- 1Infectious Diseases and Immunity in Global Health Program, Research Institute of McGill University Health Centre, Montreal, QC, Canada
- 2Department of Microbiology and Immunology, McGill University, Montreal, QC, Canada
- 3Department of Biological Sciences and CERMO-FC Research Centre, Université du Québec à Montréal, Montreal, QC, Canada
- 4Division of Infectious Diseases and Chronic Viral Illness Service, McGill University Health Centre, Montreal, QC, Canada
Despite the success of antiretroviral therapy (ART), people living with HIV continue to suffer from high burdens of respiratory infections, lung cancers and chronic lung disease at a higher rate than the general population. The lung mucosa, a previously neglected HIV reservoir site, is of particular importance in this phenomenon. Because ART does not eliminate the virus, residual levels of HIV that remain in deep tissues lead to chronic immune activation and pulmonary inflammatory pathologies. In turn, continuous pulmonary and systemic inflammation cause immune cell exhaustion and pulmonary immune dysregulation, creating a pro-inflammatory environment ideal for HIV reservoir persistence. Moreover, smoking, gut and lung dysbiosis and co-infections further fuel the vicious cycle of residual viral replication which, in turn, contributes to inflammation and immune cell proliferation, further maintaining the HIV reservoir. Herein, we discuss the recent evidence supporting the notion that the lungs serve as an HIV viral reservoir. We will explore how smoking, changes in the microbiome, and common co-infections seen in PLWH contribute to HIV persistence, pulmonary immune dysregulation, and high rates of infectious and non-infectious lung disease among these individuals.
Introduction
HIV-1 infection is characterized by chronic immune activation and inflammation, which are predictors of disease progression (1, 2). In fact, HIV+ status has been linked to higher prevalence of age-associated noncommunicable comorbidities, such as cardiovascular and renal disease, where inflammation is believed to be the driving factor (3). Furthermore, chronic inflammatory states persist in PLWH despite virologic suppression with antiretroviral therapy (ART), which has been linked to a higher non-AIDS related morbidity and mortality rate (4, 5). Chronic inflammation and the prevalence of infectious and non-infectious comorbidities is especially pertinent in regards to the lungs. Even on ART, PLWH are 25 times more likely to suffer from pneumonia and are at a higher risk of developing chronic obstructive pulmonary disease or lung cancer than healthy individuals (6–10). Rates of tuberculosis infection and influenza-associated mortality is also higher in PLWH than in general population (11, 12).
Despite the success of ART at inhibiting HIV replication, the major challenge for a functional HIV cure is viral persistence in cellular and tissue reservoirs. An HIV reservoir is an anatomical site harboring cells where a replication-competent proviral DNA with stable kinetic properties persists in its integrated form (13). As a retrovirus, HIV integrates its DNA into the genome of host cells, mainly CD4 T cells (14). After acute infection, the provirus enters a state of post-integration latency whereby viral transcription is reversibly silenced. The lack of viral protein expression on the cell surface protects the cell from detection and elimination by the immune system (15). Cellular HIV reservoirs are established during the early days of infection, whereby HIV seeds different anatomical sites such as lymph nodes, gut-associated lymphoid tissue, the central nervous system, the genitourinary tract and, based on recent evidence, the lungs (16–18).
Early viral presence in the lung, along with high rate of lung diseases in PLWH even after ART initiation raise the question on why and how the lung might serve as another anatomical HIV reservoir and what challenges does it pose on our way to a true functional HIV cure.
Lung Immunity
Just like the skin and the gastrointestinal tract, the lungs are an interface between the inner body and the outside world. Apart from ensuring adequate gas exchange, the lung must prevent harmful effects of noxious compounds, microbes, and debris on the body. The lungs are designed to facilitate optimal oxygen exchange between the environment and red blood cells. Thus, not only are they highly vascularized, but they also house around 300 million alveoli, covering a surface area of approximately 500 m2 – roughly the size of a tennis court (19, 20). This entire area must be kept clean of unwanted airborne particles and is patrolled continuously for foreign invaders – a task executed by the innate and adaptive arms of the immune system.
Physical and Biochemical Barriers
The human respiratory tract can be divided into the upper and lower parts. The upper portion is composed of the nasal cavity, pharynx, and larynx, while the lower portion includes the trachea and the lung itself, which houses part of the conducting airways (bronchi, bronchioles) that lead into the respiratory zone (the alveoli) (21). The lumen of the airways is lined with epithelium that is made up of ciliated, non-ciliated, and secretory cells (21). These are sealed shut with tight junctions, creating a physical barrier, whose permeability is controlled by transmembrane proteins called claudins (22). This barrier is further reinforced by a layer of airway surface fluid made of mucins - large heavily glycosylated proteins secreted by goblet cells that form a gel-like physical barrier that protects the underlying cells from physical and chemical stressors. Moreover, this barrier acts as an antimicrobial wall, trapping any microbes with which it comes into contact (23). In addition to mucins, airway surface fluid contains a large number of antimicrobial proteins and peptides, such as defensins and lysozymes, which collectively exhibit broad-spectrum antimicrobial activity (24). Airway mucous, along with the trapped microbes and debris within it, is pushed out continuously from the lower lung into the trachea and esophagus by the mucociliary clearance system where ciliated cells move the surface fluid via the motive force of their cilia. As we progress from the terminal bronchus into the alveolar sacs, the cellular and airway surface fluid makeup changes. Here, in the alveolar sacs, the epithelial barrier becomes extremely thin and its surface mucus becomes replaced by surfactant, a detergent-like substance that prevents alveolar collapse when we breathe (25). Since gas exchange in this zone is vital, structural damage, air flow obstruction, or uncontrolled inflammation within this tissue can have life threatening consequences (26).
Sensor Cells of the Innate Immune System
The next tier of the lung’s defense system are sensor cells, which include the aforementioned epithelial cells along with alveolar macrophages (AMs), dendritic cells (DCs), and mast cells (25). In bronchoalveolar lavage (BAL) fluid, AMs make up the majority of this cell pool (~85%) (27). Unlike most immune cells, AMs are largely derived from a unique tissue-resident cell subset that originates from a distinct hematopoietic cell lineage during embryonic development (28, 29). When quiescent, the primary role of AMs is to clear the lung of allergen particles, dead cells, and other debris to maintain tissue homeostasis. They also receive multiple inhibitory signals, such as CD200, transforming growth factor−β (TGF-β) and interleukin−10 (IL−10), from pulmonary epithelium that prevent their unnecessary activation (30). Notably, healthy lung microbiota further supports these anti-inflammatory homeostatic functions (31). However, during an active infection, these processes are interrupted. Once a pathogen is encountered and recognized by one of the aforementioned sensor cells, pro-inflammatory cytokines are released, which immediately initiate an innate immune response. The type of immune response initiated depends on the nature of the pathogen and the type of cytokines it triggers. For instance, in the case of a viral infection, epithelial cells, AMs and DCs begin producing Type I and Type III interferons (IFNs), to limit pathogen spread and induce an antiviral state of the cells in the vicinity (32).
Engaging the Adaptive Immune System
If the aforementioned mechanisms fail to clear the pathogen, tissue-resident lymphoid cells are recruited. These include innate lymphoid cells, natural killer cells, Natural Killer (NK) T-cells, conventional CD8 and CD4 T-cells, as well as mucosal-associated invariant T-cells (MAIT) and γδ T-cells. Collectively, these cells further enhance direct killing of the pathogen or infected cells and recruit more effector cells from the circulation, such as neutrophils and monocytes, to facilitate infection clearance in its early stages (25). If the pathogen is still not cleared, additional forces of the adaptive immune system are called to action. DCs are the key mediators of this process. Residing under the epithelial layer within the pulmonary interstitium, these cells can extend their dendrites across the epithelial layer to sample antigen from the lumen. Under inflammatory conditions, DCs become activated and begin to transport the microbial antigen to the draining lymph nodes and nearby mucosal associated lymphoid tissues, where they activate naïve and central memory T-cells whose T-cell receptor matches the MHC-peptide complex on DC’s surface (33–35). The efficiency of DCs to activate these cells depends on both the nature of infection and the type of T-cell it encounters. For example, during respiratory influenza virus infection, CD103+ migratory DCs are the most potent activators of naïve virus-specific CD8 T-cells (36, 37).
CD8+ cytotoxic T-cells and CD4+ Th1 cells fight intracellular microbes by killing infected cells, releasing pro-inflammatory and anti-viral cytokines (IFN-γ), and recruiting phagocytes to the infection site (25, 33, 38). CD8+ Tc2 cells and CD4+ Th2 cells fight extracellular parasites via granulocyte recruitment, mast cell activation, stimulation of mucus production by goblet cells, and promotion of B-cell class-switching to IgE (25, 38). CD4+ Th17 and CD8+ Tc17 cells are devoted to battling against extracellular bacteria and fungi. These cells amplify neutrophil recruitment, stimulate antimicrobial peptide production by the pulmonary epithelium, and promote B-cell class-switching to opsonizing antibody production (38, 39). B-cells and follicular helper T-cells (Tfh) are crucial against all classes of pathogen. With the aid of Tfh cells, B-cells expand and differentiate. Depending on the activation site and signals they receive from Tfh cells, some will traffic back to the airways and become local IgA producing plasma cells, while others will switch to IgG and home to the bone marrow to provide systemic protection (40). Notably, in a healthy respiratory tract, IgA is the major immunoglobulin and IgA deficient individuals experience higher rates of respiratory infections (20, 41).
Lastly, regulatory T-cells (Tregs) are tasked with resolution of inflammation upon infection clearance. Their job is crucial in collateral damage control caused by pro-inflammatory immune mechanisms. Originating either from the thymus (natural Tregs) or from conventional CD4+ T-cells differentiated in the periphery (induced Tregs), these cells are potent immune suppressors. They downscale immune activation, kill effector T-cells, limit growth factor availability, and promote tissue repair returning the lung back to homeostasis (42–44).
Pulmonary Immune Dysregulation During HIV Infection
Acute HIV and Lung Immunity
Within a few hours of the transmission event, HIV begins to replicate in mucosal, submucosal, and draining lymphoid tissues. Notably, lung is an early target of HIV dissemination because it is highly vascularized and houses a very large pool of target cells. Experimental SIV-infections of macaques demonstrate that the virus is seeded into the lungs shortly after intravenous infection (45–47). In fact, SIV replication in BAL cells of pigtailed macaques can be detected as early as 7 days post-inoculation, peaking at 10 days during acute infection (46). Interestingly, the CCR5-tropic HIV strain, known as HIV-1Bal, was originally isolated from the lungs (48). Viral quasispecies specific to this tissue and distinct from the circulation have also been reported (49, 50).
Upon reaching the lung, HIV is seeded into multiple cell types: CD4 T-cells, DN T-cells, and AMs. Although HIV preferentially infects CD4 T-cells, which account for ~6% of total BAL cells in healthy non-smokers, AMs, which account for ~85% of total BAL cells, are also infected (27, 51–53). Although data on pulmonary immune perturbations during primary acute HIV infection is scarce, in vitro experiments on human lung lymphocytes and in vivo animal SIV models suggest that during the acute phase of infection pulmonary interstitial CD4 T-cells are more severely and rapidly depleted compared to the blood compartment that is largely due to CCR5+ memory CD4 T-cells’ high susceptibility to CCR5-topic HIV-1 infection, which make up the vast majority of the lung CD4 T-cell pool (54, 55).
Most acute HIV-1 infections are caused by the CCR5-tropic strain (T-tropic strain), which preferentially targets T-cells. Although it’s not very efficient at infecting macrophages on its own, its infectivity is enhanced significantly during cell-to-cell contact between AMs and infected CD4 T-cells, as has been recently shown by Schiff and his group, suggesting that AM HIV entry during acute infection is CD4 T-cell dependent (53). Within the AM cell pool (CD206+) two subsets have been identified based on size (FSC) and granularity (SSC) – small and large AMs (56). Human ex vivo AM analysis shows that HIV preferentially infects monocyte-like small AMs, which show higher pro-inflammatory gene expression and greater phagocytic capacity compared to large AMs (56, 57). In addition to infecting AMs, we have also shown in a humanized mouse model of early HIV infection that the virus is preferentially seeded within lung DN T-cells, a rather novel lung HIV reservoir cell subset, which is enriched in the lungs of both HIV+ and seronegative individuals compared to other tissues (58).
In about two thirds of cases with primary HIV infection, individuals experience flu-like symptoms such as fever, chills, and swollen lymph nodes. Some individuals also suffer from respiratory symptoms such as a sore throat or a dry cough, suggesting engagement of the pulmonary innate immune response (59). Massive CD4 T-cell apoptosis during the acute infection phase is accompanied by profound immune activation, caused by release of apoptotic microparticles into the bloodstream (1, 60). Furthermore, in addition to the attack on human immune cells, HIV also compromises the integrity of the lung epithelial barrier by infecting human bronchial epithelial cells, decreasing their expression of E-cadherin, and increasing paracellular permeability (61, 62).
Untreated Chronic HIV and the Lung Immunity
At this point, viral reservoirs in deep tissues are already well established, including the reservoir in the lung. In fact, SIV-models show that during the asymptomatic phase of infection, there is no correlation between plasma and BAL fluid viral loads (46). This is further supported by human phylogenetic studies, which demonstrate HIV lung reservoir compartmentalization in untreated patients (63, 64). Furthermore, whole lung tissue biopsies of untreated HIV-infected persons harbor distinct viral quasispecies compared to those found in their blood and lymphoid tissues suggesting that the HIV reservoir may be replicating and evolving locally in that anatomical site, rather than solely spreading from the circulation (49, 50).
Prior to introduction of ART, lung disease was the leading cause of death in people living with HIV (PLWH) (65). As seen in the very first AIDS reports of Pneumocystis jirovecii pneumoniae infections in gay men in the 1980s, previously rare fungal pulmonary infections became a staple AIDS-defining illness observed in severely immunosuppressed patients (66, 67). Untreated PLWH are also at a higher risk of developing recurring bacterial pneumonia, whose rate is inversely proportional to the patients’ CD4 T-cell counts (68). They are also more prone to cancer development, such as lung cancer, compared to persons without HIV infection (69, 70).
The dysregulated pulmonary immune environment in PLWH may facilitate the development of such lung pathologies. Destruction of lung parenchyma, pulmonary inflammation, and emphysema are recognized complications of HIV infection. Up to 60% of untreated PLWH present with lymphocytic alveolitis, characterized by infiltration of B-cells, gamma-delta T-cells, as well as CD4+ and CD8+ T-cells into the lung, which is observed in absence of any respiratory symptoms (71–74). Both their pulmonary CD8 and CD4 T-cells show 2- to 3-fold greater expression of HLA-DR and CD38, which are markers of immune activation, compared to seronegative adults (75). Neff and colleagues have also shown that in untreated PLWH with lymphocytic alveolitis, lung HIV-specific CD4+ and CD8+ T-cells show impaired proliferative capacity, which is caused by high expression levels of PD-1, a classic exhaustion marker that dampens T-cell receptor signaling (71, 76). Furthermore, the lung cytokine milieu of PLWH is disrupted. Jambo et al. have shown that BAL fluid taken from ART-naïve HIV+ study participants have increased concentrations of RANTES and TNF-β and a shift towards MIP-1β, MCP-1, and IP-10 signaling network (77). Notably, RANTES is a lymphocyte chemoattractant, shown to a play a role in lung CD8 T-cell recruitment in other viral lung infections. These CD8 T-cells can in turn produce TNF-β, a potent pro-inflammatory cytokine that promotes vascular cell adhesion, chemokine production, and further immune cell infiltration into the tissue (78–80). IL-6 is another pro-inflammatory player, whose levels are associated with higher HIV RNA levels and has repeatedly been shown to be produced by monocytes and macrophages in response to HIV (81, 82). In vivo non-human-primate models further show that IL-6 expression in pulmonary interstitial macrophages of SIV-infected animals is positively correlated with monocyte turnover and lung tissue damage (83). As mentioned previously, HIV has also been shown to impair pulmonary epithelial integrity by decreasing expression of cell-to-cell adhesion molecules and promoting further release of pro-inflammatory mediators by these cells, thus accelerating the decline in lung function (61).
Chronic pulmonary inflammation further leads to increased production of matrix metalloproteinases (MMPs), a family of endopeptidases that can degrade elastin and collagen fibers (84). Notably, both elastin and collagen degradation products act as immune cell chemoattractants: while elastin fragments recruit monocytes, collagen fragments attract neutrophils (85). Collectively, MMPs play a role in tissue repair and modulate the immune response. In vitro experiments on primary human airway basal cells have shown that HIV infection can force these cells to acquire a destructive phenotype via upregulation of MMP-9 through activation of MAPK signaling, thus potentially contributing to emphysema development in PLWH (86).
On the other end of the scale between inflammation and wound repair, HIV leads to higher levels of TGF-β – an anti-inflammatory cytokine produced by Tregs and alveolar macrophages. Because PLWH experience persistent low-grade chronic inflammation, that is in part caused by bacterial translocation across the gastrointestinal mucosal barrier, the immune system tries to counteract it via anti-inflammatory cytokines, such as TGF-β (87, 88). Notably, TGF-β levels are significantly higher in PLWH compared to seronegative individuals and remain elevated regardless of ART treatment and viral load suppression (89). TGF-β can downregulate inflammatory processes by promoting Treg expansion and inhibiting effector T-cell function, as well as drive collagen deposition by fibroblasts as part of the normal wound repair process (90, 91). In PLWH long-term TGF-β elevation may contribute to irreversible tissue fibrosis of the gut, secondary lymphoid organs, and the lung (92–95). One study has also shown higher TGF-β production by AMs from PLWH compared to AMs from healthy donors, which the authors believe to be implicated in impaired IgG secretion in the alveoli (96). AMs from PLWH also show a pro-inflammatory phenotype, higher TNFα production, and impaired phagocytic ability, which in turn leads to poor pathogen clearance (57, 97–99). Untreated HIV infection also leads to loss of anti-inflammatory CD163+CD206+ AMs (100). Furthermore, AMs from SIV infected macaques show elevated levels of PD-1 which positively correlates with plasma viral load, suggesting that higher PD-1 expression on AMs may be associated with disease progression (101).
ART-Treated HIV and Lung Immunity
Although introduction of ART has greatly reduced the rate of opportunistic infections and improved the quality of life of PLWH, it does not fully restore all of immune perturbations caused by HIV infection. Instead, the spectrum of most prevalent pulmonary diseases had shifted from opportunistic infections to chronic illnesses, such as emphysema, chronic obstructive pulmonary disease (COPD), pulmonary fibrosis, and lung cancer which are discussed in more detail in a later section. Notably, although ART had greatly reduced the rate of lung infections in PLWH, they still occur more frequently in HIV infected individuals compared to the general population (7, 102).
Viral control after ART-initiation has a significant impact on lung immunity. Lymphocytic alveolitis resolves, CD8 T-cell numbers decrease, CD4 T-cell count improves, and thus CD4:CD8 T-cell ratio is ameliorated (103, 104). Notably, lung CD4 T-cell repopulation likely occurs due to local expansion of the tissue resident subset, as seen by higher Ki67 expression in BAL CD4 T-cells 1 month after starting therapy (104). Twigg et al. have also demonstrated that level of CD38 and HLA-DR on BAL lymphocytes decrease significantly after the first 6 months of therapy, especially on CD8+ T-cells (105). However, as our team has recently demonstrated, levels of HLA-DR+CD38+ BAL CD4 T-cells remain higher in ART-treated PLWH compared to healthy controls (17).
Twigg and his group also show that intracellular levels of INF-γ, TNF-β, and IL-2 in alveolar CD4+ and CD8+ T-cells, measured after mitogenic stimulation with the superantigen staphylococcal enterotoxin B, decline significantly in ART-treated PLWH (105). Similar reports were made on extracellular levels of inflammatory cytokines in BAL fluid, such as INF-γ, IL-6, and INF-γ inducible chemokines like IP-10 (105–107). In contrast, Knox et al. showed that although BAL CD4 T-cell infection rate decreases with introduction of ART, both CD4 and CD8 T-cell polyfunctional profiles (INF-γ, TNF-α, IL-2) after mitogenic stimulation remain relatively unchanged (104). Importantly, this was not the case in the peripheral blood, where CD8 T-cells showed a marked improvement in polyfunctional cytokine secretion in response to a stimulus (104). These findings further highlight that immune restoration in lung tissue during ART is incomplete, which can help explain ongoing susceptibility of treated PLWH to pulmonary infections (108, 109).
Apart from pro-inflammatory mechanisms, anti-inflammatory immune functions also remain dysregulate during ART. PLWH show higher levels of CD39+CD73+Tregs in their BAL fluid compared to peripheral blood, while no such difference is observed in seronegative controls (17). Importantly, these immunosuppressive cells can act as a double-edged sword. They can help resolve inflammation during acute lung injury, but they are also capable of promoting tumor cell survival, angiogenesis, and fibrosis (110, 111).
AM dysfunction is yet another factor contributing compromised lung immunity in PLWH on ART. Collini et al. have shown that AMs from ART-treated PLWH have defects in microbicidal mechanisms, mediate by HIV’s gp120 protein, which could inhibit macrophage apoptosis induction, caspase activation, and mROS-dependent pneumococcal killing (112). These cells also remain subjected to chronic oxidative stress despite ART. Yeligar et al. have shown that BAL fluid from treated PLWH has higher H2O2 concentration (113). Furthermore, their AMs have lower expression levels of proliferator-activated receptor (PPAR)-γ, an important player in combatting oxidative stress during acute lung injury, higher expression levels of expression of NADPH oxidases, which further promote oxidative stress and inhibit phagocytosis, and higher levels of TGF-β, a big driver of tissue fibrosis (113–116). Impaired AM phagocytic capacity and skewing in polarization has been further emphasized by Akata et al. (117). Using BAL samples from PLWH and healthy controls, they have demonstrated that out of the four macrophage subsets (non-polarized: CD40-, CD163-; M1: CD40+, CD163-; M2: CD40-, CD163+; double-polarized: CD40+, CD163+) the double-polarized subset has the highest phagocytic capacity. Notably, this subset was significantly diminished in HIV+ COPD- individuals, while the non-polarized subset, which had the lowest phagocytic capacity, was enriched (117). The collective effects of HIV on pulmonary inflammation are summarized in Figure 1.
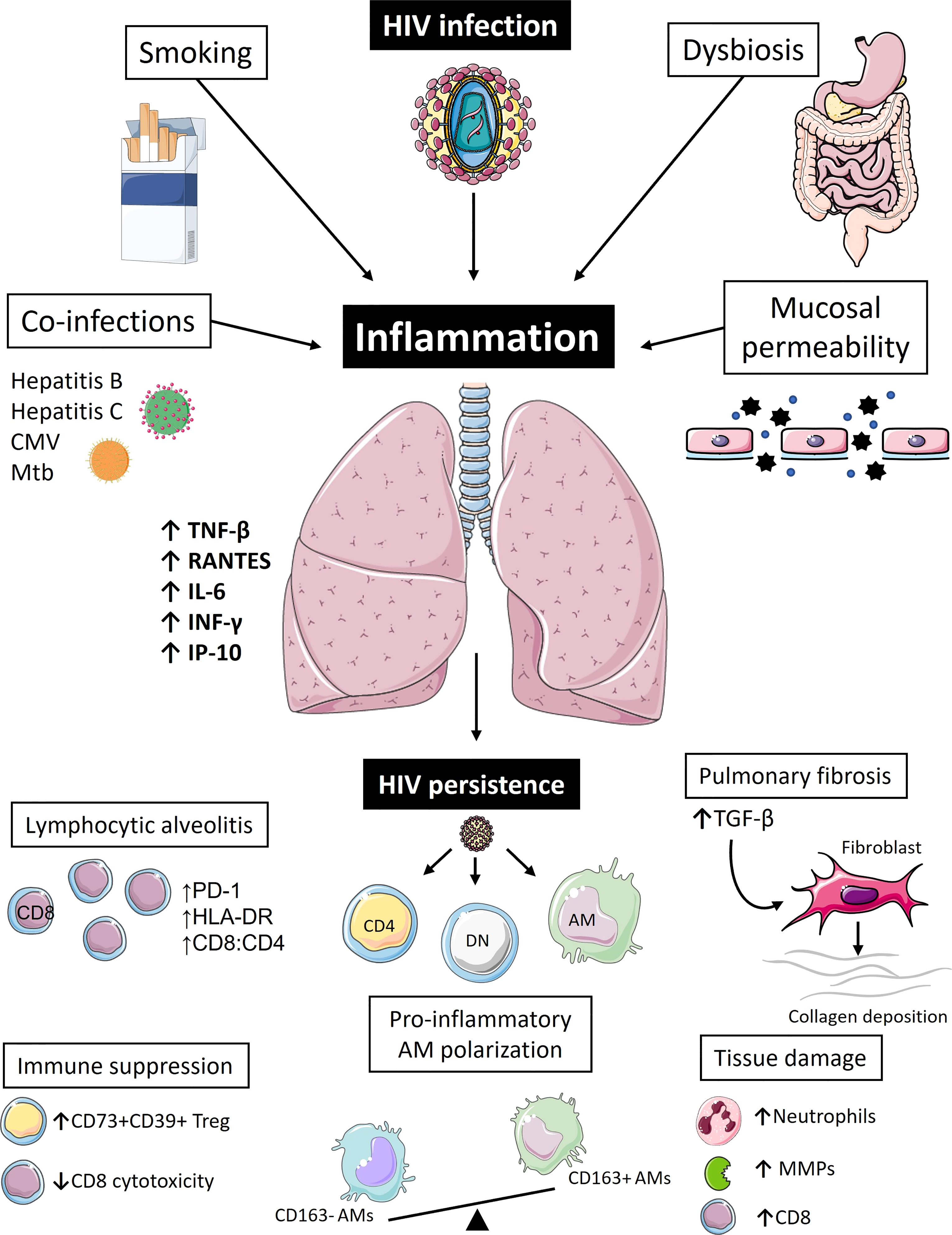
Figure 1 Pulmonary inflammation drives immune dysregulation and reservoir persistence in people living with HIV. Even during the era of antiretroviral therapy (ART), people living with HIV (PLWH) continue to suffer from high burdens of pulmonary illnesses. Inflammation is likely the biggest driver of pulmonary pathologies and lung HIV reservoir persistence in these individuals. Apart from the virus itself, which is seeded into the lung within the first few weeks of infection, other factors also contribute to pulmonary immune perturbations in PLWH even during ART, such as smoking, co-infections, changes in the microbiome, and compromised integrity of mucosal barriers. Collectively, these fuel chronic inflammatory state and pulmonary immune activation characterized by high levels of pro-inflammatory cytokines (RANTES, TNF-β, IFN-γ, IL-6, IP-10), which in turn lead to immune cell recruitment to the lung tissue, typically presenting as CD8 T-cell lymphocytic alveolitis. These CD8 T-cells appear as functionally impaired and fail to remove lung HIV reservoir that continues to persist in mucosal CD4+ T-cells and CD4-CD8- Double Negative (DN) T-cells, as well as alveolar macrophages (AMs). A vicious cycle of immune activation and residual viral replication ensues further exacerbating pulmonary immune abnormalities, such as pro-inflammatory AM polarization, extracellular matrix destruction caused by increased production of matrix metalloproteinases (MMPs), further CD8 T-cell recruitment, and increased neutrophil count. In an attempt to counteract this inflammatory process, immunoregulatory arm of the immune system could further contribute to increased risk of pulmonary co-morbidities: accumulation of immunosuppressive regulatory T-cells (CD73+CD39+Treg) might be implicated in higher lung cancer risk and higher levels of TGF-β could be the primary driver of irreversible pulmonary fibrosis. [Images adapted from Servier Medical Art licensed under CC 3.0 (smart.servier.com)].
ART Toxicity
Interestingly, there are several studies which suggest that ART toxicity might also play a role in immune dysregulation in PLWH. In vitro studies have shown that nucleoside reverse transcriptase inhibitors can decrease mitochondrial DNA content and complement-mediated phagocytosis in human monocyte-derived macrophages (118). In addition, Korencak et al. looked at CD4 T-cells from treated and untreated PLWH, as well as healthy subjects, showed that ART improves these cells’ metabolic phenotype but not the respiratory impairment, especially in patients receiving integrase inhibitors. Furthermore, they show that CD4 T-cells treated with dolutegravir and elvitegravir shift the cytokine response of these cells from a polyfunctional one to a TNF-α dominated one (119). A similar observation was made by Bowman and colleagues, which demonstrate that human macrophages exposed to tenofovir disoproxil fumarate and emtricitabine have lower mitochondrial mass and increased lipid uptake (120). These studies can help explain the observations made by Correa-Macedo et al. showing the potential adverse effect of ART on transcriptional response of AMs to Mycobacterium tuberculosis (Mtb). In their recent study, they have isolated AMs from healthy controls, PLWH on ART, as well as seronegative participants taking pre-exposure prophylaxis (PrEP). Notably, AMs from HIV+ and HIV- PrEP study groups both had a weaker transcriptional response when challenged with Mtb compared to AMs from healthy controls. Furthermore, AMs from HIV- PrEP and HIV+ donors showed no change in chromatin state upon challenge, unlike AMs from healthy controls which displayed a significant change in chromatin accessibility (121). Collectively, these findings suggest that although ART greatly improves the quality of life of PLWH and does restore many of their immune parameters it does not come without side effects. Some regimens may decrease mitochondrial function and elicit a pro-inflammatory immune cell profile, thus partially contributing to chronic inflammation in treated individuals.
Accelerated Pulmonary Co-Morbidities During HIV Infection
Accelerated Immune Aging
Many age-related co-morbidities occur in PLWH at a younger age than in the general population. This premature onset of age-related illnesses has been largely attributed to chronic inflammation and immune activation, which in turn leads to accelerated immune aging, now known as ‘inflammageing’ (4, 122). Inflammageing is characterized by high level of circulating pro-inflammatory cytokines (IL-6, IL-8, TNF-α, and IFN-γ), telomere shortening, cell senescence (loss of CD28 and increased expression of CD57 and KLRG1 on T-cells), mitochondria dysfunction, and changes in microbial composition of the microbiota, all of which have been documented in PLWH (122–125). Consequently, this accelerated immune aging affects the lungs. Leung et al. had conducted several studies on telomere shortening in PLWH (126, 127). They have shown that telomeres in circulating leukocytes of PLWH are significantly shorter than in seronegative study participants. Furthermore, their telomere length correlated with severity of poor lung function determined by forced expiratory volume (126). Notably, in their more recent publication, which included HIV+ participants with good immune recovery and undetectable viral loads, the telomere length of their small airway epithelial cells was significantly shorter than in healthy controls, even after accounting for cigarette smoke exposure (127). Moreover, HIV+ participants in that study were on average 4 years younger than the seronegative ones.
HIV and COPD
COPD is a progressive inflammatory lung condition characterized by airway obstruction, inflamed mucous membranes and alveolar damage and is the third leading causes of death worldwide with 2 million people affected in Canada alone (128, 129). Both smoking and HIV are independent risk factors for COPD development (130–132). Furthermore, COPD has often been proposed to be a disease caused by accelerated immune aging (133, 134). A recently published study conducted by Córdoba-Lanús et al. monitored telomere length of patients with COPD over a 10 year period and found an association between accelerated telomere shortening and progressive decline in lung function, such as worsening of gas exchange and lung hyperinflation in COPD patients (135).
HIV-associated COPD also further dysregulates lung immune cell function. Popescu et al. have documented that HIV+COPD+ individuals show severe CD4 T-cell loss in their BAL fluid, mediated by Fas-dependent activation-induced cell death. Their BAL CD4 T-cells also show poor HIV-specific immune response and loss of polyfunctionality compared to HIV+COPD− participants (136). Curiously, unlike lung mucosal CD4 T-cells, BAL CD8 T-cells in these individuals maintained their HIV-specific function. Moreover, increased CD8 T-cell cytotoxicity has been documented previously in COPD patients, which might contribute to this condition’s highly tissue destructive phenotype (137).
HIV and Lung Cancer
Lung cancer is yet another leading cause of death in PLWH (138). Notably, HIV and COPD are both risk factors for lung cancer development even after accounting for smoking status (139–141). Increased lung cancer risk in PLWH has been attributed to acute inflammatory insults caused by lung infections, chronic low-grade inflammation, CD8 T-cell dysregulation, compromised integrity of pulmonary epithelium, and changes in lung microbiome (142–145). Accelerated immune aging may also play a role. Klugman and colleagues have shown that in the United States PLWH were diagnosed with non-small cell lung cancer at a younger age compared to seronegative participants, with lower median survival time especially among those with a low CD4/CD8 ratio and high viral loads (146). Exhausted CD8 T-cells likely contribute to worse lung cancer outcomes in PLWH, especially in those with multiple co-infections which exacerbate this exhausted phenotype further (147, 148). Given the importance of these cells in fighting cancer, their exhaustion leads to decreased responsiveness to stimuli, low cytotoxicity, poor IFN-γ secretion, and thus compromised ability to kill tumour cells (149–151).
HIV and Pulmonary Fibrosis
The high rates of pulmonary fibrosis in PLWH compared to those in the general population could be attributed to increased TGF-β levels (95, 152). TGF-β levels are significantly higher in PLWH compared to seronegative individuals and remain elevated regardless of ART treatment and viral load suppression (89). Because TGF-β can drive collagen deposition by fibroblasts it has been implicated in irreversible tissue fibrosis of the gut, secondary lymphoid organs, and the lung in PLWH (92–95). A 2017 multi-center Lung-HIV study has shown that fibrotic lung changes have been observed in 29% of HIV-infected participants, which correlated with viral load but not ART treatment status or CD4 T-cell count (95). Of note, high levels of TGF-β in PLWH might contribute to poor non-small lung cancer outcomes, as it has already been documented in seronegative individuals, although a direct link between TGF-β levels in PLWH and lung cancer outcomes is yet to be established (96, 153–155).
HIV and Pulmonary Emphysema
In contrast to pulmonary fibrosis, emphysema is characterized by higher lung compliance, increased lung volume and lower expiratory flow rate (156). Increased risk of PLWH of pulmonary emphysema was reported as early as the 1980s (157). More recent studies further confirmed that HIV status is a risk factor for emphysema development independently of smoking (158, 159). Some of the mechanisms in PLWH that likely contribute to this risk have been mentioned previously. These include CD8 T-cell accumulation, increased oxidative stress, AM activation, and increased production of MMPs caused by chronic lung inflammatory state, which can subsequently lead to extracellular matrix destruction (85, 86, 159). Attia et al. have further demonstrated that participants in their HIV+ study group were more likely to have a greater portion of their lung to be affected and were more likely to be diagnosed with COPD compared to seronegative controls diagnosed with emphysema. Additionally, in their HIV+ study arm, low CD4 T-cell counts and high soluble CD14 levels were linked with disease severity, supporting the notion that immune activation in PLWH contributes to the risk of pulmonary emphysema development (158). Whether ART initiation decreases the risk of emphysema development remains rather unclear. Emphysema rates in the pre-ART era were reported to be 15% in PLWH versus 2% in the general population (160). In ART-treated patients, the reported incidence rates are even higher. Guaraldi et al. have reported that, of 1,446 HIV-infected patients on ART in their cohort, nearly 50% had evidence for emphysema and/or bronchiolitis based on thoracic computed tomography (CT) scans, with 13% showing signs of bronchiolitis, 19% showing emphysema, and 16% having both (161). Furthermore, among ART-treated HIV-infected participants recruited by Leung et al., emphysema progression was not associated with peripheral CD4 cell counts or CD4:CD8 ratio, HIV viral load, ART classes or duration of ART exposure (162). As proposed by others, the increase in incidence rate of chronic inflammatory conditions in the ART era, of not just emphysema and COPD but also diabetes and cardiovascular disease, can largely be explained by improved life expectancies in treated PLWH, giving them more time to develop these co-morbidities (163, 164).
Role of Smoking in Pulmonary Co-Morbidities
In a nationwide population-based cohort study conducted, Helleberg and others reported that both all-cause and non-AIDS-related mortalities are higher among HIV+ smokers compared with HIV+ non-smokers. They also highlight that smoking PLWH lose more life-years to smoking than to HIV itself (12.3 years versus 5.1 years respectively) (165). Furthermore, they show that smoking-associated risk of death was 61% among PLWH compared to 34% among healthy controls. Notably, risk of death among former smokers was reduced by 40% compared to current smokers. Around 70% of myocardial infarctions and 27% of cancers in PLWH are related to their smoking status (166). Importantly, unlike with myocardial infarction risk, cancer risk could remain elevated in former smokers even 5 years after smoking cessation (166).
Smoking further increases the risk of COPD and emphysema in PLWH (159, 167). Notably, pulmonary emphysema is more prevalent in HIV+ smokers compared to seronegative smokers and is often developed at a younger age, which could be partially attributed to immune dysregulation of AMs in PLWH (159). Indeed, previous ex vivo human studies show an increase in MMP expression in both AMs and epithelial lining fluid in HIV positive smokers with early emphysema compared to HIV negative smokers with the same lung condition (168). Previous reports also show that smoking can activate cytotoxic CD8 T-cells, which can in turn exacerbate pulmonary injury (169–171).
Lungs as an HIV Reservoir
Lungs Provide Ideal Grounds for HIV Spread
As organs, the lungs possess several features that may contribute to HIV reservoir establishment, several of which stem from their anatomy. Similarly to the gastrointestinal tract, a confirmed and well-studied HIV reservoir site (172), the lungs are an extension of the external environment. They are constantly exposed to external particles and airborne microorganisms. The antigen load in the lungs is therefore quite high. Furthermore, although lymphocytes make up only 10% of the BAL cell pool, the lymphocyte count in pulmonary interstitium is comparable to that of peripheral blood, with as many as 10 X 109 cells (7). High antigen load can in turn promote activation of these lymphocytes and other immune cells, consequently supporting HIV replication and continuous reservoir replenishment in the lung.
Because the lungs carry out the vital function of gas exchange, they are highly vascularized. Their high blood flow, cell proximity, surface area, and small arteriole size could further aid in HIV cell to cell spread and reservoir compartmentalization (7). Furthermore, the lungs of HIV-infected persons harbor distinct viral quasispecies that are tissue specific suggesting that HIV reservoir may be replicating locally in that anatomical site, rather than spreading from other infected tissues (49, 50).
HIV Persists in Multiple Lung Immune Cell Types
Early viral presence in the lung, along with high rate of co-morbidities caused by lung diseases in PLWH even after ART initiation, support the notion that lungs serve as another anatomical HIV reservoir. Our team previously assessed HIV persistence in the pulmonary milieu in individuals under long-term treatment (median 9 years) (17). We found that total HIV DNA in BAL CD4 T-cells was significantly higher than in peripheral blood mononuclear cells. Moreover, the lungs were enriched in activated memory CD4+ T-cells subsets that can further promote HIV replication and persistence (173). We also observed that pulmonary mucosal DN T cells of PLWH on ART expressed higher levels of HLA-DR and several cellular markers associated with HIV persistence (CCR6, CXCR3, and PD-1) compared to the blood (58). Importantly, CD3+CD4-CD8- DN T-cells from the BAL fluid of these participants harbored HIV DNA. Using the humanized bone marrow-liver-thymus mouse model, our group also observed higher infection frequencies of lung DN T-cells than those of the blood and spleen in both early and late HIV infection stages, meaning that apart from AMs and CD4 T-cells, HIV is also seeded in pulmonary mucosal DN T-cells early following infection and persists in these potential cellular HIV reservoirs even during long-term ART (58).
AMs pose yet a bigger challenge on our way to HIV reservoir eradication due to their abundance, longevity, and resistance to apoptosis (112, 174). As alluded to previously, HIV viral proteins remain detectable in BAL fluid of treated HIV-1 infected patients, as Collini and his group have demonstrated, which further underscores the role that AMs serve as HIV cellular reservoirs in the lung despite ART (112). Clayton and colleagues also show that HIV-infected macrophages are resistant to CD8 T-cell mediated killing, even more so than HIV-infected CD4 T-cells, which is associated with increased pro-inflammatory cytokine production (175). The relative importance of the lungs as viral reservoirs has also been highlighted by Horiike et al. in an SIV-infected Rhesus macaque model, where they found that the lungs and intestines of ART-treated animals had the largest burdens of SIV RNA, second to the lymphatic tissues (176). HIV persistence in the lung during ART has been further confirmed by Santangelo’s group using antibody-targeted positron emission tomography – a real-time, in vivo viral imaging method, showing that although lung viral signals are reduced after ART initiation they still remain detectable (177).
Lung CD8 T-Cells Show Poor HIV-Specific Response
CD8 T-cells play a key role in clearance of virus-infected cells. Once differentiated into cytotoxic T-lymphocytes, they acquire several immunological effector functions. Their arsenal includes cytokines (IFN-γ, TNF-α, IL-2), cytotoxic granules containing perforin and granzymes, and the Fas ligand (178). These allow CD8 T-cells to kill infected target cells, activate and recruit phagocytes, and mediate pro-inflammatory processes, thus orchestrating and modulating the immune response (179, 180). As mentioned in the previous sections, chronic inflammatory environment and antigen stimulation leads impaired CD8 T-cell function in lungs of PLWH, rendering them susceptible to opportunistic infections. Although some of the effector functions of CD8 T-cells in the peripheral blood do recover after ART initiation, this is not the case for the CD8 T-cells in the lungs (181, 182). Moreover, elevation in CD8/CD4 ratio contributes to non-AIDS-related morbidity (183). These cells might also induce excessive expansion of other CD8 T-cells in the vicinity via T-cell receptor independent mechanisms, known as “bystander activation” (184, 185). Excessive expansion and immune activation consequently lead to accumulation of these functionally impaired CD8 T-cells displaying reduced proliferation, poor effector functions, and high expression of inhibitory receptors, such as PD-1, in the lung (184, 186, 187).
Impaired CD8 T-cell function may further contribute to lung reservoir persistence. Several studies have shown that these cells are required for HIV infection control. Once HIV-specific CD8 T-cells rise during acute infection, peak viremia begins to subside, meaning that these cells play a crucial part in viral control during primary infection (188, 189). Furthermore, SIV-infected Rhesus Macaques whose CD8 T-cells have been depleted, show increased plasma viremia, which is reversible with CD8 T-cell repopulation (190). Moreover, HIV mutants that can escape the CD8 T-cell response appear early during infection and persist, further demonstrating that there is a strong evolutionary pressure posed on the virus yielding CD8 T-cell escape highly advantageous (191, 192). In a recent study, our team has demonstrated that pulmonary CD8 T-cells show lower perforin expression ex vivo compared with blood CD8 T-cells, regardless of HIV or smoking status (193). Pulmonary CD8 T cells also showed significantly lower in vitro degranulation ability and less effective HIV-specific CD4 killing capacity than blood CD8 T cells, potentially contributing to a suboptimal anti-HIV immune response within the lungs.
Interplay Between Chronic Inflammation and Viral Persistence Within the Lungs
Persistent Lung Immune Dysfunction and HIV Persistence
Residual inflammation in PLWH is one of the biggest contributors to immune dysfunction, chronic co-morbidities, and replenishment of the viral reservoir even on ART. Several mechanisms have been put forth to explain HIV reservoir persistence after many years of ART treatment: poor ART penetration into deep tissues, residual replication, persistent cell stimulation due to residual antigen load, and CD8 T-cell exhaustion (194–196). Residual replication is of particular importance as it can create a self-perpetuating cycle where some of the virus will continue replicating, increasing viral antigen load locally (197, 198). In turn, this antigen load will stimulate nearby immune cells, activating their transcriptional machinery, which will produce more virus if that cell harbors intact and inducible viral DNA.
Studies have shown that the size of the viral reservoir is associated with residual levels of immune activation in ART-treated PLWH. Levels of both cell-associated RNA and proviral DNA have been positively correlated with frequencies of activated (CD38+HLA-DR+) and exhausted (PD-1+) CD4+ and CD8+ T cells (199, 200). Although most of these studies are typically done in the blood, the lungs of PLWH show a similar phenomenon. All lung T-cells show high frequencies of activation (HLA-DR) (17, 58, 193). Lung CD4 T-cells from treated PLWH also show higher frequencies of senescent cells (CD57), while CD8+ and double negative T-cells show high expression of PD-1 (17, 58, 193). These high levels of immune activation likely contribute to lung HIV reservoir persistence under ART, which our team has demonstrated to be larger in BAL CD4 T-cells compared to the blood (17). They also underline the presence of HIV-infected AMs in BAL fluid from PLWH on ART, which could induce chronic activation of the innate immune response in that tissue and contribute to T-cell dysfunction, as has already been shown in monocyte-derived macrophages (97, 201). Furthermore, Collini and colleagues were able to detect residual levels of HIV viral protein gp120 in BAL fluid of HIV-1-seropositive donors with median ART treatment time of 75 months (112). This finding is of particular importance, because the ability to detect HIV viral proteins in PLWH who have been on ART for over 6 years suggests that there is ongoing residual viral replication in that tissue that contributes to chronic pulmonary inflammation. In turn, chronic inflammation will consequently fuel HIV reservoir maintenance either through active viral replication or through proliferating latently infected cells, both of which are promoted by inflammatory environment, creating a vicious cycle that ensures HIV reservoir renewal (202–204).
Dysbiosis of the Gut-Lung Axis Fuel Inflammation
Impaired lung immune function and HIV reservoir expansion are likely amplified through exacerbated inflammation caused by microbial dysbiosis in the lung and gut of PLWH (145, 205–208). Although the gastrointestinal and respiratory tracts are separate organs, they share a common mucosal immune system known as the gut–lung axis (209). Disruption of the gut microbiota has been linked with pulmonary diseases such as asthma and COPD (210, 211). Similarly, a well-balanced gut microbiome can help the lung immune system fight viral respiratory infections (212). Importantly, the lung and gut microbiomes do not exist in isolation from each other and prolonged immune activation, as seen in PLWH, affects both of them.
In PLWH, chronic inflammation isn’t just fueled by residual infection but also by damaged mucosal barriers and bacterial translocation, especially in the gut, which can further promote immune activation and HIV reservoir expansion in the lung. It is well known that HIV seeds into the gut within the first few weeks of infection and aggressively depletes memory CD4 T-cells in that tissue (213, 214). This is then followed by tight junction disruption between intestinal epithelial cells, leading to increased permeability, also known as a ‘leaky gut’, which might not be fully reversed with ART (215–217). This in turn leads to gastrointestinal dysbiosis and translocation of bacterial products, such as lipopolysaccharides, into the circulation leading to systemic innate immune activation (218–220). Notably, systemic markers of inflammation and innate defense have been associated with decreased lung function and pulmonary abnormalities in PLWH (221). Alterations in the pulmonary microbiome prior to ART initiation include decreased richness (α diversity) and increased change in species (β diversity) of microbial communities, as well as increased abundance of Streptococcus and Tropheryma whipplei in HIV-infected subjects compared to uninfected controls (144, 222). Although dysbiosis improve after treatment initiation, the lung microbiome is not always fully normalized even after effective ART. Levels of Veillonella, for instance, remain elevated in the lungs of PLWH even after 3 years of ART. Notably, Veillonella outgrowth has been previously associated with pulmonary inflammation in COPD patients characterized by higher lymphocyte and neutrophil count in BAL fluid and increased levels of exhaled nitric oxide (145, 223).
Role of Smoking and Respiratory Co-Infections in HIV Persistence Within the Lungs
Smoking and co-infections further exacerbate pulmonary inflammation and thus could promote lung HIV reservoir persistence. Several research groups have shown that common co-infections in PLWH, such as hepatitis B, hepatitis C, and cytomegalovirus, are associated with higher circulating LPS levels, increased CD8 T-cell activation, and accelerated immunologic aging (224–226). Mtb infections, are especially detrimental to HIV-infected individuals. According to UNAIDS, PLWH are 19 times more likely to fall ill with tuberculosis (TB). In 2018, around 1.5 M people died from the disease, 251 000 of which died from TB that was AIDS-related, making it the leading cause of death in PLWH (227, 228). Notably, the risk of TB stays 4-fold higher in treated HIV+ individuals, despite ART and both diseases are characterized by chronic inflammation caused by failure to clear either pathogen (229). Importantly, in vitro studies show that macrophage infection with HIV-1 leads to impairment in IL-10 secretion in response to subsequent Mtb challenge, which is further confirmed by low IL-10 levels and high IL-1β levels in PLWH with tuberculosis, meaning that HIV can exacerbate pulmonary inflammation during TB infection even during ART. Furthermore, PLWH co-infected with Mtb have increased lung HIV viral load, and increased systemic HIV heterogeneity likely because more CD4 T-cells are recruited to pulmonary granulomas, which leads to accumulation of highly permissive HIV target cells, thus facilitating viral replication and cell to cell spread (230–233).
Smoking, which is highly prevalent in HIV-infected population, has also been shown to promote inflammatory immune environment (234). Neff and colleagues have recently documented increased expression levels CCR2, TLR4, CXCR4, and program death ligand 1 (PD-L1) by AMs in smokers regardless of HIV infection status (100). Importantly, higher TLR4 and CXCR4 levels were statistically significant only in HIV-infected smokers but not in seronegative nonsmokers and HIV+ nonsmokers, suggesting an additive inflammatory effect of HIV and smoking on AMs. They also highlight that the effect of CXCR4 upregulation on AMs in smokers should not be overlooked, since its upregulation in other contexts can lead to increased viral entry of X4-tropic HIV virus in vitro and increased viral evolution in vivo (100, 235).
HIV and SARS-CoV-2 Infection
In the face of a pandemic, many contradictory statements have been made over the past two years regarding the relationship between HIV and SARS-CoV-2 infection. While some groups suggest that PLWH are more susceptible to SARS-CoV-2 infection and poor disease outcomes, other groups suggest that their immune suppression may prevent cytokine storm onset (236–241). Some groups even report that they do not see a significant difference in hospitalization rates or adverse outcomes caused by SARS-CoV-2 between PLWH and the general population (242, 243). In a meta-analysis, Ssentongo et al. examined studies published between January and December of 2020 and reported that the proportion of PLWH among SARS-CoV-2 infected patients in the cities was double compared to that found in the general population (244). Furthermore, they found that the risk of death in PLWH with SARS-CoV-2 infection was 80% greater than in HIV negative patients (244). However, the relationship between HIV and SARS-CoV-2 infection is challenging to decorticate due to many confounding factors, including multiple vulnerabilities and more often belonging to groups disproportionately affected by the pandemic than people without HIV infection (245).
Greater risk of SARS-CoV-2 infection and complications could be explained, in part, by accelerated immune aging and higher rates of co-morbidities in the HIV-infected population. Many of these co-morbidities, such as cardiovascular disease, diabetes, and cancer, are considered to be risk factors for severe SARS-CoV-2 infection outcomes independently of HIV infection (246, 247). Furthermore, given the immune perturbations of both innate and adaptive immune cells of the pulmonary mucosa, higher levels of pro-inflammatory cytokines, along with increased susceptibility of PLWH to respiratory infections, it is not surprising that they are also more likely to be infected with SARS-CoV-2 and suffer from a severe form of infection. Lastly, ART-discontinuation was also reported to be a risk factor for SARS-CoV-2 infection among PLWH, further stressing the importance of treatment-adherence in these patients (248). Importantly, preliminary data suggest that some PLWH, and especially those with low CD4 T-cell count below 250 cells/mm3 or detectable viral load, may exhibit a weaker humoral immune response to SARS-CoV-2 vaccination than persons without HIV infection, although results are mixed (249–251). Poor vaccine response in the setting of poorly controlled HIV or suboptimal CD4 T-cell recovery is well-recognized for many other pathogens, including pneumococcal pneumonia and influenza (252–255). The effects of SARS-CoV-2 on the lung HIV reservoir remain largely unknown. It is plausible that SARS-CoV-2-induced immune activation, similarly to what has been reported in PLWH with tuberculosis and other pulmonary infections, could stimulate viral replication and clonal expansion of HIV-infected cells but no studies have yet been published to support or refute this hypothesis (229, 256).
Conclusion
In summary, ART does not fully restore lung immunity in PLWH, who continue to suffer from high burdens of pulmonary illnesses. Many different factors contribute to pulmonary immune perturbations in PLWH even during ART. Inflammation is the biggest driver of pulmonary pathologies and lung HIV reservoir persistence in these individuals. High blood flow, large pool of target cells, close cell-to-cell proximity, and small arteriole size likely contribute to lung HIV infection and spread. Furthermore, because the lung mucosa is continuously exposed to a large number of airborne antigens, these could further stimulate residual HIV replication and proliferation of infected cells. A true functional HIV cure will likely need to target many different factors to subdue both systemic and pulmonary inflammation such as promoting a healthy lung and gut microbiota, restoring epithelial integrity of the mucosal barriers, reducing microbial translocation, and controlling other co-infections.
Author Contributions
YA wrote the first draft and constructed the figure. CC participated in the discussion and critically read and edited the manuscript. M-AJ designed the review and critically revised the manuscript. All authors contributed to the article and approved the submitted version.
Funding
This work was funded by the Canadian Institutes of Health Research (CIHR) (grant 153082) and by CIHR-funded Canadian HIV Cure Enterprise (CanCURE) Team Grant HB2—164064, and in part by the Réseau SIDA et maladies infectieuses du Fonds de recherche du Québec-Santé (FRQ-S) to CC and M-AJ. YA is recipient of MSc scholarship from the FRQ-S. CC holds an FRQ-S Junior 2 Clinician-researcher salary award. M-AJ holds the CIHR Canada Research Chair tier 2 in Immuno-Virology.
Conflict of Interest
The authors declare that the research was conducted in the absence of any commercial or financial relationships that could be construed as a potential conflict of interest.
Publisher’s Note
All claims expressed in this article are solely those of the authors and do not necessarily represent those of their affiliated organizations, or those of the publisher, the editors and the reviewers. Any product that may be evaluated in this article, or claim that may be made by its manufacturer, is not guaranteed or endorsed by the publisher.
References
1. McMichael AJ, Borrow P, Tomaras GD, Goonetilleke N, Haynes BF. The Immune Response During Acute HIV-1 Infection: Clues for Vaccine Development. Nat Rev Immunol (2010) 10(1):11–23. doi: 10.1038/nri2674
2. Paiardini M, Müller-Trutwin M. HIV-Associated Chronic Immune Activation. Immunol Rev (2013) 254(1):78–101. doi: 10.1111/imr.12079
3. Schouten J, Wit FW, Stolte IG, Kootstra NA, van der Valk M, Geerlings SE, et al. Cross-Sectional Comparison of the Prevalence of Age-Associated Comorbidities and Their Risk Factors Between HIV-Infected and Uninfected Individuals: The AGEhIV Cohort Study. Clin Infect Dis An Off Publ Infect Dis Soc America (2014) 59(12):1787–97. doi: 10.1093/cid/ciu701
4. Guaraldi G, Orlando G, Zona S, Menozzi M, Carli F, Garlassi E, et al. Premature Age-Related Comorbidities Among HIV-Infected Persons Compared With the General Population. Clin Infect Dis (2011) 53(11):1120–6. doi: 10.1093/cid/cir627
5. Hunt PW, Lee SA, Siedner MJ. Immunologic Biomarkers, Morbidity, and Mortality in Treated HIV Infection. J Infect Dis (2016) 214 Suppl 2(Suppl 2):S44–50. doi: 10.1093/infdis/jiw275
6. Triplette M, Justice A, Attia EF, Tate J, Brown ST, Goetz MB, et al. Markers of Chronic Obstructive Pulmonary Disease Are Associated With Mortality in People Living With HIV. AIDS (Lond Engl) (2018) 32(4):487–93. doi: 10.1097/qad.0000000000001701
7. Costiniuk CT, Jenabian M-A. The Lungs as Anatomical Reservoirs of HIV Infection. Rev Med Virol (2014) 24(1):35–54. doi: 10.1002/rmv.1772
8. Shiels MS, Cole SR, Kirk GD, Poole C. A Meta-Analysis of the Incidence of non-AIDS Cancers in HIV-Infected Individuals. J Acquired Immune Deficiency Syndromes (1999) (2009) 52(5):611–22. doi: 10.1097/QAI.0b013e3181b327ca
9. Kirk GD, Merlo C, O' Driscoll P, Mehta SH, Galai N, Vlahov D, et al. HIV Infection Is Associated With an Increased Risk for Lung Cancer, Independent of Smoking. Clin Infect Dis An Off Publ Infect Dis Soc America (2007) 45(1):103–10. doi: 10.1086/518606
10. Head BM, Trajtman A, Rueda ZV, Vélez L, Keynan Y. Atypical Bacterial Pneumonia in the HIV-Infected Population. Pneumonia (2017) 9(1):12. doi: 10.1186/s41479-017-0036-z
11. Cohen C, Simonsen L, Sample J, Kang J-W, Miller M, Madhi SA, et al. Influenza-Related Mortality Among Adults Aged 25–54 Years With AIDS in South Africa and the United States of America. Clin Infect Dis (2012) 55(7):996–1003. doi: 10.1093/cid/cis549
12. Getahun H, Gunneberg C, Granich R, Nunn P. HIV Infection—Associated Tuberculosis: The Epidemiology and the Response. Clin Infect Dis (2010) 50(Supplement_3):S201–S7. doi: 10.1086/651492
13. Blankson JN, Persaud D, Siliciano RF. The Challenge of Viral Reservoirs in HIV-1 Infection. Annu Rev Med (2002) 53(1):557–93. doi: 10.1146/annurev.med.53.082901.104024
14. Clapham PR, McKnight Á. HIV-1 Receptors and Cell Tropism. Br Med Bull (2001) 58(1):43–59. doi: 10.1093/bmb/58.1.43
15. Alexaki A, Liu Y, Wigdahl B. Cellular Reservoirs of HIV-1 and Their Role in Viral Persistence. Curr HIV Res (2008) 6(5):388–400. doi: 10.2174/157016208785861195
16. Avettand-Fenoel V, Hocqueloux L, Ghosn J, Cheret A, Frange P, Melard A, et al. Total HIV-1 DNA, a Marker of Viral Reservoir Dynamics With Clinical Implications. Clin Microbiol Rev (2016) 29(4):859–80. doi: 10.1128/cmr.00015-16
17. Costiniuk CT, Salahuddin S, Farnos O, Olivenstein R, Pagliuzza A, Orlova M, et al. HIV Persistence in Mucosal CD4+ T Cells Within the Lungs of Adults Receiving Long-Term Suppressive Antiretroviral Therapy. AIDS (Lond Engl) (2018) 32(16):2279–89. doi: 10.1097/qad.0000000000001962
18. Simon V, Ho DD, Abdool Karim Q. HIV/AIDS Epidemiology, Pathogenesis, Prevention, and Treatment. Lancet (2006) 368(9534):489–504. doi: 10.1016/S0140-6736(06)69157-5
19. Effros RM. Anatomy, Development, and Physiology of the Lungs. GI Motil Online (2006). doi: 10.1038/gimo73
20. Kato A, Hulse KE, Tan BK. Schleimer RP. B-Lymphocyte Lineage Cells and the Respiratory System. J Allergy Clin Immunol (2013) 131(4):933–57. doi: 10.1016/j.jaci.2013.02.023
21. Hewitt RJ, Lloyd CM. Regulation of Immune Responses by the Airway Epithelial Cell Landscape. Nat Rev Immunol (2021) 21(6):347–62. doi: 10.1038/s41577-020-00477-9
22. Koval M. Claudin Heterogeneity and Control of Lung Tight Junctions. Annu Rev Physiol (2013) 75(1):551–67. doi: 10.1146/annurev-physiol-030212-183809
23. Linden SK, Sutton P, Karlsson NG, Korolik V, McGuckin MA. Mucins in the Mucosal Barrier to Infection. Mucosal Immunol (2008) 1(3):183–97. doi: 10.1038/mi.2008.5
24. Laube DM, Yim S, Ryan LK, Kisich KO, Diamond G. Antimicrobial Peptides in the Airway. In: Shafer WM, editor. Antimicrobial Peptides and Human Disease. Berlin, Heidelberg: Springer Berlin Heidelberg (2006). p. 153–82.
25. Iwasaki A, Foxman EF, Molony RD. Early Local Immune Defences in the Respiratory Tract. Nat Rev Immunol (2017) 17(1):7–20. doi: 10.1038/nri.2016.117
26. Aghasafari P, George U, Pidaparti R. A Review of Inflammatory Mechanism in Airway Diseases. Inflamm Res (2019) 68(1):59–74. doi: 10.1007/s00011-018-1191-2
27. Heron M, Grutters JC, ten Dam-Molenkamp KM, Hijdra D, van Heugten-Roeling A, Claessen AME, et al. Bronchoalveolar Lavage Cell Pattern From Healthy Human Lung. Clin Exp Immunol (2012) 167(3):523–31. doi: 10.1111/j.1365-2249.2011.04529.x
28. Guilliams M, De Kleer I, Henri S, Post S, Vanhoutte L, De Prijck S, et al. Alveolar Macrophages Develop From Fetal Monocytes That Differentiate Into Long-Lived Cells in the First Week of Life. Via GM-CSF J Exp Med (2013) 210(10):1977–92. doi: 10.1084/jem.20131199
29. Gomez Perdiguero E, Klapproth K, Schulz C, Busch K, Azzoni E, Crozet L, et al. Tissue-Resident Macrophages Originate From Yolk-Sac-Derived Erythro-Myeloid Progenitors. Nature (2015) 518(7540):547–51. doi: 10.1038/nature13989
30. Hussell T, Bell TJ. Alveolar Macrophages: Plasticity in a Tissue-Specific Context. Nat Rev Immunol (2014) 14(2):81–93. doi: 10.1038/nri3600
31. Wang J, Li F, Tian Z. Role of Microbiota on Lung Homeostasis and Diseases. Sci China Life Sci (2017) 60(12):1407–15. doi: 10.1007/s11427-017-9151-1
32. Sadler AJ, Williams BRG. Interferon-Inducible Antiviral Effectors. Nat Rev Immunol (2008) 8(7):559–68. doi: 10.1038/nri2314
33. Braciale TJ, Sun J, Kim TS. Regulating the Adaptive Immune Response to Respiratory Virus Infection. Nat Rev Immunol (2012) 12(4):295–305. doi: 10.1038/nri3166
34. Hwang JY, Randall TD, Silva-Sanchez A. Inducible Bronchus-Associated Lymphoid Tissue: Taming Inflammation in the Lung. Front Immunol (2016) 7:258(258). doi: 10.3389/fimmu.2016.00258
35. Halle S, Dujardin HC, Bakocevic N, Fleige H, Danzer H, Willenzon S, et al. Induced Bronchus-Associated Lymphoid Tissue Serves as a General Priming Site for T Cells and Is Maintained by Dendritic Cells. J Exp Med (2009) 206(12):2593–601. doi: 10.1084/jem.20091472
36. Kim TS, Braciale TJ. Respiratory Dendritic Cell Subsets Differ in Their Capacity to Support the Induction of Virus-Specific Cytotoxic CD8+ T Cell Responses. PloS One (2009) 4(1):e4204. doi: 10.1371/journal.pone.0004204
37. Moltedo B, Li W, Yount JS, Moran TM. Unique Type I Interferon Responses Determine the Functional Fate of Migratory Lung Dendritic Cells During Influenza Virus Infection. PloS Pathog (2011) 7(11):e1002345. doi: 10.1371/journal.ppat.1002345
38. Annunziato F, Romagnani C, Romagnani S. The 3 Major Types of Innate and Adaptive Cell-Mediated Effector Immunity. J Allergy Clin Immunol (2015) 135(3):626–35. doi: 10.1016/j.jaci.2014.11.001
39. Weaver CT, Harrington LE, Mangan PR, Gavrieli M, Murphy KM. Th17: An Effector CD4 T Cell Lineage With Regulatory T Cell Ties. Immunity (2006) 24(6):677–88. doi: 10.1016/j.immuni.2006.06.002
40. Kiyono H, Fukuyama S. NALT- Versus PEYER'S-Patch-Mediated Mucosal Immunity. Nat Rev Immunol (2004) 4(9):699–710. doi: 10.1038/nri1439
41. Johansen F-E, Baekkevold ES, Carlsen HS, Farstad IN, Soler D, Brandtzaeg P. Regional Induction of Adhesion Molecules and Chemokine Receptors Explains Disparate Homing of Human B Cells to Systemic and Mucosal Effector Sites: Dispersion From Tonsils. Blood (2005) 106(2):593–600. doi: 10.1182/blood-2004-12-4630
42. Thorburn AN, Hansbro PM. Harnessing Regulatory T Cells to Suppress Asthma: From Potential to Therapy. Am J Respir Cell Mol Biol (2010) 43(5):511–9. doi: 10.1165/rcmb.2009-0342TR
43. Jenabian MA, Ancuta P, Gilmore N, Routy JP. Regulatory T Cells in HIV Infection: Can Immunotherapy Regulate the Regulator? Clin Dev Immunol (2012) 2012:908314. doi: 10.1155/2012/908314
44. Cipolla EM, Alcorn JF. Repair of the Lung by Regulatory T Cells. Am J Respir Cell Mol Biol (2020) 63(4):405–7. doi: 10.1165/rcmb.2020-0260ED
45. Li Y, Kang G, Duan L, Lu W, Katze MG, Lewis MG, et al. SIV Infection of Lung Macrophages. PloS One (2015) 10(5):e0125500–e. doi: 10.1371/journal.pone.0125500
46. Barber SA, Gama L, Li M, Voelker T, Anderson JE, Zink MC, et al. Longitudinal Analysis of Simian Immunodeficiency Virus (SIV) Replication in the Lungs: Compartmentalized Regulation of SIV. J Infect Dis (2006) 194(7):931–8. doi: 10.1086/507429
47. Amedee AM, Phillips B, Jensen K, Robichaux S, Lacour N, Burke M, et al. Early Sites of Virus Replication After Oral SIV(mac251) Infection of Infant Macaques: Implications for Pathogenesis. AIDS Res Hum Retroviruses (2018) 34(3):286–99. doi: 10.1089/AID.2017.0169
48. Gartner S, Markovits P, Markovitz David M, Kaplan Mark H, Gallo Robert C, Popovic M. The Role of Mononuclear Phagocytes in HTLV-III/LAV Infection. Science (1986) 233(4760):215–9. doi: 10.1126/science.3014648
49. van't Wout AB, Ran LJ, Kuiken CL, Kootstra NA, Pals ST, Schuitemaker H. Analysis of the Temporal Relationship Between Human Immunodeficiency Virus Type 1 Quasispecies in Sequential Blood Samples and Various Organs Obtained at Autopsy. J Virol (1998) 72(1):488–96. doi: 10.1128/JVI.72.1.488-496.1998
50. Alimohammadi A, Coker R, Miller R, Mitchell D, Williamson J, Clarke J. Genotypic Variants of HIV-1 From Peripheral Blood and Lungs of AIDS Patients. AIDS (Lond Engl) (1997) 11(6):831–2.
51. Jeffrey AA, Israël-Biet D, Andrieu JM, Even P, Venet A. HIV Isolation From Pulmonary Cells Derived From Bronchoalveolar Lavage. Clin Exp Immunol (1991) 84(3):488–92.
52. Almodovar S. The Complexity of HIV Persistence and Pathogenesis in the Lung Under Antiretroviral Therapy: Challenges Beyond AIDS. Viral Immunol (2014) 27(5):186–99. doi: 10.1089/vim.2013.0130
53. Schiff AE, Linder AH, Luhembo SN, Banning S, Deymier MJ, Diefenbach TJ, et al. T Cell-Tropic HIV Efficiently Infects Alveolar Macrophages Through Contact With Infected CD4+ T Cells. Sci Rep (2021) 11(1):3890. doi: 10.1038/s41598-021-82066-x
54. Corleis B, Bucsan AN, Deruaz M, Vrbanac VD, Lisanti-Park AC, Gates SJ, et al. HIV-1 and SIV Infection Are Associated With Early Loss of Lung Interstitial CD4+ T Cells and Dissemination of Pulmonary Tuberculosis. Cell Rep (2019) 26(6):1409–18.e5. doi: 10.1016/j.celrep.2019.01.021
55. Groot F, van Capel TMM, Schuitemaker JHN, Berkhout B, de Jong EC. Differential Susceptibility of Naïve, Central Memory and Effector Memory T Cells to Dendritic Cell-Mediated HIV-1 Transmission. Retrovirology (2006) 3(1):52. doi: 10.1186/1742-4690-3-52
56. Dewhurst JA, Lea S, Hardaker E, Dungwa JV, Ravi AK, Singh D. Characterisation of Lung Macrophage Subpopulations in COPD Patients and Controls. Sci Rep (2017) 7(1):7143. doi: 10.1038/s41598-017-07101-2
57. Jambo KC, Banda DH, Kankwatira AM, Sukumar N, Allain TJ, Heyderman RS, et al. Small Alveolar Macrophages Are Infected Preferentially by HIV and Exhibit Impaired Phagocytic Function. Mucosal Immunol (2014) 7(5):1116–26. doi: 10.1038/mi.2013.127
58. Meziane O, Salahuddin S, Pham TNQ, Farnos O, Pagliuzza A, Olivenstein R, et al. HIV Infection and Persistence in Pulmonary Mucosal Double Negative T Cells In Vivo. J Virol (2020) 94(24). doi: 10.1128/jvi.01788-20
59. How Can You Tell If You Have HIV? : HIV.Gov Available at: https://www.hiv.gov/hiv-basics/overview/about-hiv-and-aids/symptoms-of-hiv.
60. Gasper-Smith N, Crossman DM, Whitesides JF, Mensali N, Ottinger JS, Plonk SG, et al. Induction of Plasma (TRAIL), TNFR-2, Fas Ligand, and Plasma Microparticles After Human Immunodeficiency Virus Type 1 (HIV-1) Transmission: Implications for HIV-1 Vaccine Design. J Virol (2008) 82(15):7700–10. doi: 10.1128/jvi.00605-08
61. Brune KA, Ferreira F, Mandke P, Chau E, Aggarwal NR, D'Alessio FR, et al. HIV Impairs Lung Epithelial Integrity and Enters the Epithelium to Promote Chronic Lung Inflammation. PloS One (2016) 11(3):e0149679–e. doi: 10.1371/journal.pone.0149679
62. Devadoss D, Singh SP, Acharya A, Do KC, Periyasamy P, Manevski M, et al. Lung Bronchial Epithelial Cells are HIV Targets for Proviral Genomic Integration. bioRxiv (2020), 2020.06.01.126821. doi: 10.1101/2020.06.01.126821
63. Heath L, Fox A, McClure J, Diem K, van 't Wout AB, Zhao H, et al. Evidence for Limited Genetic Compartmentalization of HIV-1 Between Lung and Blood. PloS One (2009) 4(9):e6949. doi: 10.1371/journal.pone.0006949
64. Nakata K, Weiden M, Harkin T, Ho D, Rom WN. Low Copy Number and Limited Variability of Proviral DNA in Alveolar Macrophages From HIV-1-Infected Patients: Evidence for Genetic Differences in HIV-1 Between Lung and Blood Macrophage Populations. Mol Med (1995) 1(7):744–57. doi: 10.1007/BF03401889
65. Cribbs SK, Crothers K, Morris A. Pathogenesis of HIV-Related Lung Disease: Immunity, Infection, and Inflammation. Physiol Rev (2019) 100(2):603–32. doi: 10.1152/physrev.00039.2018
66. Masur H, Michelis MA, Greene JB, Onorato I, Stouwe RA, Holzman RS, et al. An Outbreak of Community-Acquired Pneumocystis Carinii Pneumonia: Initial Manifestation of Cellular Immune Dysfunction. N Engl J Med (1981) 305(24):1431–8. doi: 10.1056/nejm198112103052402
67. Stansell JD. Pulmonary Fungal Infections in HIV-Infected Persons. Semin Respir Infect (1993) 8(2):116–23.
68. Hirschtick RE, Glassroth J, Jordan MC, Wilcosky TC, Wallace JM, Kvale PA, et al. Bacterial Pneumonia in Persons Infected With the Human Immunodeficiency Virus. Pulmonary Complications of HIV Infection Study Group. N Engl J Med (1995) 333(13):845–51. doi: 10.1056/nejm199509283331305
69. Johnson CC, Wilcosky T, Kvale P, Rosen M, Stansell J, Glassroth J, et al. Cancer Incidence Among an HIV-Infected Cohort. Pulmonary Complications of HIV Infection Study Group. Am J Epidemiol (1997) 146(6):470–5. doi: 10.1093/oxfordjournals.aje.a009300
70. Hou W, Fu J, Ge Y, Du J, Hua S. Incidence and Risk of Lung Cancer in HIV-Infected Patients. J Cancer Res Clin Oncol (2013) 139(11):1781–94. doi: 10.1007/s00432-013-1477-2
71. Neff CP, Chain JL, MaWhinney S, Martin AK, Linderman DJ, Flores SC, et al. Lymphocytic Alveolitis is Associated With the Accumulation of Functionally Impaired HIV-Specific T Cells in the Lung of Antiretroviral Therapy-Naive Subjects. Am J Respir Crit Care Med (2015) 191(4):464–73. doi: 10.1164/rccm.201408-1521OC
72. Twigg HL, Soliman DM, Day RB, Knox KS, Anderson RJ, Wilkes DS, et al. Lymphocytic Alveolitis, Bronchoalveolar Lavage Viral Load, and Outcome in Human Immunodeficiency Virus Infection. Am J Respir Crit Care Med (1999) 159(5 Pt 1):1439–44. doi: 10.1164/ajrccm.159.5.9808031
73. Mwale A, Hummel A, Mvaya L, Kamng'ona R, Chimbayo E, Phiri J, et al. B Cell, CD8 (+) T Cell and Gamma Delta T Cell Infiltration Alters Alveolar Immune Cell Homeostasis in HIV-Infected Malawian Adults. Wellcome Open Res (2017) 2:105. doi: 10.12688/wellcomeopenres.12869.3
74. Guillon J-M, Autran B, Denis M, Fouret P, Plata F, Mayaud CM, et al. Human Immunodeficiency Virus-Related Lymphocytic Alveolitis. Chest (1988) 94(6):1264–70. doi: 10.1378/chest.94.6.1264
75. Bunjun R, Soares AP, Thawer N, Müller TL, Kiravu A, Ginbot Z, et al. Dysregulation of the Immune Environment in the Airways During HIV Infection. Front Immunol (2021) 12:707355(2630). doi: 10.3389/fimmu.2021.707355
76. Porichis F, Kaufmann DE. Role of PD-1 in HIV Pathogenesis and as Target for Therapy. Curr HIV/AIDS Rep (2012) 9(1):81–90. doi: 10.1007/s11904-011-0106-4
77. Jambo KC, Tembo DL, Kamng’ona AW, Musicha P, Banda DH, et al. HIV-Associated Disruption of Lung Cytokine Networks Is Incompletely Restored in Asymptomatic HIV-Infected Malawian Adults on Antiretroviral Therapy. ERJ Open Res (2017) 3(4):00097–2017. doi: 10.1183/23120541.00097-2017
78. Culley Fiona J, Pennycook Alasdair MJ, Tregoning John S, Dodd Jonathan S, Walzl G, Wells Timothy N, et al. Role of CCL5 (RANTES) in Viral Lung Disease. J Virol (2006) 80(16):8151–7. doi: 10.1128/JVI.00496-06
79. Jassoy C, Harrer T, Rosenthal T, Navia BA, Worth J, Johnson RP, et al. Human Immunodeficiency Virus Type 1-Specific Cytotoxic T Lymphocytes Release Gamma Interferon, Tumor Necrosis Factor Alpha (TNF-Alpha), and TNF-Beta When They Encounter Their Target Antigens. J Virol (1993) 67(5):2844–52. doi: 10.1128/jvi.67.5.2844-2852.1993
80. Calmon-Hamaty F, Combe B, Hahne M, Morel J. Lymphotoxin α Revisited: General Features and Implications in Rheumatoid Arthritis. Arthritis Res Ther (2011) 13(4):232. doi: 10.1186/ar3376
81. Trentin L, Garbisa S, Zambello R, Agostini C, Caenazzo C, Di Francesco C, et al. Spontaneous Production of Interleukin-6 by Alveolar Macrophages From Human Immunodeficiency Virus Type 1-Infected Patients. J Infect Dis (1992) 166(4):731–7. doi: 10.1093/infdis/166.4.731
82. Borges ÁH, O'Connor JL, Phillips AN, Rönsholt FF, Pett S, Vjecha MJ, et al. Factors Associated With Plasma IL-6 Levels During HIV Infection. J Infect Dis (2015) 212(4):585–95. doi: 10.1093/infdis/jiv123
83. Cai Y, Sugimoto C, Liu DX, Midkiff CC, Alvarez X, Lackner AA, et al. Increased Monocyte Turnover Is Associated With Interstitial Macrophage Accumulation and Pulmonary Tissue Damage in SIV-Infected Rhesus Macaques. J Leukoc Biol (2015) 97(6):1147–53. doi: 10.1189/jlb.4A0914-441R
84. Greenlee KJ, Werb Z, Kheradmand F. Matrix Metalloproteinases in Lung: Multiple, Multifarious, and Multifaceted. Physiol Rev (2007) 87(1):69–98. doi: 10.1152/physrev.00022.2006
85. McGarry Houghton A. Matrix Metalloproteinases in Destructive Lung Disease. Matrix Biol (2015) 44-46:167–74. doi: 10.1016/j.matbio.2015.02.002
86. Chung NPY, Ou X, Khan KMF, Salit J, Kaner RJ, Crystal RG. HIV Reprograms Human Airway Basal Stem/Progenitor Cells to Acquire a Tissue-Destructive Phenotype. Cell Rep (2017) 19(6):1091–100. doi: 10.1016/j.celrep.2017.04.026
87. Sandler NG, Douek DC. Microbial Translocation in HIV Infection: Causes, Consequences and Treatment Opportunities. Nat Rev Microbiol (2012) 10(9):655–66. doi: 10.1038/nrmicro2848
88. Theron AJ, Anderson R, Rossouw TM, Steel HC. The Role of Transforming Growth Factor Beta-1 in the Progression of HIV/AIDS and Development of Non-AIDS-Defining Fibrotic Disorders. Front Immunol (2017) 8:1461. doi: 10.3389/fimmu.2017.01461
89. Maina EK, Abana CZ, Bukusi EA, Sedegah M, Lartey M, Ampofo WK. Plasma Concentrations of Transforming Growth Factor Beta 1 in Non-Progressive HIV-1 Infection Correlates With Markers of Disease Progression. Cytokine (2016) 81:109–16. doi: 10.1016/j.cyto.2016.02.009
90. Batlle E, Massagué J. Transforming Growth Factor-β Signaling in Immunity and Cancer. Immunity (2019) 50(4):924–40. doi: 10.1016/j.immuni.2019.03.024
91. Penn JW, Grobbelaar AO, Rolfe KJ. The Role of the TGF-β Family in Wound Healing, Burns and Scarring: A Review. Int J Burns Trauma (2012) 2(1):18–28.
92. Sanchez JL, Hunt PW, Reilly CS, Hatano H, Beilman GJ, Khoruts A, et al. Lymphoid Fibrosis Occurs in Long-Term Nonprogressors and Persists With Antiretroviral Therapy But May Be Reversible With Curative Interventions. J Infect Dis (2015) 211(7):1068–75. doi: 10.1093/infdis/jiu586
93. Zeng M, Smith AJ, Wietgrefe SW, Southern PJ, Schacker TW, Reilly CS, et al. Cumulative Mechanisms of Lymphoid Tissue Fibrosis and T Cell Depletion in HIV-1 and SIV Infections. J Clin Invest (2011) 121(3):998–1008. doi: 10.1172/jci45157
94. Asmuth DM, Pinchuk IV, Wu J, Vargas G, Chen X, Mann S, et al. Role of Intestinal Myofibroblasts in HIV-Associated Intestinal Collagen Deposition and Immune Reconstitution Following Combination Antiretroviral Therapy. AIDS (2015) 29(8):877–88. doi: 10.1097/qad.0000000000000636
95. Leader JK, Crothers K, Huang L, King MA, Morris A, Thompson BW, et al. Risk Factors Associated With Quantitative Evidence of Lung Emphysema and Fibrosis in an HIV-Infected Cohort. J Acquir Immune Defic Syndr (2016) 71(4):420–7. doi: 10.1097/QAI.0000000000000894
96. Twigg Iii HL, Spain BA, Soliman DM, Bowen LK, Heidler KM, Wilkes DS. Impaired IgG Production in the Lungs of HIV-Infected Individuals. Cell Immunol (1996) 170(1):127–33. doi: 10.1006/cimm.1996.0142
97. Porcheray F, Samah B, Léone C, Dereuddre-Bosquet N, Gras G. Macrophage Activation and Human Immunodeficiency Virus Infection: HIV Replication Directs Macrophages Towards a Pro-Inflammatory Phenotype While Previous Activation Modulates Macrophage Susceptibility to Infection and Viral Production. Virology (2006) 349(1):112–20. doi: 10.1016/j.virol.2006.02.031
98. Israël-Biet D, Cadranel J, Beldjord K, Andrieu JM, Jeffrey A, Even P. Tumor Necrosis Factor Production in HIV-Seropositive Subjects. Relationship With Lung Opportunistic Infections and HIV Expression in Alveolar Macrophages. J Immunol (1991) 147(2):490.
99. Koziel H, Eichbaum Q, Kruskal BA, Pinkston P, Rogers RA, Armstrong MY, et al. Reduced Binding and Phagocytosis of Pneumocystis Carinii by Alveolar Macrophages From Persons Infected With HIV-1 Correlates With Mannose Receptor Downregulation. J Clin Invest (1998) 102(7):1332–44. doi: 10.1172/JCI560
100. Neff CP, Atif SM, Logue EC, Siebert J, Görg C, Lavelle J, et al. HIV Infection Is Associated With Loss of Anti-Inflammatory Alveolar Macrophages. J Immunol (2020) 205(9):2447. doi: 10.4049/jimmunol.2000361
101. Hunegnaw R, Mushtaq Z, Enyindah-Asonye G, Hoang T, Robert-Guroff M. Alveolar Macrophage Dysfunction and Increased PD-1 Expression During Chronic SIV Infection of Rhesus Macaques. Front Immunol (2019) 10:1537. doi: 10.3389/fimmu.2019.01537
102. Chou S-HS, Prabhu SJ, Crothers K, Stern EJ, Godwin JD, Pipavath SN. Thoracic Diseases Associated With HIV Infection in the Era of Antiretroviral Therapy: Clinical and Imaging Findings. RadioGraphics (2014) 34(4):895–911. doi: 10.1148/rg.344130115
103. Twigg HL III, Weiden M, Valentine F, Bick CTS, Bassett R, Zheng L, et al. Effect of Highly Active Antiretroviral Therapy on Viral Burden in the Lungs of HIV-Infected Subjects. J Infect Dis (2008) 197(1):109–16. doi: 10.1086/523766
104. Knox KS, Vinton C, Hage CA, Kohli LM, Twigg HL 3rd, Klatt NR, et al. Reconstitution of CD4 T Cells in Bronchoalveolar Lavage Fluid After Initiation of Highly Active Antiretroviral Therapy. J Virol (2010) 84(18):9010–8. doi: 10.1128/jvi.01138-10
105. Twigg HL 3rd, Knox KS. Impact of Antiretroviral Therapy on Lung Immunology and Inflammation. Clinics Chest Med (2013) 34(2):155–64. doi: 10.1016/j.ccm.2013.01.004
106. Morris A, Crothers K, Beck JM, Huang L. American Thoracic Society Committee on HIVPD. An Official ATS Workshop Report: Emerging Issues and Current Controversies in HIV-Associated Pulmonary Diseases. Proc Am Thorac Soc (2011) 8(1):17–26. doi: 10.1513/pats.2009-047WS
107. Twigg HL 3rd, Knox KS. HIV-Related Lung Disorders. Drug Discovery Today Dis Mech (2007) 4(2):95–101. doi: 10.1016/j.ddmec.2007.11.001
108. Segal LN, Methé BA, Nolan A, Hoshino Y, Rom WN, Dawson R, et al. HIV-1 and Bacterial Pneumonia in the Era of Antiretroviral Therapy. Proc Am Thorac Soc (2011) 8(3):282–7. doi: 10.1513/pats.201006-044WR
109. López-Palomo C, Martín-Zamorano M, Benítez E, Fernández-Gutiérrez C, Guerrero F, Rodríguez-Iglesias M, et al. Pneumonia in HIV-Infected Patients in the HAART Era: Incidence, Risk, and Impact of the Pneumococcal Vaccination. J Med Virol (2004) 72(4):517–24. doi: 10.1002/jmv.20045
110. Ehrentraut H, Clambey ET, McNamee EN, Brodsky KS, Ehrentraut SF, Poth JM, et al. CD73+ Regulatory T Cells Contribute to Adenosine-Mediated Resolution of Acute Lung Injury. FASEB J (2013) 27(6):2207–19. doi: 10.1096/fj.12-225201
111. Allard B, Longhi MS, Robson SC, Stagg J. The Ectonucleotidases CD39 and CD73: Novel Checkpoint Inhibitor Targets. Immunol Rev (2017) 276(1):121–44. doi: 10.1111/imr.12528
112. Collini PJ, Bewley MA, Mohasin M, Marriott HM, Miller RF, Geretti A-M, et al. HIV Gp120 in the Lungs of Antiretroviral Therapy-Treated Individuals Impairs Alveolar Macrophage Responses to Pneumococci. Am J Respir Crit Care Med (2018) 197(12):1604–15. doi: 10.1164/rccm.201708-1755OC
113. Yeligar SM, Ward JM, Harris FL, Brown LAS, Guidot DM, Cribbs SK. Dysregulation of Alveolar Macrophage Pparγ, NADPH Oxidases, and Tgfβ(1) in Otherwise Healthy HIV-Infected Individuals. AIDS Res Hum Retroviruses (2017) 33(10):1018–26. doi: 10.1089/AID.2016.0030
114. Yeligar SM, Harris FL, Hart CM, Brown LA. Glutathione Attenuates Ethanol-Induced Alveolar Macrophage Oxidative Stress and Dysfunction by Downregulating NADPH Oxidases. Am J Physiol Lung Cell Mol Physiol (2014) 306(5):L429–41. doi: 10.1152/ajplung.00159.2013
115. Cho HY, Gladwell W, Wang X, Chorley B, Bell D, Reddy SP, et al. Nrf2-Regulated PPAR{gamma} Expression is Critical to Protection Against Acute Lung Injury in Mice. Am J Respir Crit Care Med (2010) 182(2):170–82. doi: 10.1164/rccm.200907-1047OC
116. Meng X-M, Nikolic-Paterson DJ, Lan HY. TGF-β: The Master Regulator of Fibrosis. Nat Rev Nephrol (2016) 12(6):325–38. doi: 10.1038/nrneph.2016.48
117. Akata K, Leung JM, Yamasaki K, Filho FSL, Yang J, Yang CX, et al. Altered Polarization and Impaired Phagocytic Activity of Lung Macrophages in People With HIV and COPD. J Infect Dis (2021). doi: 10.1093/infdis/jiab506
118. Azzam R, Lal L, Goh SL, Kedzierska K, Jaworowski A, Naim E, et al. Adverse Effects of Antiretroviral Drugs on HIV-1-Infected and -Uninfected Human Monocyte-Derived Macrophages. J Acquir Immune Defic Syndr (2006) 42(1):19–28. doi: 10.1097/01.qai.0000214809.83218.88
119. Korencak M, Byrne M, Richter E, Schultz BT, Juszczak P, Ake JA, et al. Effect of HIV Infection and Antiretroviral Therapy on Immune Cellular Functions. JCI Insight (2019) 4(12). doi: 10.1172/jci.insight.126675
120. Bowman ER, Cameron C, Richardson B, Kulkarni M, Gabriel J, Kettelhut A, et al. In Vitro Exposure of Leukocytes to HIV Preexposure Prophylaxis Decreases Mitochondrial Function and Alters Gene Expression Profiles. Antimicrob Agents Chemother (2020) 65(1):e01755–20. doi: 10.1128/AAC.01755-20
121. Correa-Macedo W, Fava VM, Orlova M, Cassart P, Olivenstein R, Sanz J, et al. Alveolar Macrophages From Persons Living With HIV Show Impaired Epigenetic Response to Mycobacterium Tuberculosis. J Clin Invest (2021). doi: 10.1172/jci148013
122. Ferrucci L, Fabbri E. Inflammageing: Chronic Inflammation in Ageing, Cardiovascular Disease, and Frailty. Nat Rev Cardiol (2018) 15(9):505–22. doi: 10.1038/s41569-018-0064-2
124. Koelman L, Pivovarova-Ramich O, Pfeiffer AFH, Grune T, Aleksandrova K. Cytokines for Evaluation of Chronic Inflammatory Status in Ageing Research: Reliability and Phenotypic Characterisation. Immun Ageing (2019) 16(1):11. doi: 10.1186/s12979-019-0151-1
125. Rodriguez IJ, Lalinde Ruiz N, Llano León M, Martínez Enríquez L, Montilla Velásquez M, Ortiz Aguirre JP, et al. Immunosenescence Study of T Cells: A Systematic Review. Front Immunol (2021) 11:604591(3460). doi: 10.3389/fimmu.2020.604591
126. Liu JC, Leung JM, Ngan DA, Nashta NF, Guillemi S, Harris M, et al. Absolute Leukocyte Telomere Length in HIV-Infected and Uninfected Individuals: Evidence of Accelerated Cell Senescence in HIV-Associated Chronic Obstructive Pulmonary Disease. PloS One (2015) 10(4):e0124426. doi: 10.1371/journal.pone.0124426
127. Xu S, Vucic EA, Shaipanich T, Lam S, Lam W, Montaner JS, et al. Decreased Telomere Length in the Small Airway Epithelium Suggests Accelerated Aging in the Lungs of Persons Living With Human Immunodeficiency Virus (HIV). Respir Res (2018) 19(1):117. doi: 10.1186/s12931-018-0821-0
128. Barnes PJ, Burney PGJ, Silverman EK, Celli BR, Vestbo J, Wedzicha JA, et al. Chronic Obstructive Pulmonary Disease. Nat Rev Dis Primers (2015) 1(1):15076. doi: 10.1038/nrdp.2015.76
129. Quaderi SA, Hurst JR. The Unmet Global Burden of COPD. Glob Health Epidemiol Genom (2018) 3:e4–e. doi: 10.1017/gheg.2018.1
130. Gershon A, Anita Kozyrskyj A, Bogdanovic B, Saint-Laurent D, Marciniuk D, Rasali D, et al. Report From The Canadian Chronic Disease Surveillance System: Asthma And Chronic Obstructive Pulmonary Disease (COPD) In Canada, 2018. Ottawa: Public Health Agency of Canada (2018).
131. Lalloo UG, Pillay S, Mngqibisa R, Abdool-Gaffar S, Ambaram A. HIV and COPD: A Conspiracy of Risk Factors. Respirology (2016) 21(7):1166–72. doi: 10.1111/resp.12806
132. Bigna JJ, Kenne AM, Asangbeh SL, Sibetcheu AT. Prevalence of Chronic Obstructive Pulmonary Disease in the Global Population With HIV: A Systematic Review and Meta-Analysis. Lancet Global Health (2018) 6(2):e193–202. doi: 10.1016/S2214-109X(17)30451-5
133. Ito K, Barnes PJ. COPD as a Disease of Accelerated Lung Aging. Chest (2009) 135(1):173–80. doi: 10.1378/chest.08-1419
134. Lee J, Sandford A, Man P, Sin DD. Is the Aging Process Accelerated in Chronic Obstructive Pulmonary Disease? Curr Opin Pulm Med (2011) 17(2):90–7. doi: 10.1097/mcp.0b013e328341cead
135. Córdoba-Lanús E, Cazorla-Rivero S, García-Bello MA, Mayato D, Gonzalvo F, Ayra-Plasencia J, et al. Telomere Length Dynamics Over 10-Years and Related Outcomes in Patients With COPD. Respir Res (2021) 22(1):56. doi: 10.1186/s12931-021-01616-z
136. Popescu I, Drummond MB, Gama L, Coon T, Merlo CA, Wise RA, et al. Activation-Induced Cell Death Drives Profound Lung CD4(+) T-Cell Depletion in HIV-Associated Chronic Obstructive Pulmonary Disease. Am J Respir Crit Care Med (2014) 190(7):744–55. doi: 10.1164/rccm.201407-1226OC
137. Freeman CM, Han MK, Martinez FJ, Murray S, Liu LX, Chensue SW, et al. Cytotoxic Potential of Lung CD8(+) T Cells Increases With Chronic Obstructive Pulmonary Disease Severity and With In Vitro Stimulation by IL-18 or IL-15. J Immunol (2010) 184(11):6504–13. doi: 10.4049/jimmunol.1000006
138. Winstone TA, Man SFP, Hull M, Montaner JS, Sin DD. Epidemic of Lung Cancer in Patients With HIV Infection. Chest (2013) 143(2):305–14. doi: 10.1378/chest.12-1699
139. Sigel K, Wisnivesky J, Gordon K, Dubrow R, Justice A, Brown ST, et al. HIV as an Independent Risk Factor for Incident Lung Cancer. AIDS (2012) 26(8):1017–25. doi: 10.1097/QAD.0b013e328352d1ad
140. Koshiol J, Rotunno M, Consonni D, Pesatori AC, De Matteis S, Goldstein AM, et al. Chronic Obstructive Pulmonary Disease and Altered Risk of Lung Cancer in a Population-Based Case-Control Study. PloS One (2009) 4(10):e7380. doi: 10.1371/journal.pone.0007380
141. Agustí A, Edwards LD, Rennard SI, MacNee W, Tal-Singer R, Miller BE, et al. Persistent Systemic Inflammation Is Associated With Poor Clinical Outcomes in COPD: A Novel Phenotype. PloS One (2012) 7(5):e37483. doi: 10.1371/journal.pone.0037483
142. Sigel K, Makinson A, Thaler J. Lung Cancer in Persons With HIV. Curr Opin HIV AIDS (2017) 12(1):31–8. doi: 10.1097/COH.0000000000000326
143. Sigel K, Wisnivesky J, Crothers K, Gordon K, Brown ST, Rimland D, et al. Immunological and Infectious Risk Factors for Lung Cancer in US Veterans With HIV: A Longitudinal Cohort Study. Lancet HIV (2017) 4(2):e67–73. doi: 10.1016/S2352-3018(16)30215-6
144. Twigg HL 3rd, Knox KS, Zhou J, Crothers KA, Nelson DE, Toh E, et al. Effect of Advanced HIV Infection on the Respiratory Microbiome. Am J Respir Crit Care Med (2016) 194(2):226–35. doi: 10.1164/rccm.201509-1875OC
145. Twigg HL 3rd, Weinstock GM, Knox KS. Lung Microbiome in Human Immunodeficiency Virus Infection. Transl Res (2017) 179:97–107. doi: 10.1016/j.trsl.2016.07.008
146. Klugman M, Fazzari M, Xue X, Ginsberg M, Rohan TE, Halmos B, et al. The Associations of CD4 Count, CD4/CD8 Ratio, and HIV Viral Load With Survival From Non-Small Cell Lung Cancer in Persons Living With HIV. AIDS Care (2021), 1–8. doi: 10.1080/09540121.2021.1934380
147. Feuth T, Arends JE, Fransen JH, Nanlohy NM, van Erpecum KJ, Siersema PD, et al. Complementary Role of HCV and HIV in T-Cell Activation and Exhaustion in HIV/HCV Coinfection. PloS One (2013) 8(3):e59302. doi: 10.1371/journal.pone.0059302
148. Kared H, Saeed S, Klein MB, Shoukry NH. CD127 Expression, Exhaustion Status and Antigen Specific Proliferation Predict Sustained Virologic Response to IFN in HCV/HIV Co-Infected Individuals. PloS One (2014) 9(7):e101441. doi: 10.1371/journal.pone.0101441
149. Okoye IS, Houghton M, Tyrrell L, Barakat K, Elahi S. Coinhibitory Receptor Expression and Immune Checkpoint Blockade: Maintaining a Balance in CD8(+) T Cell Responses to Chronic Viral Infections and Cancer. Front Immunol (2017) 8:1215. doi: 10.3389/fimmu.2017.01215
150. Al-Shibli KI, Donnem T, Al-Saad S, Persson M, Bremnes RM, Busund LT. Prognostic Effect of Epithelial and Stromal Lymphocyte Infiltration in Non-Small Cell Lung Cancer. Clin Cancer Res (2008) 14(16):5220–7. doi: 10.1158/1078-0432.Ccr-08-0133
151. Prado-Garcia H, Romero-Garcia S, Aguilar-Cazares D, Meneses-Flores M, Lopez-Gonzalez JS. Tumor-Induced CD8+ T-Cell Dysfunction in Lung Cancer Patients. Clin Dev Immunol (2012) 2012:741741. doi: 10.1155/2012/741741
152. Crothers K, Huang L, Goulet JL, Goetz MB, Brown ST, Rodriguez-Barradas MC, et al. HIV Infection and Risk for Incident Pulmonary Diseases in the Combination Antiretroviral Therapy Era. Am J Respir Crit Care Med (2011) 183(3):388–95. doi: 10.1164/rccm.201006-0836OC
153. Osuji FN, Onyenekwe CC, Ahaneku JE, Ukibe NR. The Effects of Highly Active Antiretroviral Therapy on the Serum Levels of Pro-Inflammatory and Anti-Inflammatory Cytokines in HIV Infected Subjects. J BioMed Sci (2018) 25(1):88–. doi: 10.1186/s12929-018-0490-9
154. Saito A, Horie M, Nagase T. TGF-β Signaling in Lung Health and Disease. Int J Mol Sci (2018) 19(8):2460. doi: 10.3390/ijms19082460
155. Hasegawa Y, Takanashi S, Kanehira Y, Tsushima T, Imai T, Okumura K. Transforming Growth Factor-Beta1 Level Correlates With Angiogenesis, Tumor Progression, and Prognosis in Patients With Nonsmall Cell Lung Carcinoma. Cancer (2001) 91(5):964–71. doi: 10.1002/1097-0142(20010301)91:5<964::AID-CNCR1086>3.0.CO;2-O
156. Jankowich MD, Rounds SIS. Combined Pulmonary Fibrosis and Emphysema Syndrome: A Review. Chest (2012) 141(1):222–31. doi: 10.1378/chest.11-1062
157. Kuhlman JE, Knowles MC, Fishman EK, Siegelman SS. Premature Bullous Pulmonary Damage in AIDS: CT Diagnosis. Radiology (1989) 173(1):23–6. doi: 10.1148/radiology.173.1.2781013
158. Attia EF, Akgün KM, Wongtrakool C, Goetz MB, Rodriguez-Barradas MC, Rimland D, et al. Increased Risk of Radiographic Emphysema in HIV Is Associated With Elevated Soluble CD14 and Nadir CD4. Chest (2014) 146(6):1543–53. doi: 10.1378/chest.14-0543
159. Petrache I, Diab K, Knox KS, Twigg HL 3rd, Stephens RS, Flores S, et al. HIV Associated Pulmonary Emphysema: A Review of the Literature and Inquiry Into its Mechanism. Thorax (2008) 63(5):463–9. doi: 10.1136/thx.2007.079111
160. Diaz PT, King MA, Pacht ER, Wewers MD, Gadek JE, Nagaraja HN, et al. Increased Susceptibility to Pulmonary Emphysema Among HIV-Seropositive Smokers. Ann Intern Med (2000) 132(5):369–72. doi: 10.7326/0003-4819-132-5-200003070-00006
161. Guaraldi G, Besutti G, Scaglioni R, Santoro A, Zona S, Guido L, et al. The Burden of Image Based Emphysema and Bronchiolitis in HIV-Infected Individuals on Antiretroviral Therapy. PloS One (2014) 9(10):e109027. doi: 10.1371/journal.pone.0109027
162. Leung JM, Malagoli A, Santoro A, Besutti G, Ligabue G, Scaglioni R, et al. Emphysema Distribution and Diffusion Capacity Predict Emphysema Progression in Human Immunodeficiency Virus Infection. PloS One (2016) 11(11):e0167247. doi: 10.1371/journal.pone.0167247
163. Singhvi D, Bon J, Morris A. Obstructive Lung Disease in HIV—Phenotypes and Pathogenesis. Curr HIV/AIDS Rep (2019) 16(4):359–69. doi: 10.1007/s11904-019-00456-3
164. Rodriguez-Penney AT, Iudicello JE, Riggs PK, Doyle K, Ellis RJ, Letendre SL, et al. Co-Morbidities in Persons Infected With HIV: Increased Burden With Older Age and Negative Effects on Health-Related Quality of Life. AIDS Patient Care STDS (2013) 27(1):5–16. doi: 10.1089/apc.2012.0329
165. Helleberg M, Afzal S, Kronborg G, Larsen CS, Pedersen G, Pedersen C, et al. Mortality Attributable to Smoking Among HIV-1–Infected Individuals: A Nationwide, Population-Based Cohort Study. Clin Infect Dis (2013) 56(5):727–34. doi: 10.1093/cid/cis933
166. Giles ML, Gartner C, Boyd MA. Smoking and HIV: What are the Risks and What Harm Reduction Strategies do We Have at Our Disposal? AIDS Res Ther (2018) 15(1):26–. doi: 10.1186/s12981-018-0213-z
167. MacDonald DM, Melzer AC, Collins G, Avihingsanon A, Crothers K, Ingraham NE, et al. Smoking and Accelerated Lung Function Decline in HIV-Positive Individuals: A Secondary Analysis of the START Pulmonary Substudy. J Acquir Immune Defic Syndr (2018) 79(3):e85–92. doi: 10.1097/QAI.0000000000001797
168. Kaner RJ, Santiago F, Crystal RG. Up-Regulation of Alveolar Macrophage Matrix Metalloproteinases in HIV1(+) Smokers With Early Emphysema. J Leukoc Biol (2009) 86(4):913–22. doi: 10.1189/jlb.0408240
169. Nadigel J, Préfontaine D, Baglole CJ, Maltais F, Bourbeau J, Eidelman DH, et al. Cigarette Smoke Increases TLR4 and TLR9 Expression and Induces Cytokine Production From CD8+T Cells in Chronic Obstructive Pulmonary Disease. Respir Res (2011) 12(1):149. doi: 10.1186/1465-9921-12-149
170. Maeno T, Houghton AM, Quintero PA, Grumelli S, Owen CA, Shapiro SD. CD8+ T Cells Are Required for Inflammation and Destruction in Cigarette Smoke-Induced Emphysema in Mice. J Immunol (2007) 178(12):8090. doi: 10.4049/jimmunol.178.12.8090
171. Duan M-C, Huang Y, Zhong X-N, Tang H-J. Th17 Cell Enhances CD8 T-Cell Cytotoxicity via IL-21 Production in Emphysema Mice. Mediators Inflamm (2012) 2012:898053. doi: 10.1155/2012/898053
172. Dandekar S. Pathogenesis of HIV in the Gastrointestinal Tract. Curr HIV/AIDS Rep (2007) 4(1):10–5. doi: 10.1007/s11904-007-0002-0
173. Hatano H. Immune Activation and HIV Persistence: Considerations for Novel Therapeutic Interventions. Curr Opin HIV AIDS (2013) 8(3):211–6. doi: 10.1097/COH.0b013e32835f9788
174. Eguíluz-Gracia I, Schultz HH, Sikkeland LI, Danilova E, Holm AM, Pronk CJ, et al. Long-Term Persistence of Human Donor Alveolar Macrophages in Lung Transplant Recipients. Thorax (2016) 71(11):1006–11. doi: 10.1136/thoraxjnl-2016-208292
175. Clayton KL, Collins DR, Lengieza J, Ghebremichael M, Dotiwala F, Lieberman J, et al. Resistance of HIV-Infected Macrophages to CD8+ T Lymphocyte–Mediated Killing Drives Activation of the Immune System. Nat Immunol (2018) 19(5):475–86. doi: 10.1038/s41590-018-0085-3
176. Horiike M, Iwami S, Kodama M, Sato A, Watanabe Y, Yasui M, et al. Lymph Nodes Harbor Viral Reservoirs That Cause Rebound of Plasma Viremia in SIV-Infected Macaques Upon Cessation of Combined Antiretroviral Therapy. Virology (2012) 423(2):107–18. doi: 10.1016/j.virol.2011.11.024
177. Santangelo PJ, Rogers KA, Zurla C, Blanchard EL, Gumber S, Strait K, et al. Whole-Body immunoPET Reveals Active SIV Dynamics in Viremic and Antiretroviral Therapy–Treated Macaques. Nat Methods (2015) 12(5):427–32. doi: 10.1038/nmeth.3320
178. Barry M, Bleackley RC. Cytotoxic T Lymphocytes: All Roads Lead to Death. Nat Rev Immunol (2002) 2(6):401–9. doi: 10.1038/nri819
179. Gonzalez SM, Taborda NA, Rugeles MT. Role of Different Subpopulations of CD8(+) T Cells During HIV Exposure and Infection. Front Immunol (2017) 8:936. doi: 10.3389/fimmu.2017.00936
180. Lauvau G, Goriely S. Memory CD8+ T Cells: Orchestrators and Key Players of Innate Immunity? PloS Pathog (2016) 12(9):e1005722. doi: 10.1371/journal.ppat.1005722
181. Warren JA, Clutton G, Goonetilleke N. Harnessing CD8(+) T Cells Under HIV Antiretroviral Therapy. Front Immunol (2019) 10:291. doi: 10.3389/fimmu.2019.00291
182. Brenchley JM, Knox KS, Asher AI, Price DA, Kohli LM, Gostick E, et al. High Frequencies of Polyfunctional HIV-Specific T Cells Are Associated With Preservation of Mucosal CD4 T Cells in Bronchoalveolar Lavage. Mucosal Immunol (2008) 1(1):49–58. doi: 10.1038/mi.2007.5
183. Serrano-Villar S, Sainz T, Lee SA, Hunt PW, Sinclair E, Shacklett BL, et al. HIV-Infected Individuals With Low CD4/CD8 Ratio Despite Effective Antiretroviral Therapy Exhibit Altered T Cell Subsets, Heightened CD8+ T Cell Activation, and Increased Risk of Non-AIDS Morbidity and Mortality. PloS Pathog (2014) 10(5):e1004078. doi: 10.1371/journal.ppat.1004078
184. Cao W, Mehraj V, Kaufmann DE, Li T, Routy J-P. Elevation and Persistence of CD8 T-Cells in HIV Infection: The Achilles Heel in the ART Era. J Int AIDS Soc (2016) 19(1):20697–. doi: 10.7448/IAS.19.1.20697
185. Doisne JM, Urrutia A, Lacabaratz-Porret C, Goujard C, Meyer L, Chaix ML, et al. CD8+ T Cells Specific for EBV, Cytomegalovirus, and Influenza Virus are Activated During Primary HIV Infection. J Immunol (2004) 173(4):2410–8. doi: 10.4049/jimmunol.173.4.2410
186. Seddiki N, Kaufmann DE. Editorial Overview: Cell Dysfunction and Exhaustion in HIV Infection. Curr Opin HIV AIDS (2014) 9(5):437–8. doi: 10.1097/coh.0000000000000098
187. Fenwick C, Joo V, Jacquier P, Noto A, Banga R, Perreau M, et al. T-Cell Exhaustion in HIV Infection. Immunol Rev (2019) 292(1):149–63. doi: 10.1111/imr.12823
188. Borrow P, Lewicki H, Hahn BH, Shaw GM, Oldstone MB. Virus-Specific CD8+ Cytotoxic T-Lymphocyte Activity Associated With Control of Viremia in Primary Human Immunodeficiency Virus Type 1 Infection. J Virol (1994) 68(9):6103–10. doi: 10.1128/jvi.68.9.6103-6110.1994
189. Koup RA, Safrit JT, Cao Y, Andrews CA, McLeod G, Borkowsky W, et al. Temporal Association of Cellular Immune Responses With the Initial Control of Viremia in Primary Human Immunodeficiency Virus Type 1 Syndrome. J Virol (1994) 68(7):4650–5. doi: 10.1128/jvi.68.7.4650-4655.1994
190. Cartwright EK, Spicer L, Smith SA, Lee D, Fast R, Paganini S, et al. CD8(+) Lymphocytes Are Required for Maintaining Viral Suppression in SIV-Infected Macaques Treated With Short-Term Antiretroviral Therapy. Immunity (2016) 45(3):656–68. doi: 10.1016/j.immuni.2016.08.018
191. Borrow P, Lewicki H, Wei X, Horwitz MS, Peffer N, Meyers H, et al. Antiviral Pressure Exerted by HIV-1-Specific Cytotoxic T Lymphocytes (CTLs) During Primary Infection Demonstrated by Rapid Selection of CTL Escape Virus. Nat Med (1997) 3(2):205–11. doi: 10.1038/nm0297-205
192. Vanderford TH, Bleckwehl C, Engram JC, Dunham RM, Klatt NR, Feinberg MB, et al. Viral CTL Escape Mutants are Generated in Lymph Nodes and Subsequently Become Fixed in Plasma and Rectal Mucosa During Acute SIV Infection of Macaques. PloS Pathog (2011) 7(5):e1002048–e. doi: 10.1371/journal.ppat.1002048
193. Meziane O, Alexandrova Y, Olivenstein R, Dupuy FP, Salahuddin S, Thomson E, et al. Peculiar Phenotypic and Cytotoxic Features of Pulmonary Mucosal CD8 T Cells in People Living With HIV Receiving Long-Term Antiretroviral Therapy. J Immunol (2021) 206(3):641–51. doi: 10.4049/jimmunol.2000916
194. Sung JM, Margolis DM. HIV Persistence on Antiretroviral Therapy and Barriers to a Cure. In: Zhang L, Lewin SR, editors. HIV Vaccines and Cure: The Path Towards Finding an Effective Cure and Vaccine. Singapore: Springer Singapore (2018). p. 165–85.
195. Velu V, Shetty RD, Larsson M, Shankar EM. Role of PD-1 Co-Inhibitory Pathway in HIV Infection and Potential Therapeutic Options. Retrovirology (2015) 12:14. doi: 10.1186/s12977-015-0144-x
196. Twigg HL, Schnizlein-Bick CT, Weiden M, Valentine F, Wheat J, Day RB, et al. Measurement of Antiretroviral Drugs in the Lungs of HIV-Infected Patients. HIV Ther (2010) 4(2):247–51. doi: 10.2217/hiv.10.5
197. Martinez-Picado J, Deeks SG. Persistent HIV-1 Replication During Antiretroviral Therapy. Curr Opin HIV AIDS (2016) 11(4):417–23. doi: 10.1097/COH.0000000000000287
198. Lorenzo-Redondo R, Fryer HR, Bedford T, Kim E-Y, Archer J, Pond SLK, et al. Persistent HIV-1 Replication Maintains the Tissue Reservoir During Therapy. Nature (2016) 530(7588):51–6. doi: 10.1038/nature16933
199. Hatano H, Jain V, Hunt PW, Lee TH, Sinclair E, Do TD, et al. Cell-Based Measures of Viral Persistence Are Associated With Immune Activation and Programmed Cell Death Protein 1 (PD-1)-Expressing CD4+ T Cells. J Infect Dis (2013) 208(1):50–6. doi: 10.1093/infdis/jis630
200. Cockerham LR, Siliciano JD, Sinclair E, O'Doherty U, Palmer S, Yukl SA, et al. CD4+ and CD8+ T Cell Activation Are Associated With HIV DNA in Resting CD4+ T Cells. PloS One (2014) 9(10):e110731. doi: 10.1371/journal.pone.0110731
201. Akiyama H, Miller CM, Ettinger CR, Belkina AC, Snyder-Cappione JE, Gummuluru S. HIV-1 Intron-Containing RNA Expression Induces Innate Immune Activation and T Cell Dysfunction. Nat Commun (2018) 9(1):3450. doi: 10.1038/s41467-018-05899-7
202. Chomont N, El-Far M, Ancuta P, Trautmann L, Procopio FA, Yassine-Diab B, et al. HIV Reservoir Size and Persistence are Driven by T Cell Survival and Homeostatic Proliferation. Nat Med (2009) 15(8):893–900. doi: 10.1038/nm.1972
203. Massanella M, Fromentin R, Chomont N. Residual Inflammation and Viral Reservoirs: Alliance Against an HIV Cure. Curr Opin HIV AIDS (2016) 11(2):234–41. doi: 10.1097/COH.0000000000000230
204. Reeves DB, Duke ER, Wagner TA, Palmer SE, Spivak AM. Schiffer JT. A Majority of HIV Persistence During Antiretroviral Therapy is Due to Infected Cell Proliferation. Nat Commun (2018) 9(1):4811. doi: 10.1038/s41467-018-06843-5
205. Vesterbacka J, Rivera J, Noyan K, Parera M, Neogi U, Calle M, et al. Richer Gut Microbiota With Distinct Metabolic Profile in HIV Infected Elite Controllers. Sci Rep (2017) 7(1):6269. doi: 10.1038/s41598-017-06675-1
206. Nowak P, Troseid M, Avershina E, Barqasho B, Neogi U, Holm K, et al. Gut Microbiota Diversity Predicts Immune Status in HIV-1 Infection. AIDS (2015) 29(18):2409–18. doi: 10.1097/qad.0000000000000869
207. Rofael SAD, Brown J, Pickett E, Johnson M, Hurst JR, Spratt D, et al. Enrichment of the Airway Microbiome in People Living With HIV With Potential Pathogenic Bacteria Despite Antiretroviral Therapy. EClinicalMedicine (2020) 24. doi: 10.1016/j.eclinm.2020.100427
208. Koay WLA, Siems LV, Persaud D. The Microbiome and HIV Persistence: Implications for Viral Remission and Cure. Curr Opin HIV AIDS (2018) 13(1):61–8. doi: 10.1097/COH.0000000000000434
209. Budden KF, Gellatly SL, Wood DLA, Cooper MA, Morrison M, Hugenholtz P, et al. Emerging Pathogenic Links Between Microbiota and the Gut–Lung Axis. Nat Rev Microbiol (2017) 15(1):55–63. doi: 10.1038/nrmicro.2016.142
210. Bowerman KL, Rehman SF, Vaughan A, Lachner N, Budden KF, Kim RY, et al. Disease-Associated Gut Microbiome and Metabolome Changes in Patients With Chronic Obstructive Pulmonary Disease. Nat Commun (2020) 11(1):5886. doi: 10.1038/s41467-020-19701-0
211. Frati F, Salvatori C, Incorvaia C, Bellucci A, Di Cara G, Marcucci F, et al. The Role of the Microbiome in Asthma: The Gut⁻Lung Axis. Int J Mol Sci (2018) 20(1):123. doi: 10.3390/ijms20010123
212. Sencio V, Machado MG, Trottein F. The Lung–Gut Axis During Viral Respiratory Infections: The Impact of Gut Dysbiosis on Secondary Disease Outcomes. Mucosal Immunol (2021) 14(2):296–304. doi: 10.1038/s41385-020-00361-8
213. Thompson CG, Gay CL, Kashuba ADM. HIV Persistence in Gut-Associated Lymphoid Tissues: Pharmacological Challenges and Opportunities. AIDS Res Hum Retroviruses (2017) 33(6):513–23. doi: 10.1089/AID.2016.0253
214. Guadalupe M, Reay E, Sankaran S, Prindiville T, Flamm J, McNeil A, et al. Severe CD4+ T-Cell Depletion in Gut Lymphoid Tissue During Primary Human Immunodeficiency Virus Type 1 Infection and Substantial Delay in Restoration Following Highly Active Antiretroviral Therapy. J Virol (2003) 77(21):11708–17. doi: 10.1128/jvi.77.21.11708-11717.2003
215. Nwosu FC, Avershina E, Wilson R, Rudi K. Gut Microbiota in HIV Infection: Implication for Disease Progression and Management. Gastroenterol Res Pract (2014) 2014:803185. doi: 10.1155/2014/803185
216. Zicari S, Sessa L, Cotugno N, Ruggiero A, Morrocchi E, Concato C, et al. Immune Activation, Inflammation, and Non-AIDS Co-Morbidities in HIV-Infected Patients Under Long-Term ART. Viruses (2019) 11(3):200. doi: 10.3390/v11030200
217. Nazli A, Chan O, Dobson-Belaire WN, Ouellet M, Tremblay MJ, Gray-Owen SD, et al. Exposure to HIV-1 Directly Impairs Mucosal Epithelial Barrier Integrity Allowing Microbial Translocation. PloS Pathog (2010) 6(4):e1000852. doi: 10.1371/journal.ppat.1000852
218. Gootenberg DB, Paer JM, Luevano JM, Kwon DS. HIV-Associated Changes in the Enteric Microbial Community: Potential Role in Loss of Homeostasis and Development of Systemic Inflammation. Curr Opin Infect Dis (2017) 30(1):31–43. doi: 10.1097/qco.0000000000000341
219. Vázquez-Castellanos JF, Serrano-Villar S, Latorre A, Artacho A, Ferrús ML, Madrid N, et al. Altered Metabolism of Gut Microbiota Contributes to Chronic Immune Activation in HIV-Infected Individuals. Mucosal Immunol (2015) 8(4):760–72. doi: 10.1038/mi.2014.107
220. Abad-Fernández M, Vallejo A, Hernández-Novoa B, Díaz L, Gutiérrez C, Madrid N, et al. Correlation Between Different Methods to Measure Microbial Translocation and its Association With Immune Activation in Long-Term Suppressed HIV-1-Infected Individuals. J Acquir Immune Defic Syndr (2013) 64(2):149–53. doi: 10.1097/QAI.0b013e31829a2f12
221. Head BM, Mao R, Keynan Y, Rueda ZV. Inflammatory Mediators and Lung Abnormalities in HIV: A Systematic Review. PloS One (2019) 14(12):e0226347. doi: 10.1371/journal.pone.0226347
222. Lozupone C, Cota-Gomez A, Palmer BE, Linderman DJ, Charlson ES, Sodergren E, et al. Widespread Colonization of the Lung by Tropheryma Whipplei in HIV Infection. Am J Respir Crit Care Med (2013) 187(10):1110–7. doi: 10.1164/rccm.201211-2145OC
223. Segal LN, Alekseyenko AV, Clemente JC, Kulkarni R, Wu B, Gao Z, et al. Enrichment of Lung Microbiome With Supraglottic Taxa is Associated With Increased Pulmonary Inflammation. Microbiome (2013) 1(1):19. doi: 10.1186/2049-2618-1-19
224. Crane M, Avihingsanon A, Rajasuriar R, Velayudham P, Iser D, Solomon A, et al. Lipopolysaccharide, Immune Activation, and Liver Abnormalities in HIV/hepatitis B Virus (HBV)-Coinfected Individuals Receiving HBV-Active Combination Antiretroviral Therapy. J Infect Dis (2014) 210(5):745–51. doi: 10.1093/infdis/jiu119
225. Naeger DM, Martin JN, Sinclair E, Hunt PW, Bangsberg DR, Hecht F, et al. Cytomegalovirus-Specific T Cells Persist at Very High Levels During Long-Term Antiretroviral Treatment of HIV Disease. PloS One (2010) 5(1):e8886. doi: 10.1371/journal.pone.0008886
226. Hileman CO, Funderburg NT. Inflammation, Immune Activation, and Antiretroviral Therapy in HIV. Curr HIV/AIDS Rep (2017) 14(3):93–100. doi: 10.1007/s11904-017-0356-x
227. Tuberculosis and HIV. Geneva: UNAIDS (Joint United Nations Programme on HIV/AIDS) (2020). Available at: https://www.unaids.org/sites/default/files/media_asset/tuberculosis-and-hiv-progress-towards-the-2020-target_en.pdf
228. Tuberculosis and HIV — Progress Towards the 2020 Target (2019). Geneva: UNAIDS (Joint United Nations Programme on HIV/AIDS). Available at: https://www.unaids.org/sites/default/files/media_asset/tuberculosis-and-hiv-progress-towards-the-2020-target_en.pdf.
229. Bell LCK, Noursadeghi M. Pathogenesis of HIV-1 and Mycobacterium Tuberculosis Co-Infection. Nat Rev Microbiol (2018) 16(2):80–90. doi: 10.1038/nrmicro.2017.128.
230. Hoshino Y, Nakata K, Hoshino S, Honda Y, Tse DB, Shioda T, et al. Maximal HIV-1 Replication in Alveolar Macrophages During Tuberculosis Requires Both Lymphocyte Contact and Cytokines. J Exp Med (2002) 195(4):495–505. doi: 10.1084/jem.20011614
231. Toossi Z, Johnson JL, Kanost RA, Wu M, Luzze H, Peters P, et al. Increased Replication of HIV-1 at Sites of Mycobacterium Tuberculosis Infection: Potential Mechanisms of Viral Activation. JAIDS-HAGERSTOWN MD- (2001) 28(1):1–8. doi: 10.1097/00042560-200109010-00001
232. Lawn SD, Pisell TL, Hirsch CS, Wu M, Butera ST, Toossi Z. Anatomically Compartmentalized Human Immunodeficiency Virus Replication in HLA-DR+ Cells and CD14+ Macrophages at the Site of Pleural Tuberculosis Coinfection. J Infect Dis (2001) 184(9):1127–33. doi: 10.1086/323649
233. Collins Kalonji R, Quiñones-Mateu Miguel E, Wu M, Luzze H, Johnson John L, Hirsch C, et al. Human Immunodeficiency Virus Type 1 (HIV-1)Quasispecies at the Sites of Mycobacterium Tuberculosis InfectionContribute to Systemic HIV-1 Heterogeneity. J Virol (2002) 76(4):1697–706. doi: 10.1128/JVI.76.4.1697-1706.2002
234. Mdodo R, Frazier EL, Dube SR, Mattson CL, Sutton MY, Brooks JT, et al. Cigarette Smoking Prevalence Among Adults With HIV Compared With the General Adult Population in the United States: Cross-Sectional Surveys. Ann Intern Med (2015) 162(5):335–44. doi: 10.7326/m14-0954
235. Hoshino Y, Tse DB, Rochford G, Prabhakar S, Hoshino S, Chitkara N, et al. ≪Em<Mycobacterium Tuberculosis≪/Em<-Induced CXCR4 and Chemokine Expression Leads to Preferential X4 HIV-1 Replication in Human Macrophages. J Immunol (2004) 172(10):6251. doi: 10.4049/jimmunol.172.10.6251
236. Tesoriero JM, Swain C-AE, Pierce JL, Zamboni L, Wu M, Holtgrave DR, et al. COVID-19 Outcomes Among Persons Living With or Without Diagnosed HIV Infection in New York State. JAMA Netw Open (2021) 4(2):e2037069–e. doi: 10.1001/jamanetworkopen.2020.37069
237. Guo WMF, Dong Y, Zhang Q, Zhang X, Mo P, Feng Y, et al. A Survey for COVID-19 Among HIV/AIDS Patients in Two Districts of Wuhan, China. Lancet (2020). doi: 10.2139/ssrn.3550029
238. Geretti AM, Stockdale AJ, Kelly SH, Cevik M, Collins S, Waters L, et al. Outcomes of Coronavirus Disease 2019 (COVID-19) Related Hospitalization Among People With Human Immunodeficiency Virus (HIV) in the ISARIC World Health Organization (WHO) Clinical Characterization Protocol (UK): A Prospective Observational Study. Clin Infect Dis (2021) 73(7):e2095–e106. doi: 10.1093/cid/ciaa1605
239. Williamson EJ, Walker AJ, Bhaskaran K, Bacon S, Bates C, Morton CE, et al. Factors Associated With COVID-19-Related Death Using OpenSAFELY. Nature (2020) 584(7821):430–6. doi: 10.1038/s41586-020-2521-4
240. Vizcarra P, Pérez-Elías MJ, Quereda C, Moreno A, Vivancos MJ, Dronda F, et al. Description of COVID-19 in HIV-Infected Individuals: A Single-Centre, Prospective Cohort. Lancet HIV (2020) 7(8):e554–e64. doi: 10.1016/S2352-3018(20)30164-8
241. Dandachi D, Geiger G, Montgomery MW, Karmen-Tuohy S, Golzy M, Antar AAR, et al. Characteristics, Comorbidities, and Outcomes in a Multicenter Registry of Patients With Human Immunodeficiency Virus and Coronavirus Disease 2019. Clin Infect Dis (2021) 73(7):e1964–e72. doi: 10.1093/cid/ciaa1339
242. Sigel K, Swartz T, Golden E, Paranjpe I, Somani S, Richter F, et al. Coronavirus 2019 and People Living With Human Immunodeficiency Virus: Outcomes for Hospitalized Patients in New York City. Clin Infect Dis (2020) 71(11):2933–8. doi: 10.1093/cid/ciaa880
243. Shalev N, Scherer M, LaSota ED, Antoniou P, Yin MT, Zucker J, et al. Clinical Characteristics and Outcomes in People Living With Human Immunodeficiency Virus Hospitalized for Coronavirus Disease 2019. Clin Infect Dis (2020) 71(16):2294–7. doi: 10.1093/cid/ciaa635
244. Ssentongo P, Heilbrunn ES, Ssentongo AE, Advani S, Chinchilli VM, Nunez JJ, et al. Epidemiology and Outcomes of COVID-19 in HIV-Infected Individuals: A Systematic Review and Meta-Analysis. Sci Rep (2021) 11(1):6283. doi: 10.1038/s41598-021-85359-3
245. Fehr D, Lebouché B, Ruppenthal L, Brownc M, Obasc N, Bourbonnière E, et al. Characterization of People Living With HIV in a Montreal-Based Tertiary Care Center With COVID-19 During the First Wave of the Pandemic. AIDS Care (2021) 1–7. doi: 10.1080/09540121.2021.1904500
246. Ssentongo P, Ssentongo AE, Heilbrunn ES, Ba DM, Chinchilli VM. Association of Cardiovascular Disease and 10 Other Pre-Existing Comorbidities With COVID-19 Mortality: A Systematic Review and Meta-Analysis. PloS One (2020) 15(8):e0238215. doi: 10.1371/journal.pone.0238215
247. Richardson S, Hirsch JS, Narasimhan M, Crawford JM, McGinn T, Davidson KW, et al. Presenting Characteristics, Comorbidities, and Outcomes Among 5700 Patients Hospitalized With COVID-19 in the New York City Area. JAMA (2020) 323(20):2052–9. doi: 10.1001/jama.2020.6775
248. Huang J, Xie N, Hu X, Yan H, Ding J, Liu P, et al. Epidemiological, Virological and Serological Features of Coronavirus Disease 2019 (COVID-19) Cases in People Living With Human Immunodeficiency Virus in Wuhan: A Population-Based Cohort Study. Clin Infect Dis (2021) 73(7):e2086–e94. doi: 10.1093/cid/ciaa1186
249. Nault L, Marchitto L, Goyette G, Tremblay-Sher D, Fortin C, Martel-Laferrière V, et al. Covid-19 Vaccine Immunogenicity in People Living With HIV-1. bioRxiv (2021) 2021.08.13.456258. doi: 10.1101/2021.08.13.456258
250. Ruddy JA, Boyarsky BJ, Bailey JR, Karaba AH, Garonzik-Wang JM, Segev DL, et al. Safety and Antibody Response to Two-Dose SARS-CoV-2 Messenger RNA Vaccination in Persons With HIV. AIDS (2021) 35(14):2399–401. doi: 10.1097/qad.0000000000003017
251. Frater J, Ewer KJ, Ogbe A, Pace M, Adele S, Adland E, et al. Safety and Immunogenicity of the ChAdOx1 Ncov-19 (AZD1222) Vaccine Against SARS-CoV-2 in HIV Infection: A Single-Arm Substudy of a Phase 2/3 Clinical Trial. Lancet HIV (2021) 8(8):e474–85. doi: 10.1016/S2352-3018(21)00103-X
252. Klein MB, Lu Y, DelBalso L, Coté S, Boivin G. Influenzavirus Infection Is a Primary Cause of Febrile Respiratory Illness in HIV-Infected Adults, Despite Vaccination. Clin Infect Dis (2007) 45(2):234–40. doi: 10.1086/518986
253. Cooper C, Hutton B, Fergusson D, Mills E, Klein MB, Boivin G, et al. A Review of Influenza Vaccine Immunogenicity and Efficacy in HIV-Infected Adults. Can J Infect Dis Med Microbiol (2008) 19(6):419–23. doi: 10.1155/2008/419710
254. French N, Gordon SB, Mwalukomo T, White SA, Mwafulirwa G, Longwe H, et al. A Trial of a 7-Valent Pneumococcal Conjugate Vaccine in HIV-Infected Adults. Malawi Med J (2016) 28(3):115–22. doi: 10.1056/NEJMoa0903029
255. Glaser JB, Volpe S, Aguirre A, Simpkins H, Schiffman G. Zidovudine Improves Response to Pneumococcal Vaccine Among Persons With AIDS and AIDS-Related Complex. J Infect Dis (1991) 164(4):761–4. doi: 10.1093/infdis/164.4.761
Keywords: HIV, HIV reservoir, pulmonary immunity, lungs, alveolar macrophages, CD8 T-cell dysfunction, mucosal immunity, alveolar macrophage (AM)
Citation: Alexandrova Y, Costiniuk CT and Jenabian M-A (2022) Pulmonary Immune Dysregulation and Viral Persistence During HIV Infection. Front. Immunol. 12:808722. doi: 10.3389/fimmu.2021.808722
Received: 03 November 2021; Accepted: 10 December 2021;
Published: 04 January 2022.
Edited by:
Shokrollah Elahi, University of Alberta, CanadaReviewed by:
Matthias Clauss, Indiana University Bloomington, United StatesCamilla Tincati, University of Milan, Italy
Copyright © 2022 Alexandrova, Costiniuk and Jenabian. This is an open-access article distributed under the terms of the Creative Commons Attribution License (CC BY). The use, distribution or reproduction in other forums is permitted, provided the original author(s) and the copyright owner(s) are credited and that the original publication in this journal is cited, in accordance with accepted academic practice. No use, distribution or reproduction is permitted which does not comply with these terms.
*Correspondence: Mohammad-Ali Jenabian, jenabian.mohammad-ali@uqam.ca