N., Hegemier, G. A., and Innamorato, D. (1997). Seismic retrofit of RC columns with continuous carbon fiber jackets. J. Compos. Constr. 1 (2), 52–62. doi:10.1061/(asce)1090-0268(1997)1:2(52)
Shahbazi, M., Cerato, A. B., Allred, S., El Naggar, M. H., and Elgamal, A. (2020b). Damping characteristics of full-scale grouped helical piles in dense sands subjected to small and large shaking events. Can. Geotechnical J. 57 (6), 801–814. doi:10.1139/cgj-2018-0769
Shahbazi, M., Cerato, A. B., El Naggar, M. H., and Elgamal, A. (2020a). Evaluation of seismic soil–structure interaction of full-scale grouped helical piles in dense sand. Int. J. Geomechanics 20 (12), 04020228. doi:10.1061/(asce)gm.1943-5622.0001876
Shahrooz, B. M., and Moehle, J. P. (1987). “Experimental study of seismic response of RC setback buildings,” in Springfield, VA: earthquake engineering research center, college of engineering. University of California.
Shing, P. S. B., and Mahin, S. A. (1987). Elimination of spurious higher-mode response in pseudodynamic tests. Earthq. Eng. and Struct. Dyn. 15 (4), 425–445. doi:10.1002/eqe.4290150403
Sideris, P., Filiatrault, A., Leclerc, M., and Tremblay, R. (2008). “Experimental performance evaluation of inclined shelving for steel pallet type storage racks,” in 14th world conference on earthquake engineering (Beijing, China).
Silva, P. (2019). Collaborative research: self-centering pendulum shear walls in buildings via nonlinear elastic kinematics. NSF-CMMI 1762170.
Silva, P., and Kanitkar, R. (2018). “ACI 440.2 R and the new seismic strengthening guidelines using FRP,”, 327. ACI, Farmington Hills, MI: Special Publication, 20–21.
Singh, A., Hutchinson, T. C., Torabian, S., Schafer, B. W., Peterman, K. D., Padgett, L., et al. (2022). “Structural design narrative of the CFS-NHERI 10-story test building for multi-dimensional shake table testing,” in Proceedings of the cold-formed steel research consortium colloquium 2022. Baltimore, MD, USA.
Singh, A., Zhang, J., Rivera, D., Eladly, M., Jones, H., Kovac, A., et al. (2024). “CFS-NHERI 10-story building shake table test specimen: design updates,” in Proceedings of the 18th world conference on earthquake engineering (WCEE2024). Milan, Italy.
Smith-Pardo, J. P., and Ospina, C. E. (2013). “Special considerations for the seismic analysis and design of piers, wharves and container yards supported on prestressed concrete piles,”, 295. ACI, Farmington Hills, MI: Special Publication, 1–26.
Sogabe, M., Asanuma, K., Nakamura, T., Kataoka, H., Goto, K., and Tokunaga, M. (2013). Deformation behavior of ballasted track during earthquakes. Q. Rep. RTRI 54 (2), 104–111. doi:10.2219/rtriqr.54.104
Song, W., Dyke, S., and Harmon, T. (2013). Application of nonlinear model updating for a reinforced concrete shear wall. J. Eng. Mech. 139 (5), 635–649. doi:10.1061/(asce)em.1943-7889.0000519
Soroushian, S., Ryan, K. L., Maragakis, M., Wieser, J., Sasaki, T., Sato, E., et al. (2012). “NEES/E-Defense tests: seismic performance of ceiling/sprinkler piping nonstructural systems in base isolated and fixed base building,” in 15th world conference on earthquake engineering (15WCEE).
Soydan, C., Gullu, A., Hepbostanci, O. E., Yuksel, E., and Irtem, E. (2012). “Design of a special lead extrusion damper,” in 15th world conference on earthquake engineering.
Spencer, B. F., Yang, G., Carlson, J. D., and Sain, M. K. (1998). Smart dampers for seismic protection of structures: a full-scale study. Proc. second world Conf. Struct. control 1, 417–426.
Stavridis, A., Ahmadi, F., Mavros, M., Shing, P. B., Klingner, R. E., and McLean, D. (2016). Shake-table tests of a full-scale three-story reinforced masonry shear wall structure. J. Struct. Eng. 142 (10), 04016074. doi:10.1061/(asce)st.1943-541x.0001527
Steinbrugge, K. V., and Schader, E. E. (1973). “Earthquake damage and related statistics in san Fernando, California, earthquake of february 9, 1971,”, 1A. Washington DC: National Oceanic and Atmospheric Administration, 709–713.
Stewart, J. P., Smith, P. M., Whang, D. H., and Bray, J. D. (2004). Seismic compression of two compacted earth fills shaken by the 1994 Northridge earthquake. J. geotechnical geoenvironmental Eng. 130 (5), 461–476. doi:10.1061/(asce)1090-0241(2004)130:5(461)
Stojadinović, B., Goel, S. C., Lee, K. H., Margarian, A. G., and Choi, J. H. (2000). Parametric tests on unreinforced steel moment connections. J. Struct. Eng. 126 (1), 40–49. doi:10.1061/(asce)0733-9445(2000)126:1(40)
Sun, L., Didier, M., Delé, E., and Stojadinovic, B. (2015). “Probabilistic demand and supply resilience model for electric power supply system under seismic hazard,” in Proceedings of the 12th international conference on applications of statistics and probability in civil engineering (ICASP2015), 12–15.
Taghavi, S., and Miranda, E. (2003). Response assessment of nonstructural building elements, PEER report 2003/05. Berkeley: University of California, 1–96.
Takayanagi, T., and Schnobrich, W. C. (1979). Non-linear analysis of coupled wall systems. Earthq. Eng. and Struct. Dyn. 7 (1), 1–22. doi:10.1002/eqe.4290070102
Tazarv, M., and Saiid Saiidi, M. (2016). Low-damage precast columns for accelerated bridge construction in high seismic zones. J. Bridge Eng. 21 (3), 04015056. doi:10.1061/(asce)be.1943-5592.0000806
Tian, J., Symans, M. D., Pang, W., Ziaei, E., and van de Lindt, J. W. (2016). Application of energy dissipation devices for seismic protection of soft-story wood-frame buildings in accordance with FEMA guidelines. J. Struct. Eng. 142 (4), E4015009. doi:10.1061/(asce)st.1943-541x.0001269
Tinawi, R., Léger, P., Leclerc, M., and Cipolla, G. (2000). Seismic safety of gravity dams: from shake table experiments to numerical analyses. J. Struct. Eng. 126 (4), 518–529. doi:10.1061/(asce)0733-9445(2000)126:4(518)
TMS (2016). Building code requirements for masonry structures. Longmont, CO: The Masonry Society, 402–416.
Tokimatsu, K., and Seed, H. B. (1987). Evaluation of settlements in sands due to earthquake shaking. J. geotechnical Eng. 113 (8), 861–878. doi:10.1061/(asce)0733-9410(1987)113:8(861)
Tomassetti, U., Graziotti, F., Penna, A., and Magenes, G. (2016). “Out-of-plane shaking table tests on URM cavity walls,” in Brick and block masonry. 1st ed. (London: CRC Press), 10.
Torres Matos, M. A., and Rodríguez, M. E. (2014). Comportamiento sísmico de cimentación tipo candelero para puentes con columnas prefabricadas de concreto reforzado. Ing. sísmica (90), 55–87. doi:10.18867/ris.90.14
Trandafir, A. C., and Bartlett, S. F. (2010). “Seismic performance of double EPS geofoam buffer systems,” in Proc. Of fifth international conference on recent advances in geotechnical earthquake engineering and soil dynamics and symposium in honor of professor IM idriss.
Tremblay, R., Mottier, P., and Rogers, C. (2016). Seismic retrofit of existing low-rise steel buildings in eastern Canada using rocking braced frame system. Can. J. Civ. Eng.
Trifunac, M. D. (1982). A note on rotational components of earthquake motions on ground surface for incident body waves. Int. J. Soil Dyn. Earthq. Eng. 1 (1), 11–19. doi:10.1016/0261-7277(82)90009-2
Trifunac, M. D. (2009a). The role of strong motion rotations in the response of structures near earthquake faults. Soil Dyn. Earthq. Eng. 29 (2), 382–393. doi:10.1016/j.soildyn.2008.04.001
Trifunac, M. D. (2009b). Review: rotations in structural response. Bull. Seismol. Soc. Am. 99 (2B), 968–979. doi:10.1785/0120080068
Trifunac, M. D., Todorovska, M. I., and Ivanović, S. S. (1996). Peak velocities and peak surface strains during Northridge, California, earthquake of 17 January 1994. Soil Dyn. Earthq. Eng. 15 (5), 301–310. doi:10.1016/0267-7261(96)00004-8
Tsang, H. H. (2008). Seismic isolation by rubber–soil mixtures for developing countries. Earthq. Eng. and Struct. Dyn. 37 (2), 283–303. doi:10.1002/eqe.756
Van Den Einde, L., Conte, J. P., Restrepo, J. I., Bustamente, R., Halvorson, M., Hutchinson, T., et al. (2021). NHERI@UC San Diego 6-DOF large high-performance outdoor shake table facility. Front. Built Environ. 6, 580333. doi:10.3389/fbuil.2020.580333
Van Den Einde, L., Zhao, L., and Seible, F. (2003). Use of FRP composites in civil structural applications. Constr. Build. Mater. 17 (6-7), 389–403. doi:10.1016/s0950-0618(03)00040-0
Villaverde, R. (1997). Seismic design of secondary structures: state of the art. J. Struct. Eng. 123 (8), 1011–1019. doi:10.1061/(asce)0733-9445(1997)123:8(1011)
Vosooghi, A., and Buckle, I. G. (2013). “Evaluation of the performance of a conventional four-span bridge during shake table tests,” in Center for civil engineering earthquake research department of civil and environmental engineering. Reno, Nevada: University of Nevada.
Wakabayashi, M., Nakamura, T., Katagihara, A., Yogoyama, H., and Morisono, T. (1973). Experimental study on the elastoplastic behavior of braces enclosed by precast concrete panels under horizontal cyclic loading—parts 1 and 2. Summ. Tech. Pap. Annu. Meet. 6, 121–128.
Wang, D., Dai, J., Qu, Z., and Ning, X. (2016). Shake table tests of suspended ceilings to simulate the observed damage in the M s7. 0 Lushan earthquake, China. Earthq. Eng. Eng. Vib. 15 (2), 239–249. doi:10.1007/s11803-016-0319-z
Wartman, J., Seed, R. B., and Bray, J. D. (2005). Shaking table modeling of seismically induced deformations in slopes. J. Geotech. Geoenv. Eng. 131 (5), 610–622. doi:10.1061/(asce)1090-0241(2005)131:5(610)
Wierschem, N. E., Hubbard, S. A., Luo, J., Fahnestock, L. A., Spencer, B. F., McFarland, D. M., et al. (2017). Response attenuation in a large-scale structure subjected to blast excitation utilizing a system of essentially nonlinear vibration absorbers. J. Sound Vib. 389, 52–72. doi:10.1016/j.jsv.2016.11.003
Wilson, E. L., and Penzien, J. (1972). Evaluation of orthogonal damping matrices. Int. J. Numer. methods Eng. 4 (1), 5–10. doi:10.1002/nme.1620040103
Wu, R. Y., and Pantelides, C. P. (2019). Seismic experiments and analysis of repaired bridge columns using CFRP donut. Spec. Publ. 333, 80–95. doi:10.14359/51720271
Xiao, Y., Priestley, M. N., and Seible, F. (1996). Seismic assessment and retrofit of bridge column footings. Struct. J. 93 (1), 79–94. doi:10.14359/9834
Xie, Y., Zhang, J., DesRoches, R., and Padgett, J. E. (2019). Seismic fragilities of single-column highway bridges with rocking column-footing. Earthq. Eng. and Struct. Dyn. 48 (7), 843–864. doi:10.1002/eqe.3164
Yarahuaman, A., and McCartney, J. S. (2022). Seismic response of rail embankments. Proc. GeoCongress 2022. Charlotte. Mar. 20-23. GSP 336, 300–310. doi:10.1061/9780784484067.031
Yarahuaman, A., and McCartney, J. S. (2024). Full-scale seismic response test on a shallow foundation embedded in tire-derived aggregate for geotechnical seismic isolation. Soil Dyn. Earthq. Eng. 177, 108417. doi:10.1016/j.soildyn.2023.108417
Yazdanian, M., Ingham, J. M., Kahanek, C., and Dizhur, D. (2020). Damage to legged wine storage tanks during the 2013 and 2016 New Zealand earthquakes. J. Constr. Steel Res. 172, 106226. doi:10.1016/j.jcsr.2020.106226
Yegian, M. K., and Kadakal, U. (2004). Foundation isolation for seismic protection using a smooth synthetic liner. J. Geotech. Geoenv. Eng 130 (11), 1121–1130. doi:10.1061/(asce)1090-0241(2004)130:11(1121)
Youd, T. L., Hansen, C. M., and Bartlett, S. F. (2002). Revised multilinear regression equations for prediction of lateral spread displacement. J. Geotech. Geoenv. Eng. 128, 1007–1017. doi:10.1061/(asce)1090-0241(2002)128:12(1007)
Yu, C. C., Mir, F. U. H., and Whittaker, A. S. (2021). Validation of numerical models for seismic fluid-structure-interaction analysis of nuclear, safety-related equipment. Nucl. Eng. Des. 379, 111179. doi:10.1016/j.nucengdes.2021.111179
Zareian, F., and Medina, R. A. (2010). A practical method for proper modeling of structural damping in inelastic plane structural systems. Comput. and Struct. 88 (1-2), 45–53. doi:10.1016/j.compstruc.2009.08.001
Zareian, F., Sampere, C., Sandoval, V., McCormick, D. L., Moehle, J., and Leon, R. (2012). Reconnaissance of the Chilean wine industry affected by the 2010 Chile offshore Maule earthquake. Earthq. Spectra 28 (1_Suppl. 1), 503–512. doi:10.1193/1.4000048
Zareiyan, B., and Khoshnevis, B. (2017). Effects of interlocking on interlayer adhesion and strength of structures in 3D printing of concrete. Automation Constr. 83, 212–221. doi:10.1016/j.autcon.2017.08.019
Zargar, S., Medina, R. A., and Ibarra, L. (2017). “Effect of pellet-cladding bonding on the vibration of surrogate fuel rods,” in Proceedings of international high-level radioactive waste management (IHLRWM 2017) conference (Charlotte, NC).
Zayed, M., Ebeido, A., Prabhakaran, A., Kim, K., Qiu, Z., and Elgamal, A. (2020). Shake table testing: a high-resolution vertical accelerometer array for tracking shear wave velocity. Geotechnical Test. J. 44 (4), 1097–1118. doi:10.1520/gtj20190066
Zayed, M., Ebeido, A., Prabhakaran, A., Qiu, Z., and Elgamal, A. (2021). Asymmetric input motion for accumulation of lateral ground deformation in laminar container shake table testing. Can. Geotechnical J. 58 (2), 210–223. doi:10.1139/cgj-2018-0647
Zembaty, Z., and Boffi, G. (1994). Effect of rotational seismic ground motion on dynamic response of slender towers. Eur. Earthq. Eng. 8, 3–11.
Zhang, J., and Makris, N. (2001). Rocking response of free-standing blocks under cycloidal pulses. J. Eng. Mech. 127 (5), 473–483. doi:10.1061/(asce)0733-9399(2001)127:5(473)
Zhang, J., Singh, A., Eladly, M., Schafer, B. W., and Hutchinson, T. C. (2024a). “Pre-test numerical modeling of the CFS-NHERI 10-story capstone building,” in Proceedings of the 18th world conference on earthquake engineering (WCEE2024). Milan, Italy.
Zhang, J., Singh, A., Eladly, M., Schafer, B. W., and Hutchinson, T. C. (2024b). “Pre-test numerical modeling of the CFS-NHERI 10-story capstone building,” in Proceedings of the 18th world conference on earthquake engineering (WCEE2024). Milan, Italy.
Zhang, Y., Conte, J. P., Yang, Z., Elgamal, A., Bielak, J., and Acero, G. (2008). Two-dimensional nonlinear earthquake response analysis of a bridge-foundation-ground system. Earthq. Spectra 24 (2), 343–386. doi:10.1193/1.2923925
Zhang, Z., Fleischman, R. B., Restrepo, J. I., Guerrini, G., Nema, A., Zhang, D., et al. (2018c). Shake-table test performance of an inertial force-limiting floor anchorage system. Earthq. Eng. and Struct. Dyn. 47 (10), 1987–2011. doi:10.1002/eqe.3047
Zheng, Y., Fox, P. J., Shing, P. B., and McCartney, J. S. (2019a). Physical model tests of half-scale geosynthetic reinforced soil bridge abutments. I: static loading. J. Geotechnical Geoenvironmental Eng. 145 (11), 04019094. doi:10.1061/(asce)gt.1943-5606.0002152
Zheng, Y., McCartney, J. S., Shing, P. B., and Fox, P. J. (2018b). Transverse shaking table test of a half-scale geosynthetic reinforced soil bridge abutment. Geosynth. Int. 25 (6), 582–598. doi:10.1680/jgein.18.00019
Zheng, Y., McCartney, J. S., Shing, P. B., and Fox, P. J. (2019b). Physical model tests of half-scale geosynthetic reinforced soil bridge abutments. II: dynamic loading. J. Geotechnical Geoenvironmental Eng. 145 (11), 04019095. doi:10.1061/(asce)gt.1943-5606.0002158
Keywords: shake table testing, structural systems, geotechnical systems, earthquake engineering, retrofits, construction methods, design codes
Citation: McCartney JS, Van Den Einde L, Morrison M, Palermo A, Lotfizadeh K, Lai C-T and Conte JP (2025) Multi-degree of freedom shake table testing of large-scale structural and geotechnical systems with NHERI-UCSD LHPOST6. Front. Built Environ. 11:1573390. doi: 10.3389/fbuil.2025.1573390
Received: 09 February 2025; Accepted: 10 March 2025;
Published: 23 April 2025.
Edited by:
Navid Jafari, Louisiana State University, United StatesReviewed by:
Paolo Castaldo, Polytechnic University of Turin, ItalyRijalul Fikri, Syiah Kuala University, Indonesia
Copyright © 2025 McCartney, Van Den Einde, Morrison, Palermo, Lotfizadeh, Lai and Conte. This is an open-access article distributed under the terms of the Creative Commons Attribution License (CC BY). The use, distribution or reproduction in other forums is permitted, provided the original author(s) and the copyright owner(s) are credited and that the original publication in this journal is cited, in accordance with accepted academic practice. No use, distribution or reproduction is permitted which does not comply with these terms.
*Correspondence: John S. McCartney, bWNjYXJ0bmV5QHVjc2QuZWR1