- 1Laboratory for Neural Stem Cells and Functional Neurogenetics, Division of Multiple Sclerosis and Neuroimmunology, University of Connecticut Health Center, Farmington, CT, United States
- 2Center for Neurologic Diseases, Department of Neurology, Brigham and Women’s Hospital, Harvard Medical School, Boston, MA, United States
- 3Medical Scientist Training Program, University of California, Irvine, Irvine, CA, United States
- 4Department of Neurology, Columbia University Medical Center, New York City, NY, United States
- 5Institute for Medical Immunology, Charité–Universitätsmedizin Berlin, Berlin, Germany
- 6Alzheimer’s Clinical and Translational Research Center, Department of Neurology, Massachusetts General Hospital, Harvard Medical School, Boston, MA, United States
- 7Abu Haidar Neuroscience Institute, American University of Beirut Medical Center, Beirut, Lebanon
A central issue in regenerative medicine is understanding the mechanisms that regulate the self-renewal of endogenous stem cells in response to injury and disease. Interferons increase hematopoietic stem cells during infection by activating STAT1, but the mechanisms by which STAT1 regulates intrinsic programs in neural stem cells (NSCs) during neuroinflammation is less known. Here we explored the role of STAT1 on NSC self-renewal. We show that overexpressing Stat1 in NSCs derived from the subventricular zone (SVZ) decreases NSC self-renewal capacity while Stat1 deletion increases NSC self-renewal, neurogenesis, and oligodendrogenesis in isolated NSCs. Importantly, we find upregulation of STAT1 in NSCs in a mouse model of multiple sclerosis (MS) and an increase in pathological T cells expressing IFN-γ rather than interleukin 17 (IL-17) in the cerebrospinal fluid of affected mice. We find IFN-γ is superior to IL-17 in reducing proliferation and precipitating an abnormal NSC phenotype featuring increased STAT1 phosphorylation and Stat1 and p16ink4a gene expression. Notably, Stat1–/– NSCs were resistant to the effect of IFN-γ. Lastly, we identified a Stat1-dependent gene expression profile associated with an increase in the Sox9 transcription factor, a regulator of self-renewal. Stat1 binds and transcriptionally represses Sox9 in a transcriptional luciferase assay. We conclude that Stat1 serves as an inducible checkpoint for NSC self-renewal that is upregulated during chronic brain inflammation leading to decreased self-renewal. As such, Stat1 may be a potential target to modulate for next generation therapies to prevent progression and loss of repair function in NSCs/neural progenitors in MS.
Introduction
Transplantation of exogenous neural stem cells (NSCs) holds tremendous clinical potential evidenced by their success in ameliorating neurological diseases (Pluchino et al., 2003, 2005; Merzaban et al., 2015) in animals models and in humans in recent phase I trials (Pluchino et al., 2003; Warre-Cornish et al., 2020). In contrast, the repair potential of endogenous NSCs to injury and disease remains less explored. Initially, the self-renewal of endogenous NSCs (Molofsky et al., 2003) is increased by central nervous system (CNS) injury or acute inflammation, as seen early in multiple sclerosis (MS). However, aging and chronic neuroinflammation decrease NSC renewal and differentiation capacity over time (Imitola et al., 2003; Aharoni et al., 2005), thereby limiting the endogenous capacity of the brain for self-repair in MS. Such a pattern suggests a switch in the permissibility of the immune microenvironment from favorable to unfavorable to NSC self-renewal and differentiation, (Nait-Oumesmar et al., 2007; Pluchino et al., 2008; Wang et al., 2008; Rasmussen et al., 2011; Tepavčević et al., 2011). What exactly in the immune microenvironment or NSC response program triggers this switch, however, remains largely unknown.
One hypothesis for the lack of repair in chronic neuroinflammation is that NSCs and other progenitors like oligodendrocyte progenitor cells (OPCs) may participate in the immune microenvironment in MS by becoming activated direct targets of the immune response as they respond to inflammation by expressing immune-related molecules. The targeting may involve not only cell death but also cells intrinsic repair capacity dysfunction due to changes in the NSCs transcriptional programs (Imitola et al., 2004a; Kirby et al., 2019). Interferons have been shown to activate hematopoietic stem cell (HSC) self-renewal by inducing proliferation in response to infections (Essers et al., 2009; Baldridge et al., 2010); however, their role in the brain is still not clear. In particular, the IFN-γ/signal transducer and activator of transcription 1 (STAT1) pathway is an important participant in neuroinflammation. Recent work has found infiltrating T cells expressing IFN-γ in the aging subventricular zone (SVZ) NSC niche (Dulken et al., 2019), suggesting its effect in regulating NSC self-renewal. Indeed, IFN-γ regulates progenitor proliferation in vivo (Arien-Zakay et al., 2009), and activates Stat1 and p21 (Mäkelä et al., 2010) in NSCs. Furthermore, IFN-γ, through activation of its canonical STAT1 pathway, acts specifically on Nestin + progenitors to decrease progenitor proliferation and the number of cycling cells in a normal SVZ (Pereira et al., 2015). Moreover, a recent paper demonstrated type I interferon induces cell cycle arrest and quiescence of SVZ NSCs (Carvajal Ibañez et al., 2023). Still, the transcriptional targets of Stat1 and the direct role of Stat1 in the regenerative program of NSCs in neuroinflammation as seen in MS due to IFN-γ are still unknown.
Here, we investigate the role of Stat1 in the molecular program of NSCs and demonstrate Stat1 negatively controls self-renewal capacity in NSCs in a bidirectional manner during homeostasis and in response to inflammation. At a mechanistic level, we identify genes directly regulated by Stat1 in NSCs, including Sox9, through which Stat1 binds and transcriptionally represses this pro-neural stem cell gene (Scott et al., 2010). Our data suggest the inflammatory factors regulating self-renewal are stem cell-specific and are relevant to the search for novel therapies tailored to improve regeneration after chronic and progressive injury like MS.
Results
Expression of interferon-γ/Stat1 associated genes in SVZ NSCs
Because IFN-γ-expressing T cells infiltrate the aging SVZ, we hypothesized that this might trigger simultaneous increase in Stat1 expression in NSCs. To examine this possibility, we leveraged publicly available Smart-Seq2 single cell RNA-seq (scRNA-seq) data from young and old murine SVZ (Kalamakis et al., 2019); this dataset captures significantly more transcripts than any other scRNAseq datasets. We first examined whether the IFN-γ pathway is expressed in the SVZ. Except for minimal levels of Jak2, we find SVZ cell types express all members of the IFN-γ pathway, like SVZ astrocytes that are considered stem cells in the normal SVZ (Figure 1A). We next asked if Stat1 is increased in these cell types with age. Stat1 levels trend strongly toward increasing with age across all NSC populations, statistically significant on a subtype of quiescent NSCs (Figure 1B). Since Stat1 is a transcription factor, we next assessed expression of its direct target genes in the SVZ of young and old mice (promoter bound; FC > 2 with IFN-γ treatment) as defined by past ChIP-sequencing experiments (Dulken et al., 2019; Figure 1C). We found an upregulation of Stat1 target genes in older aNSCs and qNSC1, supporting the idea that inflammation associated with aging activate Stat1 and its downstream networks in the SVZ.
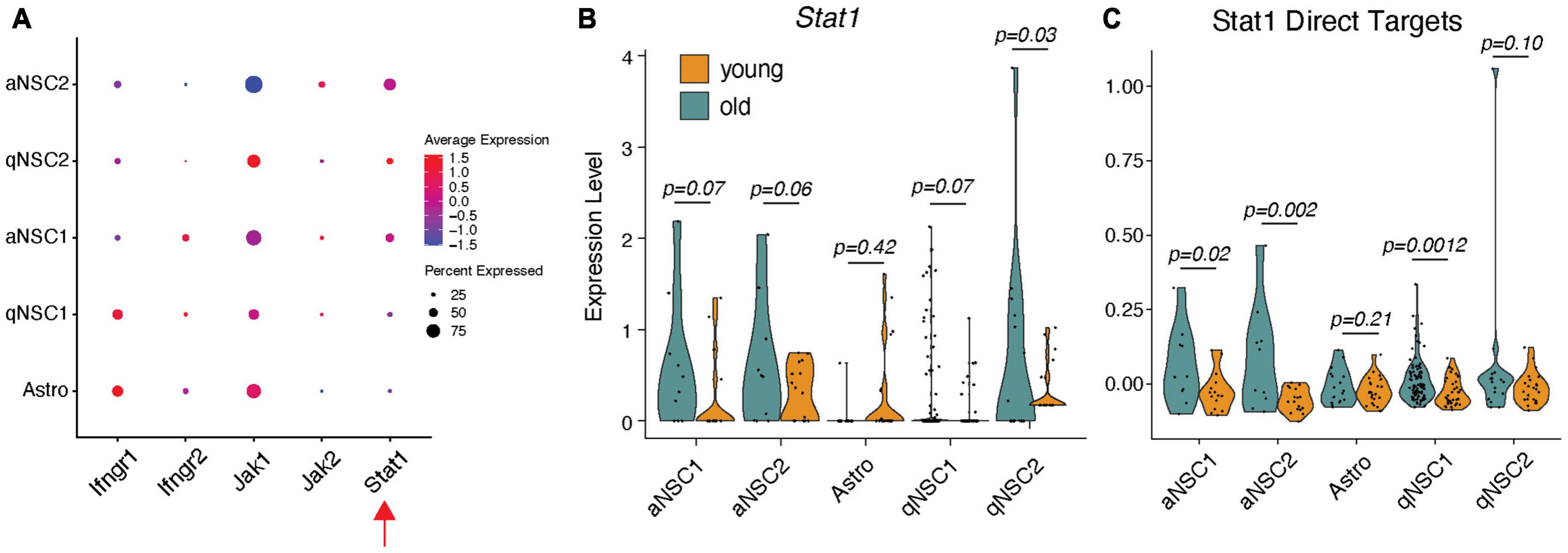
Figure 1. IFN-γR-Stat1 pathway activity in young and old mouse SVZ-NSCs. (A) Dot plot for gene expression of Stat1 pathway members by cell type using scRNA-seq data. (B) Cell-type-specific violin plots for Stat1 in different NSCs and (C) its direct transcriptional targets split by age. One-tailed t-tests. aNSC, activated NSC; Astro, astroglia; qNSC, quiescent NSCs.
Stat1 bidirectionally regulates NSC self-renewal
In a chronic model of MS, there is a decrease in NSC self-renewal in the SVZ (Pluchino et al., 2008) that is spatiotemporally correlated with increase in Stat1. To determine whether STAT1 plays a causative role in regulating self-renewal in NSCs, we delivered STAT1 to wild type NSCs isolated from the SVZ using a bicistronic retroviral vector LTR-STAT1-IRES-GFP containing the coding sequence of Stat1 and GFP (Lesinski et al., 2003). These two genes are simultaneously expressed in infected cells and a high expression of both genes is indicated by high GFP fluorescence. These NSCs were then FAC-sorted for GFP-positive single cells to ensure retroviral transduction, gene expression, and survival and plated at clonal dilution that prevent fusion of cells to accurately measure the formation of secondary neurospheres (Molofsky et al., 2003; Pluchino et al., 2008; Rasmussen et al., 2011). At clonal dilution, the resulting secondary GFP + neurosphere numbers were then counted after 7 days in vitro as a measure of self-renewal capacity (Molofsky et al., 2003; Pluchino et al., 2008; Wang et al., 2008; Rasmussen et al., 2011). Overexpression of STAT1 decreased neurosphere diameter and numbers compared to the control vector-infected NSCs (p < 0.0001; n = 3 from 2 independent experiments) (Figures 2A–C). In addition, there is a small increase in cell death in the STAT1-GFP NSCs compared to control (Supplementary Figure 1A) indicating a predominant effect in proliferation as the primary mechanism rather than cell death (Pereira et al., 2015).
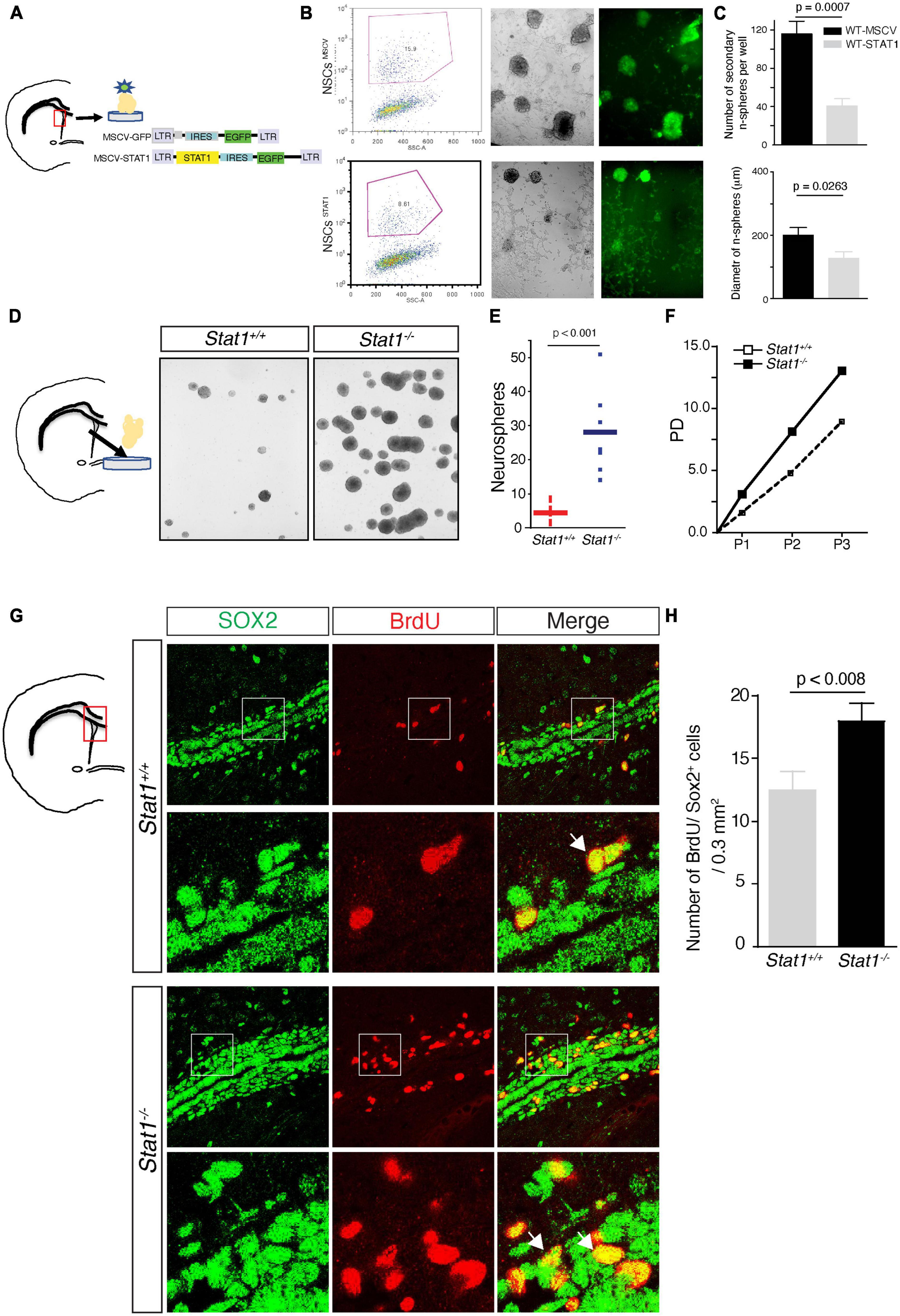
Figure 2. Self-renewal of NSCs after Stat1 overexpression or ablation. (A) Diagram showing the construction of retrovirus delivering Stat1 to NSCs and the isolation of SVZ NSCs (B) Secondary neurospheres infected with empty vector-GFP (MSCV) and Stat1-GFP vector (STAT1). NSCs were sorted and plated in self-renewing conditions, note the presence of GFP positive neurospheres in both conditions but diminished in GFP-STAT1 containing vector (C) quantification of secondary neurospheres numbers and diameter overexpressing STAT1, showing reduction of numbers and diameter compared to control n = 3 p < 0.0007 by unpaired t-test. Data is representative of two independent experiments. (D) Culture of secondary neurospheres from Stat1+/+ and Stat1–/– mice. (E) Quantification of number of neurospheres obtained from NSCs from Stat1–/– and Stat1+/+ mice there is a significant increase in the numbers of neurospheres, p < 0.001 by t-test. Data is representative of three independent experiments. (F) Quantification of population doublings, a measure of increased lifespan on NSCs from Stat1+/+ and Stat1–/– mice. (G) Representative figure of Incorporation of BrdU by Sox2 + neural progenitors in the SVZ of Stat1–/– and control adult brain by stereological methods and confocal microscopy (arrows). (H) Quantification of Sox2 + BrDU + neural progenitors in vivo in Stat1+/+ and Stat1–/– mice SVZ (n = 3) p < 0.008 by t-test. Bar: 50 μm.
We next asked whether depleting Stat1 from NSCs would have the opposite effect and increase NSC self-renewal. To test this, we measured the basal self-renewing capacity of adult NSCs isolated from the subventricular zone from Stat1–/– mice confirmed by the absence of Stat1 at the DNA and mRNA level in the isolated neurospheres (Supplementary Figures 1B, C). Under these conditions, using established methods (Molofsky et al., 2003; Pluchino et al., 2008; Wang et al., 2008; Rasmussen et al., 2011) revealed an increase in the number of secondary neurospheres (p < 0.001) and in Stat1–/– NSC population doubling (Figures 2D–F). To demonstrate the specificity of this effect, we delivered the same retroviral vector LTR-STAT1-IRES-GFP to Stat1–/– NSCs, sorted them at clonal dilution, and counted them 7 days later. Stat1 overexpression rescued the reduction in NSC self-renewal caused by Stat1–/– knockout (p < 0.001) (Supplementary Figures 1D–F).
Having established its role in vitro by isolation of SVZ NSCs, we then asked if Stat1 regulates NSC proliferation in vivo. To do this, we performed BrdU injections in adult Stat1–/– mice (that are viable and normal in appearance) and controls. BrdU was injected for 7 days and then the mice were sacrificed. Of note, these concentrations of BrDU does not change the in vivo outcomes and is widely used as reported by many groups (Pluchino et al., 2008; Li et al., 2010; Rasmussen et al., 2011; Starossom et al., 2019). We used Sox2, an established marker mostly expressed in NSCs and minimally in transient neural progenitors. We quantified the number of Sox2/BrdU-positive NSCs in the normal adult SVZ and rostral migratory stream (RMS). We found that Stat1 depletion increases the number of proliferating BrdU/Sox2 + NSCs in the SVZ (p < 0.008 n = 3 mice per group), confirming the in vitro observations (Figures 2G, H). These data demonstrate a bidirectional role of Stat1 in controlling NSC self-renewal, meaning increases of Stat1 leads to decreased NSCs self-renewal and decreases of Stat1 leads to increase in self-renewal in vitro and proliferation in vivo.
Stat1 knockout increases sensitivity to niche signals, self-renewal, and differentiation
In vivo, NSCs respond to niche signals to maintain self-renewal capacity. Our in vivo and in vitro data suggest that Stat1-deficient NSCs may respond differentially to known SVZ niche cytokines (Jackson et al., 2006; Kalani et al., 2008). To investigate the responses of NSCs from Stat1–/– mice to niche cytokines, we exposed the isolated Stat1–/– NSCs to self-renewal signals in vitro. In response to EGF and FGF-2, Stat1–/– NSCs exhibited more stabilized β-catenin, increased Akt phosphorylation, and GSK-3β inactivation demonstrated by increased phosphorylation GSK-3β at serine 9 (S9) (Figure 3A). Next, we tested the responses of Stat1–/– NSCs to PDGF-β (Jackson et al., 2006) and Wnt-3a (Kalani et al., 2008), both of which increase self-renewal and neurogenesis in the SVZ (Kalani et al., 2008; Favaro et al., 2009). In response to these cytokines, Stat1–/– NSCs increased the number of secondary neurospheres (p < 0.05) (Figures 3B, C), indicating that the machinery important for SVZ cytokine-induced self-renewal by PDGF and Wnt3 is not only functional, but increased in Stat1–/– NSCs in response to PDGF-β and Wnt-3a.
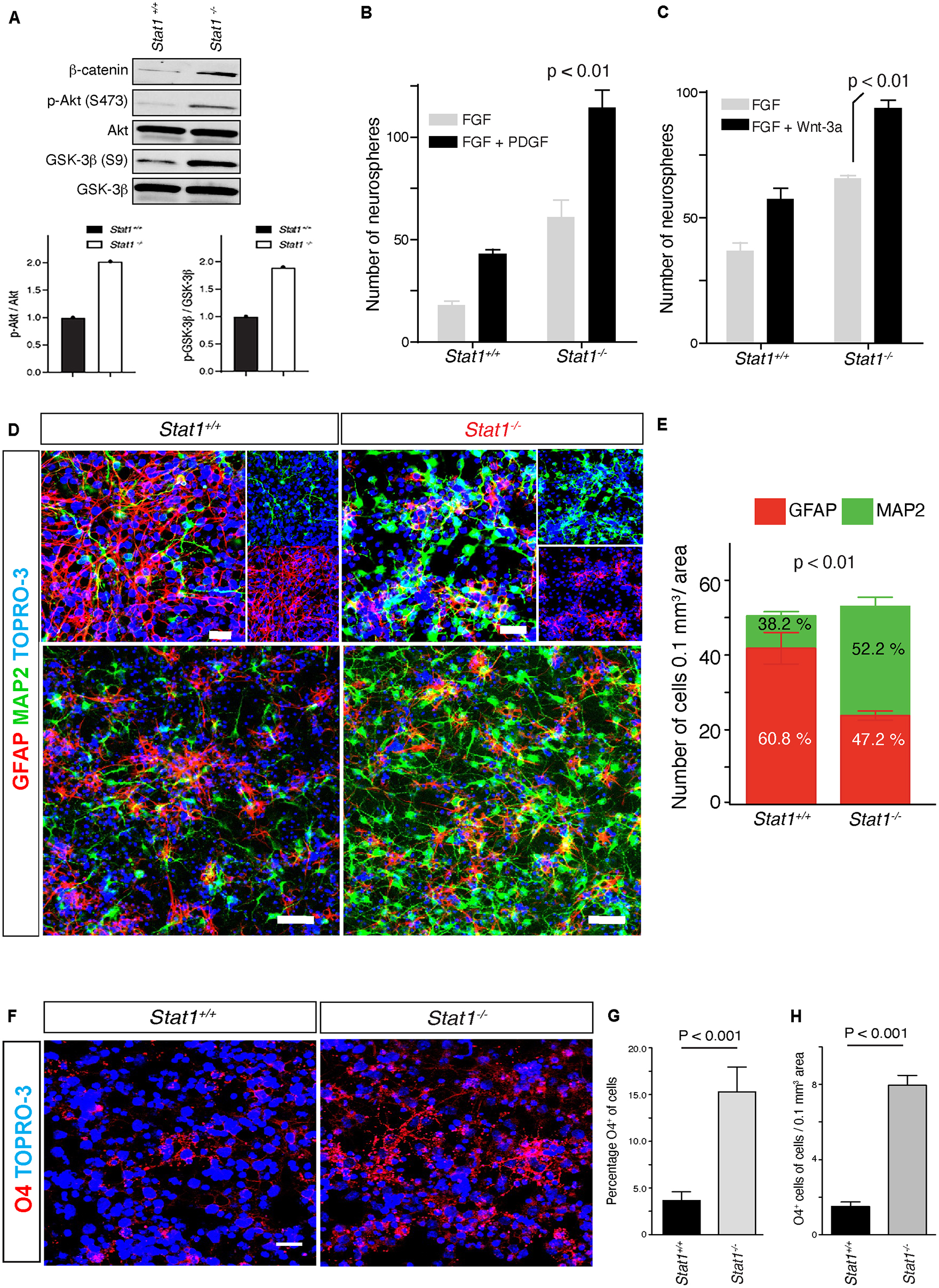
Figure 3. Stat1-deficient NSCs exhibit increased responses to SVZ self-renewal signals and differentiation in vitro. (A) Western blot and quantification of β-catenin expression, Akt phosphorylation and GSK-3β activation from Stat1–/– vs Stat+/+ NSCs. (B,C) Quantification of self-renewal response in the presence of PDGF-β and Wnt3 in Stat1+/+ and Stat1–/–, NSCs from Stat1+/+ and Stat1–/– mice, there is an increase response in Stat1–/– NSCs p < 0.01 (D) Confocal microscopy of differentiation of neurospheres from Stat1+/+ and Stat1–/– stained with (MAP2 (green) and GFAP (red) there is an increased in the numbers of MAP2 positive cells in the Stat1–/– NSCs cultured in NSCs differentiation medium. Top panel: higher magnification Lower panel: Lower magnification of different area. (E) Quantification of numbers of MAP2 and astrocytes in differentiating NSCs from Stat1+/+ and Stat1–/– showing an increased in the numbers of differentiated progenitors with increased in neurogenesis p < 0.001 by t-test. Bar: 50 μm (n = 3, representative of 3 experiments). (F) Confocal microscopy of differentiation of neurospheres from Stat1+/+ and Stat1–/– stained with O4 (red) and TOPRO-3 (blue) there is an increased in the numbers of O4 + positive cells in the Stat1–/– NSCs cultured in NSCs differentiation medium. (G,H) Quantification of numbers of O4 + progenitors in differentiating NSCs from Stat1+/+ and Stat1–/– showing an increased in the numbers and percentage of differentiated oligodendrocyte progenitors p < 0.001 by t-test. Bar: 10 μm.
Next, we studied the differentiation of NSCs in the absence of Stat1 by plating similar number of NSCs in PLL-coated dishes in neurobasal differentiation medium that halts proliferation and initiates differentiation. Seven days after plating, we counted the number of newborn neurons and differentiating astrocytes in culture. Stat1 deletion induced a relative increase in the number of MAP2 + neurons and reduced the number of GFAP + astrocytic cells (p < 0.05) (Figures 3D, E) compared to controls as shown by confocal. We confirmed this increased neurogenesis by staining for doublecortin (a marker of neuroblasts) and found a statistically significant increase in doublecortin-positive cells (p < 0.05) (Supplementary Figures 2A, B). We also observed an increase in O4 + oligodendrocyte precursor percentage and numbers per area in the Stat1-deficient NSCs (p < 0.001) (Figures 3F–H). Together, these data suggest Stat1 negatively regulates neuronal and oligodendroglia differentiation.
Stat1 is induced in the SVZ during EAE and correlates with increased presence of IFN-γ-producing T cells in the meninges and cerebrospinal fluid (CSF)
Aging is associated with inflammation of the SVZ niche characterized by the activation of Stat1 signaling on SVZ NSCs and the presence of T cells, suggesting that aging induces inflammation of the SVZ niche mediated by IFN-γ-producing T cells (Dulken et al., 2019). T cell cytokines in the cerebrospinal fluid (CSF) may target NSCs, since these cells (type B NSCs) protrude part of their cytoplasm into the ventricle (Lehtinen et al., 2011; Warre-Cornish et al., 2020; Supplementary Figure 3A). Consistent with this, we found that interferon-γ-associated genes are expressed in the NSCs in the SVZ (Supplementary Figures 3B, C), consistent with the role of IFN-γ in the aging SVZ (Dulken et al., 2019).
Next, we asked if Stat1 is likewise activated under disease conditions, such experimental autoimmune encephalomyelitis (EAE), a model of MS. We have previously found that Stat1 is increased in the SVZ in MOG-EAE (Pluchino et al., 2008). To confirm these observations in a different EAE model, we first examined Stat1 gene expression in the isolated SVZ SJL mice showing a neurological score of >2. In these mice, Stat1 increased two-fold and nine-fold in the SVZ during the acute (day 15) and chronic phases of EAE (day 30 post-immunization) (Supplementary Figure 3D). To confirm these gene expression observations at the protein level, we used NSC-specific markers. We found increased expression of STAT1 protein in Nestin + NSCs specifically in NSC cytoplasm and nuclear regions, by using quantitative confocal imaging with pixel measurements during EAE in the PLP 139–151 model in vivo (Figures 4A, B). We confirmed these findings by using Sox2, a marker for SVZ neural stem and progenitor cells repeating the quantitative confocal measurements showing similar findings (Figures 4C, D).
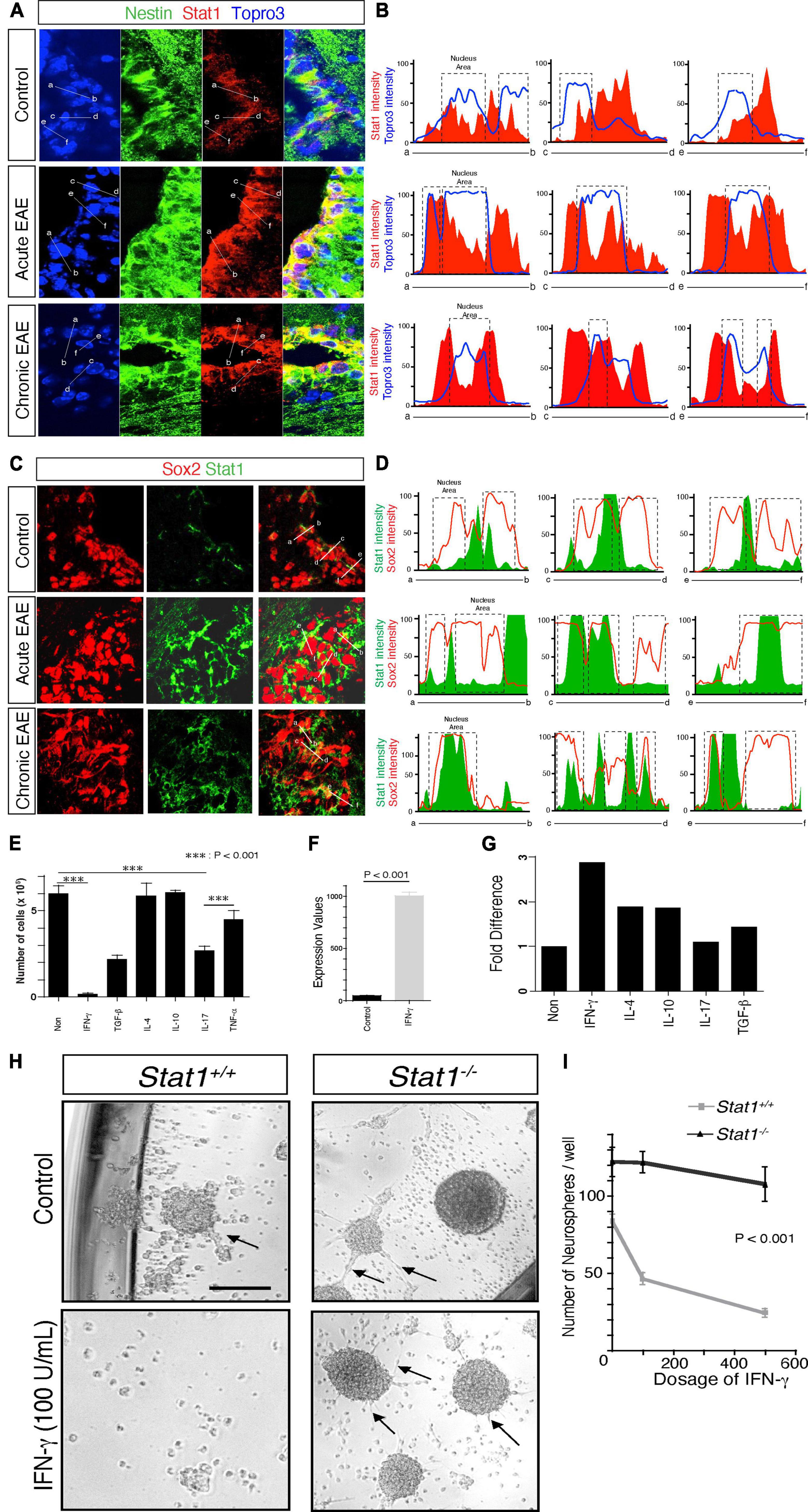
Figure 4. Increased Stat1 mediates IFN-γ alteration of self-renewal in NSCs. (A) Expression of STAT1 in nestin + positive NSCs from the SVZ in control, acute and chronic EAE in SJL mice. (B) Confocal quantitative pixel measurements of STAT1 in nestin + NSCs, indicating cytoplasmic and peri and nuclear increase of STAT1. (C) Expression of STAT1 in Sox2 positive NSCs from the SVZ in control, acute and chronic EAE in SJL mice. (D) Confocal quantitative pixel measurements of Stat1 in Sox2 positive NSCs, indicating an increase in STAT1 in cytoplasmic and peri and nuclear areas, Sox2 is highly expressed in the nucleus and colocalize with STAT1. (E) Quantification of proliferation of NSCs exposed to various cytokines, quantified at day 7 by thymidine incorporation, n = 3. p < 0.0001 by t-test. (F) Expression of Stat1 by PCR of NSCs derived from the SVZ after exposure to IFN-γ 100 u/ml (G) Quantitative PCR analysis of p16/ink4 after exposure to cytokines. there is a threefold increase in the expression of p16/ink4 after exposure to IFN-γ and other cytokines (H) Microscopy of cultured neurospheres from Stat1+/+ and Stat1–/– SVZ cells in the absence or presence of 100 U/ml IFN-γ. (I) Quantification of neurospheres from Stat1–/– and Stat1+/+ mice exposed to IFN-γ. NSCs from Stat1+/+ exhibited a reduction of the numbers of neurospheres that is dose dependent compared to Stat1–/– NSCs that are resistant to the effects of IFN-γ reduction in size and numbers, and the colonies were smoother with more spontaneous formation of chain migration (arrows) (p < 0.001) n = 4 independent experiments. Bar: 100 μm.
Next, we hypothesized that increased STAT1 signaling in our EAE SJL model may correlate with the presence of infiltrating T cells. To test this, we measured the frequency of IFN-γ+ and IL17+ CD4 + cells in the meninges and ventricular CSF obtained from SJL mice. There was a significant increase in the number of IFN-γ-producing cells during the acute and chronic phases of EAE compared to IL-17 producing cells (p < 0.001) in the CSF and meninges (Supplementary Figure 3E). To confirm this observation as a broader pathological mechanism in EAE, we used MOG EAE adoptive transfer. We already demonstrated that Stat1 is increased in the SVZ of EAE mice (Pluchino et al., 2008). To correlate this increased expression with IFN-γ + producing cells, we measured the frequency of antigen-specific IFN-γ + and IL-17 + cells in the meninges and CSF by adoptive transfer of 2D2 transgenic cells in mice with significant clinical scores > 2. The frequency of MOG-specific T cells producing IFN-γ was significantly higher than of IL17 + transgenic cells, suggesting that IFN-γ is a predominant cytokine affecting the SVZ niche in EAE in both models (Pluchino et al., 2008; Rasmussen et al., 2011; Supplementary Figure 3F).
An interferon-γ-inducible Stat1 program mediates decreased NSC self-renewal
Despite the role of IL-17 producing cells in the pathogenesis of EAE (Kebir et al., 2007; Steinman, 2007), our observations suggest that IFN-γ may play a more prominent role on NSCs in vivo. To investigate this further, we exposed NSCs to a panel of cytokines in vitro for 7 days and measured their number. We found that IFN-γ suppressed the proliferation of NSCs significantly more than IL-17 (p < 0.0001) (at the standard effective doses of both cytokines) or other cytokines such as IL-10 and IL-4 (Figure 4E). At these dosages, IFN-γ increases the expression of Stat1 and p16 in NSCs associated with cell cycle arrest (Figures 4F, G). Next, we extended these in vitro observations by examining the exposure to IFN-γ in NSC self-renewal. First, we found a dose-dependent decrease in the self-renewal capacity of NSCs at 100 U/ml, when STAT1 phosphorylation was activated (Supplementary Figures 4A, B). IFN-γ did not affect the levels of apoptosis (Supplementary Figure 4C), a result consistent with effects on proliferation that has been shown before (Pereira et al., 2015). In addition, there was an increase in Tapasin-1 (an interferon gene involved in MHC class I function) and downregulation of tenascin (a gene involved in stem cell renewal) (Garcion et al., 2004; Supplementary Figures 4D, E). These mRNA changes were reflected at the protein level. Tenascin-C was downregulated by IFN-γ (p < 0.008) and Tapasin (p < 0.008) was upregulated by IFN-γ at the protein level as revealed by confocal microscopy (Supplementary Figure 4F). More notably, we cultured early and late passage NSCs under IFN-γ for 9–12 weeks to mimic a chronic neuroinflammatory microenvironment and observed a decrease in the population doubling with lack of formation of neurospheres more pronounced in younger NSCs than late culture NSCs indicating that despite in vitro adaptation NSCs were susceptible to IFN-γ. This effect was partially reversed after IFN-γ was withdrawn from the culture. These data suggest an effect of IFN-γ on NSC lifespan that persists even in the absence of additional IFN-γ (Supplementary Figures 4G, H). To determine the specificity of Stat1 in mediating the effects of IFN-γ, we cultured NSCs from Stat1–/– and WT with IFN-γ. NSCs from WT cultured with IFN-γ showed a dose-dependent reduction of self-renewal capacity (Figure 4H) while Stat1–/– NSCs were resistant to IFN-γ (p < 0.001, of note the neurospheres in the Stat1–/– were smoother with more spontaneous formation of chain migration of neural progenitors (Figure 4I).
Stat1-deficient NSCs increases the expression of genes for self-renewal and differentiation
To determine the molecular impact of Stat1 deletion as a model for a potential therapeutic intervention on NSCs, we performed microarray analysis on WT and Stat1–/– NSCs. Despite being a transcription factor, Stat1 deletion induced a limited differential gene expression in NSCs (Figure 5A). Among the top differentially expressed genes (DEGs), we found high expression of Sox9 (important in NSC self-renewal) and Zic1 (important for neurogenic differentiation) (Figure 5B). Confirmatory qPCR validated our microarray results for several of these genes with Sox9 being the top upregulated gene (Figure 5C). Gene ontology analysis for DEGs showed an enrichment in cell cycle regulation, dentate gyrus development, where adult neurogenesis occurs, and neuronal processes, like axonal transport (Figure 5D). We next performed protein-protein interaction (PPI) analysis on DEGs, we found our PPI network to include significantly more interactions than expected (p = 0.00383), suggesting these DEGs are biologically connected including Zic1-Sox9 (Figure 5E). Finally, we explored the location of the increased gene expression signature using the Allen Gene Expression Database1 to confirm the regional and temporal expression of increased genes in Stat1 deleted cells to NSC areas by in situ hybridization patterns. This data was available in the developing embryonic mouse brain. While Gria4 showed predominant expression in the cortical plate of the expanding cortex, Zic1 and Sox9 expression localized to areas of NSC proliferation, such as the SVZ and olfactory bulb (Figures 5F-H). To examine their expression in the adult mice SVZs, we leveraged the scRNA-seq dataset of adult SVZ NSCs (Kalamakis et al., 2019). Among the differential expressed genes, we found high levels of Sox9 expression across all NSCs types including astroglia cells in the SVZ (Figure 5I).
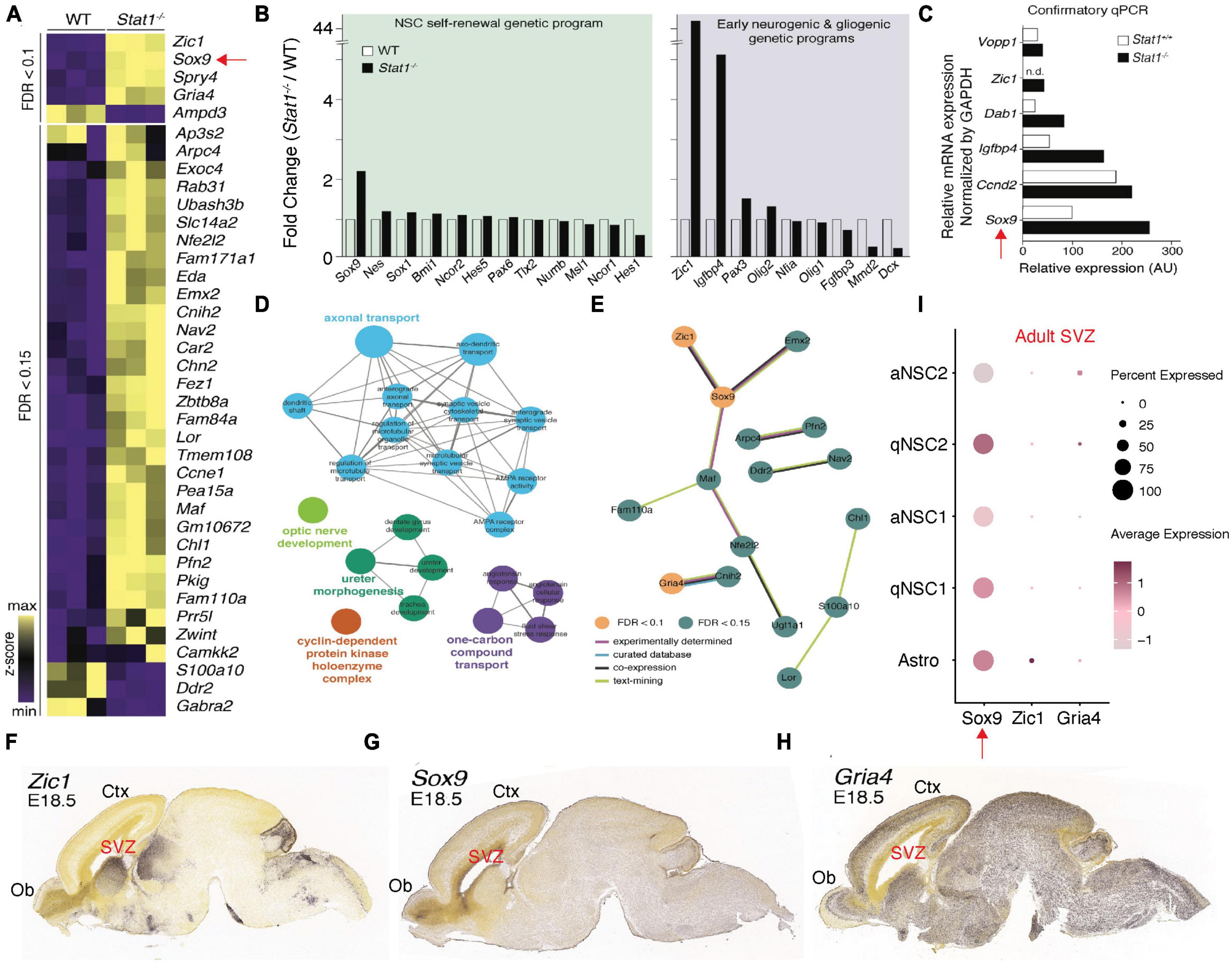
Figure 5. Differential expression of self-renewal and neurogenic genes following Stat1 deletion. (A) Heatmap of differentially expressed genes (DEGs) between Stat1+/+ and Stat1–/– NSCs at FDR < 0.1 and 0.15, respectively. (B) Fold change (Stat1–/–/Stat1+/+) of genes underlying NSC self-renewal, and early neurogenic and gliogenic programs. (C) Plot of confirmatory PCR values in Stat1+/+ and Stat1–/– NSCs for selected genes using GADPH as control (D) Gene ontology enrichment for DEGs at FDR < 0.15. Node sizes reflect level of statistical significance and are colored by group. (E) PPI network of DEGs with only interacting proteins being shown. Nodes are colored by FDR threshold and edges by evidence source. Data derived from StringDB. (F–H) In situ hybridization images of the developing mouse brain at E18.5 with probes for (F) Zic1, (G) Sox9, and (H) Gria4 from the Allen Brain Atlas. (I) Single cell RNAseq Dot plot for Sox9, Zic1, and Gria4 expression in the adult SVZ by cell type indicating high expression of Sox9 in all SVZ NSCs and SVZ astrocytes.
Stat1 transcriptionally represses Sox9
Sox9 is a well-known transcriptional regulator of self-renewal in NSCs (Scott et al., 2010). However, the signals that regulate the expression of Sox9 in NSCs are unknown. We study whether physiological and pathological states regulate the expression of Sox9 in NSCs. Indeed, we find that Sox9 expression positively correlates with signals such as hypoxia associated with increased self-renewal. On the contrary, pathological signals associated with decreases self-renewal such as IFN-γ and starvation decreases Sox9 expression (Figures 6A-C). Furthermore, we investigated whether the expression of Stat1 target genes like Stat1 and Irf1 where dependent of Stat1, we showed that these genes are dependent of the presence of Stat1 in NSCs (Figures 6C, D). Next, we asked for the mechanisms of Stat1 regulation of Sox9 in NSCs. Because of the upregulation of Sox9 upon Stat1 depletion, we hypothesized Stat1 may directly regulate Sox9 expression. To test this, we performed qPCR for Sox9 in WT and Stat1-deficient cells response to IFN-γ. We found that in the presence of IFN-γ, Sox9 only was downregulated in WT cells, but not Stat1-depleted cells, suggesting that Stat1 may directly regulate Sox9 at the transcriptional level (Figure 6F). To test this hypothesis, we searched the Sox9 promoter for potential binding sites for Stat1 using the Biobase database. We identified overlapping putative binding sites for Stat1 upstream of the transcription start site (TSS) of Sox9 in highly conserved area among vertebrates (Figure 7A). We validated three putative sites of potential binding of Sox9 as a direct target of Stat1 in NSCs by performing chromatin immunoprecipitation followed by qPCR (ChIP-qPCR) in NSCs exposed to IFN-γ. Primer sets flanking the Stat1 binding at three sites (Site1-3) in the Sox9 promoter were designed to amplify the immunoprecipitated ChIP DNA by qPCR. We observed a significant increase in Stat1 binding to these three sites in the Sox9 promoter in NSCs exposed to IFN-γ (Figure 7B). To analyze the functional relevance of the binding of Stat1 to their target sequences in the Sox9 locus, we investigated the ability of Stat1 to regulate the activity of the Sox9 promoter in reporter assays. We used four reporter constructs pGL3-Sox9 containing the firefly luciferase gene under the control of the Sox9 promoter. It should be noted that these vectors span multiple conserved genomic regions in Sox9 that are highly conserved among Human, Rhesus, Mouse, and Dog that include all our binding sites for Stat1. Notably, we found that Stat1 was able to transcriptionally repress all the Sox9-luciferase constructs, especially a highly conserved region near the transcriptional start site that is highly conserved during evolution (Figure 7C).
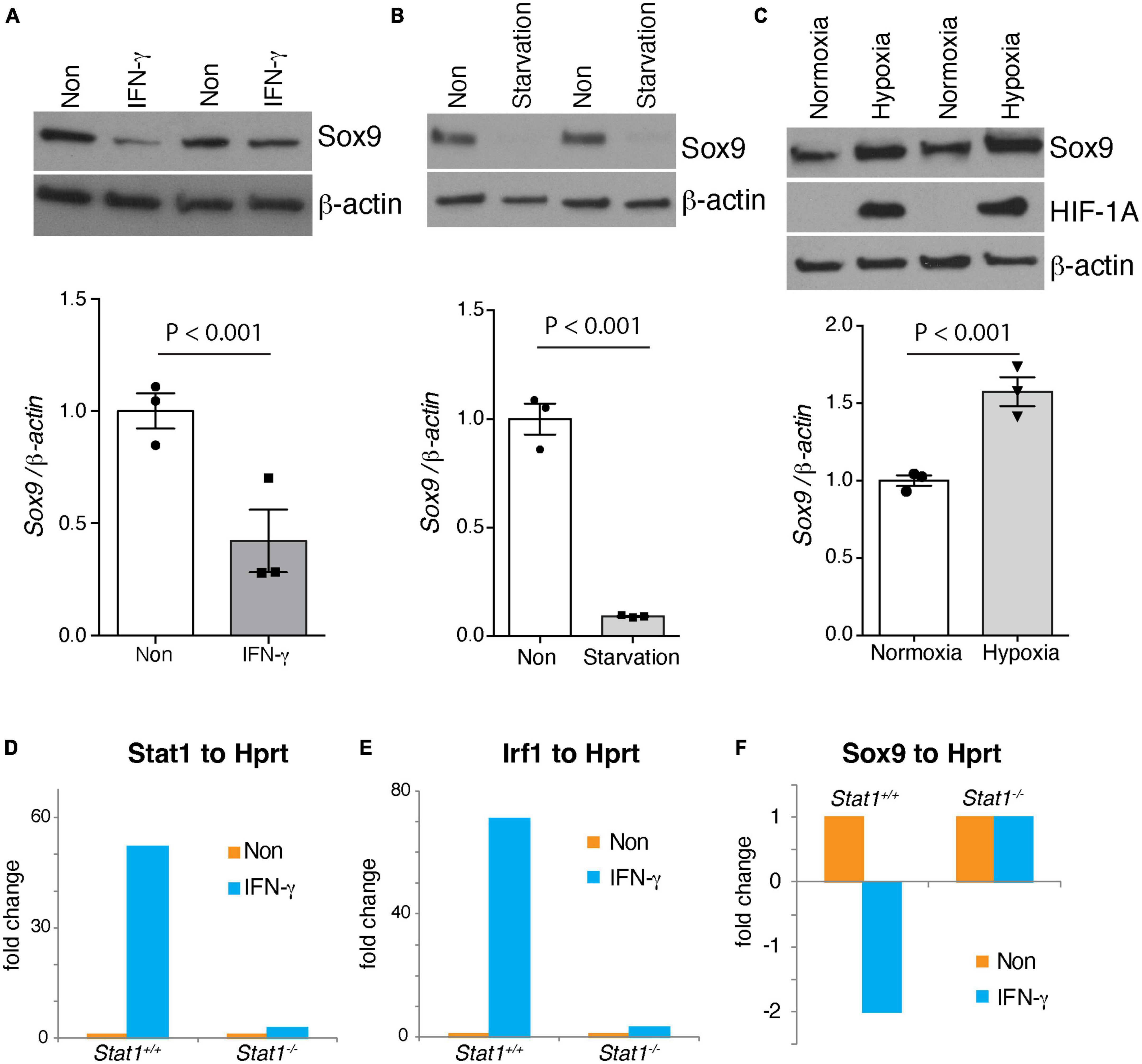
Figure 6. Regulation of Sox9 NSCs and Stat1 dependency of IFNγ effects. Western blot (top) of physiological and pathological signals regulating the SVZ, shown as technical duplicates. (A) IFNγ (B) NSCs starvation (C) and hypoxia differentially regulates Sox9 levels. (Bottom) shows quantification of Sox9 protein levels using β-actin as control, showing statistical significance differences. IFNγ and starvation of NSCs reduces Sox9 levels, while hypoxia increase Sox9 expression (D,E) Stat1 dependency of the increase of Stat1 and Irf1 gene expression by IFNγ, (F) Stat1 dependency of the reduction of Sox9 expression by IFNγ in NSCs.
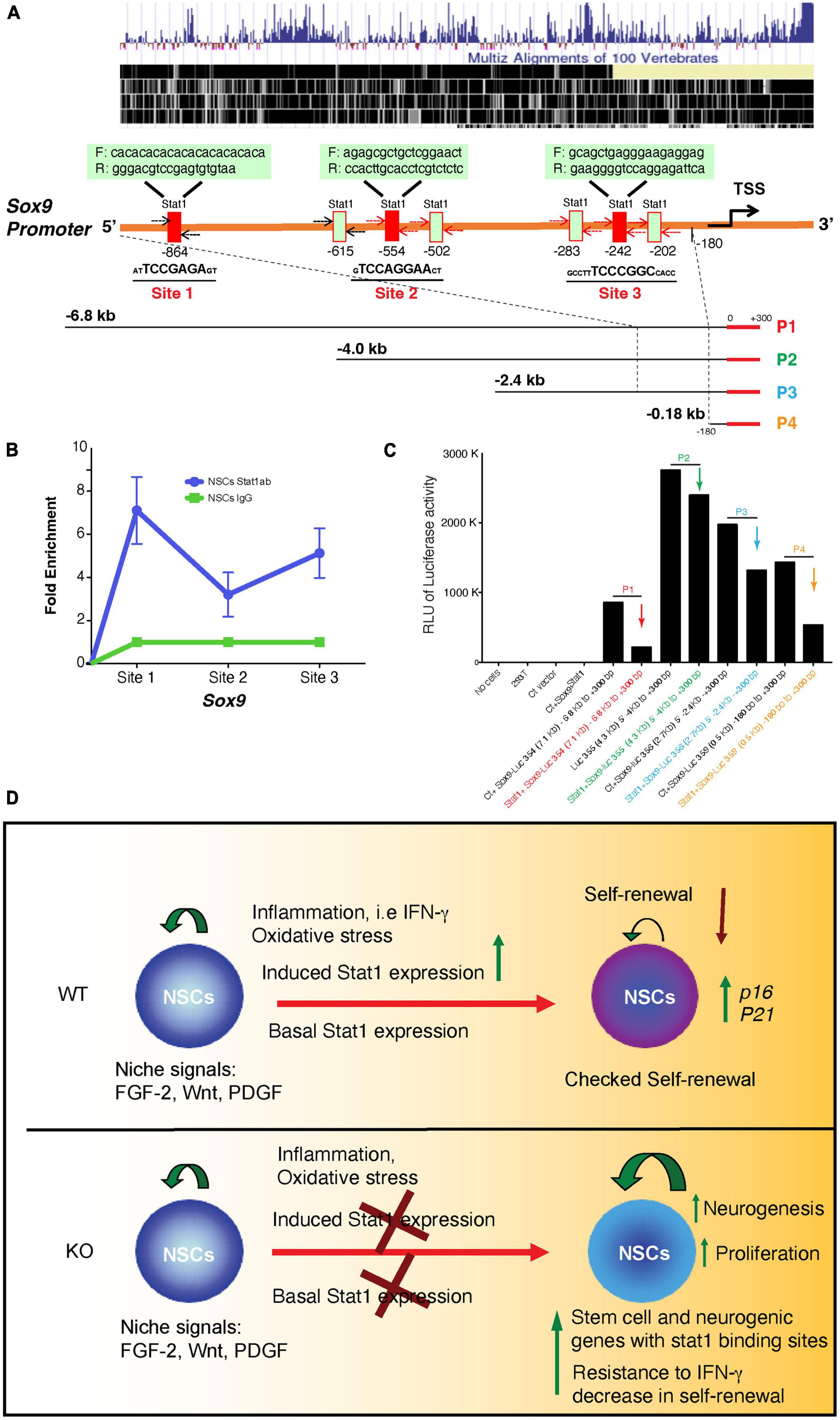
Figure 7. Direct interaction of Stat1 and Sox9 and transcriptional repression in a luciferase assay and model. (A) Conserved map of the endogenous sox9 promoter among vertebrates and Schema of positions of seven and putative Stat1 binding sites within the mouse Sox9 promoter. Three candidate binding sites were selected (underlined). Primer sets used in the PCR assays for the detection of Stat1 binding to the Sox9 promoter using the ChIP products are shown. (B) ChIP analysis of Stat1 binding to the three sites of the Sox9 promoter. Antibodies used for IP are anti-Stat1 and control IgG. Total input DNA before IP was used for normalization of data. Data are represented as fold enrichment. (C) Luciferase assay of four different Sox9 promoter regions targeted by Stat1 construct (P1-P4) demonstrating transcriptional repression. HEK 293T cells were transfected with a constant amount of four different Sox9 promoter-luciferase plasmids encoding four different regions of the Sox9 promoter as indicated (P1, P2, P3, and P4) with or without Stat1 vectors. Cells were lysed 24 h. later and luminescence was measured, data is representative of three independent experiments. (D) Model of the role of Stat1 in NSCs during physiological and neuroinflammation conditions, in the presence of chronic inflammation (i.e., chronic MS), oxidative stress and inflammatory cytokines (i.e., IFN-γ) upregulate STAT1 expression in NSCs leading to decreased self-renewal capacity, in addition to increase in senescence genes such as p16 and decreased expression of stem cell genes such as Tnc, Sox9 (top panel) as shown in our data. In the absence of STAT1, the NSCs self-renewal capacity is increased and deletion increased neurogenesis and self-renewal and neurogenic gene expression (our data). During inflammation (i.e., IFN-γ increased) STAT1 deletion may associated with resistance to IFN-γ deleterious effects.
Discussion
A central unanswered question in regenerative neuroimmunology is the mechanisms by which NSCs are activated or inactivated to initiate or halt repair during disease and how this process changes over time (Poss, 2010). Identification of the genes and molecules differentially induced by acute versus chronic states is a critical step toward developing therapies for lasting neural regeneration. Complementary to the use of exogenous NSCs, the investigation into the pathways that control endogenous NSCs during repair is more limited. For instance, the search for genes that control self-renewal capacity of stem cells like NSCs has largely focused on cell type-specific enriched genes through comparative transcriptome analysis between diverse populations of stem cells and adult tissue (Ramalho-Santos et al., 2002; Suslov et al., 2002). This approach has been successful in identifying genes that, by nature of their high expression in NSCs, have a dominant function during development and adult homeostasis. However, this approach overlooks inducible genes and programs in NSCs activated only during disease states that can change the NSCs internal molecular programs at the genetic and epigenetic level.
Our study provides new evidence of the mechanistic role of the IFNγ-receptor-Stat1 pathway in SVZ-derived NSCs in neuroinflammation. New data in pathological situations like aging involves Type II interferon in NSC modulation by T cells (Dulken et al., 2019), but the interplay between Type I and II interferon during normal and pathological states and SVZ function is still unclear. The situation during pathology — especially neuroinflammation models — may be different. For instance, in EAE there is a profound alteration in the SVZ cells that leads to a reduction of NSC self-renewal in vivo and in vitro with reduction in neurogenesis in chronic EAE; this has been shown by our group and others (Pluchino et al., 2008; Rasmussen et al., 2011; Tepavčević et al., 2011). There are major transcriptional changes in the SVZ at the molecular level during inflammation in EAE that generate sustained disease-associated programs in the SVZ in chronic stages of the disease. These changes induced by chronic inflammation are orders of magnitude higher compared to the physiological function of interferons in the normal SVZ (Starossom et al., 2011, 2019). Therefore, Type II interferon may change the transcriptional landscape of the SVZ with aberrant and sustained molecular programs by chronic exposure.
Our data show that NSCs self-renewal capacity can be modulated by IFNγ, a cytokine from the inflamed environment that induces an abnormal transcriptional program in NSCs, including an IFNγ receptor-Stat1-Sox9 pathway. By taking such an approach to identify inducible molecules, we find that during normal homeostasis Stat1 is a negative regulator of NSCs in response to normal niche molecules that may include small amounts of IFN-γ, as shown previously (Li et al., 2010). However, during inflammation such as in EAE, IFN-γ increases the Stat1 transcriptional program and results in increased p16/ink4a and Stat1, leading to decreased self-renewal and lifespan that is sustained and changes these cells’ repair capacity. In our model, IFN-γ changes the transcriptional landscape of NSCs with sustained molecular programs as shown in our in vitro experiments. In culture, IFN-γ has a sustained effect that alters the lifespan and self-renewal of NSCs. Using Stat1-deficient NSCs, we showed that the effects of IFN-γ are mediated in part by the transcriptional function of Stat1, results that could have translational relevance in the protection of NSCs from the inflamed microenvironment. More, notably we find that Stat1-deficient cells may have increased neurogenesis and oligodendrogenesis associated with an increase in Sox9, findings that have potential translational implications but need to be better defined in vivo. Finally, we demonstrate that Stat1 binds and modulates the function of Sox9. We summarize this simplified model of the role of Stat1 in NSCs in Figure 7D. As just one example, future genome-wide studies will be critical for defining other direct transcriptional targets of Stat1 in NSCs.
Our study confirms the functional role of Stat1 in regulating NSC function in vitro. Previous work identified the specific role of Stat1 in Nestin + progenitors from the SVZ by reducing Stat1 dependent NSC proliferation; this was confirmed with our data (Pereira et al., 2015). Furthermore, we expanded the role of Stat1 in NSCs by focusing on the impact of Stat1 in NSC self-renewal and differentiation in vitro. Future work will be needed to further delineate the role of Stat1 in neuroblast formation in vivo, since it has been shown that IFN-γ increases the number of neuroblast (Pereira et al., 2015), and our NSCs deficient in Stat1 in vitro show an increase in differentiation of neurons and neuroblast. Differences in methodology may explain the difference between these findings. For instance, emerging data highlight that aging and immune-related inflammation overlap in many of their molecular targets. T cells increasingly infiltrate the aging SVZ and interact with resident NSCs to temper adult neurogenesis through interferon stimulated gene (ISG) signaling (Dulken et al., 2019). This is similar to our results in EAE that show in the SJL and MOG-EAE models a reduction in olfactory bulb neurogenesis (Pluchino et al., 2008; Rasmussen et al., 2011) that has been confirmed by others (Tepavčević et al., 2011).
There is recent interest in defining the impact of interferons in the SVZ, and recent work has shown an important role of interferons in regulating SVZ NSCs in young and old mice. Type I interferon modulates the activation of SVZ NSCs and induces quiescence (Kalamakis et al., 2019; Carvajal Ibañez et al., 2023). At the same time, other groups report major contributions of type I interferon signaling in the aging choroid plexus and ventricular zones (Baruch et al., 2014). A crucial next step will be to disentangle the contributions and interplay between these different types of interferons. In addition, investigation into the role of ISG signaling in other neural stem cell niche areas like the hippocampus and aging-associated diseases like dementia could be critical for repair in neurodegeneration. Similar mechanisms of dysfunction of a stem cell niche are reported in the bone marrow where loss of normal signaling in the niche can precipitate disease (Raaijmakers et al., 2010) or exogenous signals such as immune cytokines can affect the normal regulation in the niche, leading to bone marrow failure (de Latour et al., 2010; Tang et al., 2010).
Despite the success in animal models of MS and promise from a recently completed open-label, phase one study showing the safety of intrathecal infusions of fetal-derived NSCs (Genchi et al., 2023), infusions of NSCs to MS patients face many hurdles. These questions include the sourcing of stem cells; the use of fetal allogeneic vs. induced pluripotent stem cells; the safety of cultured human stem cells such as NSCs (Imitola, 2007) or embryonic stem cells (Gore et al., 2011); their potential for tumorigenicity (Snyder, 2011); and whether exogenous NSCs may be compromised when placed in a chronically-inflamed microenvironment, becoming the target of neuroinflammation. Therefore, understanding the mechanisms by which endogenous stem cells fail to repair during disease and the dissection of inducible aberrant transcriptional programs by cytokines critical for MS, like IFN-γ in collaboration with other cytokines like TNFα and IL17 among others, thus remains of paramount importance.
Limitations of the current work includes a detail analysis of the relationship of Stat1 in multiple cell types including Dlx2, DCX neural progenitors in the SVZ. Future single-cell sequencing identification of such neuroinflammation-induced programs of NSCs in the SVZ in vivo will be a critical next step to expand the distinct molecular programs in NSCs and the role of other inflammatory cells such as T cells and microglia (Starossom et al., 2011, 2019).
In conclusion, our data expand of the role IFN-γ on NSCs and suggest that the transcriptional programs of Stat1 may regulate NSCs and other progenitors in the SVZ. Identifying the cell type-specific genetic networks that respond to injury and inflammation, such as Stat1, will be key to rationally designing pro-regenerative therapies in chronic progression of neuroinflammatory diseases such as MS.
Experimental methods
Animals
We used 6–8 weeks C57BL/6, and SJL mice obtained from The Jackson Laboratory (Bar Harbor, Maine, USA). MOG-Tg 2D2 mice were provided by Dr. Vijay K. Kuchroo. 6–8 weeks old 129S6/SvEv-Stat1TM1Rds mice containing a homozygous disruption of the Stat1 gene and complete lack of functional STAT1 proteins were obtained from Taconis Bioscience (Germantown, NY, USA). All mice were housed according to NIH guidelines and the Animal Care Committee of Harvard University approved all experiments. All NSCs experiments were done according to the regulation of the University of Connecticut Biosafety regulations.
EAE induction
All experiments were conducted under the approval of the Harvard Medical School Animal Care committee and the Animal Care. SJL/J mice were immunized subcutaneously in two sites (left and right flank) with 150 μg of PLP139-151 (New England Peptide LLC., Gardner, MA) emulsified in complete Freund’s adjuvant (CFA, Sigma Aldrich, Saint Louis, MO) containing 200 μg Mycobacterium Tuberculosis (Difco Laboratories, Detroit, MI). Mice received 200 ng pertussis toxin (PT, List Biological Laboratories Inc., Campbell, CA) in 0.2 ml PBS (Lonza, Walkersville, MD) intraperitoneally (ip) at the time of immunization and 48 h later. Control mice were immunized with CFA followed by PT (12). The mice were sacrificed at different time points. For MOG 35–55 (M-E-V-G-W-Y-R-S-P-F-S-R-O-V-H-L-Y-R-N-G-K) corresponding to mouse sequence is synthesized by QCB Inc. Division of BioSource International (Hopkinton, MA), and purified to > 99% by HPLC. Mice are immunized subcutaneously in the flanks with 150–200 μg of MOG peptide in 0.1 ml PBS and 0.1 ml CFA containing 0.4 mg Mycobacterium Tuberculosis (H37Ra, Difco, Detroit, Michigan) and injected i.p. with 200 ng Pertussis toxin (List Laboratories, Campbell, California) on the day of immunization and 2 days later. EAE is scored as follows; grade 1, limp tail or isolated weakness of gait without limp tail; grade 2, partial hind leg paralysis; grade 3, total hind leg or partial hind and front leg paralysis; grade 4, total hind leg and partial front leg paralysis; grade 5, moribund or dead animal. All animals used for immunohistochemistry experiments of acute and chronic EAE, had a clinical score of equal or > 2. For adoptive transfer, we collected 3 × 106 CD4 + MOG TCR transgenic cells from a naïve 2D2 mouse and injected iv into 4 WT females. The recipients were immunized with 150 μg MOG/CFA + PT and harvested at day 14 pi. EAE scores were 2–3. The samples were stimulated with PMA and ionomycin for 4 h before staining. MOG TCR transgenic cells were identified based on their homogenous expression of TCR Va3.2 and Vb11.
Neural stem cell isolation and culture
Primary culture of adult neural stem cells. Primary cultures of neural stem cells were isolated from adult 129 Stat1+/+ and Stat1–/– mice. Mice were deeply anesthetized in a CO2 chamber at the endpoint of the various time points. Two mm coronal sections were taken from the brains and periventricular tissue was dissected into cold HBSS. The tissue was cut into small pieces (>0.1 mm) and transferred to a digestion solution (mixture of papain, cysteine and EDTA). At the end of the enzymatic incubation the tissue was mechanical dissociated with a fire-bore narrowed Pasteur pipette in DMEM/F-12 medium containing 0.7 mg/ml ovomucoid. The dissociated cell suspension was plated in non-coated flasks in media composed of DMEM/F-12, N-2 supplement (1%), penicillin/streptomycin (1%), bFGF (20 ng/ml), EGF (20 ng/ml) and heparin (8 μg/ml). Primary stem cell proliferation was detected after 7 days in vitro and characterized by formation of spheres of undifferentiated cells. Subsequently passaging of primary spheres was performed by enzymatic incubation with versene (5 min at 37°C) and mechanically dissociating the collected spheres.
The dissociated cell suspension was then again plated in non-coated flasks for secondary neurospheres stem cell proliferation. Before each experiment NSCs were dissociated with versene and plated in PDL-coated 24 well-plates (1*105 cells/ml) in DMEM/F-12 medium for 24 h whereafter the medium was changed to neurobasal medium supplemented with B27 (1%), L-glutamine (0.5 nM) and penicillin/streptomycin (1%) for differentiation.
For differentiation of NSCs from Stat1+/+ and Stat1–/– NSCs were plated at 1 × 105 cells/ml in 24 well plates in neurobasal medium supplemented with B27 (1%), L-glutamine (0.5 nM) and penicillin/streptomycin (1%) for differentiation that stop the proliferation of NSCs and allow neuronal and glial differentiation after 7–14 days in vitro. At the end of the differentiation period cultures were placed on PFA4% in PBS for staining with antibodies for neurons (MAP2, DCX), astrocytes (GFAP) and oligodendrocyte progenitor cells (O4).
Culture of NSCs with cytokines, continuous growth curve, and population doublings
To culture NSCs with cytokines for proliferation assay, NSCs were cultured for 7 days with IFNγ 100 u/ml, IL17, 10 ng/ml. TGF-β, 1 ng/ml, IL4, 1 ng/ml, IL10, 1 ng/ml in 96 well plates and processed for thymidine incorporation to determine proliferation.
For continuous growth and population doubling. 3 × 105 NSCs were be plated in T75 flasks with or without IFN-γ at 100 U/ml and allowed to proliferate. On day 3, the cells are counted and 3 × 105 of the live NSCs are re-plated under the same conditions. The numbers of population doublings are calculated using a standard formula: PD = ln(Nf/Ni)/ln 2. Where PD = Population doubling, Ni = is the initial amount of NSC, Nf = final amount of NSCs per passage, ln = natural logarithm. Population doubling is calculated for a total of 5–10 passages (Jacobs et al., 2000). This experiment allow us to establish whether the continuous presence of IFN-γ at non-apoptotic dosages is sufficient to generate a senescent phenotype in the NSCs in vitro. This approach was used to compared Stat1–/– and Stat1+/+ NSCs.
Stat1 retrovirus infection and self-renewal neurosphere assay
The details from the retroviral virus and infections have been provided, STAT1 signaling was increased in NSCs by transduction with a murine stem cell virus–based (MSCV-based) retroviral vector as previously described and received from G. Lesinski (Lesinski et al., 2003). A brief description is provided, the genome of this virus contains 5′ and 3′ long terminal repeats (LTRs), a multiple cloning site, and an internal ribosomal entry signal (IRES) followed by the enhanced green fluorescent protein (EGFP) coding region. A plasmid containing the STAT1 coding sequence was obtained and the STAT1 sequence was excised with SacII, blunted with Klenow fragment DNA polymerase, and digested with BamHI to generate a sticky end 5′ to the STAT1 coding sequence. This strategy permitted the directional cloning of the STAT1 sequence into the BamHI/HpaI-digested MSCV retroviral genome. The genes encoding STAT1 and EGFP are expressed as a single transcript, therefore a marker of Stat1 gene overexpression. To generate retroviral supernatants, 293T cells were transiently transfected by calcium phosphate–mediated coprecipitation as previously described. Briefly, 1.5 × 107 293T cells plated overnight in 15-cm dishes with polylysine (Sigma-Aldrich) were cotransfected with plasmids encoding the gag and pol proteins from the Moloney murine leukemia virus (M-MLV) (25 μg), coat proteins from the vesicular stomatitis virus (VSV) (5 μg), and the STAT1-IRES-EGFP construct or a control construct (MSCV) lacking the STAT1 sequence (25 μg). The retroviral supernatant was harvested at 48 h after transfection, filtered (0.2 micron), and frozen at −70°C (23). NSCs cells as neurospheres for infection were washed, trypsinized, seeded in a 6-well plate at 5 × 105 cells per well. For infection, the culture medium was removed, and 1 ml of thawed retroviral supernatant was added per well in the presence of polybrene (8 μg/ml final concentration). Plates were spun for 1 h at 1,000 × g, incubated overnight at 37°C with 5% CO2, and supplemented with new media the next day. Cells were assayed 48 h after infection by flow cytometric analysis for EGFP expression using uninfected NSCs for comparison. EGFP-positive cells were sorted into a 96-well plate as single cell clones.
The number of neurospheres formed in the presence of FGF-2 divided by the number of cells plated gives the neurosphere frequency (Molofsky et al., 2003). For self-renewal capacity assay. NSCs were isolated from different ages and regions from mouse brain. A self-renewal NSCs population was obtained by culturing NSCs in DMEM/F12 with N2 supplement and bFGF (20 ng/ml) in coated Poly-l-lysine dish.
To quantify the percentage of alive vs. dead cells from the retrovirus infection, we counted the number of necrotic (GFP-) per 0.1 mm area of individual coverslip compared to the number of GFP + live cells, captured with high magnification differential interface contrast (DIC) and fluorescent images, that allows the simultaneous assessment of the GFP signal and necrotic features of NSCs.
In vivo analysis of SVZ stem cells proliferation and quantification in Stat1-/- and WT mice
Mice were injected ip (intraperitoneally) with BrdU (5-bromo-2′-deoxyuridine, 120 mg/kg body weight, Sigma Aldrich) once a day for seven consecutive days and were killed 2 h. after the last injection as previously described (Pluchino et al., 2008; Rasmussen et al., 2011).
Immunohistochemistry in vivo and in vitro and quantification
For confocal imaging animals are perfused intracardially with 10 ml of 4% paraformaldehyde in PBS. The brain and spinal cord are removed and embedded in O.C.T., quick frozen in liquid nitrogen and kept at −70°C until sectioning. Cryostat sections (10 μm) of spinal cords are fixed with acetone or 4% paraformaldehyde and then labeled with the antibody of interest, as previously described (Imitola et al., 2003, 2004b; Aharoni et al., 2005; Arien-Zakay et al., 2009). Brains were placed in 30% sucrose for at least 24 h for cryoprotection. Coronal blocks of brain tissue from bregma –2 to + 2 mm were frozen in cryo-protective O.C.T.-solution (Sakura Finetek, Torrance, CA) at –80°C. The tissue was cut into floating sections of 40 μm thickness on a freezing microtome. Floating sections were incubated in a 2% solution of sodium borohydride for 20 min to reduce autofluorescence and then denatured by incubation in 2M hydrochloric acid for 2 h. at room temperature before staining. Sections were blocked with 8% horse serum for 1 h. and incubated overnight with rat antibody against BrdU (1:250, Accurate Chemical, Westbury, NY) and neural antibodies. For differentiation markers we incubated with primary antibodies overnight and secondary antibodies for 2 h in blocking solution. We use highly cross-adsorbed secondary antibodies to avoid cross-reactivity (Alexa 488 and Alexa 594). Confocal microscopy is performed using a Zeiss Laser Scanning Microscope 3D analysis software (Zeiss, Thornwood, NY) with a multitrack acquisition protocol to avoid potential overlapping of the two fluorochromes, all antibodies used in the study are shown in Table 1.
The number of BrdU + cells in the corpus callosum was quantified from a 20 μm thick z-stack from 200 × 200 μm square right below the genu of the corpus callosum, while the number of BrdU + cells and the labeling index for Sox2 in the lateral wall were quantified from a 20 μm thick z-stack over 200 μm extending down from the corner of the SVZ. Cells labeled with Sox2 and/or BrdU were quantified using a using a 63 × water-immersion objective lens. The number of cells was averaged from four coronal sections per animal 120 μm apart starting at rostrocaudal level + 1.10 mm from bregma with four mice (Paxinos and Franklin, 2001) per experimental group.
In vitro NSCs were cultures as described above in PLL coated coverslip and at the end of the experimental setting PFA 4% was used for fixation. We performed stereological techniques to quantify neurospheres and differentiation with specific antibodies including MAP2, DCX, GFAP, and O4. Differentiated cells were counted using stereological technique using 10 areas per well, the total number of differentiated cells per antibody were counted per each well and expressed as total numbers or percentage of the total number of cells, that demonstrate switch in differentiation of typical NSCs cultures in vitro that are characterized by more astrocytic than neuronal differentiation.
Confocal analysis, reconstruction, and pixel intensity analysis
Regions of interest around the lateral ventricle were analyzed with a confocal microscope (LSM 510 Laser Scanning Microscope and LSM 3D analysis software, Linux, Ogdensburg, NY). We use pixel intensity quantitative measurements to colocalize the staining of two molecules in 3D as we have shown previously (Imitola et al., 2004a,b; Wang et al., 2008; Rasmussen et al., 2011; Starossom et al., 2019).
Collection and flow cytometry staining of CSF samples from EAE mice and controls
Mice were anesthetized with ketamine (100 mg/kg body weight) and xylazine (10 mg/kg body weight). An incision was made in the neck and the membrane covering the cisterna magna was exposed. The membrane was perforated with an ultra-fine 29G insulin syringe and 2–10 μl CSF aspirated as previously described (Kivisäkk et al., 2009). Macroscopically blood contaminated samples were discarded.
CSF cells were collected from animals that had a clinical score > 2 and were washed once with PBS containing 5% serum for intracellular cytokine staining before being stimulated in full culture medium (RPMI supplemented with 10% FCS, glutamine, 2-ME, sodium pyruvate non-essential amino acids, HEPES and antibiotics; all from BioWhittaker) containing phorbol 12-myristate 13-acetate (PMA, 50 ng/ml, Sigma), ionomycin (1 μg/ml, Sigma), and monensin (GolgiStop, 0.65 μl/ml, BD Biosciences) for 4 h at 37°C. After staining of surface markers (CD4, Vβ3.2, Vβ11, CD69, and CD25), cells were fixed and permeabilized using Cytofix/Cytoperm (BD Biosciences) according to the manufacturer’s instructions. Cells were incubated with anti-cytokine antibodies (IFN-γ and IL-17) at RT for 20 min and washed twice in Perm/Wash buffer (BD Biosciences) before analysis. The following mAbs were used: CD4 Pacific Blue (clone RM4-5), IFN-γ PE-Cy7 (XMG1.2), IL-10 APC (JES5-16E3), IL-6 PE (MP5-20F3) and TNF-a FITC (MP6-XT22) from BD Biosciences. Samples were acquired on a FACS Calibur or an LSR II flow cytometer (BD Biosciences) and analyzed using FlowJo version 8.4.3 (Tree Star, Inc.).
RNA Isolation
RNA was isolated from the cells using the RNeasy Mini Qit (Qiagen, Valencia, CA) and consistently yielded high quality RNA based on post analysis of multiple parameters including visualization of the 28S and 18S bands on a 1% agarose RNA gel, spectrophotometric readings of 1.8–2.0 as determined by [(Abs 260–Abs 320)/(Abs 280–Abs 320)], and visualization of two distinct peaks on a bioanalyzer (Agilent Technologies, Andover MA, USA) representing the 28S and 18S ribosomal RNA.
Protein extraction and western blot analysis
Cells were harvested at different time points and washed with ice-cold PBS. Whole cell lysates were prepared by extraction in lysis buffer containing 1% Triton X-100, 20 mM Tris-HCl pH 8.0, 150 mM NaCl, 3 mM sodium pyrophosphate, 10% glycerol, 2 mM sodium orthovanadate, and 20 μl/ml Protease Arrest (Genotech, St. Louis, MO, USA). Protein concentration was determined by the Bradford protein assay (Bio-Rad, Hercules, CA, USA). 40 μg of protein was analyzed by SDS-PAGE using 10% Tris-glycine gels (Invitrogen, Carlsbad, CA, USA), followed by transfer to Hybond-P membranes (Amersham, Piscataway, NJ, USA). Membranes were blocked in 5% non-fat milk in TBS containing 0.05% Tween 20 for 1 h and incubated with primary antibodies at 4°C overnight. The detection step was performed with HRP-coupled anti-rabbit IgG antibodies (Genotech). Blots were developed with the ECL plus chemiluminescence detection system (Amersham, Piscataway, NJ, USA).
We extracted proteins from NSCs and from brain tissues of adult mice in lysis buffer [1 times phosphate-buffered saline, 5 mM EDTA, 1% Triton X-100 and protease inhibitor cocktail tablet (Roche)]. We will separate immunoprecipitated proteins by SDS-PAGE, immunoblot them and incubate them with the appropriate primary antibodies overnight at 4°C and then with the corresponding horseradish peroxidase–conjugated secondary antibodies for 1–2 h at room temperature. Proteins will be detected using ECL chemiluminescence (Amersham).
Microarray and interferon stimulated gene single cell RNAseq analysis
For gene expression analysis mRNA from duplicates NSCs exposed to interferon-γ for 24 h, were processed on Affymetrix microarrays and genes with change expression values ≥ 2-fold and statistical significance by t-test with a Bonferroni correction, were confirmed using a different microarray platform, confocal microscopy, and western blot. Before determining differential expression between Stat1+/+ and Stat1–/– NSCs, genes showing less than 0.25 variance were first filtered out using the varFilter function in the genefilter R package. Next, differential expression was performed using the limma R package, comparing the knockout to control, and using to FDR to correct for multiple hypotheses (Ritchie et al., 2015). A heatmap of DEGs was then generated using the online matrix visualization software, Morpheus.2 To visualize gene ontology enrichment, the ClueGO plug-in in Cytoscape was utilized, using standard parameters, except for including GO Fusion (Bindea et al., 2009). PPI networks from all DEGs at FDR < 0.15 were produced using StringDB (Szklarczyk et al., 2019). Finally, in situ hybridization images of sagittal sections of embryonic mouse brains for selected DEGs were retrieved from Brainspan (Lein et al., 2007).
Normalized TPM values and cell annotation data for the 10× single cell RNA-seq dataset from were retrieved directly from https://github.com/Martin-Villalba-lab/Data/tree/master/Cell_2019 (Kalamakis et al., 2019). Original cell annotations were preserved and transferred to a new SeuratObject in which all genes were examined using the Seurat package in R (Hao et al., 2021). Stat1 target genes were defined using a comprehensive list from Chip-Seq analysis (Satoh and Tabunoki, 2013). Direct target genes were defined by those at which Stat1 bound to promoters, since intronic localization could be Stat1 binding enhancers of unknown far-away target genes, and genes that had at least two log-fold change increase by treatment with Stat1 activator, IFN-γ.
Real-time PCR of NSCs
Total RNA is extracted from NSCs using RNeasy mini kit (Qiagen, Valencia, CA, USA), and cDNA is synthesized using SuperScript™ III first-strand synthesis system for RT-PCR (Invitrogen, Carlsbad, CA, USA). Expression of Stat1 (as an example of a gene of interest) and glyceraldehyde phosphate dehydrogenase (GAPDH) or Hypoxanthine Guanine Phosphoribosyltransferase (HPRT) mRNA, reliable housekeeping genes for NSCs studies, (Chapman and Waldenstrom, 2015; Kang et al., 2019) were measured by PCR in separate tubes in duplicates using probes labeled with 6-carboxyfluorescein (FAM) and VIC®, respectively, TAMRA® as a quencher, TaqMan® universal PCR master mix and the ABI Prism 7700 sequence detection system (all from Applied Biosystems, Foster City, CA, USA). Primers and probe for GAPDH were purchased from Applied Biosystems. A comparative threshold cycle (CT) will be used to determine mRNA expression of Stat1 and GAPDH relative to no-template control (calibrator). CT value will be normalized for each sample using the formula: ΔCT = CT (stat1)- CT (GAPDH) and the relative expression of stat1 will be then calculated using the expression 2–Δ CT.
ChIP and qPCR and luciferase assay
Neural stem cells were isolated from in vitro cultures in the presence or absence of IFN-γ purified and ChIP was performed with ChampionChip kit (SABiosciences). Cell lysates were used for immunoprecipitation with anti-Stat1 (Abcam) and anti-Sox9 (Abcam) and were compared to control IgG. Several regions of the Sox9 promoter containing putative Stat1 binding sites were amplified by SYBR Green qPCR (Roche) and quantified in triplicate with the percent of input method. The following primers were used for Sox9: site 1F: 5′-CACACACACACACACACACACA-3′, site 1R: 5′-GGGACGTCCGAGTGTGTAA-3′; site 2F: 5′-AGAGCGCTGCTCGGAACT-3′, site 2R: 5′-CCACTTGCACCTCGTCTCTC-3′; and site 3F: 5′-GCAGCTGAGGGAAGAGGAG-3′, site 3R: 5′-GAAGGGGTCCAGGAGATTCA-3′. qPCR quantification of ChIP assays on NSCs from adult mice treated with or without IFN-γ for 4 h. Samples were immunoprecipitated with an anti-Stat1 antibody and amplified with Sox9 or irrelevant control primers. For negative controls, samples were processed without IgG antibody. Vectors for Sox9 promoters (Kumar and Lassar, 2009) were used for transcriptional luciferase assay. Multiple Reporter vectors coding for the Firefly Luciferase under the control of the Sox9 promoter encompassing nucleotides −6,028 to +300 bp, reporter assays were carried out as described previously (Elyaman et al., 2012). In brief, 293T cells were transfected with 0.4 μg of the reporter vector coding for the firefly luciferase (pGL3 basic; Promega) under the control of the Sox9 promoter and with 0.8 μg of the Stat1. Cells were cultured for 48 h. before harvesting and the relative Sox9 promoter activity was measured with Promega kit in accordance with the manufacturer’s instructions.
Statistical analysis
All data is presented as mean ± SEM, statistical analysis was performed using the unpaired, two-sided t-test comparison between EAE and control or by using one-way analysis of variance (ANOVA) with Turkey’s Multiple Comparison Test. Significant differences were assumed at the 5% level and represented as P-values (p < 0.05).
Data availability statement
The original contributions presented in the study are included in the article/Supplementary material, further inquiries can be directed to the corresponding author/s upon reasonable request.
Ethics statement
The animal study was reviewed and approved by the Animal Care Committee of Harvard University.
Author contributions
JI and SK conceived the project. JI, EH, FW, MO, WE, SS, and PK performed the experiments. JI, EH, and SK wrote the manuscript with contributions from all authors. All authors read and approved the final manuscript.
Funding
This work was supported by NIH grants (AI46374 and AI043496 to SK), NMSS RG 3945 grant to SK, and PP1236 from the National Multiple Sclerosis Society and UConn-Biogen Award to JI.
Conflict of interest
The authors declare that the research was conducted in the absence of any commercial or financial relationships that could be construed as a potential conflict of interest.
Publisher’s note
All claims expressed in this article are solely those of the authors and do not necessarily represent those of their affiliated organizations, or those of the publisher, the editors and the reviewers. Any product that may be evaluated in this article, or claim that may be made by its manufacturer, is not guaranteed or endorsed by the publisher.
Supplementary material
The Supplementary Material for this article can be found online at: https://www.frontiersin.org/articles/10.3389/fncel.2023.1156802/full#supplementary-material
Supplementary Figure 1 | Quantification of cell death after STAT1 infection, Validation of SVZ NSCs by qPCR, genotype and Retrovirus targeting of Stat1–/– NSCs. Quantification of cell death after STAT1 infection: (A) cell death quantification of GFP cells after MSCV and STAT1 vectors, there is a minimal but significant increase in the cell death of STAT1 vector expressing cells. Validation of the absence of Stat1 in SVZ NSCs by qPCR, genotype: (B) diagram of the SVZ microdissected area used to perform isolation of NSCs from brains of Stat1–/– and mice and validation of Stat1–/– genotypes used for the SVZ NSCs isolation, the gel represents typical genomic DNA confirmation in mice tissue and cultures neurospheres, this is done to verify the fidelity of the NSCs cultures from Stat1–/– and controls. (C) qPCR analysis of neurospheres to confirm reduction of expression Stat1 in NSCs from Stat1–/– deficient mice. (D) Validation of effects of Stat1 retrovirus in Stat1 Neurospheres from Stat1–/– NSCs: NSCs were dissociated and infected with retrovirus delivering STAT1-GFP, the cells were sorted by FACS 48 hours after infection based on GFP expression and plated at clonal dilution in FGF-2 medium. (E) Representative image showing the reduction of the numbers (p < 0.001 by t-test) and (F) size of secondary neurospheres in the Stat1–/– NSCs treated with the STAT1-GFP retrovirus compared to murine stem cell virus (MSCV)-GFP vector after 7 days in cultures (p = 0.019).
Supplementary Figure 2 | Differentiation of Stat1–/– NSCs compared to Stat1 + / +. (A) Staining of doublecortin DCX+ cells (green) and GFAP+ cells (red) in WT (left panel) and STAT1-/- (right panel). (B) Quantification of DCX+ and GFAP+ cells in Stat1 + / + and Stat1–/– in mm3 (p < 0.001 by t-test).
Supplementary Figure 3 | Expression of interferon related genes in subventricular zone NSCs and IFN-γ T cells in EAE CSF. (A) Diagram and Electron Microscopy of the relationship of NSCs with the CSF, ciliated NSCs cells (Type B) protrude the cytoplasm in the CSF interacting with the cytokines from the CSF. (B) Comparative microarray gene expression of interferon-γ related genes in NSCs (blue bars) compared to normal brain (red bars), showing constitutive expression of these genes in isolated SVZ NSCs expression values are given in Affymetrix mean fluorescence. (C) Expression of IFN-γ receptor-1 (green) in the ventricular and SVZ mouse brain. NSCs are co-stained with the neural stem cell regulator Bmi-1 (red) bar = 20 μm. (D) Quantitative PCR analysis of Stat1 expression of isolated SVZ from SJL mice with EAE, results from n = 3 animals per group. (E) Immune cells from draining lymph nodes, CSF, blood, and MEN of animals with EAE (day 14 post-immunization) were isolated by dissection of meninges and stained intracytoplasmically for IFN-g and IL-17 showing a higher percentage of IFN-γ producing CD4+ T cells in EAE in the PLP-relapsing remitting EAE compared to IL-17 (p < 0.001). n = 3 animals per group. (F) Confirmation in a different EAE model, using 2D2 mice, quantification of numbers of MOG-specific IFN-γ producing T cells in the CSF compared to different immune compartments. IFN-γ producing cells in CSF of diseased animals, 80% compared to 20% of IL-17 producing cells in CSF, data represent n = 3 animal per group.
Supplementary Figure 4 | Effects of IFN-γ in long term cultures of NSCs inducing alteration of lifespan. (A) Quantification of the numbers of secondary neurospheres by frequency exposed to various doses of IFN-γ showing a significant dose dependent response p < 0.001 by t-test. (B) Western blot of IFN-γ induced phosphorylation of STAT1 at tyrosine Y705 but not STAT3 in serine S727 compared to total STAT-1 and STAT-3 (bottom lane). (C) Representative FACS showing no increase apoptosis or toxicity of IFN-γ used for experiments showing decrease proliferation after day 5 in culture. (D,E) Quantification of tapasin-1 and Tenascin after IFN-γ treatment in vitro and (F) confirmation of proteins by confocal microscopy. (H) Quantification of NSCs lifespan after repeated passages of primary isolated (p5) compared to late passage (p60) NSCs in the presence of IFN-γ. The red line below the X-axis indicates the presence of IFN-γ in the culture. The blue lines are cells passaged p5 times while the red lines are the cells passaged p60 times, the dashed lines are cells cultured in the presence of IFN-γ while the continuous lines are cells not exposed to IFN-γ (I) example of neurospheres growing in the absence or presence of long term IFN-γ 100 U/ml, showing a significant decrease in the size of neurospheres and single NSCs that failed to form neurospheres but still viable (insert).
Footnotes
References
Aharoni, R., Arnon, R., and Eilam, R. (2005). Neurogenesis and neuroprotection induced by peripheral immunomodulatory treatment of experimental autoimmune encephalomyelitis. J. Neurosci. 25, 8217–8228.
Arien-Zakay, H., Lecht, S., Bercu, M., Amariglio, N., Rechavi, G., Galski, H., et al. (2009). Interferon-gamma-induced neuronal differentiation of human umbilical cord blood-derived progenitors. Leukemia 23, 1790–1800. doi: 10.1038/leu.2009.106
Baldridge, M., King, K., Boles, N., and Weksberg, D. (2010). Quiescent haematopoietic stem cells are activated by IFN-gamma in response to chronic infection. Nature 465, 793–797. doi: 10.1038/nature09135
Baruch, K., Deczkowska, A., David, E., Castellano, J., Miller, O., Kertser, A., et al. (2014). Aging-induced type I interferon response at the choroid plexus negatively affects brain function. Science 346, 89–93. doi: 10.1126/science.1252945
Bindea, G., Mlecnik, B., Hackl, H., Charoentong, P., Tosolini, M., Kirilovsky, A., et al. (2009). ClueGO: A Cytoscape plug-in to decipher functionally grouped gene ontology and pathway annotation networks. Bioinformatics 25, 1091–1093. doi: 10.1093/bioinformatics/btp101
Carvajal Ibañez, D., Skabkin, M., Hooli, J., Cerrizuela, S., Göpferich, M., Jolly, A., et al. (2023). Interferon regulates neural stem cell function at all ages by orchestrating mTOR and cell cycle. EMBO Mol. Med. 15:e16434. doi: 10.15252/emmm.202216434
Chapman, J. R., and Waldenstrom, J. (2015). With reference to reference genes: A systematic review of endogenous controls in gene expression studies. PLoS One 10:e0141853. doi: 10.1371/journal.pone.0141853
de Latour, R., Visconte, V., Takaku, T., Wu, C., Erie, A., Sarcon, A., et al. (2010). Th17 immune responses contribute to the pathophysiology of aplastic anemia. Blood 116, 4175–4184. doi: 10.1182/blood-2010-01-266098
Dulken, B., Buckley, M., Navarro Negredo, P., Saligrama, N., Cayrol, R., Leeman, D., et al. (2019). Single-cell analysis reveals T cell infiltration in old neurogenic niches. Nature 571, 205–210. doi: 10.1038/s41586-019-1362-5
Elyaman, W., Bassil, R., Bradshaw, E., Orent, W., Lahoud, Y., Zhu, B., et al. (2012). Notch receptors and Smad3 signaling cooperate in the induction of interleukin-9-producing T cells. Immunity 36, 623–634. doi: 10.1016/j.immuni.2012.01.020
Essers, M., Offner, S., Blanco-Bose, W., Waibler, Z., Kalinke, U., Duchosal, M., et al. (2009). IFNalpha activates dormant haematopoietic stem cells in vivo. Nature 458, 904–908. doi: 10.1038/nature07815
Favaro, R., Valotta, M., Ferri, A., Latorre, E., Mariani, J., Giachino, C., et al. (2009). Hippocampal development and neural stem cell maintenance require Sox2-dependent regulation of Shh. Nat. Neurosci. 12, 1248–1256. doi: 10.1038/nn.2397
Garcion, E., Halilagic, A., Faissner, A., and ffrench-Constant, C. (2004). Generation of an environmental niche for neural stem cell development by the extracellular matrix molecule tenascin C. Development 131, 3423–3432. doi: 10.1242/dev.01202
Genchi, A., Brambilla, E., Sangalli, F., Radaelli, M., Bacigaluppi, M., Furlan, R., et al. (2023). Neural stem cell transplantation in patients with progressive multiple sclerosis: An open-label, phase 1 study. Nat. Med. 29, 75–85. doi: 10.1038/s41591-022-02097-3
Gore, A., Li, Z., Fung, H., Young, J., Agarwal, S., Antosiewicz-Bourget, J., et al. (2011). Somatic coding mutations in human induced pluripotent stem cells. Nature 471, 63–67. doi: 10.1038/nature09805
Hao, Y., Hao, S., Andersen-Nissen, E., Mauck, W., Zheng, S., Butler, A., et al. (2021). Integrated analysis of multimodal single-cell data. Cell 184, 3573–3587.e29. doi: 10.1016/j.cell.2021.04.048
Imitola, J. (2007). Prospects for neural stem cell-based therapies for neurological diseases. Neurotherapeutics 4, 701–714. doi: 10.1016/j.nurt.2007.08.005
Imitola, J., Comabella, M., Chandraker, A., Dangond, F., Sayegh, M., Snyder, E., et al. (2004a). Neural stem/progenitor cells express costimulatory molecules that are differentially regulated by inflammatory and apoptotic stimuli. Am. J. Pathol. 164, 1615–1625. doi: 10.1016/S0002-9440(10)63720-0
Imitola, J., Raddassi, K., Park, K., Mueller, F., Nieto, M., Teng, Y., et al. (2004b). Directed migration of neural stem cells to sites of CNS injury by the stromal cell-derived factor 1alpha/CXC chemokine receptor 4 pathway. Proc. Natl. Acad. Sci. U.S.A. 101, 18117–18122. doi: 10.1073/pnas.0408258102
Imitola, J., Snyder, E. Y., and Khoury, S. J. (2003). Genetic programs and responses of neural stem/progenitor cells during demyelination: Potential insights into repair mechanisms in multiple sclerosis. Physiol. Genomics 14, 171–197.
Jackson, E., Garcia-Verdugo, J., Gil-Perotin, S., Roy, M., Quinones-Hinojosa, A., VandenBerg, S., et al. (2006). PDGFR alpha-positive B cells are neural stem cells in the adult SVZ that form glioma-like growths in response to increased PDGF signaling. Neuron 51, 187–199. doi: 10.1016/j.neuron.2006.06.012
Jacobs, J., Keblusek, P., Robanus-Maandag, E., Kristel, P., Lingbeek, M., Nederlof, P., et al. (2000). Senescence bypass screen identifies TBX2, which represses Cdkn2a (p19(ARF)) and is amplified in a subset of human breast cancers. Nat. Genet. 26, 291–299. doi: 10.1038/81583
Kalamakis, G., Brüne, D., Ravichandran, S., Bolz, J., Fan, W., Ziebell, F., et al. (2019). Quiescence modulates stem cell maintenance and regenerative capacity in the aging brain. Cell 176, 1407–1419.e14. doi: 10.1016/j.cell.2019.01.040
Kalani, M., Cheshier, S., Cord, B., Bababeygy, S., Vogel, H., Weissman, I., et al. (2008). Wnt-mediated self-renewal of neural stem/progenitor cells. Proc. Natl. Acad. Sci. U.S.A. 105, 16970–16975. doi: 10.1073/pnas.0808616105
Kang, I. N., Lee, C. Y., and Tan, S. C. (2019). Selection of best reference genes for qRT-PCR analysis of human neural stem cells preconditioned with hypoxia or baicalein-enriched fraction extracted from Oroxylum indicum medicinal plant. Heliyon 5:e02156.
Kebir, H., Kreymborg, K., Ifergan, I., Dodelet-Devillers, A., Cayrol, R., Bernard, M., et al. (2007). Human TH17 lymphocytes promote blood-brain barrier disruption and central nervous system inflammation. Nat. Med. 13, 1173–1175. doi: 10.1038/nm1651
Kirby, L., Jin, J., Cardona, J., Smith, M., Martin, K., Wang, J., et al. (2019). Oligodendrocyte precursor cells present antigen and are cytotoxic targets in inflammatory demyelination. Nat. Commun. 10:3887. doi: 10.1038/s41467-019-11638-3
Kivisäkk, P., Imitola, J., Rasmussen, S., Elyaman, W., Zhu, B., Ransohoff, R. M., et al. (2009). Localizing central nervous system immune surveillance: Meningeal antigen-presenting cells activate T cells during experimental autoimmune encephalomyelitis. Ann. Neurol. 65, 457–469.
Kumar, D., and Lassar, A. B. (2009). The transcriptional activity of Sox9 in chondrocytes is regulated by RhoA signaling and actin polymerization. Mol. Cell Biol. 29, 4262–4273.
Lehtinen, M., Zappaterra, M., Chen, X., Yang, Y., Hill, A., Lun, M., et al. (2011). The cerebrospinal fluid provides a proliferative niche for neural progenitor cells. Neuron 69, 893–905. doi: 10.1016/j.neuron.2011.01.023
Lein, E., Hawrylycz, M., Ao, N., Ayres, M., Bensinger, A., Bernard, A., et al. (2007). Genome-wide atlas of gene expression in the adult mouse brain. Nature 445, 168–176. doi: 10.1038/nature05453
Lesinski, G., Anghelina, M., Zimmerer, J., Bakalakos, T., Badgwell, B., Parihar, R., et al. (2003). The antitumor effects of IFN-alpha are abrogated in a STAT1-deficient mouse. J. Clin. Invest. 112, 170–180. doi: 10.1172/JCI16603
Li, L., Walker, T., Zhang, Y., Mackay, E., and Bartlett, P. (2010). Endogenous interferon gamma directly regulates neural precursors in the non-inflammatory brain. J. Neurosci. 30, 9038–9050. doi: 10.1523/JNEUROSCI.5691-09.2010
Mäkelä, J., Koivuniemi, R., Korhonen, L., and Lindholm, D. (2010). Interferon-gamma produced by microglia and the neuropeptide PACAP have opposite effects on the viability of neural progenitor cells. PLoS One 5:e11091. doi: 10.1371/journal.pone.0011091
Merzaban, J., Imitola, J., Starossom, S., Zhu, B., Wang, Y., Lee, J., et al. (2015). Cell surface glycan engineering of neural stem cells augments neurotropism and improves recovery in a murine model of multiple sclerosis. Glycobiology 25, 1392–1409. doi: 10.1093/glycob/cwv046
Molofsky, A., Pardal, R., Iwashita, T., Park, I., Clarke, M., and Morrison, S. (2003). Bmi-1 dependence distinguishes neural stem cell self-renewal from progenitor proliferation. Nature 425, 962–967. doi: 10.1038/nature02060
Nait-Oumesmar, B., Picard-Riera, N., Kerninon, C., Decker, L., Seilhean, D., Höglinger, G., et al. (2007). Activation of the subventricular zone in multiple sclerosis: Evidence for early glial progenitors. Proc. Natl. Acad. Sci. U.S.A. 104, 4694–4699. doi: 10.1073/pnas.0606835104
Paxinos, G., and Franklin, K. B. J. (2001). The mouse brain in stereotaxic coordinates. San Diego, CA: Academic Press.
Pereira, L., Medina, R., Baena, M., Planas, A., and Pozas, E. (2015). IFN gamma regulates proliferation and neuronal differentiation by STAT1 in adult SVZ niche. Front. Cell Neurosci. 9:270. doi: 10.3389/fncel.2015.00270
Pluchino, S., Muzio, L., Imitola, J., Deleidi, M., Alfaro-Cervello, C., Salani, G., et al. (2008). Persistent inflammation alters the function of the endogenous brain stem cell compartment. Brain. 131(Pt. 10), 2564–2578. doi: 10.1093/brain/awn198
Pluchino, S., Quattrini, A., Brambilla, E., Gritti, A., Salani, G., Dina, G., et al. (2003). Injection of adult neurospheres induces recovery in a chronic model of multiple sclerosis. Nature 422, 688–694. doi: 10.1038/nature01552
Pluchino, S., Zanotti, L., Rossi, B., Brambilla, E., Ottoboni, L., Salani, G., et al. (2005). Neurosphere-derived multipotent precursors promote neuroprotection by an immunomodulatory mechanism. Nature 436, 266–271. doi: 10.1038/nature03889
Poss, K. (2010). Advances in understanding tissue regenerative capacity and mechanisms in animals. Nat. Rev. Genet. 11, 710–722. doi: 10.1038/nrg2879
Raaijmakers, M., Mukherjee, S., Guo, S., Zhang, S., Kobayashi, T., Schoonmaker, J., et al. (2010). Bone progenitor dysfunction induces myelodysplasia and secondary leukaemia. Nature 464, 852–857. doi: 10.1038/nature08851
Ramalho-Santos, M., Yoon, S., Matsuzaki, Y., Mulligan, R., and Melton, D. (2002). “Stemness”: Transcriptional profiling of embryonic and adult stem cells. Science 298, 597–600. doi: 10.1126/science.1072530
Rasmussen, S., Imitola, J., Ayuso-Sacido, A., Wang, Y., Starossom, S., Kivisäkk, P., et al. (2011). Reversible neural stem cell niche dysfunction in a model of multiple sclerosis. Ann. Neurol. 69, 878–891. doi: 10.1002/ana.22299
Ritchie, M. E., Phipson, B., Wu, D., Hu, Y., Law, C. W., Shi, W., et al. (2015). Limma powers differential expression analyses for RNA-sequencing and microarray studies. Nucleic Acids Res. 43:e47.
Satoh, J., and Tabunoki, H. A. (2013). Comprehensive profile of ChIP-Seq-based STAT1 target genes suggests the complexity of STAT1-mediated gene regulatory mechanisms. Gene Regul. Syst. Bio 7, 41–56.
Scott, C., Wynn, S., Sesay, A., Cruz, C., Cheung, M., Gomez Gaviro, M., et al. (2010). SOX9 induces and maintains neural stem cells. Nat. Neurosci. 13, 1181–1189. doi: 10.1038/nn.2646
Snyder, E. (2011). The risk of putting something where it does not belong: Mesenchymal stem cells produce masses in the brain. Exp. Neurol. 230, 75–77. doi: 10.1016/j.expneurol.2011.03.012
Starossom, S., Campo Garcia, J., Woelfle, T., Romero-Suarez, S., Olah, M., Watanabe, F., et al. (2019). Chi3l3 induces oligodendrogenesis in an experimental model of autoimmune neuroinflammation. Nat. Commun. 10:217. doi: 10.1038/s41467-018-08140-7
Starossom, S., Imitola, J., Wang, Y., Cao, L., and Khoury, S. (2011). Subventricular zone microglia transcriptional networks. Brain Behav. Immun. 25, 991–999. doi: 10.1016/j.bbi.2010.11.002
Steinman, L. (2007). A brief history of T(H)17, the first major revision in the T(H)1/T(H)2 hypothesis of T cell-mediated tissue damage. Nat. Med. 13, 139–145. doi: 10.1038/nm1551
Suslov, O., Kukekov, V., Ignatova, T., and Steindler, D. (2002). Neural stem cell heterogeneity demonstrated by molecular phenotyping of clonal neurospheres. Proc. Natl. Acad. Sci. U.S.A. 99, 14506–14511. doi: 10.1073/pnas.212525299
Szklarczyk, D., Gable, A., Lyon, D., Junge, A., Wyder, S., Huerta-Cepas, J., et al. (2019). STRING v11: Protein-protein association networks with increased coverage, supporting functional discovery in genome-wide experimental datasets. Nucleic Acids Res. 47, D607–D613. doi: 10.1093/nar/gky1131
Tang, Y., Desierto, M., Chen, J., and Young, N. (2010). The role of the Th1 transcription factor T-bet in a mouse model of immune-mediated bone-marrow failure. Blood 115, 541–548. doi: 10.1182/blood-2009-03-211383
Tepavčević, V., Lazarini, F., Alfaro-Cervello, C., Kerninon, C., Yoshikawa, K., Garcia-Verdugo, J., et al. (2011). Inflammation-induced subventricular zone dysfunction leads to olfactory deficits in a targeted mouse model of multiple sclerosis. J. Clin. Invest. 121, 4722–4734. doi: 10.1172/JCI59145
Wang, Y., Imitola, J., Rasmussen, S., O’Connor, K. C., and Khoury, S. J. (2008). Paradoxical dysregulation of the neural stem cell pathway sonic hedgehog-Gli1 in autoimmune encephalomyelitis and multiple sclerosis. Ann. Neurol. 64, 417–427.
Keywords: multiple sclerosis, experimental autoimmune encephalomyelitis, neural stem cells, subventricular zone, STAT1, interferons, Sox9
Citation: Imitola J, Hollingsworth EW, Watanabe F, Olah M, Elyaman W, Starossom S, Kivisäkk P and Khoury SJ (2023) Stat1 is an inducible transcriptional repressor of neural stem cells self-renewal program during neuroinflammation. Front. Cell. Neurosci. 17:1156802. doi: 10.3389/fncel.2023.1156802
Received: 01 February 2023; Accepted: 20 July 2023;
Published: 16 August 2023.
Edited by:
Fereshteh Pourabdolhossein, Babol University of Medical Sciences, IranReviewed by:
Christian Schachtrup, University of Freiburg, GermanyShannon William Davis, University of South Carolina, United States
Copyright © 2023 Imitola, Hollingsworth, Watanabe, Olah, Elyaman, Starossom, Kivisäkk and Khoury. This is an open-access article distributed under the terms of the Creative Commons Attribution License (CC BY). The use, distribution or reproduction in other forums is permitted, provided the original author(s) and the copyright owner(s) are credited and that the original publication in this journal is cited, in accordance with accepted academic practice. No use, distribution or reproduction is permitted which does not comply with these terms.
*Correspondence: Jaime Imitola, aW1pdG9sYUB1Y2hjLmVkdQ==
†These authors have contributed equally to this work and share senior authorship