- 1Division of Applied Life Sciences, Graduate School of Life and Environmental Sciences, Kyoto Prefectural University, Kyoto, Japan
- 2Department of Sport Research, Japan Institute of Sports Sciences, Tokyo, Japan
Skeletal muscles secrete various factors, such as proteins/peptides, nucleotides, and metabolites, which are referred to as myokines. Many of these factors are transported into extracellular bodily fluids in a free or protein-bound form. Furthermore, several secretory factors have been shown to be wrapped up by small vesicles, particularly exosomes, secreted into circulation, and subsequently regulate recipient cells. Thus, exosome contents can be recognized as myokines. In recipient cells, proteins, microRNAs, and metabolites in exosomes can regulate the expression and activity of target proteins associated with nutrient metabolism and immune function. The levels of circulating exosomes and their contents are altered in muscle disorders and metabolic-related states, such as metabolic dysfunction, sarcopenia, and physical fitness. Therefore, such circulating factors could mediate various interactions between skeletal muscle and other organs and may be useful as biomarkers reflecting physiological and pathological states associated with muscular function. Here, this review summarizes secretory regulation of muscle-derived exosomes. Their metabolic and immunological roles and the significance of their circulating levels are also discussed.
Introduction
In the last 20 years, skeletal muscle has emerged as a secretory organ. Early studies showed that interleukin-6 (IL-6) is a typical secretory protein produced by muscle cells (1). Thereafter, various proteins and peptides were identified as muscle-secreted factors. Classically, muscles are known to generate and secrete a metabolite, lactate through glycolysis during muscle contraction. The majority of lactate is consumed as an energy substrate in metabolic organs, a process known as the “lactate shuttle” (2). Furthermore, novel metabolites have been reported using developed metabolomic analysis techniques. Many secretory factors are transported into the circulation and mediate the communication between skeletal muscle and other organs, referred to as the myokine theory (1). In recipient cells, myokines can regulate the expression and activity of target proteins in the muscle itself in autocrine and paracrine manners or other organs via an endocrine route, thereby contributing to physiological and pathological phenotypes, such as metabolic capacity, muscle mass, bone density, hormone secretion, cognitive function, and tumorigenesis.
Many secretory factors exist in free form or in a protein-bound form in circulation. Growing evidence shows that several secreted factors also contain in extracellular vesicles (EVs). Generally, two major subtypes of EVs have been characterized with differences of their biogenesis. In contrast to plasma membrane-derived “microvesicles”, “exosomes” are defined as the EVs derived from endosome (3). Although it is difficult to distinguish each subtype completely, EVs differ based their components and functions. A noncoding RNA, microRNA (miRNA) is a typical component. Over 60% of protein-coding genes may be regulated by miRNAs (4), and many of these miRNAs are thought to play important roles in a range of biological processes. Some intracellular miRNAs are wrapped up by small vesicles, particularly exosomes, secreted into circulation, and then regulate recipient cells. Indeed, over 1000 RNAs have been detected in exosomes (5), in addition to proteins/peptides, lipids, and metabolites. Secreted exosomes dock to the plasma membrane of target cells, where they can bind or fuse with the plasma membrane or be endocytosed and then deliver their cargo (6). Through this process, exosomes mediate cell-cell and organ-organ communication (Figure 1). Skeletal muscle cells secrete exosomes; thus, their contents can be recognized as myokines and have potential functions over free-form secretory factors.
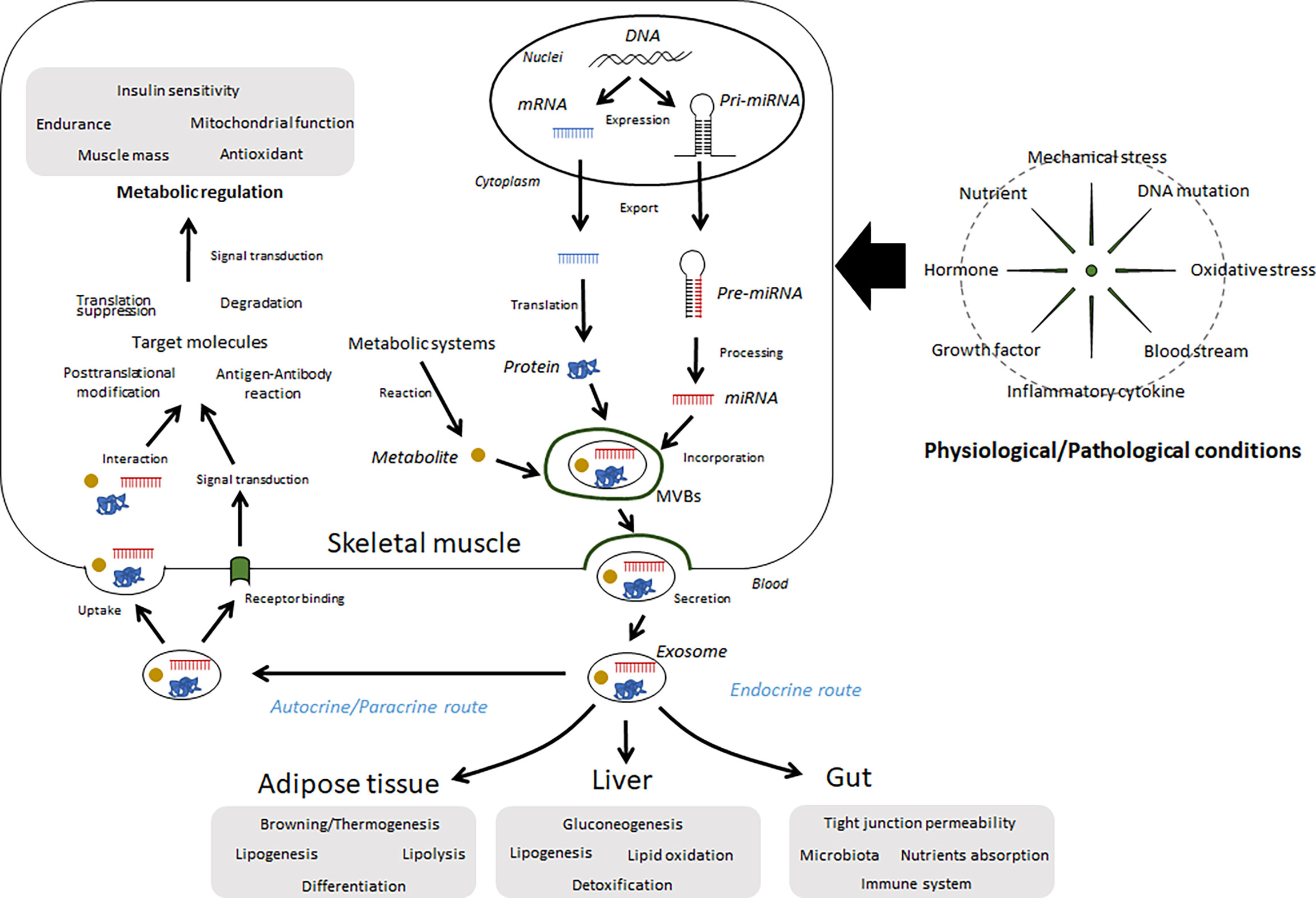
Figure 1 Summary of the regulation and functions of exosomes in skeletal muscle. Several miRNAs, proteins, and metabolites are wrapped up by intracellular vesicles, such as exosomes, in skeletal muscle cells and secreted into extracellular fluids. These components can be affected by various conditions, including physical activity, diet, diseases, and aging. Circulating exosomes migrate to the skeletal muscle as well as other organs and regulate metabolic and immunological functions.
Here, we discuss the significance of circulating exosomes and their contents in the context of various physiological, and pathological states, such as muscle disorders, lifestyle-related diseases, and physical fitness.
Exosome Components and Functions Secreted From Skeletal Muscle
The production of EVs, which includes exosomes, by skeletal muscle cells was first demonstrated by Guescini et al. (7). In a proteomic assay, muscle-derived exosomes were found to contain exosome-associated proteins and signal transduction proteins. Interestingly, mitochondrial DNA was also shown to be taken up into exosomes and released into the extracellular medium in cultures of C2C12 cells (7). In parallel, miRNAs have been reported to be present in blood. Several miRNAs are highly enriched in muscle tissues and are often referred to as myomiRs. Four myomiRs, namely, miR-1, miR-133a, miR-133b, and miR-206, together account for nearly 25% of miRNA expression in skeletal muscles in both humans and mice (8, 9) and contribute to the development of animal and human skeletal muscle cells (10, 11). Skeletal muscle cells were shown to secrete exosomes containing these four myomiRs (12, 13), which were found to be involved in myoblast differentiation into myotubes via an autocrine route. In addition, several animal and human studies have suggested the existence of some circulating myomiRs in the living body (14–16). Recently, intact skeletal muscle tissues secrete exosomes containing miR-1, miR-133a, miR-133b, and miR-206, which depends on their expression levels in the tissues (13). In addition to the four miRNAs, several muscle-enriched miRNAs, including miR-208, miR-486, and miR-499, have also been identified in circulation (14, 17). Additionally, in human satellite cells, extracellular guanosine 5’-triphosphate was shown to increase the level of myomiRs and also induce the release of exosomes containing guanosine (18). These studies suggest that circulating muscle-specific miRNAs likely can act as myogenetic factors in autocrine and paracrine manners, and some are even released into circulation (13). However, their involvement in sarcopenia and training-induced muscle hypertrophy is unclear.
Although few proteins have been identified in exosomes secreted from skeletal muscle, several proteins related to the growth and metabolism of muscle have been reported. Proteomic findings using two-dimensional gel electrophoresis and liquid chromatography tandem mass spectrometry have shown that exosomes secreted from C2C12 cells show different in compositions after differentiation into myoblasts and myotubes. Several protein components, including integrin subunit beta 1, CD9, CD81, neural cell adhesion molecule, CD44, and myoferlin, likely play key roles in the differentiation of these cells (19). Additionally, growth factors such as insulin-like growth factors and fibroblast growth factor-2 are also present in exosomes from differentiating human skeletal muscle cells (20). Such exosomes likely contribute to skeletal myogenesis. A previous study showed that mouse and human myoblasts cultured in exosome-depleted serum form fewer myotubes than myoblasts grown in normal serum (21). This effect was not reversed, even when the medium was replaced with normal medium. Moreover, the expression levels of myomiRs, such as miR-1, miR-206, and miR-133a, are decreased during myoblast proliferation. As mentioned above, exosomes containing guanosine may also contribute to regulated contents of myomiRs (18). In addition, differentiating C2C12 exosomes increase motor neuron survival in NSC-34 cells (22). Therefore, skeletal muscle-secreted exosomes can mediate the maintenance of physiological homeostasis in muscle tissue in autocrine and endocrine manners.
In general, there are two pathways for exosome formation: endosomal sorting complex required for transport (ESCRT)-dependent and ESCRT-independent pathways (23). ESCRT-dependent pathways involve four types of ESCRT complexes and the proteins that bind to them which regulate protein recruitment, vesicle budding and separation. On the other hand, the ESCRT-independent pathway is mediated by sphingolipid ceramide via neutral sphingomyelinase. Although the regulatory mechanism of their biogenesis and secretion in muscle have not been fully clarified, several studies have provided some information. Using proteomic and bioinformatic analysis, Forterre et al. (12) suggested the existence of ESCRT proteins in exosomes from C2C12 differentiation. The release of EVs from C2C12 myoblasts was found to be modulated by intracellular ceramide levels in a Ca2+-dependent manner (15). In mdx mice, the inhibition of ceramide synthesis suppressed EV secretion and improved muscle dystrophy (15).
Since the report by Valadi et al. (5), evidences regarding not only the secretory mechanism but also body dynamism of exosomes have been developed. Particularly, Aswad et al. (24) showed that information. fluorescently labeled muscle-derived exosomes injected into mice were found to be taken up into various tissues, including the lung, liver, spleen, brain, heart, pancreas, and gastrointestinal tract. In addition, a high-fat diet was shown to accelerate the release of exosomes from muscle tissues in mice (24). Muscle-derived exosomes from mice fed a high-fat diet induced myoblast proliferation and altered the expression of cell cycle and differentiation factors. These findings suggest that muscle-derived EVs have paracrine signaling functions via alteration of muscle homeostasis in response to a high-fat diet and may have endocrine functions via targeting other tissues in vivo. The same group showed that EVs from the muscles of mice on a high-fat diet increased the sizes of isolated islets in vitro and induced the proliferation of murine insulin-secreting MIN6B1 cells (25).
Exosomes in Physical Activity/Muscle Contraction
Skeletal muscles function as a supporting organ during physical activity. The muscles consume lipids and glucose as substrates and generate energy for muscle contraction; thus, exercise training adaptively leads to muscle building and endurance. In addition, daily physical activity reduces the risk of various noncommunicable diseases, such as cardiovascular disease, type 2 diabetes, and cancer. Habitual exercise also counteracts the development of age-related muscle atrophy by regulating myogenesis and protein metabolism. Muscle-secreted factors have been known to contribute to physical fitness, preventive diseases, and anti-aging effects.
Initial studies have shown that circulating miRNAs are altered in response to a single bout of exercise and training (14, 26–30). However, the miRNAs are not limited to the cargo in exosomes and their secretory sources cannot be identified. In a last decade, EVs have been isolated in circulation and their secretory characteristics examined in physiological and pathological states (Table 1). Differences in exosomal or vesicle-free contents may affect the results. For example, Guescini et al. (31) reported that muscle-enriched miR-133b and miR-181a-5p in EVs were elevated after acute exercise, and a positive correlation was found between aerobic fitness and circulating muscle-enriched miRNAs. Additionally, Yin et al. (17) examined the secretory dynamics of cell-free and exosomal muscle-enriched miRNAs with exercise patterns in rats. In cell-free miRNAs, only miR-1 and miR-133a were increased immediately after uphill exercise, whereas more miRNAs (miR-1, miR-133a, miR-133b, miR-206, miR-208a, and miR-499) increased after downhill running. In exosomes, only miR-133a increased following uphill running, whereas miR-1, miR-133a, and miR-499 increased after downhill running (17). Mild to moderate muscle-damaging exercise affects miR-31 in circulating EVs after exercise but does not alter myomiRs in EVs (32). Although the levels of exosomal miRNAs do not change as easily in response to exercise, training status can influence the response. For example, Nair et al. (33) showed that regular exercise adaptively increased baseline levels of miR-486-5p, miR-215-5p, and miR-941 and decreased the levels of miR-151b. These miRNAs were associated with IGF-1 signaling. In particular, circulating miR-486, a muscle-enriched miRNA, is altered in response to exercise and correlated with endurance capacity (14), suggesting that it may act on certain recipient cells. A major putative target of miR-486 is phosphatase and tensin homolog (PTEN), which is a negative regulator of insulin signal transduction. Therefore, exosomal miR-486 has a potential for protein synthesis through the IGF-1/Akt/mTOR pathway. Release of EVs into circulation in response to exercise is also affected by the physical characteristics of the individuals, including sex, body mass index, and fitness level (44). Furthermore, as reported by Whitham et al. (45), many proteins are contained in exosomes and small vesicles, and their profiles can be changed by exercise. Therefore, future investigations should focus on proteins and peptides as cargo in exosomes.
Muscle-Derived Exosomes and Noncommunicable Diseases
miRNAs have been shown to function as modulators of myogenesis, muscle mass, and metabolic capacity in skeletal muscle (46–48). In addition, several muscle-enriched miRNAs are released into the circulation, and these miRNAs can be altered by muscle disorders (Table 1). Therefore, several researchers have investigated the functions of these miRNAs in the muscle itself and in other organs through paracrine and endocrine routes. In animal models and human patients of Duchenne muscular dystrophy, circulating levels of miR-1, miR-133a, and miR-206 were suggested to be increased and were correlated with the progression of muscle pathology (34, 35). Another neuromuscular disorder, denervation, resulted in higher levels of miR-206 and lower levels of miR-1, miR-133a, and miR-133b in muscle-derived exosomes (16). These secretory characteristics of miRNAs may be closely involved in the development of pathological muscle conditions. Indeed, exposure of C2C12 muscle cells to EVs from the serum of mdx mice resulted in a decrease in cell death (15). Moreover, inhibition of ceramide synthesis and injection with GW4869, an inhibitor of exosome secretion, ameliorate muscular dystrophy in mdx mice, suggesting that EVs and myomiRs are closely associated with skeletal muscle degeneration.
Several exosomal contents have been shown to change with associated metabolic dysfunction. For example, miR-23a levels were found to be decreased in atrophied gastrocnemius muscles from streptozotocin-induced diabetic rats. Additionally, miR-23a was present in exosomes isolated from the medium of C2C12 myotubes, and dexamethasone treatment increased its abundance in exosomes (36). Thus, selective packaging of miR-23a into exosomes may reduce its content in muscles. Jalabert et al. (25) found that skeletal muscle-derived exosomes injected into mice could specifically target miR pancreatic islet cells and affect gene expression and proliferation of βcells. Although direct evidence has not yet shown whether muscle-derived exosomes can influence insulin secretion, regular exercise is known to promote the secretory capacity of insulin (49, 50). Karolina et al. (37) found that circulating miR-144 levels are increased in animals and humans with type 2 diabetes. This elevation is negatively correlated with insulin receptor substrate 1 in insulin-responsive tissues, including skeletal muscle, suggesting that the elevation of miR-144 levels in circulation may be associated with the development of insulin resistance. Furthermore, overexpression of miR-23a/-27a in muscle prevents diabetes-induced muscle cachexia and attenuates renal fibrosis lesions via muscle-kidney crosstalk (38). In addition, overexpression of miR-26 in muscle prevents chronic kidney disease (CKD)-induced muscle wasting via exosomal miR-26a (39). This result supports the potential therapeutic applications of exosome delivery of specific miRNAs to treat and prevent CKD and sarcopenia.
Levels of muscle-specific miRNAs, i.e., miR-1, miR-206, and miR-499, were also higher in the plasma of patients with chronic obstructive pulmonary disease (COPD), who often have a sarcopenic condition (40). In plasma samples from 103 patients with COPD and healthy controls, plasma miR-499 and miR-206 levels were associated with inflammatory responses in skeletal muscle and circulation (40). This may cause sarcopenic conditions in patients with COPD. In addition, serum levels of myomiRs (i.e., miR-1, miR-133a, miR-133b, and miR-206) were significantly higher in patients with rhabdomyosarcoma tumors than in those with non-rhabdomyosarcoma tumors (41). Ischemia-reperfusion stimulation of skeletal muscle induces different responses of circulating exosomal proteins between nuclear factor-κB-knockout and wild-type mice (43). The results showed that various proteins, including complement component C3 prepropeptide, PK-120 precursor, alpha-amylase one precursor, beta-enolase isoform 1, adenylosuccinate synthetase isozyme 1, and glyceraldehyde-3-phosphate dehydrogenase, were altered. Interestingly, exosome-like vesicles released from inflamed myotubes induce myoblast inflammation and inhibit myogenic mechanisms while stimulating atrophic signals (51), supporting the roles of muscle-derived EVs in the development of inflammatory diseases via organ-organ crosstalk.
Several studies have shown that aging and dietary habits can also affect the quality of muscle-derived exosomes. An aging-dependent miRNA, miR-29b-3p, was observed in exosomes isolated from differentiated atrophic myotubes. The miR-29b-3p-containing exosomes released from myotubes were taken up by neuronal SH-SY5Y cells, leading to downregulation of neuronal-related genes and inhibition of neuronal differentiation. Muscle-derived miR-34a also increases with age in circulating EVs and induces the senescence of bone marrow stem cells (42). Thus, such age-associated exosomes in circulation may promote aging through modulation of crosstalk between muscle cells and neurons. In addition, a high-fat diet can promote the secretion of exosomes from skeletal muscles, thereby affecting proliferation and differentiation of myoblast (24). Thus, exosomes contribute to lipid transfer through mediating communication between cells in muscle tissues. Regarding the cardiovascular system, the muscle-derived exosomes with angiogenic miRNAs such as miR-130a, induces angiogenesis via redox signaling in endothelial cells (52). This prevents age-related loss of capillarization, found in type 2 diabetes and chronic heart failure. Furthermore, the influence on the function and survival of β-cells under normal and diabetic conditions has been discussed (25). miR-133a, miR-206, and miR-16 in muscle-derived exosomes likely result in the abnormal regulation of insulin secretion in pancreatic islets (53). In contrast, miR-133a, miR-133b, and miR-206 may have a tumor suppressive function. Under several cancer conditions, the levels of these myomiRs levels in circulation are lower than in the normal conditions (54). Although the relationship between exosomes and disease is still unclear, these observations support the significant role of muscle-derived exosomes in physiological homeostasis and pathological changes.
Conclusion
In this review, we discussed the roles of exosomes in metabolic and immunological communication between muscles and other organs. Many components are contained in muscle-derived exosomes and are likely contributed to maintaining or impairing the homeostasis of muscles and organs throughout the body. Abnormalities in glucose tolerance and lipid metabolism occur as a result of impaired metabolic function of skeletal muscle. Furthermore, it is now known that age-related muscle atrophy, or sarcopenia, is associated with central diseases including respiratory and cardiovascular diseases, chronic kidney disease, dementia, and depression. There is also a negative correlation between physical activity and the risk of developing cancer, as well as an association between intestinal bacteria and leaky gut syndrome. Exosomes may be involved in these associations between skeletal muscle and multiple organs. Thus far, the search for bioactive factors in circulation has targeted free proteins, peptides, and metabolites, but in the future it is necessary to include the components encapsulated in exosomes. In addition, exosomes have potential applications as biomarkers reflecting metabolic and immunological conditions. However, it is unclear whether muscle-derived exosomes can be useful as a biomarker to reflect physical fitness, diseases, or aging. Many factors lack specificity because common factors are secreted by multiple organs and different stimuli. Therefore, special factors with highly expressed in skeletal muscle should be found in a future. In contrast to the identification of many miRNAs in exosomes, few proteins and metabolites have been identified, although it is assumed that many exist. Accordingly, further studies are needed to elucidate the detailed characteristics of exosomes under physiological and pathological conditions.
Author Contributions
WA and YT wrote the manuscript. All authors contributed to the article and approved the submitted version.
Funding
This work was supported by Grants-in-Aid for Scientific Research (B) KAKENHI Grant Number JP20H04080 (WA) from Japan Society for the Promotion of Science and the grant from the Uehara Memorial Foundation.
Conflict of Interest
The authors declare that the research was conducted in the absence of any commercial or financial relationships that could be construed as a potential conflict of interest.
Publisher’s Note
All claims expressed in this article are solely those of the authors and do not necessarily represent those of their affiliated organizations, or those of the publisher, the editors and the reviewers. Any product that may be evaluated in this article, or claim that may be made by its manufacturer, is not guaranteed or endorsed by the publisher.
References
1. Pedersen BK, Steensberg A, Fischer C, Keller C, Keller P, Plomgaard P, et al. Searching for the Exercise Factor: Is IL-6 a Candidate? J Muscle Res Cell Motil (2003) 24:113–9. doi: 10.1023/a:1026070911202
2. Gilles R, S. International Union of Biological, P. Section of Comparative, Biochemistry, P. International Congress of Comparative, and Biochemistry. Circulation, Respiration, and Metabolism Current Comparative Approaches. Springer-Verlag Berlin Heidelberg (1985). pp. 208–18.
3. Cocucci E, Meldolesi J. Ectosomes and Exosomes: Shedding the Confusion Between Extracellular Vesicles. Trends Cell Biol (2015) 25:364–72. doi: 10.1016/j.tcb.2015.01.004
4. Friedman RC, Farh KK, Burge CB, Bartel DP. Most Mammalian mRNAs Are Conserved Targets of microRNAs. Genome Res (2009) 19:92–105. doi: 10.1101/gr.082701.108
5. Valadi H, Ekstrom K, Bossios A, Sjostrand M, Lee JJ, Lotvall JO. Exosome-Mediated Transfer of mRNAs and microRNAs Is a Novel Mechanism of Genetic Exchange Between Cells. Nat Cell Biol (2007) 9:654–9. doi: 10.1038/ncb1596
6. Raposo G, Stoorvogel W. Extracellular Vesicles: Exosomes, Microvesicles, and Friends. J Cell Biol (2013) 200:373–83. doi: 10.1083/jcb.201211138
7. Guescini M, Guidolin D, Vallorani L, Casadei L, Gioacchini AM, Tibollo P, et al. C2C12 Myoblasts Release Micro-Vesicles Containing mtDNA and Proteins Involved in Signal Transduction. Exp Cell Res (2010) 316:1977–84. doi: 10.1016/j.yexcr.2010.04.006
8. Sempere LF, Freemantle S, Pitha-Rowe I, Moss E, Dmitrovsky E, Ambros V. Expression Profiling of Mammalian microRNAs Uncovers a Subset of Brain-Expressed microRNAs With Possible Roles in Murine and Human Neuronal Differentiation. Genome Biol (2004) 5:R13. doi: 10.1186/gb-2004-5-3-r13
9. McCarthy JJ. MicroRNA-206: The Skeletal Muscle-Specific Myomir. Biochim Biophys Acta (2008) 1779:682–91. doi: 10.1016/j.bbagrm.2008.03.001
10. Sweetman D, Goljanek K, Rathjen T, Oustanina S, Braun T, Dalmay T, et al. Specific Requirements of MRFs for the Expression of Muscle Specific microRNAs, miR-1, miR-206 and miR-133. Dev Biol (2008) 321:491–9. doi: 10.1016/j.ydbio.2008.06.019
11. Koutsoulidou A, Mastroyiannopoulos NP, Furling D, Uney JB, Phylactou LA. Expression of miR-1, miR-133a, miR-133b and miR-206 Increases During Development of Human Skeletal Muscle. BMC Dev Biol (2011) 11:34. doi: 10.1186/1471-213X-11-34
12. Forterre A, Jalabert A, Chikh K, Pesenti S, Euthine V, Granjon A, et al. Myotube-Derived Exosomal miRNAs Downregulate Sirtuin1 in Myoblasts During Muscle Cell Differentiation. Cell Cycle (2014) 13:78–89. doi: 10.4161/cc.26808
13. Mytidou C, Koutsoulidou A, Katsioloudi A, Prokopi M, Kapnisis K, Michailidou K, et al. Muscle-Derived Exosomes Encapsulate myomiRs and Are Involved in Local Skeletal Muscle Tissue Communication. FASEB J (2021) 35:e21279 doi: 10.1096/fj.201902468RR
14. Aoi W, Ichikawa H, Mune K, Tanimura Y, Mizushima K, Naito Y, et al. Muscle-Enriched microRNA miR-486 Decreases in Circulation in Response to Exercise in Young Men. Front Physiol (2013) 4:80. doi: 10.3389/fphys.2013.00080
15. Matsuzaka Y, Tanihata J, Komaki H, Ishiyama A, Oya Y, Ruegg U, et al. Characterization and Functional Analysis of Extracellular Vesicles and Muscle-Abundant miRNAs (miR-1, miR-133a, and miR-206) in C2C12 Myocytes and Mdx Mice. PloS One (2016) 11:e0167811. doi: 10.1371/journal.pone.0167811
16. De Gasperi R, Hamidi S, Harlow LM, Ksiezak-Reding H, Bauman WA, Cardozo CP. Denervation-Related Alterations and Biological Activity of miRNAs Contained in Exosomes Released by Skeletal Muscle Fibers. Sci Rep (2017) 7:12888. doi: 10.1038/s41598-017-13105-9
17. Yin X, Zhao Y, Zheng YL, Wang JZ, Li W, Lu QJ, et al. Time-Course Responses of Muscle-Specific MicroRNAs Following Acute Uphill or Downhill Exercise in Sprague-Dawley Rats. Front Physiol (2019) 10:1275. doi: 10.3389/fphys.2019.01275
18. Pietrangelo T, Di Filippo ES, Locatelli M, Piacenza F, Farina M, Pavoni E, et al. Extracellular Guanosine 5'-Triphosphate Induces Human Muscle Satellite Cells to Release Exosomes Stuffed With Guanosine. Front Pharmacol (2018) 9:152. doi: 10.3389/fphar.2018.00152
19. Forterre A, Jalabert A, Berger E, Baudet M, Chikh K, Errazuriz E, et al. Proteomic Analysis of C2C12 Myoblast and Myotube Exosome-Like Vesicles: A New Paradigm for Myoblast-Myotube Cross Talk? PloS One (2014) 9:e84153. doi: 10.1371/journal.pone.0084153
20. Choi JS, Yoon HI, Lee KS, Choi YC, Yang SH, Kim IS, et al. Exosomes From Differentiating Human Skeletal Muscle Cells Trigger Myogenesis of Stem Cells and Provide Biochemical Cues for Skeletal Muscle Regeneration. J Control Release (2016) 222:107–15. doi: 10.1016/j.jconrel.2015.12.018
21. Aswad H, Jalabert A, Rome S. Depleting Extracellular Vesicles From Fetal Bovine Serum Alters Proliferation and Differentiation of Skeletal Muscle Cells In Vitro. BMC Biotechnol (2016) 16:32. doi: 10.1186/s12896-016-0262-0
22. Madison RD, McGee C, Rawson R, Robinson GA. Extracellular Vesicles From a Muscle Cell Line (C2C12) Enhance Cell Survival and Neurite Outgrowth of a Motor Neuron Cell Line (NSC-34). J Extracell Vesicles (2014) 3:22865. doi: doi: 10.3402/jev.v3.22865.
23. van Niel G, D'Angelo G, Raposo G. Shedding Light on the Cell Biology of Extracellular Vesicles. Nat Rev Mol Cell Biol (2018) 19:213–28. doi: 10.1038/nrm.2017.125
24. Aswad H, Forterre A, Wiklander OP, Vial G, Danty-Berger E, Jalabert A, et al. Exosomes Participate in the Alteration of Muscle Homeostasis During Lipid-Induced Insulin Resistance in Mice. Diabetologia (2014) 57:2155–64. doi: 10.1007/s00125-014-3337-2
25. Jalabert A, Vial G, Guay C, Wiklander OP, Nordin JZ, Aswad H, et al. Exosome-Like Vesicles Released From Lipid-Induced Insulin-Resistant Muscles Modulate Gene Expression and Proliferation of Beta Recipient Cells in Mice. Diabetologia (2016) 59:1049–58. doi: 10.1007/s00125-016-3882-y
26. Small EM, O'Rourke JR, Moresi V, Sutherland LB, McAnally J, Gerard RD, et al. Regulation of PI3-Kinase/Akt Signaling by Muscle-Enriched microRNA-486. Proc Natl Acad Sci USA (2010) 107:4218–23. doi: 10.1073/pnas.1000300107
27. Sawada S, Kon M, Wada S, Ushida T, Suzuki K, Akimoto T. Profiling of Circulating microRNAs After a Bout of Acute Resistance Exercise in Humans. PloS One (2013) 8:e70823. doi: 10.1371/journal.pone.0070823
28. Bye A, Rosjo H, Aspenes ST, Condorelli G, Omland T, Wisloff U. Circulating microRNAs and Aerobic Fitness–the HUNT-Study. PloS One (2013) 8:e57496. doi: 10.1371/journal.pone.0057496
29. Banzet S, Chennaoui M, Girard O, Racinais S, Drogou C, Chalabi H, et al. Changes in Circulating microRNAs Levels With Exercise Modality. J Appl Physiol (1985) (2013) 115:1237–44. doi: 10.1152/japplphysiol.00075.2013
30. Baggish AL, Hale A, Weiner RB, Lewis GD, Systrom D, Wang F, et al. Dynamic Regulation of Circulating microRNA During Acute Exhaustive Exercise and Sustained Aerobic Exercise Training. J Physiol (2011) 589:3983–94. doi: 10.1113/jphysiol.2011.213363
31. Guescini M, Canonico B, Lucertini F, Maggio S, Annibalini G, Barbieri E, et al. Muscle Releases Alpha-Sarcoglycan Positive Extracellular Vesicles Carrying miRNAs in the Bloodstream. PloS One (2015) 10:e0125094. doi: 10.1371/journal.pone.0125094
32. Lovett JAC, Durcan PJ, Myburgh KH. Investigation of Circulating Extracellular Vesicle MicroRNA Following Two Consecutive Bouts of Muscle-Damaging Exercise. Front Physiol (2018) 9:1149. doi: 10.3389/fphys.2018.01149
33. Nair VD, Ge Y, Li S, Pincas H, Jain N, Seenarine N, et al. Sedentary and Trained Older Men Have Distinct Circulating Exosomal microRNA Profiles at Baseline and in Response to Acute Exercise. Front Physiol (2020) 11:605. doi: 10.3389/fphys.2020.00605
34. Roberts TC, Godfrey C, McClorey G, Vader P, Briggs D, Gardiner C, et al. Extracellular microRNAs Are Dynamic Non-Vesicular Biomarkers of Muscle Turnover. Nucleic Acids Res (2013) 41:9500–13. doi: 10.1093/nar/gkt724
35. Cacchiarelli D, Legnini I, Martone J, Cazzella V, D'Amico A, Bertini E, et al. miRNAs as Serum Biomarkers for Duchenne Muscular Dystrophy. EMBO Mol Med (2011) 3:258–65. doi: 10.1002/emmm.201100133
36. Hudson MB, Woodworth-Hobbs ME, Zheng B, Rahnert JA, Blount MA, Gooch JL, et al. miR-23a Is Decreased During Muscle Atrophy by a Mechanism That Includes Calcineurin Signaling and Exosome-Mediated Export. Am J Physiol Cell Physiol (2014) 306:C551–8. doi: 10.1152/ajpcell.00266.2013
37. Karolina DS, Armugam A, Tavintharan S, Wong MT, Lim SC, Sum CF, et al. MicroRNA 144 Impairs Insulin Signaling by Inhibiting the Expression of Insulin Receptor Substrate 1 in Type 2 Diabetes Mellitus. PloS One (2011) 6:e22839. doi: 10.1371/journal.pone.0022839
38. Zhang A, Li M, Wang B, Klein JD, Price SR, Wang XH. miRNA-23a/27a Attenuates Muscle Atrophy and Renal Fibrosis Through Muscle-Kidney Crosstalk. J Cachexia Sarcopenia Muscle (2018) 9:755–70. doi: 10.1002/jcsm.12296
39. Wang B, Zhang A, Wang H, Klein JD, Tan L, Wang ZM, et al. miR-26a Limits Muscle Wasting and Cardiac Fibrosis Through Exosome-Mediated microRNA Transfer in Chronic Kidney Disease. Theranostics (2019) 9:1864–77. doi: 10.7150/thno.29579
40. Donaldson A, Natanek SA, Lewis A, Man WD, Hopkinson NS, Polkey MI, et al. Increased Skeletal Muscle-Specific microRNA in the Blood of Patients With COPD. Thorax (2013) 68:1140–9. doi: 10.1136/thoraxjnl-2012-203129
41. Miyachi M, Tsuchiya K, Yoshida H, Yagyu S, Kikuchi K, Misawa A, et al. Circulating Muscle-Specific microRNA, miR-206, as a Potential Diagnostic Marker for Rhabdomyosarcoma. Biochem Biophys Res Commun (2010) 400:89–93. doi: 10.1016/j.bbrc.2010.08.015
42. Fulzele S, Mendhe B, Khayrullin A, Johnson M, Kaiser H, Liu Y, et al. Muscle-Derived miR-34a Increases With Age in Circulating Extracellular Vesicles and Induces Senescence of Bone Marrow Stem Cells. Aging (Albany NY) (2019) 11:1791–803. doi: 10.18632/aging.101874
43. Yang JC, Lin MW, Rau CS, Jeng SF, Lu TH, Wu YC, et al. Altered Exosomal Protein Expression in the Serum of NF-KappaB Knockout Mice Following Skeletal Muscle Ischemia-Reperfusion Injury. J BioMed Sci (2015) 22:40. doi: 10.1186/s12929-015-0147-x
44. Rigamonti AE, Bollati V, Pergoli L, Iodice S, De Col A, Tamini S, et al. Effects of an Acute Bout of Exercise on Circulating Extracellular Vesicles: Tissue-, Sex-, and BMI-Related Differences. Int J Obes (Lond) (2020) 44:1108–18. doi: 10.1038/s41366-019-0460-7
45. Whitham M, Parker BL, Friedrichsen M, Hingst JR, Hjorth M, Hughes WE, et al. Extracellular Vesicles Provide a Means for Tissue Crosstalk During Exercise. Cell Metab (2018) 27:237–51.e4. doi: 10.1016/j.cmet.2017.12.001
46. Aoi W. Frontier Impact of microRNAs in Skeletal Muscle Research: A Future Perspective. Front Physiol (2015) 5:495. doi: 10.3389/fphys.2014.00495
47. Sjogren RJO, Lindgren Niss MHL, Krook A. Skeletal Muscle microRNAs: Roles in Differentiation, Disease and Exercise. In: Spiegelman B, editor. Hormones, Metabolism and the Benefits of Exercise. Cham, CH: Springer, Cham (2017). p. 67–81.
48. Wang J, Yang LZ, Zhang JS, Gong JX, Wang YH, Zhang CL, et al. Effects of microRNAs on Skeletal Muscle Development. Gene (2018) 668:107–13. doi: 10.1016/j.gene.2018.05.039
49. Almeida FN, Proenca AR, Chimin P, Marcal AC, Bessa-Lima F, Carvalho CR. Physical Exercise and Pancreatic Islets: Acute and Chronic Actions on Insulin Secretion. Islets (2012) 4:296–301. doi: 10.4161/isl.21273
50. Fluckey JD, Kraemer WJ, Farrell PA. Pancreatic Islet Insulin Secretion is Increased After Resistance Exercise in Rats. J Appl Physiol (1985) (1995) 79:1100–5. doi: 10.1152/jappl.1995.79.4.1100
51. Kim S, Lee MJ, Choi JY, Park DH, Kwak HB, Moon S, et al. Roles of Exosome-Like Vesicles Released From Inflammatory C2C12 Myotubes: Regulation of Myocyte Differentiation and Myokine Expression. Cell Physiol Biochem (2018) 48:1829–42. doi: 10.1159/000492505
52. Nie Y, Sato Y, Garner RT, Kargl C, Wang C, Kuang S, et al. Skeletal Muscle-Derived Exosomes Regulate Endothelial Cell Functions via Reactive Oxygen Species-Activated Nuclear factor-kappaB Signalling. Exp Physiol (2019) 104:1262–73. doi: 10.1113/EP087396
53. Barlow JP, Solomon TP. Do Skeletal Muscle-Secreted Factors Influence the Function of Pancreatic Beta-Cells? Am J Physiol Endocrinol Metab (2018) 314:E297–307. doi: 10.1152/ajpendo.00353.2017
Keywords: skeletal muscle, myokine, exosome, metabolism, microRNA
Citation: Aoi W and Tanimura Y (2021) Roles of Skeletal Muscle-Derived Exosomes in Organ Metabolic and Immunological Communication. Front. Endocrinol. 12:697204. doi: 10.3389/fendo.2021.697204
Received: 19 April 2021; Accepted: 10 August 2021;
Published: 14 September 2021.
Edited by:
James M. Olcese, Florida State University, United StatesReviewed by:
Guo-Chang Fan, University of Cincinnati, United StatesKazuyoshi Kuwano, The Jikei University School of Medicine, Japan
Copyright © 2021 Aoi and Tanimura. This is an open-access article distributed under the terms of the Creative Commons Attribution License (CC BY). The use, distribution or reproduction in other forums is permitted, provided the original author(s) and the copyright owner(s) are credited and that the original publication in this journal is cited, in accordance with accepted academic practice. No use, distribution or reproduction is permitted which does not comply with these terms.
*Correspondence: Wataru Aoi, waoi@kpu.ac.jp