- 1 INSERM U643, Nantes, France
- 2 Institut de Transplantation et de Recherche en Transplantation, Centre Hospitalier Universitaire de Nantes, Nantes, France
- 3 Faculté de Médecine, Université de Nantes, Nantes, France
Neural transplantation is a promising therapeutic strategy for neurodegenerative diseases and other disorders of the central nervous system (CNS) such as Parkinson and Huntington diseases, multiple sclerosis or stroke. Although cell replacement therapy already went through clinical trials for some of these diseases using fetal human neuroblasts, several significant limitations led to the search for alternative cell sources that would be more suitable for intracerebral transplantation.Taking into account logistical and ethical issues linked to the use of tissue derived from human fetuses, and the immunologically special status of the CNS allowing the occurrence of deleterious immune reactions, neural stem/progenitor cells (NSPCs) appear to be an interesting cell source candidate. In addition to their ability for replacing cell populations lost during the pathological events, NSPCs also display surprising therapeutic effects of neuroprotection and immunomodulation. A better knowledge of the mechanisms involved in these specific characteristics will hopefully lead in the future to a successful use of NSPCs in regenerative medicine for CNS disorders.
The Central Nervous System, an Organ with an “Immunologically Special” Status
Inflammation is the primary response of the immune system that occurs to defend the organism against danger signals provoked by an infection or irritation consecutive to the intrusion of pathogens. The specific property of the immune system is to discriminate the self from the non-self and thus to identify and eliminate the infectious agents. From this same mechanism originates the phenomenon of rejection in transplantation. However, since Van Dooremal work in 1873 on tumor cell graft in the eye anterior chamber, it is known that specific sites in the organism display limited immune reactions. In 1948, Sir Peter Medawar, Nobel Prize of Medicine and pioneer in the field of the immunology of transplantation, proposed the term of “immune privilege” to describe a reduced inflammation after inoculation of allogeneic tissues in some organs like the brain or the eye anterior chamber (Medawar, 1948). Later, this definition was extended to the particular property of specific organs or tissues to display a prolonged, and sometimes infinite, survival when grafted in conventional sites of the organism. Thus, throughout the years, the cornea, the placenta, the eye anterior chamber (Billingham and Boswell, 1953; Hori et al., 2000), or the testis (Head et al., 1983) were examples of well-studied immune-privileged tissues. In this context, the central nervous system (CNS) and the immune system were traditionally perceived as separate morphological and functional entities, preventing the disturbance of the CNS homeostasis which is crucial to neuronal functioning. This vision that the CNS could escape the immune surveillance was supported by the discovery of the blood–brain barrier (BBB) preventing the exchange between a wide range of soluble molecules from the blood and the brain, like growth factors, cytokines, or immunoglobulins (Goldstein and Betz, 1983; Joó, 1993). In addition, there is no proof of the existence of professional antigen presenting cells like dendritic cells, B cells, and macrophages in the unlesioned CNS (Wekerle et al., 1987) preventing the initiation and propagation of antigen-specific immune responses in the brain. In physiological conditions, the expression of antigens from the major histocompatibility complex (MHC) by neural cells is very weak and even in some cases undetectable (Barker and Billingham, 1977; Mauerhoff et al., 1988) allowing these cells to escape the recognition by antigen-specific T cells. However, only the normal CNS displays such an absence of immune response.
During certain pathological processes, specific genes are activated leading to the change of this immunologically non-reactive tissue into an environment favorable to the development of inflammatory reactions. In these conditions, macrophages are recruited from the pool of blood monocytes and infiltrate the perivascular spaces. In addition, microglial cells from the CNS are activated and acquire phagocytosis and antigen presentation abilities (Aloisi, 2001). Microglia can induce the production of pro-inflammatory cytokines like TNF and IL-1β (Sivakumar et al., 2011) and, along with reactive astrocytes, become able to present antigens through class I and II MHC molecules (Höftberger et al., 2004), allowing CNS-infiltrated T cells to recognize the antigenic peptides and behave as potent immune effectors (Cornet et al., 2000). The last two decades have thus witnessed the questioning of the concept of immune privilege. Discovery of the permeability of the BBB under pathological circumstances (Kebir et al., 2007), the existence of an unconventional lymphatic drainage in the CNS (Hatterer et al., 2006), and the migration of leucocytes across the BBB on a regular basis (Hickey et al., 1991; Cayrol et al., 2008) were convincing proofs of the strong bidirectional communication between the immune and the nervous systems. The brain is now more readily considered as an organ with special immune characteristics and subject to immunological surveillance than a strictly immune-privileged tissue (Hickey, 2001). This dual status of the CNS, being both more favorable to the transplantation than the periphery but also a propitious environment for deleterious inflammatory reactions in response to pathological conditions (Kerschensteiner et al., 2009) could in part explain why fetal neuron allografts in the brain are usually well tolerated under moderate immunosuppressive treatment (Björklund and Lindvall, 2000; Bachoud-Lévi et al., 2006; Krystkowiak et al., 2007) while intracerebral xenografts are systematically rejected (Larsson et al., 2000).
Cell Replacement Therapy for the CNS
Neuronal cell death is the main characteristic of CNS acute disorders like stroke or trauma but also of neurodegenerative diseases including Alzheimer, Parkinson, and Huntington diseases. As the adult CNS displays very weak capacities of endogenous cell replacement and repair, both being necessary to attain a significant functional recovery, cell replacement therapy for the CNS represents a promising avenue for the treatment of these pathological conditions. In fact, clinical impact of cell replacement strategy has already been assessed in Parkinson disease patients by allotransplantation of fetal mesencephalic cells. The results of the clinical trials showed, in some patients, a long-term symptomatic improvement associated with a survival of the grafted cells and a partial reinnervation of the striatum (Björklund and Lindvall, 2000). A functional recovery has also been noticed in a small cohort of patients suffering from Huntington disease and grafted with human fetal striatal neuroblasts (Bachoud-Lévi et al., 2000, 2006), with one study even showing integration of cells from the donor into the host tissue (Freeman et al., 2000). Despite these promising outcomes, several issues remain to be assessed (Björklund et al., 2003). Tissue availability, ethical and logistical concerns linked to the use of human material, cell viability and purity, or appearance of deleterious side-effects like dyskinesia (Olanow et al., 2003) represent important obstacles that need to be overcome before resuming clinical trials on neural allotransplantation. Moreover, the discovery of biological signs of alloimmunization to donor’s antigens like the appearance of anti-HLA antibodies in Huntington disease patients following fetal neural grafts (Krystkowiak et al., 2007) underlined the importance of considering immune responses in the CNS as a crucial parameter for future cell transplantation strategies. In order to at least circumvent some of the problems mentioned above, alternative cell sources for intracerebral transplantation like porcine neuroblasts have been considered (Pakzaban and Isacson, 1994).
Intracerebral Xenotransplantation
Porcine fetal neural tissue has been for a long time considered as the more adequate cell source for xenotransplantation in the human brain. Indeed, swine offer the advantage of a relatively easy breeding allowing an excellent access to fetal material and can be genetically modified. In fact, porcine fetal cells have been proven to be an efficient source for cell therapy in animal models of neurodegenerative diseases. Interest in these cells started to grow after first studies revealed that xenografts derived from the ventral mesencephalon of pigs (21–26 days of gestation) could survive in the brain of a rat model of Parkinson disease under immunosuppression (Freeman et al., 1988; Huffaker et al., 1989). Beyond the long-term survival, xenotransplanted neural cells also displayed a long-distance axonal outgrowth (Wictorin et al., 1990; Deacon et al., 1994). Isacson et al. (1995) have shown that neurons isolated from several areas of the porcine fetal brain and transplanted in homotypic or ectopic lesioned cerebral regions of an adult rat could project axons in the deafferented zones, thus rebuilding the brain parenchyma cytoarchitecture. This inter-species and targeted axonal growth of porcine dopaminergic neuroblasts significantly restored in several months an efficient dopaminergic innervation. A significant functional recovery in transplanted rats was also observed and was correlated to the number of tyrosine hydroxylase (TH)-positive cells and to the size of the graft (Galpern et al., 1996). Encouraging results obtained from animal models of Parkinson disease led to small-scale clinical trials. Isacson’s group performed a study where 12 Parkinson disease patients received a unilateral striatal graft constituted of a porcine ventral mesencephalon (25–28 days of gestation) cell suspension (Schumacher et al., 2000). Even though a significant decrease in Unified Parkinson’s Disease Rating Scale (UPDRS) scores was observed, positron emission tomography (PET) analyses did not show any increase in the [18F]fluorodopa uptake, underlining a lack of improvement in axon termination density of dopaminergic neurons from the graft and no increase in dopa-decarboxylase activity. Moreover, a postmortem study revealed that only 638 TH-positive cells out of the 12 millions of porcine neuroblasts transplanted had survived at that stage, with a lymphocytic infiltration at the border and within the graft, despite the fact that the patient was under cyclosporine A treatment (Deacon et al., 1997). If some work has shown that neural xenografts are able to survive for a long time in the CNS without the use of immunosuppressors (Björklund et al., 1982; Daniloff et al., 1985), most of the recent studies, including those from our group, indicate that intracerebral xenografts trigger a strong immune reaction leading to the fast destruction of the graft through the invasion of microglial cells/macrophages, T lymphocytes, and dendritic cells within 5– 7 weeks post-transplantation (Rémy et al., 2001; Melchior et al., 2002; Michel et al., 2006; Figure 1). Xenograft rejection is more aggressive than that observed with allotransplantation and mainly occurs through the involvement of T cells. A genetically modified swine has been engineered to express CTLA4-Ig, a human molecule inhibiting T cells, under the neuron-specific enolase promoter. This construction allowed porcine transgenic neurons to locally deliver an immunosuppressive molecule after transplantation in the brain (Martin et al., 2005). In the CNS, xenograft survival has been significantly prolonged by administration of immunosuppressive drugs targeting T cells like cyclosporine A. However, this immunosuppressor at doses required to inhibit the rejection process has strong sides effects (Rezzani, 2006) and is only transiently efficient. Graft rejection has also been delayed when the T cell receptor (TCR) and the IL-2 receptor a chain (CD25) were inactivated, and in the case of CD4-positive T cell depletion (Honey et al., 1990; Okura et al., 1997). The administration of two successive high doses of anti-CD4 monoclonal antibodies resulted in a longer survival of discordant xenografts (between species differing from the expression of the gal epitope, like pig and human), although not prolonged, suggesting that other immune components than T cells are implicated (Wood et al., 1996). Indeed, B cells and immunoglobulins have been shown to intervene in the survival and function of the graft. The importance of immunoglobulins has been highlighted by comparing the survival of a cell suspension derived from porcine ventral mesencephalon xenografted in the brain of wild-type (WT) or immunoglobulin-deficient (Ig-KO) mice. Most of the Ig-KO mice displayed for more than 4 weeks a functional graft with very little immune cell infiltration while xenograft survival beyond 4 days remained exceptional in WT mice. After a prolonged survival of about 4 weeks, an immune cellular response with a high proportion of CD8-positive T cells occurred and led to the rejection of the graft in Ig-KO mice (Larsson et al., 1999). These observations indicate that immunoglobulins play an important part in the fast initiation of discordant neural xenografts rejection, a phenomenon that appears to be unavoidable despite all the efforts made to control it. Overall, these pre-clinical and clinical studies led to disappointing results and limited the enthusiasm and hope initially raised by the use of fetal xenogeneic neuroblasts in regenerative medicine. It is now important to find more adequate cell sources for intracerebral transplantation. In theory, the ideal candidate should be tolerogenic and safe and have the properties to be easily amplified, resist to long-term storage, be efficient for cell replacement and allow manipulation to adjust to a specific pathology. Until these days, no cell type possesses all those characteristics and several cells at different stages of the neuronal lineage have been tested in animal models and clinical trials (Guillaume and Zhang, 2008). Among them, neural stem/progenitor cells (NSPCs) seem to represent a good candidate.
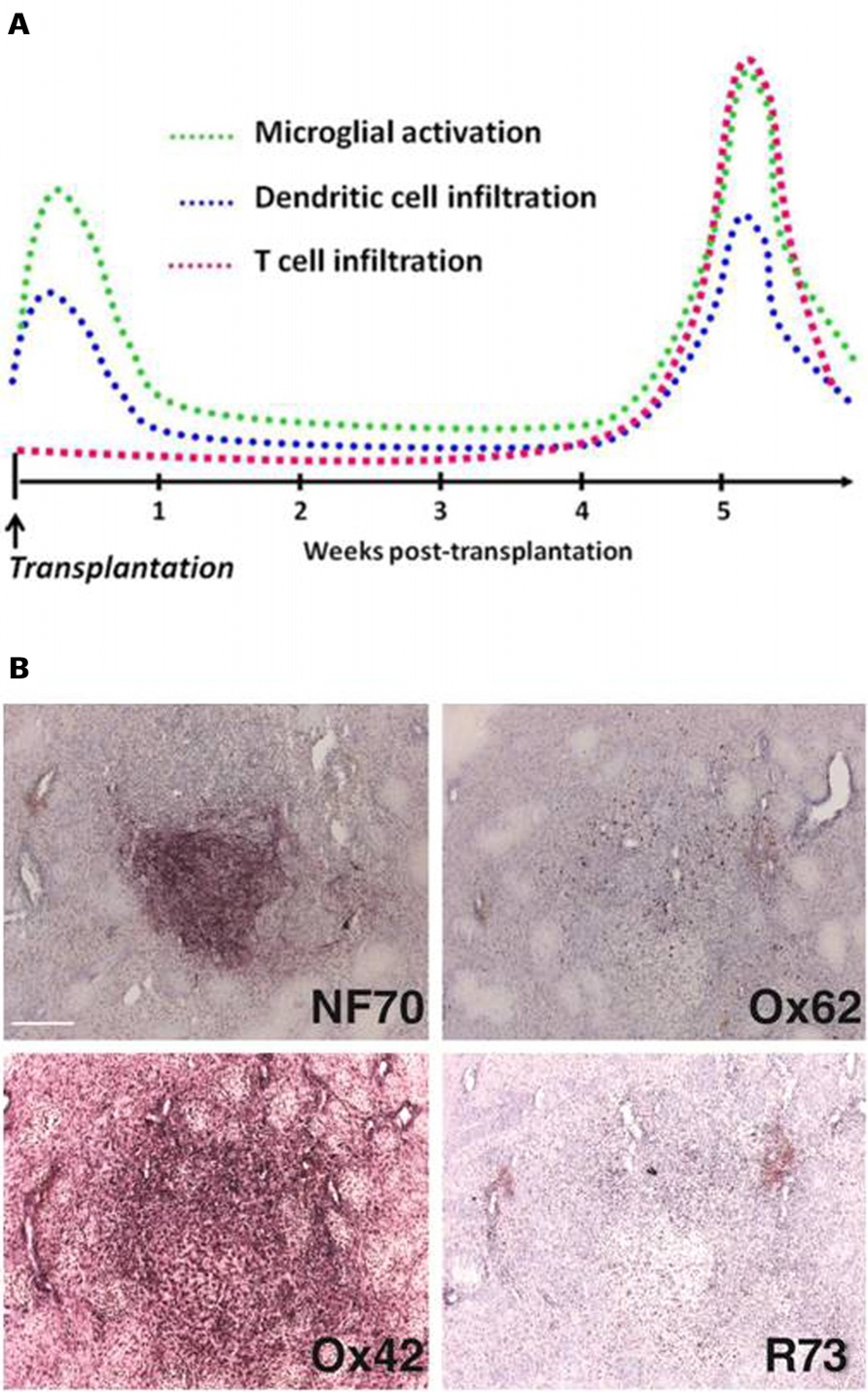
FIGURE 1. Characterization of xenograft infiltration by activated immune cells. Kinetics (A). In the absence of immunosuppressive treatment, the rejection process of fetal neurons xenografted in the rat striatum involves cells from the macrophagic lineage. After a peak of microglial activation and dendritic cell infiltration in the first days post-transplantation, probably consecutive to the surgery, a latency phase is observed. Around 5 weeks post-transplantation, rejection process is initiated and strong microglial cell activation at the border but also within the graft is observed. This phenomenon is strongly correlated with a massiveT cell and dendritic cell infiltration. Immunostaining (B). Specific porcine neurofilament is revealed by NF70 antibody and allows visualization of the graft. Immune reaction is assessed by O×62 (dendritic cells), O×42 (microglial cells/macrophages), and R73 (T cells) antibodies. Scale bar: 200 μm.
NSPCs, the Future of Intracerebral Transplantation?
Stem cells are defined by their ability to self renew to maintain the pool of undifferentiated cells, to proliferate to give rise to lineage-restricted progenitors, and to differentiate into a range of mature cell types. Among them, multipotent neural stem cells (NSCs), derived from embryonic or adult nervous systems, can be cultivated in vitro as neurospheres, a mixture of neural stem cells and progenitors (NSPCs), in presence of growth factors such as bFGF (Vescovi et al., 1993; Gritti et al., 1996), and EGF (Reynolds and Weiss, 1992). Withdrawal of growth factors induces their differentiation into neurons, astrocytes, and oligodendrocytes (Reynolds and Weiss, 1992; Reynolds et al., 1992). These properties make them an interesting source of cells for neural repair after injury or disease. Besides their differentiation potential, NSPCs seem to have a selective advantage for their survival when transplanted in the brain. In a xenotransplantation context, Armstrong and colleagues as well as our group demonstrated that porcine NSPCs were able to survive longer than porcine neuroblasts when grafted into the striatum of non-immunosuppressed rats (Armstrong et al., 2001; Michel-Monigadon et al., 2011). As NSPCs were reported to express no or low levels of MHC molecules (Klassen et al., 2001; Hori et al., 2003), this phenomenon was firstly linked to a lesser immunogenicity of these cells compared to more mature cells (Odeberg et al., 2005). However, immunogenic properties of NSPCs cannot totally justify their delayed rejection, and recent studies confirmed the expression of MHC class I and class II molecules by NSPCs, under normal or inflammatory conditions (Sergent-Tanguy et al., 2006; Johansson et al., 2008; Yin et al., 2008; Laguna Goya et al., 2011). Beneficial effects of NSPC transplantation have been shown in pre-clinical models of several neurologic disorders such as Parkinson disease (Richardson et al., 2005), Huntington disease (McBride et al., 2004), or multiple sclerosis but also in other pathologies including renal ischemia-reperfusion (Wang et al., 2009b). However, the different mechanisms by which these cells exert their therapeutic effect remain unclear. The replacement of cells that have been lost or damaged was for a long time thought to be the main function of transplanted stem cells but it is now clear that somatic stem cells could also induce several beneficial effects far beyond the cell replacement itself. For instance, it has recently been demonstrated that several stem cell types (embryonic, mesenchymal, or neural) display a strong immunosuppressive potential (Fändrich et al., 2002; Zappia et al., 2005; Einstein et al., 2007), favorable to their use in transplantation strategies for immune-related diseases like multiple sclerosis. It is also possible that NSPCs induce neural repair through intrinsic properties of neuroprotection and immunomodulationbyreleasing directlyatthegraft sitea range of molecules (immunomodulatory molecules, growth factors, stem cell regulatory factors) spatially and temporally orchestrated by the microenvironment (Pluchino et al., 2009).
Administration Route and Source of Transplanted NSPCs
Defining the best route of administration of cells represents a constraint for NSPC transplantation and is very dependent on the type of CNS lesions (focal or multifocal). Anatomic and pathologic characteristics of CNS focal disorders like Parkinson disease, spinal cord lesions, Huntington disease, or stroke suggest that intracerebraltransplantationof cells directly at the site of the lesion would be the more appropriate strategy to facilitate tissue regeneration. However, the presence of several lesion areas in diseases like multiple sclerosis or epilepsy represents a major limit for intralesional cellular transplantation approaches, and some groups were able to show a therapeutic effect of NSPC systemic transplantation by intravenous or intrathecal route (Pluchino et al., 2003; Einstein et al., 2007). Efficiency of restorative transplantation can also depend on the differentiation stage of the grafted cells. In some cases where a specific cell type is selectively lost during the pathogenic event, like the dopaminergic neurons in Parkinson disease, transplantation of pre-differentiated cells sharing similar properties in the affected region could allow a better functional recovery (Kim et al., 2002; Lévesque et al., 2009). However, the use of multipotent undifferentiated cells could be the best strategy in the case of a lesion or disease affecting several cell types in extended areas. Indeed these cells could spontaneously differentiate in vivo under the influence of the microenvironment in cells with desired phenotypes. It has recently been show that undifferentiated human NSPCs could survive and differentiate into neurons and glial cells after xenotransplantation into the rat spinal cord (Mothe et al., 2011).
Auto, Allo, and Xenotransplantation of NSPCs
Autologous NSPCs derived from rodent adult nervous tissue and transplanted in the CNS can functionally integrate in the cerebral parenchyma, confirming their potential use in therapy (Taupin and Gage, 2002). Isolation of NSPCs from the human adult brain offers the opportunity to perform autologous transplantation, in which NSPCs would be isolated from an unlesioned region of the CNS, amplified in culture and reimplanted in the patient to repair cerebral or spinal damage without the need for immunosuppressive treatment (Pfeifer et al., 2006). To date, autologous transplantation of differentiated NSPCs was performed in one Parkinson disease patient with encouraging results (Lévesque et al., 2009). If the invasive surgery to isolate NSPCs may have critical consequences like permanent damage in the taking area, this strategy presents the advantage to avoid immune compatibility problems and could allow patient-specific gene modifications of the cells prior to transplantation (Stojkovic and Lako, 2011). Genetic engineering of autologous NSPCs could for example be used to repair potential genetic damage linked to the patient’s disease (like in Huntington disease), stimulate dopaminergic differentiation in the case of Parkinson disease (Wagner et al., 1999) or dedifferentiate NSPCs toward a pluripotent state using the Oct4 gene (Kim et al., 2009). Despite the immunologically special status of the CNS, long-term survival of neural allografts is compromised in the host without immunosuppression (Krystkowiak et al., 2007; Rota Nodari et al., 2010). Immunogenicity is therefore an important parameter to consider for the survival of transplanted cells. NSPCs express class I MHC and co-stimulatory molecules (Imitola et al., 2004; Sergent-Tanguy et al., 2006) but the expression of MHC class II molecules remain undetectable in physiological conditions (Hori et al.,2003). However in the case of an inflammatory process, in particular under IFN? exposure, the expression of immunogenic molecules at NSPC’s surface is strongly increased (Johansson et al., 2008; Laguna Goya et al., 2011) which compromises their survival and consequently their long-term effects. Moreover, NSPC allotransplantation studies have revealed that NSPCs derived from adult tissue could be less efficient than those derived from the fetal CNS (Guillaume and Zhang, 2008). Indeed, the latter have demonstrated a greater capacity of proliferation in vitro and would more easily differentiate into neurons in vivo. NSPCs derived from the adult brain display weak propensity to integrate in the cerebral tissue, show limited synapse formation and their survival remains relatively short (Dziewczapolski et al., 2003), compromising a potential functional recovery. These observations suggest that NSPCs should preferentially be isolated from the fetal CNS prior to transplantation. However, one must keep in mind that the use of human fetal NSPCs, like ES cells, is limited by ethical and logistical issues. The use of NSPCs from animal species like swine could alleviate problems linked to the use of fetal human cells. In several studies, porcine NSPCs have been shown to have a reduced immunogenicity that could optimize their survival in vivo (Armstrong et al., 2001). Porcine NSPCs are also particularly interesting because of their multipotency. Barker’s group has demonstrated that porcine NSPCs xenografted in cyclosporine A-immunosuppressed rats presenting a 6-hydroxydopamine lesion to model Parkinson disease could differentiate into dopaminergic neurons (Armstrong et al., 2002). In addition, the xenografted cells have proven to significantly integrate in the host tissue and partially reconstitute damaged neuronal circuitry (Harrower et al., 2006). In a recent study, our group has shown that NSPCs derived from the cerebral cortex of pig embryos at 28 days of gestation could survive significantly longer than porcine neuroblasts isolated from the ventral mesencephalon after transplantation in the brain of non-immunosuppressed rats. This prolonged survival was associated with an absence of T lymphocyte, dendritic and activated microglial cell infiltration within the NSPC healthy grafts and with a trophic effect of the grafted cells on the host dopaminergic system (Michel-Monigadon et al., 2011). However, we did not observe any dopaminergic differentiation suggesting that NSPCs could be beneficial far beyond the cell replacement process itself.
Therapeutic Effects of Transplanted NSPCs
Cell Replacement
Human NSPCs transplanted in the brain of immunodeficient mice can proliferate, migrate, and differentiate depending on the injection site (Tamaki et al., 2002). Allotransplantation of NSPCs has proven to be efficient in animal models of stroke and spinal cord lesion. Murine NSPCs from C17.2 cell line implanted in an ischemic mouse brain could survive, integrate, and differentiate into neurons and glial cells (Snyder et al., 1997). Animal models of cerebral ischemia have also been used to demonstrate that NSPCs delivered by intraventricular injection could migrate toward and through the damaged tissue, integrate, and differentiate into the main neural cell types (Riess et al., 2002). Moreover, NSPCs genetically modified to overexpress NT-3 have shown a potent integration and a significant regenerative capacity in a model of hypoxic and ischemic cerebral lesion, associated with a decrease in the severity of brain parenchyma damage, an improvement of axonal outgrowth, and a diminution of the glial scar (Park et al., 2006). These studies suggest that, under certain conditions, the lesioned CNS could represent a permissive environment for the maintenance of transplanted NSPCs. Functional integration and reconstruction of the neuronal circuitry is an important goal for restorative therapy. In this context, several groups have shown that NSPCs cultured in vitro could give rise to functional neurons connected and electrically active (Auerbach et al., 2000) that could integrate in the host cortical connections after transplantation (Snyder et al., 1997; Lundberg et al., 2002; Park et al., 2002). Despite these encouraging results, proofs of the ability of NSPCs to differentiate into a sufficient number of functional neurons that could regenerate lost functions by massive cell replacement remain relatively rare. Functional benefits resulting from NSPC transplantation are hardly correlated to the number of fully differentiated neural cells obtained from the grafted cells. This inefficiency to complete the differentiation process and the tendency to maintain an undifferentiated phenotype within the host tissue suggest that transplanted NSPCs partially exert their therapeutic effect by alternative mechanisms.
Neuroprotection
Transplanted NSPCs can significantly improve the survival and the functions of endogenous glial and neuronal progenitor cells that have survived the pathologic event. NSPCs display a strong tropism for tissue lesions and seem to migrate toward these critical sites to release molecules preventing death and facilitating regeneration of targeted cell populations (Ourednik et al., 2002). Several groups are interested in this particular tropism of NSPCs and stem cells in general for damaged zones. This property could indeed be used to deliver therapeutic molecules directly in the lesioned area using genetically engineered stem cells (Müller et al., 2006). This type of strategy would especially be appropriate in ischemic pathologies where altered blood flow decreases the access to the lesion site by systemic administration route. NSPC’s neuroprotective effect often goes along with an increase in the bioavailability of the main neurotrophic factors like NGF, BDNF, CNTF, and GDNF (Carletti et al., 2011). This has been demonstrated in rodents suffering from primary central inflammatory diseases like multiple sclerosis (Pluchino et al., 2003), spinal cord lesions (Lu et al., 2003), or stroke, and also in rodent models of neurodegenerative disorders associated with an immune reaction such as Parkinson and Huntington diseases (McBride et al., 2004; Ryu et al.,2004; Richardson et al., 2005). Cellular and molecular mechanisms implicated in this phenomenon remain unclear but may reside in the intrinsic properties of neurospheres, cellular artifacts resulting from NSPC culture and from which most of transplanted NSPCs are derived. Typically, neu-rospheres are generated in vitro after about 10 days in culture in the absence of serum and in the presence of high concentrations of growth factors (EGF/bFGF). This culture protocol allows the selection of NSPCs responding to those factors. Thus, after transplantation, neurosphere-derived NSPCs might be more sensitive to environmental signals (especially bFGF) in the host tissue triggering neurotrophin secretion by neighboring cells (Lu et al., 2003).
Immunomodulation
Accumulating evidence suggest that stem cells, like mesenchymal stem cells (MSCs) or embryonic stem cells, could interact with components of the immune system, leading to the modulation of many effector functions (Fändrich et al., 2002; Zappia et al., 2005). For instance, MSCs are known to suppress T cell proliferation in vitro (Di Nicola et al., 2002) and to induce long-term graft survival in an allogeneic context (Bartholomew et al., 2002; Chabannes et al., 2007). Regarding NSPCs, a growing number of studies highlighted their immunomodulatory properties, in vivo and in vitro (Bonnamain et al., 2011). It was clearly demonstrated that NSPCs were able to attenuate experimental autoimmune encephalomyelitis (EAE) when injected centrally or peripherally (Einstein et al., 2003, 2007; Pluchino et al., 2005). If the site of action (i.e., CNS or lymph nodes) is not clearly defined, NSPCs have the capacity to inhibit the proliferation of lymph nodes-derived T cells, in response to either concanavalin A (ConA) or to myelin oligodendrocyte glycoprotein, in vitro (Einstein et al., 2003). A recent study showed that syngenic NSPCs transplanted in a model of focal spinal cord injury were able to interact with activated-macrophages in situ to decrease their number and increase the proportion of regulatory T cells (Cusimano et al., 2012). This confirms the importance of the interactions between NSPCs and immune cells to reconfigure the deleterious inflammatory environment and thus promote the healing or regeneration processes. As systemic transplantation of NSPCs was shown to be beneficial in animal models of autoimmune disease like multiple sclerosis (Einstein et al., 2007), it could be hypothesized that NSPCs exert their immunomodulatory effect through a strong paracrine mechanism. Previous studies revealed the expression of immune molecules like TGFβ-1 by NSPCs (Klassen et al., 2003), or inducible nitric oxide synthase (iNOS) and prostaglandin E2 in NSPC cell line (Wang et al., 2009a). Even if the implication of some of these molecules in the immunosuppressive effect of NSPC cell line has already been suggested, the mechanism remains poorly understood.
Conclusion
Significant progresses in stem cell biology have generated considerable enthusiasm for the use of these cells in therapeutic strategies for CNS disorders. Numerous studies have challenged the common knowledge that the CNS was an immunologically privileged tissue deprived of any immune reaction and that transplanted NSPCs were only efficient through mechanisms of neuronal replacement. It is now clear that neuroprotection and immunomodulation capacities of these cells play a major part in the beneficial effects observed in pre-clinical models of neurodegenerative diseases and other CNS affections. Elucidation of these mechanisms may be a crucial step in the control and improvement of NSPC transplantation as a major therapeutic strategy in regenerative medicine.
Conflict of Interest Statement
The authors declare that the research was conducted in the absence of any commercial or financial relationships that could be construed as a potential conflict of interest.
References
Armstrong, R. J., Harrower, T. P., Hurelbrink, C. B., McLaughin, M., Ratcliffe, E. L., Tyers, P., Richards, A., Dunnett, S. B., Rosser, A. E., and Barker, R. A. (2001). Porcine neural xenografts in the immunocompetent rat: immune response following grafting of expanded neural precursor cells. Neuroscience 106, 201–216.
Armstrong, R. J. E., Hurelbrink, C. B., Tyers, P., Ratcliffe, E. L., Richards, A., Dunnett, S. B., Rosser, A. E., and Barker, R. A. (2002). The potential for circuit reconstruction by expanded neural precursor cells explored through porcine xenografts in a rat model of Parkinson’s disease. Exp. Neurol. 175, 98–111.
Auerbach, J. M., Eiden, M. V., and McKay, R. D. (2000). Transplanted CNS stem cells form functional synapses in vivo. Eur. J. Neurosci. 12, 1696–1704.
Bachoud-Lévi, A.-C., Gaura, V., Brugières, P., Lefaucheur, J.-P., Boissé, M.-F., Maison, P., Baudic, S., Ribeiro, M.-J., Bourdet, C., Remy, P., Cesaro, P., Hantraye, P., and Peschanski, M. (2006). Effect of fetal neural transplants in patients with Huntington’s disease 6 years after surgery: a long-term follow-up study. Lancet Neurol. 5, 303–309.
Bachoud-Lévi, A. C., Rémy, P., Nguyen, J. P., Brugières, P., Lefaucheur, J. P., Bourdet, C., Baudic, S., Gaura, V., Maison, P., Haddad, B., Boissé, M. F., Grandmougin, T., Jény, R., Bartolomeo, P., Dalla Barba, G., Degos, J. D., Lisovoski, F., Ergis, A. M., Pailhous, E., Cesaro, P., Hantraye, P., and Peschanski, M. (2000). Motor and cognitive improvements in patients with Huntington’s disease after neural transplantation. Lancet 356, 1975–1979.
Barker, C. F., and Billingham, R. E. (1977). Immunologically privileged sites. Adv. Immunol. 25, 1–54.
Bartholomew, A., Sturgeon, C., Siatskas, M., Ferrer, K., McIntosh, K., Patil, S., Hardy, W., Devine, S., Ucker, D., Deans, R., Moseley, A., and Hoffman, R. (2002). Mesenchymal stem cells suppress lymphocyte proliferation in vitro and prolong skin graft survival in vivo. Exp. Hematol. 30, 42–48.
Billingham, R. E., and Boswell, T. (1953). Studies on the problem of corneal homografts. Proc. R. Soc. Lond. B Biol. Sci. 141, 392–406.
Björklund, A., Dunnett, S. B., Brundin, P., Stoessl, A. J., Freed, C. R., Breeze, R. E., Levivier, M., Peschanski, M., Studer, L., and Barker, R. (2003). Neural transplantation for the treatment of Parkinson’s disease. Lancet Neurol. 2, 437–445.
Björklund, A., and Lindvall, O. (2000). Cell replacement therapies for central nervous system disorders. Nat. Neurosci. 3, 537–544.
Björklund, A., Stenevi, U., Dunnett, S. B., and Gage, F. H. (1982). Cross-species neural grafting in a rat model of Parkinson’s disease. Nature 298, 652–654.
Bonnamain, V., Neveu, I., and Naveilhan, P. (2011). In vitro analyses of the immunosuppressive properties of neural stem/progenitor cells using anti-CD3/CD28-activated T cells. Methods Mol. Biol. 677, 233–243.
Carletti, B., Fpiemonte, F., and Rossi, F. (2011). Neuroprotection: the emerging concept of restorative neural stem cell biology for the treatment of neurodegenerative diseases. Curr. Neuropharmacol. 9, 313–317.
Cayrol, R., Wosik, K., Berard, J. L., Dodelet-Devillers, A., Ifergan, I., Kebir, H., Haqqani, A. S., Kreymborg, K., Krug, S., Moumdjian, R., Bouthillier, A., Becher, B., Arbour, N., David, S., Stanimirovic, D., and Prat, A. (2008). Activated leukocyte cell adhesion molecule promotes leukocyte trafficking into the central nervous system. Nat. Immunol. 9, 137–145.
Chabannes, D., Hill, M., Merieau, E., Rossignol, J., Brion, R., Soulillou, J. P., Anegon, I., and Cuturi, M. C. (2007). A role for heme oxygenase-1 in the immunosuppressive effect of adult rat and human mesenchymal stem cells. Blood 110, 3691–3694.
Cornet, A., Bettelli, E., Oukka, M., Cambouris, C., Avellana-Adalid, V., Kosmatopoulos, K., and Liblau, R. S. (2000). Role of astrocytes in antigen presentation and naive T-cell activation. J. Neuroimmunol. 106, 69–77.
Cusimano, M., Biziato, D., Brambilla, E., Donegà, M., Alfaro-Cervello, C., Snider, S., Salani, G., Pucci, F., Comi, G., Garcia-Verdugo, J. M., De Palma, M., Martino, G., and Pluchino, S. (2012). Transplanted neural stem/precursor cells instruct phagocytes and reduce secondary tissue damage in the injured spinal cord. Brain 35(Pt. 2), 447–460.
Daniloff, J. K., Low, W. C., Bodony, R. P., and Wells, J. (1985). Cross-species neural transplants of embryonic septal nuclei to the hippocampal formation of adult rats. Exp. Brain Res. 59, 73–82.
Deacon, T., Schumacher, J., Dinsmore, J., Thomas, C., Palmer, P., Kott, S., Edge, A., Penney, D., Kassissieh, S., Dempsey, P., and Isacson, O. (1997). Histological evidence of fetal pig neural cell survival after transplantation into a patient with Parkinson’s disease. Nat. Med. 3, 350–353.
Deacon, T. W., Pakzaban, P., Burns, L. H., Dinsmore, J., and Isacson, O. (1994). Cytoarchitectonic development, axon–glia relationships, and long distance axon growth of porcine striatal xenografts in rats. Exp. Neurol. 130, 151–167.
Di Nicola, M., Carlo-Stella, C., Magni, M., Milanesi, M., Longoni, P. D., Matteucci, P., Grisanti, S., and Gianni, A. M. (2002). Human bone marrow stromal cells suppress T-lymphocyte proliferation induced by cellular or nonspecific mitogenic stimuli. Blood 99, 3838–3843.
Dziewczapolski, G., Lie, D. C., Ray, J., Gage, F. H., and Shults, C. W. (2003). Survival and differentiation of adult rat-derived neural progenitor cells transplanted to the striatum of hemiparkinsonian rats. Exp. Neurol. 183, 653–664.
Einstein, O., Fainstein, N., Vaknin, I., Mizrachi-Kol, R., Reihartz, E., Grigoriadis, N., Lavon, I., Baniyash, M., Lassmann, H., and Ben-Hur, T. (2007). Neural precursors attenuate autoimmune encephalomyelitis by peripheral immunosuppression. Ann. Neurol. 61, 209–218.
Einstein, O., Karussis, D., Grigoriadis, N., Mizrachi-Kol, R., Reinhartz, E., Abramsky, O., and Ben-Hur, T. (2003). Intraventricular transplantation of neural precursor cell spheres attenuates acute experimental allergic encephalomyelitis. Mol. Cell. Neurosci. 24, 1074–1082.
Fändrich, F., Dresske, B., Bader, M., and Schulze, M. (2002). Embryonic stem cells share immune-privileged features relevant for tolerance induction. J. Mol. Med. 80, 343–350.
Freeman, T. B., Cicchetti, F., Hauser, R. A., Deacon, T. W., Li, X.-J., Hersch, S. M., Nauert, G. M., Sanberg, P. R., Kordower, J. H., Saporta, S., and Isacson, O. (2000). Transplanted fetal striatum in Huntington’s disease: phenotypic development and lack of pathology. Proc. Natl. Acad. Sci. U.S.A. 97, 13877–13882.
Freeman, T. B., Wojak, J. C., Brandeis, L., Michel, J. P., Pearson, J., and Flamm, E. S. (1988). Cross-species intracerebral grafting of embryonic swine dopaminergic neurons. Prog. Brain Res. 78, 473–477.
Galpern, W. R., Burns, L. H., Deacon, T. W., Dinsmore, J., and Isacson, O. (1996). Xenotransplantation of porcine fetal ventral mesencephalon in a rat model of Parkinson’s disease: functional recovery and graft morphology. Exp. Neurol. 140, 1–13.
Goldstein, G. W., and Betz, A. L. (1983). Recent advances in understanding brain capillary function. Ann. Neurol. 14, 389–395.
Gritti, A., Parati, E. A., Cova, L., Frolichsthal, P., Galli, R., Wanke, E., Faravelli, L., Morassutti, D. J., Roisen, F., Nickel, D. D., and Vescovi, A. L. (1996). Multipotential stem cells from the adult mouse brain proliferate and self-renew in response to basic fibroblast growth factor. J. Neurosci. 16, 1091–1100.
Guillaume, D. J., and Zhang, S.-C. (2008). Human embryonic stem cells: a potential source of transplantable neural progenitor cells. Neurosurg. Focus 24, E3.
Harrower, T. P., Tyers, P., Hooks, Y., and Barker, R. A. (2006). Long-term survival and integration of porcine expanded neural precursor cell grafts in a rat model of Parkinson’s disease. Exp. Neurol. 197, 56–69.
Hatterer, E., Davoust, N., Didier-Bazes, M., Vuaillat, C., Malcus, C., Belin, M.-F., and Nataf, S. (2006). How to drain without lymphatics? Dendritic cells migrate from the cerebrospinal fluid to the B-cell follicles of cervical lymph nodes. Blood 107, 806–812.
Head, J. R., Neaves, W. B., and Billingham, R. E. (1983). Immune privilege in the testis. I. Basic parameters of allograft survival. Transplantation 36, 423–431.
Hickey, W. F. (2001). Basic principles of immunological surveillance of the normal central nervous system. Glia 36, 118–124.
Hickey, W. F., Hsu, B. L., and Kimura, H. (1991). T-lymphocyte entry into the central nervous system. J. Neurosci. Res. 28, 254–260.
Höftberger, R., Aboul-Enein, F., Brueck, W., Lucchinetti, C., Rodriguez, M., Schmidbauer, M., Jellinger, K., and Lassmann, H. (2004). Expression of major histocompatibility complex class I molecules on the different cell types in multiple sclerosis lesions. Brain Pathol. 14, 43–50.
Honey, C. R., Clarke, D. J., Dallman, M. J., and Charlton, H. M. (1990). Human neural graft function in rats treated with anti-interleukin II receptor antibody. Neuroreport 1, 247–249.
Hori, J., Joyce, N., and Streilein, J. W. (2000). Epithelium-deficient corneal allografts display immune privilege beneath the kidney capsule. Invest. Ophthalmol. Vis. Sci. 41, 443–452.
Hori, J., Ng, T. F., Shatos, M., Klassen, H., Streilein, J. W., and Young, M. J. (2003). Neural progenitor cells lack immunogenicity and resist destruction as allografts. Stem Cells 21, 405–416.
Huffaker, T. K., Boss, B. D., Morgan, A. S., Neff, N. T., Strecker, R. E., Spence, M. S., and Miao, R. (1989). Xenografting of fetal pig ventral mesencephalon corrects motor asymmetry in the rat model of Parkinson’s disease. Exp. Brain Res. 77, 329–336.
Imitola, J., Comabella, M., Chandraker, A. K., Dangond, F., Sayegh, M. H., Snyder, E. Y., and Khoury, S. J. (2004). Neural stem/progenitor cells express costimulatory molecules that are differentially regulated by inflammatory and apoptotic stimuli. Am. J. Pathol. 164, 1615–1625.
Isacson, O., Deacon, T. W., Pakzaban, P., Galpern, W. R., Dinsmore, J., and Burns, L. H. (1995). Transplanted xenogeneic neural cells in neurodegenerative disease models exhibit remarkable axonal target specificity and distinct growth patterns of glial and axonal fibres. Nat. Med. 1, 1189–1194.
Johansson, S., Price, J., and Modo, M. (2008). Effect of inflammatory cytokines on major histocompatibility complex expression and differentiation of human neural stem/progenitor cells. Stem Cells 26, 2444–2454.
Kebir, H., Kreymborg, K., Ifergan, I., Dodelet-Devillers, A., Cayrol, R., Bernard, M., Giuliani, F., Arbour, N., Becher, B., and Prat, A. (2007). Human TH17 lymphocytes promote blood–brain barrier disruption and central nervous system inflammation. Nat. Med. 13, 1173–1175.
Kerschensteiner, M., Meinl, E., and Hohlfeld, R. (2009). Neuro-immune crosstalk in CNS diseases. Neuroscience 158, 1122–1132.
Kim, J. B., Sebastiano, V., Wu, G., Araúzo-Bravo, M. J., Sasse, P., Gentile, L., Ko, K., Ruau, D., Ehrich, M., van den Boom, D., Meyer, J., Hübner, K., Bernemann, C., Ortmeier, C., Zenke, M., Fleischmann, B. K., Zaehres, H., and Schöler, H. R. (2009). Oct4-induced pluripotency in adult neural stem cells. Cell 136, 411–419.
Kim, J.-H., Auerbach, J. M., Rodríguez-Gómez, J. A., Velasco, I., Gavin, D., Lumelsky, N., Lee, S.-H., Nguyen, J., Sánchez-Pernaute, R., Bankiewicz, K., and McKay, R. (2002). Dopamine neurons derived from embryonic stem cells function in an animal model of Parkinson’s disease. Nature 418, 50–56.
Klassen, H., Schwartz, M. R., Bailey, A. H., and Young, M. J. (2001). Surface markers expressed by multipotent human and mouse neural progenitor cells include tetraspanins and non-protein epitopes. Neurosci. Lett. 312, 180–182.
Klassen, H. J., Imfeld, K. L., Kirov, I. I., Tai, L., Gage, F. H., Young, M. J., and Berman, M. A. (2003). Expression of cytokines by multipotent neural progenitor cells. Cytokine 22, 101–106.
Krystkowiak, P., Gaura, V., Labalette, M., Rialland, A., Remy, P., Peschanski, M., and Bachoud-Lévi, A.-C. (2007). Alloimmunisation to donor antigens and immune rejection following foetal neural grafts to the brain in patients with Huntington’s disease. PLoS ONE 2, e166. doi: 10.1371/journal.pone.0000166
Laguna Goya, R., Busch, R., Mathur, R., Coles, A. J., and Barker, R. A. (2011). Human fetal neural precursor cells can up-regulate MHC class I and class II expression and elicit CD4 and CD8 T cell proliferation. Neurobiol. Dis. 41, 407–414.
Larsson, L. C., Czech, K. A., Brundin, P., and Widner, H. (2000). Intrastriatal ventral mesencephalic xenografts of porcine tissue in rats: immune responses and functional effects. Cell Transplant. 9, 261–272.
Larsson, L. C., Czech, K. A., Widner, H., and Korsgren, O. (1999). Discordant neural tissue xenografts survive longer in immunoglobulin deficient mice. Transplantation 68, 1153–1160.
Lévesque, M. F., Neuman, T., and Rezak, M. (2009). Therapeutic microinjection of autologous adult human neural stem cells and differentiated neurons for Parkinson’s disease: five-year post-operative outcome. Open Stem Cell J. 1, 20–29.
Lu, P., Jones, L. L., Snyder, E. Y., and Tuszynski, M. H. (2003). Neural stem cells constitutively secrete neurotrophic factors and promote extensive host axonal growth after spinal cord injury. Exp. Neurol. 181, 115–129.
Lundberg, C., Englund, U., Trono, D., Björklund, A., and Wictorin, K. (2002). Differentiation of the RN33B cell line into forebrain projection neurons after transplantation into the neonatal rat brain. Exp. Neurol. 175, 370–387.
Martin, C., Plat, M., Nerriére-Daguin, V., Coulon, F., Uzbekova, S., Venturi, E., Condé, F., Hermel, J.-M., Hantraye, P., Tesson, L., Anegon, I., Melchior, B., Peschanski, M., Le Mauff, B., Boeffard, F., Sergent-Tanguy, S., Neveu, I., Naveilhan, P., Soulillou, J. P., Terqui, M., Brachet, P., and Vanhove, B. (2005). Transgenic expression of CTLA4-Ig by fetal pig neurons for xenotransplantation. Transgenic Res. 14, 373–384.
Mauerhoff, T., Pujol-Borrell, R., Mirakian, R., and Bottazzo, G. F. (1988). Differential expression and regulation of major histocompatibility complex (MHC) products in neural and glial cells of the human fetal brain. J. Neuroimmunol. 18, 271–289.
McBride, J. L., Behrstock, S. P., Chen, E.-Y., Jakel, R. J., Siegel, I., Svendsen, C. N., and Kordower, J. H. (2004). Human neural stem cell transplants improve motor function in a rat model of Huntington’s disease. J. Comp. Neurol. 475, 211–219.
Medawar, P. B. (1948). Immunity to homologous grafted skin; the fate of skin homografts transplanted to the brain, to subcutaneous tissue, and to the anterior chamber of the eye. Br. J. Exp. Pathol. 29, 58–69.
Melchior, B., Rémy, S., Nerrière-Daguin, V., Heslan, J.-M., Soulillou, J.-P., and Brachet, P. (2002). Temporal analysis of cytokine gene expression during infiltration of porcine neuronal grafts implanted into the rat brain. J. Neurosci. Res. 68, 284–292.
Michel, D. C., Nerrière-Daguin, V., Josien, R., Brachet, P., Naveilhan, P., and Neveu, I. (2006). Dendritic cell recruitment following xenografting of pig fetal mesencephalic cells into the rat brain. Exp. Neurol. 202, 76–84.
Michel-Monigadon, D., Bonnamain, V., Nerrière-Daguin, V., Dugast, A.-S., Lévèque, X., Plat, M., Venturi, E., Brachet, P., Anegon, I., Vanhove, B., Neveu, I., and Naveilhan, P. (2011). Trophic and immunoregulatory properties of neural precursor cells: benefit for intracerebral transplantation. Exp. Neurol. 230, 35–47.
Mothe, A. J., Zahir, T., Santaguida, C., Cook, D., and Tator, C. H. (2011). Neural stem/progenitor cells from the adult human spinal cord are multipotent and self-renewing and differentiate after transplantation. PLoS ONE 6, e27079. doi: 10.1371/journal.pone.0027079
Müller, F.-J., Snyder, E. Y., and Loring, J. F. (2006). Gene therapy: can neural stem cells deliver? Nat. Rev. Neurosci. 7, 75–84.
Odeberg, J., Piao, J.-H., Samuelsson, E.-B., Falci, S., and Akesson, E. (2005). Low immunogenicity of in vitro-expanded human neural cells despite high MHC expression. J. Neuroimmunol. 161, 1–11.
Okura, Y., Tanaka, R., Ono, K., Yoshida, S., Tanuma, N., and Matsumoto, Y. (1997). Treatment of rat hemiparkinson model with xenogeneic neural transplantation: tolerance induction by anti-T-cell antibodies. J. Neurosci. Res. 48, 385–396.
Olanow, C. W., Goetz, C. G., Kordower, J. H., Stoessl, A. J., Sossi, V., Brin, M. F., Shannon, K. M., Nauert, G. M., Perl, D. P., Godbold, J., and Freeman, T. B. (2003). A double-blind controlled trial of bilateral fetal nigral transplantation in Parkinson’s disease. Ann. Neurol. 54, 403–414.
Ourednik, J., Ourednik, V., Lynch, W. P., Schachner, M., and Snyder, E. Y. (2002). Neural stem cells display an inherent mechanism for rescuing dysfunctional neurons. Nat. Biotechnol. 20, 1103–1110.
Pakzaban, P., and Isacson, O. (1994). Neural xenotransplantation: reconstruction of neuronal circuitry across species barriers. Neuroscience 62, 989–1001.
Park, K. I., Himes, B. T., Stieg, P. E., Tessler, A., Fischer, I., and Snyder, E. Y. (2006). Neural stem cells may be uniquely suited for combined gene therapy and cell replacement: evidence from engraftment of neurotrophin-3-expressing stem cells in hypoxic-ischemic brain injury. Exp. Neurol. 199, 179–190.
Park, K. I., Teng, Y. D., and Snyder, E. Y. (2002). The injured brain interacts reciprocally with neural stem cells supported by scaffolds to reconstitute lost tissue. Nat. Biotechnol. 20, 1111–1117.
Pfeifer, K., Vroemen, M., Caioni, M., Aigner, L., Bogdahn, U., and Weidner, N. (2006). Autologous adult rodent neural progenitor cell transplantation represents a feasible strategy to promote structural repair in the chronically injured spinal cord. Regen. Med. 1, 255–266.
Pluchino, S., Quattrini, A., Brambilla, E., Gritti, A., Salani, G., Dina, G., Galli, R., Del Carro, U., Amadio, S., Bergami, A., Furlan, R., Comi, G., Vescovi, A. L., and Martino, G. (2003). Injection of adult neurospheres induces recovery in a chronic model of multiple sclerosis. Nature 422, 688–694.
Pluchino, S., Zanotti, L., Brini, E., Ferrari, S., and Martino, G. (2009). Regeneration and repair in multiple sclerosis: the role of cell transplantation. Neurosci. Lett. 456, 101–106.
Pluchino, S., Zanotti, L., Rossi, B., Brambilla, E., Ottoboni, L., Salani, G., Martinello, M., Cattalini, A., Bergami, A., Furlan, R., Comi, G., Constantin, G., and Martino, G. (2005). Neurosphere-derived multipotent precursors promote neuroprotection by an immunomodulatory mechanism. Nature 436, 266–271.
Rémy, S., Canova, C., Daguin-Nerrière, V., Martin, C., Melchior, B., Neveu, I., Charreau, B., Soulillou, J. P., and Brachet, P. (2001). Different mechanisms mediate the rejection of porcine neurons and endothelial cells transplanted into the rat brain. Xenotransplantation 8, 136–148.
Reynolds, B. A., Tetzlaff, W., and Weiss, S. (1992). A multipotent EGF-responsive striatal embryonic progenitor cell produces neurons and astrocytes. J. Neurosci. 12, 4565–4574.
Reynolds, B. A., and Weiss, S. (1992). Generation of neurons and astrocytes from isolated cells of the adult mammalian central nervous system. Science 255, 1707–1710.
Rezzani, R. (2006). Exploring cyclosporine A-side effects and the protective role-played by antioxidants: the morphological and immunohistochemical studies. Histol. Histopathol. 21, 301–316.
Richardson, R. M., Broaddus, W. C., Holloway, K. L., and Fillmore, H. L. (2005). Grafts of adult subependymal zone neuronal progenitor cells rescue hemiparkinsonian behavioral decline. Brain Res. 1032, 11–22.
Riess, P., Zhang, C., Saatman, K. E., Laurer, H. L., Longhi, L. G., Raghupathi, R., Lenzlinger, P. M., Lifshitz, J., Boockvar, J., Neugebauer, E., Snyder, E. Y., and McIntosh, T. K. (2002). Transplanted neural stem cells survive, differentiate, and improve neurological motor function after experimental traumatic brain injury. Neurosurgery 51, 1043–1052; discussion 1052–1054.
Rota Nodari, L., Ferrari, D., Giani, F., Bossi, M., Rodriguez-Menendez, V., Tredici, G., Delia, D., Vescovi, A. L., and De Filippis, L. (2010). Long-term survival of human neural stem cells in the ischemic rat brain upon transient immunosuppression. PLoS ONE 5, e14035. doi: 10.1371/ journal.pone.0014035
Ryu, J. K., Kim, J., Cho, S. J., Hatori, K., Nagai, A., Choi, H. B., Lee, M. C., McLarnon, J. G., and Kim, S. U. (2004). Proactive transplantation of human neural stem cells prevents degeneration of striatal neurons in a rat model of Huntington disease. Neurobiol. Dis. 16, 68–77.
Schumacher, J. M., Ellias, S. A., Palmer, E. P., Kott, H. S., Dinsmore, J., Dempsey, P. K., Fischman, A. J., Thomas, C., Feldman, R. G., Kassissieh, S., Raineri, R., Manhart, C., Penney, D., Fink, J. S., and Isacson, O. (2000). Transplantation of embryonic porcine mesencephalic tissue in patients with PD. Neurology 54, 1042–1050.
Sergent-Tanguy, S., Véziers, J., Bonnamain, V., Boudin, H., Neveu, I., and Naveilhan, P. (2006). Cell surface antigens on rat neural progenitors and characterization of the CD3 (+)/CD3 (-) cell populations. Differentiation 74, 530–541.
Sivakumar, V., Foulds, W. S., Luu, C. D., Ling, E., and Kaur, C. (2011). Retinal ganglion cell death is induced by microglia derived pro-inflammatory cytokines in the hypoxic neonatal retina. J. Pathol. 224, 245–260.
Snyder, E. Y., Yoon, C., Flax, J. D., and Macklis, J. D. (1997). Multipotent neural precursors can differentiate toward replacement of neurons undergoing targeted apoptotic degeneration in adult mouse neocortex. Proc. Natl. Acad. Sci. U.S.A. 94, 11663–11668.
Stojkovic, M., and Lako, M. (2011). Neural stem cells, a step closer to clinic? Stem Cells 29, 1477–1478.
Tamaki, S., Eckert, K., He, D., Sutton, R., Doshe, M., Jain, G., Tushinski, R., Reitsma, M., Harris, B., Tsukamoto, A., Gage, F., Weissman, I., and Uchida, N. (2002). Engraftment of sorted/expanded human central nervous system stem cells from fetal brain. J. Neurosci. Res. 69, 976–986.
Taupin, P., and Gage, F. H. (2002). Adult neurogenesis and neural stem cells of the central nervous system in mammals. J. Neurosci. Res. 69, 745–749.
Vescovi, A. L., Reynolds, B. A., Fraser, D. D., and Weiss, S. (1993). bFGF regulates the proliferative fate of unipotent (neuronal) and bipotent (neuronal/astroglial) EGF-generated CNS progenitor cells. Neuron 11, 951–966.
Wagner, J., Akerud, P., Castro, D. S., Holm, P. C., Canals, J. M., Snyder, E. Y., Perlmann, T., and Arenas, E. (1999). Induction of a midbrain dopaminergic phenotype in Nurr1-overexpressing neural stem cells by type 1 astrocytes. Nat. Biotechnol. 17, 653–659.
Wang, L., Shi, J., van Ginkel, F. W., Lan, L., Niemeyer, G., Martin, D. R., Snyder, E. Y., and Cox, N. R. (2009a). Neural stem/progenitor cells modulate immune responses by suppressing T lymphocytes with nitric oxide and prostaglandin E2. Exp. Neurol. 216, 177–183.
Wang, P. H. M., Schwindt, T. T., Barnabé, G. F., Motta, F. L. T., Semedo, P., Beraldo, F. C., Mazzali, M., Dos Reis, M. A., Teixeira Vde, P. A., Pacheco-Silva, A., Mello, L. E., and Câmara, N. O. (2009b). Administration of neural precursor cells ameliorates renal ischemia-reperfusion injury. Nephron Exp. Nephrol. 112, e20–e28.
Wekerle, H., Sun, D., Oropeza-Wekerle, R. L., and Meyermann, R. (1987). Immune reactivity in the nervous system: modulation of T-lymphocyte activation by glial cells. J. Exp. Biol. 132, 43–57.
Wictorin, K., Clarke, D. J., Bolam, J. P., Brundin, P., Gustavii, B., Lindvall, O., and Björklund, A. (1990). Extensive efferent projections of intra-striatally transplanted striatal neurons as revealed by a species-specific neurofilament marker and anterograde axonal tracing. Prog. Brain Res. 82, 391–399.
Wood, M. J., Sloan, D. J., Wood, K. J., and Charlton, H. M. (1996). Indefinite survival of neural xenografts induced with anti-CD4 monoclonal antibodies. Neuroscience 70, 775–789.
Yin, L., Fu, S.-L., Shi, G.-Y., Li, Y., Jin, J.-Q., Ma, Z.-W., and Lu, P.-H. (2008). Expression and regulation of major histocompatibility complex on neural stem cells and their lineages. Stem Cells Dev. 17, 53–65.
Keywords: transplantation, immunomodulation, immune reactions, stem cells, regenerative medicine
Citation: Bonnamain V, Neveu I and Naveilhan P (2012) Neural stem/progenitor cells as promising candidates for regenerative therapy of the central nervous system. Front. Cell. Neurosci. 6:17. doi: 10.3389/fncel.2012.00017
Received: 15 February 2012; Paper pending published: 06 March 2012;
Accepted: 26 March 2012; Published online: 11 April 2012.
Edited by:
Afsaneh Gaillard, INSERM, University of Poitiers, FranceReviewed by:
Corette Wierenga, Utrecht University, NetherlandsMohamed Jaber, INSERM, University of Poitiers, France
Copyright: © 2012 Bonnamain, Neveu and Naveilhan. This is an open-access article distributed under the terms of the Creative Commons Attribution Non Commercial License, which permits noncommercial use, distribution, and reproduction in other forums, provided the original authors and source are credited.
*Correspondence: Philippe Naveilhan, INSERM U643, 30 Boulevard Jean Monnet, 44093 Nantes Cedex 01, France. e-mail:cGhpbGlwcGUubmF2ZWlsaGFuQHVuaXYtbmFudGVzLmZy