- Department of Medical Biotechnology, Institute of Biotechnology, Saveetha School of Engineering, Saveetha Institute of Medical and Technical Sciences, Chennai, Tamil Nadu, India
Introduction: Liver fibrosis is the abnormal accumulation of extracellular matrix and eventual formation of fibrous scar in response to chronic liver injury, which can be triggered by increased levels of reactive oxygen species. The brain-liver axis is a crucial communication pathway that significantly influences the intricate interactions between hepatic function and brain health. Selenium, as a source of selenoproteins, plays a vital role in antioxidant defense systems. The extraction of selenium from mussels leverages their natural bioaccumulation, providing a biocompatible source. Selenium nanoparticles are known for their potential antioxidant activity and can be employed to regulate ROS levels to overcome hepatic damage.
Methods: Selenium nanoparticles were synthesized from mussel-extracted selenium and stabilized with bovine serum albumin. The zebrafish models exposed to copper sulfate were treated with selenium nanoparticles (5-25 μg/ml). This study evaluated their potential role as antioxidants against hepatic damage induced by copper sulfate in vivo in the zebrafish model.
Results: The bovine serum albumin stabilized selenium nanoparticles reduced for 30 minutes and 1 hour were spherical with a size of 19 and 16 nm. Stabilized selenium nanoparticles reduced for 30 minutes (25 μg/ml) showed significant in vitro reactive oxygen species scavenging activity and improved in vivo antioxidant enzyme levels by decreasing lipid peroxidation and nitric oxide levels. Histopathological examination revealed a delay in the progression of copper sulfate-induced hepatic damage, and upregulated the expression of antioxidants, while the hepatic and mitochondrial damage markers were downregulated.
Conclusion: In conclusion, bovine serum albumin-reduced selenium nanoparticles can be a promising therapeutic antioxidant for protecting against reactive oxygen species-induced hepatic damage and neurodegeneration.
Highlights
• Green-synthesized and stabilized nano-selenium is a potential therapeutic agent for controlling Cu-induced ROS, which can lead to neurodegeneration.
• In zebrafish, nano-Se ultimately reduces Cu-induced hepatic damage by maintaining the integrity of liver cells.
• 25 μg/mL Se-NPs are potential in vitro ROS scavengers. They increase SOD, CAT, GSH, and GPX levels while reducing the levels of LPO and NO in treated zebrafish.
• Se-NPs show promise as neuroprotective agents for restoring AChE levels and cognitive functions in zebrafish
1 Introduction
Liver fibrosis is a pathological condition characterized by excessive accumulation of extracellular matrix proteins, including collagen, that results from chronic liver injury. It represents a wound-healing response to liver damage, where the normal liver tissue is replaced by scar tissue (Bataller and Brenner, 2005; Addissouky et al., 2024). This fibrotic process occurs in response to various factors such as viral hepatitis (hepatitis B and C), chronic alcohol abuse, non-alcoholic fatty liver disease, and autoimmune diseases (Athuluri-Divakar and Hoshida, 2019). Recent studies suggest that liver health extends beyond its functions, influencing the brain through the brain–liver axis. This communication network is crucial for maintaining metabolic balance (Vegas-Suárez et al., 2022; De Cól et al., 2024). The liver is a vital organ with a remarkable capacity to regenerate (Qian et al., 2022). However, when repeated or persistent injury occurs, the balance between tissue repair and destruction is disrupted (Schilrreff and Alexiev, 2022). This triggers the activation of hepatic stellate cells, the main cell type responsible for fibrogenesis, leading to the deposition of fibrous scar tissue (Chen et al., 2024). Over time, the accumulation of scar tissue impairs liver function and architecture (Luangmonkong et al., 2018). A key factor in its development is the overproduction of reactive oxygen species (ROS). Exposure to copper sulfate (CuSO4) is known to induce liver fibrosis by generating excessive ROS and causing oxidative stress (Seffner et al., 1997; Wang et al., 2016). Oxidative stress, caused by an imbalance between the production of ROS and the body’s ability to detoxify these reactive molecules, is a major contributing factor to various diseases, including liver fibrosis and neurodegenerative disorders (Chen et al., 2020).
With growing interest in sustainable and biocompatible nanomaterials, research is increasingly focused on optimizing nanoparticle synthesis, improving biocompatibility, and understanding their interactions within biological systems (Ranjha et al., 2022). Nanoparticles can be synthesized through diverse methods, such as environmentally friendly green synthesis and physical and conventional chemical techniques (Ijaz et al., 2020; Osman et al., 2024). This versatility enables innovative solutions in fields such as medicine, environmental remediation, energy, and materials science, contributing to advancements (Sen et al., 2024). Among the various nanomaterials, selenium nanoparticles (Se-NPs) have gained significant attention due to their distinctive characteristics and potential applications in multiple fields, particularly in biomedicine (Karthik et al., 2024; Umapathy et al., 2024).
Selenium is an essential micronutrient that is crucial in maintaining cellular health, primarily due to its involvement in the body’s antioxidant defense systems (Bjørklund et al., 2022). It is a key component of selenoproteins, which are enzymes that help neutralize the effects of harmful ROS and protect cells from damage caused by oxidative stress (Jomova et al., 2023). By reducing ROS, selenium helps protect cells from damage and prevent the progression of these conditions (Zhang F. et al., 2023). Mussels are particularly valuable as a natural source of selenium due to their ability of bioaccumulating this element from their environment. Through a process known as bioaccumulation, mussels concentrate selenium in their tissues, making them a rich and sustainable source of this vital micronutrient (Kristan et al., 2015; Abderrahmani et al., 2021; Dey et al., 2024).
However, when selenium is reduced to its nanoparticle form, its surface area increases, allowing for more efficient interaction with ROS and other reactive molecules. This nano-formulation significantly enhances the antioxidant capacity of Se-NPs to mitigate oxidative stress by scavenging ROS more effectively than selenium alone (Zhao et al., 2018; Bisht et al., 2022). This ability to modulate biological pathways and enhance the immune response has opened new avenues for treating conditions such as neurodegenerative diseases, liver disorders, and cancer (Khurana et al., 2019; Chen et al., 2022). This study focuses on exploring the therapeutic potential of Se-NPs reduced with bovine serum albumin (BSA) for their efficacy in protecting against hepatic damage and neurodegeneration caused by exposure to CuSO4 in zebrafish.
2 Materials and methods
2.1 Extraction of selenium from mussels
2.1.1 Collection and preparation of mussel
Mussels (domain: Eukaryota; kingdom: Animalia; phylum: Mollusca; class: Bivalvia; order: Mytilida; family: Mytilidae; genus: Perna; species: viridis) were freshly collected whole from Kasimedu Fishing Harbor, Tondiarpet (N 13° 7′22.4292″, E 80° 17′36.4272″), Chennai. Sand and other detritus were thoroughly cleaned out of the mussels. After cracking open the shells, the tissue was carefully scraped and cleaned with distilled water. Excess water in the collected tissue was removed and dried overnight. The dried mussel tissue was then stored at 4°C for use in further experiments.
2.1.2 Extraction of selenium
2.1.2.1 Extraction with 0.8% saline
A saline solution (0.8%) was prepared by dissolving 8 g of sodium chloride (NaCl) in 1,000 mL of distilled water. Using a mortar and pestle, 8.5 g of mussel tissue was ground along with 100 mL of the 0.8% saline solution. The mixture was centrifuged at room temperature (37°C) for 30 min at 5,000 rpm until no particulates were visible. The collected pellet was dried in a hot-air oven at 60°C and then pulverized with a mortar and pestle (Kristan et al., 2015).
2.1.2.2 Extraction with 50 mM Tris–HCl buffer pH 7.4
Fresh Tris–HCl buffer (pH 7.4) was prepared by dissolving 4.44 g of HCl and 2.65 g of Tris base in 1,000 mL of distilled water. An amount of 8.5 g of the mussel tissue was mashed with 100 mL of the Tris–HCl buffer using a mortar and pestle. Fine selenium powder was obtained following the outlined procedure for selenium purification (Kristan et al., 2015).
2.2 Synthesis of Se-NPs
Se-NPs were synthesized by reducing and stabilizing mussel-derived selenium using BSA. In summary, 0.2 g of mussel-derived selenium was dissolved in 50 mL of distilled water using a sonicator. In a round-bottom flask, 50 mL of the dissolved mussel-derived selenium was mixed with 25 mL of 1% BSA. The process was carried out at two different intervals of 30 min and 1 h, both without and with a stabilizer (5% BSA) using a heating mantle. The mixture was centrifuged at 5,000 rpm for 30 min at 37°C. The collected pellets were dried at 60°C in a hot-air oven. Once completely dried, the pellets were ground into a fine powder using a mortar and pestle (Nie et al., 2016; Salem et al., 2022). These synthesized Se-NPs were utilized for further experiments.
2.3 Characterization of mussel-extracted selenium and Se-NPs
The Se-NPs were extensively analyzed using various analytical methods. PerkinElmer Optima 5300 DV Inductively Coupled Plasma Optical Emission Spectroscopy (ICP-OES) was utilized to determine trace element concentrations (Machat et al., 2002). A Bruker D8 Advance Powder XRD was employed for X-ray diffraction (XRD) and crystallographic investigations within a range of 10 and 80 degrees of 2θ (Ramamurthy et al., 2013). The presence of functional groups was examined using Fourier-transform infrared (FTIR) spectroscopy. Thermo Fisher Scientific’s Nicolet Summit FTIR instrument, operated in the diffuse reflectance mode, was used for this analysis. A total of 16 scans covering a wavenumber range of 400 to 4,000 cm−1 were obtained using the DTGS KBr detectors (Ramamurthy et al., 2013). The obtained spectra were plotted with the wavenumber (cm−1) on the X-axis and transmittance (%) on the Y-axis using OriginPro 8.5 Software. Zeta potential was determined using the nanoPartica SZ-100V2 (Horiba) (Ramamurthy et al., 2013). To analyze the surface morphology of the Se-NPs, a Hitachi Model S-3400 N scanning electron microscope (SEM) was used. The SEM was operated with semiconductor secondary electron (SE) detection in the high vacuum mode at 15 kV. The obtained images were further analyzed for size distribution using ImageJ software (Ramamurthy et al., 2013).
2.4 In vitro antioxidant studies
Utilizing procedures described in earlier research (Dumore and Mukhopadhyay, 2020; Issac and Velumani, 2024), DPPH and ABTS experiments were carried out to assess the in vitro antioxidant capacity of stabilized Se-NPs. Stock solutions containing 50 µM ascorbic acid and 1 mg/mL of Se-NPs were prepared, from which working solutions of 5, 10, 15, 20, and 25 μg/mL were derived. These concentrations were fixed based on previously reported studies (Kora, 2018). The entire investigation was conducted using the mentioned doses.
2.4.1 DPPH assay
In a 96-well ELISA plate, 50 µM ascorbic acid (CAS: 50-81-7; SRL) and varying doses of stabilized Se-NPs (5, 10, 15, 20, and 25 μg/mL) were added to 300 µM DPPH solution (CAS: 1898-66-4; SRL). The plates were then left in the dark for half an hour. A Thermo Fisher Scientific® microplate reader measured the absorbance at 517 nm.
2.4.2 ABTS assay
A reaction mixture containing 7 mM ABTS salt (CAS: 30931-67-0; SRL) and 2.45 mM potassium persulfate (1:1) was left to stand at room temperature in the dark for a day. The ABTS solution was diluted with 20X PBS to achieve an absorbance of 0.7 ± 2 at 734 nm. As a positive control, 50 µM ascorbic acid was employed. Stabilized Se-NPs at concentrations of 5, 10, 15, 20, and 25 μg/mL were used. The prepared mixtures were added to a 96-well ELISA plate containing ABTS solution and incubated for 60 min in the dark at room temperature. Using a Thermo Fisher Scientific® microplate reader, the samples were measured at 734 nm.
2.5 In vivo developmental toxicity studies
2.5.1 Zebrafish maintenance and embryo collection
Adult male and female zebrafish were obtained from Tarun Fish Farm in Manimangalam (latitude N 12° 55′1″ and longitude E 80° 2′29″), Chennai. Following Institutional Ethical Committee Guidelines (SU/CLAR/RD/001/2023), adult zebrafish were housed in a 19-L glass tank at 28.5°C with a 14-/10-h light/dark cycle and fed live Artemia salina (brine shrimp) three times daily. Breeding was initiated after 20 days of acclimatization, with two breeding groups placed in a spawning tank with a ratio of 1:1 (male: female). To prevent the female fish from swallowing the eggs, a mesh was placed at the bottom of the spawning tank. Embryos were collected 30 min after the onset of light, rinsed with freshly prepared E3 medium (Williams and Renquist, 2016), and kept at 26°C ± 1°C until the experiment (OECD, 2013; OECD, 2019).
2.5.2 In vivo developmental toxicity test
Four hours post-fertilization (hpf), zebrafish embryos were placed in a 12-well plate, with 10 embryos (n = 30/group) per well. The control group embryos were left untreated, while the stress group embryos were exposed to CuSO4 (20 µM) (Nguyen et al., 2020; Herrera et al., 2021; Gao et al., 2023; Zhang P. et al., 2023), and the treatment group embryos were exposed to CuSO4 and treated with five different concentrations of stabilized Se-NPs (5–25 μg/mL) every 24 h. These concentrations were fixed based on previously reported studies (Kalishwaralal et al., 2015; 2016; Gao et al.,2023) and were consistently utilized throughout the study. The experiment was conducted in triplicate. Zebrafish embryo development was monitored under a microscope with 4X magnification (Issac et al., 2021; Wang et al., 2021).
2.6 Estimation of ROS levels in zebrafish larvae
Tricaine was used to anesthetize the larvae in each group (n = 6 per group), including the control, stress, and stabilized Se-NP treatment groups. The larvae were washed using E3 medium stained with DCFDA (20 μg/mL) dye and incubated in the dark for 1 h at room temperature. The stained larvae were washed, mounted on the clean glass, and observed visually using a fluorescent microscope (CKX53 Microscope, Japan). ImageJ software was utilized for analysis with parameters of image type: 8 bit and dark background, and the region of interest was measured with the rectangle tool (Sudhakaran et al., 2023).
2.7 Estimation of LPO levels in zebrafish larvae
Tricaine was used to anesthetize the larvae in each group (n = 6 per group), including the control, stress, and stabilized Se-NP treatment groups. The larvae were washed using the E3 medium and stained with the DPPP (25 μg/mL) dye and incubated at room temperature for 30 min. The stained larvae were washed, mounted on the clean glass, and visualized under a fluorescent microscope (CKX53 Microscope, Japan). ImageJ software was used for analysis with parameters of image type: 8 bit and dark background, and the region of interest was measured with the rectangle tool (Sudhakaran et al., 2023).
2.8 In vivo antioxidant studies
For enzymatic analysis, CuSO4-exposed larvae (n = 20/group) and adult zebrafish (n = 2/group) treated with stabilized Se-NPs (5–25 μg/mL) were homogenized in a solution containing 100 mM Tris–HCl buffer (pH 7.8 at 4°C) with 150 mM potassium chloride and 1 mM EDTA at 96 hpf. After centrifugation for 15 min at 10,000 rpm, the supernatant was utilized for further enzymatic examination (Lite et al., 2022). Protein estimation was performed using Bradford’s technique (Bradford, 1976). All experiments were conducted in triplicate.
2.8.1 Superoxide dismutase (SOD) assay
An amount of 50 µL of the homogenate was mixed with a reaction mixture containing 50 mM phosphate buffer (pH 7.8), 100 µM EDTA, 750 μM NBT, 130 mM methionine, and 20 µM riboflavin. The mixture was exposed to light for 20 min, and the absorbance was measured at 560 nm (Issac et al., 2021).
2.8.2 Catalase (CAT) assay
An amount of 50 µL of the sample was mixed with 100 µL of buffered H2O2 to measure catalase activity. Absorbance was recorded at 240 nm for using spectrophotometry for 2 min with 15-second intervals. This assay was conducted based on a previously published study (Velayutham et al., 2021).
2.8.3 Lipid peroxidation (LPO) assay
MDA levels were measured using the thiobarbituric acid method (Velayutham et al., 2021). An amount of 100 µL of homogenized larvae and adult zebrafish sample was treated with 0.1 mL of 5% trichloroacetic acid and was incubated on ice for 15 min. Then, 0.2 mL of 0.67% thiobarbituric acid was added and incubated at 100°C in a water bath for 30 min. After chilling on ice for 20 min, the sample was centrifuged at 2,000 rpm (4°C) for 10 min, and the absorbance was measured at 535 nm (Issac et al., 2024).
2.8.4 Nitric oxide (NO) assay
NO levels were measured using the Griess technique with slight modifications (Schulz et al., 1999). An amount of 100 µL of homogenized larval and adult zebrafish sample was mixed with 100 µL of Griess reagent and left at room temperature for 25 min. The absorbance was read at 540 nm.
2.8.5 Reduced glutathione (GSH) and glutathione S-transferase (GST) assay
GSH and GST levels were assessed following a previously described method (Issac et al., 2021), with slight adjustments. An amount of 100 μL larval and adult zebrafish sample was combined with 50 μL of 20 mM DTNB and 150 μL of 100 mM potassium phosphate buffer at pH 7.4, and the absorbance was measured at 412 nm. For GST evaluation, 100 μL of the reaction mixture containing 10 μM GSH and 60 μM 1-chloro 2,4-dinitrobenzene was mixed with 50 μL of the larval and adult zebrafish sample, and the absorbance was noted at 340 nm.
2.8.6 Estimation of acetylcholinesterase (AChE)
After exposure to CuSO4 and treatment with stabilized Se-NPs, samples were analyzed for AChE levels. DTNB (3.3 mM) was added to homogenized samples and incubated for 20 min, followed by the addition of acetylcholine iodide. The absorbance was measured at 412 nm at 1-minute intervals (Li et al., 2018; Issac and Velumani, 2024).
2.9 Cognitive behavior analysis
The locomotor abnormalities of zebrafish larvae were assessed by examining their swimming behavior pattern (Krishnan and Kang, 2019). Each exposure group (n = 3 larvae per well) was allowed to acclimatize for 10 min in a white chambered ice tray (2.5 × 3.5 cm) with 2 mL of the E3 medium without methylene blue 7 days post-fertilization. The movement of the larvae was recorded by using a commercial smartphone for 60-second at 60 frames per second in a noise-free environment at the beginning of the light cycle. Locomotion was plotted using UMATracker software (Yamanaka and Takeuchi, 2018).
2.10 Establishment of liver fibrosis in adult zebrafish
Healthy adult zebrafish were selected, divided into groups (n = 10/group), and placed in individual tanks—control group, CuSO4 group (20 μM), and CuSO4 + Se-NPs group (25 μg/mL)—following a 21-day exposure period. The 21-day exposure period was selected to ensure the development of chronic hepatic damage and fibrosis induced by CuSO4 exposure. This exposure duration was chosen in relevance to the previously reported studies (Li et al., 2023; Zhang J. et al., 2023). Following the Institutional Ethical Committee Guidelines (SU/CLAR/RD/001/2023), the fish were housed in a 19-L glass tank at 28.5°C with a 14-/10-hour light/dark cycle. They were fed live Artemia salina (brine shrimp) three times a day and had half of their water replaced with fresh dechlorinated water every 2 days. Three replicates were conducted for each treatment. Antioxidant enzymatic levels in adult zebrafish were evaluated through enzymatic analysis as per the protocol mentioned earlier.
2.11 Histological staining
Adult zebrafish (n = 2/group/week) were euthanized using tricaine and then immersed in fixative (4% paraformaldehyde) at room temperature for 24 to 48 h. The fixed zebrafish were dehydrated in ethanol, transferred to xylene for 1 h to remove excess ethanol, and then immersed in melted paraffin wax at 58°C–60°C overnight. The paraffin-infiltrated tissues were then transferred to molds to solidify. The prepared paraffin blocks were sectioned using a microtome, and the wax sections were flattened on glass slides by floating them on warm water. The hematoxylin and eosin (H&E) staining method was used, where tissue sections were stained in hematoxylin for 5–10 min, rinsed in water, washed in acid alcohol, stained in eosin for 2 min, dehydrated in ethanol, and cleared with xylene. The slides were then observed under a bright-field microscope (CKX53 Microscope, Japan) (Arman, 2021).
2.12 Antioxidant gene expression by real-time polymerase chain reaction (RT-PCR)
RNA was isolated from the homogenates of adult zebrafish (n = 2/group) and larvae (n = 20/group) using RDP Trio™ Reagent (SKU: MB566; HiMedia). Primers for fibrotic genes, DNA damage, and antioxidant enzymes were designed using NCBI’s Primer-BLAST (see Table 1). Gene expression was analyzed with the help of the 2x One-Step RT-PCR Master Mix (ABT018; AURA Biotech). The reverse transcription process started with a single cycle at a temperature ranging between 44°C and 50°C lasting for 15 min, followed by gene activation at 95°C for 3 min. Denaturation was repeated for 40 cycles at 95°C for 10 s. Annealing was carried out at 60°C for 45 s, and the extension process was carried out at 72°C for 15 s. The housekeeping gene β-actin is a reference gene used for normalization as it is stably expressed in most cell types. Normalization against β-actin reduces the variability in the expression levels of target genes (Santhi et al., 2025). The 2−ΔΔCT method was utilized to calculate the fold change (Issac and Velumani, 2024). The analysis was conducted using Applied Biosystems StepOnePlus™.
2.13 Statistical analysis
All experiments in this study were conducted in triplicate and are represented as mean ± standard deviation (SD). The data were analyzed using one-way analysis of variance (ANOVA) and Dunnett’s multiple comparison test in GraphPad Prism 5.0 (GraphPad Software, Inc., San Diego, CA) (Qamar et al., 2020). The data were denoted by the symbol “*” and were considered significant with p < 0.05.
3 Results
3.1 Synthesis and characterization of selenium extracted from mussels
Saline (0.8%) and Tris–HCl buffer extraction methods were used to determine which is preferable for extracting selenium from mussels. ICP-OES results showed a distinct peak at 196.026 nm with concentrations of 0.927 mg/L for the saline extraction method (Figure 1A) and 0.509 mg/L for the buffer extraction method (Figure 1B). XRD results for selenium extracted using the saline method showed peaks at angles of 26.5076°, 31.5757°, 45.3321°, 56.3082°, 66.1258°, and 75.1616°, which correspond to the planes (100), (110), (111), (112), (210), and (113), respectively (Figure 1D). In contrast, the buffer method of extracting selenium revealed peaks at 22.1635° and 26.7682°, corresponding to planes (100) and (110), respectively (Figure 1E).
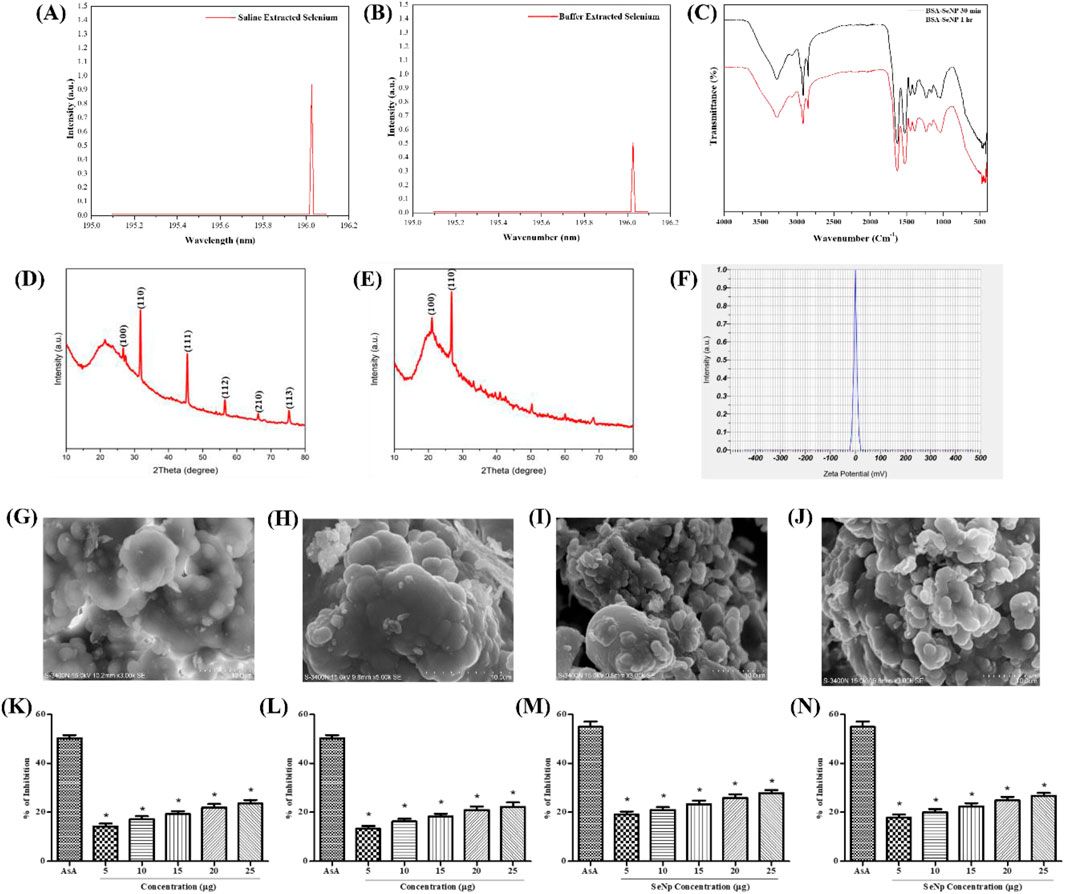
Figure 1. Synthesis and characterization of Se and Se-NPs: x-ray diffraction analysis of mussel-extracted selenium: (A) saline extraction; (B) buffer extraction; FTIR spectra of (C) stabilized Se-NPs reduced for 30 min and 1 h with BSA; ICP-OES of selenium extracted from mussel concentration of (D) saline extracted selenium; (E) buffer extracted selenium. Zeta potential of (F) stabilized Se-NPs reduced for 30 min with BSA. Scanning electron microscope imaging at 5x10-3 magnification: (G) non-stabilized Se-NPs reduced for 30 min; (H) non-stabilized Se-NPs reduced for 1 h; (I) stabilized Se-NPs reduced for 30 min; (J) stabilized Se-NPs reduced for 1 h with BSA. In vitro antioxidant activity: DPPH assay of (K) stabilized Se-NPs reduced with BSA for 30 min; (L) stabilized Se-NPs reduced with BSA for 1 h. ABTS assay of (M) stabilized Se-NPs reduced with BSA for 30 min; (N) stabilized Se-NPs reduced with BSA for 1 h. The data were considered significant (p < 0.05) and marked by the symbol “*.”
3.2 Synthesis and characterization of Se-NPs extracted from mussels
Se-NPs were synthesized using BSA as a reducing agent for two different periods (30 min and 1 h) and were stabilized to control aggregation. The chemical properties of the stabilized Se-NPs were detected using FTIR. The smooth and sharp peaks observed were at 3,277.830–3,274.074 cm−1 (medium, sharp C–H stretching alkene), 2,919.169–2,851.293 cm−1 (medium, sharp C–H stretching alkane), 1,628.229–1,628.660 cm−1 (medium, C=C stretching di-substituted alkene), 1,530.819–1,530.101 cm-1 (strong, N–O stretching nitro-compound), 1,454.547–1,454.339 cm−1 (strong, S=O stretching sulfonyl chloride), 1,396.690–1,234.989 cm−1 (strong, C–O stretching alkyl aryl ether), 1,042.220–1,039.471 cm−1 (strong, S=O stretching sulfoxide), and 471.609–409.849 cm−1 (strong, metal–ligand stretching) for stabilized Se-NPs reduced for both 30 min and 1 h with BSA (Figure 1C). The obtained peaks indicated the presence of metal and the reducing agent used. This also confirmed that the reduction process and duration did not alter the functional groups of the nanoparticles. Zeta potential was measured at 0.0 mV, indicating that the particles which tend to aggregate have a very low surface charge or are nearly neutral. The sharp peak implies a uniform distribution of zeta potential values (Figure 1F).
The synthesized Se-NPs showed spherical morphology. Lesser aggregation was observed in stabilized nanoparticles, compared to non-stabilized Se-NPs. The size of the non-stabilized Se-NPs was observed to be 39 and 26 nm when reduced for 30 min and 1 h, respectively (Figures 1G,H). In contrast, the size of the stabilized Se-NPs was observed to be 19 and 16 nm, showing that stabilization altered the size of the nanoparticle (Figures 1I,J). Based on the variation in size and aggregate reduction, BSA played a crucial role in enhancing the size of the nanoparticles.
3.3 In vitro antioxidant analysis of stabilized Se-NPs
3.3.1 DPPH scavenging assay
The scavenging activity of ROS was assessed using the DPPH scavenging test. Ascorbic acid (AsA) showed 50% scavenging activity as a positive control. The DPPH scavenging activity of stabilized Se-NPs reduced with BSA for 30 min and 1 h was concentration-dependent. At a dose of 25 μg/mL, the highest scavenging activity was observed with 23.60% and 22.28% inhibition (Figures 1K,L).
3.3.2 ABTS scavenging assay
The ROS scavenging activity was measured using the ABTS scavenging test. As a positive control, AsA exhibited 55% scavenging activity. The scavenging activity of all stabilized Se-NPs reduced with BSA for 30 min and 1 h was concentration-dependent. At a concentration of 25 μg/mL, the maximum activity was recorded with percentages of 27.79% and 24.88% (Figures 1M,N). Therefore, stabilized Se-NPs proved to be effective ROS scavengers.
3.4 Developmental toxicity assessment
The developmental toxicity assessment of Se-NPs was conducted using zebrafish embryos. In the control group, no abnormal morphological changes were observed. Groups treated with stabilized Se-NPs reduced with BSA for 30 min and 1 h (5–25 μg/mL) for 0–72 hpf showed no malformation compared to the CuSO4-exposed group, which exhibited malformations such as yolk-sac edema (YSE) and pericardial edema (PCE) (Figures 2A,B). In comparison to the CuSO4-exposed group, stabilized Se-NPs reduced with BSA for 30 min and 1 h showed a lower mortality rate at 96 hpf (Figures 2C,D).
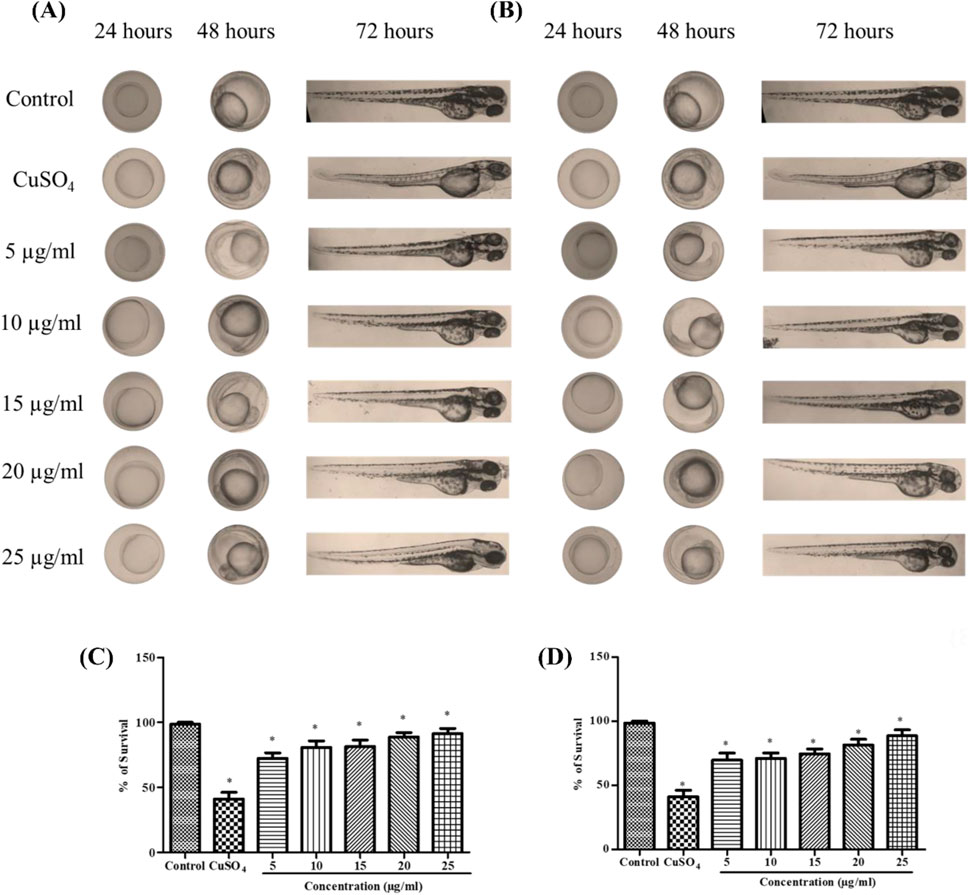
Figure 2. In vivo developmental toxicity analysis in zebrafish embryos and larvae representing the control group, stress group (CuSO4-induced stress), and stabilized Se-NP (5–25 μg/mL) treatment group. (A) Stabilized Se-NPs reduced with BSA for 30 min; (B) stabilized Se-NPs reduced with BSA for 1 h. Percentage of survival: (C) stabilized Se-NPs reduced with BSA for 30 min; (D) stabilized Se-NPs reduced with BSA for 1 h. The data were considered significant (p < 0.05) and marked by the symbol “*.”
3.5 Localization of cellular ROS
Using DCFDA fluorescent labeling, zebrafish larvae (96 hpf) were assessed for intracellular ROS. The mean fluorescence intensity (MFI) for the control group was 9.23. ROS levels in zebrafish larvae exposed to CuSO4 were 60.72. In CuSO4-induced zebrafish larvae, treatment with stabilized Se-NPs significantly (p < 0.05) decreased cellular ROS levels compared to that in the untreated stress group (Figures 3A,B; Supplementary Figure S1). Lower ROS levels were observed at concentrations of 12.60 and 17.97 MFI in the 25 μg/mL stabilized Se-NPs treated with BSA for 30 min and 1 h (Figures 3C,D).
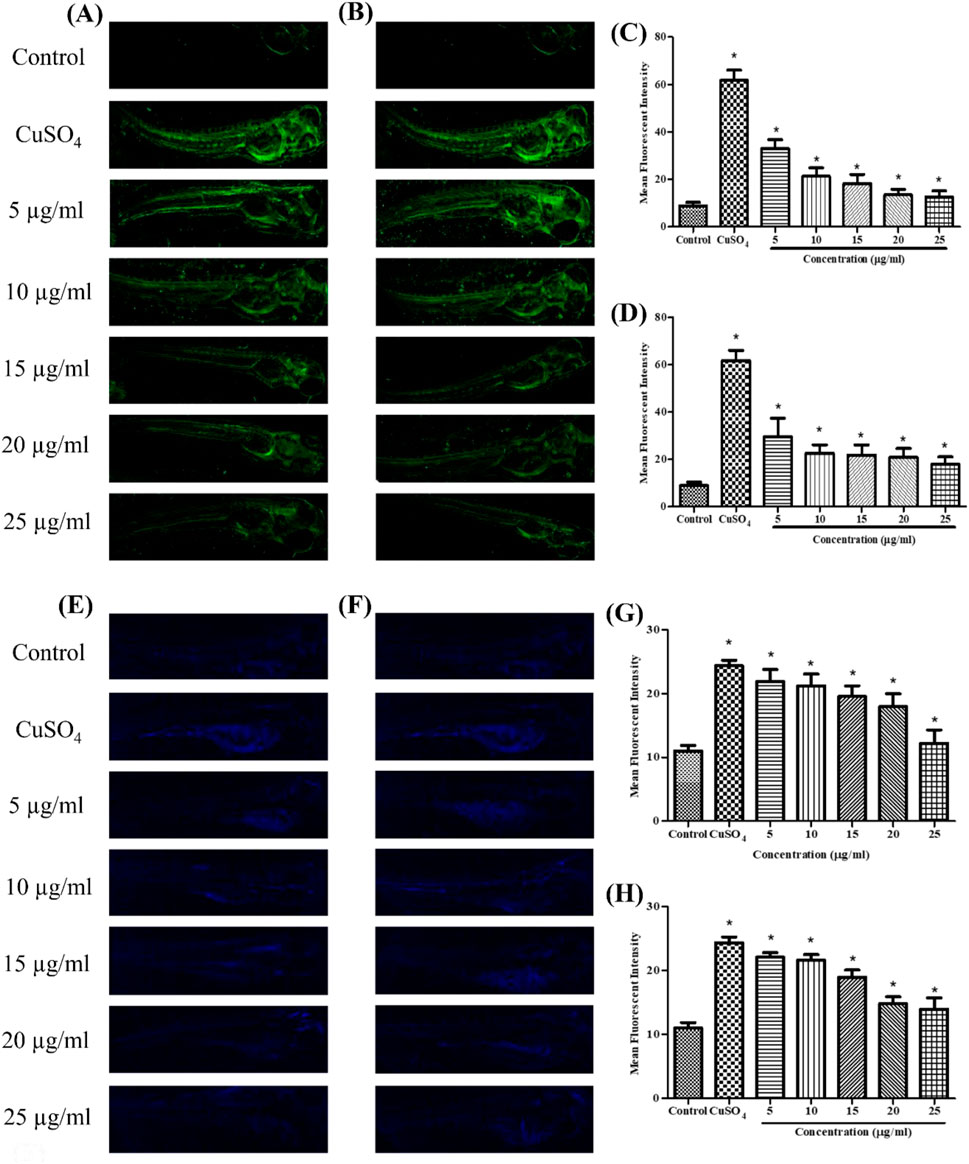
Figure 3. Live cell imaging: in vivo DCFDA and DPPP staining in the zebrafish larvae model at 96 hpf. DCFDA staining: (A) stabilized Se-NPs reduced with BSA for 30 min; (B) stabilized Se-NPs reduced with BSA for 1 h. Mean fluorescent intensity: (C) stabilized Se-NPs reduced with BSA for 30 min; (D) stabilized Se-NPs reduced with BSA for 1 h. DPPP staining: (E) stabilized Se-NPs reduced with BSA for 30 min; (F) stabilized Se-NPs reduced with BSA for 1 h. Mean fluorescent intensity: (G) stabilized Se-NPs reduced with BSA for 30 min; (H) stabilized Se-NPs reduced with BSA for 1 h. The data were considered significant (p < 0.05) and marked by the symbol “*.”
3.6 Determination of live cell lipid peroxidation
Using DPPP fluorescent staining, zebrafish larvae (96 hpf) were observed for LPO. The control group showed 11.02 MFI. ROS levels in zebrafish larvae exposed to CuSO4 were 24.35 MFI. In CuSO4-exposed zebrafish larvae, treatment with stabilized Se-NPs significantly decreased the LPO compared to that in the untreated stress group (Figures 3E,F; Supplementary Figure S2). Stabilized Se-NPs at the 25 μg/mL concentration reduced the LPO to 11.35 and 13.17 MFI (Figures 3G,H).
3.7 In vivo enzymatic assay of stabilized Se-NPs
3.7.1 SOD assay
A homogenized sample of zebrafish larvae treated with stabilized Se-NPs after exposure to CuSO4 was used to measure SOD levels. Comparing the CuSO4-exposed group to the control group (16.90 U/mg of protein), the SOD levels were observed to drop significantly to 7.33 U/mg of protein (a 57% reduction). The concentration-dependent rise in enzymatic activity was demonstrated by 25 μg/mL stabilized Se-NPs, which was reduced with BSA for 30 min and 1 h, restoring the enzymatic levels to 13.65 U/mg of protein and 11.97 U/mg of protein, respectively (Figures 4A,B).
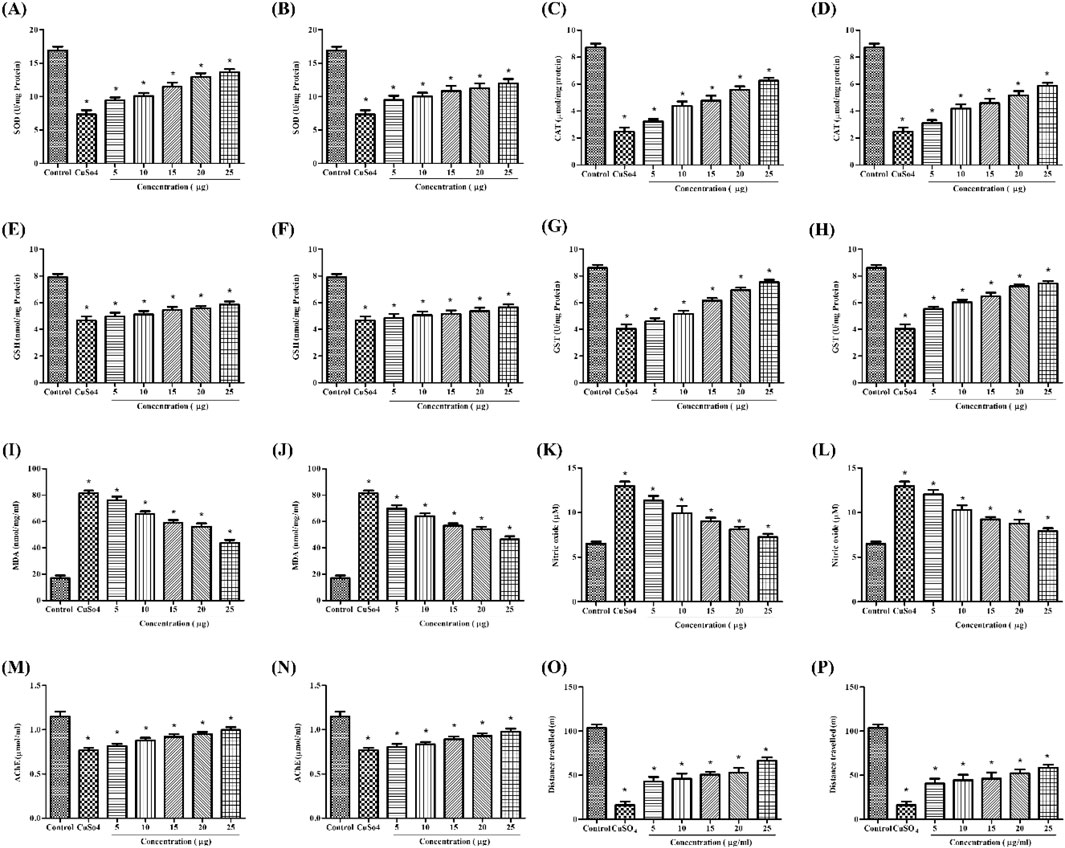
Figure 4. In vivo antioxidant activity: SOD assay of (A) stabilized Se-NPs reduced with BSA for 30 min and (B) stabilized Se-NPs reduced with BSA for 1 h. CAT assay of (C) stabilized Se-NPs reduced with BSA for 30 min and (D) stabilized Se-NPs reduced with BSA for 1 h. GSH assay of (E) stabilized Se-NPs reduced with BSA for 30 min and (F) stabilized Se-NPs reduced with BSA for 1 h. GST assay of (G) stabilized Se-NPs reduced with BSA for 30 min and (H) stabilized Se-NPs reduced with BSA for 1 h. LPO assay of (I) stabilized Se-NPs reduced with BSA for 30 minand (J) stabilized Se-NPs reduced with BSA for 1 h. NO assay of (K) stabilized Se-NPs reduced with BSA for 30 min and (L) stabilized Se-NPs reduced with BSA for 1 h. AChE assay of (M) stabilized Se-NPs reduced with BSA for 30 min and (N) stabilized Se-NPs reduced with BSA for 1 h. Locomotory behavior of (O) stabilized Se-NPs reduced with BSA for 30 min and (P) stabilized Se-NPs reduced with BSA for 1 h. The data were considered significant (p < 0.05) and marked by the symbol “*”.
3.7.2 CAT assay
A homogenized sample of zebrafish larvae treated with stabilized Se-NPs after exposure to CuSO4 was used to measure CAT levels. CAT levels were significantly lower in the CuSO4-exposed group (2.49 μmol/mg of protein) than in the control group (8.72 μmol/mg of protein). CuSO4-exposed zebrafish larvae treated with stabilized Se-NPs reduced with BSA for 30 min and 1 h at the concentration of 25 μg/mL showed concentration-dependent increases in total CAT levels of 6.25 μmol/mg and 5.86 μmol/mg, respectively (Figures 4C,D).
3.7.3 Estimation of GSH activity
GSH activity was significantly lower in the CuSO4-exposed group (4.67 nmol/mg protein) than in the control group (7.89 nmol/mg protein). In contrast, CuSO4-exposed zebrafish larvae treated with 25 μg/mL stabilized Se-NPs reduced with BSA for 30 min and 1 h greatly improved the GSH activity. As seen in Figures 4E,F, the GSH levels retained were 5.85 and 5.64 nmol/mg of protein, respectively.
3.7.4 Estimation of the GST activity
In comparison to the control group (8.59 U/mg protein), the CuSO4-exposed group exhibited a substantial decrease in GST activity (4.04 U/mg protein). After treatment with stabilized Se-NPs reduced with BSA (25 μg/mL) for 30 min and 1 h, GST activity increased markedly to 7.52 and 7.41 U/mg of protein, respectively (Figures 4G,H).
3.7.5 Lipid peroxidation assay
MDA levels were significantly higher in the CuSO4-exposed stress group (81.69 nmol/mg/mL) than in the control group (17.09 nmol/mg/mL). In zebrafish larvae exposed to CuSO4, stabilized Se-NPs reduced with BSA for 30 min and 1 h at a dose of 25 μg/mL considerably decreased the MDA levels by 43.77 and 46.49 nmol/mg/mL, respectively (Figures 4I,J).
3.7.6 Estimation of NO levels
The Griess reagent assay was used to measure NO levels. Zebrafish larvae exposed to CuSO4 exhibited higher NO levels (12.9 µM) than those in the control group (6.49 µM). NO levels decreased dose-dependently, and a significant decline was observed with 25 μg/mL stabilized Se-NPs reduced with BSA for 30 min and 1 h, showing values of 7.27 µM and 7.93 µM, respectively (Figures 4K,L).
3.7.7 Estimation of AChE activity
Zebrafish larvae exposed to CuSO4 demonstrated a considerable drop in AChE levels, from 1.14 μmol/mL to 0.76 μmol/mL, compared to the control group. When CuSO4-exposed zebrafish larvae were treated with 25 μg/mL stable Se-NPs decreased with BSA for 30 min and 1 h, respectively, AChE levels increased in a dose-dependent manner to reach 1.04 and 0.97 μmol/mL, respectively (Figures 4M,N).
3.8 Estimation of locomotor activity
The neurobehavior of zebrafish larvae was assessed by measuring the distance they traveled (in meters), allowing us to determine their locomotor activity and cognitive changes in the central nervous system. Zebrafish larvae exposed to CuSO4 traveled 16.2 m, likely due to stress effects. In the CuSO4-exposed zebrafish larval group, treatment with 25 μg/mL of stabilized Se-NPs reduced with BSA for 30 min and 1 h enhanced cognitive behavior by restoring their locomotor activity, thus covering distances up to 66.26 m and 58.57 m. The control group traveled 102.60 m, demonstrating normal cognitive behavior (Figures 4O,P).
3.9 Histopathology analysis
The histopathology of adult zebrafish liver was analyzed to determine morphological alterations. In control zebrafish, hepatocytes and nuclei were observed (Figures 5A–C). Liver histology of zebrafish exposed to CuSO4 distinctly showed the formation of vacuoles on day 7, dilation in the sinusoidal area, and hepatocyte degeneration on days 14 and 21, with noticeable alterations (Figures 5D–G). However, the treatment group showed damage in liver morphology such as vacuolation on day 14 and sinusoidal dilation on day 21, but the progression was observed to be delayed (Figures 5H–J). Degeneration of hepatocytes was not observed in the treatment group.
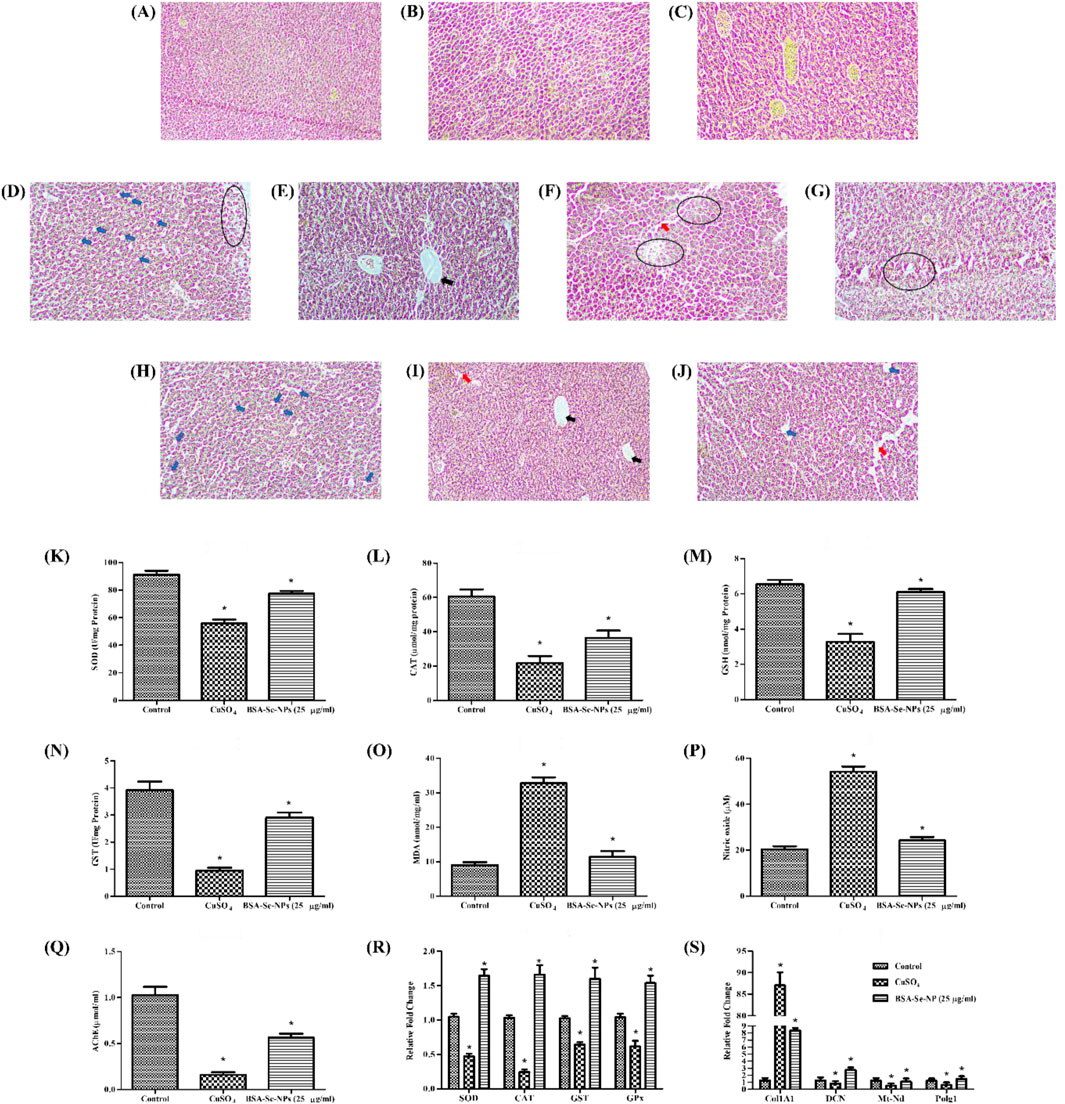
Figure 5. In vivo hepatic fibrosis model and antioxidant activity: histopathology of control (healthy) at (A) day 7, (B) day 14, and (C) day 21; stress group (CuSO4) at (D) day 7, (E,F) day 14, and (G) day 21; treatment group (25 μg/mL stabilized Se-NPs reduced with BSA for 30 min) at (H) day 7, (I) day 14, and (J) day 21. The symbols indicate the following: hepatic stellate cells (blue arrow); sinusoidal dilation (red arrow); vacuolation (black arrow), and degeneration (round), with scale bar (10 µm). Antioxidant enzymatic estimation: (K) SOD assay; (L) CAT assay; (M) GSH assay; (N) GST assay; (O) LPO assay; (P) NO assay; (Q) AChE assay. RT-PCR of (R) antioxidant genes and (S) hepatic fibrosis markers. The data were considered significant (p < 0.05) and marked by the symbol “*.”
3.10 Enzymatic assays
After the treatment period, adult zebrafish were homogenized to study the antioxidant enzymatic levels. The control group had 91.27 U/mg of SOD and 60.54 μmol/mg of CAT. Compared to the CuSO4-induced stress group with an SOD level of 55.88 U/mg and CAT level of 21.78 μmol/mg, the SOD and CAT levels were higher in the stabilized Se-NP (25 μg/mL)-treated group with 77.64 U/mg and 36.35 μmol/mg (Figures 5K,L). GSH and GST levels were significantly maintained in the stabilized Se-NP-treated group with 6.10 nmol/mg and 2.90 U/mg. In contrast, the CuSO4-induced stress group had 3.27 nmol/mg of GSH and 0.94 U/mg of GST. The control group had 6.53 nmol/mg of GSH and 3.91 U/mg of GST (Figures 5M,N).
MDA levels in the CuSO4 stress group were notably increased to 32.73 nmol/mg/mL compared to that in the control group, which was 9.01 nmol/mg/mL. Stabilized Se-NPs reduced the MDA levels to 11.35 nmol/mg/mL compared to that in the stress group (Figure 5O). The control group showed lower NO levels with 20.38 µM. In contrast, the stress group exhibited 54.13 µM, which was regulated to 24.20 µM in the treatment group that received stabilized Se-NPs (Figure 5P). The neuroprotectant AChE was significantly low in the CuSO4-induced stress group with 0.15 μmol/mL. Stabilized Se-NPs protected the AChE levels up to 0.56 μmol/mL compared to that in the stress group. The control group had higher AChE levels of 1.02 μmol/mL when compared to the stress group and treatment group (Figure 5Q).
3.11 Expression of antioxidant genes
Cellular homeostasis is disrupted by ROS generation, which is triggered by a deficiency of selenium, a crucial component of antioxidant enzymes. The larvae were subjected to CuSO4-induced stress, which effectively influenced the expression of antioxidant genes. CuSO4-exposed zebrafish larvae treated with stabilized Se-NPs reduced for 30 min and 1 h with BSA efficiently mitigated ROS, as demonstrated by the significant upregulation of SOD (1.64-fold), CAT (1.65-fold), glutathione-S-transferase (GST) (1.59-fold), and glutathione peroxidase (GPX) (1.53-fold) genes (Figure 5R). Likewise, stabilized Se-NPs also delayed hepatic damage, confirmed by the downregulation of Col1A1 (8.34-fold) and upregulation of DCN (2.75-fold), Mt-nd (1.07-fold), and PolG (1.49-fold) genes (Figure 5S).
4 Discussion
Selenium extraction from mussels was performed using saline and buffer extraction methods (Kristan et al., 2015). Our extraction method was cost-effective and sustainable, utilizing mussels as a natural source of selenium and reducing the need for expensive chemicals like sodium selenite. The procedure involves low-energy steps, making it feasible for large-scale applications. Se-NPs were synthesized using BSA as the reducing agent (Chandramohan et al., 2019). BSA is a stabilizing agent (Cao et al., 2019). Selenium peaks were observed at 196.03 (Tyburska et al., 2010), confirming its presence. The study showed that mussel-derived selenium exhibited peaks similar to those reported by Hassanien et al. on dye degradation, with XRD peaks at 26.5076°, 31.5757°, and 45.3321° corresponding to the (100), (110), and (111) planes for Se-NPs, demonstrating selenium’s presence (Hassanien et al., 2019). FTIR spectrum peaks at 3,277.830, 2,919.169, 1,628.229, 1,530.819, 1,454.547, 1,396.690, and 1,042.220 cm−1 represented functional groups, while those at 471.609 and 409.849 cm−1 indicated metal–ligand stretching, respectively. The presence of hydroxyl or amide groups from BSA was indicated by the peak at 3,277.830 cm−1, while that at 2,919.169 cm−1 likely came from BSA–protein backbones. The absorption peaks at 1,628.229 and 1,530.819 indicated amide bands and secondary structure changes upon the Se-NP interaction, with the peak at 1,396.690 showing the presence of ether or carboxyl groups, which were potentially involved in Se-NP stabilization, and those at 471.609 and 409.849 cm−1 indicating Se-NP binding with protein functional groups. In 2016, a study by Kalishwaralal et al. on a novel one-pot green synthesis of Se-NPs observed similar peaks (Kalishwaralal et al., 2016). SEM observation showed that non-stabilized Se-NPs were spherical, while stabilized Se-NPs were less aggregated than Se-NPs. A study by Chung et al. on green-synthesized BSA-coated Se-NPs reported that the nanoparticles were spherical in shape with a size ranging from 12.2 to 53.9 nm (Chung et al., 2020). Se-NPs potentially scavenged ROS, which was confirmed through cell-free DPPH and ABTS assays. A study by Wang et al. in 2024 on the biosynthesis of selenium nanoparticles by Bacillus licheniformis showed a similar scavenging activity (Wang et al., 2024). In 2024, a study by Vasanthakumar et al. on green synthesis, characterization, and functional validation of bio-transformed Se-NPs reported that Se-NPs exhibited 74% ROS scavenging activity at a concentration of 1 mg/mL (Vasanthakumar et al., 2024). A study on the antimicrobial and antioxidant potential of Se-NPs with Olea ferruginea fruit extract showed 81.12% ABTS scavenging activity (Hassan et al., 2022).
This study highlights the role of metals, such as copper, in inducing oxidative stress and organ damage, which is a key factor contributing to hepatic dysfunction and neurodegeneration via the brain–liver axis (Zhong et al., 2023; Hake et al., 2025). Metal-induced ROS generation can lead to mitochondrial dysfunction, inflammatory responses, and cellular damage, all of which are implicated in neurodegenerative disorders such as Parkinson’s and Alzheimer’s disease (Cheng et al., 2021). This preliminary research was conducted using the zebrafish model, which shares ∼70% genome similarity with humans and is a widely recognized model for studying oxidative stress, liver toxicity, and neurodegeneration (Howe et al., 2013; Adhish and Manjubala, 2023).
A previous study on embryo toxicity profile by Kalishwaralal et al. reported that at 15–25 μg/mL, malformations such as tail malformation and pericardium-sac edema were observed (Kalishwaralal et al., 2016). In our study, malformations were noted in the CuSO4-induced stress group, while the larvae treated with Se-NPs did not show any developmental malformations. Fluorescent staining showed a concentration-dependent decrease in ROS and LPO levels. Raju et al. reported a similar methodology to measure the ROS and LPO levels in zebrafish larvae exposed to H2O2 stress, which revealed higher intensity in the stress group than in the treatment groups (Raju et al., 2021).
Enzymatic analysis evaluated the decline and restoration of anti-oxidative enzymes, showing that CuSO4 exposure led to a significant impact by decreasing the first-line defense including SOD and CAT, which eventually triggered the levels of MDA and NO in both larvae and adult zebrafish. Se-NP treatment restored the SOD and CAT levels, although the larvae and adult zebrafish were exposed to CuSO4-induced stress. A study by Zhou et al. reported that CuSO4 diminished the SOD levels (Zhou et al., 2023). In 2023, Au et al. stated that Se-NPs improved the SOD levels in plasma (Li et al., 2022; Au et al., 2023). A study by Zhou et al. on CuSO4 exposure to oxidative stress stated that CuSO4 reduced the CAT levels (Zhou et al., 2023). Dawit Moges et al. stated that Se-NPs enhanced CAT levels and improved hepatic antioxidant defense mechanism (Dawit Moges et al., 2022), thus regulating the MDA and NO levels. A study by Salaramoli et al. reported that Se-NPs significantly reduced the MDA levels and improved the total antioxidant capacity (Salaramoli et al., 2023). A study by Buacheen et al. on antioxidant and anti-inflammatory activities of nano-selenium reported that Se-NPs exhibited significant anti-inflammatory activity by downregulating the NO levels (Buacheen et al., 2023). Similarly, levels of detoxifying enzymes such as GSH and GST were restored in Se-NP-treated larvae and adult zebrafish, showing the protective effects of Se-NPs against oxidative stress. In 2022, Othman et al. stated that Se-NPs notably increased GSH levels (Othman et al., 2022). Zahran et al. reported that GST levels were upregulated upon treatment with Se-NPs (Zahran et al., 2024).
To determine their effect on cerebral health, AChE levels and locomotor behavior were analyzed. The results showed that AChE levels were maintained in the group treated with Se-NPs, while the stress group had reduced levels of AChE, confirming that Se-NPs can be an effective neuroprotector. A study by Khalil et al. on Se-NPs imparting robust neuroprotection showed that Se-NPs significantly restored AChE levels and acted as a neuroprotector (Khalil et al., 2022). In 2010, a study by Pinton et al. reported that Se-NPs can retain memory and learning impairments triggered by streptozotocin (Pinton et al., 2010). The larvae that received treatment efficiently traveled farther distances than the larvae in the stress group. In 2019, a study on behavioral impairments and oxidative stress by Sarasamma et al. reported that behavioral alteration is greatly associated with the central nervous system and can be assessed based on their swimming pattern (Sarasamma et al., 2019).
The CuSO4-exposed zebrafish showed marked liver damage, characterized by the formation of vacuoles, sinusoidal dilation, and hepatocyte degeneration, with the damage progressively worsening over 7, 14, and 21 days. These findings suggest that CuSO4-induced oxidative stress severely impacts liver morphology, aligning with its known hepatotoxic effects. However, the treatment group, which received Se-NPs, significantly attenuated these damaging effects. While vacuolation and sinusoidal dilation were still present on days 14 and 21, respectively, the damage progression was notably delayed compared to that in the CuSO4 stress group. Most importantly, the absence of hepatocyte degeneration in the treatment group suggests that Se-NPs effectively preserved liver cell integrity. The delayed onset of liver pathology further underscores the potential of Se-NPs as a therapeutic intervention for liver injury (Arman, 2021). A study by Loeschner et al. (2014) demonstrated that Se-NPs exhibited distinct pharmacokinetic properties compared to ionic selenium, with prolonged circulation time, controlled release, and selective tissue accumulation (Loeschner et al., 2014). Similarly, Li et al. (2024) highlighted the role of nanoparticle surface chemistry in enhancing bioavailability and cellular uptake (Li et al., 2024). These findings suggest that the physicochemical properties of Se-NPs, such as size, charge, and coating, likely influence their absorption and biodistribution in zebrafish.
The study demonstrated that Se-NPs stabilized with BSA mitigated CuSO4-induced oxidative stress in zebrafish larvae by significantly enhancing antioxidant defenses and delaying hepatic damage. Notably, Se-NPs increased the expression of key antioxidant genes, indicating robust ROS neutralization, which helps maintain cellular homeostasis. The observed downregulation of Col1A1 suggests reduced collagen deposition, potentially slowing fibrosis, while the upregulation of DCN supports matrix remodeling, which aids in preserving liver structure (Zhu et al., 2013; Liang et al., 2021; Aljagthmi et al., 2024). Additionally, increased levels of Mt-nd and PolG suggest enhanced mitochondrial function and DNA repair, which are essential for cell recovery and resilience against oxidative stress (Valdivieso et al., 2007; Foote et al., 2018; Zhu et al., 2023). Collectively, Se-NPs showed a protective role in managing oxidative stress-induced liver damage, offering a promising strategy for liver damage prevention (Figure 6).
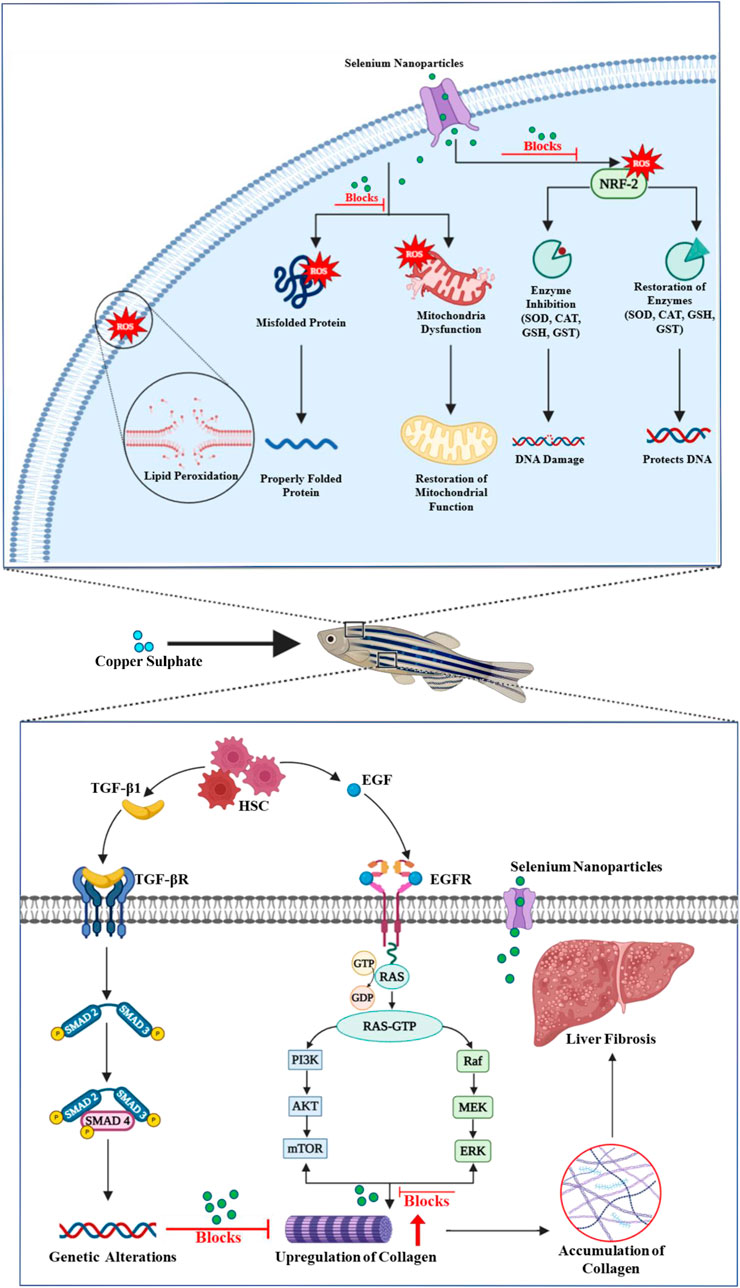
Figure 6. Schematic representation of the multifaceted protective role of selenium nanoparticles in preventing copper sulfate-induced liver fibrosis and maintaining cellular and mitochondrial integrity.
5 Conclusion
Liver fibrosis is a significant global health concern and an early stage in the progression toward cirrhosis and liver failure. CuSO4 is a common hepatotoxic agent that induces oxidative stress, leading to liver cell injury and fibrosis progression. Excess ROS generation in the liver accelerates fibrosis development. Extracting selenium from mussels offers an eco-friendly approach to obtaining this nutrient, which can be further processed into bioactive forms such as Se-NPs. Stabilized Se-NPs show better size reduction than non-stabilized Se-NPs. Treatment with stabilized Se-NPs effectively reduces developmental malformations and free radicals.
In vivo enzymatic assays showed that stabilized Se-NP treatment improved the antioxidant enzymatic levels in CuSO4-exposed larvae by reducing LPO and NO levels. Stabilized Se-NPs also enhanced the AChE levels and protected the cognitive behavior of larvae. The histopathological results further confirmed that Se-NP treatment delayed the progression of liver damage such as hepatic stellate cells, vacuoles, sinusoidal dilation, and hepatocyte degeneration. Gene expressions confirmed that Se-NPs effectively mitigated CuSO4-induced hepatic damage in zebrafish, as evidenced by the upregulation of antioxidant, DCN, Mt-nd, and PolG genes and the downregulation of the Col1A1 gene. The ability of Se-NPs to interact more efficiently with ROS, combined with their enhanced bioavailability, makes them a powerful tool in the fight against liver fibrosis and other oxidative stress-related conditions. These findings suggest that Se-NPs can regulate ROS levels and protect against oxidative stress-induced cellular and hepatic damage. Moreover, the neuroprotective potential of Se-NPs, linked through the brain–liver axis, opens promising avenues for further research on their therapeutic applications in neurodegenerative disorders.
Data availability statement
The datasets presented in this study can be found in online repositories. The names of the repository/repositories and accession number(s) can be found in the article/Supplementary Material.
Ethics statement
The animal study was approved by the Institutional Ethical Committee Guidelines (SU/CLAR/RD/001/2023). The study was conducted in accordance with the local legislation and institutional requirements.
Author contributions
SU: Formal Analysis, Investigation, Methodology, Software, Writing – original draft. IP: Conceptualization, Formal Analysis, Funding acquisition, Investigation, Project administration, Resources, Supervision, Validation, Visualization, Writing – original draft, Writing – review and editing.
Funding
The author(s) declare that no financial support was received for the research and/or publication of this article.
Acknowledgments
The authors extend our heartfelt gratitude to the Saveetha School of Engineering, Saveetha Institute of Medical and Technical Sciences, Saveetha University, for providing the essential infrastructure that enabled the successful completion of this work. The authors are especially grateful to Dr. Praveen Kumar I, Professor and Head, and Jenila John Santhi, Research Scholar in the Department of Medical Biotechnology, for their invaluable support throughout the execution of the zebrafish studies.
Conflict of interest
The authors declare that the research was conducted in the absence of any commercial or financial relationships that could be construed as a potential conflict of interest.
Generative AI statement
The author(s) declare that no Generative AI was used in the creation of this manuscript.
Publisher’s note
All claims expressed in this article are solely those of the authors and do not necessarily represent those of their affiliated organizations, or those of the publisher, the editors and the reviewers. Any product that may be evaluated in this article, or claim that may be made by its manufacturer, is not guaranteed or endorsed by the publisher.
Supplementary material
The Supplementary Material for this article can be found online at: https://www.frontiersin.org/articles/10.3389/fgene.2025.1522370/full#supplementary-material
References
Abderrahmani, K., Boulahdid, M., Bendou, N., Guenachi, B., Hacene, O. R., Masino, F., et al. (2021). Partitioning of trace elements in the tissues of Mediterranean mussels (Mytilus galloprovincialis) sampled from industrial sites along the Algerian coast. Mar. Pollut. Bull. 173, 113006. doi:10.1016/j.marpolbul.2021.113006
Addissouky, T. A., Ali, M. M. A., Sayed, I. E. T.El, and Wang, Y. (2024). Emerging advanced approaches for diagnosis and inhibition of liver Fibrogenesis. Egypt. J. Intern. Med. 36, 19. doi:10.1186/s43162-024-00283-y
Adhish, M., and Manjubala, I. (2023). Effectiveness of zebrafish models in understanding human diseases—a review of models. Heliyon 9, e14557. doi:10.1016/j.heliyon.2023.e14557
Aljagthmi, W. A., Alasmari, M. A., Daghestani, M. H., Al-Kharashi, L. A., Al-Mohanna, F. H., and Aboussekhra, A. (2024). Decorin (DCN) downregulation activates breast stromal fibroblasts and promotes their pro-carcinogenic effects through the IL-6/STAT3/AUF1 signaling. Cells 13, 680. doi:10.3390/cells13080680
Arman, S. (2021). Effects of acute triclosan exposure on gill and liver tissues of zebrafish (Danio rerio). Ann. Limnol. - Int. J. Limnol. 57, 6. doi:10.1051/limn/2021004
Athuluri-Divakar, S. K., and Hoshida, Y. (2019). Molecular prognosis in liver cirrhosis, in Genomic and precision medicine (Elsevier), 413–427. doi:10.1016/B978-0-12-801496-7.00021-6
Au, A., Mojadadi, A., Shao, J.-Y., Ahmad, G., and Witting, P. K. (2023). Physiological benefits of novel selenium delivery via nanoparticles. Int. J. Mol. Sci. 24, 6068. doi:10.3390/ijms24076068
Bataller, R., and Brenner, D. A. (2005). Liver fibrosis. J. Clin. Invest. 115, 209–218. doi:10.1172/JCI24282
Bisht, N., Phalswal, P., and Khanna, P. K. (2022). Selenium nanoparticles: a review on synthesis and biomedical applications. Mat. Adv. 3, 1415–1431. doi:10.1039/D1MA00639H
Bjørklund, G., Shanaida, M., Lysiuk, R., Antonyak, H., Klishch, I., Shanaida, V., et al. (2022). Selenium: an antioxidant with a critical role in anti-aging. Molecules 27, 6613. doi:10.3390/molecules27196613
Bradford, M. M. (1976). A rapid and sensitive method for the quantitation of microgram quantities of protein utilizing the principle of protein-dye binding. Anal. Biochem. 72, 248–254. doi:10.1016/0003-2697(76)90527-3
Buacheen, P., Chaipuang, A., Karinchai, J., Nuchuchua, O., Imsumran, A., Wongnoppavich, A., et al. (2023). Stabilization of antioxidant and anti-inflammatory activities of nano-selenium using anoectochilus burmannicus extract as a potential novel functional ingredient. Nutrients 15, 1018. doi:10.3390/nu15041018
Cao, H., Xiao, J., and Liu, H. (2019). Enhanced oxidase-like activity of selenium nanoparticles stabilized by chitosan and application in a facile colorimetric assay for mercury (II). Biochem. Eng. J. 152, 107384. doi:10.1016/j.bej.2019.107384
Chandramohan, S., Sundar, K., and Muthukumaran, A. (2019). Reducing agents influence the shapes of selenium nanoparticles (SeNPs) and subsequently their antibacterial and antioxidant activity. Mat. Res. Express 6, 0850i2. doi:10.1088/2053-1591/ab29d6
Chen, G., Yang, F., Fan, S., Jin, H., Liao, K., Li, X., et al. (2022). Immunomodulatory roles of selenium nanoparticles: novel arts for potential immunotherapy strategy development. Front. Immunol. 13, 956181. doi:10.3389/fimmu.2022.956181
Chen, S.-Y., Chen, X., Zhu, S., Xu, J.-J., Li, X.-F., Yin, N.-N., et al. (2024). miR-324-3p suppresses hepatic stellate cell activation and hepatic fibrosis via regulating SMAD4 signaling pathway. Mol. Biotechnol. 67, 673–688. doi:10.1007/s12033-024-01078-w
Chen, Z., Tian, R., She, Z., Cai, J., and Li, H. (2020). Role of oxidative stress in the pathogenesis of nonalcoholic fatty liver disease. Free Radic. Biol. Med. 152, 116–141. doi:10.1016/j.freeradbiomed.2020.02.025
Cheng, H., Yang, B., Ke, T., Li, S., Yang, X., Aschner, M., et al. (2021). Mechanisms of metal-induced mitochondrial dysfunction in neurological disorders. Toxics 9, 142. doi:10.3390/toxics9060142
Chung, S., Zhou, R., and Webster, T. J. (2020). Green synthesized BSA-coated selenium nanoparticles inhibit bacterial growth while promoting mammalian cell growth. Int. J. Nanomedicine 15, 115–124. doi:10.2147/IJN.S193886
Dawit Moges, F., Hamdi, H., Al-Barty, A., Zaid, A. A., Sundaray, M., Parashar, S. K. S., et al. (2022). Effects of selenium nanoparticle on the growth performance and nutritional quality in Nile Tilapia, Oreochromis niloticus. PLoS One 17, e0268348. doi:10.1371/journal.pone.0268348
De Cól, J. P., de Lima, E. P., Pompeu, F. M., Cressoni Araújo, A., de Alvares Goulart, R., Bechara, M. D., et al. (2024). Underlying Mechanisms behind the brain–gut–liver Axis and metabolic-associated fatty liver disease (MAFLD): an update. Int. J. Mol. Sci. 25, 3694. doi:10.3390/ijms25073694
Dey, S., Rajak, P., and Sen, K. (2024). Bioaccumulation of metals and metalloids in seafood: a comprehensive overview of mobilization, interactive effects in eutrophic environments, and implications for public health risks. J. Trace Elem. Miner. 8, 100141. doi:10.1016/j.jtemin.2024.100141
Dumore, N. S., and Mukhopadhyay, M. (2020). Antioxidant properties of aqueous selenium nanoparticles (ASeNPs) and its catalysts activity for 1, 1-diphenyl-2-picrylhydrazyl (DPPH) reduction. J. Mol. Struct. 1205, 127637. doi:10.1016/j.molstruc.2019.127637
Foote, K., Reinhold, J., Yu, E. P. K., Figg, N. L., Finigan, A., Murphy, M. P., et al. (2018). Restoring mitochondrial DNA copy number preserves mitochondrial function and delays vascular aging in mice. Aging Cell 17, e12773. doi:10.1111/acel.12773
Gao, F., Yuan, Z., Zhang, L., Peng, Y., Qian, K., and Zheng, M. (2023). Toxic effects of copper fungicides on the development and behavior of zebrafish in early-life stages. Nanomaterials 13, 2629. doi:10.3390/nano13192629
Hake, G., Mhaske, A., Shukla, R., and Flora, S. J. S. (2025). Copper-induced neurodegenerative disorders and therapeutic potential of curcumin-loaded nanoemulsion. Toxics 13, 108. doi:10.3390/toxics13020108
Hassan, H. U., Raja, N. I., Abasi, F., Mehmood, A., Qureshi, R., Manzoor, Z., et al. (2022). Comparative study of antimicrobial and antioxidant potential of Olea ferruginea fruit extract and its mediated selenium nanoparticles. Molecules 27, 5194. doi:10.3390/molecules27165194
Hassanien, R., Abed-Elmageed, A. A. I., and Husein, D. Z. (2019). Eco-friendly approach to synthesize selenium nanoparticles: photocatalytic degradation of sunset yellow azo dye and anticancer activity. ChemistrySelect 4, 9018–9026. doi:10.1002/slct.201901267
Herrera, K. J., Panier, T., Guggiana-Nilo, D., and Engert, F. (2021). Larval zebrafish use olfactory detection of sodium and chloride to avoid salt water. Curr. Biol. 31, 782–793.e3. doi:10.1016/j.cub.2020.11.051
Howe, K., Clark, M. D., Torroja, C. F., Torrance, J., Berthelot, C., Muffato, M., et al. (2013). The zebrafish reference genome sequence and its relationship to the human genome. Nature 496, 498–503. doi:10.1038/nature12111
Ijaz, I., Gilani, E., Nazir, A., and Bukhari, A. (2020). Detail review on chemical, physical and green synthesis, classification, characterizations and applications of nanoparticles. Green Chem. Lett. Rev. 13, 223–245. doi:10.1080/17518253.2020.1802517
Issac, P. K., Guru, A., Velayutham, M., Pachaiappan, R., Arasu, M. V., Al-Dhabi, N. A., et al. (2021). Oxidative stress induced antioxidant and neurotoxicity demonstrated in vivo zebrafish embryo or larval model and their normalization due to morin showing therapeutic implications. Life Sci. 283, 119864. doi:10.1016/j.lfs.2021.119864
Issac, P. K., Santhi, J. J., Janarthanam, V. A., and Velumani, K. (2024). Diosgenin-conjugated zinc oxide nanoparticles: a sustainable approach to counter antibiotic-induced oxidative stress in the aquatic environment using the in vivo zebrafish larvae model (Danio rerio). Bionanoscience 14, 903–918. doi:10.1007/s12668-024-01383-3
Issac, P. K., and Velumani, K. (2024). Rutin trihydrate conjugated zinc oxide nanoparticles targeting oxidative stress pathways for the protection of gut microbiome dysfunction and neurodegenerative diseases. Bionanoscience 14, 5310–5326. doi:10.1007/s12668-024-01430-z
Jomova, K., Raptova, R., Alomar, S. Y., Alwasel, S. H., Nepovimova, E., Kuca, K., et al. (2023). Reactive oxygen species, toxicity, oxidative stress, and antioxidants: chronic diseases and aging. Arch. Toxicol. 97, 2499–2574. doi:10.1007/s00204-023-03562-9
Kalishwaralal, K., Jeyabharathi, S., Sundar, K., and Muthukumaran, A. (2015). Comparative analysis of cardiovascular effects of selenium nanoparticles and sodium selenite in zebrafish embryos. Artif. Cells, Nanomedicine, Biotechnol. 44, 990–996. doi:10.3109/21691401.2015.1008507
Kalishwaralal, K., Jeyabharathi, S., Sundar, K., and Muthukumaran, A. (2016). A novel one-pot green synthesis of selenium nanoparticles and evaluation of its toxicity in zebrafish embryos. Artif. Cells, Nanomedicine, Biotechnol. 44, 471–477. doi:10.3109/21691401.2014.962744
Karthik, K. K., Cheriyan, B. V., Rajeshkumar, S., and Gopalakrishnan, M. (2024). A review on selenium nanoparticles and their biomedical applications. Biomed. Technol. 6, 61–74. doi:10.1016/j.bmt.2023.12.001
Khalil, H. M. A., Azouz, R. A., Hozyen, H. F., Aljuaydi, S. H., AbuBakr, H. O., Emam, S. R., et al. (2022). Selenium nanoparticles impart robust neuroprotection against deltamethrin-induced neurotoxicity in male rats by reversing behavioral alterations, oxidative damage, apoptosis, and neuronal loss. Neurotoxicology 91, 329–339. doi:10.1016/j.neuro.2022.06.006
Khurana, A., Tekula, S., Saifi, M. A., Venkatesh, P., and Godugu, C. (2019). Therapeutic applications of selenium nanoparticles. Biomed. Pharmacother. 111, 802–812. doi:10.1016/j.biopha.2018.12.146
Kora, A. J. (2018). Tree gum stabilised selenium nanoparticles: characterisation and antioxidant activity. IET nanobiotechnology 12, 658–662. doi:10.1049/iet-nbt.2017.0310
Krishnan, M., and Kang, S. C. (2019). Vitexin inhibits acrylamide-induced neuroinflammation and improves behavioral changes in zebrafish larvae. Neurotoxicol. Teratol. 74, 106811. doi:10.1016/j.ntt.2019.106811
Kristan, U., Planinšek, P., Benedik, L., Falnoga, I., and Stibilj, V. (2015). Polonium-210 and selenium in tissues and tissue extracts of the mussel Mytilus galloprovincialis (Gulf of Trieste). Chemosphere 119, 231–241. doi:10.1016/j.chemosphere.2014.05.017
Li, F., Lin, J., Liu, X., Li, W., Ding, Y., Zhang, Y., et al. (2018). Characterization of the locomotor activities of zebrafish larvae under the influence of various neuroactive drugs. Ann. Transl. Med. 6, 173. doi:10.21037/atm.2018.04.25
Li, Q., Cao, Y., Wang, Y., Long, X., Sun, W., Yang, H., et al. (2023). Toxic effects of copper sulfate and trichlorfon on the intestines of zebrafish (Danio rerio). Aquac. Res. 2023, 1–9. doi:10.1155/2023/8360334
Li, W., Lu, X., Jiang, L., and Wang, X. (2024). Biosafety and pharmacokinetic characteristics of polyethylene pyrrolidone modified nano selenium in rats. BMC Biotechnol. 24, 98. doi:10.1186/s12896-024-00915-9
Li, Y., Fan, M., Qiu, Q., Wang, Y., Shen, X., and Zhao, K. (2022). Nano-selenium and macleaya cordata extracts improved immune function and reduced oxidative damage of sows and IUGR piglets after heat stress of sows in late gestation. Biol. Trace Elem. Res. 200, 5081–5090. doi:10.1007/s12011-022-03103-y
Liang, X., Wan, Y., Shen, Z., Liu, Y., Li, D., Li, L., et al. (2021). Molecular characterization of Hsp47 in grass carp (Ctenopharyngodon idella) and its correlation with type I collagen in response to fish aerobic exercise. Fishes 6, 17. doi:10.3390/fishes6020017
Lite, C., Guru, A., Juliet, M., and Arockiaraj, J. (2022). Embryonic exposure to butylparaben and propylparaben induced developmental toxicity and triggered anxiety-like neurobehavioral response associated with oxidative stress and apoptosis in the head of zebrafish larvae. Environ. Toxicol. 37, 1988–2004. doi:10.1002/tox.23545
Loeschner, K., Hadrup, N., Hansen, M., Pereira, S. A., Gammelgaard, B., Møller, L. H., et al. (2014). Absorption, distribution, metabolism and excretion of selenium following oral administration of elemental selenium nanoparticles or selenite in rats. Metallomics 6, 330–337. doi:10.1039/c3mt00309d
Luangmonkong, T., Suriguga, S., Mutsaers, H. A. M., Groothuis, G. M. M., Olinga, P., and Boersema, M. (2018). Targeting oxidative stress for the treatment of liver fibrosis. Rev. Physiol. Biochem. Pharmacol. 175, 71–102. doi:10.1007/112_2018_10
Machat, J., Otruba, V., and Kanicky, V. (2002). Spectral and non-spectral interferences in the determination of selenium by inductively coupled plasma atomic emission spectrometry. J. Anal. At. Spectrom. 17, 1096–1102. doi:10.1039/B202167F
Nguyen, T. H., Le, H. D., Nguyen Thi Kim, T., Pham The, H., Nguyen, T. M., Cornet, V., et al. (2020). Anti–inflammatory and antioxidant properties of the ethanol extract of clerodendrum Cyrtophyllum Turcz in copper sulfate-induced inflammation in zebrafish. Antioxidants 9, 192. doi:10.3390/antiox9030192
Nie, T., Wu, H., Wong, K. H., and Chen, T. (2016). Facile synthesis of highly uniform selenium nanoparticles using glucose as the reductant and surface decorator to induce cancer cell apoptosis. J. Mat. Chem. B 4, 2351–2358. doi:10.1039/c5tb02710a
OECD (2013). Test No. 236: Fish Embryo Acute Toxicity (FET) Test. OECD Guidel. Test. Chem. Sect. 2, OECD Publ., 1–22.
Osman, A. I., Zhang, Y., Farghali, M., Rashwan, A. K., Eltaweil, A. S., Abd El-Monaem, E. M., et al. (2024). Synthesis of green nanoparticles for energy, biomedical, environmental, agricultural, and food applications: a review. Environ. Chem. Lett. 22, 841–887. doi:10.1007/s10311-023-01682-3
Othman, M. S., Obeidat, S. T., Al-Bagawi, A. H., Fareid, M. A., Fehaid, A., and Abdel Moneim, A. E. (2022). Green-synthetized selenium nanoparticles using berberine as a promising anticancer agent. J. Integr. Med. 20, 65–72. doi:10.1016/j.joim.2021.11.002
Pinton, S., da Rocha, J. T., Zeni, G., and Nogueira, C. W. (2010). Organoselenium improves memory decline in mice: involvement of acetylcholinesterase activity. Neurosci. Lett. 472, 56–60. doi:10.1016/j.neulet.2010.01.057
Qamar, N., John, P., and Bhatti, A. (2020). Toxicological and anti-Rheumatic potential of Trachyspermum ammi derived biogenic selenium nanoparticles in arthritic balb/c mice. Int. J. Nanomedicine 15, 3497–3509. doi:10.2147/IJN.S243718
Qian, Y., Shang, Z., Gao, Y., Wu, H., and Kong, X. (2022). Liver regeneration in chronic liver injuries: basic and clinical applications focusing on macrophages and natural killer cells. Cell. Mol. Gastroenterol. Hepatol. 14, 971–981. doi:10.1016/j.jcmgh.2022.05.014
Raju, S. V., Mukherjee, A., Sarkar, P., Issac, P. K., Lite, C., Paray, B. A., et al. (2021). RM12 similar to substance P from tachykinin of freshwater murrel Channa striatus influence intracellular ROS in vitro fish erythrocytes and developmental toxicity and antioxidant enzymes in vivo zebrafish embryo. Fish. Physiol. Biochem. 47, 1073–1085. doi:10.1007/s10695-021-00950-9
Ramamurthy, C., Sampath, K. S., Arunkumar, P., Kumar, M. S., Sujatha, V., Premkumar, K., et al. (2013). Green synthesis and characterization of selenium nanoparticles and its augmented cytotoxicity with doxorubicin on cancer cells. Bioprocess Biosyst. Eng. 36, 1131–1139. doi:10.1007/s00449-012-0867-1
Ranjha, M. M. A. N., Shafique, B., Rehman, A., Mehmood, A., Ali, A., Zahra, S. M., et al. (2022). Biocompatible nanomaterials in food science, Technology, and nutrient drug delivery: recent developments and applications. Front. Nutr. 8, 778155. doi:10.3389/fnut.2021.778155
Salaramoli, S., Amiri, H., Joshaghani, H. R., Hosseini, M., and Hashemy, S. I. (2023). Bio-synthesized selenium nanoparticles ameliorate Brain oxidative stress in Parkinson disease rat models. Metab. Brain Dis. 38, 2055–2064. doi:10.1007/s11011-023-01222-6
Salem, S. S., Badawy, M. S. E. M., Al-Askar, A. A., Arishi, A. A., Elkady, F. M., and Hashem, A. H. (2022). Green biosynthesis of selenium nanoparticles using orange peel waste: characterization, antibacterial and antibiofilm activities against multidrug-resistant bacteria. Life 12, 893. doi:10.3390/life12060893
Santhi, J. J., Issac, P. K., Velayutham, M., Rajan, P. S. S., Hussain, S. A., Shaik, M. R., et al. (2025). Neurotoxic effects of chronic exposure to perfluorobutane sulfonate in adult zebrafish (Danio Rerio). Comp. Biochem. Physiol. Part C Toxicol. Pharmacol. 292, 110162. doi:10.1016/j.cbpc.2025.110162
Sarasamma, S., Audira, G., Samikannu, P., Juniardi, S., Siregar, P., Hao, E., et al. (2019). Behavioral impairments and oxidative stress in the brain, muscle, and gill caused by chronic exposure of C70 nanoparticles on adult zebrafish. Int. J. Mol. Sci. 20, 5795. doi:10.3390/ijms20225795
Schilrreff, P., and Alexiev, U. (2022). Chronic inflammation in non-healing skin wounds and promising natural bioactive compounds treatment. Int. J. Mol. Sci. 23, 4928. doi:10.3390/ijms23094928
Schulz, K., Kerber, S., and Kelm, M. (1999). Reevaluation of the griess method for determining NO/NO−2 in aqueous and protein-containing samples. Nitric Oxide 3, 225–234. doi:10.1006/niox.1999.0226
Seffner, W., Schiller, F., Lippold, U., Dieter, H. H., and Hoffmann, A. (1997). Experimental induction of liver fibrosis in young Guinea pigs by combined application of copper sulphate and aflatoxin B1. Toxicol. Lett. 92, 161–172. doi:10.1016/S0378-4274(97)00052-0
Sen, A., Kurian, M. S., Narayanan, D. P., Abraham, A., Thomas, T. S., Jayalakshmi, P. S., et al. (2024). Bio-nanocomposites: innovative solutions for addressing issues in health, agriculture, energy and environmental domains. Nano-Structures and Nano-Objects 39, 101270. doi:10.1016/j.nanoso.2024.101270
Sudhakaran, G., Chandran, A., Sreekutty, A. R., Madesh, S., Pachaiappan, R., Almutairi, B. O., et al. (2023). Ophthalmic intervention of naringenin decreases vascular endothelial growth factor by counteracting oxidative stress and cellular damage in In Vivo zebrafish. Molecules 28, 5350. doi:10.3390/molecules28145350
Tyburska, A., Jankowski, K., Ramsza, A., Reszke, E., Strzelec, M., and Andrzejczuk, A. (2010). Feasibility study of the determination of selenium, antimony and arsenic in drinking and mineralwater by ICP-OES using a dual-flow ultrasonic nebulizer and direct hydride generation. J. Anal. At. Spectrom. 25, 210–214. doi:10.1039/B916729C
Umapathy, S., Pan, I., Issac, P. K., Kumar, M. S. K., Giri, J., Guru, A., et al. (2024). Selenium nanoparticles as neuroprotective agents: insights into molecular mechanisms for Parkinson’s disease treatment. Mol. Neurobiol. doi:10.1007/s12035-024-04253-x
Valdivieso, Á. G., Marcucci, F., Taminelli, G., Guerrico, A. G., Álvarez, S., Teiber, M. L., et al. (2007). The expression of the mitochondrial gene MT-ND4 is downregulated in cystic fibrosis. Biochem. Biophys. Res. Commun. 356, 805–809. doi:10.1016/j.bbrc.2007.03.057
Vasanthakumar, S., Manikandan, M., and Arumugam, M. (2024). Green synthesis, characterization and functional validation of bio-transformed selenium nanoparticles. Biochem. Biophys. Rep. 39, 101760. doi:10.1016/j.bbrep.2024.101760
Vegas-Suárez, S., Simón, J., Martínez-Chantar, M. L., and Moratalla, R. (2022). Metabolic diffusion in neuropathologies: the relevance of brain-liver Axis. Front. Physiol. 13, 864263. doi:10.3389/fphys.2022.864263
Velayutham, M., Ojha, B., Issac, P. K., Lite, C., Guru, A., Pasupuleti, M., et al. (2021). NV14 from serine O -acetyltransferase of cyanobacteria influences the antioxidant enzymes in vitro cells, gene expression against H 2 O 2 and other responses in vivo zebrafish larval model. Cell Biol. Int. 45, 2331–2346. doi:10.1002/cbin.11680
Wang, T., Chen, X., Long, X., Liu, Z., and Yan, S. (2016). Copper nanoparticles and copper sulphate induced cytotoxicity in hepatocyte primary cultures of Epinephelus coioides. PLoS One 11, e0149484. doi:10.1371/journal.pone.0149484
Wang, Y., Tian, J., Shi, F., Li, X., Hu, Z., and Chu, J. (2021). Protective effect of surfactin on copper sulfate-induced inflammation, oxidative stress, and hepatic injury in zebrafish. Microbiol. Immunol. 65, 410–421. doi:10.1111/1348-0421.12924
Wang, Z., Li, N., Zhou, X., Wei, S., Zhu, Y., Li, M., et al. (2024). Optimization of fermentation parameters to improve the biosynthesis of selenium nanoparticles by Bacillus licheniformis F1 and its comprehensive application. BMC Microbiol. 24, 271. doi:10.1186/s12866-024-03410-5
Williams, S. Y., and Renquist, B. J. (2016). High throughput Danio rerio energy expenditure assay. J. Vis. Exp., e53297. doi:10.3791/53297
Yamanaka, O., and Takeuchi, R. (2018). UMATracker: an intuitive image-based tracking platform. J. Exp. Biol. 221, jeb182469. doi:10.1242/jeb.182469
Zahran, E., Elbahnaswy, S., Ahmed, F., Risha, E., Mansour, A. T., Alqahtani, A. sultan, et al. (2024). Dietary microalgal-fabricated selenium nanoparticles improve Nile tilapia biochemical indices, immune-related gene expression, and intestinal immunity. BMC Vet. Res. 20, 107. doi:10.1186/s12917-024-03966-4
Zhang, F., Li, X., and Wei, Y. (2023a). Selenium and selenoproteins in health. Biomolecules 13, 799. doi:10.3390/biom13050799
Zhang, J., Zhu, M., Wang, Q., and Yang, H. (2023b). The combined use of copper sulfate and trichlorfon exerts stronger toxicity on the liver of zebrafish. Int. J. Mol. Sci. 24, 11203. doi:10.3390/ijms241311203
Zhang, P., Liu, N., Xue, M., Zhang, M., Liu, W., Xu, C., et al. (2023c). Anti-inflammatory and antioxidant properties of β-Sitosterol in copper sulfate-induced inflammation in zebrafish (Danio rerio). Antioxidants 12, 391. doi:10.3390/antiox12020391
Zhao, G., Wu, X., Chen, P., Zhang, L., Yang, C. S., and Zhang, J. (2018). Selenium nanoparticles are more efficient than sodium selenite in producing reactive oxygen species and hyper-accumulation of selenium nanoparticles in cancer cells generates potent therapeutic effects. Free Radic. Biol. Med. 126, 55–66. doi:10.1016/j.freeradbiomed.2018.07.017
Zhong, G., Li, L., Li, Y., Ma, F., Liao, J., Li, Y., et al. (2023). Cuproptosis is involved in copper-induced hepatotoxicity in chickens. Sci. Total Environ. 866, 161458. doi:10.1016/j.scitotenv.2023.161458
Zhou, S., Yang, Q., Song, Y., Cheng, B., and Ai, X. (2023). Effect of copper sulphate exposure on the oxidative stress, gill transcriptome and external microbiota of yellow catfish, Pelteobagrus fulvidraco. Antioxidants 12, 1288. doi:10.3390/antiox12061288
Zhu, J.-N., Chen, R., Fu, Y.-H., Lin, Q.-X., Huang, S., Guo, L.-L., et al. (2013). Smad3 inactivation and MiR-29b upregulation mediate the effect of carvedilol on attenuating the acute myocardium infarction-induced myocardial fibrosis in rat. PLoS One 8, e75557. doi:10.1371/journal.pone.0075557
Keywords: liver fibrosis, reactive oxygen species, selenium nanoparticles, antioxidant, zebrafish
Citation: Umapathy S and Pan I (2025) Evaluating the therapeutic potential of BSA-reduced mussel-derived selenium nanoparticles to mitigate copper sulfate-induced hepatic damage and neurodegeneration in a zebrafish model. Front. Genet. 16:1522370. doi: 10.3389/fgene.2025.1522370
Received: 04 November 2024; Accepted: 23 April 2025;
Published: 19 May 2025.
Edited by:
Paolo Abondio, IRCCS Institute of Neurological Sciences of Bologna (ISNB), ItalyReviewed by:
Tin Yan Wong, Hong Kong University of Science and Technology, Hong Kong SAR, ChinaAhmed M. A. Akabawy, Helwan University, Egypt
Copyright © 2025 Umapathy and Pan. This is an open-access article distributed under the terms of the Creative Commons Attribution License (CC BY). The use, distribution or reproduction in other forums is permitted, provided the original author(s) and the copyright owner(s) are credited and that the original publication in this journal is cited, in accordance with accepted academic practice. No use, distribution or reproduction is permitted which does not comply with these terms.
*Correspondence: Ieshita Pan, Ym9ueS5pZXNrQGdtYWlsLmNvbQ==, aWVzaGl0YXBhbi5zc2VAc2F2ZWV0aGEuY29t