- 1Department of Neurology, Poznan University of Medical Sciences, Poznan, Poland
- 2Department of Immunology, Poznan University of Medical Sciences, Poznan, Poland
- 3Department of Neurology, Collegium Medicum, University of Zielona Gora, Zielona Gora, Poland
Introduction: Adaptive immunity after a stroke results in a shift of T cells between compartments, leading to peripheral lymphopenia and an increased number of T cells within the brain lesion. Stroke-associated infection (SAI) presents a clinically significant challenge in stroke units. The role of T-cell subsets in the post-stroke immune response and in SAI remains unclear. Thus, we aimed to observe the quantitative changes of circulating CD4+, CD8+, double-negative T cells, and the CD4+/CD8+ ratio in stroke and SAI.
Methods: We prospectively assessed circulating CD4+, CD8+, and double-negative T cells using flow cytometry in 52 patients on days 1, 3, 10, and 90 after ischemic stroke. We compared the results to those obtained from age-, sex-, and vascular risk factor-matched controls. We analyzed lymphocyte parameters in relation to clinical outcome, SAI, infarct lesion volume, and risk factor burden.
Results: There were no differences in the studied parameters between stroke patients and controls, as well as between subjects with and without SAI. A higher percentage of CD4+ T cells and a higher CD4+/CD8+ ratio correlated with better clinical status in the acute and subacute phases, while CD8+ T cells showed the opposite correlation. The percentage of CD8+ T cells positively correlated with CRP levels during the acute and subacute phases of stroke, as well as in the control group. A negative correlation was noted between the percentage of CD4+ T cells on D1 and the serum CRP level on D10 after stroke. Similarly, the CD4+/CD8+ ratio on D1 negatively correlated with CRP on D1, D3, and D10. In patients with a history of hypertension (HT), there was a higher percentage of CD8+ T cells and a lower percentage of CD4+ T cells in the acute phase of stroke than those without HT.
1 Introduction
Stroke-induced immunodepression syndrome, characterized by lymphopenia, contributes to the increased risk of infection in patients hospitalized due to ischemic stroke. The likelihood of pneumonia or urinary tract infection in these patients ranges from 21 to 65% (Chamorro et al., 2007). Thirty percent of patients with stroke-associated infections (SAI) will die, highlighting the clinical significance of SAI. The immunological reaction to stroke is mostly concerned with the adaptive response (Meisel et al., 2005).
T-lymphocytes are the main representatives of adaptive response cells in the central nervous system (Giunti et al., 2003). T cells circulate in the cerebrospinal fluid of healthy individuals, constantly invigilating the central nervous system (De Graaf et al., 2010; Ransohoff et al., 2003). CD4+ and CD8+ T cells make up the majority of T-lymphocytes. The proportion of CD4+ and CD8+ T cells in cerebrospinal fluid is 3.5:1, and some are memory cells (Giunti et al., 2003; Stuve et al., 2006). CD4+ T cells mediate immune response by secreting specific cytokines and activating B cells, cytotoxic T cells, and non-immune cells, and also play a critical role in the suppression of immune reaction. The subsets of CD4+ T cells include classical T-helper 1 (Th1) and T-helper 2 (Th2) cells, T-helper 17 (Th17), follicular helper T cells (Tfh), induced T-regulatory cells (iTreg), and regulatory type 1 cells (Tr1) and possibly distinct T-helper 9 (Th9) (Luckheeram et al., 2012). Th1 cells are involved in the elimination of intracellular pathogens and are associated with organ-specific autoimmunity. In contrast, Th2 cells are involved in immune responses against extracellular parasites and play a major role in asthma and other allergic diseases (Del Prete, 1992). CD8+ T cells are crucial for immune defense against intracellular pathogens, including viruses and bacteria, as well as for tumor surveillance. CD8+ T cells activated by antigens from infected or malignant cells present a cytotoxic ability, releasing perforin and granzymes or eliminating target cells through Fas/FasL interactions (Catalfamo and Reali, 2020).
CD4−CD8− T cells, called double-negative T cells (DNTs), constitute a small subpopulation of peripheral T cells (Brandt and Hedrich, 2018). The origin and function of DNT cells are still unclear. In healthy individuals, they form a rare and heterogeneous T-cell subset (1–5% of the total T-cell population). However, the number of DNT cells increases in various inflammatory conditions, where they exhibit different effector phenotypes and infiltrate inflamed tissues (Brandt and Hedrich, 2018; Doyle et al., 2015).
Since the brain is no longer considered a site of immune privilege, despite the presence of the blood–brain barrier and lack of conventional lymphatic vessels, many immune cells can infiltrate the central nervous system and may contribute to immune surveillance in the brain. After an ischemic stroke, T and B cells, together with macrophages and neutrophils, infiltrate the brain and interact with the glial cells (Hersh and Yang, 2018). While the number of T cells decreases peripherally, at the same time it increases in the ischemic brain lesion. Intriguingly, it happens within the first 24 h and can persist for a longer period (Gelderblom et al., 2009). The extent of the infiltration of T cells varies among stroke models (Gill and Veltkamp, 2016). A significant increase in CD4+ and CD8+ T cells was seen in human ischemic infarct lesions, with the dominant population of CD8+ T-cells (Zrzavy et al., 2018). The experimental stroke model showed infiltration of CD8+ T cells in an ischemic area within 3 h since vessel occlusion and CD4+ T cells during the first 24 h (Grønberg et al., 2013). Other research revealed infiltration of CD4+ and CD8+ T cells on day 3 (Stevens et al., 2002), day 5 (Liesz et al., 2011), or even 7 weeks after stroke onset (Doyle et al., 2015). In mice with stroke, CD4+ T cell accumulation was noticed for a 30-day period, with a peak on the 14th day of stroke (Gill and Veltkamp, 2016). It shows that antigen-specific reactions to brain ischemia can take place in acute, subacute, and chronic phases. Furthermore, the accumulation of DNT was observed in the area of the ischemic hemisphere in 1–3 days after reperfusion, which suggested an important role of these cells in inflammation (Gelderblom et al., 2009; Meng et al., 2019). Despite these findings, it is still unclear whether the T cells that infiltrated the brain during acute post-stroke neuroinflammation support or hinder functional recovery (Selvaraj and Stowe, 2017). It has been reported that the adaptive transfer of lymphocytes against myelin antigen exacerbates stroke lesions and that proinflammatory lymphocytes are detrimental during an early stage of ischemic brain injury (Jin et al., 2018). Transgenic animals deficient in CD4+ or CD8+ T cells consistently have smaller infarcts in different stroke models (Liesz et al., 2009a; Liesz et al., 2011; Kleinschnitz et al., 2010; Yilmaz et al., 2006). Moreover, antibody-mediated depletion of CD4+ and CD8+ T cells reduced infarct volume and improved functional outcome, respectively (Mracsko et al., 2014; Gelderblom et al., 2012; Shichita et al., 2009). Antigen-specific activation of cytotoxic CD8+ T cells leads to targeted neuronal cell death by close intercellular interaction and via the perforin–granzyme pathway (Mracsko et al., 2014).
Although ischemic stroke reduces the numbers of lymphocytes in the circulation and lymphoid organs (Prass et al., 2003), subjects with acute ischemic stroke had a significantly higher number of circulating CD4+ T cells in peripheral blood compared to control subjects without acute ischemic lesions (Tuttolomondo et al., 2015). There are also qualitative shifts, and among dominating CD4+ T cells, type Th2 prevails more than Th1. The goal of the response with the domination of Th2 type, a source of anti-inflammatory cytokines, is probably neuroprotection and minimization of stroke lesions (Zhang et al., 2018). One of the SAI risk factors, next to older age, severe clinical status on admission, and large size of infarct lesions, is a decreased number of CD4+ T cells in the initial phase of stroke (Jiang et al., 2017).
Considering the biological role of peripheral immunosuppression and questioning whether it diminishes the deleterious effect of post-stroke systemic inflammation, we aimed to observe the quantitative changes in CD4+ T cells, CD8+ T cells, and DNT, as well as the CD4+/CD8+ ratio, in stroke and SAI. We also considered factors such as stroke lesion volume, neurological status, and comorbidities, particularly those related to vascular disease risk factors.
2 Materials and methods
2.1 Subjects
We studied 52 patients with acute ischemic stroke and 34 control subjects. Patients were recruited from the Stroke Unit at the Department of Neurology and Cerebrovascular Disorders of Poznan University of Medical Sciences, Poznan, Poland, between November 2018 and May 2019. A total of 161 consecutive patients admitted with suspected stroke were prospectively screened. The inclusion criteria were: ischemic stroke, symptom onset within 24 h before clinical evaluation and blood sampling, and age over 40. The clinical diagnosis of ischemic stroke was confirmed based on radiological evidence in cranial computed tomography (CT) scans and/or cranial MRI at admission. Patients with TIA, intracerebral hemorrhage, malignancies, autoimmune diseases, past/acute coronary syndrome, and/or peripheral artery disease within 12 months preceding entry into the study, hematological disorders, liver and renal failure, alcohol, and/or drug abuse, and a history of infection and/or the use of antibiotics and/or immunosuppressants and/or steroids within the preceding 3 months were excluded (Supplementary material 1). Physical and neurological examinations were performed in the acute phase of the stroke on days 1 (D1) and 3 (D3), in the subacute phase on day 10 (D10), and in the convalescent phase, 90 ± 3 days (D90) after stroke. To measure stroke severity, the Scandinavian Stroke Scale (SSS) was used (on the SSS the higher the score, the lesser the neurological deficit) (Table 1). The etiology of the stroke was classified according to the TOAST classification. The diagnostic measures included blood pressure and BMI (individual’s weight in kilograms divided by the square of height in meters). The laboratory investigations include blood counts with automatic smear tests, biochemical, coagulation, and urine tests. Clinical and laboratory assessments were supplemented by chest radiographs, ECG, color Doppler duplex ultrasonography of cervical and vertebral arteries, transcranial Doppler ultrasonography (TCCD), and transthoracic echocardiography. Imaging of the brain with MRI was performed within 24 h from stroke onset and 90 ± 3 days after the stroke (Table 2). We also documented medical history details, including the presence of hypertension, diabetes mellitus, hypercholesterolemia, atrial fibrillation, heart ischemic disease, obesity, smoking, previous strokes, and use of acetylsalicylic acid, anticoagulants, statins, hypotensive drugs, oral diabetics, and non-steroid anti-inflammation drugs (Table 3). All stroke patients were assessed for SAI on day 10 after the stroke. SAI was diagnosed when the following criteria were met: (1) body temperature > 37.8°C in a patient with symptoms suggestive of infection; (2) white blood cell (WBC) count >11,000/mL or <4,000/mL; (3) inflammatory lesions in the chest X-ray; (4) blood or urine culture positive for pathogen; and/or (5) antibiotic or chemotherapeutic therapy, all within 7 days of the onset of stroke symptoms.
The disease control (DC) group consisted of 34 subjects matched for age, sex, and vascular disease risk factors. DC subjects had at least two vascular disease risk factors but had never experienced clinical symptoms of acute cerebral, coronary, or peripheral ischemia. Disease controls underwent the same laboratory tests and procedures as stroke patients except for chest radiograph, ECG, color Doppler duplex ultrasonography of cervical and vertebral arteries, transcranial Doppler ultrasonography (TCCD), transthoracic echocardiography, and blood sampling, which was performed only once.
The study protocol was approved by the bioethics committee of the Poznan University of Medical Sciences (no. 139/2018). Informed consent was obtained from all study subjects.
2.2 Flow cytometry
To detect the absolute number and percentage of CD4+ and CD8+ T cells and CD4−CD8− T cells (DNT), flow cytometry combined with counting was used. In the stroke group, blood samples were collected within 24 h of symptoms onset (D1—the first wave of immune activation), as well as on days 3 (D3—early inflammatory window), 10 (D10—peak in adaptive immune response), and 90 ± 3 (D90—long-term immune changes) after the stroke. In the DC group, blood samples were taken once. Cell immunophenotyping was performed using flow cytometry with the direct fluorescence method. The cells were stained with combinations of the following antibodies: anti-CD3 APC-Cy7, anti-CD4 PE-Cy7, and CD8 PerCp. Test tubes with 15 μL of anti-CD3/CD4/CD8 cocktail combined with 100 μL of peripheral blood, previously sampled with K2EDTA (ethylenediaminetetraacetic acid dipotassium salt) in vacutainer tubes, were gently mixed by a vortex and incubated at room temperature in the dark for 20 min. Then, 500 μL lysis buffer (FASC lysing solution, Becton Dickinson, USA) was added to each test tube, and the tubes were incubated for 10 min. Lysis was stopped when the phosphate-buffered saline solution (PBS, Roche Diagnostic, Germany) was added. The samples were washed by spinning at 250 × g for 4 min to separate residually lysed erythrocytes, plasma proteins, and other blood elements from leukocytes. This step was performed twice. The cell pellets were resuspended in 200 μL of PBS. Data acquisition and analysis were performed using the FACSCantoTM II flow cytometry system and FACS DivaTM software (BD Biosciences) with a standard 6-color filter configuration. Lymphocytes were identified based on cell characteristic properties in the forward (FSC) and side (SSC) scatter. For additional analyses, gates were restricted to CD3+, CD3+CD4+CD8+, and CD3+CD4−CD8−. For each examined antibody, the percentage of positive cells and mean fluorescence intensity (MFI) were determined.
2.3 Infarct volume measurement
To assess infarct lesion volume, MRI sequences on a Siemens, AVANTO 1.5 T, Erlangen, Germany clinical scanner were performed. The standard imaging protocol included: FLAIR, T2-weighted images, diffusion-weighted image (DWI) sequencing with an increasing b-factor, ADC map, and T1-weighted images. Recent hyperintense ischemic lesions were traced according to the segmentation method from DWI with b = 1,000 s/mm2. The measured surface area was multiplied by the thickness of the layers within the visualized ischemic region. In the follow-up MRI examination, performed after 90 ± 3 days, the volume of the lesion was determined using the same method, but in FLAIR sequences. The lesion volume measurement was expressed in milliliters (mL). The typical imaging parameters were as follows: DWI: b = 0–500–1,000–2,000 s/mm2, TR/TE ≈ 4700/113 ms; FLAIR: ≈9,000/86 ms; TI ≈ 2,200 ms; FOV = 24 cm, matrix = 256 × 256; NEX = 1; and base resolution = 256, where: TR—repetition time, TE—echo time, TI—inversion time, FOV—field of view, NEX—number of acquisitions. The concordance of the intra-rater readings was 89.1% [κ = 0.76; 95% confidence interval (CI) 0.64–0.83 for DWI and FLAIR sequences].
2.4 Statistical analyses
Statistical analyses and database management were performed with the software system Statistica, version 13 (Statistica, 2017), and GraphPad Prism, version 8.0.1 (GraphPad Software, San Diego, CA, USA). The sample size was evaluated a priori with standard statistical criteria for the sample size estimation and statistical power. For the data with a normal distribution, the Shapiro–Wilk and Kolmogorov–Smirnov tests were used. The non-normal distribution data were analyzed using non-parametric methods and presented as median values and interquartile range values. Normal distribution data were presented as means ± SD. For comparisons of normally distributed data, the parametric Student’s t-test, ANOVA, and post-hoc Tukey’s test were used. Multiple comparisons within the stroke group were performed using the Wilcoxon signed-rank test with Bonferroni correction; differences were assumed to be significant at a p-value of <0.01. To compare data between patients and the control group, the Mann–Whitney U-test was used. Categorical data were compared using the chi-squared test or Fisher’s exact test, where appropriate. The Spearman rank correlation test was used to test for possible relationships between the studied parameters. A p-value of <0.05 was assumed to be significant, where not indicated otherwise.
3 Results
We analyzed T cells in the peripheral blood of 52 stroke patients (48% were female), with a mean age of 69 ± 12 years. Table 1 shows the demographic and clinical characteristics. On D3, 47 subjects were evaluated—5 patients refused to continue blood sampling; on D10, 45 subjects were evaluated—1 more patient declined to participate in the follow-up visit and 1 patient died; and on D90, 33 subjects were evaluated—11 patients refused to participate in the last follow-up visit due to disability and dependency or inpatient rehabilitation at that time and 1 patient died. The controls were matched with the stroke patient according to the vascular disease risk factors, but atrial fibrillation was more prevalent in the stroke group (42.2% vs. 0%, p < 0.0001) (Table 3). The median time from stroke onset to blood sampling on D1 was 17 h (12–22).
3.1 Kinetics of circulating lymphocytes
The absolute number and the percentage of T cells on post-stroke D1, D3, and D10 were lower than those observed in the controls, and on D1 the percentage of T cells was significantly lower than that measured on D90 (Table 4). The peripheral blood leukocyte count (WBC), the absolute number, and the percentage of CD4+ and CD8+ T cells in the population of CD3+ lymphocytes or CD4−CD8− T cells in the population of CD3+ lymphocytes did not change significantly during the time after the stroke. The values did not differ significantly from those observed in the control group either (Table 5; Figures 1, 2). The CD4+/CD8+ ratio did not change significantly at the time after stroke or when compared to the control group (Figure 3).
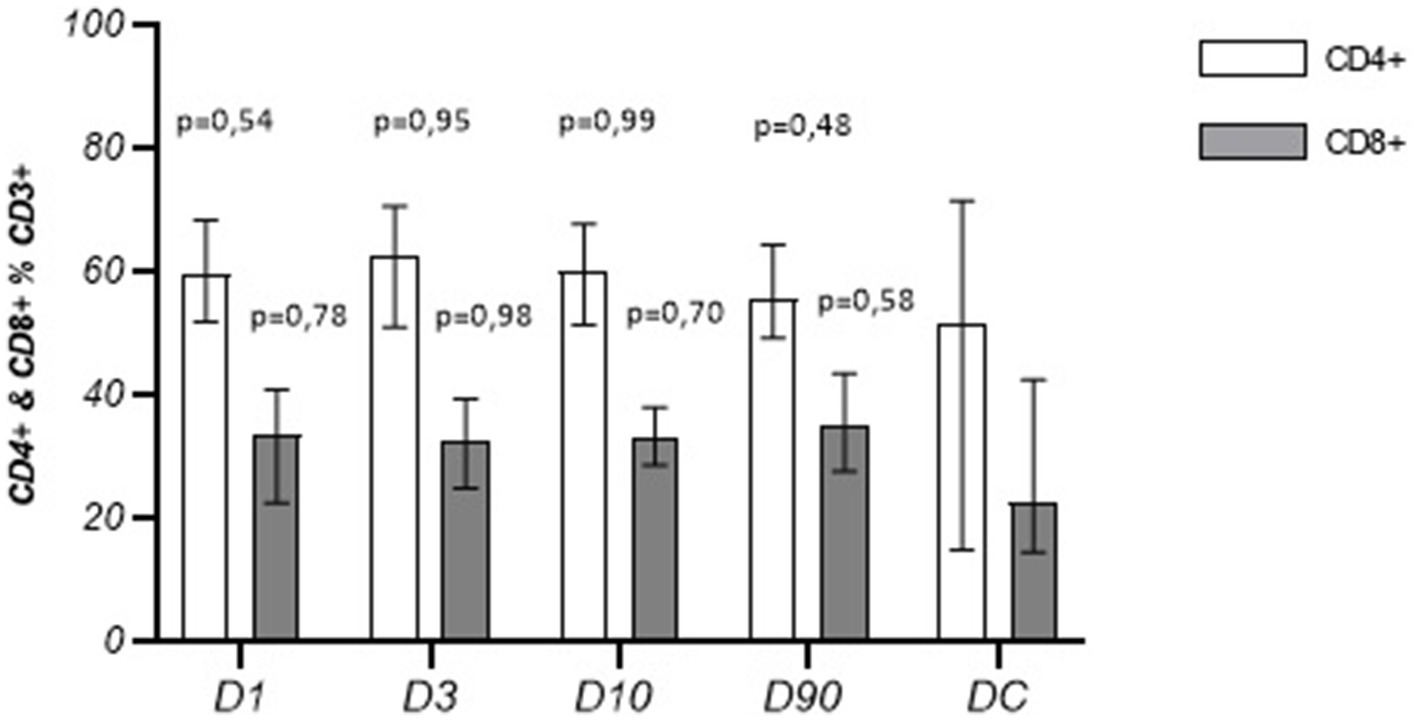
Figure 1. Percentage of CD4+ and CD8+ lymphocytes in the following stroke days in comparison to the disease control group (Me ± QR).
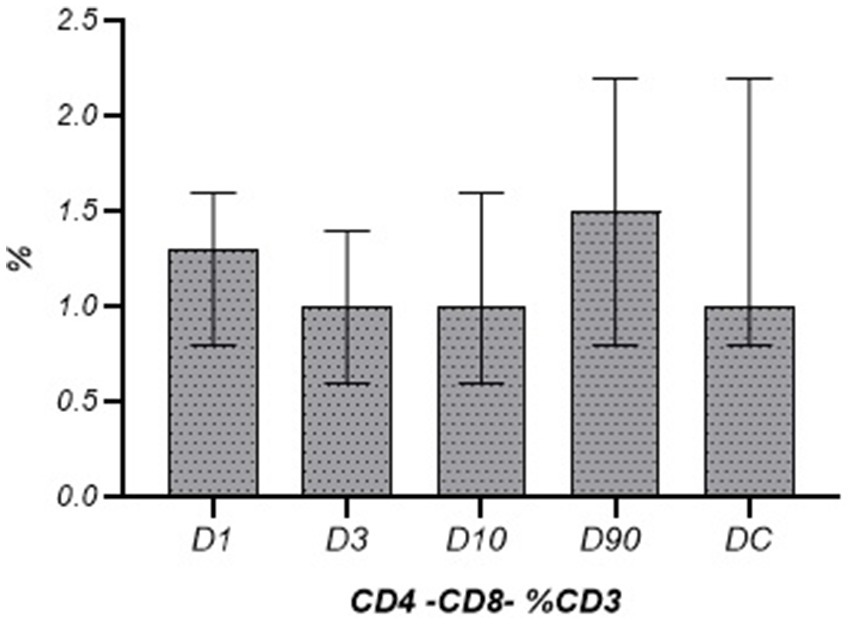
Figure 2. Percentage of CD4−CD8− lymphocytes in the following stroke days in comparison to the disease control group (Me ± QR).
3.2 Lymphocyte subsets and clinical parameters
3.2.1 Neurological status
To determine neurological deficits, patients were assessed on SSS. Significant differences were found between the clinical status of patients on D1 and at other studied time points. The lowest scores in SSS were on D1, while on D3 (p < 0.0001), D10 (p < 0.0001), and D90 (p < 0.0001)—the SSS score increased (p = 0.001). Moreover, a higher score in SSS was revealed on D90 compared to D10 (p = 0.003) and D3 (p = 0.026) (Table 6).
We found a correlation between the percentage of CD4+ or CD8+ T cells and clinical status assessed with SSS. The percentage of CD4+ T cells on D1 positively correlated with clinical status assessed with SSS on D1, D3, and D10. The percentage of CD4+ T cells on D3 and D10 positively correlated with clinical status assessed with SSS on D1 and D3. At the same time points, CD8+ T cells showed an opposite correlation (Table 7). The CD4+/CD8+ ratio on D1 positively correlated with clinical status on D1, D3, and D10, while the CD4+/CD8+ ratio on D3 and D10 positively correlated with clinical status on D1 and D3. This indicates that a higher CD4+/CD8+ ratio correlated with better clinical status during the acute and subacute phases of stroke. No statistically relevant correlation between CD4−CD8− T cells and clinical status was revealed.
3.2.2 SAI and the volume of infarct lesion
Twenty-five patients (48%) met the criteria for SAI. Most of them (56%) presented symptoms of pneumonia, 36% were diagnosed with urinary tract infections, and 8% had other infections. There were no differences between SAI+ and SAI− subjects as well as between SAI+ stroke patients and disease controls in the percentage and the absolute number of circulating CD4+ and CD8+ T cells at any time point after stroke. Nevertheless, the percentage of CD8+ T cells in the acute phase (D1) positively correlated with CRP levels during the acute and subacute phases of stroke (D1, D3, D10), as well as in the control group (Table 8). On the other hand, a negative correlation was noted between the percentage of CD4+ T cells on D1 and the serum level of CRP on D10 after stroke (Table 9). Similarly, the CD4+/CD8+ ratio on D1 negatively correlated with CRP on D1, D3, and D10. No association between the percentage of CD4−CD8− T cells and CRP in stroke patients and controls was found.
There were no associations between infarct volume measured at either D1 or D90, and the percentage of CD4+ T cells, CD8+ T cells, DNT, or the CD4+/CD8+ ratio at any time point after stroke.
3.2.3 Vascular disease risk factors
Forty-two (81%) of the patients were diagnosed with hypertension before stroke. They presented a higher percentage of CD8+ T cells and a lower percentage of CD4+ T cells in the serum than patients without a history of hypertension [CD8+ T cells: 35 (24–43) % vs. 27 (19–33) %, p = 0.023; CD4+ T cells: 57 (49–67) % vs. 66 (64–74) %, p = 0.025] (Figure 4). Such a difference was not found in the disease controls. There were no differences in the CD4+ and CD8+ T cell subpopulations regarding other vascular disease risk factors such as diabetes, hyperlipidemia, atrial fibrillation, or smoking in both studied groups.
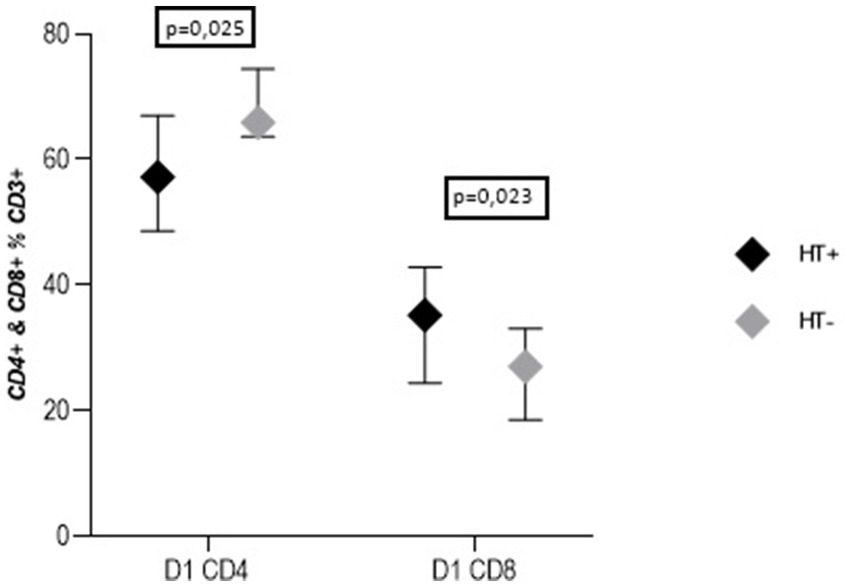
Figure 4. Percentage of CD4+ and CD8+ lymphocytes on day 1 of stroke in patients with hypertension in medical history (HT+) and without hypertension (HT−) (Me ± QR).
4 Discussion
Although leukocytosis and lymphocytopenia are well-known phenomena in systemic reactions to brain infarction, the biological significance of T cell changes in stroke is still being explored. Moreover, the CD4+/CD8+ ratio was never used as a marker of stroke patients’ status.
In the presented study, a decreased absolute number of circulating lymphocytes and a decreased percentage of lymphocytes within WBC during acute and subacute stroke phases were revealed, in comparison to the control group. The absolute number and percentage of CD4+ and CD8+ T cells in a population of CD3+ T cells neither differ in comparison to the control group nor change with the time after stroke. Similarly, T-cell lymphocytopenia, occurring within a few hours after the stroke onset has been described by Chamorro et al. (2006), Ross et al. (2007), Haeusler et al. (2008), Klehmet et al. (2009), and Adamski et al. (2014). Haeusler et al. (2008), Klehmet et al. (2009), and Vogelgesang et al. (2019) observed a rapid decrease in CD4+ T cells, together with a lack of changes within the CD8+ T cells subgroup. Adamski et al. (2014) did not observe any changes in CD4+ and CD8+ T cells, while Jiang et al. (2017) and Liesz et al. (2009b) showed a decreased number of CD4+ and CD8+ T cells in a population of CD3+ T cells in the acute stroke phase. In Hug et al. (2011) research, no changes in CD4+ after stroke were observed.
In the present study, a relevant connection between clinical status and CD4+ or CD8+ T cell percentage was shown. We found that the higher the percentage of CD4+ T cells in the acute and subacute phases of stroke, the better the clinical status, while the higher the percentage of CD8+ T cells, the worse the clinical status. In other words, a higher CD4+/CD8+ ratio related to better clinical conditions for our patients at the beginning of the stroke. A similar correlation was revealed by Vogelgesang et al. (2008), where a higher number of T CD4+ T cells was observed in patients with better clinical status in comparison to those with worse clinical status. Nevertheless, the patients examined by Tuttolomondo et al. (2015) showed an increased number of CD4+ CD28− T cells in the blood associated with stroke severity and serum levels of proinflammatory cytokines. CD4+ CD28− T cells are an interesting subset of T cells that enhance effector functions, are associated with senescent T cells, and expand under inflammatory conditions (Dumitriu, 2015). However, considering post-stroke changes in the proportion of T cells, with a predominance of CD4+ T cells, especially the neuroprotective type of CD4+ T cells—Th2 type (Prass et al., 2003) our findings may confirm the potential benefits of this phenomenon.
Moreover, we observed a positive correlation between CRP levels on D1, D3, and D10 and the percentage of CD8+ T cells on D1. This may indicate a link between CD8+ T cells and inflammation, as well as the potential detrimental role of CD8+ T cells at the very early stage of ischemia. This finding is astonishing, as CD8+ T cells are crucial in the adaptive immune response, and their harmful effects are typically seen in later phases due to their adaptive nature (Selvaraj et al., 2021). Considering the unexpected speed and readiness of the immune system to develop an adaptive response to brain damage in very early stage of stroke, we can establish a hypothesis that immunological changes exist even before the stroke onset. A potential explanation for this situation may be a clinical condition that increase the risk of stroke, such as acute or chronic inflammatory conditions. We also observed a relevant, negative correlation between the percentage of CD4+ T cells on D1 and CRP levels on D10, suggesting that a lower CD4+/CD8+ ratio contributes to inflammation development. On the other hand, this finding may imply a direct influence of CRP on specific T cell populations in stroke. This topic has been explored for years, with researchers investigating receptors for CRP on T cells or other mediating molecules (Nimmerjahn and Ravetch, 2008; Li et al., 2012; Du Clos, 2013). As described by Yoshida et al. (2020), patients with melanoma could bind CRP to CD4+ and CD8+ T cells, inhibiting the proliferation, expansion, and antigen presentation of lymphocytes. Research by Zhang et al. (2015) showed that direct binding of CRP to native CD4+ T cells affects the Th1/Th2 ratio, leading to Th1 suppression, and consequently, regulating the inflammatory response.
Our study found an increased percentage of CD8+ T cells and a decreased percentage of CD4+ T cells during the acute phase of stroke in patients with a history of hypertension (HT), compared to those without HT. The activation of the adaptive immune system in the development of HT has already been demonstrated, with CD8+ T cells identified as responsible for the detrimental effects in HT (Drummond et al., 2019). Recently, a decreased number of CD8+ T cells, with no changes in CD4+ T cell numbers, was observed in stroke patients with a history of HT and a decreased number of CD8+ T cells in patients with HT from the control group in comparison to patients without HT diagnosis (Adamski et al., 2014). In addition to changes in numbers, increased activity of CD8+ T cells, confirmed in experimental studies, highlights their pathological influence (Trott et al., 2014). This led to the conclusion that in patients with HT, activated CD8+ T cells producing proinflammatory cytokines may lead to the promotion of inflammation during the acute phase of stroke.
In the present study, we did not find any statistically significant changes, neither in the absolute number nor percentage of DNT cells, at any time after stroke. We also did not reveal any correlations between DNTs and SAI or clinical outcomes. Our findings are in opposition to Meng et al. (2019) study, where a significantly upregulated DNT population in the peripheral blood of patients with acute stroke was found, suggesting their role in ischemic stroke. The number of DNTs also increased in the brain, and infiltrating DNTs were mainly located close to microglia in the ischemic brain lesions of both stroke patients and mice. Moreover, infiltrating DNTs enhanced immune and inflammatory responses and exacerbated ischemic brain injury (Meng et al., 2019). The reasons for the discrepancies observed are not fully understood, but in both studies, the small sample sizes may limit the statistical analysis and the ability to demonstrate significant and independent correlations.
5 Limitations
Our findings and interpretations should considered within the context of several limitations. These include the single-center research design and small group size, making multivariate statistical analyses unachievable. Additionally, there was a disproportion in the control group with respect to vascular risk factors, such as atrial fibrillation. Laboratory tests were limited to the quantitative assessment of peripheral blood, without functional studies or cytokine matching.
6 Conclusion
In the prospective assessment, the percentage of CD4+ or CD8+ T cells, the CD4+/CD8+ ratio, and DNTs did not change after stroke and showed no significant difference compared to controls. A higher CD4+/CD8+ ratio correlated with better clinical status in the acute and subacute phases of stroke, suggesting a detrimental effect of CD8+ T cells and a beneficial effect of CD4+ T cells already at the early stage of ischemia. The positive correlation between the percentage of CD8+ T cells and CRP levels, along with the predominance of CD8+ T cells in patients with HT, one of the most important risk factors of stroke, may indicate their proinflammatory potential in stroke pathogenesis.
Data availability statement
The raw data supporting the conclusions of this article will be made available by the authors without undue reservation.
Ethics statement
The studies involving humans were approved by Bioethics Committee of the Poznan University of Medical Sciences (no. 139/2018). The studies were conducted in accordance with the local legislation and institutional requirements. The participants provided their written informed consent to participate in this study.
Author contributions
MT: Conceptualization, Data curation, Formal analysis, Investigation, Methodology, Project administration, Software, Supervision, Validation, Visualization, Writing – original draft, Writing – review & editing. MF: Conceptualization, Data curation, Investigation, Methodology, Software, Supervision, Writing – original draft. RK: Conceptualization, Supervision, Writing – review & editing. IW: Data curation, Methodology, Writing – review & editing. GD: Conceptualization, Formal analysis, Methodology, Supervision, Validation, Visualization, Writing – review & editing. WK: Formal analysis, Resources, Supervision, Writing – review & editing. MŁ: Conceptualization, Formal analysis, Funding acquisition, Investigation, Methodology, Project administration, Resources, Software, Supervision, Validation, Visualization, Writing – original draft, Writing – review & editing.
Funding
The author(s) declare that financial support was received for the research and/or publication of this article. This study was supported financially by the governmental research grant National Science Centre [2014/15/B/NZ4/00736] and statutory sources of Poznan University of Medical Sciences.
Conflict of interest
The authors declare that the research was conducted in the absence of any commercial or financial relationships that could be construed as a potential conflict of interest.
Generative AI statement
The authors declare that no Gen AI was used in the creation of this manuscript.
Publisher’s note
All claims expressed in this article are solely those of the authors and do not necessarily represent those of their affiliated organizations, or those of the publisher, the editors and the reviewers. Any product that may be evaluated in this article, or claim that may be made by its manufacturer, is not guaranteed or endorsed by the publisher.
Supplementary material
The Supplementary material for this article can be found online at: https://www.frontiersin.org/articles/10.3389/fncel.2025.1547905/full#supplementary-material
References
Adamski, M. G., Li, Y., Wagner, E., Yu, H., Seales-Bailey, C., Durkin, H., et al. (2014). Pre-existing hypertension dominates γδT cell reduction in human ischemic stroke. PLoS One 9:e97755. doi: 10.1371/journal.pone.0097755
Brandt, D., and Hedrich, C. M. (2018). TCRαβ+CD3+CD4−CD8− (double negative) T cells in autoimmunity. Autoimmun. Rev. 17, 422–430. doi: 10.1016/j.autrev.2018.02.001
Catalfamo, M., and Reali, E. (2020). The latest advances on CD8 T cell biology in health and disease. Mol. Immunol. 124, 198–199. doi: 10.1016/j.molimm.2020.06.009
Chamorro, A., Amaro, S., Vargas, M., Obach, V., Cervera, A., Torres, F., et al. (2006). Interleukin 10, monocytes and increased risk of early infection in ischaemic stroke. J. Neurol. Neurosurg. Psychiatry 77, 1279–1281. doi: 10.1136/jnnp.2006.100800
Chamorro, A., Urra, X., and Planas, A. M. (2007). Infection aftr acute ischemic stroke: a manifestation of brain-induced immunodepression. Stroke 38, 1097–1103. doi: 10.1161/01.STR.0000258346.68966.9d
De Graaf, M. T., Smitt, P. A. E. S., Luitwieler, R. L., van Velzen, C., van den Broek, P. D. M., Kraan, J., et al. (2010). Central memory CD4+ T cells dominate the normal cerebrospinal fluid. Cytometry B Clin. Cytom. 80B, 43–50. doi: 10.1002/cyto.b.20542
Del Prete, G. (1992). Human Th1 and Th2 lymphocytes: their role in the pathophysiology of atopy. Allergy 47, 450–455. doi: 10.1111/j.1398-9995.1992.tb00662.x
Doyle, K. P., Quach, L. N., Sole, M., Axtell, R. C., Nguyen, T. V. V., Soler-Llavina, G. J., et al. (2015). B-lymphocyte-mediated delayed cognitive impairment following stroke. J. Neurosci. 35, 2133–2145. doi: 10.1523/JNEUROSCI.4098-14.2015
Drummond, G. R., Vinh, A., Guzik, T. J., and Sobey, C. G. (2019). Immune mechanisms of hypertension. Nat. Rev. Immunol. 19, 517–532. doi: 10.1038/s41577-019-0160-5
Du Clos, T. W. (2013). Pentraxins: structure, function, and role in inflammation. ISRN Inflamm. 2013:379040. doi: 10.1155/2013/379040
Dumitriu, I. E. (2015). The life (and death) of CD4+ CD28(null) T cells in inflammatory diseases. Immunology 146, 185–193. doi: 10.1111/imm.12506
Gelderblom, M., Leypoldt, F., Steinbach, K., Behrens, D., Choe, C. U., Siler, D. A., et al. (2009). Temporal and spatial dynamics of cerebral immune cell accumulation in stroke. Stroke 40, 1849–1857. doi: 10.1161/STROKEAHA.108.534503
Gelderblom, M., Weymar, A., Bernreuther, C., Velden, J., Arunachalam, P., Steinbach, K., et al. (2012). Neutralization of the IL-17 axis diminishes neutrophil invasion and protects from ischemic stroke. Blood 120, 3793–3802. doi: 10.1182/blood-2012-02-412726
Gill, D., and Veltkamp, R. (2016). Dynamics of T cell responses after stroke. Curr. Opin. Pharmacol. 26, 26–32. doi: 10.1016/j.coph.2015.09.009
Giunti, D., Borsellino, G., Benelli, R., Marchese, M., Capello, E., Valle, M. T., et al. (2003). Phenotypic and functional analysis of T cells homing into the CSF of subjects with inflammatory diseases of the CNS. J. Leukoc. Biol. 73, 584–590. doi: 10.1189/jlb.1202598
Grønberg, N. V., Johansen, F. F., Kristiansen, U., and Hasseldam, H. (2013). Leukocyte infiltration in experimental stroke. J. Neuroinflammation 10, 1–9. doi: 10.1186/1742-2094-10-115
Haeusler, K. G., Schmidt, W. U. H., Föhring, F., Meisel, C., Helms, T., Jungehulsing, G. J., et al. (2008). Cellular immunodepression preceding infectious complications after acute ischemic stroke in humans. Cerebrovasc. Dis. 25, 50–58. doi: 10.1159/000111499
Hersh, J., and Yang, S. H. (2018). Glia–immune interactions post-ischemic stroke and potential therapies. Exp. Biol. Med. 243, 1302–1312. doi: 10.1177/1535370218818172
Hug, A., Liesz, A., Muerle, B., Zhou, W., Ehrenheim, J., Lorenz, A., et al. (2011). Reduced efficacy of circulating costimulatory cells after focal cerebral ischemia. Stroke 42, 3580–3586. doi: 10.1161/STROKEAHA.111.620948
Jiang, C., Kong, W., Wang, Y., Ziai, W., Yang, Q., Zuo, F., et al. (2017). Changes in the cellular immune system and circulating inflammatory markers of stroke patients. Oncotarget 8, 3553–3567. doi: 10.18632/oncotarget.12201
Jin, W. N., Gonzales, R., Feng, Y., Wood, K., Chai, Z., Liu, Q., et al. (2018). Brain ischemia induces diversified neuroantigen-specific T-cell responses that exacerbate brain injury. Stroke 49, 1471–1478. doi: 10.1161/STROKEAHA.118.020203
Klehmet, J., Harms, H., Richter, M., Prass, K., Volk, H. D., Dirnagl, U., et al. (2009). Stroke-induced immunodepression and post-stroke infections: lessons from the preventive antibacterial therapy in stroke trial. Neuroscience 158, 1184–1193. doi: 10.1016/j.neuroscience.2008.07.044
Kleinschnitz, C., Schwab, N., Kraft, P., Hagedorn, I., Dreykluft, A., Schwarz, T., et al. (2010). Early detrimental T-cell effects in experimental cerebral ischemia are neither related to adaptive immunity nor thrombus formation. Blood 115, 3835–3842. doi: 10.1182/blood-2009-10-249078
Li, D., Romain, G., Flamar, A. L., Duluc, D., Dullaers, M., Li, X. H., et al. (2012). Targeting self- and foreign antigens to dendritic cells via DC-ASGPR generates IL-10-producing suppressive CD4+ T cells. J. Exp. Med. 209, 109–121. doi: 10.1084/jem.20110399
Liesz, A., Hagmann, S., Zschoche, C., Adamek, J., Zhou, W., and Sun, L. (2009a). The spectrum of systemic immune alterations after murine focal ischemia: immunodepression versus immunomodulation. Stroke 40, 2849–2858. doi: 10.1161/STROKEAHA.109.549618
Liesz, A., Suri-Payer, E., Veltkamp, C., Doerr, H., Sommer, C., Rivest, S., et al. (2009b). Regulatory T cells are key cerebroprotective immunomodulators in acute experimental stroke. Nat. Med. 15, 192–199. doi: 10.1038/nm.1927
Liesz, A., Zhou, W., Mracsko, E., Karcher, S., Bauer, H., Schwarting, S., et al. (2011). Inhibition of lymphocyte trafficking shields the brain against deleterious neuroinflammation after stroke. Brain 134, 704–720. doi: 10.1093/brain/awr008
Luckheeram, R. V., Zhou, R., Verma, A. D., and Xia, B. (2012). CD4+T cells: differentiation and functions. Clin. Dev. Immunol. 2012:925135. doi: 10.1155/2012/925135
Meisel, C., Schwab, J. M., Prass, K., Meisel, A., and Dirnagl, U. (2005). Central nervous system injury-induced immune deficiency syndrome. Nat. Rev. Neurosci. 6, 775–786. doi: 10.1038/nrn1765
Meng, H., Zhao, H., Cao, X., Hao, J., Zhang, H., Liu, Y., et al. (2019). Double-negative T cells remarkably promote neuroinflammation after ischemic stroke. Proc. Natl. Acad. Sci. 116, 5558–5563. doi: 10.1073/pnas.1814394116
Mracsko, E., Liesz, A., Stojanovic, A., Lou, W. P., Osswald, M., Zhou, W., et al. (2014). Antigen dependently activated cluster of differentiation 8-positive T cells cause perforin-mediated neurotoxicity in experimental stroke. J. Neurosci. 34, 16784–16795. doi: 10.1523/JNEUROSCI.1867-14.2014
Nimmerjahn, F., and Ravetch, J. V. (2008). Fc gamma receptors as regulators of immune responses. Nat. Rev. Immunol. 8, 34–47. doi: 10.1038/nri2206
Prass, K., Meisel, C., Höflich, C., Braun, J., Halle, E., Wolf, T., et al. (2003). Stroke- induced immunodeficiency promotes spontaneous bacterial infections and is mediated by sympathetic activation reversal by poststroke T helper cell type 1-like immunostimulation. J. Exp. Med. 198, 725–736. doi: 10.1084/jem.20021098
Ransohoff, R. M., Kivisakk, P., and Kidd, G. (2003). Three or more routes for leukocyte migration into the central nervous system. Nat. Rev. Immunol. 3, 569–581. doi: 10.1038/nri1130
Ross, A. M., Hurn, P., Perrin, N., Wood, L., Carlini, W., and Potempa, K. (2007). Evidence of the peripheral inflammatory response in patients with transient ischemic attack. J. Stroke Cerebrovasc. Dis. 16, 203–207. doi: 10.1016/j.jstrokecerebrovasdis.2007.05.002
Selvaraj, U. M., and Stowe, A. M. (2017). Long-term T cell responses in the brain after an ischemic stroke. Discov. Med. 24, 323–333
Selvaraj, U. M., Ujas, T. A., Kong, X., Kumar, A., Plautz, E. J., Zhang, S., et al. (2021). Delayed egress of CD8 T cells contributes to long-term pathology after ischemic stroke in male mice. Brain Behav. Immun. 95, 502–513. doi: 10.1016/j.bbi.2021.05.001
Shichita, T., Sugiyama, Y., Ooboshi, H., Sugimori, H., Nakagawa, R., Takada, I., et al. (2009). Pivotal role of cerebral interleukin-17–producing γδT cells in the delayed phase of ischemic brain injury. Nat. Med. 15, 946–950. doi: 10.1038/nm.1999
Stevens, S. L., Bao, J., Hollis, J., Lessov, N. S., Clark, W. M., and Stenzel-Poore, M. P. (2002). The use of flow cytometry to evaluate temporal changes in inflammatory cells following focal cerebral ischemia in mice. Brain Res. 932, 110–119. doi: 10.1016/s0006-8993(02)02292-8
Stuve, O., Marra, C. M., Bar-Or, A., Niino, M., Cravens, P. D., Cepok, S., et al. (2006). Altered CD4+/CD8+ T-cell ratios in cerebrospinal fluid of natalizumab-treated patients with multiple sclerosis. Arch. Neurol. 63, 1383–1387. doi: 10.1001/archneur.63.10.1383
Trott, D. W., Thabet, S. R., Kirabo, A., Saleh, M. A., Itani, H., Norlander, A. E., et al. (2014). Oligoclonal CD8+ T cells play a critical role in the development of hypertension. Hypertension 64, 1108–1115. doi: 10.1161/HYPERTENSIONAHA.114.04147
Tuttolomondo, A., Pecoraro, R., Casuccio, A., Di Raimondo, D., Buttà, C., Clemente, G., et al. (2015). Peripheral frequency of CD4+ CD28- cells in acute ischemic stroke: relationship with stroke subtype and severity markers. Medicine 94:e813. doi: 10.1097/MD.0000000000000813
Vogelgesang, A., Grunwald, U., Langner, S., Jack, R., Broker, B. M., Kessler, C., et al. (2008). Analysis of lymphocyte subsets in patients with stroke and their influence on infection after stroke. Stroke 39, 237–241. doi: 10.1161/STROKEAHA.107.493635
Vogelgesang, A., Witt, C., Heuer, C., Schulze, J., Gellrich, J., von Sarnowski, B., et al. (2019). Clinical improvement following stroke promptly reverses post-stroke cellular immune alterations. Front. Neurol. 10:414. doi: 10.3389/fneur.2019.00414
Yilmaz, G., Arumugam, T. V., Stokes, K. Y., and Granger, D. N. (2006). Role of T lymphocytes and interferon-gamma in ischemic stroke. Circulation 113, 2105–2112. doi: 10.1161/CIRCULATIONAHA.105.593046
Yoshida, T., Ichikawa, J., Giuroiu, I., Laino, A. S., Hao, Y., Krogsgaard, M., et al. (2020). C reactive protein impairs adaptive immunity in immune cells of patients with melanoma. J. Immunother. Cancer 8:e000234. doi: 10.1136/jitc-2019-000234
Zhang, L., Liu, S. H., Wright, T. T., Shen, Z. Y., Li, H. Y., Zhu, W., et al. (2015). C- reactive protein directly suppresses Th1 cell differentiation and alleviates experimental autoimmune encephalomyelitis. J. Immunol. 194, 5243–5252. doi: 10.4049/jimmunol.1402909
Zhang, S. R., Piepke, M., Chu, H. X., Broughton, B. R. S., Shim, R., Wong, C. H. Y., et al. (2018). IL-33 modulates inflammatory brain injury but exacerbates systemic immunosuppression following ischemic stroke. JCI Insight 3:e121560. doi: 10.1172/jci.insight.121560
Keywords: ischemic stroke, stroke associated infection, CD4+ T cells, CD8+ T cells, CD4+/CD8+ratio, double negative T cells
Citation: Telec M, Frydrychowicz M, Kazmierski R, Wojtasz I, Dworacki G, Kozubski W and Łukasik M (2025) Circulating CD4+, CD8+, and double-negative T cells in ischemic stroke and stroke-associated infection: a prospective case-control study. Front. Cell. Neurosci. 19:1547905. doi: 10.3389/fncel.2025.1547905
Edited by:
Sabrina Petralla, Heidelberg University, GermanyReviewed by:
Esra Yalcin, Boston Children’s Hospital and Harvard Medical School, United StatesMayur Doke, University of Miami Health System, United States
Copyright © 2025 Telec, Frydrychowicz, Kazmierski, Wojtasz, Dworacki, Kozubski and Łukasik. This is an open-access article distributed under the terms of the Creative Commons Attribution License (CC BY). The use, distribution or reproduction in other forums is permitted, provided the original author(s) and the copyright owner(s) are credited and that the original publication in this journal is cited, in accordance with accepted academic practice. No use, distribution or reproduction is permitted which does not comply with these terms.
*Correspondence: Magdalena Telec, bXRlbGVjQHVtcC5lZHUucGw=