- 1School of Ecology and Nature Conservation, Beijing Forestry University, Beijing, China
- 2Department of Molecular Genetics and Microbiology, School of Medicine, Duke University, Durham, NC, United States
- 3College of Grassland Science, Beijing Forestry University, Beijing, China
Inverted repeat (IR) regions in the plastomes from land plants induce homologous recombination, generating isomeric plastomes. While the plastomes of Taxaceae species often lose one of the IR regions, considerable isomeric plastomes were created in Taxaceae species with a hitherto unclarified mechanism. To investigate the detailed mechanism underpinning the IR-independent genesis of plastomic diversity, we sequenced four Taxaceae plastomes, including Taxus cuspidata Siebold & Zuccarini, Taxus fauna Nan Li & R. R. Mill, and two individuals of Taxus wallichiana Zuccarini. Then we compared these structures with those of previously reported Taxaceae plastomes. Our analysis identified four distinct plastome forms that originated from the rearrangements of two IR-flanking inverted fragments. The presence of isomeric plastomes was then verified in T. cuspidata individuals. Both rearrangement analyses and phylogenetic results indicated that Taxaceae were separated into two clades, one including Taxus and Pseudotaxus and another formed by Amentotaxus and Torreya. Our reconstructed scenario suggests that the minimum number of inversion events required for the transformation of the plastome of Cephalotaxus oliveri Masters into the diversified Taxaceae plastomes ranged from three to six. To sum up, our study reveals a distinct pattern and the mechanism driving the structural diversification of Taxaceae plastomes, which will advance our understanding of the maintenance of plastomic diversity and complexity in conifers.
Introduction
Chloroplast (cp) is the organelle responsible for photosynthesis and providing energy for plants and photosynthetic algae (Dyall et al., 2004). Each chloroplast has its own genome (plastome) with a typical circular organization (Palmer, 1991). With the development of next-generation sequencing (NGS) and other methods for obtaining the plastomic sequences, the availability of plastome sequences has increased dramatically for land plants, offering opportunities for the comprehensive comparison and dissection of plastomic structure and variation (Du et al., 2015).
The plastomes of most land plants and algae are composed of four parts, namely, two copies of large inverted repeats (IRs) that contain four ribosomal RNA genes (rrn16, rrn23, rrn4.5, and rrn5), a large single copy (LSC) and a small single copy (SSC) region (Wang et al., 2008; Wicke et al., 2011; Davis et al., 2014). However, not all gymnosperms follow this rule. Gymnosperms are generally categorized into five groups, namely, cycads, ginkgo, gnetophytes, Pinaceae (conifers I), and cupressophytes (conifers II) (Chaw et al., 1997; Rai et al., 2008). While the plastomes of cycads, ginkgo, and gnetophytes are known to be quadripartite, recent comparative analyses of conifer plastomes have revealed that Pinaceae and cupressophyte species possess reduced IR copies. Comparative analysis have demonstrated that the Pinaceae have lost inverted repeat region B (IRB) and the cupressophytes have lost inverted repeat region A (IRA), suggesting the IR loss is homoplasious rather than synapomorphic (Wu et al., 2011; Wu and Chaw, 2014).
So far, two mechanisms have been proposed to explain the genesis of isomeric plastomes. For plants wherein the two IRs are present, the IRs can trigger homologous recombination, leading to the coexistence of two isomeric plastomes within individual plants (Palmer, 1983). However, conifers wherein only one IR is present have evolved short IRs that are associated with inversions in their plastomes (Wu and Chaw, 2016). In Pinaceae, a 40- to 50-kb inversion that can be used to distinguish Pseudotsuga species from that of Pinus has been found (Tsai and Strauss, 1989) and a 42-kb inversion was detected in Abies and Tsuga species (Tsumura et al., 2000). In cupressophytes, recombination of plastomes always occurs with a 34- to 36-kb inversion containing trnQ-UUG, as evidenced in Cephalotaxus (Yi et al., 2013) and Juniperus species (Guo et al., 2014). Although inversion has been shown to contribute to the genesis of isomeric plastomes in plants, the underlying mechanism remains not well studied (Tsumura et al., 2000; Hirao et al., 2008; Wu et al., 2011; Hsu et al., 2014; Wu and Chaw, 2014). Therefore, it is of scientific interest to elucidate the mechanism driving the diversification of plastomic organization in plants with reduced IR copies.
Taxaceae, the smallest family of conifers, belongs to the conifer II group. It consists of four genera, namely, Amentotaxus, Pseudotaxus, Taxus, and Torreya (Fu et al., 1999; Cheng et al., 2000). Some Taxaceae species have been commercially exploited for the extraction of the anticancer chemotherapeutic drug Taxol since 1990s (e.g., Poudel et al., 2013). Up to now, most of the studies concerning Taxaceae plants focused on the improvement of Taxol production (Wang et al., 2001; Li et al., 2017). For plastomic studies, genetic analyses using plastome fragments have been conducted to reconstruct the phylogeny of the Taxaceae family (e.g., Ran et al., 2010), to develop barcodes to differentiate different species (Gao et al., 2017; Liu et al., 2018), and to explore the demographic history of certain species or species complexes (Ge et al., 2015; Su et al., 2018). Recently, several groups released a number of Taxaceae plastomes (Li et al., 2016; Tao et al., 2016; Jia and Liu, 2017), and both intraspecific and interspecific isomeric arrangements have been reported (Hsu et al., 2014; Fu et al., 2019). However, the relative location and genomic organization of the inverted fragments in Taxaceae plastomes have not been comprehensively studied yet.
In this study, we firstly sequenced and analyzed four newly obtained Taxus plastomes from Taxus cuspidata Siebold & Zuccarini, Taxus fauna Nan Li & R. R. Mill, and two individuals of Taxus wallichiana Zuccarini. Next, we compared them with 11 other published Taxaceae plastomes to explore the evolutionary pattern underlying the structural diversification of Taxaceae plastomes. The information provided here will significantly advance our understanding of the functional significance of inversion and the nature of Taxaceae plastomes.
Materials and Methods
Sampling, DNA Extraction, and Processing
Fresh leaves were sampled from four individuals of three Taxus species including T. cuspidata, T. fauna, T. wallichiana XI3, and T. wallichiana XU10 (Supplementary Table S1). The vouchers of these species were deposited in Beijing Forestry University, Beijing, China. The chloroplast DNA was extracted and processed following the protocol developed by our group (Du et al., 2015). Next, 5 µg of the purified rolling circle amplification (RCA) product of chloroplast DNA from individual plants was used for library preparation.
Sequencing, Assembly, and Annotation
The paired-end sequencing was performed on an Illumina-HiSeq 2000 system (Illumina, USA) by OE Biotech Co., China. For each species, more than 10 Gb of clean data were generated. We assembled the plastomes using NOVOPlasty 2.6.3.pl (Dierckxsens et al., 2017) and the plastome of Taxus wallichiana var. chinensis (Pilger) Florin (KX431996) was used as a reference (Jia and Liu, 2017). The gaps were bridged using PCR with designed primers (Supplementary Table S2). We annotated the sequences with CpGAVAS (Liu et al., 2012; http://47.96.249.172:16014/analyzer/home) and verified all transfer RNA (tRNA) genes using tRNAscan-SE search server (Lowe and Eddy, 1997; http://lowelab.ucsc.edu/tRNAscan-SE/). The plastome sequences were deposited in GenBank under the following accession numbers: MF095888 for the plastome of T. cuspidata, MF278259 for the plastome of T. fauna, MF850258 for the plastome of T. wallichiana XI3, and MG011728 for the plastome of T. wallichiana XU10 (Table 1). The circular plastome maps of the four Taxus species were drawn using OGDRAW (Lohse et al., 2013; https://chlorobox.mpimp-golm.mpg.de/OGDraw.html).
Comparison of Plastomic Structure
Fifteen Taxaceae plastomes (Table 1) were used to conduct comparative genomic analyses using Cephalotaxus oliveri Masters (KC136217) as reference (Ran et al., 2010; Ran et al., 2018). Sequences were downloaded from the National Center for Biotechnology Information (NCBI) (https://www.ncbi.nlm.nih.gov/). Dot-plot analysis between the plastomes of Taxaceae species and that of C. oliveri was conducted using the Blast program (https://blast.ncbi.nlm.nih.gov). In order to better understand the structure of Taxaceae plastomes, locally co-linear blocks (LCBs) among the 16 plastomes were identified using Mauve v2.4.0 (Darling et al., 2010). For this analysis, the start point of each genome was manually set to the start codon of psbA. The REPuter software (https://bibiserv.cebitec.uni-bielefeld.de/reputer/) was used to locate inverted repeats which flanked the inverted fragment with a minimum repeat size of 8 bp and sequence identity greater than 80% (Kurtz et al., 2001). The minimum number of inversion events required for the gene order transformations was estimated by GRIMM (Tesler, 2002; http://grimm.ucsd.edu/cgi-bin/grimm.cgi).
Phylogenetic Analyses
To determine the evolutionary relationship of Taxaceae, we performed phylogenetic analyses using 73 protein-coding genes shared by all plastomes. After alignment by MAFFT v7 (Katoh and Standley, 2013), the third codon sites were deleted by DAMBE (Xia and Xie, 2001). We found model “GTR+I+G” is the fittest model for phylogenetic construction by jModelTest (Posada, 2008). Finally, Bayesian inference (BI) and maximum parsimony (MP) phylogenomic tree of 16 plastome sequences based on the protein-coding genes without the third codon were constructed. BI phylogenetic analyses were performed in MrBayes v3.2.3 (Ronquist and Huelsenbeck, 2003). The Markov chain Monte Carlo (MCMC) algorithm was run for 1,000,000 generations with trees sampled every 500 generations. MP phylogenetic analyses were performed in PAUP v4 (Swofford, 2003) using 1,000 bootstrap replicates.
Detection of Isomeric Plastomes
We investigated the presence of isomeric plastomes in three Taxus species (Supplementary Table S3). Voucher specimens of these samples were deposited in Beijing Forestry University, Beijing, China. Primer pairs listed in Supplementary Table S4 were used to amplify DNA fragments specific to the four isomeric plastomes from Taxaceae species. Each of a 25-µl PCR reaction mixture contained 1.5 µl of total genomic DNA as the template, 0.75 µl of each of the primers (10 µmol/L), 12.5 µl of 2× PCR buffer for KOD FX, 5 µl of 2 mM dNTPs, 0.5 µl of KOD FX, and 4 µl of double-distilled water. The PCR conditions were 94°C for 2 min, followed by 35 cycles of 98°C for 10 s, 56°C for 30 s, and 68°C for 1 min.
Results
Characteristics of the Four Newly Obtained Plastomes
Using T. wallichiana var. chinensis as reference (Jia and Liu, 2017), we assembled the four newly sequenced Taxus plastomes with NOVOPlasty 2.6.3.pl (Dierckxsens et al., 2017) and obtained circular sequences of size ranging from 128,001 to 129,022 bp (Table 1 and Figure 1). Annotation by CpGAVAS and tRNAscan-SE search server suggests that each plastome contained 82 protein-coding genes, 28 tRNA genes, and four ribosomal RNA (rRNA) genes. Among these genes, only trnI-CAU and trnQ-UUG had two copies. When compared with the other six Taxus plastomes, namely, Taxus baccata, Taxus mairei, T. mairei SNJ046, T. mairei WC052, T. mairei NN014, and T. wallichiana var. chinensis, these 10 plastomes were comparable regarding plastome size, gene number, and guanine–cytosine (GC) content (34.59–34.76%; Table 1). Overall, the contents of protein-coding genes were relatively consistent among Taxaceae species. The plastomes of Amentotaxus and Torreya species were larger than 130 kb and harbored more genes (Table 1). However, the plastome of Pseudotaxus chienii was smaller and contained only three rRNA genes (rrn4.5, rrn5, and rrn23), suggesting that it might have evolved toward reduced size (Wu and Chaw, 2014; Wu and Chaw, 2016).
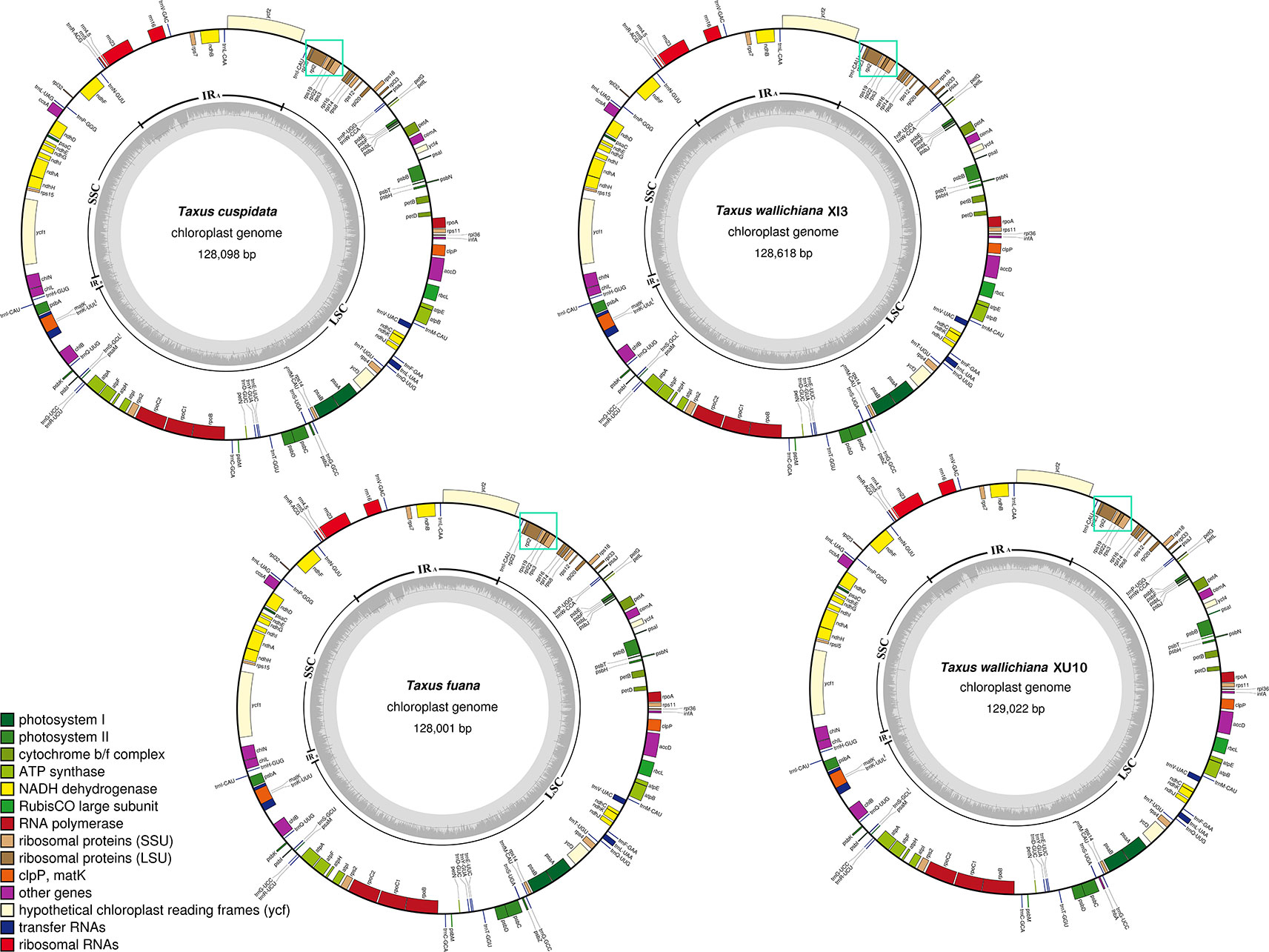
Figure 1 Circular map of the four newly sequenced Taxaceae plastomes. The genes that are transcribed clockwise are depicted on the outside of the circle, while those transcribed counterclockwise are depicted inside. The genes belonging to different functional groups are shown in different colors. Dashed area in the inner circle indicates the GC content of the plastomes. The cyan solid boxes stand for the rpl23-rps3 clusters that are always located downstream of the IRB region.
Diversification in the Structure of Taxaceae Plastomes
In this study, we found that the rpl23-rps3 cluster (cyan solid boxes in Figure 1) was adjacent to the unique rRNA operon (rrn16, rrn23, rrn4.5, and rrn5). Through a careful check of the conserved gene order as suggested by Wu et al. (2011), we further determined that the lost IR copy was likely to be IRA, rather than IRB.
Our dot-plot analyses indicated that there were two inverted fragments. Fragment of infA-rps12 was approximately 18 kb in length and hereafter termed as R1. Fragment of trnQ-IR was approximately 34 kb in length and resulted from the full duplication of trnQ-UUG gene, so it was hereafter termed as R2. A relocated fragment of petN-psbM in R2 separated R2 into two parts, namely, psbK to trnC-GCA (~18 kb) and trnD-GUC to trnT-UGU (~16 kb) (Figures 2 and 3 and Supplementary Figure 1).
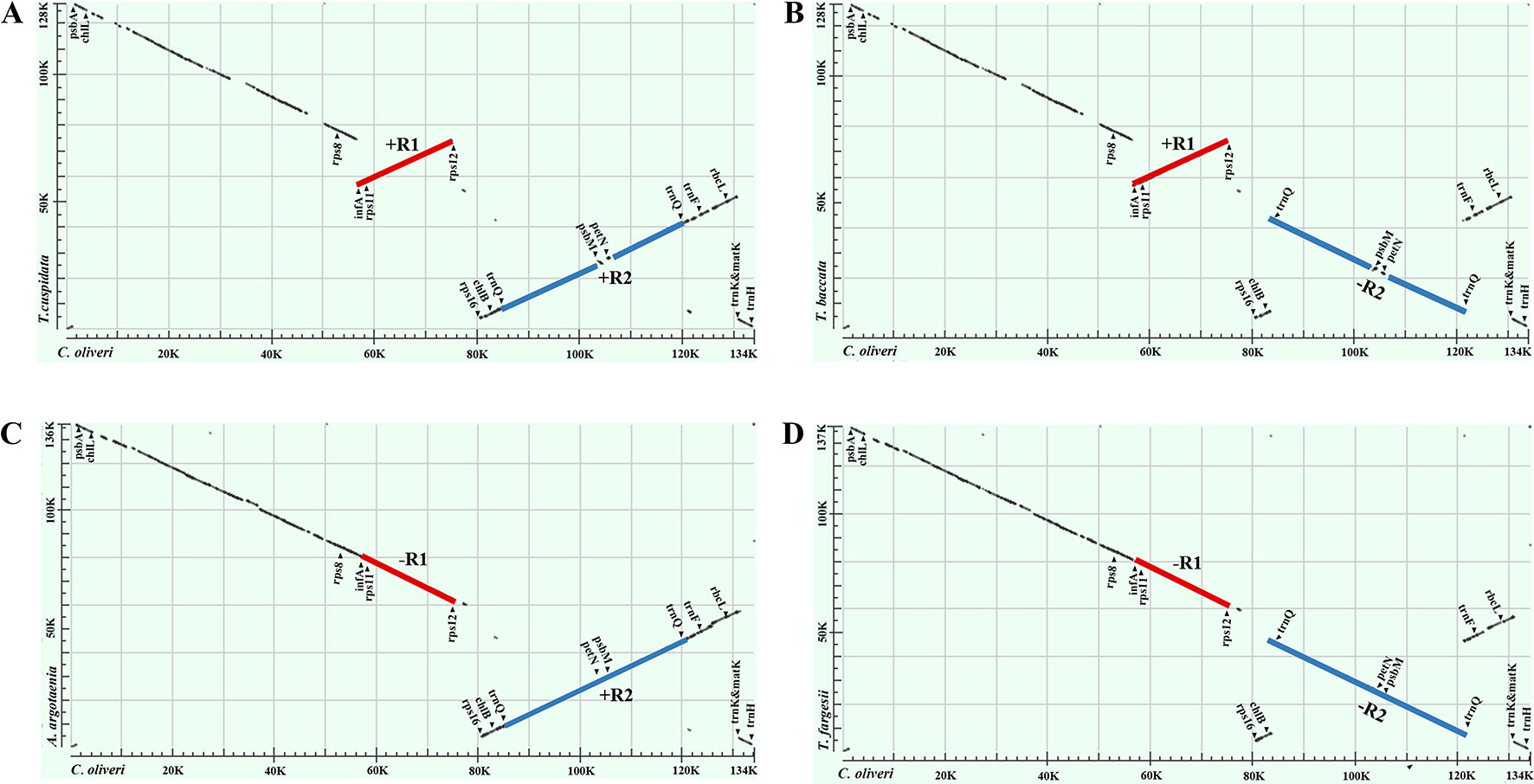
Figure 2 Dot-plot analyses of the four Taxaceae plastomes. The Cephalotaxus oliveri plastome (horizontal axes) was used as the reference. A positive slope denotes that the plastomic sequences of the two species can be aligned in the same orientation, whereas a negative one indicates that the two sequences are in the opposite orientations. Labeling of the genes is based on their corresponding positions in the C. oliveri plastome. The red line represents the region between infA and rps12 and the blue line represents the region between trnQ-IRs. These two regions were termed R1 and R2, respectively. The form (A) plastomes were characterized by +R1 and +R2, the form (B) plastomes were characterized by +R1 and −R2, the form (C) plastomes were characterized by −R1 and +R2 and the form (D) plastomes were characterized by −R1 and −R2.
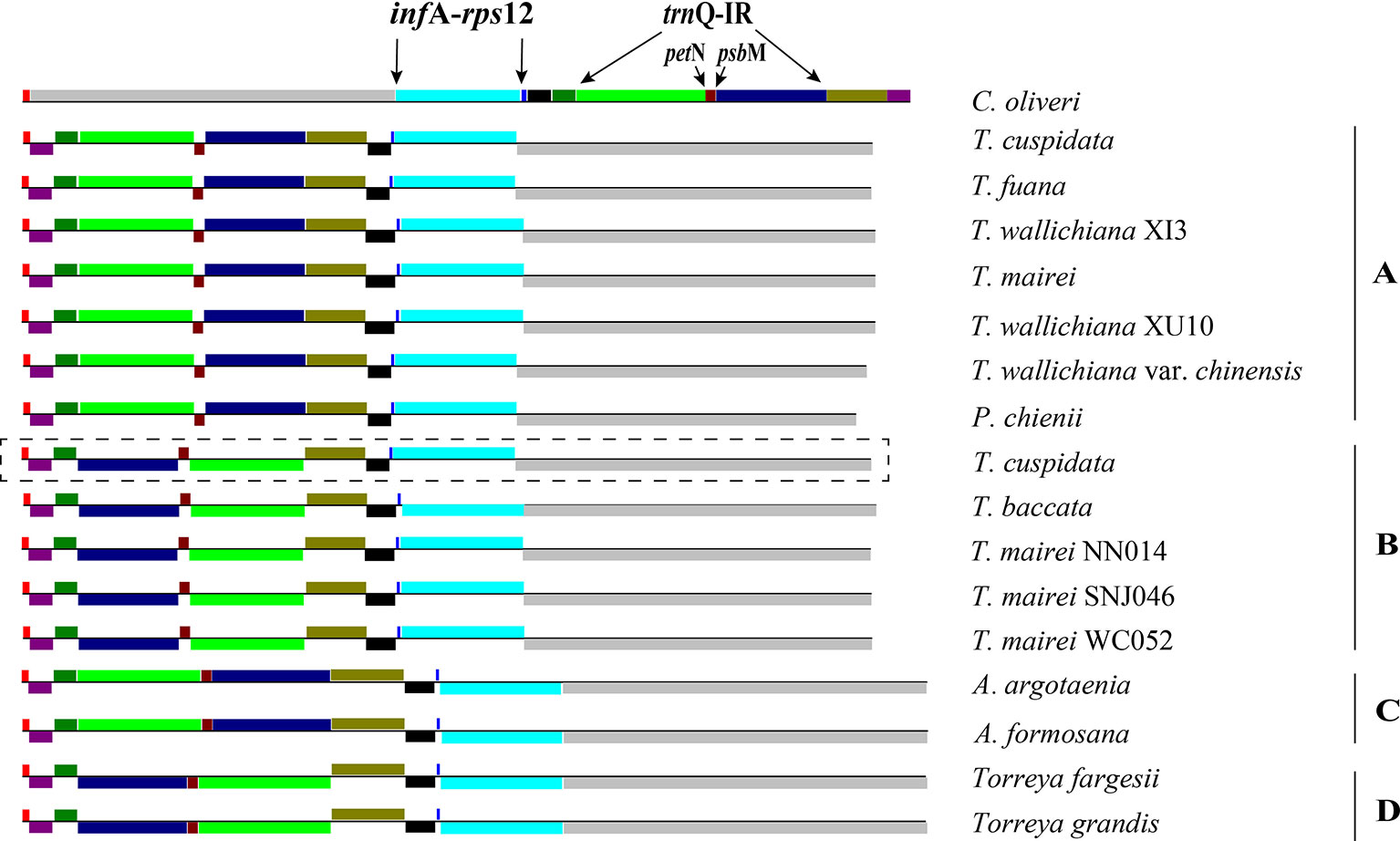
Figure 3 Structures of Taxaceae plastomes. The 11 locally collinear blocks (LCBs) are depicted with different colors on the left side. LCBs below the horizontal line represent opposite orientations when compared to that of Cephalotaxus oliveri. (A–D) after species indicates the categorization of its plastomic structure. Plastome form of Taxus cuspidata that showed in the rectangle with dashed lines represents the structure discovered by PCR. Plastome form of T. cuspidata that showed without the rectangle is based on the plastome sequence assembled from next-generation sequencing data.
We categorized the Taxaceae plastomes into four forms, namely, A, B, C, and D, based on the relative location and orientation of R1 and R2 (Figure 2 and Supplementary Figure 1). Plastome assembly form A is considered as the reference hereafter. Form B plastomes carried an inverted R2, resulting in increased adjacencies between chlB and rps4, psbK and trnL and reduced adjacencies between chlB and psbK, rps4 and trnL (Figure 4). Form C plastomes differed from those of form A in the arrangement of R1 and petN-psbM and exhibited increased adjacencies between clpP and rps12, infA and rps8, trnD and psbM, and petN and trnC and reduced adjacencies between clpP and infA, rps12 and rps8, trnC and psbM, and petN and trnD (Figure 4). Form D plastomes had R1 and R2 arrangements distinct from those of the other three forms.
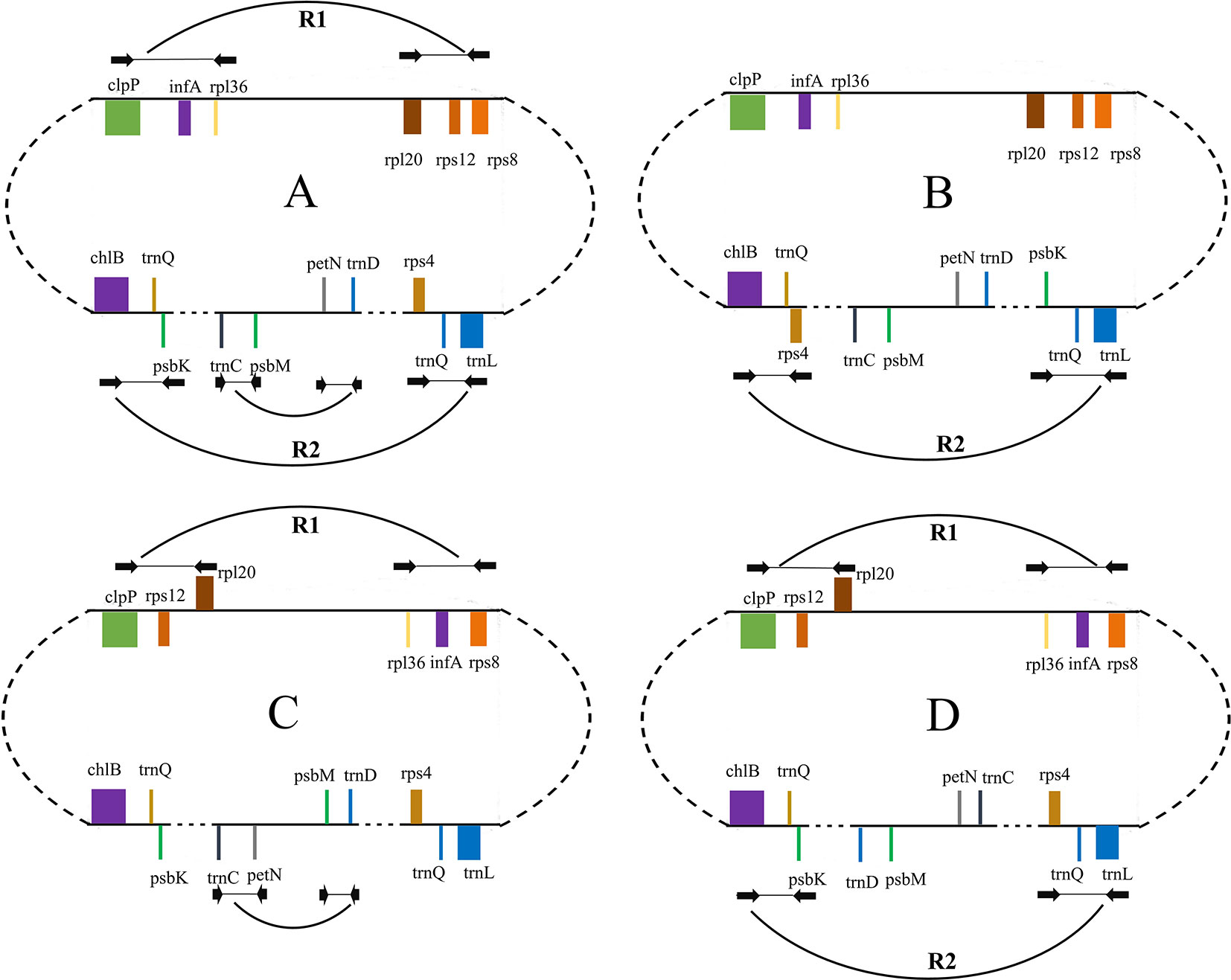
Figure 4 Schematic representation of different forms of isomeric plastomes in Taxus cuspidata individuals. Form (A) is the map of the plastomes obtained in our genome assembly shown in Figure 1. Form (B) differs from form (A) by an inversion of trnQ-IR fragment. Forms (A) and (C) differ by the inversion of infA-rps12 and petN-psbM fragments. Form (D) differs from form (A) by the inversion of infA-rps12 and trnQ-IR fragments. The paired PCR primers designed for the detection of isomeric plastomes are shown as black arrows. Ends of these inversion fragments are connected with breaking arcs.
To better understand the plastome organization of Taxaceae species, we compared the plastomes of 15 Taxaceae species or varieties with that of C. oliveri and determined 11 LCBs (Figure 3). We found the form A plastomes were characterized by +R1 and +R2 (+ denotes the forward strand and − denotes the reverse strand); for example, in Pseudotaxus chienii W. C. Cheng and six other Taxus species or varieties, namely, T. cuspidata, T. fauna, T. mairei, T. wallichiana var. chinensis, T. wallichiana XI3, and T. wallichiana XU10. The form B plastomes, characterized by +R1 and −R2, were found in four Taxus species, namely, T. baccata, T. mairei NN014, T. mairei SNJ046, and T. mairei WC052. The form C plastomes, characterized by −R1 and +R2, were found in Amentotaxus argotaenia Pilger and Amentotaxus formosana H. L. Li. The form D plastomes were characterized by −R1 and −R2 and were found in Torreya fargesii Franchet and Torreya grandis Fortune. Among the 15 Taxaceae plastomes, we observed that the organization of T. mairei plastome was of form A and the plastomes from three Taiwan T. mairei individuals (NN014, SNJ046, and WC052) were of form B, indicating that plastomes of these two forms are present in T. mairei (Figure 3).
Experimental Validation of the Four Arrangement Forms
We next performed PCR to verify the presence of these arrangement forms in plants of Taxaceae species (Figure 4). We found that 20 T. cuspidata individuals from 20 natural populations harbored two isomeric plastomes, namely, forms A and B, but not forms C and D (Figure 3, Supplementary Figure 2, and Supplementary Table S5). However, we did not find isomeric plastomes in T. fauna and T. wallichiana.
Evolutionary Path of Plastome IR Formation in Taxaceae
We detected several pairs of 9- to 12-bp inverted short repeats that specifically flanked the region of R1 in Taxaceae species, and these repeats were similar to those in species within the same genus (Figure 5). However, we failed to detect any inverted short repeats larger than 9 bp within the petN-psbM fragment. The inverted fragment R2 was flanked by the trnQ-IR that resulted from the full duplication of the trnQ-UUG gene of which the length ranged from 114 to 560 bp in the 15 Taxaceae plastomes (Table 2).
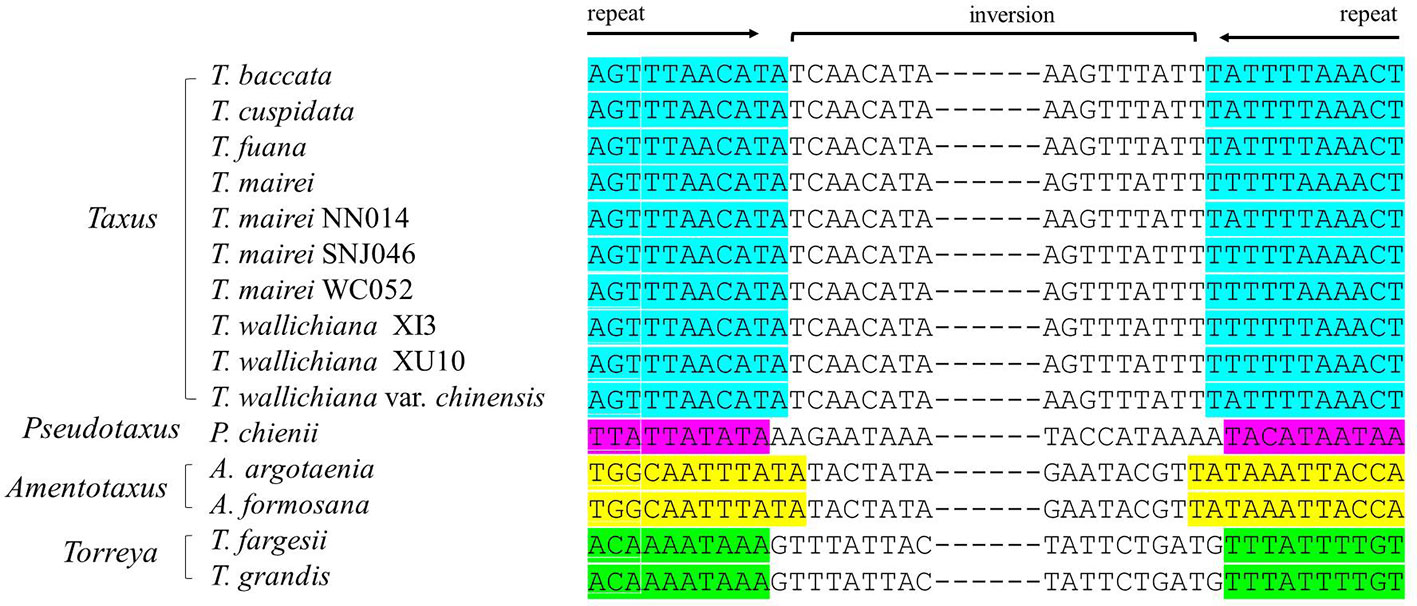
Figure 5 Pairs of short inverted repeats at the edges of the infA-rps12 fragment in Taxaceae plastomes. Repeats are represented using different colors.
As aforementioned, we identified 11 LCBs among the plastomes of the 15 Taxaceae individuals (Figure 3). The arrangements of these LCBs and that of C. oliveri were used to infer the most parsimonious rearrangement scenario that will provide information for the dissection of plastomic inversion history in Taxaceae species. The minimum number of inversion events required for the transformation from the C. oliveri plastome to the plastomes of forms A, B, C, and D was five, six, three, and four, respectively (Figure 6). As for the first transformation, the inversion of block 4 (clpP) took place, and then the inversion of block 8 (petN-psbM) occurred in one clade, generating the Taxus and Pseudotaxus plastomes. The other clade underwent the inversion of block 1 (psbA) and became the Amentotaxus and Torreya plastomes.
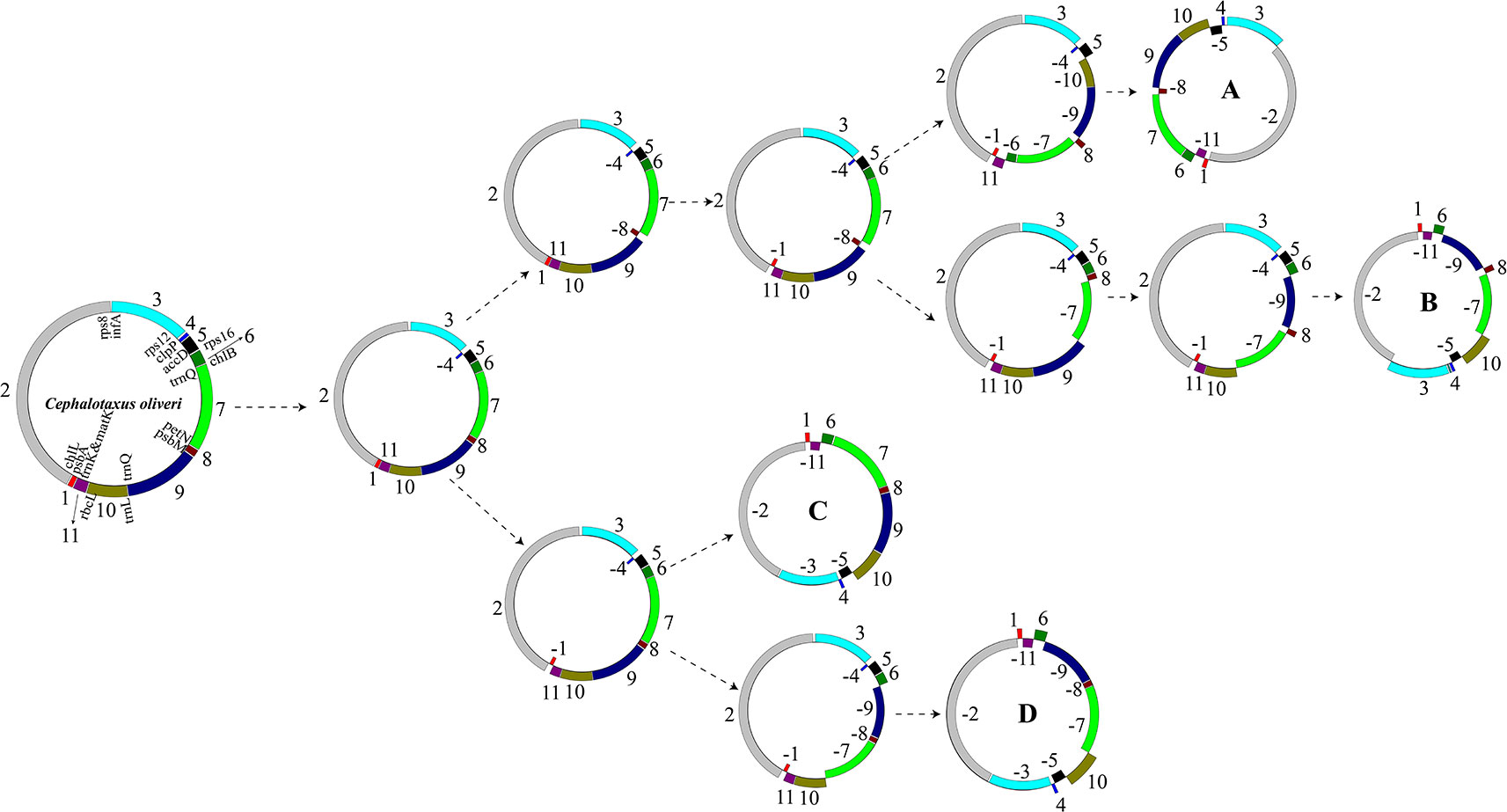
Figure 6 The putative transformation from the plastome of Cephalotaxus oliveri to the four diversified Taxaceae plastomes. Eleven locally collinear blocks (LCBs) were denoted using different colors. Gene orientations were shown by placing them inside or outside of the circles. The minimum number of inversion events required for the transformation from the plastome of C. oliveri to the Taxaceae plastomes was calculated using GRIMM.
A total of 41,596 aligned sites, including 5,357 variable and 2,849 parsimony-informative sites, were used to reconstruct the phylogenetic tree. MP and BI results both suggest that Taxaceae are separated into two clades: one including Taxus and Pseudotaxus and another formed by Amentotaxus and Torreya at the basal position (Supplementary Figure 3). These phylogenetic relationships are consistent with our GRIMM analysis results which also recovered these two clades (the A/B and C/D plastome forms) (Figure 6). Additionally, species from the same genus were placed on a single clade. However, neither T. wallichiana nor T. mairei appear to be monophyletic species in our tree (Supplementary Figure 3).
Discussion
Issues related to the IR region have been a research focus for several decades (Kolodner and Tewari, 1979; Zhu et al., 2016). In recent years, the rapidly increasing genomic resources of plants in the five gymnosperm groups have revealed considerable variations in plastomic structure (Raubeson and Jansen, 1992; Wu et al., 2009; Lin et al., 2012). The presence of only one IR copy in Pinaceae and Cupressophytes species was considered as a homologous character (Raubeson and Jansen, 1992). However, recent comparative analyses of conifer plastomes uncovered distinct evolutionary patterns in Pinaceae and cupressophytes plastomes, namely, the former lost IRB and the latter lost IRA (Wu et al., 2011; Hao et al., 2016). Due to the presence of isomeric plastomes, it has been difficult to determine which IR copy was lost (Yi et al., 2013). In this study, using a comparative plastome profiling, we revealed that the plastomes of all the 12 species from four different genera of Taxaceae contained an rpl23-rps3 cluster that is located downstream of the IRB, thus demonstrating that Taxaceae plastomes lack IRA (Figure 1).
IRs trigger homologous recombination and the coexistence of two isomeric plastomes has been found at the infraspecific level in numerous species (Palmer, 1983). Despite the absence of IRs, conifers contain isomeric plastomes that are produced by inversions, and these inversions are mediated by specific short IRs (Wu and Chaw, 2016). In our study, we revealed four new forms of organization in Taxaceae plastomes, which have possibly been generated by the rearrangements of two large inverted fragments (R1: infA-rps12 and R2: trnQ-IR) that are flanked by specific short repeats (Figure 5 and Table 2). We experimentally verified the presence of the isomeric arrangements of R2 inversion in T. cuspidata. However, we failed to identify isoforms in T. fauna and T. wallichiana. Diversified plastomic structure has also been found to be ubiquitous in other conifers. Four distinct types, generated by two inverted fragments (F1: trnR-UCU to trnE-UUC; F2: rps4 to trnG-GCC), have been uncovered in Pinaceae, and these two inverted fragments are flanked by Pinaceae-specific repeats (Tsumura et al., 2000; Wu et al., 2011). In cupressophytes, several types of inversion have been discovered. For example, a 36-kb inversion mediated by the 216- to 552-bp trnQ-IR has been found in the isomeric plastomes of Cephalotaxus (Yi et al., 2013), Juniperus (Guo et al., 2014), Pseudotaxus, and Taxus species (Fu et al., 2019). Besides, a 73-kb inversion in the isomeric plastomes of Sciadopitys species is mediated by a 370-bp IR (Hsu et al., 2016), while the isomeric plastomes of Calocedrus species are found to harbor a 34-kb rpl23-trnL/CAA inversion mediated by an 11-bp IR (Qu et al., 2017). Dealing with the rearrangements of two specific inverted fragments, our study provides novel insights into the structural diversification of Taxaceae plastomes.
Phylogeny of the Taxaceae genera has been controversial for many years (Lu et al., 2014; Wu and Chaw, 2016; Zhang et al., 2019). In this study, 73 shared protein-coding genes were used to determine the relationships among the four genera. We successfully obtained a high resolution of evolutionary relationships for the four genera and placed them on two clades of Taxaceae. Both BI and MP trees showed Amentotaxus and Torreya to branch just after the outgroup (Supplementary Figure 3). Our results are consistent with the phylogenic tree based on the rps3 gene (Ran et al., 2010) and transcriptomic data (Ran et al., 2018). Our rearrangement analyses by GRIMM provided the most parsimonious evolutionary scenario, which is consistent with the phylogenic tree (Figure 6).
It should be noted that several issues remain to be addressed. For example, the identification of the diversified plastomic structures here was based on the published Taxaceae plastomic sequences. Whether these diversified structures exist in other uncharacterized Taxaceae species needs further investigation. Besides, the presence of isomeric plastomes in Taxaceae species was only verified within only a few individuals. At the population level, however, their distribution remains unknown. Further exploration, such as determining whether there is a bias in the structure of plastome at the population level, will surely provide more information regarding the evolution of plastomes.
Conclusions
Using next-generation sequencing and de novo assembly, we obtained the complete plastomes of four individuals from three Taxus species and profiled their structures along with 11 published Taxaceae plastomes. Our analyses revealed that in Taxaceae plastomes, the rearrangements of two large fragments could lead to the genesis of the four distinct plastome forms. Further experiments verified the presence of two isomeric plastomes in T. cuspidata individuals. Based on these findings, both rearrangement analyses and phylogenetic results indicated that Taxaceae were separated into two clades, one including Taxus and Pseudotaxus and another formed by Amentotaxus and Torreya. These findings highlight that the evolution of plastomes may be more complicated than previously thought. The information provided here will significantly advance our understanding of the dynamic and complex evolution of plastomes in conifers.
Data Availability Statement
The datasets generated for this study can be found in NCBI SRA BioProject IDs PRJNA554197, PRJNA554072, PRJNA554062 and PRJNA553347.
Author Contributions
FD designed the study. YZ, YX, and HC performed the analysis. FD, YZ, KY and LW wrote the manuscript. All the authors revised the manuscript.
Funding
This work was supported by the National Key Research and Development Plan “Research on protection and restoration of typical small populations of wild plants” (grant no. 2016YFC0503106), Fundamental Research Funds for the Central Universities (no. 2015ZCQ-LX-03), and the National Science Foundation of China (grant 41671039) to FD.
Conflict of Interest
The authors declare that the research was conducted in the absence of any commercial or financial relationships that could be construed as a potential conflict of interest.
Acknowledgments
We thank suggestions from Dr. Saneyoshi Ueno working in Forest Research and Forest Products Research Institute, Japan, for comments on the ms; Prof. Yanhong Liu, Dr. Wande Liu, Prof. Deyou Qiu, Prof. Zhongling Guo, Prof. Gang Pan, Dr. Bin Tian, and Jia Song for sampling; and Dr. Jinhua Ran for discussion of the phylogeny of Taxaceae.
Supplementary Material
The Supplementary Material for this article can be found online at: https://www.frontiersin.org/articles/10.3389/fgene.2019.01295/full#supplementary-material
Supplementary Figure S1 | Comparative dot-plot analyses between the plastome of Cephalotaxus oliveri and the four Taxaceae plastomes. A positive slope denotes that the two sequences (horizontal and vertical axes) are matched in the same orientation, whereas a negative one indicates that the two sequences can be aligned but in the opposite orientations. Genes are labeled based on their corresponding positions in the C. oliveri plastomes.
Supplementary Figure S2 | The PCR results of the verification of isomeric plastomes in 20 individuals of T. cusipidata. The codes in the picture stand for different combination of individuals and primer pairs can be found in Table S5.
Supplementary Figure S3 | Phylogenetic relationships of the Taxaceae plastomes. The trees inferred from Bayesian inference (BI) and Maximum Parsimony (MP) are based on a nucleotide supermatrix of 73 shared protein-coding genes excluding the third codon positions. The BI topology is shown with BI posterior probability/MP bootstrap values given at each node.
References
Chaw, S. M., Zharkikh, A., Sung, H. M., Lau, T. C., Li, W. H. (1997). Molecular phylogeny of extant gymnosperms and seed plant evolution: analysis of nuclear 18S rRNA sequences. Mol. Biol. Evol. 14 (1), 56–68. doi: 10.1093/oxfordjournals.molbev.a025702
Cheng, Y., Nicolson, R. G., Tripp, K., Chaw, S. M. (2000). Phylogeny of Taxaceae and Cephalotaxaceae genera inferred from chloroplast matK gene and nuclear rDNA ITS region. Mol. Phylogenet. Evol. 14 (3), 353–365. doi: 10.1006/mpev.1999.0710
Darling, A. E., Mau, B., Perna, N. T. (2010). progressiveMauve: multiple genome alignment with gene gain, loss and rearrangement. PloS One 5 (6), e11147. doi: 10.1371/journal.pone.0011147
Davis, C. C., Xi, Z., Mathews, S. (2014). Plastid phylogenomics and green plant phylogeny: almost full circle but not quite there. BMC Biol. 12 (1), 11. doi: 10.1186/1741-7007-12-11
Dierckxsens, N., Mardulyn, P., Smits, G. (2017). NOVOPlasty: de novo assembly of organelle genomes from whole genome data. Nucleic Acids Res. 45 (4), e18. doi: 10.1093/nar/gkw955
Du, F. K., Lang, T., Lu, S., Wang, Y., Li, J., Yin, K. (2015). An improved method for chloroplast genome sequencing in non-model forest tree species. Tree Genet. Genomes 11 (6), 114. doi: 10.1007/s11295-015-0942-2
Dyall, S. D., Brown, M. T., Johnson, P. J. (2004). Ancient invasions: from endosymbionts to organelles. Science 304 (5668), 253–257. doi: 10.1126/science.1094884
Fu, C. N., Wu, C. S., Ye, L. J., Mo, Z. Q., Liu, J., Chang, Y. W., et al. (2019). Prevalence of isomeric plastomes and effectiveness of plastome super-barcodes in yews (Taxus) worldwide. Sci. Rep. 9 (1), 2773. doi: 10.1038/s41598-019-39161-x
Gao, L. M., Li, Y., Phan, L. K., Yan, L. J., Thomas, P., Phan, L. K., et al. (2017). DNA barcoding of East Asian Amentotaxus (Taxaceae): potential new species and implications for conservation. J. Syst. Evol. 55 (1), 16–24. doi: 10.1111/jse.12207
Ge, X. J., Hung, K. H., Ko, Y. Z., Hsu, T. W., Gong, X, Chiang, T. Y., et al. (2015). Genetic divergence and biogeographical patterns in Amentotaxus argotaenia species complex . Plant Mol. Biol. Rep. 33 (2), 264–280. doi: 10.1007/s11105-014-0742-0
Guo, W., Grewe, F., Cobo-Clark, A., Fan, W., Duan, Z., Adams, R. P., et al. (2014). Predominant and substoichiometric isomers of the plastid genome coexist within Juniperus plants and have shifted multiple times during cupressophyte evolution. Genome Biol. Evol. 6 (3), 580–590. doi: 10.1093/gbe/evu046
Hao, Z., Cheng, T., Zheng, R., Xu, H., Zhou, Y., Li, M., et al. (2016). The complete chloroplast genome sequence of a relict conifer Glyptostrobus pensilis: Comparative analysis and insights into dynamics of chloroplast genome rearrangement in cupressophytes and Pinaceae. PloS One 11 (8), e0161809. doi: 10.1371/journal.pone.0161809
Hirao, T., Watanabe, A., Kurita, M., Kondo, T., Takata, K. (2008). Complete nucleotide sequence of the Cryptomeria japonica D. Don. chloroplast genome and comparative chloroplast genomics: diversified genomic structure of coniferous species. BMC Plant Biol. 8 (1), 70. doi: 10.1186/1471-2229-8-70
Hsu, C. Y., Wu, C. S., Chaw, S. M. (2014). Ancient nuclear plastid DNA in the yew family (Taxaceae). Genome Biol. Evol. 6 (8), 2111–2121. doi: 10.1093/gbe/evu165
Hsu, C. Y., Wu, C. S., Chaw, S. M. (2016). Birth of four chimeric plastid gene clusters in Japanese umbrella pine. Genome Biol. Evol. 8 (6), 1776–1784. doi: 10.1093/gbe/evw109
Jia, X. M., Liu, X. P. (2017). Characterization of the complete chloroplast genome of the Chinese yew Taxus chinensis (Taxaceae), an endangered and medicinally important tree species in China. Conserv. Genet. Resour. 9 (2), 197–199. doi: 10.1007/s12686-016-0649-1
Katoh, K., Standley, D. M. (2013). MAFFT multiple sequence alignment software version 7: improvements in performance and usability. Mol. Biol. Evol. 30 (4), 772–780. doi: 10.1093/molbev/mst010
Kolodner, R., Tewari, K. K. (1979). Inverted repeats in chloroplast DNA from higher plants. Proc. Natl. Acad. Sci. U.S.A. 76 (1), 41–45. doi: 10.1073/pnas.76.1.41
Kurtz, S., Choudhuri, J. V., Ohlebusch, E., Schleiermacher, C., Stoye, J., Giegerich, R. (2001). REPuter: the manifold applications of repeat analysis on a genomic scale. Nucleic Acids Res. 29 (22), 4633–4642. doi: 10.1093/nar/29.22.4633
Li, J., Gao, L., Tao, K., Su, Y., Wang, T. (2016). The complete chloroplast genome sequence of Amentotaxus argotaenia (Taxaceae). Mitochondrial DNA A DNA Mapp. Seq. Anal. 27 (4), 2919–2920. doi: 10.3109/19401736.2015.1060439
Li, B. J., Wang, H., Gong, T., Chen, J. J., Chen, T. J., Yang, J. L., et al. (2017). Improving 10-deacetylbaccatin III-10-β-O-acetyltransferase catalytic fitness for Taxol production. Nat. Commun. 8, 15544. doi: 10.1038/ncomms15544
Lin, C. P., Wu, C. S., Huang, Y. Y., Chaw, S. M. (2012). The complete chloroplast genome of Ginkgo biloba reveals the mechanism of inverted repeat contraction. Genome Biol. Evol. 4 (3), 374–381. doi: 10.1093/gbe/evs021
Liu, C., Shi, L., Zhu, Y., Chen, H., Zhang, J., Lin, X., et al. (2012). CpGAVAS, an integrated web server for the annotation, visualization, analysis, and GenBank submission of completely sequenced chloroplast genome sequences. BMC Genomics 13 (1), 715. doi: 10.1186/1471-2164-13-715
Liu, J., Milne, R. I., Moller, M., Zhu, G. F., Ye, L. J., Luo, L. H., et al. (2018). Integrating a comprehensive DNA barcode reference library with the global map of yews (Taxus L.) for species identification. Mol. Ecol. Resour. 18 (5), 1115–1131. doi: 10.1111/1755-0998.12903
Lohse, M., Drechsel, O., Kahlau, S., Bock, R. (2013). Organellar genome DRAW-a suite of tools for generating physical maps of plastid and mitochondrial genomes and visualizing expression data sets. Nucleic Acids Res. 41 (W1), W575–W581. doi: 10.1093/nar/gkt289
Lowe, T. M., Eddy, S. R. (1997). tRNAscan-SE: a program for improved detection of transfer RNA genes in genomic sequence. Nucleic Acids Res. 25 (5), 955–964. doi: 10.1093/nar/25.5.955
Lu, Y., Ran, J. H., Guo, D. M., Yang, Z. Y., Wang, X. Q. (2014). Phylogeny and divergence times of gymnosperms inferred from single-copy nuclear genes. PloS One 9 (9), e107679. doi: 10.1371/journal.pone.0107679
Miu, Z. P., Zhang, J. M., Li, J. H., Hong, X., Pan, T. (2018). The complete chloroplast genome sequence of an conifer plant Torreya grandis (Pinales, Taxaceae). Mitochondrial DNA B Resour. 3 (2), 1152–1153. doi: 10.1080/23802359.2018.1522975
Palmer, J. D. (1983). Chloroplast DNA exists in two orientations. Nature 301, 92–93. doi: 10.1038/301092a0
Palmer, J. D. (1991). “Plastid chromosomes: structure and evolution” in The Molecular Biology of Plastids. Eds. Bogorad, L., Vasil, I. K. (San Diego, CA: Academic Press), 5–53. doi: 10.1016/B978-0-12-715007-9.50009-8
Posada, D. (2008). jModelTest: phylogenetic model averaging. Mol. Biol. Evol. 25 (7), 1253–1256. doi: 10.1093/molbev/msn083
Poudel, R. C., Gao, L. M., Möller, M., Baral, S. R., Uprety, Y., Liu, J., et al. (2013). Yews (Taxus) along the Hindu Kush-Himalayan region: exploring the ethnopharmacological relevance among communities of Mongol and Caucasian origins. J. Ethnopharmacol. 147 (1), 190–203. doi: 10.1016/j.jep.2013.02.031
Qu, X. J., Wu, C. S., Chaw, S. M., Yi, T. S. (2017). Insights into the existence of isomeric plastomes in Cupressoideae (Cupressaceae). Genome Biol. Evol. 9 (4), 1110–1119. doi: 10.1093/gbe/evx071
Rai, H. S., Reeves, P. A., Peakall, R., Olmstead, R. G., Graham, S. W. (2008). Inference of higher-order conifer relationships from multi-locus plastid dataset. Botany. 86 (7), 658–669. doi: 10.1139/B08-062
Ran, J. H., Gao, H., Wang, X. Q. (2010). Fast evolution of the retroprocessed mitochondrial rps3 gene in Conifer II and further evidence for the phylogeny of gymnosperms. Mol. Phylogenet. Evol. 54 (1), 136–149. doi: 10.1016/j.ympev.2009.09.011
Ran, J. H., Shen, T. T., Wang, M. M., Wang, X. Q. (2018). Phylogenomics resolves the deep phylogeny of seed plants and indicates partial convergent or homoplastic evolution between Gnetales and angiosperms. Proc. Biol. Sci. 285 (1881), 20181012. doi: 10.1098/rspb.2018.1012
Raubeson, L. A., Jansen, R. K. (1992). A rare chloroplast DNA structure mutation is shared by all conifers. Biochem. Syst. Ecol. 20 (1), 17–24. doi: 10.1016/0305-1978(92)90067-N
Ronquist, F., Huelsenbeck, J. P. (2003). MrBayes 3: Bayesian phylogenetic inference under mixed models. Bioinformatics 19 (12), 1572–1574. doi: 10.1093/bioinformatics/btg180
Su, J. Y., Yan, Y., Song, J., Li, J., Mao, J. F., Wang, N., et al. (2018). Recent fragmentation may not alter genetic patterns in endangered long-lived species: evidence from Taxus cuspidata. Front. Plant Sci. 9, 1571. doi: 10.3389/fpls.2018.01571
Swofford, D. L. (2003). PAUP*. Phylogenetic Analysis Using Parsimony (and Other Methods). Version 4. (Sunderland, MA: Sinauer Associates).
Tao, K., Gao, L., Li, J., Chen, S., Su, Y., Wang, T. (2016). The complete chloroplast genome of Torreya fargesii (Taxaceae). Mitochondrial DNA A DNA Mapp. Seq. Anal. 27 (5), 3512–3513. doi: 10.3109/19401736.2015.1074195
Tesler, G. (2002). GRIMM: genome rearrangements web server. Bioinformatics 18 (3), 492–493. doi: 10.1093/bioinformatics/18.3.492
Tsai, C. H., Strauss, S. H. (1989). Dispersed repetitive sequences in the chloroplast genome of Douglas-fir. Curr. Genet. 16, 211–218. doi: 10.1007/BF00391479
Tsumura, Y., Suyama, Y., Yoshimura, K. (2000). Chloroplast DNA inversion polymorphism in populations of Abies and Tsuga. Mol. Biol. Evol. 17 (9), 1302–1312. doi: 10.1093/oxfordjournals.molbev.a026414
Wang, C., Wu, J., Mei, X. (2001). Enhancement of taxol production and excretion in Taxus chinensis cell culture by fungal elicitation and medium renewal. Appl. Microbiol. Biotechnol. 55 (4), 404–410. doi: 10.1007/s002530000567
Wang, L. L., Shi, Y. L., Wang, C. X., Li, X. (2019). The complete chloroplast genome of the white-berry yew Pseudotaxus chienii (Cupressales: Taxaceae), a rare and endangered relict plant endemic to southern China. Mitochondrial DNA B Resour. 4 (1), 760–761. doi: 10.1080/23802359.2019.1565971
Wang, R. J., Cheng, C. L., Chang, C. C., Wu, C. L., Su, T. M., Chaw, S. M. (2008). Dynamics and evolution of the inverted repeat-large single copy junctions in the chloroplast genomes of monocots. BMC Evol. Biol. 8 (1), 36. doi: 10.1186/1471-2148-8-36
Wicke, S., Schneeweiss, G. M., dePamphilis, C. W., Muller, K. F., Quandt, D. (2011). The evolution of the plastid chromosome in land plants: gene content, gene order, gene function. Plant Mol. Biol. 76 (3-5), 273–297. doi: 10.1007/s11103-011-9762-4
Wu, C. S., Chaw, S. M. (2014). Highly rearranged and size-variable chloroplast genomes in conifers II clade (cupressophytes): evolution towards shorter intergenic spacers. Plant Biotechnol. J. 12 (3), 344–353. doi: 10.1111/pbi.12141
Wu, C. S., Chaw, S. M. (2016). Large-scale comparative analysis reveals the mechanisms driving plastomic compaction, reduction, and inversions in conifers II (cupressophytes). Genome Biol. Evol. 8 (12), 3740–3750. doi: 10.1093/gbe/evw278
Wu, C. S., Lai, Y. T., Lin, C. P., Wang, Y. N., Chaw, S. M. (2009). Evolution of reduced and compact chloroplast genomes (cpDNAs) in gnetophytes: Selection toward a lower-cost strategy. Mol. Phylogenet. Evol. 52 (1), 115–124. doi: 10.1016/j.ympev.2008.12.026
Wu, C. S., Wang, Y. N., Hsu, C. Y., Lin, C. P., Chaw, S. M. (2011). Loss of different inverted repeat copies from the chloroplast genomes of Pinaceae and cupressophytes and influence of heterotachy on the evaluation of gymnosperm phylogeny. Genome Biol. Evol. 3, 1284–1295. doi: 10.1093/gbe/evr095
Xia, X., Xie, Z. (2001). DAMBE: software package for data analysis in molecular biology and evolution. J. Hered. 92 (4), 371–373. doi: 10.1093/jhered/92.4.371
Yi, X., Gao, L., Wang, B., Su, Y. J., Wang, T. (2013). The complete chloroplast genome sequence of Cephalotaxus oliveri (Cephalotaxaceae): evolutionary comparison of Cephalotaxus chloroplast DNAs and insights into the loss of inverted repeat copies in gymnosperms. Genome Biol. Evol. 5 (4), 688–698. doi: 10.1093/gbe/evt042
Zhang, Y., Ma, J., Yang, B., Li, R., Zhu, W., Sun, L., et al. (2014). The complete chloroplast genome sequence of Taxus chinensis var. mairei (Taxaceae): Loss of an inverted repeat region and comparative analysis with related species. Gene 540 (2), 201–209. doi: 10.1016/j.gene.2014.02.037
Zhang, X., Zhang, H. J., Landis, J. B., Deng, T., Meng, A. P., Sun, H., et al. (2019). Plastome phylogenomic analysis of Torreya (Taxaceae). J. Syst. Evol. 9999, 1–9. doi: 10.1111/jse.12482
Keywords: inversion, rearrangement, isomeric plastomes, inverted repeat, phylogenetics, yew
Citation: Zhang Y, Xu Y, Chen H, Wang L, Yin K and Du FK (2020) Comparative Genomic Analysis Reveals the Mechanism Driving the Diversification of Plastomic Structure in Taxaceae Species. Front. Genet. 10:1295. doi: 10.3389/fgene.2019.01295
Received: 07 July 2019; Accepted: 25 November 2019;
Published: 14 January 2020.
Edited by:
Denis Baurain, University of Liège, BelgiumReviewed by:
Daniel Pacheco Bruschi, Federal University of Paraná, BrazilQiang Fan, Sun Yat-sen University, China
Copyright © 2020 Zhang, Xu, Chen, Wang, Yin and Du. This is an open-access article distributed under the terms of the Creative Commons Attribution License (CC BY). The use, distribution or reproduction in other forums is permitted, provided the original author(s) and the copyright owner(s) are credited and that the original publication in this journal is cited, in accordance with accepted academic practice. No use, distribution or reproduction is permitted which does not comply with these terms.
*Correspondence: Fang K. Du, dufang325@bjfu.edu.cn