- 1CCMAR Centre of Marine Sciences, Universidade do Algarve, Faro, Portugal
- 2OLSPS International, Unipessoal Lda, Lisboa, Portugal
- 3La Rochelle Université, La Rochelle, France
- 4CIIMAR/CIMAR Interdisciplinary Centre of Marine and Environmental Research, Universidade do Porto, Matosinhos, Portugal
Crustacean bottom trawling in southern Portugal is an economic and culturally important fishing activity but may result in considerable bycatch of deep-sea elasmobranchs (DSE). Due to DSE life-history strategies, at-vessel mortality (AVM) rates in crustacean bottom trawl fisheries are expectedly high but require further investigations. This study assessed the at-vessel condition of 18 species of DSE, and AVM rates and stress of four deep-sea shark species (Etmopterus pusillus, E. spinax, Galeus melastomus, and Scymnodon ringens), to understand the impact of bottom trawling on these animals. Opportunistic sampling on a crustacean trawler in the southern Portuguese coast, revealed that 95% of specimens were either dead (n = 1,258) or in poor condition (n = 224) upon collection, underscoring their minimal chance of post-release survival. General linear model analyses showed that AVM was species-specific and highest in smaller sharks, as well as in those from hauls that exhibited larger temperature differences between bottom and surface waters, and those caught in hauls with heavier codend weight using a 55 mm codend mesh (targeting shrimp and prawns) instead of those caught in hauls using a 70 mm codend mesh (targeting Norway lobster). Stress, evaluated through metabolites and electrolytes levels in sharks' plasma, indicated significant differences in potassium, urea, and magnesium levels between live and deceased specimens of E. pusillus and G. melastomus, suggesting these as reliable mortality markers. Elevated lactate levels in G. melastomus further pointed to high post-release mortality risk. These findings highlight an urgent need to find solutions to mitigate the impacts of bottom trawling on those DSE, which are thoroughly discussed. A coordinated, multi-stakeholder approach involving researchers, the fishing industry, and regulatory bodies is crucial for developing and implementing effective, and more sustainable fisheries management and protection of DSE populations.
1 Introduction
Bottom trawling is an important fishing practice in Europe. In Portugal, trawl fleet landings rank third in national seafood landings (1), with crustacean trawlers specifically targeting commercially valuable species such as Nephrops norvegicus (Linnaeus, 1758), Aristeus antennatus (Risso, 1816), and Aristaeomorpha foliacea (Risso, 1827) (2). This fleet primarily operates at depths of 200–800 m in the South and Southwest regions off Portugal [e.g., (3, 4)]. The proximity of southern Portuguese ports, like Olhão and Portimão, to Spain facilitates trade with Spanish buyers, which further enhances the economic importance of crustacean trawling in this area (3).
Trawl fisheries bycatch, particularly in deeper waters, includes deep-sea elasmobranchs (DSE; i.e., sharks and skates), which can constitute up to ca. 60% of the total catch in weight (5–9, 133). Deep-sea elasmobranchs, are classified as meso- and top predators [e.g., (10–13)], and predominantly inhabit slopes at depths over 400 m (14, 15), though it is generally conceived that deep-sea species are defined as those dwelling at depths >200 m. They play a crucial role in maintaining ecosystem balance and thus, their population decline could impact other species' populations, potentially leading to structural shifts in these ecosystems (16–19).
According to the International Union for Conservation of Nature (IUCN) Red List of Threatened Species, one in seven (14%) DSE are threatened with an elevated risk of extinction globally, and 43 of the 283 (15%) deep-sea shark species are classified as threatened with a high risk of extinction (20). This vulnerability primarily stems from their biological characteristics, including slow growth, late maturity, and low reproductive rates (21, 22). The European Union (EU) has implemented legislation aimed at reducing the capture of these species in European waters. The EU Regulation 2023/194 acknowledges that even minimal fishing activity poses a serious conservation risk for several shark species. As a result, a ban was established that prohibits EU fishing vessels, as well as vessels from non-EU countries operating in EU waters, from fishing, retaining on board, transshipping, or landing certain prohibited shark species. Since 2010, a zero Total Allowable Catch (TAC) for deep-sea sharks has been enforced within the EU. In compliance with this regulation, any prohibited shark species caught must be promptly released unharmed, preventing their fins, meat, and liver oil from entering the market. However, despite these measures, the survival of released animals is not guaranteed.
For most deep-sea sharks, data on at-vessel mortality (AVM) rates are extremely rare. The few available estimates show considerable variability, ranging from 0 to 100% in longline fisheries (23–25) and from 85 to 91% in bottom trawl fisheries (26). Information on post-release mortality (PRM) is even scarcer, mainly with estimates from longline fisheries, presenting great variation among the studies [14–83%; (23–25), (27)]. These rates indicate that mortality is likely both fishery- and species-specific, and that is generally positively correlated with depth and inversely correlated with body size (24, 26). When captured during fishing activities, deep-sea sharks are often exposed to various environmental stressors, including sudden changes in water temperature, pressure, and oxygen levels (28), which frequently result in mortality. For instance, a mortality study conducted in the Mediterranean found that fishing on the slope increases deep-sea sharks' mortality rates compared to fishing for coastal species at shallower depths (26). Additionally, the AVM of demersal shark species is affected by factors such as fishing effort, as well as the composition and weight of the catch (29). Post-release mortality, on the other hand, is influenced by factors like the amount of time specimens spend on deck before being returned to the sea (30, 31).
The evaluation of the at-vessel condition of a specimen using vitality scores (e.g., excellent, good, poor dead conditions) is a subjective but simple, quick, and cost-effective method, providing important estimates of AVM, though not reporting information on PRM which is generally assessed through tag-telemetry studies (32, 33). However, some holding and tagging studies suggest that PRM could be somewhat predicted [e.g., (34–36)]. Specimens scored in poor condition are likely to die after being discarded due to several underlying mechanisms, including physiological stress that may further impact behavior, and reproduction, and ultimately lead to PRM (37, 38). Hence, the assessment of physiological stress parameters could inform about the fate of discarded animals.
Animal stress is generally defined as a disruption of an organism's homeostasis caused by internal or external stimuli, which triggers compensatory behavioral or physiological responses (39). These responses often entail large metabolic costs, diverting energy away from growth and reproduction and toward respiration, movement, and tissue repair (39), a shift which may potentially lead to mortality. Understanding the impacts on animal stress responses is essential for developing measures aimed at reducing mortality among specimens captured in fisheries and for informing species-specific management and conservation strategies (40–42).
Stressful events such as those occurring during fishing activities, prompt a cascade of physiological responses, often due to exertion as animals struggle to escape the stressor [e.g., (43)]. This triggers a first response through the rapid release of stress hormones, such as catecholamines and corticosteroids, along with a rise in blood sugar levels, which depletes the animal's energy reserves while it also leads to lactic acid accumulation in muscles and plasma (44, 45). Such responses disrupt the balance of ions, water, and other essential substances in blood (39). Thus, secondary stress responses in animals can be measured through plasma biomarkers, such as pH, pCO2, lactate, glucose, hematocrit, and osmolality (46). These indicators help assess an animal's condition following exposure to stressors, like those encountered during capture (32, 47–49). While the level of stress responses may be largely species-specific [e.g., (38, 50–52)], factors such as fishing gear type and duration, environmental conditions and the shark's respiratory mode [either buccal pumping or ram ventilation; (52–55)] as well as the individual condition, will also determine an animal's response to stress and the variations in the above mentioned indicators.
To determine if an animal is experiencing stress or if a particular activity induces stress, baseline (or “stress-free”) values of secondary stress responses are essential. However, acquiring these baseline values is challenging, as sample collection itself can trigger stress responses in specimens, and this is further exacerbated in fisheries. Consequently, researchers often rely on data obtained from studies with animals in captivity (52, 56, 57) and/or estimated using mathematical models which relies on baseline values (58). Laboratory studies aim to minimize capture time (51) and often include behavioral analyses (59–62). However, these methods are insufficient for studying free-ranging sharks, particularly deep-sea species, as they cannot be kept in captivity or sampled quickly enough to obtain true baseline values (46). The lack of established baseline parameters for sharks indicates that most of the stress studies lack proper control groups, often limiting findings to descriptive analyses that report plasma ion concentrations without definitive conclusions on whether animals were genuinely stressed. Utilizing data from deceased animals may provide valuable control for free-ranging sharks, as suggested by Wosnick et al. (63) and discussed in prior studies (64–66). Evaluating metabolites and electrolytes levels in the plasma of deceased animals can help determine when animals approach critical conditions, potentially improving release protocols. This alternative approach would shift the point of view from “the closer to baseline, the less stressed” to “the closer to death-reference levels, the more stressed and vulnerable.”
This study aimed to evaluate the impact of bottom trawling on the at-vessel condition of DSE species caught during crustacean bottom trawling operations off the southern coasts of Portugal. To achieve this, DSE arriving onboard a crustacean bottom trawler were classified as dead, or in poor, good, or excellent condition. The influence of environmental factors and fishing practices on AVM of deep-sea sharks was analyzed using logistic regression models. Deep-sea sharks were further subjected to analyses of secondary stress responses concentrations assessed through metabolites (glucose, lactate, urea) and electrolytes (calcium, sodium, phosphorus, potassium, chloride, magnesium). Metabolites and electrolytes levels were compared between deceased and alive specimens to test the potential of using values from deceased animals as reference points (63). Our hypotheses were that: (1) DSE would primarily arrive onboard dead or in poor condition (26); (2) AVM in deep-sea sharks would be influenced by factors such as depth, body size (24, 26), water temperature (23–25), species (26, 67, 68), and fishing effort [e.g., (67, 69)]; and (3) deep-sea sharks would exhibit elevated stress levels (70).
2 Materials and methods
2.1 Ethics statement
This study was conducted following the Guidelines of the European Union Council (2010/63/UE) and Portuguese legislation “The protection of Animals Used for Scientific Purposes” (DL 113/2013). All the procedures were approved by CCMAR Animal Welfare Committee (ORBEA CCMAR—Organization Responsible for Animal Welfare of CCMAR) and the Direção-Geral de Alimentação e Veterinária (DGAV) of the Portuguese Government. All animal protocols were performed under Group-C licenses from the DGAV, Ministério da Agricultura, do Desenvolvimento Rural e das Pescas, Portugal.
2.2 Field campaigns
This study was conducted on a commercial crustacean bottom trawler in the South (37°-36°N; 9°-7.5°W) and Southwest (37.9°-36.7° N; 7.7°-9.6° W) coasts of Portugal (Figure 1). Ten fishing trips with a total of 77 hauls were performed from June 2020 to May 2022, summing up to 351 h of fishing effort. The target species were Norway lobster (Nephrops norvegicus), shrimps and prawns [Aristeus antennatus, Aristaeomorpha foliacea, Aristaeopsis edwardsiana (Johnson, 1868), Parapenaeus longirostris (Lucas, 1846), and Penaeus monodon, Fabricius, 1798] using net codend (i.e., the terminal section of a trawling net) mesh sizes of 70 mm (mean depth of 483 m) and 55 mm (mean depth of 636 m) respectively.
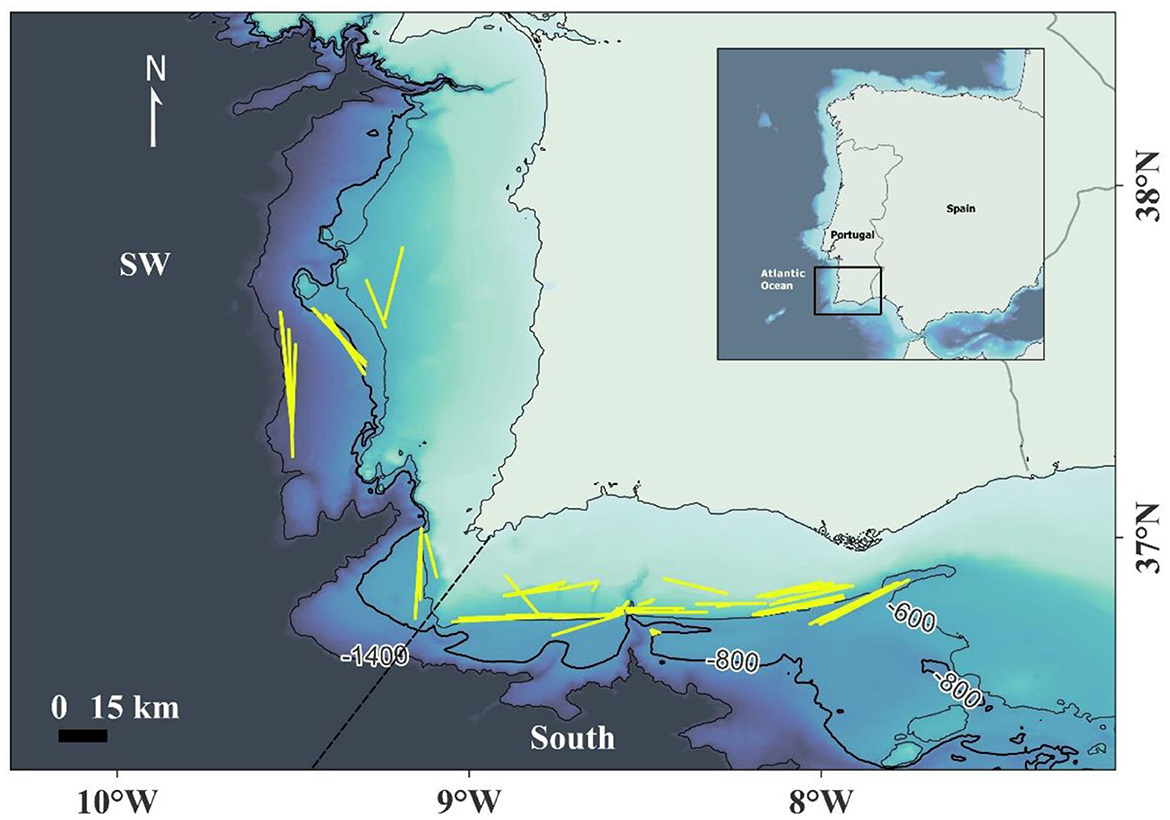
Figure 1. Study area in Portugal's South and Southwest (SW) coasts. The yellow lines represent the sites where hauls were conducted.
Fishing hauls lasted 2.3–9 h, with a velocity of 1.5–3.7 nm/h, and were conducted across all seasons of the year. Fishing effort was computed in hours from the moment the net reached the bottom of the ocean up to the moment it began to be hauled back to the vessel. Capture and handling time was computed in hours since the start of a haul until the start of the sorting of the catch by fishers. The weight of the net codend (kg) was a visual estimation given by the skipper when the net was lifted from the water at the end of every haul, or when the catch was already inside the “pond” (an area below deck where the codend is offloaded). Water temperature (°C) at the bottom/fishing depth was either collected by a Scanmar® or by a mini DST-CTD logic® Star-Oddi® attached to the net (temperature was recorded at every 5 min for hauls < 1,000 m and every 10 min for hauls >1,000 m). Surface water temperature was collected by the DST-CTD whenever a reading was performed near the surface (< 1 m), otherwise, data from the Copernicus website on the sea surface temperature for the sampled regions, dates and hour of each haul (to ensure the precision of the estimate), was used instead. Technical (i.e., codend mesh size, codend weight, and fishing effort), environmental (i.e., water temperature, and fishing depth), and biological data (i.e., sharks' total length and maturity stage) were registered into the Electronic Logbook (eLog) Olrac Dynamic Data logger (Olrac DDL®) where information per specimen was inserted. Capture and handling duration (h) was assessed from the moment each haul started up to the moment the sorting of the catch started.
2.3 At-vessel condition
The DSE caught during the fishing events, were sorted by species. Each specimen was identified following Ebert et al. (71) and Last et al. (72) and measured [total length (TL) to the nearest 0.5 cm: from the tip of snout to the tip of the caudal fin]. DSE's at-vessel condition was assessed following the criteria of Benoît et al. (73) and Catchpole et al. (74). Both methods include a rapid observation of possible injuries and reflex impairments (i.e., reduction or loss of responses to stimuli) to classify specimens into a small number of ordinal vitality categories (Table 1). After this evaluation, the alive specimens were kept inside acclimatized holding tanks until the moment they were discarded, i.e., after the end of all the analyses with the DSE from the same haul, which took up to 1 h in some instances.
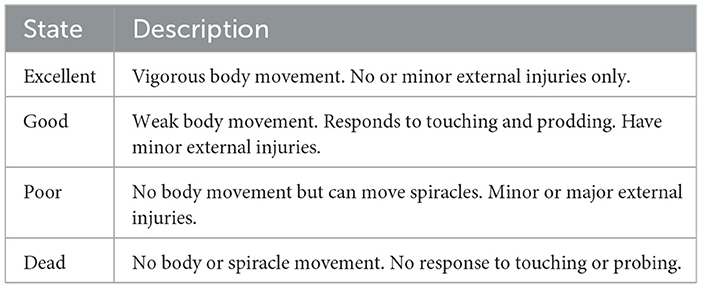
Table 1. At-vessel condition of deep-sea elasmobranchs' specimens, assessed through vitality categories.
2.3.1 At-vessel mortality
The most frequently caught deep-sea shark species (hereafter referred to as “sharks”)—comprising three species from the order Squaliformes [Etmopterus pusillus (Lowe, 1839), E. spinax (Linnaeus, 1758), and Scymnodon ringens Barbosa du Bocage and de Brito Capello, 1864] and one species from the order Carcharhiniformes (Galeus melastomus Rafinesque, 1810; Table 2)—were selected for modeling the influences of variables on their AVM rates. At-vessel mortality rates were based on the assigned vitality category of each specimen (Table 1). Specimens categorized as dead were from the vitality category “dead,” while those categorized as alive were from the vitality categories “poor,” “good,” or “excellent” (Table 1).
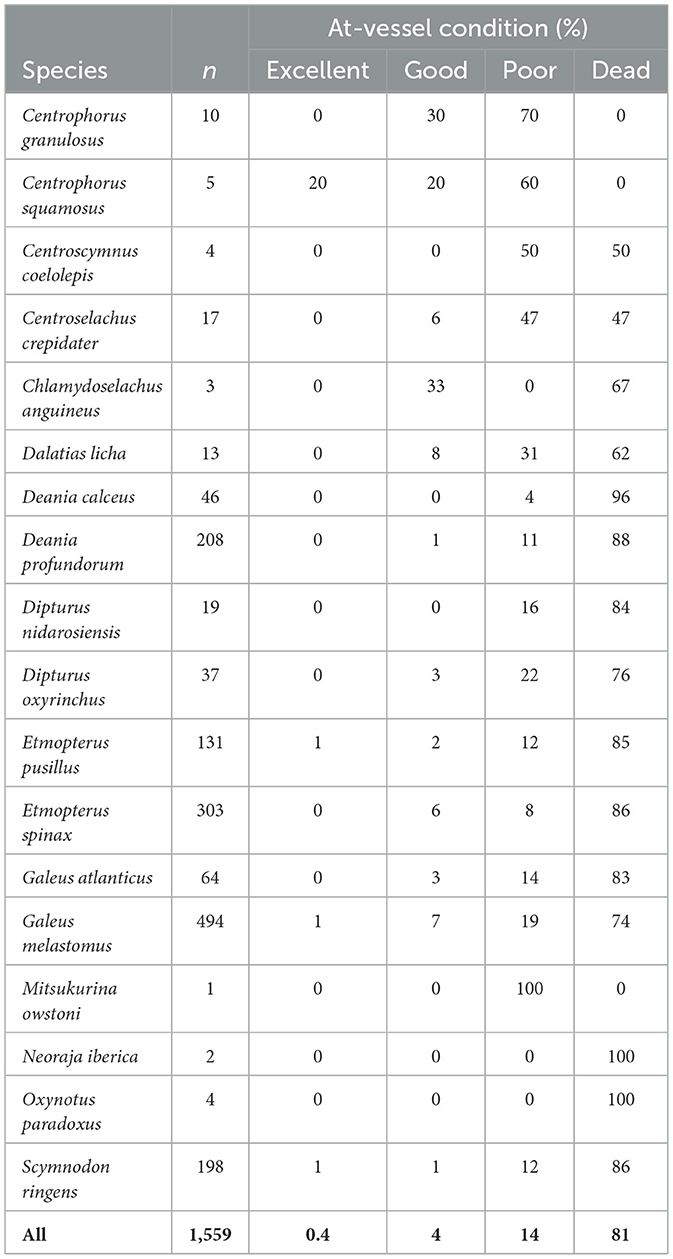
Table 2. Percentages (%) of the at-vessel condition of deep-sea elasmobranchs caught by a crustacean trawler at the southern region of Portugal.
2.4 Capture and handling stress
Blood sampling was conducted over four field trips between May and August 2021 and February and April 2022 along the South coast of Portugal. Hauls were randomly selected for sampling, with five alive and five dead sharks from frequently caught species (Etmopterus spp., G. melastomus, and S. ringens) randomly chosen from each selected haul. Blood samples were collected as quickly as possible from dead sharks via the caudal peduncle using a 1 ml heparinized syringe, followed by sampling from alive sharks. Dead sharks were prioritized over live ones, as blood flow decreases over time post-mortem, making collection increasingly difficult. Because each fishing trip takes ca. three full days, plasma was obtained by centrifugation of blood samples on board within 2 h post-collection, stored at −20°C and kept frozen until laboratory analysis. The alive sharks were released after blood collection. At the laboratory, the concentrations of metabolites (i.e., glucose, lactate and urea) and electrolytes (i.e., phosphorus, chloride, magnesium, and calcium) were measured by colorimetric assays using Spinreact® kits (Girona, Spain) with a Multi-Mode Microplate Reader BioTek Synergy™ 4 (BioTek® Instruments, Winooski, VT, USA). Sodium and potassium concentrations were determined using a flame photometer (BWB-XP PerformancePlus, BWB Technologies, UK).
2.5 Statistical analysis
2.5.1 At-vessel mortality
A binomial Generalized Linear Model (GLM) was used to evaluate the influence of several predictors on the likelihood of shark mortality (dead or alive). To ensure predictors were suitable for the GLM, an initial Logit model was run using the package lessR (75), which allowed for identifying potential collinearity among predictors. The initial predictor variables included codend weight, codend mesh size, total length, fishing velocity, fishing effort, fishing depth, species, and temperature differences between surface and bottom waters (hereafter simply “temperature differences”). If in this first step, a collinearity is identified by the Tolerance coefficient—as was the case with the variable “depth” found to exhibit high collinearity (Tolerance = 0.17)—than this variable is excluded from further GLM analyses.
The glmulti package (76) was used to identify the best predictor variables (excluding “depth”) by fitting a series of binomial GLMs with AVM as the binary response variable. The logit link function ensured response probabilities remained within the 0–1 range, appropriate for binary outcomes. Model selection relied on the Akaike Information Criterion (AIC), where a lower AIC (lower than 2 units) indicates a better model balance between fit and complexity. Only the main effects of each variable were considered, as the data were collected randomly and did not provide sufficient differences between some predictor combinations. This approach helped prevent potential overfitting issues from interaction terms that lacked adequate contrast. The glmulti package identified the importance of terms to be used in the final GLM, using a diagnostic plot to highlight predictors with an importance higher than 50%, hence suggesting exclusion of predictors that fell below that threshold. Finally, a GLM was conducted with the selected predictors, followed by a check of the model's suitability, which was once more conducted by the Logit model in the lessR package. The following diagnostics were conducted with the Logit: (1) the absence of multivariate outliers, (2) linear relationships between continuous predictors and the logit-transformed response, and (3) no collinearity among predictors.
2.5.2 Capture and handling stress
To test the usefulness of using dead animals' values as reference values, stress responses concentrations (i.e., metabolites and electrolytes concentrations) in plasma were compared between dead and alive specimens of E. pusillus and G. melastomus using the t-Test or the equivalent non-parametric Mann-Whitney test. The latter was applied whenever the assumptions of normality and homoscedasticity of each evaluated response were not met using the Shapiro-Wilk test and the Bartlet, test respectively (at a p < 0.05). Due to the low number of specimens for the species E. spinax and S. ringens (< 5 observations per dead or alive specimens) only mean (SD) and median (IQR) values on the plasma related stress responses concentrations were reported.
All statistical analyses were done with the open-source R environment (77).
3 Results
3.1 At-vessel condition
A total of 1,559 specimens belonging to 18 deep-sea species of sharks (15 species) and skates (3 species) were evaluated. Collectively they were mostly dead (n = 1,258) or in a poor condition (n = 224), with very low numbers of specimens in good condition (n = 70) and even lower in excellent conditions (7). All species presented a much higher number of deceased specimens (mean = 84) or specimens in poor condition (mean =15), than in excellent (mean = 2) and/or good conditions (mean = 6; Table 2). Species that presented the worst condition were Oxynotus paradoxus and Neoraja iberica with all specimens dead (however with low number of evaluated specimens), followed by Deania calceus and D. profundorum (Table 2). Species that presented comparatively better at-vessel condition (i.e., higher rates in good and excellent conditions) were the Centrophorus spp. with no deceased specimen. Mitsukurina owstoni also did not present immediate AVM but only one specimen was sampled (Table 2).
3.1.1 At-vessel mortality
Of a total of 1,126 specimens, the majority belonged to G. melastomus, followed by E. spinax, S. ringens, and E. pusillus (Table 2). At-vessel mortality presented a much greater proportion of dead (81%) than alive (19%) specimens (Table 3), where the species Galeus melastomus presented the lowest proportion of dead specimens in relation to alive specimens in comparison with the other sharks (Table 2).
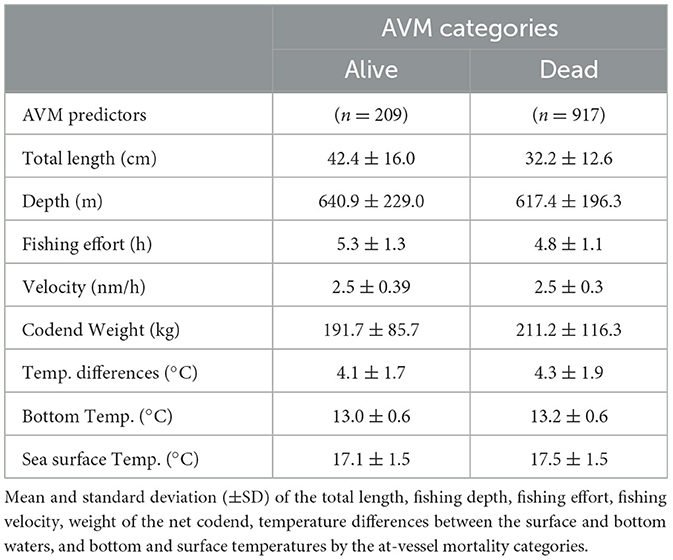
Table 3. Total number of deep-sea sharks' specimens sampled by at-vessel mortality (AVM) categories (Alive and Dead).
Dead specimens were more numerous in hauls using a codend mesh size of 55 mm (846; 75%) than 70 mm (71; 6%). Additionally, codend weight and temperatures (surface, bottom and differences among the two) showed higher mean values in deceased specimens compared to alive ones, while the sharks' total length, fishing effort, and depth presented greater values in alive specimens (Table 3).
The best GLM model included predictors such as total length (TL), codend mesh size and weight, species, fishing effort, and temperature differences (Table 4), achieving an overall prediction accuracy of 83.5%, with 97.4% of mortality predicted and 22.5% of survivorship predicted. The results indicate that several predictors significantly affect the AVM categories in different ways (Table 4). Species differences played a strong role: Etmopterus spinax and Galeus melastomus have significantly lower probabilities of mortality compared to the species (S. ringens), while E. pusillus shows significantly higher probabilities of mortality relative to S. ringens (Table 4). Additionally, a 70 mm codend mesh size corresponds to a significantly lower mortality rate compared to 55 mm mesh. Larger TL and increased fishing effort also correlate with lower mortality rates (Table 4). In contrast, increasing codend weight and temperature differences are associated with higher mortality likelihood (Table 4).
3.2 Capture and handling stress
Stress analyses were conducted at 65 specimens of four deep-sea sharks' species. Alive E. pusillus and G. melastomus were larger, generally caught at greater depths, and presented greater duration of capture and handling procedures when compared with dead specimens (Table 5). For E. spinax the same was observed but that comparison was made considering only one dead specimen. Alive S. ringens presented larger size and were mainly caught at shallower depths. In this species, however, the capture and handling times between dead and alive specimens were similar (Table 5).
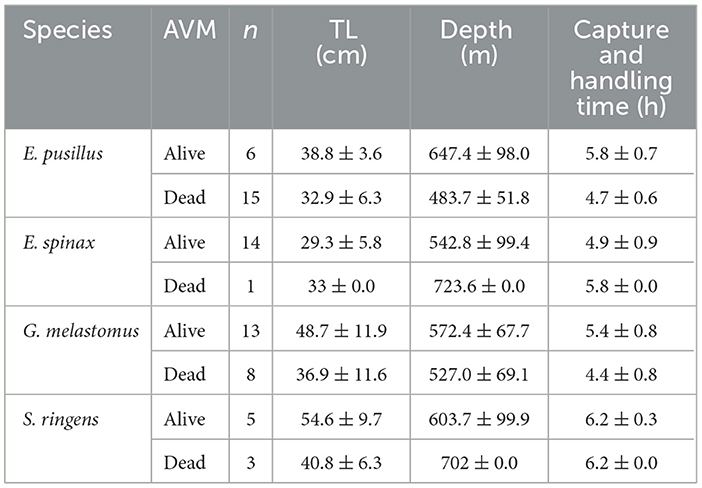
Table 5. Mean, minimum and maximum values of total length (TL, cm), depth of fishing (m) and capture and handling time (h) for sharks' species (Etmopterus pusillus; E. spinax; Galeus melastomus and Scymnodon ringens) and at-vessel mortality (AVM) categories (alive and dead) with the respective number of specimens.
Plasma analyses revealed varying concentrations of metabolites and electrolytes for the different species (Table S1). Glucose and urea presented significantly higher concentrations for alive specimens of the species E. pusillus and G. melastomus and higher concentrations for alive S. ringens in comparison with dead specimens. However, urea presented higher values for deceased S. ringens in relation to alive specimens (Table S2). Lactate concentrations were not significantly different but were also higher for alive specimens of G. melastomus and S. ringens (Figure 2). For E. pusillus higher concentrations were found for deceased specimens in comparison with alive ones (Figure 2). Alive specimens of E. spinax presented the highest maximum values of all metabolites in relation to the dead specimen (Figure 2).
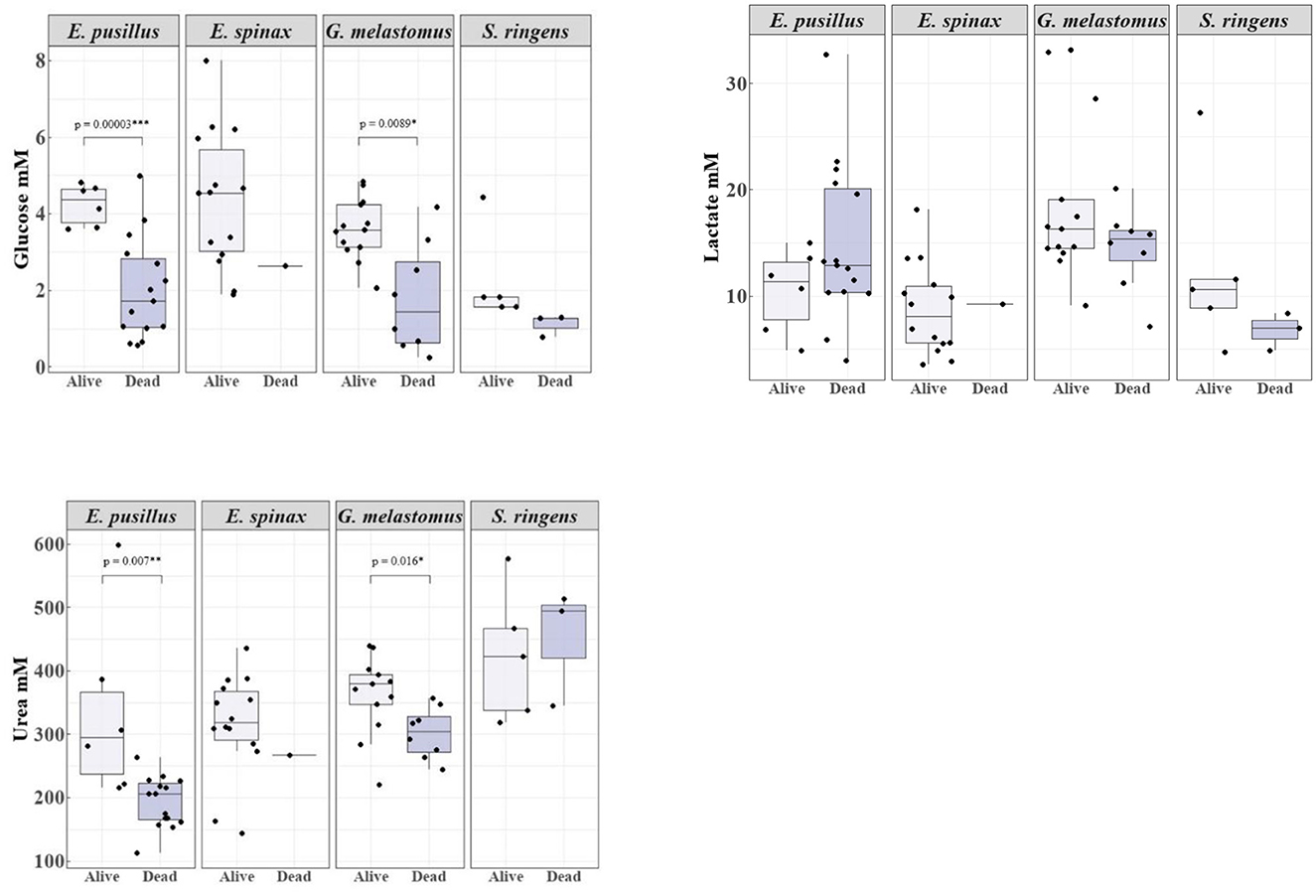
Figure 2. Boxplot representation of the concentrations of the plasma metabolites (glucose, lactate and urea) in mM for alive and deceased specimens of the sharks Etmopterus pusillus, E. spinax, Galeus melastomus and Scymnodon ringens. The boxplots show the median (horizontal lines) with 50% (boxes) and 95% intervals (vertical lines). Significant differences in glucose and urea concentrations between deceased and alive specimens of E. pusillus and G. melastomus are presented through the p-value of the t-Test (glucose) and Mann-Whitney test (urea).
In relation to the electrolytes, significant differences were found for potassium and magnesium (Figure 3; Table S2). Potassium concentrations were significantly higher for dead specimens of E. pusillus and G. melastomus (Figure 3; Table S2) and higher for dead specimens of S. ringens (Figure 3). Magnesium concentrations were significantly higher for G. melastomus deceased specimens but higher for E. pusillus and S. ringens alive specimens (Figure 3; Table S2). Calcium was higher for alive specimens of E. pusillus and S. ringens but for G. melastomus it was higher for dead specimens (Figure 3). Chloride concentrations were higher for alive specimens of E. pusillus and G. melastomus whereas S. ringens deceased specimens presented higher concentrations (Figure 3). Sodium concentrations were higher in deceased G. melastomus and S. ringens but higher for alive E. pusillus (Figure 3). Phosphorus concentrations were higher in deceased E. pusillus and G. melastomus and a bit higher in alive S. ringens. The only dead specimen of E. spinax presented the highest value of sodium (449.7 mM), in comparison with alive specimens of the same species and all the other specimens of the other species (Table S2; Figure 3). Chloride, potassium and sodium were all greater for this dead E. spinax specimen when compared to the alive specimens, whilst calcium, and magnesium were greater for alive specimens in comparison with the dead specimen (Figure 3).
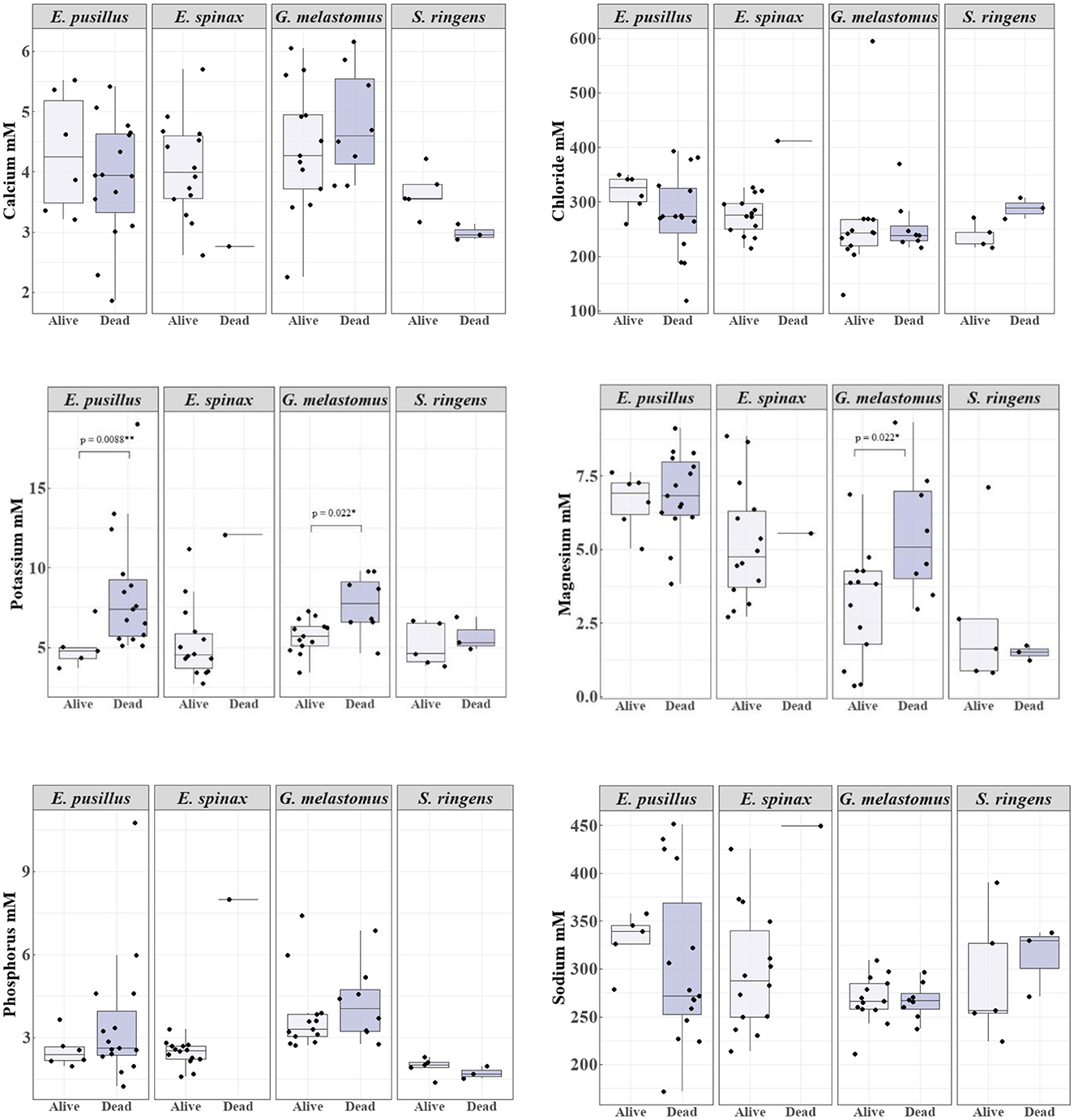
Figure 3. Boxplot representation of the concentrations of the plasma electrolytes (calcium, chloride, magnesium, phosphorus, potassium, and sodium) in mM for alive and deceased specimens of the sharks Etmopterus pusillus, E. spinax, Galeus melastomus and Scymnodon ringens. The boxplots show the median (horizontal lines) with 50% (boxes) and 95% intervals (vertical lines). Significant differences in potassium concentrations between deceased and alive specimens of E. pusillus and G. melastomus are presented through the p-value of the Mann-Whitney. For magnesium, significant differences between deceased and alive specimens of G. melastomus are presented by the p-value of the t-Test.
4 Discussion
4.1 At-vessel condition
This study presents data on the at-vessel condition of DSE caught in bottom trawling operations, where most specimens were either dead upon retrieval (80.7%; n = 1,258) or in poor condition (14.4%; n = 224). Only a few were in excellent (0.4%; n = 7) or good condition (4.5%; n = 70). Specimens classified as in poor condition are unlikely to survive when released back into the sea [e.g., (23, 25, 32, 52, 78)]. Consequently, the PRM (including dead specimens or in poor condition) of the studied DSE species is expected to be extremely high, averaging 95% across all of the 18 DSE species sampled. This includes exceptionally high estimated PRM for the most numerous species like G. melastomus (93%), E. spinax (94%), E. pusillus (97%), S. ringens (98%) and D. profundorum (99%). Additionally, PRM is thought to be critically high for species considered endangered in Europe by the International Union for Conservation of Nature [IUCN; (78)], including Centroscymnus coelolepis and Deania calceus (100%), as well as Dalatias licha (93%). However, some species of conservation concern showed a higher proportion of specimens in relatively better condition compared to other DSE species. These include the critically endangered (78) Centrophorus granulosus (30% in good condition), the endangered Centrophorus squamosus (20% in excellent and 20% in good condition), and the least-concern but rare Chlamydoselachus anguineus (33% in good condition).
Most at-vessel condition studies on sharks and skates focus on demersal species caught by longline and gillnets presenting varying mortality estimates (0–100%) but generally lower than for bottom trawling (25, 53, 79, 80). Studies specifically on bottom trawling are scarcer (81) and mainly involve resilient demersal species like Scyliorhinus canicula, which showed relatively low mortality rates between 2 and 53% (30, 31, 57, 82). Only recently has research assessed the condition of a few DSE species in bottom trawling, as in a study of 12 demersal elasmobranch species in the Asinara Gulf, where specimens were classified into “active,” “inactive,” or “dead” conditions (26). Scacco et al. (26) reported high rates of inactive or dead specimens for Dipturus oxyrinchus (85%), E. spinax (88%), and G. melastomus (91%), aligning with our findings. Though our estimates of dead or poor-condition specimens were still slightly higher (98, 94, and 93%, respectively).
Using several technical, environmental and biological predictors, the GLM achieved a high prediction of 83.5% of sharks' AVM (dead and alive), which was specifically linked to several factors which included specimen size (TL), codend weight and mesh size, temperature differences, species, and fishing effort. Mortality rates were inversely related to shark size, with smaller specimens more likely to die in bottom trawling procedures than larger ones. Similarly, Ellis et al. (83) reported higher mortality in skates under 50 cm in the North Sea trawling fishery, and Talwar et al. (25) observed higher mortality in smaller Squalus cubensis in the Exuma Sound longline fishery. This trend is also consistent with findings for other elasmobranchs (24, 26) and bony fishes (84–86). Larger specimens generally exhibit greater swimming endurance and are less prone to injury. In contrast, smaller specimens are more vulnerable to physical damage and higher mortality rates when caught in trawling nets, primarily due to the increased volume and composition of the catch in the codend. Higher catch volumes intensify contact and abrasion among organisms, raising the risk of injury for smaller species (29). This agrees with the present study findings where heavier codend presented significantly higher probability of mortality (although with a small effect), than lighter codend. However, caution is needed when interpreting these results, as codend weight was estimated visually by the skipper, introducing the potential for under- or overestimation of the weight. Future studies could use a codend weigher, a tool that measures the codend's weight as it is brought on board (87). This would enhance the accuracy of codend weight measurements and contribute to a better understanding of how codend weight affects mortality rates in sharks.
Another important technical predictor on the sharks' mortality was codend mesh size, where hauls using smaller mesh sizes (55 mm) targeting prawns and shrimp had significantly higher mortality rates than those with larger mesh sizes (70 mm) targeting Norway lobster. Smaller mesh sizes increase retention, causing the net to clog more quickly and capturing a wider range of specimen sizes (88, 89). This congestion may cause mortality by compression and asphyxia. This would suggest that adjusting mesh sizes based on target species and fishing depth could help reduce mortality in non-target deep-sea shark populations. However, the relationship between codend mesh size and mortality is so far only supported in studies with bony fish. Studies with bony fish suggest that larger mesh sizes reduce injuries, and scale loss by allowing more fish to escape (90–92), although some research found no significant impact of mesh size on escapee survival (84, 85). In this study, depth—a key predictor of mortality—was excluded from the GLM due to collinearity with other factors, as smaller mesh sizes (55 mm) were predominantly used at greater average depths (623 m ± 286 m), while larger meshes (70 mm) were used at shallower depths (473 m ± 58 m). This does not rule out the potential impact of mesh size on shark mortality, but further research using varied mesh sizes at similar depths and conditions could provide clearer insights into its effects specifically on mortality rates in deep-sea sharks.
With larger temperature differences (between bottom and surface waters) there was an increase in mortality in the sharks analyzed for this study. Similar results were reported in deep-sea sharks caught by longliners in the Cantabrian Sea [NE Atlantic; (24)] and in Bahamian waters where the sea surface temperature reached 30°C (23, 25). Deep-sea species inhabit cold waters, and when brought to warmer surface temperature may increase the stress and mortality (33, 70, 93–95). Most sharks are ectothermic which means that they regulate their body temperature with the surrounding water and have a relatively narrow range of temperature in which they can thrive and reproduce (96). Water temperature was already suggested as a proxy to determine time/area closures when it reaches a certain threshold (94). This approach may become even more important as global water temperature rises due to climate change but will require an understanding of species-specific reactions to local conditions.
Fishing effort is generally positively related to mortality, as prolonged procedures elevate mortality rates (67, 69, 94). In static gear like longlines and gillnets, longer soak times increase mortality, particularly for species reliant on ram ventilation for oxygenation (81, 97). However, in this study, higher fishing effort was related with a slightly increased odds of finding alive specimens than dead ones. Unlike static gear, trawling does not allow for pinpointing the exact moment of capture, so fishing effort in this study is likely overestimated, measured from the start of each haul rather than the exact entry time of each specimen in the net. Hauls in this study ranged from 2:54 to 8:36 h, meaning that specimens were exposed to at least 2:54 h of fishing activity. It is possible that the alive specimens from longer hauls were captured closer to the end of the haul, increasing their chance of survival. This could suggest that shorter hauls, potentially < 2:54 h, may reduce shark mortality. However, longline studies have shown significant mortality in deep-sea sharks even with soak times under 3 h (24, 25). Additional controlled studies could help identify if there is an optimal haul duration that would minimize shark mortality.
Shark species exhibited markedly different mortality likelihoods, aligning with findings in other studies (26, 67, 68). Deep-sea squaliform sharks tend to have particularly vulnerable and conservative life histories which includes lower fecundity and smaller litter size, making them less resilient to the pressures exerted by fisheries (21, 22, 98). Indeed, the squaliform sharks (Etmopterus spp. and S. ringens) showed the highest mortality rates, contrasting with the carcharhiniform shark (G. melastomus). These differences may be partly attributed to the presence of an anal fin in G. melastomus and its absence in squaliform species. The anal fin is thought to enhance swimming stability (99), which could provide G. melastomus with improved swimming abilities, potentially reducing its mortality in trawling situations. However, this adaptation does not prevent its capture by trawlers. Further research is required to confirm this hypothesis.
4.2 Capture and handling stress
In general, specimens appeared stressed by the conditions and procedures conducted during fishing activities, as indicated by plasma concentrations of secondary stress response indicators (e.g., metabolites and electrolytes). These concentrations were different when compared to baseline values for elasmobranchs from previous studies (100, 101) and stress indicators for demersal sharks caught by bottom trawlers [e.g., (102, 103)]. In this study, significant differences in glucose, urea, magnesium, and potassium concentrations were observed between deceased and alive specimens of E. pusillus and G. melastomus.
Potassium and urea levels could act as mortality markers for E. pusillus and G. melastomus, with deceased specimens showing significantly higher potassium and lower urea levels. For G. melastomus, significantly higher magnesium levels were also observed in deceased individuals. Although no statistical differences were noted for S. ringens and E. spinax due to low sample numbers, deceased specimens exhibited higher potassium levels. Post-mortem potassium and urea concentrations have previously been linked to mortality in Galeocerdo cuvier and Prionace glauca, alongside other markers like lactate, phosphorus, and calcium (63, 64). High potassium levels may lead to hyperkalemia, disrupting muscle cell membrane excitability, causing myocardial dysfunction in S. acanthias and muscle tetany in Mustelus antarcticus (47, 100, 104). Plasma potassium above 7 mM in this study consistently indicated poor at-vessel condition or death, supporting previous findings (105), and suggesting cell disruption leakage. Similarly, low urea can signal osmotic imbalance, possibly increasing ion exchange across osmoregulatory tissues—a stress response linked to capture in S. acanthias (50, 106).
Stressed fish generally exhibit significant increases in glucose levels (46, 51, 52), which is attributed to the mobilization of glucose for energy production [e.g., (107, 108)]. However, in the present study, glucose was lower for dead specimens of S. ringens and significantly lower for dead specimens of E. pusillus and G. melastomus in comparison with alive ones. Talwar et al. (25) also found that, lower blood glucose levels, resulted in a higher likelihood of mortality of deep-sea sharks in the Bahamas. Cliff and Thurman (47) observed that moribund or dead C. obscurus specimens had lower glucose levels compared to those that were alive. This might imply that, an inability to further mobilize glycogen stores and glucose depletion may contribute to metabolic failure and ultimately, death.
Lactate has been proposed as a mortality marker (51, 63, 64), given that increased lactate levels indicate a strong physiological response, often due to anaerobic respiration triggered by high stress or intense activity in low-oxygen conditions (46, 107, 108). In this study, lactate levels did not significantly differ between deceased and alive specimens of E. pusillus and G. melastomus, though across all four species studied, lactate levels were high when compared to the unstressed 5 mM level reported for species like Carcharhinus obscurus, C. plumbeus, and S. acanthias (47, 109, 110). Pelagic sharks with lactate levels above 16 mM often show high mortality (46). Notably, in this study, maximum lactate values for G. melastomus (33.12 mM) and S. ringens (27.27 mM) were among the highest recorded for elasmobranchs, comparable to species like Alopias vulpinus [27 mM; (111)] and Carcharhinus brachyurus [42 mM; (53)]. Elevated lactate levels in G. melastomus and some other alive specimens from this study suggest potential PRM, warranting further investigation into species-specific lactate responses (51).
Comparing interspecific responses, S. ringens displayed distinct concentrations of phosphorus, magnesium, and urea. This could indicate either a unique response to fishing procedures on the crustacean bottom trawler or naturally different baseline levels of these metabolites and electrolytes. Prohaska et al. (70) found species-specific and ecological differences in stress responses among deep-sea sharks, with deeper-dwelling species showing distinct reactions compared to those at shallower depths. Scymnodon ringens is a larger species and was generally found at greater depths than other studied species, particularly among live specimens. This size difference may relate to reduced fight intensity, potentially helping larger sharks better manage stress (25).
The observed differences in plasma secondary stress responses between alive and deceased and the high dispersion within a single species suggest individual variability in coping mechanisms (112) and that stress responses may be triggered at an individual level (63). Variability within parameters and species also likely reflect the differences each animal experiences while caught which are impossible to estimate in this study—i.e., actual time in codend, level of contact with the gear and other organisms, place within the organism's mass in the codend and opportunity to struggle or even access to oxygenated water. Studies on elasmobranchs indicate that plasma metabolites and electrolytes reach peak levels over different timeframes: lactate (~2 h), urea (~6 h), and sodium [~5 h; (47, 56, 100–102, 113)]. This highlights the importance of further species-specific studies where animals are exposed to various stressors in different fisheries, with blood sampling over extended time intervals to fully characterize stress responses following events like capture or air exposure (114). However, samples collected immediately after capture may not fully reflect physiological impact. Conducting controlled experiments with deep-sea elasmobranchs poses challenges due to the need to replicate their natural environment (low temperature, high pressure, darkness) and minimize captivity-related stress. Thus, endpoint concentrations of metabolites are often assessed in deceased specimens (63–66). In this study, only potassium, magnesium, and urea showed potential as markers for mortality in deceased E. pusillus and G. melastomus, suggesting that further studies should include additional species, sample sizes, and varied fishing conditions.
4.3 Recommendations for decreasing impacts of bottom trawling on deep-sea elasmobranchs
In the European Union, DSE are protected by regulations such as Regulation 2024/257, which prohibits the landing of several shark species, effectively setting a zero TAC to prevent targeted fishing. However, despite this protection, DSE continues to be caught as bycatch in bottom trawling operations and are subsequently discarded, often dead or dying, and under stress, as observed in this study. This is particularly concerning, as discarded bycatch is rarely recorded in fisheries logbooks, limiting our understanding of DSE's population dynamics and distribution. The ongoing mortality of DSE, combined with the absence of accurate catch and discard records, underscores a significant yet unrecorded impact on these vulnerable populations. This raises concerns about the long-term sustainability of DSE species in EU waters, as the true extent of their decline remains largely unknown. Given the unknown limits to which these species can withstand fishing pressures, a precautionary approach is necessary by avoiding the capture of DSE in the first place.
Studies on gear modifications provide a valuable foundation for mitigating bycatch. Turtle excluder devices and the Nordmøre grid have proven effective in reducing bycatch and are widely applied in elasmobranch studies [e.g., (115–119)]. These are angled panels with spaced bars, that allows smaller target crustaceans through while excluding larger, unwanted species such as sea turtles and elasmobranchs without impacting greatly the target catch rates (115–119). A modified version of the Nordmøre grid has been applied in Portuguese crustacean trawl fisheries, where it effectively reduced bony fish bycatch while maintaining the commercial catch of crustaceans (120). However, while effective for larger elasmobranchs, these devices still allow smaller DSE to pass through, meaning smaller-bodied DSE remain at risk. Therefore, further actions and gear modifications are encouraged to address the bycatch of smaller-bodied DSE species.
Adjusting the codend mesh size and weight in bottom trawling presents a viable approach to reducing bycatch and mortality of sharks. In Portugal, research on bottom trawler's codend mesh configuration suggests that increasing mesh size and switching from diamond to square mesh significantly enhances selectivity, allowing smaller specimens to escape (121–125). Diamond mesh tends to close under tension, trapping smaller species, while square mesh stays open, facilitating the escape of non-target species without reducing the catch of commercially valuable fish (121, 122, 132). Codend weight also plays a significant role in bycatch mortality as previously discussed. Heavier codend increase mortality not only directly, through the physical injuries caused by increased contact and abrasion among organisms (29), but also indirectly by prolonging sorting time onboard, thereby raising the risk of air exposure. Although air exposure was not measured in this study, the benefits of reducing fishing duration suggest that adjusting effort duration and codend weight could further reduce bycatch mortality. Shortening fishing hauls, when possible, could reduce both the codend's weight and handling time, minimizing air exposure and stress for non-target species.
Closures based on specific areas, seasons, and/or depths have proven effective in reducing bycatch, as they help to protect critical elasmobranchs' aggregations (126). For instance, a depth-based ban on bottom trawling below 800 m was implemented in the Northeast Atlantic in 2017 (Regulation 2016/2336) to protect deep-sea species, including DSE. Yet, for further spatial-temporal closures to be optimized, especially in Portuguese waters, a comprehensive understanding of DSE pupping and breeding grounds, would facilitate the establishment of targeted closures, maximizing conservation benefits for DSE populations. This can be achieved using a spatial distribution modeling approach (127, 128). While DSE distribution patterns have been modeled for the Azores (129), mainland Portugal still lacks crucial data on DSE distribution and habitat use. Integrating electronic monitoring programs, alongside onboard observers, can also support data collection on DSE bycatch, enhancing data on abundance and distributions, ensuring compliance and enabling adaptive management.
Implement onboard handling DSE protocols may aid in the mitigation of their mortality rates. Studies on elasmobranch PRM demonstrate that careful handling increases survival likelihood, even for species prone to stress-related mortality (126, 130, 131). Despite the high AVM rates in this study, improved handling techniques could be beneficial to minimize physical trauma, especially for conservation concern species like C. coelolepis, Centrophorus squamosus, C. granulosus, and D. calceus. Training fishers in these techniques, informed by the high mortality rates observed for DSE in bottom trawling, would be an actionable step toward reducing bycatch impacts.
5 Conclusion
The vast majority (95%) of the DSE caught in trawling operations conducted for this study were either dead upon retrieval or are unlikely to survive after being discarded. At-vessel mortality rates of deep-sea sharks were species-specific and influenced by some factors, including the small size of specimens, large temperature differences between bottom and surface waters, and the use of 55 mm codend mesh (targeting prawns and shrimps) instead of 70 mm (targeting Norway lobster), and heavier weight in the net codend. Metabolites and electrolytes levels suggest that the studied sharks are stressed, with potassium, urea, and magnesium identified as potential mortality indicators for certain species, while high lactate levels in G. melastomus pointed to elevated PRM. Species-specific and individual stress responses, as indicated by varying metabolite and electrolyte levels, suggest that further research is needed to clarify the stress pathways in different species and improve bycatch survival rates. To address these issues, immediate actions should focus on a precautionary approach, preventing DSE bycatch where possible. Complementary measures should focus on mitigating the impacts of bottom trawling activities on these vulnerable species. Translating research findings into actionable conservation strategies requires a coordinated, multi-stakeholder effort involving researchers, the fishing industry, and regulatory bodies. This collaboration is essential for developing effective management strategies that safeguard DSE populations while promoting sustainable practices in crustacean bottom trawl fisheries.
Data availability statement
The raw data supporting the conclusions of this article will be made available by the authors, without undue reservation.
Ethics statement
The animal study was approved by Organization Responsible for Animal Welfare of CCMAR. The study was conducted in accordance with the local legislation and institutional requirements.
Author contributions
SG: Conceptualization, Data curation, Formal analysis, Funding acquisition, Investigation, Methodology, Project administration, Resources, Validation, Visualization, Writing – original draft, Writing – review & editing. ATe: Conceptualization, Funding acquisition, Methodology, Project administration, Resources, Supervision, Writing – review & editing. TMa: Investigation, Visualization, Writing – review & editing. PP: Investigation, Writing – review & editing. TMo: Conceptualization, Funding acquisition, Methodology, Resources, Writing – review & editing. PG: Conceptualization, Methodology, Resources, Writing – review & editing. ATa: Investigation, Writing – review & editing. AA: Conceptualization, Methodology, Resources, Writing – review & editing. PR: Conceptualization, Funding acquisition, Investigation, Methodology, Validation, Writing – review & editing. ED: Conceptualization, Funding acquisition, Investigation, Methodology, Project administration, Resources, Supervision, Writing – review & editing.
Funding
The author(s) declare financial support was received for the research and/or publication of this article. This research was mainly supported by the EEA Grants (PT-Innovation-0007) and also by Save our Seas Foundation (SOSF 501), and National Funds through FCT projects—Foundation for Science and Technology within the scope of UIDB/04423/2020, UIDP/04423/2020, UIDB/04326/2020, UIDP/04326/2020, and LA/P/0101/2020. SG (https://doi.org/10.54499/SFRH/BD/147493/2019) and ED (DL57/2016/CP1344/CT0021) were supported by FCT.
Acknowledgments
We would first like to thank the vessel owner, onboard crew and skipper for allowing us to join their daily activities and collect this important data. To CCMAR colleagues, especially from the Fisheries Biodiversity and Conservation group, that helped and allowed us to use their facilities to treat the data and Elsa Anjinho do Couto from the Laboratory of Comparative Endocrinology and Integrative Biology that helped with the plasma analysis. To Bianca Rangel, Raquel Lubambo and Luís Reis for the gathering of literature on plasma secondary indicators of elasmobranchs. The company OLSPS Marine manager, technicians, and developers for developing and providing the software Olrac® iEMR to collect and store onboard data and for their ongoing support throughout this process. Universidade do Algarve also acknowledges the project Sustainable Horizons in Higher Education Institutions (SHEs) 101071300 funded by the European Commission.
Conflict of interest
TM was employed by the company OLSPS International.
The remaining authors declare that the research was conducted in the absence of any commercial or financial relationships that could be construed as a potential conflict of interest.
Publisher's note
All claims expressed in this article are solely those of the authors and do not necessarily represent those of their affiliated organizations, or those of the publisher, the editors and the reviewers. Any product that may be evaluated in this article, or claim that may be made by its manufacturer, is not guaranteed or endorsed by the publisher.
Supplementary material
The Supplementary Material for this article can be found online at: https://www.frontiersin.org/articles/10.3389/frish.2025.1473376/full#supplementary-material
References
1. Instituto Nacional de Estatística (INE). Estatísticas da pesca. (2023). Available online at: https://www.ine.pt (accessed December, 2024).
2. Campos A, Fonseca P, Fonseca T, Parente J. Definition of fleet components in the Portuguese bottom trawl fishery. Fish Res. (2007) 83:185–91. doi: 10.1016/j.fishres.2006.09.012
3. Bueno-Pardo J, Ramalho SP, García-Alegre A, Morgado M, Vieira RP, Cunha MR, et al. Deep-sea crustacean trawling fisheries in Portugal: quantification of effort and assessment of landings per unit effort using a vessel monitoring system (VMS). Sci Rep. (2017) 7:40795. doi: 10.1038/srep40795
4. Campos A, Henriques V, Erzini K, Castro M. Deep-sea trawling off the Portuguese continental coast: spatial patterns, target species, and impact of a prospective EU-level ban. Marine Policy. (2021) 128:104466. doi: 10.1016/j.marpol.2021.104466
5. Borges TC, Erzini K, Bentes L, Costa ME, Gonçalves JMS, Lino PG, et al. By-catch and discarding practices in five Algarve (southern Portugal) métiers. J Appl Ichthyol. (2001) 17:104–14. doi: 10.1046/j.1439-0426.2001.00283.x
6. Monteiro P, Araújo A, Erzini K, Castro M. Discards of the algarve (southern Portugal) crustacean trawl fishery. Hidrobiologia. (2001) 449:267–77. doi: 10.1023/A:1017575429808
7. Carbonell A, Alemany F, Merella P, Quetglas A, Roman E. The bycatch of sharks in the western Mediterranean (Balearic Islands) fishery. Fish Res. (2003) 61:7–18. doi: 10.1016/S0165-7836(02)00242-4
8. Coelho R, Erzini K, Bentes L, Correia C, Lino PG, Monteiro P, et al. Semi-pelagic longline and trammel net elasmobranch catches in southern Portugal: catch composition, catch rates, and discards. J Northwest Atl Fish Sci. (2005) 35:531–7. doi: 10.2960/J.v35.m482
9. Costa ME, Erzini K, Borges TC. Bycatch of crustacean and fish bottom trawl fisheries from southern Portugal (Algarve). Sci Mar. (2008) 72:801–14. doi: 10.3989/scimar.2008.72n4801
10. Graça Aranha S, Teodósio A, Baptista V, Erzini K, Dias E. A glimpse into the trophic ecology of deep-water sharks in an important crustacean fishing ground. J Fish Biol. (2023) 102:655–68. doi: 10.1111/jfb.15306
11. Cortés E. Standardized diet compositions and trophic levels of sharks. ICES J Mar Sci. (1999) 56:707–17. doi: 10.1006/jmsc.1999.0489
12. Bizzarro JJ, Robinson HJ, Rinewalt CS, Ebert DA. Comparative feeding ecology of four sympatric skate species off central California, USA. Environ Biol Fishes. (2007) 80:197–220. doi: 10.1007/s10641-007-9241-6
13. Churchill DA, Heithaus MR, Vaudo JJ, Grubbs RD, Gastrich K, Castro JI. Trophic interactions of common elasmobranchs in deep-sea communities of the Gulf of Mexico revealed through stable isotope and stomach content analysis. Deep Sea Res Part II Topical Stud Oceanogr. (2015) 115:92–102. doi: 10.1016/j.dsr2.2014.10.011
14. ICES. NEAFC and OSPAR joint request on the status and distribution of deep-water elasmobranchs (ICES Advice 2020, sr.2020.09). Copenhagen, Denmark: ICES Advisory Committee (2020).
15. O'Hea B, Davie S, Johnston G, O'Dowd L. Assemblages of deepwater shark species along the northeast Atlantic continental slope. Deep Sea Res Part I Oceanogr Res Pap. (2020) 157:103207. doi: 10.1016/j.dsr.2019.103207
16. Ruppert JLW, Travers MJ, Smith LL, Fortin MJ, Meekan MG. Caught in the middle: combined impacts of shark removal and coral loss on the fish communities of coral reefs. PLoS ONE. (2013) 8:e74648. doi: 10.1371/journal.pone.0074648
17. Barley S, Meekan M, Meeuwig J. Species diversity, abundance, biomass, size, and trophic structure of fish on coral reefs in relation to shark abundance. Mar Ecol Prog Ser. (2017) 565:163–79. doi: 10.3354/meps11981
18. Valls M, Rueda L, Quetglas A. Feeding strategies and resource partitioning among elasmobranchs and cephalopods in Mediterranean deep-sea ecosystems. Deep Sea Res Part I Oceanogr Res Pap. (2017) 129:49–62. doi: 10.1016/j.dsr.2017.09.002
19. Shipley ON, Matich P, Hussey NE, Brooks AML, Chapman D, Frisk MG, et al. Energetic connectivity of diverse elasmobranch populations: implications for ecological resilience. Proc Biol Sci. (2023) 290:20230262. doi: 10.1098/rspb.2023.0262
20. Finucci B, Pacoureau N, Rigby CL, Matsushiba JH, Faure-Beaulieu N, Sherman CS, et al. Fishing for oil and meat drives irreversible defaunation of deepwater sharks and rays. Science. (2024) 383:1135–41. doi: 10.1126/science.ade9121
21. García VB, Lucifora LO, Myers RA. The importance of habitat and life history to extinction risk in sharks, skates, rays, and chimaeras. Proc R Soc B Biol Sci. (2008) 275:83–9. doi: 10.1098/rspb.2007.1295
22. Simpfendorfer CA, Kyne PM. Limited potential to recover from overfishing raises concerns for deep-sea sharks, rays, and chimaeras. Environ Conserv. (2009) 36:97–103. doi: 10.1017/S0376892909990191
23. Brooks EJ, Brooks AML, Williams S, Jordan LKB, Abercrombie D, Chapman DD, et al. First description of deep-water elasmobranch assemblages in the Exuma Sound, The Bahamas. Deep Sea Res Part II. (2015) 115:81–91. doi: 10.1016/j.dsr2.2015.01.015
24. Rodríguez-Cabello C, Sánchez F. Catch and post-release mortalities of deep-water sharks caught by bottom longlines in the Cantabrian Sea (NE Atlantic). J Sea Res. (2017) 130:248–55. doi: 10.1016/j.seares.2017.04.004
25. Talwar B, Brooks E, Mandelman J, Grubbs R. Stress, post-release mortality, and recovery of commonly discarded deep-sea sharks caught on longlines. Mar Ecol Prog Ser. (2017) 582:147–61. doi: 10.3354/meps12334
26. Scacco U, Fortibuoni T, Baini M, Franceschini G, Giani D, Concato M, et al. Gradients of variation in the at-vessel mortality rate between twelve species of sharks and skates sampled through a fishery-independent trawl survey in the Asinara Gulf (NW Mediterranean Sea). Biology. (2023) 12:363. doi: 10.3390/biology12030363
27. Daley RK, Williams A, Green M, Barker B, Brodie P. Can marine reserves conserve vulnerable sharks in the deep sea? A case study of Centrophorus zeehaani (Centrophoridae), examined with acoustic telemetry. Deep Sea Res Part II Topical Stud Oceanogr. (2015) 115:127–36. doi: 10.1016/j.dsr2.2014.05.017
28. Fauconnet L, Catarino D, Das D, Giacomello E, Gonzalez-Irusta JM, Afonso P, et al. Challenges in avoiding deep-water shark bycatch in Azorean hook-and-line fisheries. ICES J Mar Sci. (2023) 80:605–19. doi: 10.1093/icesjms/fsac178
29. Broadhurst MK, Suuronen P, Hulme A. Estimating collateral mortality from towed fishing gear. Fish Fisheries. (2006) 7:180–218. doi: 10.1111/j.1467-2979.2006.00213.x
30. Revill AS, Dulvy NK, Holst R. The survival of discarded lesser-spotted dogfish (Scyliorhinus canicula) in the Western English channel beam trawl fishery. Fish Res. (2005) 71:121–4. doi: 10.1016/j.fishres.2004.07.006
31. Rodríguez-Cabello C, Fernández A, Olaso I, Sánchez F. Survival of small-spotted catshark (Scyliorhinus canicula) discarded by trawlers in the Cantabrian Sea. J Mar Biol Assoc U K. (2005) 85:1145–50. doi: 10.1017/S002531540501221X
32. Skomal GB. Evaluating the physiological and physical consequences of capture on post-release survivorship in large pelagic fishes. Fish Manag Ecol. (2007) 14:81–9. doi: 10.1111/j.1365-2400.2007.00528.x
33. Braccini M, Van Rijn J, Frick L. High post-capture survival for sharks, rays, and chimaeras discarded in the main shark fishery of Australia? PLoS ONE. (2012) 7:e32547. doi: 10.1371/journal.pone.0032547
34. Van Beek FA, Van Leeuwen PI, Rijnsdorp AD. On the survival of plaice and sole discards in the otter-trawl and beam-trawl fisheries in the North Sea. Netherlands J Sea Res. (1990) 26:151–60. doi: 10.1016/0077-7579(90)90064-N
35. Hueter RE, Manire CA. Bycatch and Catch-Release Mortality of Small Sharks in the Gulf Coast Nursery Grounds of Tampa Bay and Charlotte Harbor (Report No. 368). Mote Marine Laboratory Technical Report, Sarasota, FL (1994). Available online at: https://aquadocs.org/mapping/15269/1/368.pdf
36. Richards LJ, Fargo J, Schnute JT. Factors influencing bycatch mortality of trawl-caught Pacific halibut. North Am J Fish Manag. (1995) 15:266–76. doi: 10.1577/1548-8675(1995)015<0266:FIBMOT>2.3.CO;2
37. Wedemeyer GA, Barton BA, McLeay DJ. “Stress and acclimation.” In:C. B. Schreck and P. B. Moyle, , editors. Methods for Fish Biology. American Fisheries Society (1990). p. 451–89. Available online at: https://pubs.usgs.gov/publication/70180779
38. Gallagher AJ, Orbesen ES, Hammerschlag N, Serafy JE. Vulnerability of oceanic sharks as pelagic longline bycatch. Glob Ecol Conserv. (2014) 1:50–9. doi: 10.1016/j.gecco.2014.06.003
39. Wendelaar Bonga SE. The stress response in fish. Physiol Rev. (1997) 77:591–625. doi: 10.1152/physrev.1997.77.3.591
40. Ferguson RA, Tufts BL. Physiological effects of brief air exposure in exhaustively exercised rainbow trout (Oncorhynchus mykiss): implications for ‘catch and release' fisheries. Can J Fish Aquat Sci. (1992) 49:1157–62. doi: 10.1139/f92-129
41. Wikelski M, Cooke SJ. Conservation physiology. Trends Ecol Evol. (2006) 21:38–46. doi: 10.1016/j.tree.2005.10.018
42. Young JL, Bornik ZB, Marcotte ML, Charlie KN, Wagner GN, Hinch SG, et al. Integrating physiology and life history to improve fisheries management and conservation. Fish Fisheries. (2006) 7:262–83. doi: 10.1111/j.1467-2979.2006.00225.x
43. Barton BA. Stress in fishes: a diversity of responses with particular reference to changes in circulating corticosteroids. Integr Comp Biol. (2002) 42:517–25. doi: 10.1093/icb/42.3.517
44. Mazeaud MM, Mazeaud F, Donaldson EM. Primary and secondary effects of stress in fish: Some new data with a general review. Trans Am Fish Soc. (1977) 106:201–12.
45. Randall DJ, Ferry SF. Catecholamines. Cardiovasc Syst. (1992) 255–300. doi: 10.1016/S1546-5098(08)60011-4
46. Skomal G, Bernal D. “Physiological responses to stress in sharks.” In: Sharks and Their Relatives II. Boca Raton, FL: CRC Press (2010). p. 459–90. doi: 10.1201/9781420080483-c11
47. Cliff G, Thurman GD. Pathological and physiological effects of stress during capture and transport in the juvenile dusky shark (Carcharhinus obscurus). Comp Biochem Physiol A. (1984) 78:167–73. doi: 10.1016/0300-9629(84)90111-7
48. Wells RMG, McIntyre RH, Morgan AK, Davie PS. Physiological stress responses in big gamefish after capture: observations on plasma chemistry and blood factors. Comp Biochem Physiol A. (1986) 84:565–71. doi: 10.1016/0300-9629(86)90366-X
49. Harrenstien LA, Tornquist SJ, Miller-Morgan TJ, Fodness BG, Clifford KE. Evaluation of a point-of-care blood analyzer and determination of reference ranges for blood parameters in rockfish. J Am Vet Med Assoc. (2005) 226:255–65. doi: 10.2460/javma.2005.226.255
50. Mandelman JW, Skomal GB. Differential sensitivity to capture stress assessed by blood acid–base status in five carcharhinid sharks. J Comp Physiol B. (2009) 179:267–77. doi: 10.1007/s00360-008-0306-4
51. Marshall H, Field L, Afiadata A, Sepulveda C, Skomal G, Bernal D. Hematological indicators of stress in longline-captured sharks. Comp Biochem Physiol A Mol Integr Physiol. (2012) 162:121–9. doi: 10.1016/j.cbpa.2012.02.008
52. Skomal GB, Mandelman JW. The physiological response to anthropogenic stressors in marine elasmobranch fishes: a review with a focus on the secondary response. Comp Biochem Physiol A Mol Integr Physiol. (2012) 162:146–55. doi: 10.1016/j.cbpa.2011.10.002
53. Dapp DR, Huveneers C, Walker TI, Drew M, Reina RD. Moving from measuring to predicting bycatch mortality: predicting the capture condition of a longline-caught pelagic shark. Front Mar Sci. (2016) 2:126. doi: 10.3389/fmars.2015.00126
54. Guida L, Walker TI, Reina RD. Temperature insensitivity and behavioural reduction of the physiological stress response to longline capture by the gummy shark, Mustelus antarcticus. PLoS ONE. (2016) 11:e0148829. doi: 10.1371/journal.pone.0148829
55. Mohan JA, Jones ER, Hendon JM, Falterman B, Boswell KM, Hoffmayer ER, Wells RJD. Capture stress and post-release mortality of blacktip sharks in recreational charter fisheries of the Gulf of Mexico. Conserv Physiol. (2020) 8:coaa041. doi: 10.1093/conphys/coaa041
56. Frick LH, Walker TI, Reina RD. Immediate and delayed effects of gill-net capture on acid–base balance and intramuscular lactate concentration of gummy sharks, Mustelus antarcticus. Comp Biochem Physiol A Mol Integr Physiol. (2012) 162:88–93. doi: 10.1016/j.cbpa.2011.02.023
57. Barragán-Méndez C, Ruiz-Jarabo I, Fuentes J, Mancera JM, Sobrino I. Survival rates and physiological recovery responses in the lesser-spotted catshark (Scyliorhinus canicula) after bottom-trawling. Comp Biochem Physiol A Mol Integr Physiol. (2019) 233:1–9. doi: 10.1016/j.cbpa.2019.03.016
58. Skomal GB. The Physiological Effects of Capture Stress on Post-Release Survivorship of Sharks, Tunas, and Marlin (Doctoral dissertation, Boston University). ProQuest Dissertations and Theses Global (2006).
59. Manire C, Hueter R, Hull E, Spieler R. Serological changes associated with gill-net capture and restraint in three species of sharks. Trans Am Fish Soc. (2001) 130:1038–48. doi: 10.1577/1548-8659(2001)130 < 1038:SCAWGN>2.0.CO;2
60. Skomal G, Lobel PS, Marshall G. The use of animal-borne imaging to assess post-release behavior as it relates to capture stress in grey reef sharks, Carcharhinus amblyrhynchos. Mar Technol Soc J. (2007) 41:44–8. doi: 10.4031/002533207787441999
61. Hyatt MW, Anderson PA, O'Donnell PM. Behavioral release condition score of bull and bonnethead sharks as a coarse indicator of stress. J Coast Res. (2016) 32:1464. doi: 10.2112/JCOASTRES-D-15-00108.1
62. Whitney NM, Lear KO, Gaskins LC, Gleiss AC. The effects of temperature and swimming speed on the metabolic rate of the nurse shark (Ginglymostoma cirratum, Bonaterre). J Exp Mar Bio Ecol. (2016) 477:40–6. doi: 10.1016/j.jembe.2015.12.009
63. Wosnick N, Bornatowski H, Ferraz C, Afonso A, Sousa Rangel B, Hazin FHV, et al. Talking to the dead: using post-mortem data in the assessment of stress in tiger sharks (Galeocerdo cuvier) (Péron and Lesueur, 1822). Fish Physiol Biochem. (2017) 43:165–78. doi: 10.1007/s10695-016-0276-5
64. Moyes CD, Fragoso N, Musyl MK, Brill RW. Predicting postrelease survival in large pelagic fish. Trans Am Fish Soc. (2006) 135:1389–97. doi: 10.1577/T05-224.1
65. Hight BV, Holts D, Graham JB, Kennedy BP, Taylor V, Sepulveda CA, et al. Plasma catecholamine levels as indicators of the post-release survivorship of juvenile pelagic sharks caught on experimental drift longlines in the Southern California Bight. Mar Freshw Res. (2007) 58:145. doi: 10.1071/MF05260
66. Hutchinson M, Itano D, Muir J, Holland K. Post-release survival of juvenile silky sharks captured in a tropical tuna purse seine fishery. Mar Ecol Prog Ser. (2015) 521:143–54. doi: 10.3354/meps11073
67. Morgan A, Carlson JK. Capture time, size, and hooking mortality of bottom longline-caught sharks. Fish Res. (2010) 101:32–7. doi: 10.1016/j.fishres.2009.09.004
68. Braccini JM, Waltrick D. Species-specific at-vessel mortality of sharks and rays captured by demersal longlines. Marine Policy. (2019) 99:94–8. doi: 10.1016/j.marpol.2018.10.033
69. Díaz GA, Serafy JE. Longline-caught blue shark (Prionace glauca): factors affecting the numbers available for live release. Fish Bull. (2005) 103:720–4.
70. Prohaska BK, Talwar BS, Grubbs RD. Blood biochemical status of deep-sea sharks following longline capture in the Gulf of Mexico. Conserv Physiol. (2021) 9:coaa113. doi: 10.1093/conphys/coaa113
72. Last PR, White WT, de Carvalho MR, Seret B, Stehmann MFW, Naylor GJP. Rays of the World. Melbourne, VIC: CSIRO Publishing (2016). doi: 10.1071/9780643109148
73. Benoît HP, Hurlbut T, Chass, é J. Assessing the factors influencing discard mortality of demersal fishes using a semi-quantitative indicator of survival potential. Fish Res. (2010) 106:436–47. doi: 10.1016/j.fishres.2010.09.018
74. Catchpole T, Wright S, Bendall V, Hetherington S, Randall P, Ross E, et al. Ray Discard Survival: Enhancing Evidence of the Discard Survival of Ray Species. Report Cefas (2017).
75. Gerbing DW. Enhancement of the command-line environment for use in the introductory statistics course and beyond. J Stat Data Sci Educ. (2021) 29:251–6. doi: 10.1080/26939169.2021.1999871
76. Calcagno V, de Mazancourt C. Glmulti: an R package for easy automated model selection with (generalized) linear models. J Stat Softw. (2010) 34:1–29. doi: 10.18637/jss.v034.i12
77. R Development Core Team. R: A Language for Environment and Statistical Computing. (2024). Available online at: http://www.r-project.org/
78. Nieto A, Ralph GM, Comeros-Raynal MT, Kemp J, Criado MG, Allen DJ, et al. European Red List of Marine Fishes. Luxembourg: European Commission (2015).
79. Revill A. Survival of Discarded Fish. A Rapid Review of Studies on Discard Survival Rates. Brussels: European Commission, Directorate General for Maritime Affairs and Fisheries (2012).
80. Ellis JR, McCully Phillips SR, Poisson F. A review of capture and post-release mortality of elasmobranchs. J Fish Biol. (2016) 90:653–722. doi: 10.1111/jfb.13197
81. Dapp DR, Walker TI, Huveneers C, Reina RD. Respiratory mode and gear type are important determinants of elasmobranch immediate and post-release mortality. Fish Fisheries. (2015) 17:507–24. doi: 10.1111/faf.12124
82. Kaiser M, Spencer B. Survival of by-catch from a beam trawl. Mar Ecol Prog Ser. (1995) 126:31–8. doi: 10.3354/meps126031
83. Ellis JR, Clarke MW, Cortés E, Heessen HJL, Apostolaki P, Carlson JK, Kulka DW. Management of elasmobranch fisheries in the North Atlantic. In: Advances in Fisheries Science. Oxford: Blackwell (2008). p. 184–228. doi: 10.1002/9781444302653.ch9
84. Suuronen P, Erickson D, Orrensalo A. Mortality of herring escaping from pelagic trawl codends. Fish Res. (1996) 25:305–21. doi: 10.1016/0165-7836(95)00446-7
85. Wileman DA, Sangster GI, Breen M, Ulmestrand M, Soldal AV, Harris RR. Roundfish and Nephrops Survival After Escape from Commercial Fishing Gear. Final Report. EC Contract FAIR-CT95-0753. Brussels, European Commission (1999).
86. Ingolfsson O, Soldal AV, Huse I. Mortality and injuries of haddock, cod, and saithe escaping through codend meshes and sorting grids. ICES. (2002) 32:22.
87. Caslake R. Codend Weigher Report (SR616). Seafish Research and Development, UK (2009). Available online at: https://www.seafish.org/media/Publications/SR616_CodendWeigherFinal.pdf
88. Murawski SA. Factors influencing bycatch and discard rates: analyses from multispecies/multifishery sea sampling. J Northwest Atl Fish Sci. (1996) 19:31–9. doi: 10.2960/J.v19.a3
89. Broadhurst MK. Modifications to reduce bycatch in prawn trawls: a review and framework for development. Rev Fish Biol Fish. (2000) 10:27–60. doi: 10.1023/A:1008936820089
91. Sangster GI, Lehmann KM, Breen M. Commercial fishing experiments to assess the survival of haddock and whiting after escape from four sizes of diamond mesh codends. Fish Res. (1996) 25:323–46. doi: 10.1016/0165-7836(95)00430-0
92. Lowry N, Sangster GI, Breen M. Codend Selectivity and Fish Mortality. Final Report of the European Commission Study Contract No. 1994/005. Brussels, European Commission (1996).
93. Davis MW. Key principles for understanding fish bycatch discard mortality. Can J Fish Aquat Sci. (2002) 59:1834–43. doi: 10.1139/f02-139
94. Morgan A, Burgess GH. At-vessel fishing mortality for six species of sharks caught in the Northwest Atlantic and Gulf of Mexico. Gulf Caribb Res. (2007) 19:123–9. doi: 10.18785/gcr.1902.15
95. Weltersbach MS, Strehlow HV. Dead or alive—estimating post-release mortality of Atlantic cod in the recreational fishery. ICES J Mar Sci. (2013) 70:864–72. doi: 10.1093/icesjms/fst038
96. Elliot JM. “Some aspects of thermal stress on freshwater teleost.” In:Pickering AD, , editor, Stress and Fish. London: Academic Press (1981). p. 209–45.
97. Carlson J, Goldman K, Lowe C. “Metabolism, energetic demand, and endothermy.” In: Biology of Sharks and Their Relatives. CRC Press (2004). p. 203–24. doi: 10.1201/9780203491317.ch7
98. Neat FC, Burns F, Jones E, Blasdale T. The diversity, distribution, and status of deep-water elasmobranchs in the Rockall Trough, northeast Atlantic Ocean. J Fish Biol. (2015) 87:1469–88. doi: 10.1111/jfb.12822
99. Ebert DA, Dando M, Fowler S, editor. Sharks of the World. Princeton University Press (2021). doi: 10.1515/9780691210872
100. Frick LH, Reina RD, Walker TI. Stress-related physiological changes and post-release survival of Port Jackson sharks (Heterodontus portusjacksoni) and gummy sharks (Mustelus antarcticus) following gill-net and longline capture in captivity. J Exp Mar Biol Ecol. (2010) 385:29–37. doi: 10.1016/j.jembe.2010.01.013
101. Heard M, Van Rijn JA, Reina RD, Huveneers C. Impacts of crowding, trawl duration, and air exposure on the physiology of stingarees (Family: Urolophidae). Conserv Physiol. (2014) 2:cou040. doi: 10.1093/conphys/cou040
102. Ruiz-Jarabo I, Paullada-Salmerón JA, Jerez-Cepa I, Gonçalves Neto JB, Bystriansky JS, Mancera JM. Acute stress in lesser-spotted catshark (Scyliorhinus canicula Linnaeus, 1758) promotes amino acid catabolism and osmoregulatory imbalances. Animals. (2022) 12:1192. doi: 10.3390/ani12091192
103. Falco F, Bono G, Cammarata M, Cavalca J, Vazzana I, Dara M, et al. Stress-related blood values in Scyliorhinus canicula as live-indicators of physiological status after bottom trawling capture activity. Comp Biochem Physiol B Biochem Mol Biol. (2023) 263:110802. doi: 10.1016/j.cbpb.2022.110802
104. Martini FH. Effects of Capture and Fasting Confinement on an Elasmobranch, Squalus acanthias. [Unpublished doctoral dissertation]. Cornell University (1974).
105. Butcher PA, Peddemors VM, Mandelman JW, McGrath SP, Cullis BR. At-vessel mortality and blood biochemical status of elasmobranchs caught in an Australian commercial longline fishery. Glob Ecol Conserv. (2015) 3:878–89. doi: 10.1016/j.gecco.2015.04.012
106. Piermarini PM, Evans DH. Effects of environmental salinity on Na+/K+-ATPase in the gills and rectal gland of a euryhaline elasmobranch (Dasyatis sabina). J Exp Biol. (2000) 203:2957–66. doi: 10.1242/jeb.203.19.2957
107. Dobson GP, Hochachka PW. Role of glycolysis in adenylate depletion and repletion during work and recovery in teleost white muscle. J Exp Biol. (1987) 129:125–40. doi: 10.1242/jeb.129.1.125
108. Girard SS, Milligan CL. The metabolic fate of blood-borne lactate in winter flounder (Pseudopleuronectes americanus) during recovery from strenuous exercise. Physiol Zool. (1992) 65:1114–34. doi: 10.1086/physzool.65.6.30158271
109. Spargo A. The Physiological Effects of Catch and Release Angling on the Post-Release Survivorship of Juvenile Sandbar Sharks (Carcharhinus plumbeus). [Master's thesis, University of Rhode Island] (2001).
110. Mandelman JW, Farrington MA. The estimated short-term discard mortality of a trawled elasmobranch, the spiny dogfish (Squalus acanthias). Fish Res. (2007) 83:238–45. doi: 10.1016/j.fishres.2006.10.001
111. Heberer C, Aalbers SA, Bernal D, Kohin S, DiFiore B, Sepulveda CA. Insights into catch-and-release survivorship and stress-induced blood biochemistry of common thresher sharks (Alopias vulpinus) captured in the southern California recreational fishery. Fish Res. (2010) 106:495–500. doi: 10.1016/j.fishres.2010.09.024
112. Edwards JR. The determinants and consequences of coping with stress. In:Cooper CL, Payne R, , editors. Causes, Coping and Consequences of Stress and Work. New York, NY: Wiley (1988). p. 233–66.
113. Chapman CA, Renshaw GMC. Hematological responses of the grey carpet shark (Chiloscyllium punctatum) and the epaulette shark (Hemiscyllium ocellatum) to anoxia and re-oxygenation. J Exp Zool Part A Ecol Genet Physiol. (2009) 311A:422–38. doi: 10.1002/jez.539
114. Frick LH, Reina RD, Walker TI. The physiological response of Port Jackson sharks and Australian swellsharks to sedation, gill-net capture, and repeated sampling in captivity. North Am J Fish Manag. (2009) 29:127–39. doi: 10.1577/M08-031.1
115. Isaksen B, Valdemarsen JW, Larsen RB, Karlsen L. Reduction of fish by-catch in shrimp trawl using a rigid separator grid in the aft belly. Fish Res. (1992) 13:335–52. doi: 10.1016/0165-7836(92)90086-9
116. Brewer D, Rawlinson N, Eayrs S, Burridge C. An assessment of bycatch reduction devices in a tropical Australian prawn trawl fishery. Fish Res. (1998) 36:195–215. doi: 10.1016/S0165-7836(98)00096-4
117. Brewer D, Heales D, Milton D, Dell Q, Fry G, Venables B, Jones P. The impact of turtle excluder devices and bycatch reduction devices on diverse tropical marine communities in Australia's northern prawn trawl fishery. Fish Res. (2006) 81:176–88. doi: 10.1016/j.fishres.2006.07.009
118. Chosid DM, Pol M, Szymanski M, Mirarchi F, Mirarchi A. Development and observations of a spiny dogfish (Squalus acanthias) reduction device in a raised footrope silver hake (Merluccius bilinearis) trawl. Fish Res. (2012) 114:66–75. doi: 10.1016/j.fishres.2011.03.007
119. Vasapollo C, Virgili M, Petetta A, Bargione G, Sala A, Lucchetti A. Bottom trawl catch comparison in the Mediterranean Sea: flexible turtle excluder device (TED) vs traditional gear. PLoS ONE. (2019) 14:e0216023. doi: 10.1371/journal.pone.0216023
120. Fonseca P, Campos A, Larsen R, Borges TC, Erzini K. Using a modified Nordmøre grid for by-catch reduction in the Portuguese crustacean trawl fishery. Fish Res. (2005) 71:223–39. doi: 10.1016/j.fishres.2004.08.018
121. Campos A, Fonseca P, Erzini K. Size selectivity of diamond and square mesh codends for rose shrimp (Parapenaeus longirostris) and Norway lobster (Nephrops norvegicus) off the Portuguese south coast. Fish Res. (2002) 58:281–301. doi: 10.1016/S0165-7836(01)00396-4
122. Campos A, Fonseca P. Reduction of unwanted by-catch in the Portuguese crustacean trawl fishery through the use of square mesh windows. J Fish Aquat Sci. (2007) 2:17–26. doi: 10.3923/jfas.2007.17.26
123. Campos A, Fonseca P, Erzini K. Size selectivity of diamond and square mesh codends for four by-catch species in the crustacean fishery off the Portuguese south coast. Fish Res. (2003) 60:79–97. doi: 10.1016/S0165-7836(02)00061-9
124. Campos A, Fonseca P, Henriques V. Size selectivity for four fish species of the deep groundfish assemblage off the Portuguese southwest coast: evidence of mesh size, mesh configuration, and cod end catch effects. Fish Res. (2003) 63:213–33. doi: 10.1016/S0165-7836(03)00060-2
125. Fonseca P, Campos A, Millar RB. Codend selection in the deep-water crustacean trawl fishery in Portuguese southern waters. Fish Res. (2007) 85:49–60. doi: 10.1016/j.fishres.2006.11.036
126. Gupta T, Booth H, Arlidge W, Rao C, Manoharakrishnan M, Namboothri N, et al. Mitigation of elasmobranch bycatch in trawlers: a case study in Indian fisheries. Front Mar Sci. (2020) 7:571. doi: 10.3389/fmars.2020.00571
127. Guisan A, Thuiller W. Predicting species distribution: offering more than simple habitat models. Ecol Lett. (2005) 8:993–1009. doi: 10.1111/j.1461-0248.2005.00792.x
128. Elith J, Leathwick JR. Species distribution models: ecological explanation and prediction across space and time. Annu Rev Ecol Evol Syst. (2009) 40:677–97. doi: 10.1146/annurev.ecolsys.110308.120159
129. Das D, Gonzalez-Irusta JM, Morato T, Fauconnet L, Catarino D, Afonso P, et al. Distribution models of deep-sea elasmobranchs in the Azores, Mid-Atlantic Ridge, to inform spatial planning. Deep Sea Res Part I Oceanogr Res Pap. (2022) 182:103707. doi: 10.1016/j.dsr.2022.103707
130. Musyl MK, Brill RW, Curran DS, Fragoso NM, McNaughton LM, Nielsen A, Moyes CD. Postrelease survival, vertical and horizontal movements, and thermal habitats of five species of pelagic sharks in the central Pacific Ocean. Fish Bull. (2011) 109:341–68.
131. Poisson F, Séret B, Vernet A-L, Goujon M, Dagorn L. Collaborative research: development of a manual on elasmobranch handling and release best practices in tropical tuna purse-seine fisheries. Marine Policy. (2014) 44:312–20. doi: 10.1016/j.marpol.2013.09.025
132. Campos A, Fonseca P, Henriques V, Parente J. Reducing by-catch in Portuguese trawl fisheries with aview on the future discard-ban at EU level—a technological approach. In:Soares CG, Pena FL, , editors. Developments in Maritime Transportation and Exploitation of Sea Resources. IMAM 2013. Vol. 2. London: Taylor and Francis Group (2014).
Keywords: at-vessel mortality, condition, survival, plasma, secondary responses, Iberian Peninsula, Portugal
Citation: Graça Aranha S, Teodósio A, Marsili T, Pires da Rocha P, Modesto T, Guerreiro PM, Tambutte A, Alves A, Relvas P and Dias E (2025) Under pressure: deep-sea elasmobranchs experience high mortality and stress in a crustacean trawling fishery. Front. Fish Sci. 3:1473376. doi: 10.3389/frish.2025.1473376
Received: 30 July 2024; Accepted: 06 March 2025;
Published: 11 April 2025.
Edited by:
Johann Mourier, Université de Montpellier, FranceReviewed by:
Brittany Finucci, National Institute of Water and Atmospheric Research, New ZealandFlorencia Cerutti, Marbec-Ifremer, France
Copyright © 2025 Graça Aranha, Teodósio, Marsili, Pires da Rocha, Modesto, Guerreiro, Tambutte, Alves, Relvas and Dias. This is an open-access article distributed under the terms of the Creative Commons Attribution License (CC BY). The use, distribution or reproduction in other forums is permitted, provided the original author(s) and the copyright owner(s) are credited and that the original publication in this journal is cited, in accordance with accepted academic practice. No use, distribution or reproduction is permitted which does not comply with these terms.
*Correspondence: Sofia Graça Aranha, c2dyYW1vc0B1YWxnLnB0