- 1Department of Experimental and Clinical Medicine, Faculty of Medicine and Surgery, Università Politecnica delle Marche, Ancona, Italy
- 2Center for Neurobiology of Aging, IRCCS INRCA, Ancona, Italy
- 3Fondazione di Medicina Molecolare, Università Politecnica delle Marche, Ancona, Italy
γ-Aminobutyric acid (GABA) transporter (GAT)-1, the major GABA transporter in the brain, plays a key role in modulating GABA signaling and is involved in the pathophysiology of several neuropsychiatric diseases, including epilepsy. The original description of GAT-1 as a neuronal transporter has guided the interpretation of the findings of all physiological, pharmacological, genetic, or clinical studies. However, evidence published in the past few years, some of which is briefly reviewed herein, does not seem to be consistent with a neurocentric view of GAT-1 function and calls for more detailed analysis of its localization. We therefore performed a thorough systematic assessment of GAT-1 localization in neocortex and subcortical white matter. In line with earlier work, we found that GAT-1 was robustly expressed in axon terminals forming symmetric synapses and in astrocytic processes, whereas its astrocytic expression was more diffuse than expected and, even more surprisingly, immature and mature oligodendrocytes and microglial cells also expressed the transporter. These data indicate that the era of “neuronal” and “glial” GABA transporters has finally come to a close and provide a wider perspective from which to view GABA-mediated physiological phenomena. In addition, given the well-known involvement of astrocytes, oligodendrocytes, and microglial cells in physiological as well as pathological conditions, the demonstration of functional GAT-1 in these cells is expected to provide greater insight into the phenomena occurring in the diseased brain as well as to prompt a reassessment of earlier findings.
Introduction
γ-Aminobutyric acid (GABA) transporter (GAT)-1 is a highly conserved molecule that is encoded by SLC6A1 and transports GABA in a high-affinity, Na+- and Cl−-dependent manner (Kanner, 1978; Guastella et al., 1990; Borden, 1996). As the major GABA transporter in the brain, it plays a key role in modulating GABA signaling (Cherubini and Conti, 2001; Scimemi, 2014). Besides being involved in a broad range of brain functions (Cherubini and Conti, 2001; Bragina et al., 2008; Conti et al., 2011; Kinjo et al., 2013; Scimemi, 2014; Savtchenko et al., 2015; Zafar and Jabeen, 2018), GAT-1 has also been implicated in the pathophysiology of a number of neuropsychiatric disorders including anxiety, depression, epilepsy, Alzheimer’s disease, and schizophrenia (Lai et al., 1998; Nägga et al., 1999; Pierri et al., 1999; Sundman-Eriksson and Allard, 2002; Conti et al., 2004; Lewis and Gonzalez-Burgos, 2006; Cope et al., 2009; Bitanihirwe and Woo, 2014; Carvill et al., 2015; Gong et al., 2015; Fuhrer et al., 2017; Mattison et al., 2018).
GABA uptake by GAT-1 is heavily inhibited by cis-3-aminocyclohexane carboxylic acid (ACHC) and, to a lower extent, by 2,4-diaminobutyric acid, but not by β-alanine (Guastella et al., 1990; Keynan et al., 1992; Liu et al., 1993), two features that have often been considered typical of “neuronal” transporters. This view has been bolstered by the demonstration that GAT-1 is strongly expressed in axon terminals (Minelli et al., 1995; Conti et al., 1998)—despite the fact that the same studies also clearly documented an astrocytic localization—and is still widely used to interpret physiological, pharmacological, genetic, and clinical investigations. However, the findings of several studies published in the past few years call for a more detailed analysis of GAT-1 localization.
Recent Studies Suggest a Less Simplistic Scenario
After reports of SLC6A1 variants in patients with myoclonic atonic epilepsy (Dikow et al., 2014; Carvill et al., 2015; Mattison et al., 2018; Cai et al., 2019; Posar and Visconti, 2019), clinical, neurophysiological, and genetic examination of a relatively large cohort of subjects (n = 34) bearing SLC6A1 mutations demonstrated that 97% of them exhibited varying degrees of intellectual disability (ID) and that 91% had been diagnosed with epilepsy (absence, myoclonic, or atonic) based on EEG patterns characterized by irregular, high, ample, generalized spikes, and wave discharges (Johannesen et al., 2018). Notably, more than 60% of these subjects had suffered from moderate or significant ID before epilepsy onset, whereas in a limited number of cases, the ID was not accompanied by epilepsy. Although genetic analysis of the SLC6A1 variants suggested that the probable disease mechanism was loss of GAT-1 function, assessment of the clinical characteristics associated to them disclosed a wide phenotypic spectrum where the dominant sign, ID, is not quite a “pure” neuronal disorder (Di Marco et al., 2016; Iwase et al., 2017; Maglorius Renkilaraj et al., 2017).
Earlier this year, Inaba et al. (2019) used a model of chronic brain hypoperfusion to assess the protective effects conferred by the anticonvulsant levetiracetam (LEV) on the white matter of mice subjected to bilateral common carotid artery stenosis (BCAS). They found that LEV: (i) did confer protection against learning and memory impairment and white matter injury; (ii) induced PKA/CREB activation; (iii) raised the number of (GFAP-labeled) astrocytes in a time-dependent manner; (iv) reduced Iba-1-positive (+) microglial cells; and (v) increased oligodendrocytes and their precursor cells (Inaba et al., 2019). According to the evidence published to date, synaptic vesicle protein SV2A is the sole receptor for LEV (Lynch et al., 2004). However, an earlier report that LEV increases GAT-1 expression (Ueda et al., 2007), presumably through protein–protein interactions—as recently shown for other vesicular proteins (Marcotulli et al., 2017)—suggests that at least some of the effects described by Inaba et al. (2019) might be mediated through GAT-1.
In 1990, Braestrup and colleagues reported that tiagabine [(3R)-1-[4,4-bis(3-methylthiophen-2-yl)but-3-en-1-yl]piperidine-3-carboxylic acid] nipecotic acid] binds GAT-1 with high affinity (Braestrup et al., 1990). Subsequently, after GAT-1 cloning and functional characterization (Guastella et al., 1990), tiagabine was demonstrated to interact specifically with it (Borden et al., 1994; Borden, 1996) and to be a clinically effective antiepileptic drug (Suzdak and Jansen, 1995; Schousboe and White, 2009; Froestl, 2011). The selectivity of tiagabine for GAT-1 confines its action to those regions of the central nervous system where the transporter plays a large role (neocortex, cerebellum, and hippocampus; Jasmin et al., 2004). Tiagabine has also been found to exert antinociceptive, anxiolytic-like, sedative, and antidepressant-like actions (Jasmin et al., 2004; Sałat et al., 2015). Finally, tiagabine monotherapy appears to improve the performance of epilepsy patients on a number of neuropsychological tests (Dodrill et al., 1998), an effect that seems to relate to the report that heterozygous mice show greater learning and memory compared to wild-type and homozygous GAT-1−/− mice (Shi et al., 2012).
In 2015, two articles revived the interest in the effects of tiagabine. In a study of cerebellar GABA signaling using a mouse model of diffuse white matter injury (DWMI), a severe neurological syndrome characterized by hypomyelination and disruption of subcortical white matter development and involving behavioral, cognitive, and motor deficits, Zonouzi et al. (2015) demonstrated that tiagabine enhances the progression of NG2 (oligodendrocyte precursor) cells and promotes oligodendrogenesis and myelination. The same year, Liu and coworkers documented that in a methyl-4-phenyl-1,2,3,6-tetrahydropyridine (MPTP) mouse model of Parkinson’s disease, tiagabine pretreatment attenuates microglial activation, it confers partial protection on the nigrostriatal axis, and it alleviates motor deficits, but its protective function is abolished in GAT-1 knockout mice challenged with MPTP. The authors also found that tiagabine suppresses microglial activation in mice treated by intranigral lipopolysaccharide infusion, an alternative model of Parkinson’s disease (Liu et al., 2015). Although neither study clarified the mechanism(s) underlying tiagabine’s action, it is conceivable that the effects described by Zonouzi et al. (2015) and Liu et al. (2015) depend on a direct action on GAT-1 expression by microglial cells and oligodendrocytes, which may go some way toward explaining the findings of the two groups.
Evidence for a Widespread Cellular Expression of GAT-1
Some years ago, while investigating GAT-1 immunoreactivity in subcortical white matter, we detected GAT-1 cells of different sizes and morphologies (Figure 1). Some were small and round with small processes (Figure 1A), and others were medium-sized, rounded or oval with regular profiles; some medium-sized cells had a pyramidal shape with long and intensely stained processes, whereas other cells were large and elongated. The frequency distribution of their diameter is reported in Figure 1B. The broad difference in the size and morphology of these subcortical white matter cells suggested to us that they might belong to different types. We therefore set up a study to examine them in detail.
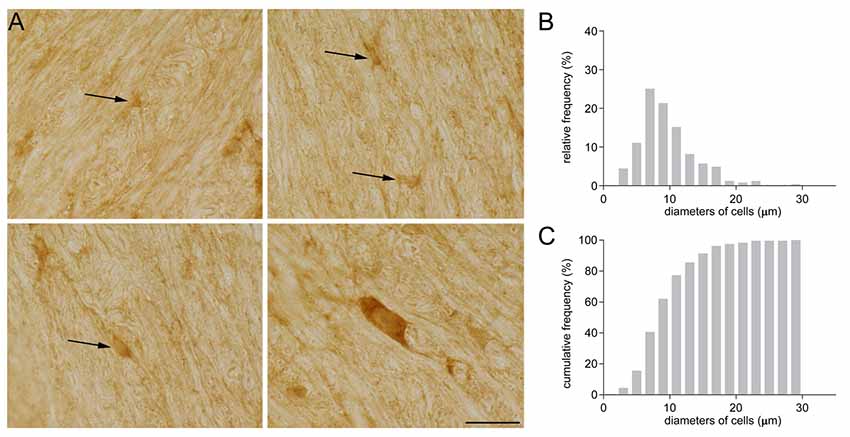
Figure 1. (A) GAT-1 immunoreactivity in the subcortical white matter reveals the presence of numerous cells of small and medium size (arrows) and of different morphology. (B) Frequency and (C) cumulative frequency distribution of the diameter of GAT-1-positive cells. Bar: 20 μm (modified from Fattorini et al., 2017).
In line with earlier work (Minelli et al., 1995; Conti et al., 1998), electron microscopic (EM) observation demonstrated that GAT-1 was robustly expressed in axon terminals forming symmetric synapses and in astrocytic processes. However, its astrocytic expression was more diffuse than expected and, even more surprisingly, immature and mature oligodendrocytes and microglial cells also expressed the transporter (Figure 2).
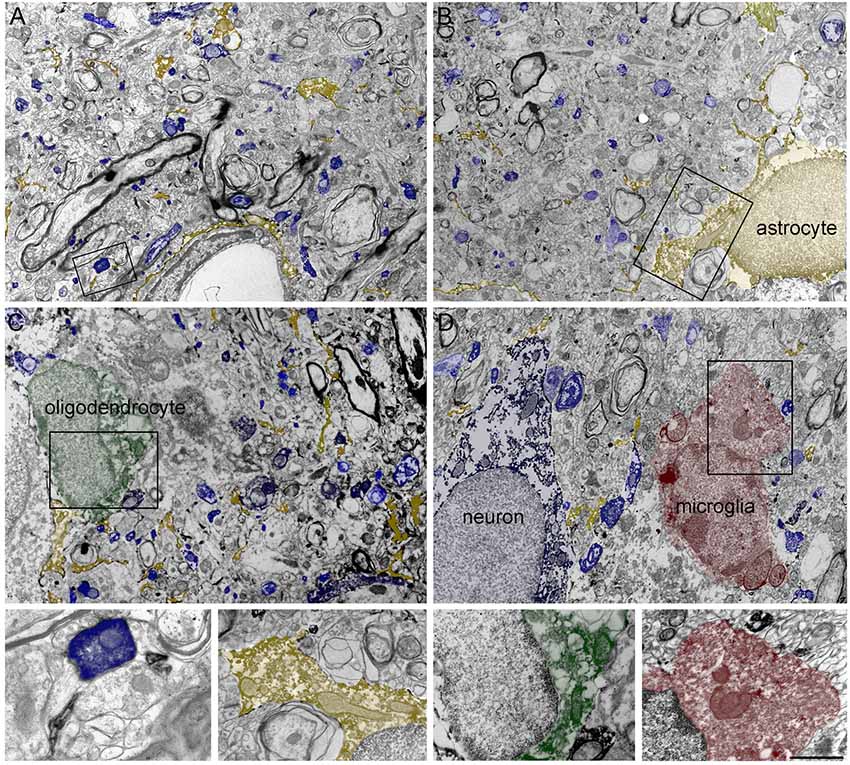
Figure 2. (A–D) Four low-magnification electron microscopic (EM) fields showing GAT-1 immunoreactivity in cerebral neocortex (layers II–III of rat parietal cortex). Colored profiles code for different GAT-1-positive cell types and/or profiles: blue, axon terminals, axon, and neuron; yellow, astrocyte and astrocytic processes; green, oligodendrocyte; red, microglial cell. Framed regions in (A–D) are reproduced and enlarged, in the lowest portion of the figure. Bar: 2.5 μm for (A–D); 0.8 and 1 μm for enlarged frames of (A) and (B–D), respectively (modified from Melone et al., 2015; Fattorini et al., 2017, 2020).
Astrocytes
Recently, quantitative EM analysis, performed in our laboratory, disclosed hitherto unknown features of astrocytic GAT-1 localization in rat cerebral cortex; in particular, we found that: (i) approximately 43% of GAT-1+ profiles in the cortical neuropil are astrocytic processes; (ii) at synaptic loci, GAT-1+ astrocytic processes lie close to the pre- and postsynaptic elements of symmetric as well as asymmetric synapses; and (iii) astrocytic GAT-1 expression at symmetric synapses is not homogeneous, since in ~15% of cases it is associated to GAT-1+ axon terminals and in ~22% of cases it is exclusively localized in astrocytic processes associated to symmetric synapses (i.e., not expressing GAT-1 in axon terminals). The latter fraction of astrocytic GAT-1 increases to up to ~38% in GABAergic synapses targeting distal dendrites and spines, where GAT-1+ axon terminals are less numerous (Melone et al., 2014). Immunogold EM demonstrated that the density of GAT-1 molecules in astrocytic process membranes was ~3.5 times higher than in axon terminals and displayed a continuous distribution from perisynaptic to extrasynaptic regions (respectively within and over 300 nm from the borders of the symmetric synapse specializations), with peaks of concentration at ~950 nm; in contrast, GAT-1 molecules in the membranes of axon terminals showed a preferential perisynaptic localization (Melone et al., 2015).
Oligodendrocytes
EM analysis revealed GAT-1 immunoreactivity in immature and mature oligodendrocytes both in gray matter and in subcortical white matter. Co-localization studies of GAT-1 and specific oligodendrocyte markers (NG2 and RIP) demonstrated that approximately 12% of GAT-1+ cells in white matter were immature oligodendrocytes and that about 15% were mature oligodendrocytes. Studies of radiolabeled GABA uptake, performed to establish whether GAT-1 localized in oligodendrocytes was functional, demonstrated significant inhibition of Na+-dependent GABA uptake in the presence of tiagabine, indicating that GABA uptake in oligodendrocytes is driven by GAT-1 (Fattorini et al., 2017).
Microglial Cells
EM analysis also demonstrated GAT-1 immunoreactivity in the soma of microglial cells in subcortical white matter and cortical gray matter as well as in microglial processes, where GAT-1 was localized predominantly in the proximal portion. To quantify GAT-1 protein in microglial cells, we measured the volume of the cells containing the GAT-1 protein signal (in cx3cr1+/gfp animals) and found that it was ~3% in subcortical white matter and ~8% in cortical gray matter. We also established that Na+-dependent GABA uptake was significantly inhibited by NNC-711, a potent GABA uptake inhibitor with high affinity and selectivity for GAT-1 (Borden et al., 1994). In addition, we documented that, like neurons, microglial cells can regulate the membrane expression of GAT-1 in a syntaxin1A-dependent manner (Deken et al., 2000), since syntaxin1A-specific cleavage by botulin toxin C1 (Schiavo et al., 1995; Deken et al., 2000) completely blocks GAT-1-dependent modulation of GABA uptake (Fattorini et al., 2020).
Discussion
The notion that GAT-1 is not an exclusively “neuronal” transporter appears to be gaining momentum. Indeed, quantitative analysis of GAT-1 in the cerebral cortex, performed in our laboratory, showed that 54% of GAT-1 + profiles were neuronal and that no less than 42% were astrocytic (Melone et al., 2015). More recently, we reported significant GAT-1 expression in oligodendrocytes and microglia (Fattorini et al., 2017, 2020; Figure 2). In this connection, it is worth noting that GAT-3, a putative “glial” transporter (see Minelli et al., 1996 for the neocortex), also seems to be expressed in brainstem and cortical neurons, at least in certain experimental conditions (Clark et al., 1992; Melone et al., 2003, 2005, 2015), and that GAT-2, another putative “glial” transporter, is expressed in epithelial cells and, although at a very low level, also in neurons (Conti et al., 1999). It therefore seems that the era of “neuronal” and “glial” GABA transporters has finally come to a close.
The demonstration that all major brain cells express GAT-1 will conceivably contribute to generate a wider framework through which to assess (and indeed reassess) numerous cerebral GABA-mediated phenomena that occur in physiological conditions. This requires tackling first the issue of the physiological role of GAT-1 in oligodendrocytes and microglial cells. Given the well-established involvement of astrocytes, oligodendrocytes, and microglial cells in pathophysiological conditions (Verkhratsky and Butt, 2013), the demonstration of functional GAT-1 in these cells is expected to provide greater insight into the phenomena occurring in the diseased brain and to prompt a reappraisal of earlier findings. Notably, one of the studies that stimulated the present reassessment (Zonouzi et al., 2015) can now be interpreted as showing that the contribution of GAT-1 to the pathophysiology of DWMI may be mediated by oligodendrocytes, and a similar situation may well arise for the ID seen in some forms of epilepsy. Also, the findings reported by Liu et al. (2015) could simply be interpreted as indicating that GAT-1 expression by microglia may be the direct mechanism by which the transporter contributes to the pathophysiology of Parkinson’s disease.
Author Contributions
GF, MM, and FC discussed the project, realized the figures, and wrote the article.
Funding
This work was supported by Ministero dell’Istruzione, dell’Università e della Ricerca (MIUR; 2015H4K2CR_002) and Università Politecnica delle Marche (PSA2018). We are indebted to the colleagues who collaborated in the original studies and to NC Brecha (Los Angeles, CA, USA) for providing the GAT-1 antibody.
Conflict of Interest
The authors declare that the research was conducted in the absence of any commercial or financial relationships that could be construed as a potential conflict of interest.
References
Bitanihirwe, B. K. Y., and Woo, T. U. W. (2014). Transcriptional dysregulation of γ-aminobutyric acid transporter in parvalbumin-containing inhibitory neurons in the prefrontal cortex in schizophrenia. Psychiatry Res. 220, 1155–1159. doi: 10.1016/j.psychres.2014.09.016
Borden, L. A. (1996). GABA transporter heterogeneity: pharmacology and cellular localization. Neurochem. Int. 29, 335–356. doi: 10.1016/0197-0186(95)00158-1
Borden, L. A., Dhar, T. G. M., Smith, K. E., Weinshank, R. L., Branchek, T. A., and Gluchowski, C. (1994). Tiagabine, SK & F 89976-A, CI-966, and NNC-711 are selective for the cloned GABA transporter GAT-1. Eur. J. Pharmacol. 269, 219–224. doi: 10.1016/0922-4106(94)90089-2
Braestrup, C., Nielsen, E. B., Sonnewald, U., Knutsen, L. J. S., Andersen, K. E., Jansen, J. A., et al. (1990). (R)-N-[4,4-bis(3-methyl-2-thienyl)but-3-en-yl]nipecotic acid binds with high affinity to the brain gamma-aminobutyric acid uptake carrier. J. Neurosci. 54, 639–647. doi: 10.1111/j.1471-4159.1990.tb01919.x
Bragina, L., Marchionni, I., Omrani, A., Cozzi, A., Pellegrini-Giampietro, D. E., Cherubini, E., et al. (2008). GAT-1 regulates both tonic and phasic GABAA receptor-mediated inhibition in the cerebral cortex. J. Neurochem. 105, 1781–1793. doi: 10.1111/j.1471-4159.2008.05273.x
Cai, K., Wang, J., Eissman, J., Wang, J., Nwosu, G., Shen, W., et al. (2019). A missense mutation in SLC6A1 associated with Lennox–Gastaut syndrome impairs GABA transporter 1 protein trafficking and function. Exp. Neurol. 320:112973. doi: 10.1016/j.expneurol.2019.112973
Carvill, G. L., McMahon, J. M., Schneider, A., Zemel, M., Myers, C. T., Saykally, J., et al. (2015). Mutations in the GABA transporter SLC6A1 cause epilepsy with myoclonic-atonic seizures. Am. J. Hum. Genet. 96, 808–815. doi: 10.1016/j.ajhg.2015.02.016
Cherubini, E., and Conti, F. (2001). Generating diversity at GABAergic synapses. Trends Neurosci. 24, 155–162. doi: 10.1016/s0166-2236(00)01724-0
Clark, J. A., Deutch, A. Y., Gallipoli, P. Z., and Amara, S. G. (1992). Functional expression and CNS distribution of a β-alanine-sensitive neuronal GABA transporter. Neuron 9, 337–348. doi: 10.1016/0896-6273(92)90172-a
Conti, F., Melone, M., De Biasi, S., Minelli, A., Brecha, N. C., and Ducati, A. (1998). Neuronal and glial localization of GAT-1, a high-affinity γ-aminobutyric acid plasma membrane transporter, in human cerebral cortex: with a note on its distribution in monkey cortex. J. Comp. Neurol. 396, 51–63. doi: 10.1002/(sici)1096-9861(19980622)396:1<51::aid-cne5>3.0.co;2-h
Conti, F., Melone, M., Fattorini, G., Bragina, L., and Ciappelloni, S. (2011). A role for GAT-1 in presynaptic GABA homeostasis? Front. Cell. Neurosci. 5:2. doi: 10.3389/fncel.2011.00002
Conti, F., Minelli, A., and Melone, M. (2004). GABA transporters in the mammalian cerebral cortex: localization, development and pathological implications. Brain Res. Rev. 45, 196–212. doi: 10.1016/j.brainresrev.2004.03.003
Conti, F., Zuccarello, L. V., Barbaresi, P., Minelli, A., Brecha, N. C., and Melone, M. (1999). Neuronal, glial, and epithelial localization of γ-aminobutyric acid transporter 2, a high-affinity γ-aminobutyric acid plasma membrane transporter, in the cerebral cortex and neighboring structures. J. Comp. Neurol. 409, 482–494. doi: 10.1002/(SICI)1096-9861(19990705)409:3<482::AID-CNE11>3.0.CO;2-O
Cope, D. W., Di Giovanni, G., Fyson, S. J., Orbán, G., Errington, A. C., Lrincz, M. L., et al. (2009). Enhanced tonic GABAA inhibition in typical absence epilepsy. Nat. Med. 15, 1392–1398. doi: 10.1038/nm.2058
Deken, S. L., Beckman, M. L., Boos, L., and Quick, M. W. (2000). Transport rates of GABA transporters: regulation by the N-terminal domain and syntaxin 1A. Nat. Neurosci. 3, 998–1003. doi: 10.1038/79939
Di Marco, B., M. Bonaccorso, C., Aloisi, E., D’Antoni, S., and V. Catania, M. (2016). Neuro-inflammatory mechanisms in developmental disorders associated with intellectual disability and autism spectrum disorder: a neuro-immune perspective. CNS Neurol. Disord. Drug Targets 15, 448–463. doi: 10.2174/1871527315666160321105039
Dikow, N., Maas, B., Karch, S., Granzow, M., Janssen, J. W. G., Jauch, A., et al. (2014). 3p25.3 microdeletion of GABA transporters SLC6A1 and SLC6A11 results in intellectual disability, epilepsy and stereotypic behavior. Am. J. Med. Genet. A 164A, 3061–3068. doi: 10.1002/ajmg.a.36761
Dodrill, C. B., Arnett, J. L., Shu, V., Pixton, G. C., Lenz, G. T., and Sommerville, K. W. (1998). Effects of tiagabine monotherapy on abilities, adjustment, and mood. Epilepsia 39, 33–42. doi: 10.1111/j.1528-1157.1998.tb01271.x
Fattorini, G., Catalano, M., Melone, M., Serpe, C., Bassi, S., Limatola, C., et al. (2020). Microglial expression of GAT-1 in the cerebral cortex. Glia 68, 646–655. doi: 10.1002/glia.23745
Fattorini, G., Melone, M., Sánchez-Gómez, M. V., Arellano, R. O., Bassi, S., Matute, C., et al. (2017). GAT-1 mediated GABA uptake in rat oligodendrocytes. Glia 65, 514–522. doi: 10.1002/glia.23108
Froestl, W. (2011). An historical perspective on GABAergic drugs. Future Med. Chem. 3, 163–175. doi: 10.4155/fmc.10.285
Fuhrer, T. E., Palpagama, T. H., Waldvogel, H. J., Synek, B. J. L., Turner, C., Faull, R. L., et al. (2017). Impaired expression of GABA transporters in the human Alzheimer’s disease hippocampus, subiculum, entorhinal cortex and superior temporal gyrus. Neuroscience 351, 108–118. doi: 10.1016/j.neuroscience.2017.03.041
Gong, X., Shao, Y., Li, B., Chen, L., Wang, C., and Chen, Y. (2015). γ-aminobutyric acid transporter-1 is involved in anxiety-like behaviors and cognitive function in knockout mice. Exp. Ther. Med. 10, 653–658. doi: 10.3892/etm.2015.2577
Guastella, J., Nelson, N., Nelson, H., Czyzyk, L., Keynan, S., Miedel, M. C., et al. (1990). Cloning and expression of a rat brain GABA transporter. Science 249, 1303–1306. doi: 10.1126/science.1975955
Inaba, T., Miyamoto, N., Hira, K., Ueno, Y., Yamashiro, K., Watanabe, M., et al. (2019). Protective role of levetiracetam against cognitive impairment and brain white matter damage in mouse prolonged cerebral hypoperfusion. Neuroscience 414, 255–264. doi: 10.1016/j.neuroscience.2019.07.015
Iwase, S., Bérubé, N. G., Zhou, Z., Kasri, N. N., Battaglioli, E., Scandaglia, M., et al. (2017). Epigenetic etiology of intellectual disability. J. Neurosci. 37, 10773–10782. doi: 10.1523/jneurosci.1840-17.2017
Jasmin, L., Wu, M. V., and Ohara, P. T. (2004). GABA puts a stop to pain. Curr. Drug Targets CNS Neurol. Disord. 3, 487–505. doi: 10.2174/1568007043336716
Johannesen, K. M., Gardella, E., Linnankivi, T., Courage, C., de Saint Martin, A., Lehesjoki, A.-E., et al. (2018). Defining the phenotypic spectrum of SLC6A1 mutations. Epilepsia 59, 389–402. doi: 10.1111/epi.13986
Kanner, B. I. (1978). Active transport of γ-aminobutyricacid by membrane vesicles isolated from rat brain. Biochemistry 17, 1207–1211. doi: 10.1021/bi00600a011
Keynan, S., Suh, Y. J., Kanner, B. I., and Rudnick, G. (1992). Expression of a cloned γ-aminobutyric acid transporter in mammalian cells. Biochemistry 31, 1974–1979. doi: 10.1021/bi00122a011
Kinjo, A., Koito, T., Kawaguchi, S., and Inoue, K. (2013). Evolutionary history of the GABA transporter (GAT) group revealed by marine invertebrate GAT-1. PLoS One 8:e82410. doi: 10.1371/journal.pone.0082410
Lai, C. T., Tanay, V. A. M. I., Charrois, G. J. R., Baker, G. B., and Bateson, A. N. (1998). Effects of phenelzine and imipramine on the steady-state levels of mRNAs that encode glutamic acid decarboxylase (GAD67 and GAD65), the GABA transporter GAT-1 and GABA transaminase in rat cortex. Naunyn. Schmiedebergs Arch. Pharmacol. 357, 32–38. doi: 10.1007/pl00005135
Lewis, D. A., and Gonzalez-Burgos, G. (2006). Pathophysiologically based treatment interventions in schizophrenia. Nat. Med. 12, 1016–1022. doi: 10.1038/nm1478
Liu, J., Huang, D., Xu, J., Tong, J., Wang, Z., Huang, L., et al. (2015). Tiagabine protects dopaminergic neurons against neurotoxins by inhibiting microglial activation. Sci. Rep. 5:15720. doi: 10.1038/srep15720
Liu, Q. R., López-Corcuera, B., Mandiyan, S., Nelson, H., and Nelson, N. (1993). Molecular characterization of four pharmacologically distinct γ-aminobutyric acid transporters in mouse brain. J. Biol. Chem. 268, 2106–2112.
Lynch, B. A., Lambeng, N., Nocka, K., Kensel-Hammes, P., Bajjalieh, S. M., Matagne, A., et al. (2004). The synaptic vesicle protein SV2A is the binding site for the antiepileptic drug levetiracetam. Proc. Natl. Acad. Sci. U S A 101, 9861–9866. doi: 10.1073/pnas.0308208101
Maglorius Renkilaraj, M. R. L., Baudouin, L., Wells, C. M., Doulazmi, M., Wehrlé, R., Cannaya, V., et al. (2017). The intellectual disability protein PAK3 regulates oligodendrocyte precursor cell differentiation. Neurobiol. Dis. 98, 137–148. doi: 10.1016/j.nbd.2016.12.004
Marcotulli, D., Fattorini, G., Bragina, L., Perugini, J., and Conti, F. (2017). Levetiracetam affects differentially presynaptic proteins in rat cerebral cortex. Front. Cell. Neurosci. 11:389. doi: 10.3389/fncel.2017.00389
Mattison, K. A., Butler, K. M., Inglis, G. A. S., Dayan, O., Boussidan, H., Bhambhani, V., et al. (2018). SLC6A1 variants identified in epilepsy patients reduce γ-aminobutyric acid transport. Epilepsia 59, e135–e141. doi: 10.1111/epi.14531
Melone, M., Barbaresi, P., Fattorini, G., and Conti, F. (2005). Neuronal localization of the GABA transporter GAT-3 in human cerebral cortex: a procedural artifact? J. Chem. Neuroanat. 30, 45–54. doi: 10.1016/j.jchemneu.2005.04.002
Melone, M., Ciappelloni, S., and Conti, F. (2014). Plasma membrane transporters GAT-1 and GAT-3 contribute to heterogeneity of GABAergic synapses in neocortex. Front. Neuroanat. 8:72. doi: 10.3389/fnana.2014.00072
Melone, M., Ciappelloni, S., and Conti, F. (2015). A quantitative analysis of cellular and synaptic localization of GAT-1 and GAT-3 in rat neocortex. Brain Struct. Funct. 220, 885–897. doi: 10.1007/s00429-013-0690-8
Melone, M., Cozzi, A., Pellegrini-Giampietro, D. E., and Conti, F. (2003). Transient focal ischemia triggers neuronal expression of GAT-3 in the rat perilesional cortex. Neurobiol. Dis. 14, 120–132. doi: 10.1016/s0969-9961(03)00042-1
Minelli, A., Brecha, N. C., Karschin, C., DeBiasi, S., and Conti, F. (1995). GAT-1, a high-affinity GABA plasma membrane transporter, is localized to neurons and astroglia in the cerebral cortex. J. Neurosci. 15, 7734–7746. doi: 10.1523/jneurosci.15-11-07734.1995
Minelli, A., DeBiasi, S., Brecha, N. C., Zuccarello, L. V., and Conti, F. (1996). GAT-3, a high-affinity GABA plasma membrane transporter, is localized to astrocytic processes and it is not confined to the vicinity of GABAergic synapses in the cerebral cortex. J. Neurosci. 16, 6255–6264. doi: 10.1523/jneurosci.16-19-06255.1996
Nägga, K., Bogdanovic, N., and Marcusson, J. (1999). GABA transporters (GAT-1) in Alzheimer’s disease. J. Neural Transm. 106, 1141–1149. doi: 10.1007/s007020050230
Pierri, J. N., Chaudry, A. S., Woo, T. U. W., and Lewis, D. A. (1999). Alterations in chandelier neuron axon terminals in the prefrontal cortex of schizophrenic subjects. Am. J. Psychiatry 156, 1709–1719. doi: 10.1176/ajp.156.11.1709
Posar, A., and Visconti, P. (2019). Mild phenotype associated with SLC6A1 gene mutation: a case report with literature review. J. Pediatr. Neurosci. 14, 100–102. doi: 10.4103/jpn.jpn_2_19
Sałat, K., Podkowa, A., Kowalczyk, P., Kulig, K., Dziubina, A., Filipek, B., et al. (2015). Anticonvulsant active inhibitor of GABA transporter subtype 1, tiagabine, with activity in mouse models of anxiety, pain and depression. Pharmacol. Rep. 67, 465–472. doi: 10.1016/j.pharep.2014.11.003
Savtchenko, L., Megalogeni, M., Rusakov, D. A., Walker, M. C., and Pavlov, I. (2015). Synaptic GABA release prevents GABA transporter type-1 reversal during excessive network activity. Nat. Commun. 6:6597. doi: 10.1038/ncomms7597
Schiavo, G., Shone, C. C., Bennett, M. K., Scheller, R. H., and Montecucco, C. (1995). Botulinum neurotoxin type C cleaves a single Lys-Ala bond within the carboxyl-terminal region of syntaxins. J. Biol. Chem. 270, 10566–10570. doi: 10.1074/jbc.270.18.10566
Schousboe, A., and White, H. S. (2009). “Glial modulation of excitability via glutamate and GABA transporters,” in Encyclopedia of Basic Epilepsy Research, ed. P. A. Scheartzkroin (Oxford: Academic Press), 397–401.
Scimemi, A. (2014). Structure, function, and plasticity of GABA transporters. Front. Cell. Neurosci. 8:161. doi: 10.3389/fncel.2014.00161
Shi, J., Cai, Y., Liu, G., Gong, N., Liu, Z., Xu, T., et al. (2012). Enhanced learning and memory in GAT1 heterozygous mice. Acta Biochim. Biophys. Sin. 44, 359–366. doi: 10.1093/abbs/gms005
Sundman-Eriksson, I., and Allard, P. (2002). [3H]Tiagabine binding to GABA transporter-1 (GAT-1) in suicidal depression. J. Affect. Disord. 71, 29–33. doi: 10.1016/s0165-0327(01)00349-4
Suzdak, P. D., and Jansen, J. A. (1995). A review of the preclinical pharmacology of tiagabine: a potent and selective anticonvulsant GABA uptake inhibitor. Epilepsia 36, 612–626. doi: 10.1111/j.1528-1157.1995.tb02576.x
Ueda, Y., Doi, T., Nagatomo, K., Tokumaru, J., Takaki, M., and Willmore, L. J. (2007). Effect of levetiracetam on molecular regulation of hippocampal glutamate and GABA transporters in rats with chronic seizures induced by amygdalar FeCl3 injection. Brain Res. 1151, 55–61. doi: 10.1016/j.brainres.2007.03.021
Verkhratsky, A., and Butt, A. (2013). Glial Physiology and Pathophysiology. Oxford: John Wiley & Sons.
Zafar, S., and Jabeen, I. (2018). Structure, function and modulation of γ-aminobutyric, acid transporter 1 (GAT1) in neurological disorders: a pharmacoinformatic prospective. Front. Chem. 6:397. doi: 10.3389/fchem.2018.00397
Keywords: GAT-1, GABA transporters, astrocytes, oligodendrocytes, microglia
Citation: Fattorini G, Melone M and Conti F (2020) A Reappraisal of GAT-1 Localization in Neocortex. Front. Cell. Neurosci. 14:9. doi: 10.3389/fncel.2020.00009
Received: 29 November 2019; Accepted: 13 January 2020;
Published: 13 February 2020.
Edited by:
Eleonora Palma, Sapienza University of Rome, ItalyReviewed by:
Annalisa Scimemi, University at Albany, United StatesEnrico Cherubini, European Brain Research Institute, Italy
Copyright © 2020 Fattorini, Melone and Conti. This is an open-access article distributed under the terms of the Creative Commons Attribution License (CC BY). The use, distribution or reproduction in other forums is permitted, provided the original author(s) and the copyright owner(s) are credited and that the original publication in this journal is cited, in accordance with accepted academic practice. No use, distribution or reproduction is permitted which does not comply with these terms.
*Correspondence: Fiorenzo Conti, Zi5jb250aUB1bml2cG0uaXQ=
† ORCID Giorgia Fattorini orcid.org/0000-0002-2497-2942
Marcello Melone orcid.org/0000-0003-4173-0774
Fiorenzo Conti orcid.org/0000-0001-5853-1566