- 1 Department of Biochemistry and Molecular Biology, Center for Brain Research, Medical University of Vienna, Vienna, Austria
- 2 INSERM U1024, CNRS UMR8197, Institut de Biologie de l’Ecole Normale Supérieure, Ecolé Normale Supérieure, Paris, France
γ-Aminobutyric acid type A receptors (GABAARs) are the major inhibitory neurotransmitter receptors in the central nervous system, and importantly contribute to the functional regulation of the nervous system. Several studies in the last few decades have convincingly shown that GABA can be co-localized with other neurotransmitters in the same synapse, and can be co-released with these neurotransmitters either from the same vesicles or from different vesicle pools. The co-released transmitters may act on post-synaptically co-localized receptors resulting in a simultaneous activation of both receptors. Most of the studies investigating such co-activation observed a reduced efficacy of GABA for activating GABAARs and thus, a reduced inhibition of the post-synaptic neuron. Similarly, in several cases activation of GABAARs has been reported to suppress the response of the associated receptors. Such a receptor cross-talk is either mediated via a direct coupling between the two receptors or via the activation of intracellular signaling pathways and is used for fine tuning of inhibition in the nervous system. Recently, it was demonstrated that a direct interaction of different receptors might already occur in intracellular compartments and might also be used to specifically target the receptors to the cell membrane. In this article, we provide an overview on such cross-talks between GABAARs and several other neurotransmitter receptors and briefly discuss their possible physiological and clinical importance.
Introduction
GABA (γ-aminobutyric acid) is the major inhibitory transmitter in the brain. Depending on the brain region, about 20–50% of all synapses use GABA as a transmitter. GABA, released from the pre-synaptic terminal can exert its action via two types of receptors, ionotropic GABAA (GABAARs) and metabotropic GABAB receptors (GABABRs). Most of the actions of GABA are mediated via GABAARs. These are chloride ion channels composed of five subunits that can belong to different subunit classes. In mammalian brain there are a total of 19 different subunits derived from 8 different subunit classes (6α, 3β, 3γ, δ, ε, π, θ, 3ρ), giving rise to a multiplicity of different GABAAR subtypes with distinct subunit composition and pharmacology (Sieghart, 1995; Olsen and Sieghart, 2009). The majority of GABAARs, however, is composed of 2α, 2β, and 1γ subunit. Like other neurotransmitter receptors, GABAARs are located at synapses as synaptic clusters (Moss and Smart, 2001; Renner et al., 2008; Bannai et al., 2009) where they are stabilized by scaffold proteins such as gephyrin (Kneussel et al., 1999; Tretter et al., 2008). Recent evidence, however, indicates that “clusters” of these receptors are also present at extrasynaptic locations (Lévi et al., 2008; Kasugai et al., 2010; Kneussel, 2010; Shrivastava et al., 2011). Synaptic as well as extrasynaptic clusters presumably consist of multi-molecular aggregates localized in the plane of the plasma membrane, which can contain not only multiple GABAARs and interacting proteins, but also other types of receptors. In fact, several of such interacting receptors from different transmitter classes have been described to be closely associated with GABAAR clusters. Interestingly, most if not all of the studies addressing GABAAR interactions with other receptors have observed a suppression of GABAergic inhibition upon co-activation of the associated receptors. Additionally, in several studies activation of GABAARs has been reported to suppress the response of the associated receptors. Such a functional inhibition of one receptor activity by activation of a second receptor is often termed as “cross-talk” between these receptors.
Here we summarize what is known on the post-synaptic co-localization and cross-talk of GABAARs with other receptors. Such a cross-talk can be mediated by a direct physical contact of the receptors and subsequent allosteric modulation of their properties on binding of the one, or the other, or of both ligands, by indirect modulation of receptor properties via a second messenger system activated by the associated receptor, or by both mechanisms together. A more remote interaction between different receptors, in which one receptor modulates the release of the transmitter activating the other receptor, will not be systematically considered in this review, because each receptor located pre-synaptically can modulate the release of a transmitter. It only will be mentioned when a possible additional direct cross-talk between a receptor pair seems to exist. Furthermore, modulation of GABAergic transmission by second messenger mechanisms elicited via other synapses will also not be discussed here, because many receptors located in the post-synaptic neuron can cause such modulation. Accordingly, we use the following criteria as supportive arguments for receptor cross-talk: (i) their neurotransmitters are either co-released or can simultaneously interact with both receptors at the post-synaptic membrane, (ii) the receptors are co-localized in certain synapses of the brain, (iii) they physically interact with each other (iv) simultaneous activation of the two receptors produces a non-additive current (current occlusion; Figure 1).
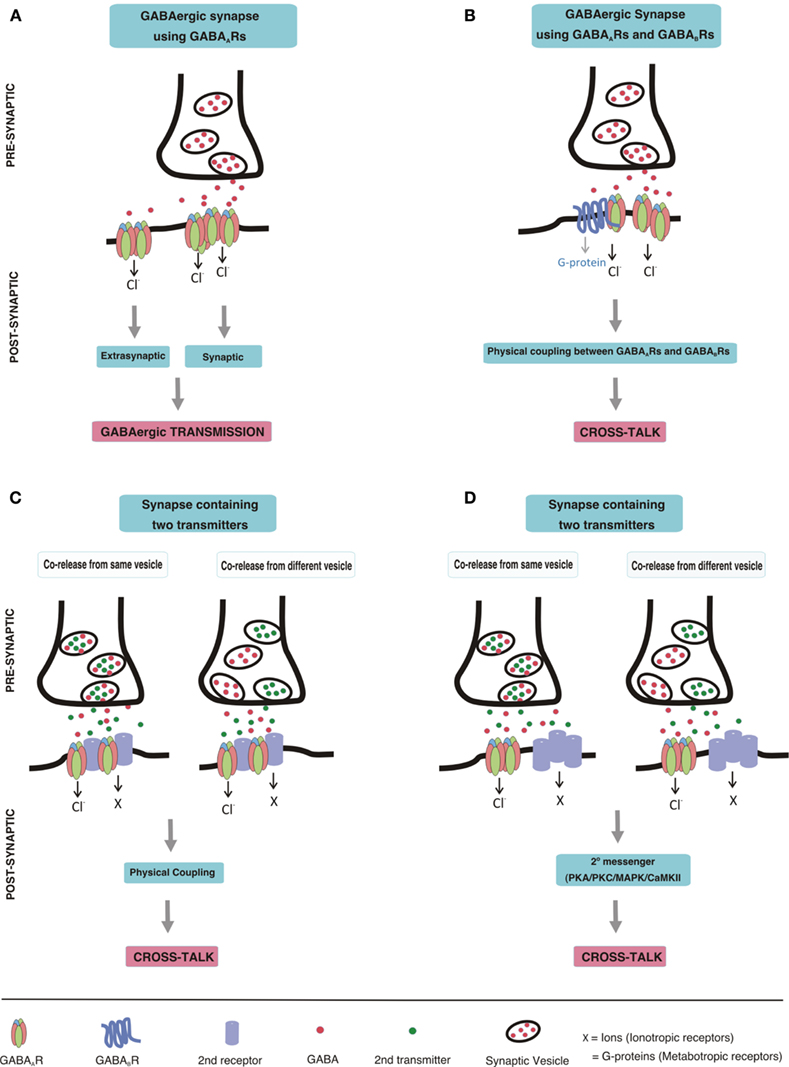
Figure 1. Schematic drawing depicting GABAergic transmission and postsynaptic cross-talk between GABAARs and other receptors (A) GABAergic transmission. GABA, released from a pre-synaptic terminal, acts on post-synaptic GABAAR clusters resulting in phasic transmission. Spillover GABA can also act on extrasynaptic GABAARs, thereby eliciting tonic currents. (B) Cross-talk between GABAARs and GABABRs. Direct physical coupling between GABAARs and GABABRs results in cross-talk at GABAergic synapses expressing both of these receptors. (C,D) Cross-talk of GABAARs with other receptors. Co-release of neurotransmitters from pre-synaptic terminal may occur either from the same vesicles or from different vesicles. Co-released transmitters, then may act on post-synaptically co-localized receptors leading to cross-talk. The cross-talk could be mediated by direct physical coupling of GABAARs with other receptors (C), or by second messenger pathways (D).
The review has been split into the following chapters to separately address individual receptor pairs:
i Cross-talk of GABAA with GABAB receptors
ii Cross-talk of GABAA with glycine receptors
iii Cross-talk of GABAA with nicotinic acetylcholine receptors
iv Cross-talk of GABAA with serotonin receptors
v Cross-talk of GABAA with dopamine receptors
vi Cross-talk of GABAA with purinergic P2X receptors
vii Cross-talk of GABAA with adenosine receptors
viii Cross-talk of GABAA with NMDA receptors
Cross-Talk of GABAA with GABAB Receptors
Pre-synaptically released GABA not only acts on GABAARs but also on GABABRs. GABABRs are metabotropic G-protein coupled receptors, which exist as heteromers of GABAB1 and GABAB2 subunits (Schwenk et al., 2010). These receptors are localized both pre- and post-synaptically (Pinard et al., 2010). Several studies have demonstrated that activation of pre-synaptic GABABRs reduced post-synaptic GABAergic transmission by reducing GABA release (Deisz and Prince, 1989; Davies et al., 1991; Mott and Lewis, 1991; Kardos et al., 1994). Other studies, however, are consistent with an additional cross-talk of post-synaptic GABAA and GABABRs. Immuno-localization studies in the basal ganglion of the human brain indicated that the GABAAα1-subunit and the GABAB1 subunit are co-localized on GABAergic striatal interneurons and on neurons in the globus pallidus and substantia nigra pars reticulata but not on those of substantia nigra pars compacta (Waldvogel et al., 2004). In addition, both receptors were found to be co-localized at symmetrical synapses and possibly also at extrasynaptic sites (Smith et al., 2001). Another study demonstrated a direct interaction of GABAB1 subunits with γ2S subunits of GABAARs in rat brain lysate (Balasubramanian et al., 2004) and results indicated that this subunit regulates GABABR trafficking in multiple ways. This study also demonstrated that GABAB1 subunit co-expression with GABAARs increased the potency of GABA for activating GABAARs.
In addition, earlier studies provide some evidence that the two receptors indeed exhibit cross-talk. Thus, Kardos et al. (1994), reported that the inhibitory action of GABA on K+-evoked glutamate release in cultured cerebral granule cells was similar to that observed by the concerted action of the GABAAR agonist isoguvacine and the GABABR agonist, baclofen. Surprisingly this effect was smaller than the sum of the inhibitory actions of isoguvacine and baclofen added separately. These results for the first time proposed that the two GABA receptors may functionally interact with each other in such a way that the final outcome results in a reduced inhibition (=disinhibition; Kardos et al., 1994; Schousboe, 1999). The functional inhibition of GABAARs by activation of GABABRs was additionally observed using Ca2+-imaging in developing hypothalamic neurons, in which GABAARs are excitatory and raise cytosolic Ca2+ level on activation. Dose-dependent administration of baclofen depressed GABAAR-mediated Ca2+-influx in these cells (Obrietan and van den Pol, 1998). Although the authors confirmed a stronger involvement of pre-synaptic GABABRs on electrically evoked GABA release, they could additionally observe depression of muscimol-evoked GABAAR-mediated Ca2+-influx by baclofen when blocking pre-synaptic activity by tetrodotoxin, suggesting a possible involvement of post-synaptic GABABRs too. Whether these effects were caused by a direct interaction of GABAA and GABAB receptors or by a second messenger mechanism, however, was not investigated.
These studies seem to indicate that pre-synaptically released GABA can act on post-synaptically co-localized GABAA and GABABRs resulting in a reduction of GABAergic signaling. However, more studies have to be performed to understand the possible mechanism of this cross-talk. Additionally, whether GABAAR can also suppress GABABRs mediated signaling, and whether any second messenger system is involved, still needs to be clarified. As GABABRs are highly abundant in the central nervous system (CNS), their simultaneous activation and cross-talk with GABAARs may play an important role in fine tuning of inhibition in the nervous system. Thus, it might limit hyperpolarization caused by excess of GABA release, and simultaneously overcome a failure of inhibition caused by a local change of the Cl− reversal potential (Segal and Barker, 1984; Staley et al., 1995) by GABABR activated K+ channels.
Cross-Talk of GABAA with Glycine Receptors
Glycine receptors (GlyRs), like GABAARs, are members of the cys-loop receptor family and share the load of inhibition with GABAARs in the CNS. GlyRs are highly abundant in spinal cord, brain stem and retina, where GABAARs are also expressed. The neurotransmitters GABA and glycine are co-localized in many synapses, and can be co-released from them. Actually, co-release of GABA and glycine was demonstrated to occur from the same vesicle in interneuron–motoneurons synapses in spinal cord slices (Jonas et. al, 1998). Co-release of GABA and glycine has additionally been reported in brain stem slice preparations at the hypoglossal motoneuron synapse (O’Brien and Berger, 1999) and the abducens motoneuron synapse (Russier et al., 2002). Other studies not only demonstrated the co-existence of GABA and glycine, but also a co-localization of GABAARs and GlyRs at synapses in spinal cord neurons (Triller et al., 1987; Bohlhalter et al., 1994; Todd et al., 1996; Shrivastava et al., 2011). Another recent study provided a quantitative estimate of such mixed GABAergic/glycinergic synapses (Lévi et al., 2008) suggesting that a substantial proportion of spinal cord inhibitory synapses house both of these inhibitory receptors.
Surprisingly, even before the co-release of these two neurotransmitters was demonstrated, a partial inhibition of the GABA response by glycine was reported in rat medullary neurons (Lewis and Faber, 1993). Later, Trombley et al. (1999), using whole-cell voltage clamp recordings, studied this in more detail in rat olfactory bulb neurons, where they observed that in the majority of these neurons, glycine inhibited GABA-evoked currents and GABA inhibited glycine-evoked currents. They additionally observed that on co-application of saturating concentrations of GABA and glycine to these neurons the current amplitude was less than the sum of the currents evoked by GABA and glycine alone, demonstrating current occlusion. Later, similar cross-inhibition was reported in neurons from rat sacral dorsal commissural nucleus (Li et al., 2003). The authors additionally observed that this cross-inhibition was asymmetric (GABA-evoked currents were more affected by glycine than glycine-evoked responses by GABA). In addition, it was reversible, and depended on the phosphorylation of GABAARs. This indicates that more complex signaling pathways might be involved in this cross-talk. Taken together, all these results suggest that the two receptors are in close proximity, but whether they also are physically interacting remains to be clarified.
Recent findings, however, offer alternative explanations for a possible cross-talk of GABAARs and GlyRs, that does not require direct physical interaction of these receptors. Thus, it has been demonstrated that at physiological concentrations GABA acts as an endogenous ligand (weak partial agonist) for synaptic GlyRs (Jonas et al., 1998; De Saint Jan et al., 2001; Lu et al., 2008; Singer, 2008). GABA thus not only activates GABAARs but also to a lesser extent GlyRs. The GlyR activation by GABA, however, would be gone as soon as glycine is simultaneously applied, resulting in non-additive effects. In addition it was reported that GlyRs activated by very high concentrations of GABA have deactivation times 10 times faster than receptors activated by glycine (Fucile et al., 1999). Thus, by directly activating GlyRs, GABA can narrow the time window for effective glycinergic inhibition (Lu et al., 2008) resulting in an additional mechanism of cross-talk of the GABAergic and glycinergic system. Finally, strong simultaneous activation of GABAARs and GlyRs could cause an increase of the local chloride concentration in the cell, thus reducing further chloride influx (Segal and Barker, 1984; Staley et al., 1995). Further experiments will have to analyze whether such mechanisms could explain the observed receptor “cross-talk.”
In any case, GABAAR and GlyR mediated cross-talk in spinal cord and brain stem may precisely regulate the time course of the post-synaptic conductance during development. This may play an important role in motor coordination and the generation of locomotor patterns (Jonas et al., 1998; O’Brien and Berger, 1999), however this possibility so far has not been investigated. The interaction possibly also helps in shaping odor information processing in developing olfactory bulb (Trombley et al., 1999) and acoustic information processing in auditory synapses (Lu et al., 2008). Finally, it provides a mechanism that ensures efficient synaptic inhibition even in the case of a mutation in the one or the other transmitter receptor types.
Cross-Talk of GABAA with Nicotinic Acetylcholine Receptors
Nicotinic acetylcholine receptors (nAChRs) are other major members of the cys-loop receptor family. In contrast to GABAARs, that are ligand-gated anion channels, nAChRs are ligand-gated cation channels. Co-localization of GABA and the ACh-synthesizing enzyme choline acetyltransferase has been demonstrated in rodent cortical neurons (Hallanger et al., 1986), chick retina amacrine-like cells (O’Malley and Masland, 1989; Santos et al., 1998) and in the nerve fibers innervating adrenal gland (Iwasa et al., 1999). Moreover, several studies have convincingly demonstrated that GABA can be co-released with ACh from cholinergic terminals (O’Malley and Masland, 1989; O’Malley et al., 1992; Lee et al., 2010). Unlike co-release of GABA and glycine, which involves release from the same vesicles, all these studies suggested the involvement of different vesicle pools in the co-release of GABA and ACh (Santos et al., 1998; Lee et al., 2010). Even though it is well established that GABA can be co-released at cholinergic nerve terminals, very few studies have focused on post-synaptic co-localization of GABAA and nACh receptors. There is some evidence that the α7-subtype containing nAChRs co-localize with GABAARs in interneurons of embryonic hippocampus (Kawai et al., 2002; Zago et al., 2006). It remains to be elucidated, however, if other subtypes of nAChRs apart from the α7-subtype also co-localize with GABAARs in brain regions where GABA and acetylcholine are co-released.
Direct evidence of a post-synaptic cross-talk between GABAARs and α7-nAChRs comes from recent studies on rodent hippocampal interneurons and on chick ciliary ganglion neurons (Wanaverbecq et al., 2007; Zhang and Berg, 2007). Using whole-cell voltage clamp recording, it was demonstrated that activation of post-synaptically localized α7-nAChRs reversibly inhibited GABAergic inhibitory post-synaptic currents (IPSCs) by generating a calcium influx, resulting in Ca2+/calmodulin-dependent kinase II (CaMKII), Mitogen-activated protein kinase (MAPK; Zhang and Berg, 2007), and protein kinase C (PKC; Wanaverbecq et al., 2007) dependent phosphorylation of GABAARs. This inhibition was observed in autonomic neurons where α7-nAChRs are concentrated on the soma along with GABAARs, and on hippocampal interneurons where α7-nAChRs often co-cluster with GABAARs on dendrites and filopodia (Zhang and Berg, 2007). It however remains to be determined if Ca2+ influx via α7-nAChRs may additionally result in phosphorylation and/or internalization of synaptic GABAARs. In any case, as hippocampal interneurons are spontaneously active by generating rhythms (Cobb et al., 1995) even when glutamatergic transmission is blocked, their regulation by α7-nAChRs, which are highly enriched in interneurons, possibly plays an important role in modulating inhibitory neurotransmission.
In addition, pre-synaptic nAChRs depending on their subunit composition are known to facilitate action-potential dependent GABA release in hippocampal interneurons (Alkondon et al., 1997, 1999; Ji and Dani, 2000; Buhler and Dunwiddie, 2002), superficial superior colliculus neurons (Endo et al., 2005), and ventral tegmental area dopamine neurons (Yang et al., 2011). Surprisingly, Wanaverbecq et al. (2007), in their study, also observed an increase in pre-synaptic GABA release along with post-synaptic suppression of GABAAR-mediated IPSCs, effects that should oppose each other. In any case, cholinergic input can both facilitate as well as suppress GABAergic neurotransmission via complex pre-synaptic as well post-synaptic mechanisms. Further studies addressing regulation of GABAARs by both pre- and post-synaptic nAChRs are required to provide clues on the mechanism and function of this fine tuning.
Cross-Talk of GABAA with Serotonin Receptors
Serotonin (5-Hydroxytryptamine, 5-HT) is a monoamine transmitter that exerts its action by binding to serotonin receptors (5-HTRs) that are widely expressed in the nervous system as well as in non-neuronal tissue. Apart from the 5-HT3R subtype, which is a ligand-gated ion channel and member of the cys-loop receptor family, all other serotonin receptors (5-HT1, 2, 4–7) belong to the G-protein coupled receptor family (Hoyer et al., 2002; Kitson, 2007). So far, the only evidence for a co-localization of the transmitters GABA and serotonin comes from a recent study in the early vertebrate sea lamprey, where serotonin and GABA was demonstrated to co-exist in several brain regions (Barreiro-Iglesias et al., 2009a). Whether such a co-existence of these neurotransmitters and their receptors is also present in the mammalian nervous system, still needs to be determined. Furthermore, a co-release of serotonin with GABA (along with ATP) has only been reported in non-neuronal rat pancreatic beta cells (Braun et al., 2007), and thus, a possible co-release in the CNS still needs to be investigated.
However, a recent study for the first time described a cross-talk between 5-HT3Rs and GABAARs in myenteric neurons using whole-cell recordings (Miranda-Morales et al., 2007), by providing evidence for current occlusion on simultaneous activation of these two receptors. The cross-talk as observed at saturating concentration of agonists for both receptors occurred immediately after the application of the two agonists, possibly suggesting that the two receptors are located very close to each other. The authors provided additional evidence for an allosteric interaction as they observed current occlusion even in the absence of Ca2+ and also in the presence of staurosporine, a general protein kinase inhibitor. Further experiments will have to investigate the mechanism of interaction of these receptors. As 5-HT3R have a preferential localization on a sub-population of GABAergic interneurons in hippocampus and cortex, cross-talk between 5-HT3Rs and GABAARs possibly may play a crucial role in the control of the balance between excitation and inhibition in the CNS (Chameau and van Hooft, 2006). It may also be important to note that the co-localization as reported for nACh/GABAA and 5-HT3/GABAA receptors have been primarily observed in interneurons suggesting that nAChRs and 5-HT3Rs possibly participate in fine tuning of inter-neuron functioning.
So far, there is no evidence of any post-synaptic cross-talk between GABAARs and G-protein coupled serotonin receptors. However, pre-synaptic potentiation of GABA release by serotonin, as well as by 5-HT2 and 5-HT3 receptors has been documented in spinal cord (Xu et al., 1998; Li et al., 2000; Fukushima et al., 2009) and hippocampal neurons (Dorostkar and Boehm, 2007). Whether these receptors additionally cross-talk with GABAARs receptors post-synaptically, still remains to be clarified.
Cross-Talk of GABAA with Dopamine Receptors
Dopamine receptors are G-protein coupled receptors consisting of five different subtypes, namely D1–D5 receptors. These receptors act via adenylyl cyclase mediated second messenger systems and are thus responsible for long-term regulation of brain functioning (Girault and Greengard, 2004). Even though there are only very few dopaminergic neurons, they have been described to actively regulate post-synaptic GABAergic signaling. Virtually all dopaminergic pre-synaptic terminals in glomerulus were reported to be glutamic acid decarboxylase (GAD) positive (Gall et al., 1987), suggesting a possible co-existence and co-release of the transmitters dopamine and GABA. This conclusion was supported by demonstrating co-localization of tyrosine hydroxylase and GAD in periglomerular cells in mouse brain slices (Maher and Westbrook, 2008). Additional evidence for the co-existence of dopamine and GABA came from a study on the brain of early vertebrate sea lamprey, where these two neurotransmitters were observed to co-exist in several brain regions (Barreiro-Iglesias et al., 2009b). Finally, Maher and Westbrook, in their study also reported the co-release of GABA and dopamine from dopaminergic nerve terminals of periglomerular cells.
Several studies have demonstrated that most, if not all members of the dopamine receptor family modulate post-synaptic GABAAR-mediated neurotransmission in different regions of the brain. However, so far only dopamine D5 receptors have been demonstrated to directly bind to and cross-talk with GABAARs (Liu et al., 2000). It was demonstrated that D5, but not D1 receptor antibodies precipitated hippocampal GABAARs, indicating complex formation between these two receptors in vivo. Additionally, it was shown that the C-terminus of dopamine D5, but not D1 receptors could directly interact with the intracellular loop between the third and fourth transmembrane domain of the γ2-subunit of GABAARs. In addition, the authors reported that co-activation of GABAA and D5 receptors suppressed dopamine induced cAMP accumulation and resulted in current occlusion through GABAARs. In agreement with the absence of co-precipitation with D1 receptors, the authors observed no functional cross-talk. Actually, this was one of the first studies demonstrating that cross-talk could indeed occur via direct coupling between two receptors; however an additional involvement of adenylyl cyclase induced intracellular signaling following the activation of D1 receptors cannot be ruled out. Surprisingly, most of the other studies investigating dopamine/GABAA receptor interaction only dealt with this second possibility.
Although Liu et al. (2000), observed no interaction and cross-talk of GABAAR with D1 receptors in hippocampus, three other studies using whole-cell patch-clamp recordings, reported a suppression of GABAAR-mediated chloride influx following activation of dopamine D1 receptors in olfactory bulb neurons (Brünig et al., 1999) and in the striatum (Flores-Hernandez et al., 2000; Goffin et al., 2010). Using radiolabeling and immunoprecipitation experiments, it was initially demonstrated that activation of D1 receptors increased the phosphorylation of β1/β3 subunit of GABAARs via PKA/DARPP-32/PP1 (protein kinase A/Dopamine- and cAMP-regulated phosphoprotein/Protein phosphatase 1) signaling cascade, which in turn leads to reduced GABA-evoked currents in neostriatal medium spiny neurons (Flores-Hernandez et al., 2000). Recently, Goffin et al. (2010) additionally showed that 10 min to 7 days treatment of striatal neurons with D1 receptor agonist resulted in a decreased number of GABAARs at the cell surface, eventually leading to a reduction in the number of GABAergic synapses and in GABAAR cluster size. Surprisingly, they observed that the reduction in number of GABAARs was due to endocytosis followed by dephosphorylation of β-subunits by a protein phosphatase 2A-dependent pathway (PP2A; Goffin et al., 2010). Thus it seems likely, that the activation of D1 receptors results in rapid PKA-dependent phosphorylation of GABAARs, which over time, gets dephosphorylated by PP2A and eventually endocytosed, leading to sustained inhibition. The effect of GABAAR activation on D1 receptor signaling and whether there is a direct interaction between the two receptors in striatal neurons was not investigated in these studies.
In similar lines, Goffin et al. (2010), additionally observed a D2 receptor-mediated down-regulation of surface GABAARs following dephosphorylation of β-subunits involving protein phosphatase 1 dependent pathway, eventually resulting in a smaller inhibitory response in the medium spiny neurons of the striatum (Goffin et al., 2010). In contrast, Brünig et al. (1999), had previously reported that D2 receptor activation resulted in an enhancement of GABAergic transmission due to a phosphorylation of GABAARs involving the protein kinase C pathway in mitral/tufted cells in the olfactory bulb. These discrepancies may possibly suggest a cell-type-specific modulation by dopamine receptors. More studies in this direction have to be performed to clarify this discrepancy. The reciprocal suppression of D2 receptor function by activation of GABAARs has been demonstrated in another study using dopamine D2 receptor radio-ligand binding assays in membrane preparation from rat neostriatum. It was demonstrated that the activation of GABAARs significantly increased the dissociation constant of the high affinity selective D2 receptor antagonist, raclopride, (Pérez de la Mora et al., 1997) which could be reversed by use of the GABAAR antagonist bicuculline. Altogether, these results suggest a high probability of reciprocal cross-talk and possibly direct interaction between GABAA/D2 receptors in similar lines as proposed for GABAA/D5 receptors. However, this needs to be investigated further.
Finally, using whole-cell patch-clamp recordings in various brain regions, the last two members of the dopamine receptor family, the D3 and D4 receptors, have also been demonstrated to reduce GABAergic inhibition by stimulating endocytosis of GABAARs via the PKA/protein phosphatase I pathway. D3 receptors seem to reduce GABAAR-mediated current by inactivating PKA activity, resulting in dephosphorylation of β-subunits, followed by clathrin/activator protein 2-mediated endocytosis of GABAARs in the nucleus accumbens (Chen et al., 2006) and hippocampus (Swant et al., 2008). Similarly, PKA/protein phosphatase I pathway-dependent rundown of GABAARs by D4 receptors has been reported in prefrontal cortex (Wang et al., 2002) and globus pallidus neurons (Shin et al., 2003). Functional experiments on dopamine receptors will clarify whether D3 or D4 receptors are also reciprocally inhibited followed by GABAAR activation.
Based on all these studies, it seems clear that complex intracellular signaling pathways can be activated by dopamine receptors, which then determines the fate of nearby localized GABAARs. In addition, a direct coupling and a reciprocal cross-talk between dopamine and GABAA receptors cannot be ruled out. In any case, it seems that dopamine receptors are important regulators of surface GABAAR expression. As GABAARs are highly mobile and continuously exchange between synaptic and extrasynaptic sites as well as by exocytosis and endocytosis, it is apparent that interactions with dopamine receptors may be one of the determinants for such rapid transition and therefore directly defining the strength of inhibitory synapse. Loss of coordination between the GABAergic and dopaminergic system could contribute to psychomotor and neuropsychiatric diseases in striatum and cortex, which affects both these pathways (Liu et al., 2000). Inhibition of GABAAR-mediated IPSCs by dopamine receptors could enhance oscillations between globus pallidus neurons and subthalamic nucleus, thereby ameliorating Parkinson’s disease (Shin et al., 2003). Additionally the action of dopamine on GABAergic inhibitory circuit in olfactory bulb could assist in odor detection and olfactory learning (Brünig et al., 1999).
Cross-Talk of GABAA with P2X Receptors
P2X receptors (P2XRs) are ligand-gated ion channels that are activated by adenosine-5′-triphosphate (ATP). Seven different subtypes of these receptors are now known (P2X1–P2X7) which assemble to form predominantly homo-trimeric but also hetero-trimeric receptors (Burnstock, 2006). Even though ATP got late recognition as a neurotransmitter, it is now well established that it acts as a fast excitatory neurotransmitter and co-transmitter in several regions of the CNS. Synaptic co-release of ATP with GABA but not glutamate has been demonstrated in the rat spinal cord and mouse lateral hypothalamus (Jo and Schlichter, 1999; Jo and Role, 2002). Moreover, co-localization studies have clearly demonstrated the co-existence of P2X2R-subtype with GABAARs in dorsal root ganglion (Labrakakis et al., 2003) and spinal cord neurons (Shrivastava et al., 2011), suggesting a possible physical association between these receptors.
A direct cross-talk between GABAARs and P2XRs was first demonstrated using whole-cell patch-clamp recording in rat dorsal root ganglion cells. It was demonstrated that co-application of the agonists of GABAARs and P2XRs produced a total current much smaller than the predicted linear summation of individual responses (Sokolova et al., 2001). This interaction was shown to be dependent on Ca2+ influx through endogenous P2XRs expressed in dorsal root ganglion neurons. Another study, investigated the mechanism of this cross-inhibition in more detail in a recombinant system after the co-expression of GABAARs and P2X2Rs in Xenopus oocytes (Boué-Grabot et al., 2004b). Surprisingly, the authors observed the cross-talk to be independent of Ca2+influx via P2X2Rs. Additionally, they demonstrated that the intracellular C-terminus of P2X2Rs and of the intracellular loop (between transmembrane domain III and IV) of GABAAR β-subunit is essential for the interaction, which was additionally verified in our recent study (Shrivastava et al., 2011). In agreement with the previous study, Ca2+-independent cross-talk was also reported in myenteric neurons (Karanjia et al., 2006). Finally, a similar cross-talk between P2X3Rs/GABAARs and P2X4Rs/GABAARs was reported recently (Toulmé et al., 2007; Jo et al., 2011).
We studied this interaction in more detail by employing co-immunoprecipitation and FRET imaging in transiently transfected HEK cells, in combination with single particle tracking and quantitative immunocytochemistry in primary neurons of spinal cord. We demonstrated that GABAARs and P2X2Rs interact with each other already intracellularly possibly within the endoplasmic reticulum and are then co-transported to the cell membrane where they are primarily co-localized extrasynaptically in mice spinal cord neurons (Shrivastava et al., 2011). Additionally we observed that upon activation of P2X2Rs by ATP or P2X2R agonists, this transient complex gets dissociated. This agonist-induced dissociation seems to be both Ca2+ dependent and thus mediated via signaling mechanisms and Ca2+ independent and thus mediated via a conformational change. Although this cannot explain the cross-talk observed by electrophysiological studies due to the much longer time course used in our studies, it appears that following activation of one of the two receptors, an initial Ca2+-independent (Boué-Grabot et al., 2004b; Karanjia et al., 2006) conformational change results in current occlusion, followed by a Ca2+-dependent (Sokolova et al., 2001; Shrivastava et al., 2011) dissociation of this receptor–receptor complex. Whether this Ca2+-influx additionally leads to activation of Ca2+-dependent intracellular signaling pathways, as reported for nACh and dopamine receptors, remains a highly intriguing question to be answered.
The importance of cross-talk involving members of P2XR family can be estimated by the fact that P2XRs not only cross-talk with GABAARs, but are now known to also cross-talk with most of the other members of cys-loop receptor family, possibly in a similar way (Zhou and Galligan, 1998; Khakh et al., 2000, 2005; Sokolova et al., 2001; Boué-Grabot et al., 2004a,2004b; Karanjia et al., 2006; Decker and Galligan, 2009). P2XR-mediated regulation of GABAAR number at the cell surface can have direct implications on modulating pain transmission in spinal cord (Zeilhofer et al., 2009; Shrivastava et al., 2011) or on epileptic seizures in hippocampus (Kang et al., 2003).
Cross-Talk of GABAA with Adenosine Receptors
Adenosine receptors are members of the G-protein coupled purine receptor family, with adenosine being their natural ligand. Four different adenosine receptors are known, namely A1, A2A, A2B, and A3 receptors (Burnstock, 2006). ATP released from pre-synaptic terminals rapidly degrades to ADP and adenosine by ectonucleotidases that are present in the synaptic cleft (Abbracchio et al., 2009). Thus, ATP co-released from GABAergic terminals (Jo and Schlichter, 1999) can also give rise to adenosine. An additional source of ATP and hence adenosine are astrocytes surrounding the neurons. Thus, it is highly probable that even in the absence of a direct co-release with GABA, adenosine may modulate GABAAR signaling if adenosine receptors are in the vicinity of GABAARs. Although to the best of our knowledge no co-localization study of adenosine receptors and GABAARs has been published so far, there have been several reports suggesting a possible post-synaptic cross-talk of adenosine receptors and GABAARs.
Cross-talk between GABAA and A1 receptors has been reported in dorsal root ganglion neurons and sacral dorsal commissural nucleus neurons where a suppression of post-synaptic GABAAR-mediated chloride current was observed in the presence of adenosine and the A1 receptor agonist N6-cyclohexyladenosine but not the A2A receptor agonist 2-[p-(2-carboxyethyl)-phenethylamino]-5’-N-ethylcarboxamidoadenosine (Hu and Li, 1997; Li et al., 2004) using voltage-clamped whole-cell patch recordings. The suppression effect of adenosine could be blocked specifically by the A1 receptor antagonist, 8-cyclopentyl-1,3-dipropylxanthine. Interestingly, this inhibition of GABAAR-mediated chloride flux was observed to occur via a Ca2+-independent–PKC-dependent pathway and also did not involve PKA-dependent pathways. Although the involvement of PKC strongly suggests that post-synaptic A1 receptors were mediating this response, a possible inhibition of GABA release by pre-synaptic A1 receptors cannot be ruled out. Surprisingly, no study was published in the last few years investigating this cross-talk further. However, a couple of recent studies suggest an important functional role of adenosine receptors in the regulation of GABAergic transmission under pathological conditions. After injecting neurosurgically resected epileptic human brain tissue in Xenopus oocytes, the oocytes use the mRNAs present in the tissue to synthesize the respective proteins and receptors that then can be investigated electrophysiologically by whole-cell recordings. When GABAARs expressed by these oocytes were investigated, the authors observed a rundown of the GABA-induced current on repetitive application of GABA, which could be significantly reduced by antagonists of A2A, A2B, and A3 receptors and surprisingly not by those of A1 receptors. A similar effect was found in rodent brain (Roseti et al., 2008, 2009). The mechanism behind this effect remains to be elucidated, however the authors speculate on the possible involvement of MAPK and/or PKA-dependent pathway regulating the rundown of GABAAR-mediated current. Adenosine receptor-mediated regulation of GABAAR functioning has additionally been implicated in hypoxia, ischemia, and pain, which is supported by that fact that under the conditions of tissue injury and inflammation, an increase in the levels of purines in the neuronal system is observed (Gourine et al., 2007). It is highly interesting to speculate that a functional cross-talk between GABAARs and adenosine receptors may play an important role under conditions of neuro-inflammation.
Cross-Talk of GABAA with N-Methyl-D-Aspartate Receptors (NMDA) Receptors
Several studies have demonstrated that glutamate and GABA can be co-released from inhibitory as well as excitatory nerve terminals. This is not surprising because glutamate is the precursor for the synthesis of GABA. In one of the first studies, the presence and release of glutamate and aspartate, in addition to GABA, was demonstrated from the anti-GAD immune-purified GABAergic synaptosomal preparations from brain cortex (Docherty et al., 1987). These GABAergic synaptosomes released glutamate in response to depolarizing treatments with either potassium or veratrine, a sodium channel activator. However, whether these synaptosomal preparations were in fact purely GABAergic and not contaminated with glutamatergic synaptosomes cannot be answered retrospectively. Recent studies have identified the type 3 vesicular glutamate transporter (VGLUT3) to be co-localized extensively with vesicular GABA transporter (VGAT) around unstained pyramidal cells and granule cells in the hippocampus (Boulland et al., 2004) and in developing auditory medial nucleus of the trapezoid body (Gillespie et al., 2005). Boulland et al. (2004), additionally observed a dramatic increase in co-localization of VGAT and VGLUT3 in GABAergic nerve terminals during early stages of development suggesting co-release of glutamate along with GABA plays a crucial role during development. In fact, a very recent work demonstrated that the co-transmission of glutamate in developing GABA/glycinergic sound localization pathway is crucial for synaptic reorganization of inhibitory circuits and disruption of glutamate co-transmission prevented the strengthening of inhibitory connections that would normally occur with maturation (Noh et al., 2010). In a very recent study, Fattorini et al. (2009), observed that GABAergic synapses can express VGLUT1 and glutamatergic synapses can express VGAT. This was further supported by immunoisolation experiments demonstrating that anti-VGAT immunoisolated vesicles contained VGLUT1 and anti-VGLUT1 immunoisolated vesicles contained VGAT (Fattorini et al., 2009). A more recent work using postembedding immunogold double labeling additionally revealed that VGLUT1, VGLUT2, and VGAT coexist in mossy fiber terminals of the hippocampal CA3 area and cerebellar mossy fiber terminals. The presence of VGLUT2 was also demonstrated in cerebellar GABAergic basket cells in this study (Zander et al., 2010).
The co-localization of pre-synaptic transporters for co-released glutamate and GABA suggests a possible co-localization of their respective receptors at post-synaptic terminals. So far, co-localization of glutamate receptors at GABAergic synapses has not been extensively investigated. Recently, however, the existence of NMDA receptors in hippocampal CA1 and CA3c GABAergic synapses has been demonstrated (Szabadits et al., 2011). In these synapses NMDA receptors triggered a retrograde nitric oxide-cGMP cascade, thus modulating GABAergic inhibition in an activity-dependent manner. Since GABAergic inhibition plays a central role in the control of pyramidal cell ensemble activities, such a regulation is able to fine-tune network patterns.
In contrast, localization of GABAARs at the post-synaptic membrane of glutamatergic synapses has been demonstrated in several studies. Thus, using electron microscopic immunogold labeling, it was demonstrated that the α6, but not the α1 subunit of GABAARs was also concentrated in glutamatergic cerebral mossy fiber synapses (Nusser et al., 1996). More functional evidence on the existence of GABAARs at glutamatergic synapses comes from a very recent study where the authors observed that at puberty, expression of inhibitory α4 and δ subunits of GABAARs increased by 700% on the plasma membrane perisynaptically to asymmetric glutamatergic synapses of hippocampal CA1 pyramidal neurons (Shen et al., 2010). Among other subunits, the primarily extrasynaptically localized α5 subunit has also been detected at excitatory synapses (Crestani et al., 2002). The existence of post-synaptic GABAARs at excitatory synapses may serve to regulate the time course of excitation and to limit glutamatergic overexcitation and subsequent excitotoxicity.
Post-synaptically, glutamate exerts its action via ionotropic NMDARs, AMPARs, and kainate receptors, or metabotropic glutamate receptors (Dingledine et al., 1999). Among these, NMDA receptors have been best studied and shown to directly modulate GABAAR functioning in a Ca2+ dependent manner. NMDA receptors are highly permeable to Ca2+and are localized to post-synaptic densities, where they are structurally organized in characteristic spines (Lau and Zukin, 2007). Several studies demonstrate that activation of NMDARs resulted in the suppression of GABAAR function due to calcium dependent activation of phosphatase 2B/calcineurin and consecutive dephosphorylation of GABAARs in hippocampus (Stelzer and Shi, 1994; Chen and Wong, 1995; Marsden et al., 2007; Bannai et al., 2009) and cerebellum granule cells (Robello et al., 1997). Following dephosphorylation of serine-327 in the intracellular loop of GABAARs γ2-subunit, synaptic GABAARs then escape to extrasynaptic sites (Wang et al., 2003; Bannai et al., 2009; Muir et al., 2010). In contradiction to all these observations, one study reported that NMDAR activation resulted in the removal of AMPARs from the membrane while simultaneously increased expression of surface GABAARs (Marsden et al., 2007). However, this pathway was dependent on Ca2+/calmodulin-dependent protein kinase II (CaMKII). This discrepancy possibly could be explained on the basis of the Ca2+ level in the cell followed by activation of NMDARs. NMDAR activation of CaMKII is generally associated with high levels of Ca2+ influx (Silva et al., 1992), so depending on the level of Ca2+ influx through NMDARs, phosphatases (calcineurin), or kinases (CaMKII) may be activated resulting in a decrease or increase of surface GABAARs, respectively. Thus, it seems clear that glutamate primarily acting via NMDARs can modulate the expression of GABAARs, thus modulating inhibition. It would be interesting to see whether GABA acting via GABAARs can also modulate NMDAR expression or function. However a direct cross-talk of NMDARs and GABAARs has not been investigated in these studies.
Conclusion
Recently it was demonstrated that several members of the G-protein coupled receptors can heteromerize, thus producing functional entities that possess different biochemical characteristics with respect to the individual components of the heteromer. In addition, the qualitative or quantitative aspects of the signaling generated by stimulation of any of the individual receptor units in the heteromer are different from those obtained during co-activation, they thus exhibit “cross-talk” (Ferré et al., 2007). A similar heteromerization obviously can also occur between different types of ligand-gated ion channels and ligand-gated ion channels and G-protein coupled receptors. These interactions allow cross-talk between different signaling pathways, but to date, their molecular nature and functional implications are poorly understood. Here we provide an overview on the current knowledge on the interactions of GABAAR with other ligand-gated ion channels or metabotropic G-protein coupled receptors at the post-synaptic membrane.
GABAARs have been clearly demonstrated to heteromerize with GABABRs (Balasubramanian et al., 2004), dopamine D5 receptors (Liu et al., 2000), and purinergic P2XRs (Jo et al., 2011; Shrivastava et al., 2011). All these receptors have also been demonstrated to cross-talk with GABAARs, resulting in a decrease in GABAergic neurotransmission. As other members of the dopamine receptor family are also known to cross-talk with GABAARs, other dopamine receptors (D1–D4) may also heteromerize with GABAARs. Additional experiments will have to be performed to investigate this possibility. Although there is strong evidence of a functional interaction and co-localization of GABAARs and GlyRs, a direct coupling between them so far has not been demonstrated. In cross-talk studies involving serotonin 5-HT3Rs, the authors proposed a very close proximity with GABAARs, as they observed current occlusion even in the absence of Ca2+or protein kinase inhibitor. As the cross-talk reported was similar to the one described for GABAA/P2X receptors, a possible direct coupling remains a challenging issue to be addressed. Among other receptors known to cross-talk with GABAARs are nAChRs and adenosine receptors. However, so far evidence is too weak to speculate on a direct interaction of these receptors with GABAARs. Lastly, NMDAR mediated down-regulation of GABAARs and thus disinhibition of the respective neuron possibly involves only intracellular signaling mechanisms.
Whereas heteromerization of GABAAR with other receptors in most cases has been demonstrated at synapses, such interactions may also occur at extrasynaptic sites, as observed for GABAA/P2X2 receptor interactions regulating tonic inhibition. In addition, it was demonstrated that these receptors already interact with each other in intracellular compartments, possibly providing a mechanism of specifically targeting the heteromer to extrasynaptic sites. A similar intracellular interaction followed by co-trafficking to the cell membrane has also been observed for GABAAR and GABABRs (Balasubramanian et al., 2004). Similar studies have not been performed so far with other receptors that heteromerize with GABAARs.
From most of these studies it is evident that a direct or indirect interaction of GABAAR with other receptors resulted in a decrease in GABAergic neurotransmission and in several cases, also in a decreased function of the interacting receptor. Receptor heteromers thus integrate signals from two different neurotransmitter systems, enabling the one to control the effects of the other on neurotransmission (Ferré et al., 2007). So far, no evidence exists for a change in the pharmacological properties of individual receptors of a directly interacting receptor heteromer. But changes in pharmacology elicited by directly interacting proteins are quite plausible (Olsen and Sieghart, 2009; Zhang et al., 2010). Novel drugs addressing the changed pharmacology of these receptor heteromers might thus be developed that could exhibit quite selective properties. In addition, development of drugs interfering with receptor–receptor interactions might be another strategy for modulating not only the function of GABAARs, but also that of associated receptors. Overall, it might well be that cross-talk between receptors is a general phenomenon in the nervous system that provides an additional complexity in the regulation of the post-synaptic response. Further studies will have to clarify the mechanism involved and the possible importance of such receptor heteromerizations in health and disease.
Conflict of Interest Statement
The authors declare that the research was conducted in the absence of any commercial or financial relationships that could be construed as a potential conflict of interest.
Acknowledgments
Financial support was provided by the International PhD program “Cell Communication in Health and Disease” of the Medical University of Vienna and the Austrian Science Fund. Additional support was provided by INSERM (U1024) and Institut de Biologie de l’Ecole Normale Supérieure, Paris.
References
Abbracchio, M. P., Burnstock, G., Verkhratsky, A., and Zimmermann, H. (2009). Purinergic signalling in the nervous system: an overview. Trends Neurosci. 32, 19–29.
Alkondon, M., Pereira, E. F., Barbosa, C. T., and Albuquerque, E. X. (1997). Neuronal nicotinic acetylcholine receptor activation modulates gamma-aminobutyric acid release from CA1 neurons of rat hippocampal slices. J. Pharmacol. Exp. Ther. 283, 1396–1411.
Alkondon, M., Pereira, E. F., Eisenberg, H. M., and Albuquerque, E. X. (1999). Choline and selective antagonists identify two subtypes of nicotinic acetylcholine receptors that modulate GABA release from CA1 interneurons in rat hippocampal slices. J. Neurosci. 19, 2693–2705.
Balasubramanian, S., Teissére, J. A., Raju, D. V., and Hall, R. A. (2004). Hetero-oligomerization between GABAA and GABAB receptors regulates GABAB receptor trafficking. J. Biol. Chem. 279, 18840–18850.
Bannai, H., Lévi, S., Schweizer, C., Inoue, T., Launey, T., Racine, V., Sibarita, J. B., Mikoshiba, K., and Triller, A. (2009). Activity-dependent tuning of inhibitory neurotransmission based on GABAAR diffusion dynamics. Neuron 62, 670–682.
Barreiro-Iglesias, A., Cornide-Petronio, M. E., Anadón, R., and Rodicio, M. C. (2009a). Serotonin and GABA are colocalized in restricted groups of neurons in the larval sea lamprey brain: insights into the early evolution of neurotransmitter colocalization in vertebrates. J. Anat. 215, 435–443.
Barreiro-Iglesias, A., Villar-Cerviño, V., Anadón, R., and Rodicio, M. C. (2009b). Dopamine and gamma-aminobutyric acid are colocalized in restricted groups of neurons in the sea lamprey brain: insights into the early evolution of neurotransmitter colocalization in vertebrates. J. Anat. 215, 601–610.
Bohlhalter, S., Mohler, H., and Fritschy, J. M. (1994). Inhibitory neurotransmission in rat spinal cord: co-localization of glycine- and GABAA-receptors at GABAergic synaptic contacts demonstrated by triple immunofluorescence staining. Brain Res. 642, 59–69.
Boué-Grabot, E., Emerit, M. B., Toulmé, E., Séguéla, P., and Garret, M. (2004a). Cross-talk and co-trafficking between rho1/GABA receptors and ATP-gated channels. J. Biol. Chem. 279, 6967–6975.
Boué-Grabot, E., Toulmé, E., Emerit, M. B., and Garret, M. (2004b). Subunit-specific coupling between gamma-aminobutyric acid type A and P2X2 receptor channels. J. Biol. Chem. 279, 52517–52525.
Boulland, J. L., Qureshi, T., Seal, R. P., Rafiki, A., Gundersen, V., Bergersen, L. H., Fremeau, R. T. Jr., Edwards, R. H., Storm-Mathisen, J., and Chaudhry, F. A. (2004). Expression of the vesicular glutamate transporters during development indicates the widespread corelease of multiple neurotransmitters. J. Comp. Neurol. 480, 264–280.
Braun, M., Wendt, A., Karanauskaite, J., Galvanovskis, J., Clark, A., MacDonald, P. E., and Rorsman, P. (2007). Corelease and differential exit via the fusion pore of GABA, serotonin, and ATP from LDCV in rat pancreatic beta cells. J. Gen. Physiol. 129, 221–231.
Brünig, I., Sommer, M., Hatt, H., and Bormann, J. (1999). Dopamine receptor subtypes modulate olfactory bulb gamma-aminobutyric acid type A receptors. Proc. Natl. Acad. Sci. U.S.A. 96, 2456–2460.
Buhler, A. V., and Dunwiddie, T. V. (2002). Alpha7 nicotinic acetylcholine receptors on GABAergic interneurons evoke dendritic and somatic inhibition of hippocampal neurons. J. Neurophysiol. 87, 548–557.
Burnstock, G. (2006). Historical review: ATP as a neurotransmitter. Trends Pharmacol. Sci. 27, 166–176.
Chameau, P., and van Hooft, J. A. (2006). Serotonin 5-HT(3) receptors in the central nervous system. Cell Tissue Res. 326, 573–581.
Chen, G., Kittler, J. T., Moss, S. J., and Yan, Z. (2006). Dopamine D3 receptors regulate GABAA receptor function through a phospho-dependent endocytosis mechanism in nucleus accumbens. J. Neurosci. 26, 2513–2521.
Chen, Q. X., and Wong, R. K. (1995). Suppression of GABAA receptor responses by NMDA application in hippocampal neurones acutely isolated from the adult guinea-pig. J. Physiol. (Lond.) 482, 353–362.
Cobb, S. R., Buhl, E. H., Halasy, K., Paulsen, O., and Somogyi, P. (1995). Synchronization of neuronal activity in hippocampus by individual GABAergic interneurons. Nature 378, 75–78.
Crestani, F., Keist, R., Fritschy, J.-M., Benke, D., Vogt, K., Prut, L., Blüthmann, H., Möhler, H., and Rudolph, U. (2002). Trace fear conditioning involves hippocampal alpha5 GABA(A) receptors. Proc. Natl. Acad. Sci. U.S.A. 99, 8980–8985.
Davies, C. H., Starkey, S. J., Pozza, M. F., and Collingridge, G. L. (1991). GABA autoreceptors regulate the induction of LTP. Nature 349, 609–611.
De Saint Jan, D., David-Watine, B., Korn, H., and Bregestovski, P. (2001). Activation of human alpha1 and alpha2 homomeric glycine receptors by taurine and GABA. J. Physiol. (Lond.) 535, 741–555.
Decker, D. A., and Galligan, J. J. (2009). Cross-inhibition between nicotinic acetylcholine receptors and P2X receptors in myenteric neurons and HEK-293 cells. Am. J. Physiol. Gastrointest. Liver Physiol. 296, 1267–1276.
Deisz, R. A., and Prince, D. A. (1989). Frequency-dependent depression of inhibition in guinea-pig neocortex in vitro by GABAB receptor feed-back on GABA release. J. Physiol. (Lond.) 412, 513–541.
Dingledine, R., Borges, K., Bowie, D., and Traynelis, S. F. (1999). The glutamate receptor ion channels. Pharmacol. Rev. 51, 7–61.
Docherty, M., Bradford, H. F., and Wu, J. Y. (1987). Co-release of glutamate and aspartate from cholinergic and GABAergic synaptosomes. Nature 330, 64–66.
Dorostkar, M. M., and Boehm, S. (2007). Opposite effects of presynaptic 5-HT3 receptor activation on spontaneous and action potential-evoked GABA release at hippocampal synapses. J. Neurochem. 100, 395–405.
Endo, T., Yanagawa, Y., Obata, K., and Isa, T. (2005). Nicotinic acetylcholine receptor subtypes involved in facilitation of GABAergic inhibition in mouse superficial superior colliculus. J. Neurophysiol. 94, 3893–3902.
Fattorini, G., Verderio, C., Melone, M., Giovedí, S., Benfenati, F., Matteoli, M., and Conti, F. (2009). VGLUT1 and VGAT are sorted to the same population of synaptic vesicles in subsets of cortical axon terminals. J. Neurochem. 110, 1538–1546.
Ferré, S., Ciruela, F., Woods, A. S., Lluis, C., and Franco, R. (2007). Functional relevance of neurotransmitter receptor heteromers in the central nervous system. Trends Neurosci. 30, 440–446.
Flores-Hernandez, J., Hernandez, S., Snyder, G. L., Yan, Z., Fienberg, A. A., Moss, S. J., Greengard, P., and Surmeier, D. J. (2000). D(1) dopamine receptor activation reduces GABA(A) receptor currents in neostriatal neurons through a PKA/DARPP-32/PP1 signaling cascade. J. Neurophysiol. 83, 2996–3004.
Fucile, S., Saint Jan, D., de David-Watine, B., Korn, H., and Bregestovski, P. (1999). Comparison of glycine and GABA actions on the zebrafish homomeric glycine receptor. J. Physiol. (Lond.) 517, 369–383.
Fukushima, T., Ohtsubo, T., Tsuda, M., Yanagawa, Y., and Hori, Y. (2009). Facilitatory actions of serotonin type 3 receptors on GABAergic inhibitory synaptic transmission in the spinal superficial dorsal horn. J. Neurophysiol. 102, 1459–1471.
Gall, C. M., Hendry, S. H., Seroogy, K. B., Jones, E. G., and Haycock, J. W. (1987). Evidence for coexistence of GABA and dopamine in neurons of the rat olfactory bulb. J. Comp. Neurol. 266, 307–318.
Gillespie, D. C., Kim, G., and Kandler, K. (2005). Inhibitory synapses in the developing auditory system are glutamatergic. Nat. Neurosci. 8, 332–338.
Girault, J. A., and Greengard, P. (2004). The neurobiology of dopamine signaling. Arch. Neurol. 61, 641–644.
Goffin, D., Ali, A. B., Rampersaud, N., Harkavyi, A., Fuchs, C., Whitton, P. S., Nairn, A. C., and Jovanovic, J. N. (2010). Dopamine-dependent tuning of striatal inhibitory synaptogenesis. J. Neurosci. 30, 2935–2950.
Gourine, A. V., Dale, N., Llaudet, E., Poputnikov, D. M., Spyer, K. M., and Gourine, V. N. (2007). Release of ATP in the central nervous system during systemic inflammation: real-time measurement in the hypothalamus of conscious rabbits. J. Physiol. (Lond.) 585, 305–316.
Hallanger, A. E., Wainer, B. H., and Rye, D. B. (1986). Colocalization of gamma-aminobutyric acid and acetylcholinesterase in rodent cortical neurons. Neuroscience 19, 763–769.
Hoyer, D., Hannon, J. P., and Martin, G. R. (2002). Molecular, pharmacological and functional diversity of 5-HT receptors. Pharmacol. Biochem. Behav. 71, 533–554.
Hu, H. Z., and Li, Z. W. (1997). Modulation by adenosine of GABA-activated current in rat dorsal root ganglion neurons. J. Physiol. (Lond.) 501, 67–75.
Iwasa, K., Oomori, Y., and Tanaka, H. (1999). Colocalization of gamma-aminobutyric acid immunoreactivity and acetylcholinesterase activity in nerve fibers of the mouse adrenal gland. J. Vet. Med. Sci. 61, 631–635.
Ji, D., and Dani, J. A. (2000). Inhibition and disinhibition of pyramidal neurons by activation of nicotinic receptors on hippocampal interneurons. J. Neurophysiol. 83, 2682–2690.
Jo, Y. H., Donier, E., Martinez, A., Garret, M., Toulme, E., and Boue-Grabot, E. (2011). Crosstalk between P2X4 and GABA-A receptors determines synaptic efficacy at central synapses. J. Biol. Chem. 286, 19993–20004.
Jo, Y. H., and Schlichter, R. (1999). Synaptic corelease of ATP and GABA in cultured spinal neurons. Nat. Neurosci. 2, 241–245.
Jo, Y.-H., and Role, L. W. (2002). Coordinate release of ATP and GABA at in vitro synapses of lateral hypothalamic neurons. J. Neurosci. 22, 4794–4804.
Jonas, P., Bischofberger, J., and Sandkühler, J. (1998). Corelease of two fast neurotransmitters at a central synapse. Science 281, 419–424.
Kang, T. C., An, S. J., Park, S. K., Hwang, I. K., and Won, M. H. (2003). P2X2 and P2X4 receptor expression is regulated by a GABA(A) receptor-mediated mechanism in the gerbil hippocampus. Brain Res. Mol. Brain Res. 116, 168–175.
Karanjia, R., García-Hernández, L. M., Miranda-Morales, M., Somani, N., Espinosa-Luna, R., Montaño, L. M., and Barajas-López, C. (2006). Cross-inhibitory interactions between GABAA and P2X channels in myenteric neurones. Eur. J. Neurosci. 23, 3259–3268.
Kardos, J., Elster, L., Damgaard, I., Krogsgaard-Larsen, P., and Schousboe, A. (1994). Role of GABAB receptors in intracellular Ca2+ homeostasis and possible interaction between GABAA and GABAB receptors in regulation of transmitter release in cerebellar granule neurons. J. Neurosci. Res. 39, 646–655.
Kasugai, Y., Swinny, J. D., Roberts, J. D. B., Dalezios, Y., Fukazawa, Y., Sieghart, W., Shigemoto, R., and Somogyi, P. (2010). Quantitative localisation of synaptic and extrasynaptic GABA(A) receptor subunits on hippocampal pyramidal cells by freeze-fracture replica immunolabelling. Eur. J. Neurosci. 32, 1868–1888.
Kawai, H., Zago, W., and Berg, D. K. (2002). Nicotinic alpha 7 receptor clusters on hippocampal GABAergic neurons: regulation by synaptic activity and neurotrophins. J. Neurosci. 22, 7903–7912.
Khakh, B. S., Fisher, J. A., Nashmi, R., Bowser, D. N., and Lester, H. A. (2005). An angstrom scale interaction between plasma membrane ATP-gated P2X2 and alpha4beta2 nicotinic channels measured with fluorescence resonance energy transfer and total internal reflection fluorescence microscopy. J. Neurosci. 25, 6911–6920.
Khakh, B. S., Zhou, X., Sydes, J., Galligan, J. J., and Lester, H. A. (2000). State-dependent cross-inhibition between transmitter-gated cation channels. Nature 406, 405–410.
Kneussel, M. (2010). Extracellular neurotransmitter receptor clustering: think outside the box. J. Mol. Cell Biol. 2, 107–109.
Kneussel, M., Brandstätter, J. H., Laube, B., Stahl, S., Müller, U., and Betz, H. (1999). Loss of postsynaptic GABA(A) receptor clustering in gephyrin-deficient mice. J. Neurosci. 19, 9289–9297.
Labrakakis, C., Tong, C. K., Weissman, T., Torsney, C., and MacDermott, A. B. (2003). Localization and function of ATP and GABAA receptors expressed by nociceptors and other postnatal sensory neurons in rat. J. Physiol. 549, 131–142.
Lau, C. G., and Zukin, R. S. (2007). NMDA receptor trafficking in synaptic plasticity and neuropsychiatric disorders. Nat. Rev. Neurosci. 8, 413–426.
Lee, S., Kim, K., and Zhou, Z. J. (2010). Role of ACh-GABA cotransmission in detecting image motion and motion direction. Neuron 68, 1159–1172.
Lévi, S., Schweizer, C., Bannai, H., Pascual, O., Charrier, C., and Triller, A. (2008). Homeostatic regulation of synaptic GlyR numbers driven by lateral diffusion. Neuron 59, 261–273.
Lewis, C. A., and Faber, D. S. (1993). GABA responses and their partial occlusion by glycine in cultured rat medullary neurons. Neuroscience 52, 83–96.
Li, H., Lang, B., Kang, J. F., and Li, Y. Q. (2000). Serotonin potentiates the response of neurons of the superficial laminae of the rat spinal dorsal horn to gamma-aminobutyric acid. Brain Res. Bull. 52, 559–565.
Li, H., Wu, L., and Li, Y. Q. (2004). Adenosine suppresses GABAA receptor-mediated responses in rat sacral dorsal commissural neurons. Auton. Neurosci. 111, 71–79.
Li, Y., Wu, L.-J., Legendre, P., and Xu, T. L. (2003). Asymmetric cross-inhibition between GABAA and glycine receptors in rat spinal dorsal horn neurons. J. Biol. Chem. 278, 38637–38645.
Liu, F., Wan, Q., Pristupa, Z. B., Yu, X. M., Wang, Y. T., and Niznik, H. B. (2000). Direct protein-protein coupling enables cross-talk between dopamine D5 and gamma-aminobutyric acid A receptors. Nature 403, 274–280.
Lu, T., Rubio, M. E., and Trussell, L. O. (2008). Glycinergic transmission shaped by the corelease of GABA in a mammalian auditory synapse. Neuron 57, 524–535.
Maher, B. J., and Westbrook, G. L. (2008). Co-transmission of dopamine and GABA in periglomerular cells. J. Neurophysiol. 99, 1559–1564.
Marsden, K. C., Beattie, J. B., Friedenthal, J., and Carroll, R. C. (2007). NMDA receptor activation potentiates inhibitory transmission through GABA receptor-associated protein-dependent exocytosis of GABA(A) receptors. J. Neurosci. 27, 14326–14337.
Miranda-Morales, M., García-Hernández, L. M., Ochoa-Cortés, F., Espinosa-Luna, R., Naranjo-Rodríguez, E. B., and Barajas-López, C. (2007). Cross-talking between 5-HT3 and GABAA receptors in cultured myenteric neurons. Synapse 61, 732–740.
Moss, S. J., and Smart, T. G. (2001). Constructing inhibitory synapses. Nat. Rev. Neurosci. 2, 240–250.
Mott, D. D., and Lewis, D. V. (1991). Facilitation of the induction of long-term potentiation by GABAB receptors. Science 252, 1718–1720.
Muir, J., Arancibia-Carcamo, I. L., Macaskill, A. F., Smith, K. R., Griffin, L. D., and Kittler, J. T. (2010). NMDA receptors regulate GABAA receptor lateral mobility and clustering at inhibitory synapses through serine 327 on the gamma2 subunit. Proc. Natl. Acad. Sci. U.S.A. 107, 11679–11684.
Noh, J., Seal, R. P., Garver, J. A., Edwards, R. H., and Kandler, K. (2010). Glutamate co-release at GABA/glycinergic synapses is crucial for the refinement of an inhibitory map. Nat. Neurosci. 13, 232–238.
Nusser, Z., Sieghart, W., Stephenson, F. A., and Somogyi, P. (1996). The alpha 6 subunit of the GABAA receptor is concentrated in both inhibitory and excitatory synapses on cerebellar granule cells. J. Neurosci. 16, 103–114.
O’Brien, J. A., and Berger, A. J. (1999). Cotransmission of GABA and glycine to brain stem motoneurons. J. Neurophysiol. 82, 1638–1641.
Obrietan, K., and van den Pol, A. N. (1998). GABAB receptor-mediated inhibition of GABAA receptor calcium elevations in developing hypothalamic neurons. J. Neurophysiol. 79, 1360–1370.
Olsen, R. W., and Sieghart, W. (2009). GABA A receptors: subtypes provide diversity of function and pharmacology. Neuropharmacology 56, 141–148.
O’Malley, D. M., and Masland, R. H. (1989). Co-release of acetylcholine and gamma-aminobutyric acid by a retinal neuron. Proc. Natl. Acad. Sci. U.S.A. 86, 3414–3418.
O’Malley, D. M., Sandell, J. H., and Masland, R. H. (1992). Co-release of acetylcholine and GABA by the starburst amacrine cells. J. Neurosci. 12, 1394–1408.
Pérez de la Mora, M., Ferré, S., and Fuxe, K. (1997). GABA-dopamine receptor-receptor interactions in neostriatal membranes of the rat. Neurochem. Res. 22, 1051–1054.
Pinard, A., Seddik, R., and Bettler, B. (2010). GABAB Receptors: physiological functions and mechanisms of diversity. Adv. Pharmacol. 58, 231–255.
Renner, M., Specht, C. G., and Triller, A. (2008). Molecular dynamics of postsynaptic receptors and scaffold proteins. Curr. Opin. Neurobiol. 18, 532–540.
Robello, M., Amico, C., and Cupello, A. (1997). A dual mechanism for impairment of GABAA receptor activity by NMDA receptor activation in rat cerebellum granule cells. Eur. Biophys. J. 25, 181–187.
Roseti, C., Martinello, K., Fucile, S., Piccari, V., Mascia, A., Di Gennaro, G., Quarato, P. P., Manfredi, M., Esposito, V., Cantore, G., Arcella, A., Simonato, M., Fredholm, B. B., Limatola, C., Miledi, R., and Eusebi, F. (2008). Adenosine receptor antagonists alter the stability of human epileptic GABA A receptors. Proc. Natl. Acad. Sci. U.S.A. 105, 15118–15123.
Roseti, C., Palma, E., Martinello, K., Fucile, S., Morace, R., Esposito, V., Cantore, G., Arcella, A., Giangaspero, F., Aronica, E., Mascia, A., Di Gennaro, G., Quarato, P. P., Manfredi, M., Cristalli, G., Lambertucci, C., Marucci, G., Volpini, R., Limatola, C., and Eusebi, F. (2009). Blockage of A2A and A3 adenosine receptors decreases the desensitization of human GABA(A) receptors microtransplanted to Xenopus oocytes. Proc. Natl. Acad. Sci. U.S.A. 106, 15927–15931.
Russier, M., Kopysova, I. L., Ankri, N., Ferrand, N., and Debanne, D. (2002). GABA and glycine co-release optimizes functional inhibition in rat brainstem motoneurons in vitro. J. Physiol. (Lond.) 541, 123–137.
Santos, P. F., Carvalho, A. L., Carvalho, A. P., and Duarte, C. B. (1998). Differential acetylcholine and GABA release from cultured chick retina cells. Eur. J. Neurosci. 10, 2723–2730.
Schousboe, A. (1999). Pharmacologic and therapeutic aspects of the developmentally regulated expression of GABA(A) and GABA(B) receptors: cerebellar granule cells as a model system. Neurochem. Int. 34, 373–377.
Schwenk, J., Metz, M., Zolles, G., Turecek, R., Fritzius, T., Bildl, W., Tarusawa, E., Kulik, A., Unger, A., Ivankova, K., Seddik, R., Tiao, J. Y., Rajalu, M., Trojanova, J., Rohde, V., Gassmann, M., Schulte, U., Fakler, B., and Bettler, B. (2010). Native GABA(B) receptors are heteromultimers with a family of auxiliary subunits. Nature 465, 231–235.
Segal, M., and Barker, J. L. (1984). Rat hippocampal neurons in culture: voltage-clamp analysis of inhibitory synaptic connections. J. Neurophysiol. 52, 469–487.
Shen, H., Sabaliauskas, N., Sherpa, A., Fenton, A. A., Stelzer, A., Aoki, C., and Smith, S. S. (2010). A critical role for alpha4betadelta GABAA receptors in shaping learning deficits at puberty in mice. Science 327, 1515–1518.
Shin, R. M., Masuda, M., Miura, M., Sano, H., Shirasawa, T., Song, W. J., Kobayashi, K., and Aosaki, T. (2003). Dopamine D4 receptor-induced postsynaptic inhibition of GABAergic currents in mouse globus pallidus neurons. J. Neurosci. 23, 11662–11672.
Shrivastava, A. N., Triller, A., Sieghart, W., and Sarto-Jackson, I. (2011). Regulation of gamma-aminobutyric acid a (GABAA) receptor dynamics by interaction with purinergic P2X2 receptors. J. Biol. Chem. 286, 14455–14468.
Sieghart, W. (1995). Structure and pharmacology of gamma-aminobutyric acidA receptor subtypes. Pharmacol. Rev. 47, 181–234.
Silva, A. J., Stevens, C. F., Tonegawa, S., and Wang, Y. (1992). Deficient hippocampal long-term potentiation in alpha-calcium-calmodulin kinase II mutant mice. Science 257, 201–206.
Singer, J. H. (2008). GABA is an endogenous ligand for synaptic glycine receptors. Neuron 57, 475–477.
Smith, Y., Charara, A., Paquet, M., Kieval, J. Z., Paré, J. F., Hanson, J. E., Hubert, G. W., Kuwajima, M., and Levey, A. I. (2001). Ionotropic and metabotropic GABA and glutamate receptors in primate basal ganglia. J. Chem. Neuroanat. 22, 13–42.
Sokolova, E., Nistri, A., and Giniatullin, R. (2001). Negative cross talk between anionic GABA A and cationic P2X ionotropic receptors of rat dorsal root ganglion neurons. J. Neurosci. 21, 4958–4968.
Staley, K. J., Soldo, B. L., and Proctor, W. R. (1995). Ionic mechanisms of neuronal excitation by inhibitory GABAA receptors. Science 269, 977–981.
Stelzer, A., and Shi, H. (1994). Impairment of GABAA receptor function by N-methyl-D-aspartate-mediated calcium influx in isolated CA1 pyramidal cells. Neuroscience 62, 813–828.
Swant, J., Stramiello, M., and Wagner, J. J. (2008). Postsynaptic dopamine D3 receptor modulation of evoked IPSCs via GABA(A) receptor endocytosis in rat hippocampus. Hippocampus 18, 492–502.
Szabadits, E., Cserép, C., Szonyi, A., Fukazawa, Y., Shigemoto, R., Watanabe, M., Itohara, S., Freund, T. F., and Nyiri, G. (2011). NMDA Receptors in hippocampal GABAergic synapses and their role in nitric oxide signaling. J. Neurosci. 31, 5893–5904.
Todd, A. J., Watt, C., Spike, R. C., and Sieghart, W. (1996). Colocalization of GABA, glycine, and their receptors at synapses in the rat spinal cord. J. Neurosci. 16, 974–982.
Toulmé, E., Blais, D., Léger, C., Landry, M., Garret, M., Séguéla, P., and Boué-Grabot, E. (2007). An intracellular motif of P2X(3) receptors is required for functional cross-talk with GABA(A) receptors in nociceptive DRG neurons. J. Neurochem. 102, 1357–1368.
Tretter, V., Jacob, T. C., Mukherjee, J., Fritschy, J. M., Pangalos, M. N., and Moss, S. J. (2008). The clustering of GABA(A) receptor subtypes at inhibitory synapses is facilitated via the direct binding of receptor alpha 2 subunits to gephyrin. J. Neurosci. 28, 1356–1365.
Triller, A., Cluzeaud, F., and Korn, H. (1987). gamma-Aminobutyric acid-containing terminals can be apposed to glycine receptors at central synapses. J. Cell Biol. 104, 947–956.
Trombley, P. Q., Hill, B. J., and Horning, M. S. (1999). Interactions between GABA and glycine at inhibitory amino acid receptors on rat olfactory bulb neurons. J. Neurophysiol. 82, 3417–3422.
Waldvogel, H. J., Billinton, A., White, J. H., Emson, P. C., and Faull, R. L. M. (2004). Comparative cellular distribution of GABAA and GABAB receptors in the human basal ganglia: immunohistochemical colocalization of the alpha 1 subunit of the GABAA receptor, and the GABABR1 and GABABR2 receptor subunits. J. Comp. Neurol. 470, 339–356.
Wanaverbecq, N., Semyanov, A., Pavlov, I., Walker, M. C., and Kullmann, D. M. (2007). Cholinergic axons modulate GABAergic signaling among hippocampal interneurons via postsynaptic alpha 7 nicotinic receptors. J. Neurosci. 27, 5683–5693.
Wang, J., Liu, S., Haditsch, U., Tu, W., Cochrane, K., Ahmadian, G., Tran, L., Paw, J., Wang, Y., Mansuy, I., Salter, M. M., and Lu, Y. M. (2003). Interaction of calcineurin and type-A GABA receptor gamma 2 subunits produces long-term depression at CA1 inhibitory synapses. J. Neurosci. 23, 826–836.
Wang, X., Zhong, P., and Yan, Z. (2002). Dopamine D4 receptors modulate GABAergic signaling in pyramidal neurons of prefrontal cortex. J. Neurosci. 22, 9185–9193.
Xu, T. L., Pang, Z. P., Li, J. S., and Akaike, N. (1998). 5-HT potentiation of the GABA(A) response in the rat sacral dorsal commissural neurones. Br. J. Pharmacol. 124, 779–787.
Yang, K., Buhlman, L., Khan, G. M., Nichols, R. A., Jin, G., McIntosh, J. M., Whiteaker, P., Lukas, R. J., and Wu, J. (2011). Functional nicotinic acetylcholine receptors containing 6 subunits are on GABAergic neuronal boutons adherent to ventral tegmental area dopamine neurons. J. Neurosci. 31, 2537–2548.
Zago, W. M., Massey, K. A., and Berg, D. K. (2006). Nicotinic activity stabilizes convergence of nicotinic and GABAergic synapses on filopodia of hippocampal interneurons. Mol. Cell. Neurosci. 31, 549–559.
Zander, J. F., Münster-Wandowski, A., Brunk, I., Pahner, I., Gómez-Lira, G., Heinemann, U., Gutiérrez, R., Laube, G., and Ahnert-Hilger, G. (2010). Synaptic and vesicular coexistence of VGLUT and VGAT in selected excitatory and inhibitory synapses. J. Neurosci. 30, 7634–7645.
Zeilhofer, H. U., Möhler, H., and Di Lio, A. (2009). GABAergic analgesia: new insights from mutant mice and subtype-selective agonists. Trends Pharmacol. Sci. 30, 397–402.
Zhang, C., Atasoy, D., Arac, D., Yang, X., Fucillo, M. V., Robinson, A. J., Ko, J., Brunger, A. T., and Sudof, T. C. (2010). Neurexins physically and functionally interact with GABA(A) receptors. Neuron 66, 403–416.
Zhang, J., and Berg, D. K. (2007). Reversible inhibition of GABAA receptors by alpha7-containing nicotinic receptors on the vertebrate postsynaptic neurons. J. Physiol. (Lond.) 579, 753–763.
Keywords: GABAAR, cross-talk, ionotropic receptors, metabotropic receptors, targeting
Citation: Shrivastava AN, Triller A and Sieghart W (2011) GABAA receptors: post-synaptic co-localization and cross-talk with other receptors. Front. Cell. Neurosci. 5:7. doi: 10.3389/fncel.2011.00007
Received: 14 April 2011;
Paper pending published: 23 April 2011;
Accepted: 06 June 2011;
Published online: 22 June 2011.
Edited by:
Enrico Cherubini, International School for Advanced Studies, ItalyReviewed by:
Stefano Vicini, Georgetown University School of Medicine, USAAndrea Nistri, Scuola Internazionale Superiore di Studi Avanzati, Italy
Copyright: © 2011 Shrivastava, Triller and Sieghart. This is an open-access article subject to a non-exclusive license between the authors and Frontiers Media SA, which permits use, distribution and reproduction in other forums, provided the original authors and source are credited and other Frontiers conditions are complied with.
*Correspondence: Werner Sieghart, Department of Biochemistry and Molecular Biology of the Nervous System, Center for Brain Research, Medical University of Vienna, Spitalgasse 4, A-1090 Vienna, Austria. e-mail:d2VybmVyLnNpZWdoYXJ0QG1lZHVuaXdpZW4uYWMuYXQ=