- 1Department of Ophthalmology, Klinikum der Ludwig-Maximilians-Universität München, Munich, Germany
- 2Department of Pharmacy, Ludwig-Maximilians-Universität München, Munich, Germany
Microglia are the main resident immune cells of the nervous system and as such they are involved in multiple roles ranging from tissue homeostasis to response to insults and circuit refinement. While most knowledge about microglia comes from brain studies, some mechanisms have been confirmed for microglia cells in the retina, the light-sensing compartment of the eye responsible for initial processing of visual information. However, several key pieces of this puzzle are still unaccounted for, as the characterization of retinal microglia has long been hindered by the reduced population size within the retina as well as the previous lack of technologies enabling single-cell analyses. Accumulating evidence indicates that the same cell type may harbor a high degree of transcriptional, morphological and functional differences depending on its location within the central nervous system. Thus, studying the roles and signatures adopted specifically by microglia in the retina has become increasingly important. Here, we review the current understanding of retinal microglia cells in physiology and in disease, with particular emphasis on newly discovered mechanisms and future research directions.
Introduction
Microglia cells are the main resident immune component of the central nervous system (CNS), as opposed to those elements of the immune system that are circulating or do not reside in the parenchymal part of a tissue. First found by Franz Nissl at the end of the 19th century and addressed as “rod cells”, it is only after the characterization of neurons and astrocytes that Ramon y Cajal’s student del Rio-Hortega used the term microglia to indicate a “third component” of the brain that was distinct from oligodendrocytes (1). The initial source of this cell population has found little consensus for many years, during which several researchers supported the theory of the neuroglial origin advanced by Ford Robertson and Ramon y Cajal (2–5), as opposed to del Rio-Hortega’s theory of the myeloid origin. The latter has been long controversial, only to be accredited when the expression of markers typical of macrophages, which originate from myeloid precursors, was confirmed also in microglia (6–11). Not surprisingly, microglia are considered the main resident macrophage population of the CNS (12).
The retina is the neural region of the eye where visual stimuli are detected and receive a first elaboration prior to being sent to the visual cortex. During late embryonic development, it consists of retinal progenitor cells organized in a neuroblast layer (NbL), which progressively give rise to all cell populations in a timely regulated manner (13, 14). At later postnatal and adult stages, this highly specialized tissue is organized in a well-characterized circuitry, consisting of nuclear and plexiform layers. Rod and cone photoreceptors, bipolar and ganglion cells belong to the first category and constitute outer nuclear (ONL), inner nuclear (INL) and ganglion cell layer (GCL), respectively (14, 15).
Other cell types residing in these layers are Müller glia (in the INL), horizontal (INL) and amacrine cells (within INL and GCL). A synaptic layer between ONL and INL as well as between INL and GCL constitute the outer (OPL) and inner plexiform layers (IPL), respectively (14, 15).
“The only thing I know is that I know nothing” - the famous Socratic paradox holds partly true for retinal microglia, on which relatively little is known compared to the brain counterpart. Hence, it comes to no surprise that most literature on retinal microglia simply relies on the assumption that this population performs the same tasks observed in brain microglia. However, already within the brain, at least part of the microglial population harbors diverse degrees of heterogeneity depending on their localization (16–19). Further complicating the scenario, microglia display a high context-dependent plasticity, making the classification of all acquired identities (according to morphology, molecular signature and function) a matter of active debate (20). As such, in this review we will step back to have an unbiased look specifically at the microglia population in the Mammalian retina and to address its known roles in retinal physiology and pathology. We will try to shed some light on recently discovered mechanisms and evidence from experiments carried out specifically in retinal microglia, all the while bringing the attention to the many persisting gaps of knowledge.
Origin and consolidation of retinal microglia
Origin and retina colonization
In comparison to the brain, pinpointing the original source and temporal appearance of microglia cells in the retina has proven more challenging, possibly for the physical separation of this compartment from other CNS areas and for the relatively reduced size of this population (21, 22).
Although the origin of retinal microglia has not been confirmed specifically, fate-mapping studies performed on murine models have shown that microglia population as a whole is generated by erythro-myeloid progenitors located in the yolk sac (23–30), in particular from a sub-population that does not express the transcription factor Myb (31, 32). Several studies have determined that a cohort of transcriptional regulators, such as Spi1 (encoding for the protein PU.1), Irf8 (10, 24) and Csfr1 (33–35) is crucial for microglia generation. The mutation or deletion of either of these genes impairs, and in some cases completely abolishes, the production of microglia.
After differentiation, microglia invade the CNS in two consecutive waves. Following the first wave, microglia cells reach the brain around embryonic day (E)8.5. The colonization of the retina, instead, has been determined as early as E11.5 in rodents (10 weeks of gestation in humans), though earlier time-points have not been extensively addressed (36–39) (Figure 1). At this time, vascularization has yet to take place and the vitreo-retinal interface and/or the ciliary margin zone (CMZ) have been indicated as main entry routes (36, 40, 41). The second wave is observed around birth, when the retinal vasculature is in the process of being established (Figure 1). Initially, microglia cells migrate tangentially at the interface with the ganglion cell layer, where the primary plexus of the vasculature is located (7, 42). Until postnatal day (P)10, they progressively invade the retinal tissue migrating in a radial fashion, in order to colonize the IPL first and the OPL later, while following the formation of the deeper vasculature plexus (7, 36, 40, 43, 44) (Figure 1).
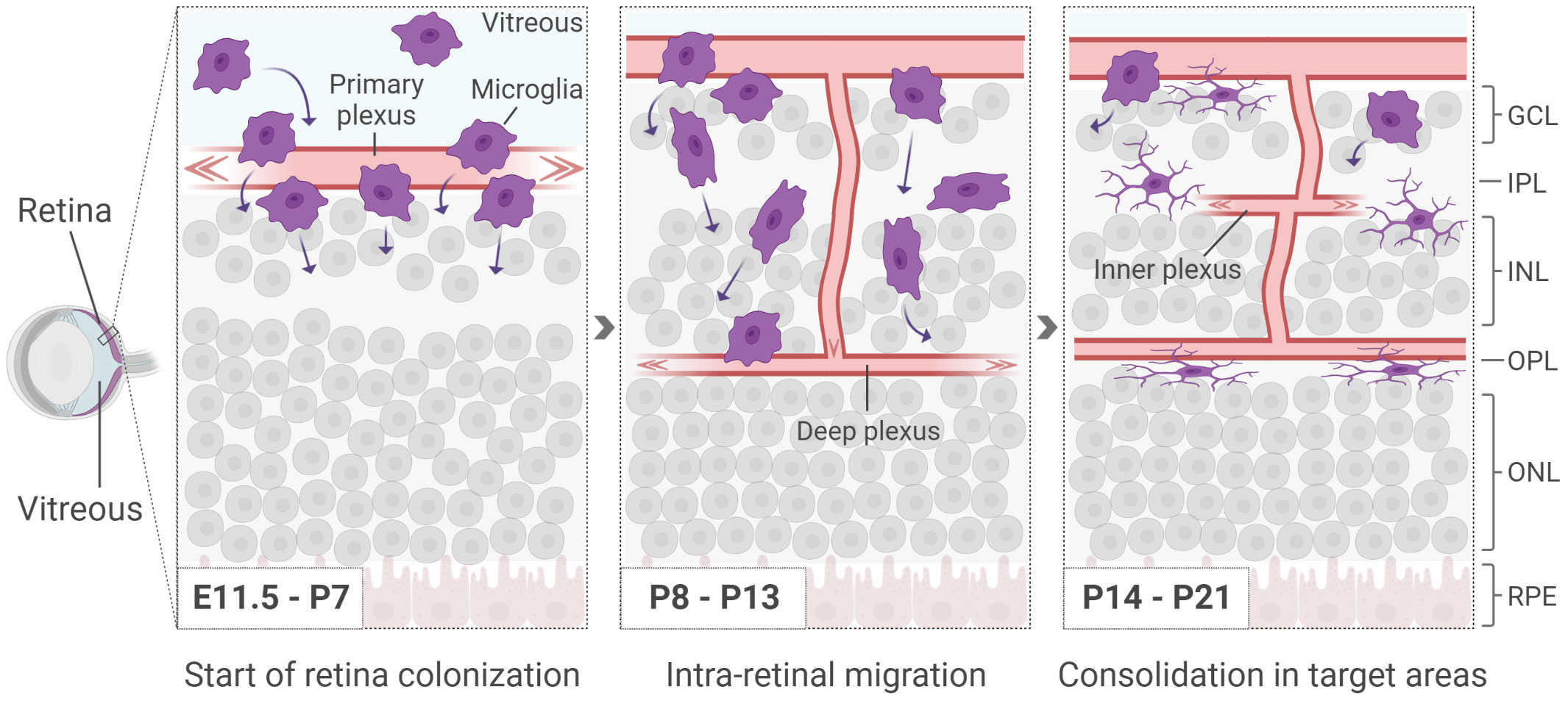
Figure 1 Microglia start appearing in the retina around E11.5, before the formation of the inner vasculature. At early postnatal days, as the deeper vasculature plexus develop, microglia infiltrate the retina in a radial manner, colonizing first the IPL and subsequently the OPL. Retinal cells are shown simplified. GCL, ganglion cell layer; IPL, inner plexiform layer; INL, inner nuclear layer; OPL, outer plexiform layer; ONL, outer nuclear layer; RPE, retinal pigmented epithelium.
The key drivers of the radial migration are not fully uncovered yet. However, microglia have been shown to be naturally attracted to sites with increased stiffness such as the ONL, a process referred to as durotaxis (45–47). Once the IPL and OPL have been colonized, microglia persist in these territories until adulthood, forming a network with a constant intercellular distance that is likely to be acquired via repulsive mechanisms (48). Additionally, microglia are found in the retinal nerve fiber layer (NFL) adjacent to ganglion cells and occasionally with their soma in the INL. The ONL is the only retinal region where no microglia are observed in physiological conditions (36).
Repopulation routes and microglia turnover
The events underlying retina colonization have been additionally explored at adult stages, in order to shed light on the migratory behavior of microglia in a context where circuitries are already established and consolidated. To this end, murine experiments of pharmacologic or genetic ablation of microglia have shown that this cell population is capable of rapidly repopulating the retina and that the employed routes partly differ from those followed by microglia during development (49, 50). Indeed, following ablation at adult stages, most microglia cells origin from infiltration through the optic nerve and subsequent center-to-periphery migration, while repopulation occurs only to a lesser extent via invasion from the CMZ (49, 50). Cells that have already migrated, as well as cells that have survived ablation, further contribute to repopulation through local proliferation. Interestingly, migration within the retina seems to follow the same steps seen during development, with a radial distribution pattern starting at the interface of the GCL followed by colonization of IPL and OPL (49, 50). Thus, while cellular tropism per se relies on rather conserved mechanisms, and the retina environment remains seemingly permissive to (re)colonization even at adult stages, the cells contributing to repopulation are different in identity and location source, and mainly consist in microglia cells within the optic nerve that have survived the ablation as well as, to some extent, infiltrating macrophages (49, 51–53).
Supporting this evidence, retinal microglia show very little turnover in physiological conditions (29, 30, 54, 55), indicating that local proliferation might be mainly restricted to extreme cases of retinal tissue alteration and/or microglia depletion. Indeed, past studies making use of irradiation as a mean to deplete microglial population suggested a higher turnover rate promoted by infiltration of peripheral monocytes (56, 57). However, these results were the direct consequence of the impairment of the blood-retinal barrier caused by the experimental methods adopted, rather than of physiological turnover.
Thus, retinal microglia appear as a highly stable cell population, which, however, retains the ability to properly colonize and integrate in the adult retina following an alteration of its numbers.
Retinal microglia morphotypes across development
During the processes leading to retina colonization and proper integration, as seen across development, microglia cells adopt different morphologies. Similarly, the pathophysiological condition of a tissue and the location within the CNS correlate with microglial differences in size, polarization and complexity of arborization. In the retina, the first microglia cells entering the tissue are amoeboid in shape (Figure 1). Once at their final destination, their morphology changes either to a shape with long radial ramifications (within the IPL) or an elongated shape with highly polarized ramifications (within the OPL; Figure 2) (22, 47). The ramified morphology established during development has been indicated as a sign of quiescence, leading to the definition of “resting microglia”. Though the term might suggest the contrary, microglia in a resting state are rather active in tissue surveillance, constantly scanning the environment for any sign of physiological or pathological alterations (58–64). These processes are thought to be mediated by several extracellular cues, as already extensively described in other literature reviews (65–67). Examples of such cues are Cx3CL1, CD200 and ATP/ADP, which are detected by microglial receptors Cx3CR1, CD200R and P2RY12, respectively (68–73). Although it was suggested that the differential tissue stiffness of INL and ONL may impose the degree of microglial polarization (47), it remains unclear whether in homeostasis microglial morphotypes merely reflect the diversity a) in the tissue environment, b) in the functional states in response to local cues (59, 74–79), c) in the intrinsic molecular program, or d) in a mix of all of these. The evidence of distinct molecular signatures for some microglia sub-populations in various areas of the CNS might support the presence of a diverse molecular program defining not only retinal microglia as a whole (16–19, 30, 79–81), but also microglia within the same tissue, i.e., in IPL versus OPL regions. Recent findings have revealed that only IPL microglia cells depend on the Csfr1-IL-34 signaling (29), further prompting the importance of non-cell autonomous cues in the definition of microglial states and, possibly, identities. Additional studies are necessary to fully uncover the molecular program characterizing the IPL and OPL sub-populations, the factors influencing the correct positioning in either one of these plexiform layers and the distinct roles they might play in health and disease.
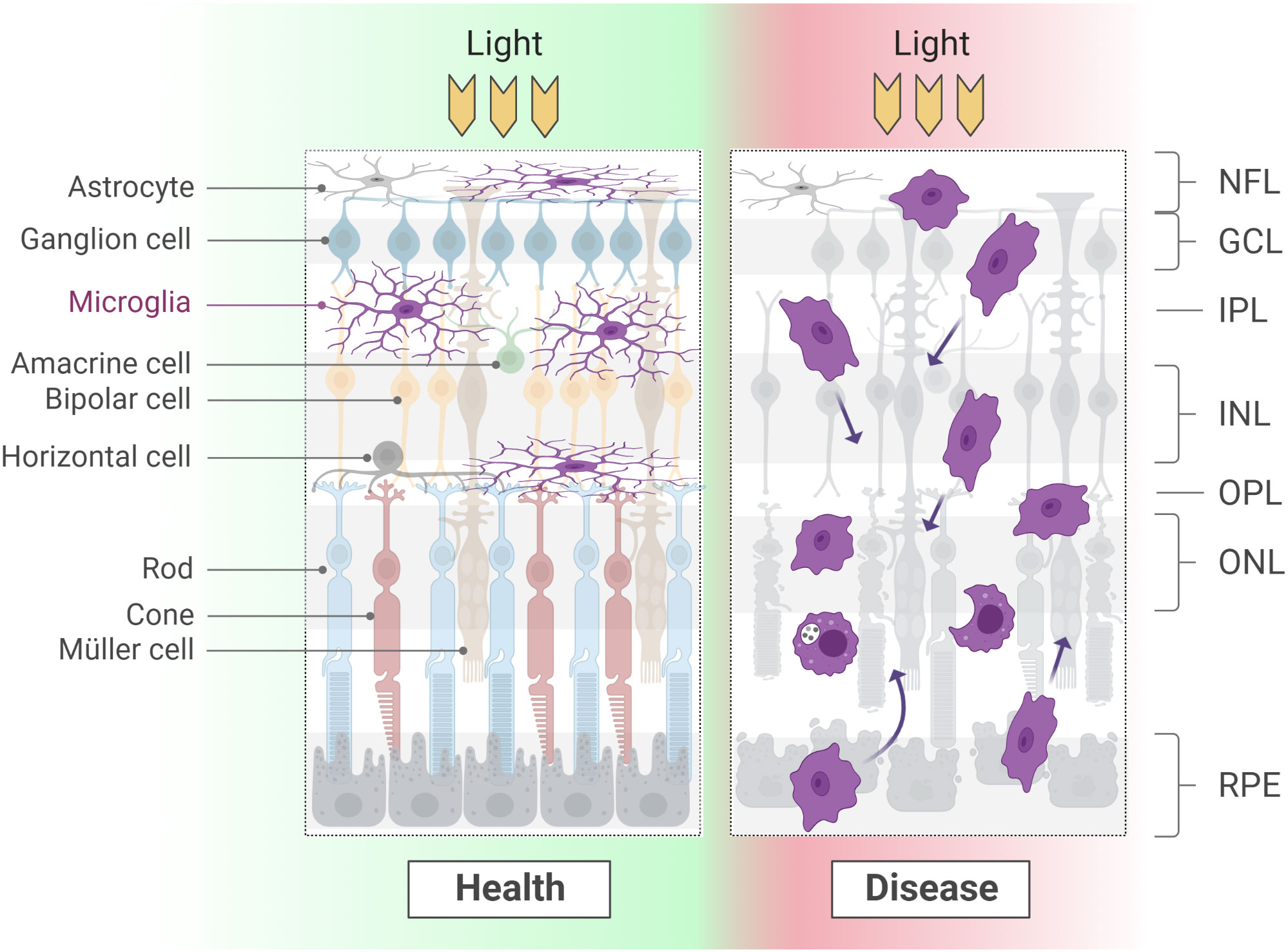
Figure 2 Microglial morphology varies considerably according to the developmental and physio-pathological state of the tissue. At adult stages, a long and thin arborization confers microglia the ability to scan large areas of the surrounding environment. When a degenerative process starts, microglia retract the branches and display high motility and phagocyting activity. Of note, while in health microglia occupy the synaptic layers and line ganglion cells fibers, during degeneration these boundaries are lost. NFL, nerve fiber layer; GCL, ganglion cell layer; IPL: inner plexiform layer; INL, inner nuclear layer; OPL, outer plexiform layer; ONL, outer nuclear layer; RPE, retinal pigment epithelium.
At postnatal and adult stages, the acquisition of an amoeboid morphology is instead associated with a reactive state, following the onset of a degenerative pathology or the occurrence of an injury (82–85) (Figure 2; see section Roles of microglia in pathology).
Roles of microglia in physiology
As discussed in the previous section, the presence of microglia in the retina has been determined as early as E11.5. From this point, the density of microglia in the mouse retina increases to such an extent that by P7 it accounts for double the density observed in the adult (22, 36, 86). As local cell proliferation has been ruled out during this phase, the likely explanation for the observed increase is the ingress of new microglia cells (36). Conversely, a decrease in density follows during the second postnatal week and it has been accredited to the expansion of the retinal size together with the absence of new microglia cells entering the tissue (36, 41). Of note, changes in absolute numbers are not known and selective cell death at these stages has not been ruled out. During physiological aging, instead, the number of microglia cells increases in the subretinal and perivascular space (87).
In order to elucidate the roles retinal microglia play during development, the attention has been turned to the physiological events taking place in the retina during microglia invasion and during their changes in density, using the acquired knowledge on brain microglia as reference. In the following sections, we will mainly address the result of studies in the murine retina, unless differently specified.
Vasculature development
As addressed in section Origin and retina colonization, early microglia progenitors colonize the retina in two consecutive waves. Particularly the second wave takes place around the time retinal vasculature appears and shares with it the same pattern of invasion, i.e., from vitreo-retinal interface to deep retina layers (7, 36, 40, 43, 44, 88). This temporal and spatial correlation raised the question of a potential link between microglia cells and formation of new blood vessels in the retina. Indeed, during development microglia are found in close proximity to nascent blood vessels and extend branches to contact endothelial cells and pericytes (37, 88, 89). Several receptors, including Mas1 and Notch1, are likely to mediate the active localization of microglia in these regions, as mice lacking their expression show reduced numbers of microglia near sprouting blood vessels and general defects in vasculature formation (90, 91).
Further substantiating a role for microglia cells in retinal vascularization, inducing microglia ablation around birth causes a reduction of the area covered by blood vessels and a general impairment of the vasculature, whose growth is, however, rescued when new microglia cells are transplanted (88, 92). Transforming growth factor (TGF) β1, in particular, has been indicated as the main microglial mediator of vascular growth and its tight regulation is key in promoting physiological vascularization (47, 93).
During postnatal development, microglia are additionally involved in vasculature regression to help refine the blood vessel landscape, as seen both in mouse (94) and human retina (92). This process seems to be mediated by several signalling factors, such as Wnt, macrophage colony stimulating factor (M-CSF) and angiopoietin (94–97). In addition to vascular regression, microglia/macrophages mediate suppression of blood vessel branching via vascular endothelial growth factor (VEGF)-Flt1 axis, acting in conjunction with non-canonical Wnt signalling (98).
All in all, experimental proof supports the contribution of microglia in the development of retinal vasculature, especially by exercising an important inhibitory role on vascular sprouting. Of note, astrocyte progenitors are, instead, known to actively promote vascularization (40, 99, 100). Therefore, microglia are seemingly involved in vasculature development also indirectly, via regulation of the numbers of retinal astrocytes (101).
Synaptic refinement
Aside from the already mentioned role during vascularization (47, 88, 92, 94–96, 98, 102–104)(see previous section), shaping the nascent synapses is considered one of the main functions of microglia. Synapses in the retina are established on the one hand among photoreceptors, horizontal and bipolar cells, on the other hand among bipolar, amacrine and ganglion cells. At late embryonic stages in the murine retina, the synaptic marker synaptophysin is detected in the photoreceptor outer segments (13). As early as P5, the same marker can be found in both IPL and cones, becoming restricted to pre-synaptic terminals of photoreceptors from P10 and, finally, being detected only in IPL and OPL from the second postnatal week (13, 105). Arborizations and synapses keep on being consolidated and refined from eye opening (P13-14), following an activity-dependent scheme and resulting in more spontaneous synaptic inputs (106).
Temporally and spatially, microglia invasion of the retina follows the same pattern seen in the development of cell-cell connections. Together with this observation, the known role of brain microglia in pruning of synapses during development (106–108) prompted the idea that similar mechanisms might concur to shape the retina circuitry. In this regard, it was shown that microglia regulate the extent to which the dendrites of horizontal cells are extended via the complement protein C1q (107). As such, C1q-depleted mice show a progressive invasion of horizontal cells dendrites into the ONL from P13 on, at the time when the synapses in the OPL are being consolidated. While any other direct evidence of retinal microglia involvement in pruning during development has yet to be uncovered, brain microglia have been described to refine presynaptic terminals of ganglion cells in the lateral geniculate nucleus (LGN) through complement protein activity (108, 109). Interestingly, the impairment of Cx3CL1-Cx3CR1 signaling in mice, particularly important for microglia activity (110), was associated with the extension of microglia branches inside the ONL and morphological and functional alterations in photoreceptors during early postnatal development, particularly in cones (111). Similarly, specific depletion of microglia at adult stages was shown to affect photoreceptor synapses and visual performance (112, 113). Of note, an additional role for microglia in axon guidance of ganglion cells through nerve growth factor (NGF) signaling was suggested (114), thus corroborating a multi-faceted spectrum of activities for microglia also in the context of the retina.
Developmental cell death
Strictly interconnected with synaptic refinement, and, therefore, proper neuronal integration, is the regulation of cell numbers. The early developmental phases of the CNS have been associated with extensive cell death. Both cell autonomous and non-cell autonomous processes concur to eliminate exuberating cells and/or newborn neurons that fail to establish a proper connection with their targets (115, 116). Such mechanism also takes place within the retina, where three waves of programmed cell death (PCD) have been described: morphogenic, early neural and neurotrophic cell death (117–120).
Morphogenic cell death is found in the neuroepithelium of the eye primordia during embryonic development, peaking at E10.5 and extending until E14.5 (117, 121). Its name reflects the contribution of this PCD to the processes that shape the optic cup during this time. Early neural cell death, instead, occurs during E15-E17 and involves retinal ganglion and amacrine cells, namely the first cell types derived from retinal progenitors (122–124). NGF signaling acting through p75 receptor has been indicated as the trigger of this apoptotic wave (125). Finally, late or neurotrophic cell death coincides with the establishment of connections with extra-retinal structures at postnatal stages and affects several cell populations (118, 126, 127). Between P4 and P10, TGF signaling triggers the correct expression levels of NGF, which, contrary to the detrimental effects mediated by p75 during embryonic development, promotes survival of retinal neurons, possibly through activation of TrkA receptor (128). The same is observed in the adult retina, where TGF-β1 is involved in the maintenance of ganglion cells (129). Of note, a bystander cell death effect has also been described at early postnatal stages (130).
The role of microglia throughout these steps is still mostly circumstantial. Microglia in early phases of retina colonization has been observed in proximity of dying cells (7, 39, 114). From E12.5 until birth, retinal microglia cells predominantly localize in the compartment containing already differentiated cells, while in the NbL almost 50% of microglia cells establishes contacts with newborn migrating ganglion cells. Interestingly, mainly non-apoptotic ganglion cells undergo phagocytosis after being marked with complement proteins (41), a process defined phagoptosis (131). However, a previous study indicated that microglia do uptake remnants of apoptotic ganglion cells that had been retrogradely labelled with Fluorogold or (4-[4-didecylaminostyryl]-N-methylpyridinium iodide (4Di-10ASP) (44). Thus, it is unclear whether microglia merely adopt a scavenging role or rather an active role in the phagocytosis of dying retinal cells.
Moreover, aside from regulating the number of ganglion cells, microglia seem to influence the number of other retinal populations, such as astrocytes (101).
Cell survival and proliferation
In addition to cell death, proliferation and cell survival act as direct regulators of cell numbers. Similar to the previously addressed roles, recent evidence links brain microglia population and progenitor cell behavior. While a bidirectional cross-talk with neural stem cells and progenitors has been extensively described both during development and at adult stages (132, 133), the contribution of microglia to similar mechanisms in the retina is limited to selected examples. During early postnatal development, the number of proliferating progenitors can be increased by lipopolysaccharide (LPS) administration, whose primary effect is microglia activation and proliferation (86). Accordingly, microglia depletion results in decreased progenitor proliferation. Knockout of microglial-expressed gene progranulin (grn) in mice has been shown to result in a decrease in numbers of both microglia and retinal progenitors (86). In agreement with this, activated microglia have also been shown to promote cell survival in retinal explants from P10 postnatal mice, as interfering with their activation or promoting their ablation leads to decreased viability of retinal cells (134).
As already addressed, it is worth noting that microglia have also been associated with proper cone maturation via Cx3CL1-Cx3CR1 signaling and maintenance of cone homeostasis at adult stages (111, 112). Though intriguing, the nature of this selective relationship between microglia and cone cells has yet to be fully characterized.
Roles of microglia in pathology
As befitting cells participating in the immune response of an organism, microglia adopt a more active (and overall, better characterized) role in pathology. Consequently, understanding the behavior of microglia in disease can help uncover additional functions adopted in physiological conditions.
Several factors expressed in the healthy mouse, rat and human retina serve as signals for microglia to remain in a non-reactive state, e.g., CD200 and Cx3CL1 (60, 70, 71, 135). The loss of retinal homeostasis resulting from a physical insult or a degenerative process causes an alteration in the levels of these and additional factors, thus leading to microglia activation and recruitment. These events correlate with the acquisition of an amoeboid morphology and a highly migratory behavior that brings microglia cells to invade nuclear layers (particularly the ONL) commonly devoid of immune cells (82–85). It was proposed that activated microglia adopt distinct transcriptional features depending on whether a mostly pro-inflammatory (M1) or anti-inflammatory (M2) program is initiated. However, these definitions are largely stereotyped and possibly more suitable for monocytes (20, 74, 136–140). The rapid expansion of high-throughput methods, particularly single-cell RNA sequencing, has recently enabled a more granular characterization of several phenotypes (and morphotypes) acquired by microglia in different brain areas and conditions (18–20, 138, 141, 142). Most of these definitions, including disease-associated microglia (DAM), proliferative-region-associated microglia (PAM), axon tract-associated microglia (ATM) and cd11c+ microglia, have recently found correlation also in mouse retina, specifically at postnatal stages during developmental apoptosis and in several paradigms of degeneration (143–149).
Although categorizations often suggest stereotyped responses in well-defined contexts, the role of retinal microglia during degeneration is multifaceted. Here, we will review the current literature on microglia in the context of severe sight-threatening eye diseases that are predominantly associated with retinal degeneration.
Retinitis pigmentosa
Among inherited retinal diseases (IRDs), retinitis pigmentosa (RP) is the most prevalent form and includes a spectrum of diseases that can be generally categorized in syndromic (= affecting various organs) and non-syndromic (affecting only the retina) (150, 151). Initially characterized by rod photoreceptor loss in a peripheral-to-central gradient, the degeneration soon extends also to cones. RP patients notice nyctalopia and impaired dark adaptation already at early stages of the disease, and suffer from a progressive, usually concentric, visual field impairment. Currently, no remedy exists for the various forms of RP, although several different approaches have been tried and many others are currently under investigation (152, 153). The sole important exception is represented by voretigene neparvovec, an AAV-based gene therapy specifically designed for the treatment of retinal dystrophies caused by RPE65 bi-allelic mutations, although the latter ones account only for a very small proportion of all RP cases (152–154).
Early RP is characterized by progressive loss of rod photoreceptors. As discussed in the opening of this chapter, the loss of photoreceptors per se deprives microglia of signals important for their homeostasis, though sudden hyperoxidative environment, oxidative stress and alteration of retinal metabolism may also concur to foster and sustain microglial response (155–160). Similar to what happens throughout the CNS, retinal microglia usually respond by adopting an amoeboid morphology and migrating to the area of insult, ultimately proliferating to amplify their numbers and acting to resolve the ongoing degeneration. In the context of RP, as seen in mouse models, activation of microglia cells leads to their migration into the ONL, production of pro-inflammatory cytokines and phagocytosis of viable photoreceptors, which further contributes to disease progression (65, 161–164).
Thus, it is reasonable to speculate that activating pathways involved in microglia homeostasis might concur to ameliorate the effects of the degeneration. In this scenario, Cx3CL1 administration to well-established mouse models of RP (Pde6brd1 and Pde6brd10 mutants, also known as rd1 and rd10, respectively (165, 166), has been shown to improve survival of photoreceptors, though the positive effects may not be necessarily mediated by microglia (167, 168). In agreement with the homeostatic role of Cx3CL1-Cx3CR1 signaling, loss of photoreceptors is exacerbated in mice lacking Cx3CR1, possibly due to the inability to activate downstream pathways that would normally maintain microglia in a resting state both in mice and humans (72, 162). A similar result was described for insulin growth factor (IGF-1), whose anti-apoptotic effect seems to be mediated by newly recruited microglia cells (169). In addition to Cx3CL1 and IGF-1, TGF-β1 administration has also been shown to ameliorate cone survival in mice by reducing the pro-inflammatory effect of microglia (170).
Although involved in removal of hindered photoreceptors and in scavenging of cellular debris, microglia have been shown to additionally phagocytize seemingly healthy photoreceptors (171–173), as already observed for ganglion cells during development (41). These observations prompted to think that microglia depletion, rather than mere modulation, would considerably hinder disease progression. Indeed, interfering with phagocytosis or selectively inducing microglia cell death has been shown to ameliorate retinal cytoarchitecture and overall visual function in Pde6brd10 mice (171). However, a recent work has challenged this view by indicating a protective effect of microglia on cones in the same mouse model (174), as suggested by the discussed role of microglia in cone homeostasis (111). In rats, treatments affecting microglia numbers have resulted in reduced visual function, as a consequence of an increased apoptotic rate in retinal neurons (172, 175). Partially explaining this protective role, microglia are not only involved in phagocytosis of stressed photoreceptors, but also in refinement and clearance of improper synaptic connections attempted by bipolar cells with remaining photoreceptors. Such role seems to be mediated by C1q expression in the dendrites of bipolar cells (172), while both complement protein C3 and its receptor (C3R) have been implicated in pruning of photoreceptor synapses (173). Genetic depletion of either C3 or C3R aggravated photoreceptor death in the already affected retina. Similar results hinting at a neuroprotective role of microglia were obtained in a mouse model of retinal detachment (176) and of excitotoxicity (177). Interestingly, signs of excitotoxicity were also found in Pde6brd1 mutants, possibly justifying similar microglia responses in different degenerative contexts (158, 178).
Thus, it is conceivable that microglia attempt to contain the degeneration by targeting both dying and viable (albeit already compromised) photoreceptors. Impairing microglia numbers or their phagocytic ability soon after infiltrating the ONL leads to an accumulation of stressed and/or dying photoreceptors, which seems to transiently ameliorate the progression of the disease. In later stages of the disease, however, the ONL is affected in the same way as in untreated retinae, possibly due to the inability of the remaining photoreceptors to maintain proper connections with bipolar cells. Microglia cells surviving the depletion might also concur to aggravate the degeneration when their phagocytic ability is impaired.
Glaucoma
Aside from IRDs, the most common cause of irreversible blindness as of 2020 is glaucoma (179), which encompasses a spectrum of heterogenous diseases whose underlying etiology is still largely unknown (180, 181). The common leitmotif in affected patients is a gradual degeneration of the retinal ganglion cells, the associated optic nerve and the supporting connective tissue, which become ophthalmologically apparent as an excavation of the optic disc (180, 181). An elevated intraocular pressure (IOP) is the most common risk factor and, currently, the main target of treatment (182).
Although the principal factors driving glaucoma are still under investigation, the involvement of the immune system in the pathology is rather established (183–191). Indeed, complement and endothelin systems have been found to be overrepresented in both patients and animal models of glaucoma even before the appearance of other phenotypic effects (183, 190, 192–210). Interestingly, antagonizing C1qa protein produced by ganglion cells, microglia and macrophages exerts positive effects on glaucoma progression (183, 195, 197, 211), whereas mice genetically depleted of C3, produced by astrocytes, show an aggravation of the phenotype (196). In contrast, attenuation of C3 production specifically in the retina resulted in increased survival of ganglion cells, suggesting that the levels of certain complement factors in this locally restricted context play a complex role in the onset and progression of the pathology (194).
In the context of glaucomatous optic atrophy, microglia localize to the optic nerve head, where they locally proliferate and release inflammatory molecules such as tumor necrosis factor (TNF)-α and TGF-β (212–214). Their recruitment precedes or coincides with the actual onset of degeneration, possibly as a consequence of the local complement accumulation (191, 208, 215). Following ganglion cell damage and subsequent degeneration, retrograde labeling of the optic nerve leads to simultaneous labeling of retinal microglia, indicating a central role of the latter population in the clearance of debris and removal of dying ganglion cells (216, 217). Interestingly, in models of unilateral glaucoma induction, e.g., via laser-induced ocular hypertension (OHT), microglia cells have been shown to react in the unaffected contralateral control eye, though to a lesser extent (218–221). In addition to the canonical signs of activation, such as increase in overall cell size, migratory behavior and reduction in arborization, the extension of microglial processes from the OPL to the photoreceptor outer segments is particularly pronounced in the control eye (219, 220). Such feature has also been observed in some mouse models of age-related macular degeneration (AMD) (222). These activated microglia cells seem to adopt a more pro-inflammatory state, as the expression of M2-polarization marker Ym1 was not detected (221, 223). However, microglia have been shown to assume a rather anti-inflammatory identity in other glaucoma models (224). These observations highlight the importance of both context and choice of disease models and further substantiate the complexity and plasticity of the microglia population in response to different stimuli.
Not less importantly, microglia have been shown to facilitate monocytes recruitment and infiltration, two processes that are usually mediated by the presence of the chemokine Ccl2 in the tissue and the expression of its receptor Ccr2 on these cells (225), though also activated microglia is able to express Ccr2 in particular degenerative conditions (226). Monocytes have been shown to infiltrate the retina of rodent glaucoma models and to promote degeneration. Accordingly, their depletion via radiation treatment, or inhibition of their entry via chemical treatment or Itgam knockout (also known as Cd11b, the alpha subunit of C3R), results in extended neuroprotection (187, 188, 209, 227). Similar results were obtained by hindering monocyte recruitment through the inhibition of TNF-α or IL-1β activity (228). Of note, while low doses of Ccl2 promote survival of retinal ganglion cells, seemingly mediated by microglia, studies performed in rats suggest that high doses accelerate their loss (229). Thus, a key aspect of microglia behavior is the context-dependent modulation of its state/activation. However, the mechanisms linking Ccr2 to such dual role are not fully uncovered.
Age-related macular degeneration
AMD represents the most common cause of irreversible central vision loss and its prevalence is expected to increase dramatically within the next decades (179, 230, 231). Early and intermediate AMD is characterized by the formation and expansion of extracellular deposits (so-called drusen) beneath the retinal pigment epithelium (RPE) or in the subretinal space (231, 232). In advanced stages, AMD either progresses to a neovascular (wet) form or leads to progressive cell death without any neovascular component (dry AMD with geographic atrophy). Wet AMD is associated with the development of new blood vessels (choroidal neovascularization, CNV), leading to rapid vision loss through exudative macular edema, subretinal/intraretinal bleedings and progressive scarring of the central retina. Dry AMD progresses to geographic atrophy, characterized by a complete loss of both photoreceptors and RPE in the macula (233). Importantly, these two late forms of AMD are not completely exclusive, as either one can eventually evolve into the other (231, 232).
Similar to glaucoma, the presence of complement proteins in drusen is per se a strong indication of the involvement of the innate immune system in AMD and, indeed, macrophages and microglia have been found in its association (234–239). This observation is especially true for the neovascular form of AMD, where complement has been shown to be associated with an increase in the levels of the pro-angiogenic VEGF (234, 240), partly contributed by microglia and macrophages (241–246). Anti-VEGF drugs are indeed to date the only approved therapy for AMD, though only the exudative form is treatable (247, 248).
Microglia exceptionally found in subretinal space is one of the hallmarks of AMD and is thought to have both protective and detrimental effects on RPE and photoreceptors (55, 249–253). The colonization of this area is achieved by the mobilization and subsequent proliferation of the retinal microglia population as well as infiltration of circulating monocytes (30, 55, 64, 243, 253–258). Aged mice lacking Ccr2, its ligand Ccl2 or Cx3CR1 have been shown to develop retinal lesions that are reminiscent of human AMD (240, 259–263) and, indeed, mutations in CX3CR1 gene have been found also in patients affected by AMD (259, 264–267), but not mutations in CCR2 (268). The infiltration of microglia in the subretinal space of these mouse models is likely to induce para-inflammation and determine cellular death (260). Moreover, microglia could also be involved in (and/or be activated by) the production of reactive oxygen species (269), which, together with changes in cellular metabolism, are considered among the possible causes of AMD (270–272). Notably, another cohort of studies showed that mice deficient for Cx3CR1 and/or Ccl2 do not develop AMD and that only the latter mouse line displays microglia/macrophages accumulation in the subretinal space (273). Such an accumulation of lipid-bloated immune cells can be easily mistaken for AMD-related lesions during ophthalmologic inspections, but would rather be a natural consequence of aging, as observed in aged control mice (87, 261, 262, 274). Possibly unifying these seemingly contradictory results is the discovery of the confounding mutation rd8 in C57Bl6/N mice, which were used to generate most of these mutant mouse lines (275). However, a common view on the matter has not been achieved and the subject remains under debate (222, 276, 277).
The presence of both microglia and infiltrating monocytes/macrophages, which share many molecular characteristics and functional properties, renders distinguishing their individual contribution to diseases particularly challenging (278). Nevertheless, potential treatments alleviating or hindering the progression of AMD through the modulation of the immune system are likely to act on both cellular populations. Among those, interferon-beta (IFN-β) administration in the laser-induced model of CNV was shown to attenuate the phagocytic activity of both microglia and macrophages, whereas the knockout of IFN-β receptor (Ifnar1) promoted neovascularization (279). Similarly, the reduction or loss in TGF-β signaling has been associated with microglia reactivity and aggravation (but not causation) of AMD (254). However, the literature on this topic is still largely controversial, partly due to the high degree of pleiotropism displayed by TGF-β signaling both in animal models and in patients (280–286).
Furthermore, the blockade of adenosine receptor A2AR activity has been shown to decrease reactivity and complement deposition in human microglia cells, all the while enhancing clearance of photoreceptor debris and improving cell survival (287).
Interestingly, the endolysosomal system can also be manipulated in order to alleviate wet AMD conditions. Indeed, our group has recently shown that interfering with two-pore channel 2 (TPC2) function on endolysosomes in a model of laser-induced AMD leads to reduced recruitment of retinal microglia/macrophages (288). Moreover, Tpc2 knockout determines a reduction in neovascularization via a decrease in both VEGF and IL-1β levels, the latter being a pro-inflammatory interleukin that has been already associated with photoreceptor degeneration and pathological neovascularization (288–290). Further studies are currently undergoing to better characterize the mechanisms linking TPC2 to the beneficial effects observed.
Retinal vascular disorders
Microglia are involved in multiple additional retinopathies, although their role is not always fully characterized. Among those whose incidence is expected to increase in the coming years there is diabetic retinopathy (DR) – a condition triggered by systemic diabetes and initially determining vascular abnormalities in the retina (291). Both in mice and human, ganglion cells, amacrine cells and, to a lesser extent, photoreceptors undergo apoptosis already at early stages (292–294), but signs of neurodegeneration may occasionally appear even before changes in retinal vasculature become appreciable (295–297). At advanced stages, ischemia and reactive oxygen species aggravate the scenario by triggering de novo vascularization in vitreous and vitreo-retinal interface (298, 299). As a consequence of all these vascular alterations, vision loss ensues from diffuse edema, in particular in the macular region (298).
Per se, ischemia and hyperglycemia are potent activators of microglia, as indicated in several in vitro studies (300) and further observed in mice (88, 301–303) and rats in which type 1 diabetes was induced with streptozotocin (STZ) (89, 304–308) as well as in human patients (294, 309, 310). As described for other pathologies, activation includes events like proliferation, retraction of processes and acquisition of an amoeboid shape. In DR, microglia undergo these morphological changes before cell death is observed, supporting their involvement at very early stages, but not clarifying their role in disease onset and progression (311). Interestingly, in a mouse model of DR, systemic and local level of cytokines (such as IL-4, IL-13, TNF-α and IL-1β) indicated that microglia cells initially engage in an anti-inflammatory response, which is then almost completely lost in favor of a pro-inflammatory response at later stages (312). Thus, while microglia might not contribute substantially to DR onset, they rather sustain its progression, but only when attempts aiming at quenching the increasing inflammation are exhausted.
Retinal microglia have been shown to contact pericytes and blood vessels and regulate blood flow through Cx3CL1-Cx3CR1 axis, particularly by promoting vasoconstriction when the pathway is activated (37, 88, 89). At early stages in rat models of DR, in the absence of any sign of activation, microglia increase contact with capillaries and pericytes, thus causing an overall reduction in retinal blood flow (89). However, in a similar time window, a different work has determined that fractalkine levels in the retina are lower, with consequent increase in retinal inflammation (308). Further studies are required in order to assess the contribution of Cx3CL1-Cx3CR1 axis in DR and the associated role of microglia.
Similar to all other retinopathies, alterations in the complement system have been described for patients affected by DR and provide an additional link between microglia activity and disease progression (313, 314). Interestingly, though the mechanisms are not entirely elucidated, IgG-laden exosomes in the plasma have been found increased in DR and associated to complement activation (315). Additional efforts are needed to pinpoint the correlation between exosomes and DR onset.
Aside from DR, retinal vascular occlusion (RVO) is a common cause of vision loss, with vein occlusions being more common than arterial occlusions. The prevalence of both vein and arterial occlusions rises with age and is strongly associated with cardiovascular risk factors such as hypertension, diabetes and cardiovascular disease (316). Typically, an ischemic injury results from impaired blood circulation and leads to inflammatory responses, including microglia activation and proliferation. Moreover, cell death eventually affects retinal ganglion cells, which are particularly vulnerable to ischemic conditions (317–324).
In a well-established rat model of high intraocular pressure, a strong increase in retinal microglia was observed following an ischemic insult and suggested the involvement of this cell population in RVO (318, 322). As such, it was speculated that microglia depletion and/or modulation could result in reduction of the local inflammation and general neuroprotection. Indeed, microglia depletion in mice with experimental branch RVO exerts a protective effect on retinal ganglion cells (325–327). Additionally, modulating microglial activity via selective inhibition of A2A receptor prevents microglia-mediated neuroinflammation and protects retinal ganglion cells from transient ischemic injury (322, 328). Similar neuroprotective effects are exerted by treatment with the antibiotic minocycline, specifically via induction of IL-4 expression and polarization of microglia/macrophages towards an M2 phenotype, as observed in mice with induced retinal ischemia (324).
Thus, these examples illustrate the relevance of microglia in disease development and its therapeutic potential in retinal vascular occlusion. Inhibition of retinal microglia may represent a promising approach for modulating inflammatory responses and/or promoting neuroprotection.
Discussion
Microglia have been in the spotlight since their discovery, but, despite receiving considerable and increasing attention over the years, many aspects of their biology and behavior remain unknown. From a developmental standpoint, it is interesting to notice how the main resident immune cell population arises from a different embryonic source from all other neural cells. This aspect imposes microglial progenitors to migrate extensively in order to colonize their neural areas of destination, while neurons, astrocytes and oligodendrocytes are generated in situ and are subject to more local migratory events. Provided that retinal microglia belong to the same population colonizing the rest of the CNS, an assumption that has not been corroborated by evidence yet, this feature translates into a delayed invasion of the retinal anlage compared, e.g., to the brain. However, the process still happens in a timely manner and precisely when synaptic connections are being developed within the retina. The latter correlation has been often pointed out, and although to date it has not been sufficiently explored, it could help to answer some questions regarding the temporal and spatial cues underlying this migration.
A second aspect worth highlighting is the complementary role adopted by macroglial cells in immune reaction. Particularly in the brain, part of the tasks seen for microglia are, in fact, mediated by astrocytes, with which microglia establish a tight and continuous cross-talk (329, 330). Per se, the fact that astrocytes already actively participate in the immune response program prompts the question of the evolutionary decision to rely on the non-neuroectoderm derived-microglia to cover and/or initiate most immune-related functions in the brain. Specifically for the retina, this point concerns mostly Müller cells, since astrocytes are only found at the interface of ganglion cells and are, therefore, physically separated from most retinal cells (65, 331). However, Müller cells and (cerebral) astrocytes are two biologically distinct cell types that only partially share functions, in turn suggesting that microglia in the retina might act differently or require different partners to accomplish the same tasks as microglia in the brain. Given the dissimilar cellular composition of the retina compared to the brain, one example being the exclusive presence of Müller glia in the former and of oligodendrocytes in the latter, and the segregation of retinal microglia in specific compartments (IPL and OPL) in contrast to the rather homogeneous distribution in the brain, it is conceivable that a regional distinction persists and concurs to define the identity of microglia subpopulations. Additionally, retina and brain environments differ in other physical properties, such as extracellular matrix composition and vasculature coverage (332–335). Together with the cellular composition, which is reflected by different cellular players (e.g., Müller cells vs. oligodendrocytes) and overall proportion of cellular subtypes (e.g., neurons vs. glia), differences in these aspects confer unique stiffness landscapes as well as specific metabolic environments in brain and retina, which could influence both microglia regional identity and their behavior in disease (336–338). With this in mind, the implicit assumption that has driven and continues to drive the field, namely that the same cell type performs the same tasks regardless of the CNS region, albeit being sensible, must necessarily find more experimental support. As pointed out in this review, a first publication ended up underlying differences in IPL versus OPL microglia (29). Nevertheless, it is not clear which additional cue defines these two spatially segregated populations and guides their positioning during early development. Similarly, the contribution of microglia to physiological events in the retina, such as circuitry refinement and tissue homeostasis, has found little evidence to date.
The role of microglia in pathology has instead received more attention. Though the core of the microglial response is in principle quite stereotyped, i.e., by secreting and/or reacting to specific cytokines (or the lack thereof), exploiting the complement system, displaying hypertrophy and process retraction, adopting a highly migratory and phagocytic phenotype to remove affected cells, several differences persist depending on the pathology and the strategy used to model it. Of note, abolishing microglia or interfering with its function does not necessarily result in an improvement of the degeneration. An explanation for this seemingly counterintuitive effect could reside in the versatility of microglia, which can adopt a detrimental or a pro-survival role in a context-dependent manner. An additional explanation could be the beneficial functions that microglia have in homeostasis and that are lost following its depletion. Cone photoreceptors, in particular, seem to benefit from the presence of microglia, as demonstrated by studies underlying the involvement of the latter ones in their maturation and maintenance and the development of structural abnormalities when microglia are depleted. These findings suggest a very specific connection between these two cell types, although the nature and underlying mechanisms of this relationship have not been extensively addressed.
Given the high degree of complexity and variety in microglial behavior, leading to both beneficial and detrimental effects in the degenerative context, therapeutic strategies could benefit from an approach in which microglia are modulated/repurposed rather than depleted. Indeed, not only would depletion harbor the risk to aggravate and accelerate the ongoing degeneration, as observed in several models of retinal dystrophy, but it would also not be easily achieved. Recent studies focusing on the ablation of the retinal microglia population have acknowledged a rapid repopulation, triggered by infiltration and proliferation of microglia having survived the treatment. Since monocytes/macrophages have been shown to be able to infiltrate the retina and become resident microglia cells (55), strategies focusing on microglia depletion might bear limited effects in those contexts where a pro-inflammatory function of microglia is observed. Conversely, treatments that would end up redirecting to a more neuroprotective phenotype, though of difficult application, could result in increased neuronal protection and would not deprive surrounding cells of important homeostatic mechanisms attributed to microglia.
Further studies are required to shed more light on the functions of microglia in physiology, specifically in the retina, and on the singularities of retinal tissue compared to the rest of the CNS. While microglia populations across the CNS share many common features, different contexts may directly or indirectly impose distinct characteristics and novel functions. In parallel, precious information can be inferred from studying the immune reaction in retinal disease and from phenotyping microglial behavior in response to different pathologies.
All in all, many gaps await to be filled, making the field undoubtedly exciting, but all the while urging the community to turn a well-earned attention to retinal microglia.
Author contributions
EM and SM contributed to the conception of the manuscript. EM wrote the manuscript. M-JG, MB and SM contributed to writing selected part of the manuscript. All authors contributed to manuscript revision, read, and approved the submitted version.
Funding
This work was supported by Deutsche Forschungsgemeinschaft (MI 1238/4-1 and SFB1309).
Acknowledgments
We thank Dr. Hanaa Ghanawi for insightful comments on the manuscript. Figures were created with BioRender.com.
Conflict of interest
The authors declare that the research was conducted in the absence of any commercial or financial relationships that could be construed as a potential conflict of interest.
Publisher’s note
All claims expressed in this article are solely those of the authors and do not necessarily represent those of their affiliated organizations, or those of the publisher, the editors and the reviewers. Any product that may be evaluated in this article, or claim that may be made by its manufacturer, is not guaranteed or endorsed by the publisher.
References
2. Kitamura T, Miyake T, Fujita S. Genesis of resting microglia in the gray matter of mouse hippocampus. J Comp Neurol (1984) 226(3):421–33. doi: 10.1002/cne.902260310
3. Ginhoux F, Lim S, Hoeffel G, Low D, Huber T. Origin and differentiation of microglia. Front Cell Neurosci (2013) 0(MAR):45.
4. Hao C, Richardson A, Fedoroff S. Macrophage-like cells originate from neuroepithelium in culture: Characterization and properties of the macrophage-like cells. Int J Dev Neurosci (1991) 9(1):1–14. doi: 10.1016/0736-5748(91)90067-V
5. Fedoroff S, Zhai R, Novak JP. Microglia and astroglia have a common progenitor cell. J Neurosci Res (1997) 50:477–86. doi: 10.1002/(SICI)1097-4547(19971101)50:3<477::AID-JNR14>3.0.CO;2-3
6. Perry VH, Hume DA, Gordon S. Immunohistochemical localization of macrophages and microglia in the adult and developing mouse brain. Neuroscience (1985) 15(2):313–26. doi: 10.1016/0306-4522(85)90215-5
7. Hume DA, Perry VH, Gordon S. Immunohistochemical localization of a macrophage-specific antigen in developing mouse retina: phagocytosis of dying neurons and differentiation of microglial cells to form a regular array in the plexiform layers. J Cell Biol (1983) 97(1):253–7. doi: 10.1083/jcb.97.1.253
8. Akiyama H, McGeer PL. Brain microglia constitutively express β-2 integrins. J Neuroimmunol (1990) 30(1):81–93. doi: 10.1016/0165-5728(90)90055-R
9. Beers DR, Henkel JS, Xiao Q, Zhao W, Wang J, Yen AA, et al. Wild-type microglia extend survival in PU.1 knockout mice with familial amyotrophic lateral sclerosis. Proc Natl Acad Sci (2006) 103(43):16021–6. doi: 10.1073/pnas.0607423103
10. McKercher SR, Torbett BE, Anderson KL, Henkel GW, Vestal DJ, Baribault H, et al. Targeted disruption of the PU.1 gene results in multiple hematopoietic abnormalities. EMBO J (1996) 15(20):5647–58. doi: 10.1002/j.1460-2075.1996.tb00949.x
11. Alliot F, Godin I, Pessac B. Microglia derive from progenitors, originating from the yolk sac, and which proliferate in the brain. Dev Brain Res (1999) 117(2):145–52. doi: 10.1016/S0165-3806(99)00113-3
12. Prinz M, Jung S, Priller J. Microglia biology: One century of evolving concepts. Cell (2019) 179(2):292–311. doi: 10.1016/j.cell.2019.08.053
13. Fan WJ, Li X, Yao HL, Deng JX, Liu HL, Cui ZJ, et al. Neural differentiation and synaptogenesis in retinal development. Neural Regener Res (2016) 11(2):312. doi: 10.4103/1673-5374.177743
14. Amini R, Rocha-Martins M, Norden C. Neuronal migration and lamination in the vertebrate retina. Front Neurosci (2018) 11:742. doi: 10.3389/fnins.2017.00742
15. Masland RH. The neuronal organization of the retina. Neuron (2012) 76(2):266–80. doi: 10.1016/j.neuron.2012.10.002
16. Masuda T, Sankowski R, Staszewski O, Prinz M. Microglia heterogeneity in the single-cell era. Cell Rep (2020) 30(5):1271–81. doi: 10.1016/j.celrep.2020.01.010
17. Tan YL, Yuan Y, Tian L. Microglial regional heterogeneity and its role in the brain. Mol Psychiatry (2020) 25(2):351–67. doi: 10.1038/s41380-019-0609-8
18. Li Q, Cheng Z, Zhou L, Darmanis S, Neff NF, Okamoto J, et al. Developmental heterogeneity of microglia and brain myeloid cells revealed by deep single-cell RNA sequencing. Neuron (2019) 101(2):207–223.e10. doi: 10.1016/j.neuron.2018.12.006
19. Hammond TR, Dufort C, Dissing-Olesen L, Giera S, Young A, Wysoker A, et al. Single-cell RNA sequencing of microglia throughout the mouse lifespan and in the injured brain reveals complex cell-state changes. Immunity (2019) 50(1):253–271.e6. doi: 10.1016/j.immuni.2018.11.004
20. Stratoulias V, Venero JL, Tremblay M, Joseph B. Microglial subtypes: diversity within the microglial community. EMBO J (2019) 38(17):e101997. doi: 10.15252/embj.2019101997
21. Macosko EZ, Basu A, Satija R, Nemesh J, Shekhar K, Goldman M, et al. Highly parallel genome-wide expression profiling of individual cells using nanoliter droplets. Cell (2015) 161(5):1202–14. doi: 10.1016/j.cell.2015.05.002
22. Li F, Jiang D, Samuel MA. Microglia in the developing retina. Neural Dev (2019) 14(1):12. doi: 10.1186/s13064-019-0137-x
23. Utz SG, See P, Mildenberger W, Thion MS, Silvin A, Lutz M, et al. Early fate defines microglia and non-parenchymal brain macrophage development. Cell (2020) 181(3):557–573.e18. doi: 10.1016/j.cell.2020.03.021
24. Kierdorf K, Erny D, Goldmann T, Sander V, Schulz C, Perdiguero EG, et al. Microglia emerge from erythromyeloid precursors via Pu.1- and Irf8-dependent pathways. Nat Neurosci (2013) 16(3):273–80. doi: 10.1038/nn.3318
25. Hagemeyer N, Kierdorf K, Frenzel K, Xue J, Ringelhan M, Abdullah Z, et al. Transcriptome-based profiling of yolk sac-derived macrophages reveals a role for Irf8 in macrophage maturation. EMBO J (2016) 35(16):1730–44. doi: 10.15252/embj.201693801
26. Gomez Perdiguero E, Klapproth K, Schulz C, Busch K, Azzoni E, Crozet L, et al. Tissue-resident macrophages originate from yolk-sac-derived erythro-myeloid progenitors. Nature (2014) 518(7540):547–51. doi: 10.1038/nature13989
27. Bennett FC, Bennett ML, Yaqoob F, Mulinyawe SB, Grant GA, Hayden Gephart M, et al. A combination of ontogeny and CNS environment establishes microglial identity. Neuron (2018) 98(6):1170–1183.e8. doi: 10.1016/j.neuron.2018.05.014
28. Yona S, Kim KW, Wolf Y, Mildner A, Varol D, Breker M, et al. Fate mapping reveals origins and dynamics of monocytes and tissue macrophages under homeostasis. Immunity (2013) 38(1):79–91. doi: 10.1016/j.immuni.2012.12.001
29. O’Koren EG, Yu C, Klingeborn M, Wong AYW, Prigge CL, Mathew R, et al. Microglial function is distinct in different anatomical locations during retinal homeostasis and degeneration. Immunity (2019) 50(3):723–737.e7. doi: 10.1016/j.immuni.2019.02.007
30. Wieghofer P, Hagemeyer N, Sankowski R, Schlecht A, Staszewski O, Amann L, et al. Mapping the origin and fate of myeloid cells in distinct compartments of the eye by single-cell profiling. EMBO J (2021) 40(6):e105123. doi: 10.15252/embj.2020105123
31. Hoeffel G, Chen J, Lavin Y, Low D, Almeida FF, See P, et al. C-myb+ erythro-myeloid progenitor-derived fetal monocytes give rise to adult tissue-resident macrophages. Immunity (2015) 42(4):665–78. doi: 10.1016/j.immuni.2015.03.011
32. Schulz C, Perdiguero EG, Chorro L, Szabo-Rogers H, Cagnard N, Kierdorf K, et al. A lineage of myeloid cells independent of myb and hematopoietic stem cells. Sci (1979) (2012) 336(6077):86–90. doi: 10.1126/science.1219179
33. Ginhoux F, Greter M, Leboeuf M, Nandi S, See P, Gokhan S, et al. Fate mapping analysis reveals that adult microglia derive from primitive macrophages. Sci (1979) (2010) 330(6005):841–5. doi: 10.1126/science.1194637
34. Dai XM, Ryan GR, Hapel AJ, Dominguez MG, Russell RG, Kapp S, et al. Targeted disruption of the mouse colony-stimulating factor 1 receptor gene results in osteopetrosis, mononuclear phagocyte deficiency, increased primitive progenitor cell frequencies, and reproductive defects. Blood (2002) 99(1):111–20. doi: 10.1182/blood.V99.1.111
35. Rojo R, Raper A, Ozdemir DD, Lefevre L, Grabert K, Wollscheid-Lengeling E, et al. Deletion of a Csf1r enhancer selectively impacts CSF1R expression and development of tissue macrophage populations. Nat Commun (2019) 10(1):1–17. doi: 10.1038/s41467-019-11053-8
36. Santos AM, Calvente R, Tassi M, Carrasco MC, Martín-Oliva D, Marín-Teva JL, et al. Embryonic and postnatal development of microglial cells in the mouse retina. J Comp Neurol (2008) 506(2):224–39. doi: 10.1002/cne.21538
37. Ashwell KWS, Holländer H, Streit W, Stone J. The appearance and distribution of microglia in the developing retina of the rat. Vis Neurosci (1989) 2(5):437–48. doi: 10.1017/S0952523800012335
38. Diaz-Araya CM, Provis JM, Penfold PL, Billson FA. Development of microglial topography in human retina. J Comp Neurol (1995) 363(1):53–68. doi: 10.1002/cne.903630106
39. Bejarano-Escobar R, Holguín-Arévalo MS, Montero JA, Francisco-Morcillo J, Martín-Partido G. Macrophage and microglia ontogeny in the mouse visual system can be traced by the expression of cathepsins b and d. Dev Dynamics (2011) 240(7):1841–55. doi: 10.1002/dvdy.22673
40. Fruttiger M. Development of the retinal vasculature. Angiogenesis (2007) 10(2):77–88. doi: 10.1007/s10456-007-9065-1
41. Anderson SR, Zhang J, Steele MR, Romero CO, Kautzman AG, Schafer DP, et al. Complement targets newborn retinal ganglion cells for phagocytic elimination by microglia. J Neurosci (2019) 39(11):2025–40. doi: 10.1523/JNEUROSCI.1854-18.2018
42. Chen L, Yang P, Kijlstra A. Distribution, markers, and functions of retinal microglia. Ocular Immunol Inflamm (2002) 10:27–39. doi: 10.1076/ocii.10.1.27.10328
43. Marín-Teva JL, Almendros A, Calvente R, Cuadros MA, Navascué J. Tangential migration of ameboid microglia in the developing quail retina: Mechanism of migration and migratory behavior. Glia (1998) 22:31–52. doi: 10.1002/(SICI)1098-1136(199801)22:1<31::AID-GLIA4>3.0.CO;2-B
44. Bodeutsch N, Thanos S. Migration of phagocytotic cells and development of the murine intraretinal microglial network: An in vivo study using fluorescent dyes. Glia (2000) 32(1):91–101. doi: 10.1002/1098-1136(200010)32:1<91::AID-GLIA90>3.0.CO;2-X
45. Bollmann L, Koser DE, Shahapure R, Gautier HOB, Holzapfel GA, Scarcelli G, et al. Microglia mechanics: Immune activation alters traction forces and durotaxis. Front Cell Neurosci (2015) 9(September):1–16. doi: 10.3389/fncel.2015.00363
46. Ambekar YS, Singh M, Scarcelli G, Rueda EM, Hall BM, Poché RA, et al. Characterization of retinal biomechanical properties using brillouin microscopy. J Biomed (2020) 25(9):090502. doi: 10.1117/1JBO259090502
47. Dudiki T, Meller J, Mahajan G, Liu H, Zhevlakova I, Stefl S, et al. Microglia control vascular architecture via a TGFβ1 dependent paracrine mechanism linked to tissue mechanics. Nat Commun (2020) 11(1):986. doi: 10.1038/s41467-020-14787-y
48. Endo Y, Asanuma D, Namiki S, Sugihara K, Hirose K, Uemura A, et al. Quantitative modeling of regular retinal microglia distribution. Sci Rep (2021) 11(1):1–13. doi: 10.1038/s41598-021-01820-3
49. Huang Y, Xu Z, Xiong S, Qin G, Sun F, Yang J, et al. Dual extra-retinal origins of microglia in the model of retinal microglia repopulation. Cell Discov (2018) 4(1):9. doi: 10.1038/s41421-018-0011-8
50. Zhang Y, Zhao L, Wang X, Ma W, Lazere A, Qian HH, et al. Repopulating retinal microglia restore endogenous organization and function under CX3CL1-CX3CR1 regulation. Sci Adv (2018) 4(3):eaap8492. doi: 10.1126/sciadv.aap8492
51. McPherson SW, Heuss ND, Lehmann U, Roehrich H, Abedin MD, Gregerson DS. The retinal environment induces microglia-like properties in recruited myeloid cells. J Neuroinflamm (2019) 16(1):1–19. doi: 10.1186/s12974-019-1546-9
52. Heuss ND, Pierson MJ, Roehrich H, McPherson SW, Gram AL, Li L, et al. Optic nerve as a source of activated retinal microglia post-injury. Acta Neuropathol Commun (2018) 6(1):66. doi: 10.1186/s40478-018-0571-8
53. Kezic J, McMenamin PG. Differential turnover rates of monocyte-derived cells in varied ocular tissue microenvironments. J Leukoc Biol (2008) 84(3):721–9. doi: 10.1189/jlb.0308166
54. O’Koren EG, Mathew R, Saban DR. Fate mapping reveals that microglia and recruited monocyte-derived macrophages are definitively distinguishable by phenotype in the retina. Sci Rep (2016) 6(1):20636. doi: 10.1038/srep20636
55. Ma W, Zhang Y, Gao C, Fariss RN, Tam J, Wong WT. Monocyte infiltration and proliferation reestablish myeloid cell homeostasis in the mouse retina following retinal pigment epithelial cell injury. Sci Rep (2017) 7(1):8433. doi: 10.1038/s41598-017-08702-7
56. Xu H, Chen M, Mayer EJ, Forrester JV, Dick AD. Turnover of resident retinal microglia in the normal adult mouse. Glia (2007) 55(11):1189–98. doi: 10.1002/glia.20535
57. Alt C, Runnels JM, Mortensen LJ, Zaher W, Lin CP. In vivo imaging of microglia turnover in the mouse retina after ionizing radiation and dexamethasone treatment. Invest Ophthalmol Vis Sci (2014) 55(8):5314–9. doi: 10.1167/iovs.14-14254
58. Nimmerjahn A. Resting microglial cells are highly dynamic surveillants of brain parenchyma in vivo. Sci (1979) (2005) 308(5726):1314–8. doi: 10.1126/science.1110647
59. Fontainhas AM, Wang M, Liang KJ, Chen S, Mettu P, Damani M, et al. Microglial morphology and dynamic behavior is regulated by ionotropic glutamatergic and GABAergic neurotransmission. PloS One (2011) 6(1):e15973. doi: 10.1371/journal.pone.0015973
60. Lee JE, Liang KJ, Fariss RN, Wong WT. Ex vivo dynamic imaging of retinal microglia using time-lapse confocal microscopy. Invest Ophthalmol Vis Sci (2008) 49(9):4169–76. doi: 10.1167/iovs.08-2076
61. Joseph A, Power D, Schallek J, Schallek J, Schallek J, Schallek J. Imaging the dynamics of individual processes of microglia in the living retina in vivo. Biomed Optics Express (2021) 12(10):6157–83. doi: 10.1364/BOE.426157
62. Miller EB, Zhang P, Ching K, Pugh EN, Burns ME. In vivo imaging reveals transient microglia recruitment and functional recovery of photoreceptor signaling after injury. Proc Natl Acad Sci (2019) 116(33):201903336. doi: 10.1073/pnas.1903336116
63. Wahl DJ, Sarunic MV, Ju MJ, Ng R, Jian Y. Sensorless adaptive optics multimodal en-face small animal retinal imaging. Biomed Optics Express (2019) 10(1):252–67. doi: 10.1364/BOE.10.000252
64. Paques M, Simonutti M, Augustin S, Goupille O, El Mathari B, Sahel JA. In vivo observation of the locomotion of microglial cells in the retina. Glia (2010) 58(14):1663–8. doi: 10.1002/glia.21037
65. Rashid K, Akhtar-Schaefer I, Langmann T. Microglia in retinal degeneration. Front Immunol (2019) 10:1975/full. doi: 10.3389/fimmu.2019.01975/full
66. Guo L, Choi S, Bikkannavar P, Cordeiro MF. Microglia: Key players in retinal ageing and neurodegeneration. Front Cell Neurosci (2022) 16:86. doi: 10.3389/fncel.2022.804782
67. Rathnasamy G, Foulds WS, Ling EA, Kaur C. Retinal microglia – a key player in healthy and diseased retina. Prog Neurobiol Pergamon (2019) 173:18–40. doi: 10.1016/j.pneurobio.2018.05.006
68. Haynes SE, Hollopeter G, Yang G, Kurpius D, Dailey ME, Gan WB, et al. The P2Y12 receptor regulates microglial activation by extracellular nucleotides. Nat Neurosci (2006) 9(12):1512–9. doi: 10.1038/nn1805
69. Liang KJ, Lee JE, Wang YD, Ma W, Fontainhas AM, Fariss RN, et al. Regulation of dynamic behavior of retinal microglia by CX3CR1 signaling. Invest Ophthalmol Vis Sci (2009) 50(9):4444–51. doi: 10.1167/iovs.08-3357
70. Broderick C, Hoek RM, Forrester JV, Liversidge J, Sedgwick JD, Dick AD. Constitutive retinal CD200 expression regulates resident microglia and activation state of inflammatory cells during experimental autoimmune uveoretinitis. Am J Pathol (2002) 161(5):1669–77. doi: 10.1016/S0002-9440(10)64444-6
71. Zhang M, Xu G, Liu W, Ni Y, Zhou W. Role of fractalkine/CX3CR1 interaction in light-induced photoreceptor degeneration through regulating retinal microglial activation and migration. PloS One (2012) 7(4):e35446. doi: 10.1371/journal.pone.0035446
72. Zabel MK, Zhao L, Zhang Y, Gonzalez SR, Ma W, Wang X, et al. Microglial phagocytosis and activation underlying photoreceptor degeneration is regulated by CX3CL1-CX3CR1 signaling in a mouse model of retinitis pigmentosa. Glia (2016) 64(9):1479–91. doi: 10.1002/glia.23016
73. Bernier LP, Bohlen CJ, York EM, Choi HB, Kamyabi A, Dissing-Olesen L, et al. Nanoscale surveillance of the brain by microglia via cAMP-regulated filopodia. Cell Rep (2019) 27(10):2895–2908.e4. doi: 10.1016/j.celrep.2019.05.010
74. Tam WY, Ma CHE. Bipolar/rod-shaped microglia are proliferating microglia with distinct M1/M2 phenotypes. Sci Rep (2014) 4(1):1–7. doi: 10.1038/srep07279
75. Au NPB, Ma CHE. Recent advances in the study of Bipolar/Rod-shaped microglia and their roles in neurodegeneration. Front Aging Neurosci (2017) 0(MAY):128. doi: 10.3389/fnagi.2017.00128
76. Taylor SE, Morganti-Kossmann C, Lifshitz J, Ziebell JM. Rod microglia: A morphological definition. PloS One (2014) 9(5):e97096. doi: 10.1371/journal.pone.0097096
77. Choi S, Guo L, Cordeiro MF. Retinal and brain microglia in multiple sclerosis and neurodegeneration. Cells (2021) 10(6):1507. doi: 10.3390/cells10061507
78. Torres-Platas SG, Comeau S, Rachalski A, Bo GD, Cruceanu C, Turecki G, et al. Morphometric characterization of microglial phenotypes in human cerebral cortex. J Neuroinflamm (2014) 11(1):1–14. doi: 10.1186/1742-2094-11-12
79. Grabert K, Michoel T, Karavolos MH, Clohisey S, Baillie JK, Stevens MP, et al. Microglial brain region–dependent diversity and selective regional sensitivities to aging. Nat Neurosci (2016) 19(3):504–16. doi: 10.1038/nn.4222
80. Masuda T, Sankowski R, Staszewski O, Böttcher C, Amann L, Sagar , et al. Spatial and temporal heterogeneity of mouse and human microglia at single-cell resolution. Nature (2019) 566(7744):388–92. doi: 10.1038/s41586-019-0924-x
81. Ronning KE, Karlen SJ, Miller EB, Burns ME. Molecular profiling of resident and infiltrating mononuclear phagocytes during rapid adult retinal degeneration using single-cell RNA sequencing. Sci Rep (2019) 9(1):4858. doi: 10.1038/s41598-019-41141-0
82. Karperien A, Ahammer H, Jelinek HF. Quantitating the subtleties of microglial morphology with fractal analysis. Front Cell Neurosci (2013) 7:1–34. doi: 10.3389/fncel.2013.00003
83. Zanier ER, Fumagalli S, Perego C, Pischiutta F, De Simoni MG. Shape descriptors of the “never resting” microglia in three different acute brain injury models in mice. Intensive Care Med Exp (2015) 3(1):1–18. doi: 10.1186/s40635-015-0039-0
84. Megjhani M, Rey-Villamizar N, Merouane A, Lu Y, Mukherjee A, Trett K, et al. Population-scale three-dimensional reconstruction and quantitative profiling of microglia arbors. Bioinformatics (2015) 31(13):2190–8. doi: 10.1093/bioinformatics/btv109
85. Hovens IB, Nyakas C, Schoemaker RG. A novel method for evaluating microglial activation using ionized calcium-binding adaptor protein-1 staining: cell body to cell size ratio. Neuroimmunol Neuroinflamm (2014) 1(2):82–8. doi: 10.4103/2347-8659.139719
86. Kuse Y, Ohuchi K, Nakamura S, Hara H, Shimazawa M. Microglia increases the proliferation of retinal precursor cells during postnatal development. Mol Vis (2018) 24:536–45.
87. Xu H, Chen M, Manivannan A, Lois N, Forrester JV. Age-dependent accumulation of lipofuscin in perivascular and subretinal microglia in experimental mice. Aging Cell (2008) 7(1):58–68. doi: 10.1111/j.1474-9726.2007.00351.x
88. Checchin D, Sennlaub F, Levavasseur E, Leduc M, Chemtob S. Potential role of microglia in retinal blood vessel formation. Invest Opthalmol Visual Sci (2006) 47(8):3595. doi: 10.1167/iovs.05-1522
89. Mills SA, Jobling AI, Dixon MA, Bui BV, Vessey KA, Phipps JA, et al. Fractalkine-induced microglial vasoregulation occurs within the retina and is altered early in diabetic retinopathy. Proc Natl Acad Sci USA (2021) 118(51):e2112561118. doi: 10.1073/pnas.2112561118
90. Outtz HH, Tattersall IW, Kofler NM, Steinbach N, Kitajewski J. Notch1 controls macrophage recruitment and notch signaling is activated at sites of endothelial cell anastomosis during retinal angiogenesis in mice. Blood (2011) 118(12):3436–9. doi: 10.1182/blood-2010-12-327015
91. Foulquier S, Caolo V, Swennen G, Milanova I, Reinhold S, Recarti C, et al. The role of receptor MAS in microglia-driven retinal vascular development. Angiogenesis (2019) 22(4):481–9. doi: 10.1007/s10456-019-09671-3
92. Diez-Roux G, Lang RA. Macrophages induce apoptosis in normal cells in vivo. Development (1997) 124(18):3633–8. doi: 10.1242/dev.124.18.3633
93. Walshe TE, Saint-Geniez M, Maharaj ASR, Sekiyama E, Maldonado AE, D’Amore PA. TGF-β is required for vascular barrier function, endothelial survival and homeostasis of the adult microvasculature. PloS One (2009) 4(4):e5149. doi: 10.1371/journal.pone.0005149
94. Lobov IB, Rao S, Carroll TJ, Vallance JE, Ito M, Ondr JK, et al. WNT7b mediates macrophage-induced programmed cell death in patterning of the vasculature. Nature (2005) 437(7057):417–21. doi: 10.1038/nature03928
95. Fantin A, Vieira JM, Gestri G, Denti L, Schwarz Q, Prykhozhij S, et al. Tissue macrophages act as cellular chaperones for vascular anastomosis downstream of VEGF-mediated endothelial tip cell induction. Blood (2010) 116(5):829–40. doi: 10.1182/blood-2009-12-257832
96. Kubota Y, Takubo K, Shimizu T, Ohno H, Kishi K, Shibuya M, et al. M-CSF inhibition selectively targets pathological angiogenesis and lymphangiogenesis. J Exp Med (2009) 206(5):1089–102. doi: 10.1084/jem.20081605
97. Rao S, Lobov IB, Vallance JE, Tsujikawa K, Shiojima I, Akunuru S, et al. Obligatory participation of macrophages in an angiopoietin 2-mediated cell death switch. Development (2007) 134(24):4449–58. doi: 10.1242/dev.012187
98. Stefater JA III, Lewkowich I, Rao S, Mariggi G, Carpenter AC, Burr AR, et al. Regulation of angiogenesis by a non-canonical wnt–Flt1 pathway in myeloid cells. Nature (2011) 474(7352):511–5. doi: 10.1038/nature10085
99. West H, Richardson WD, Fruttiger M. Stabilization of the retinal vascular network by reciprocal feedback between blood vessels and astrocytes. Development (2005) 132(8):1855–62. doi: 10.1242/dev.01732
100. O’Sullivan ML, Puñal VM, Kerstein PC, Brzezinski JA, Glaser T, Wright KM, et al. Astrocytes follow ganglion cell axons to establish an angiogenic template during retinal development. Glia (2017) 65(10):1697–716. doi: 10.1002/glia.23189
101. Puñal VM, Paisley CE, Brecha FS, Lee MA, Perelli RM, Wang J, et al. Large-Scale death of retinal astrocytes during normal development is non-apoptotic and implemented by microglia. PloS Biol (2019) 17(10):e3000492. doi: 10.1371/journal.pbio.3000492
102. Alves CH, Fernandes R, Santiago AR, Ambrósio AF. Microglia contribution to the regulation of the retinal and choroidal vasculature in age-related macular degeneration. Cells Multidiscip Digital Publishing Institute (2020) 9:1217. doi: 10.3390/cells9051217
103. Chen S, Tisch N, Kegel M, Yerbes R, Hermann R, Hudalla H, et al. CNS macrophages control neurovascular development via CD95L. Cell Rep (2017) 19(7):1378–93. doi: 10.1016/j.celrep.2017.04.056
104. Penfold PL, Provis JM, Madigan MC, van Driel D, Billson FA. Angiogenesis in normal human retinal development the involvement of astrocytes and macrophages. Graefe’s Arch Clin Exp Ophthalmol (1990) 228(3):255–63. doi: 10.1007/BF00920031
105. Furukawa T, Ueno A, Omori Y. Molecular mechanisms underlying selective synapse formation of vertebrate retinal photoreceptor cells. Cell Mol Life Sci (2019) 77(7):1251–66. doi: 10.1007/s00018-019-03324-w
106. Tian N. Visual experience and maturation of retinal synaptic pathways. in: Vision research. Pergamon; (2004) 44:3307–16. doi: 10.1016/j.visres.2004.07.041
107. Burger CA, Jiang D, Li F, Samuel MA. C1q regulates horizontal cell neurite confinement in the outer retina. Front Neural Circuits (2020) 0:64. doi: 10.3389/fncir.2020.583391
108. Schafer DP, Lehrman EK, Kautzman AG, Koyama R, Mardinly AR, Yamasaki R, et al. Microglia sculpt postnatal neural circuits in an activity and complement-dependent manner. Neuron (2012) 74(4):691–705. doi: 10.1016/j.neuron.2012.03.026
109. Kalish BT, Cheadle L, Hrvatin S, Nagy MA, Rivera S, Crow M, et al. Single-cell transcriptomics of the developing lateral geniculate nucleus reveals insights into circuit assembly and refinement. Proc Natl Acad Sci (2018) 115(5):E1051–60. doi: 10.1073/pnas.1717871115
110. Mizutani M, Pino PA, Saederup N, Charo IF, Ransohoff RM, Cardona AE. The fractalkine receptor but not CCR2 is present on microglia from embryonic development throughout adulthood. J Immunol (2012) 188(1):29–36. doi: 10.4049/jimmunol.1100421
111. Jobling AI, Waugh M, Vessey KA, Phipps JA, Trogrlic L, Greferath U, et al. The role of the microglial Cx3cr1 pathway in the postnatal maturation of retinal photoreceptors. J Neurosci (2018) 38(20):4708–23. doi: 10.1523/JNEUROSCI.2368-17.2018
112. Wang X, Zhao L, Zhang J, Fariss RN, Ma W, Kretschmer F, et al. Requirement for microglia for the maintenance of synaptic function and integrity in the mature retina. J Neurosci (2016) 36(9):2827–42. doi: 10.1523/JNEUROSCI.3575-15.2016
113. Du X, Penalva R, Little K, Kissenpfennig A, Chen M, Xu H. Deletion of Socs3 in LysM+ cells and Cx3cr1 resulted in age-dependent development of retinal microgliopathy. Mol Neurodegener (2021) 16(1):1–17. doi: 10.1186/s13024-021-00432-9
114. Rodríguez-Gallardo L, Lineros-Domínguez M del C, Francisco-Morcillo J, Martín-Partido G. Macrophages during retina and optic nerve development in the mouse embryo: Relationship to cell death and optic fibres. Anat Embryol (Berl) (2005) 210(4):303–16. doi: 10.1007/s00429-005-0051-3
115. Takahashi T, Goto T, Miyama S, Nowakowski RS, Caviness VS. Sequence of neuron origin and neocortical laminar fate: Relation to cell cycle of origin in the developing murine cerebral wall. J Neurosci (1999) 19(23):10357–71. doi: 10.1523/JNEUROSCI.19-23-10357.1999
116. Yamaguchi Y, Miura M. Programmed cell death in neurodevelopment. Dev Cell (2015) 32(4):478–90. doi: 10.1016/j.devcel.2015.01.019
117. Valenciano AI, Boya P, de la Rosa EJ. Early neural cell death: numbers and cues from the developing neuroretina. Int J Dev Biol (2009) 53(8-9–10):1515–28. doi: 10.1387/ijdb.072446av
118. Péquignot MO, Provost AC, Sallé S, Taupin P, Sainton KM, Marchant D, et al. Major role of BAX in apoptosis during retinal development and in establishment of a functional postnatal retina. Dev Dynamics (2003) 228(2):231–8. doi: 10.1002/dvdy.10376
119. Francisco-Morcillo J, Bejarano-Escobar R, Rodríguez-León J, Navascués J, Martín-Partido G. Ontogenetic cell death and phagocytosis in the visual system of vertebrates. Dev Dynamics (2014) 243(10):1203–25. doi: 10.1002/dvdy.24174
120. Young RW. Cell death during differentiation of the retina in the mouse. J Comp Neurol (1984) 229(3):362–73. doi: 10.1002/cne.902290307
121. Bähr M. Live or let die - retinal ganglion cell death and survival during development and in the lesioned adult CNS. Trends Neurosci (2000) 23:483–90. doi: 10.1016/S0166-2236(00)01637-4
122. Farah MH, Easter SS. Cell birth and death in the mouse retinal ganglion cell layer. J Comp Neurol (2005) 489(1):120–34. doi: 10.1002/cne.20615
123. Nguyen-ba-charvet KT, Rebsam A. Neurogenesis and specification of retinal ganglion cells. Int J Mol Sci (2020) 21:451. doi: 10.3390/ijms21020451
124. Galli-Resta L, Ensini M. An intrinsic time limit between genesis and death of individual neurons in the developing retinal ganglion cell layer. J Neurosci (1996) 16(7):2318–24. doi: 10.1523/JNEUROSCI.16-07-02318.1996
125. Frade JM, Barde YA. Genetic evidence for cell death mediated by nerve growth factor and the neurotrophin receptor p75 in the developing mouse retina and spinal cord. Development (1999) 126(4):683–90. doi: 10.1242/dev.126.4.683
126. Guimarães CA, Benchimol M, Amarante-Mendes GP, Linden R. Alternative programs of cell death in developing retinal tissue. J Biol Chem (2003) 278(43):41938–46. doi: 10.1074/jbc.M306547200
127. Trachsel-Moncho L, Benlloch-Navarro S, Fernández-Carbonell Á, Ramírez-Lamelas DT, Olivar T, Silvestre D, et al. Oxidative stress and autophagy-related changes during retinal degeneration and development. Cell Death Dis (2018) 9(8):812. doi: 10.1038/s41419-018-0855-8
128. Braunger BM, Pielmeier S, Demmer C, Landstorfer V, Kawall D, Abramov N, et al. TGF-β signaling protects retinal neurons from programmed cell death during the development of the mammalian eye. J Neurosci (2013) 33(35):14246–58. doi: 10.1523/JNEUROSCI.0991-13.2013
129. Walshe TE, Leach LL, D’Amore PA. TGF-β signaling is required for maintenance of retinal ganglion cell differentiation and survival. Neuroscience (2011) 189:123–31. doi: 10.1016/j.neuroscience.2011.05.020
130. Cusato K, Bosco A, Rozental R, Guimarães CA, Reese BE, Linden R, et al. Gap junctions mediate bystander cell death in developing retina. J Neurosci (2003) 23(16):6413–22. doi: 10.1523/JNEUROSCI.23-16-06413.2003
131. Brown GC, Neher JJ. Microglial phagocytosis of live neurons. Nat Rev Neurosci (2014) 15(4):209–16. doi: 10.1038/nrn3710
132. Sirerol-Piquer MS, Belenguer G, Morante-Redolat JM, Duart-Abadia P, Perez-Villalba A, Fariñas I. Physiological interactions between microglia and neural stem cells in the adult subependymal niche. Neuroscience (2019) 405:77–91. doi: 10.1016/j.neuroscience.2019.01.009
133. Rosin JM, Marsters CM, Malik F, Far R, Adnani L, Schuurmans C, et al. Embryonic microglia interact with hypothalamic radial glia during development and upregulate the TAM receptors MERTK and AXL following an insult. Cell Rep (2021) 34(1):108587. doi: 10.1016/j.celrep.2020.108587
134. Ferrer-Martín RM, Martín-Oliva D, Sierra-Martín A, Carrasco MC, Martín-Estebané M, Calvente R, et al. Microglial activation promotes cell survival in organotypic cultures of postnatal mouse retinal explants. block ML, editor. PloS One (2015) 10(8):e0135238. doi: 10.1371/journal.pone.0135238
135. Dick AD, Broderick C, Forrester JV, Wright GJ. Distribution of OX2 antigen and OX2 receptor within retina. Invest Ophthalmol Vis Sci (2001) 42(1):170–6.
136. Orihuela R, McPherson CA, Harry GJ. Microglial M1/M2 polarization and metabolic states. Br J Pharmacol (2016) 173:649–65. doi: 10.1111/bph.13139
137. Zhou T, Huang Z, Sun X, Zhu X, Zhou L, Li M, et al. Microglia polarization with M1/M2 phenotype changes in rd1 mouse model of retinal degeneration. Front Neuroanat (2017) 11:77. doi: 10.3389/fnana.2017.00077
138. Holtman IR, Skola D, Glass CK. Transcriptional control of microglia phenotypes in health and disease. J Clin Invest (2017) 127(9):3220–9. doi: 10.1172/JCI90604
139. Morrison H, Young K, Qureshi M, Rowe RK, Lifshitz J. Quantitative microglia analyses reveal diverse morphologic responses in the rat cortex after diffuse brain injury. Sci Rep (2017) 7(1):1–12. doi: 10.1038/s41598-017-13581-z
140. Guo M, Schwartz TD, Dunaief JL, Cui QN. Myeloid cells in retinal and brain degeneration. FEBS J (2022) 289:2337–61. doi: 10.1111/febs.16177
141. Sousa C, Golebiewska A, Poovathingal SK, Kaoma T, Pires-Afonso Y, Martina S, et al. Single-cell transcriptomics reveals distinct inflammation-induced microglia signatures. EMBO Rep (2018) 19(11):e46171. doi: 10.15252/embr.201846171
142. Keren-Shaul H, Spinrad A, Weiner A, Matcovitch-Natan O, Dvir-Szternfeld R, Ulland TK, et al. A unique microglia type associated with restricting development of alzheimer’s disease. Cell (2017) 169(7):1276–90.e17. doi: 10.1016/j.cell.2017.05.018
143. Rosmus DD, Wieghofer P. Guardians of the eye: new tales about retinal microglia and other ocular macrophages. Neural Regener Res (2022) 17(6):1275–7. doi: 10.4103/1673-5374.327335
144. Ozaki E, Delaney C, Campbell M, Doyle SL. Minocycline suppresses disease-associated microglia (DAM) in a model of photoreceptor cell degeneration. Exp Eye Res (2022) 217:108953. doi: 10.1016/j.exer.2022.108953
145. Anderson SR, Roberts JM, Zhang J, Steele MR, Romero CO, Bosco A, et al. Developmental apoptosis promotes a disease-related gene signature and independence from CSF1R signaling in retinal microglia. Cell Rep (2019) 27(7):2002–2013.e5. doi: 10.1016/j.celrep.2019.04.062
146. Anderson SR, Roberts JM, Ghena N, Irvin EA, Schwakopf J, Cooperstein IB, et al. Neuronal apoptosis drives remodeling states of microglia and shifts in survival pathway dependence. Elife (2022) 11. doi: 10.7554/eLife.76564
147. Tang PH, Pierson MJ, Heuss ND, Gregerson DS. A subpopulation of activated retinal macrophages selectively migrated to regions of cone photoreceptor stress, but had limited effect on cone death in a mouse model for type 2 leber congenital amaurosis. Mol Cell Neurosci (2017) 85:70–81. doi: 10.1016/j.mcn.2017.09.002
148. Bisht K, Sharma KP, Lecours C, Gabriela Sánchez M, Hajj H, Milior G, et al. Dark microglia: A new phenotype predominantly associated with pathological states. Glia (2016) 64(5):826–39. doi: 10.1002/glia.22966
149. Dando SJ, Naranjo Golborne C, Chinnery HR, Ruitenberg MJ, McMenamin PG. A case of mistaken identity: CD11c-eYFP+ cells in the normal mouse brain parenchyma and neural retina display the phenotype of microglia, not dendritic cells. Glia (2016) 64(8):1331–49. doi: 10.1002/glia.23005
151. Hartong DT, Berson EL, Dryja TP. Retinitis pigmentosa. Lancet (2006) 368:1795–809. doi: 10.1016/S0140-6736(06)69740-7
152. Wang AL, Knight DK, Vu TTT, Mehta MC. Retinitis pigmentosa: Review of current treatment. Int Ophthalmol Clin (2019) 59(1):263–80. doi: 10.1097/IIO.0000000000000256
153. Liu W, Liu S, Li P, Yao K. Retinitis pigmentosa: Progress in molecular pathology and biotherapeutical strategies. Int J Mol Sci (2022) 23(9):4883. doi: 10.3390/ijms23094883
154. Sallum JMF, Kaur VP, Shaikh J, Banhazi J, Spera C, Aouadj C, et al. Epidemiology of mutations in the 65-kDa retinal pigment epithelium (RPE65) gene-mediated inherited retinal dystrophies: A systematic literature review. Adv Ther (2022) 39(3):1179–98. doi: 10.1007/s12325-021-02036-7
155. Murakami Y, Nakabeppu Y, Sonoda KH. Oxidative stress and microglial response in retinitis pigmentosa. Int J Mol Sci (2020) 21(19):7170. doi: 10.3390/ijms21197170
156. Du J, Rountree A, Cleghorn WM, Contreras L, Lindsay KJ, Sadilek M, et al. Phototransduction influences metabolic flux and nucleotide metabolism in mouse retina. J Biol Chem (2016) 291(9):4698–710. doi: 10.1074/jbc.M115.698985
157. Weiss ER, Osawa S, Xiong Y, Dhungana S, Carlson J, McRitchie S, et al. Broad spectrum metabolomics for detection of abnormal metabolic pathways in a mouse model for retinitis pigmentosa. Exp Eye Res (2019) 184:135–45. doi: 10.1016/j.exer.2019.03.007
158. Murenu E, Kostidis S, Lahiri S, Geserich AS, Imhof A, Giera M, et al. Metabolic analysis of vitreous/lens and retina in wild type and retinal degeneration mice. Int J Mol Sci (2021) 22(5):1–22. doi: 10.3390/ijms22052345
159. Jiang K, Mondal AK, Adlakha YK, Gumerson J, Aponte A, Gieser L, et al. Multi-omics analyses reveal early metabolic imbalance and mitochondrial stress in neonatal photoreceptors leading to cell death in Pde6brd1/rd1 mouse model of retinal degeneration. Hum Mol Genet (2022) 31:2137–54. doi: 10.1093/hmg/ddac013
160. Venkatesh A, Ma S, Le YZ, Hall MN, Rüegg MA, Punzo C. Activated mTORC1 promotes long-term cone survival in retinitis pigmentosa mice. J Clin Invest (2015) 125(4):1446–58. doi: 10.1172/JCI79766
161. Zeiss CJ, Johnson EA. Proliferation of microglia, but not photoreceptors, in the outer nuclear layer of the rd-1 mouse. Invest Ophthalmol Vis Sci (2004) 45(3):971–6. doi: 10.1167/iovs.03-0301
162. Peng B, Xiao J, Wang K, So KF, Tipoe GL, Lin B. Suppression of microglial activation is neuroprotective in a mouse model of human retinitis pigmentosa. J Neurosci (2014) 34(24):8139–50. doi: 10.1523/JNEUROSCI.5200-13.2014
163. Roche SL, Wyse-Jackson AC, Byrne AM, Ruiz-Lopez AM, Cotter TG. Alterations to retinal architecture prior to photoreceptor loss in a mouse model of retinitis pigmentosa. Int J Dev Biol (2016) 60(4-5–6):127–39. doi: 10.1387/ijdb.150400tc
164. Blank T, Goldmann T, Koch M, Amann L, Schön C, Bonin M, et al. Early microglia activation precedes photoreceptor degeneration in a mouse model of CNGB1-linked retinitis pigmentosa. Front Immunol (2018) 8:1930. doi: 10.3389/fimmu.2017.01930
165. Keeler CE. The inheritance of a retinal abnormality in white mice. Proc Natl Acad Sci (1924) 10(7):329–33. doi: 10.1073/pnas.10.7.329
166. Chang B, Hawes NL, Hurd RE, Davisson MT, Nusinowitz S, Heckenlively JR. Retinal degeneration mutants in the mouse. Vision Res (2002) 42(4):517–25. doi: 10.1016/S0042-6989(01)00146-8
167. Wang SK, Xue Y, Rana P, Hong CM, Cepko CL. Soluble CX3CL1 gene therapy improves cone survival and function in mouse models of retinitis pigmentosa. Proc Natl Acad Sci USA (2019) 116(20):10140–9. doi: 10.1073/pnas.1901787116
168. Roche SL, Wyse-Jackson AC, Ruiz-Lopez AM, Byrne AM, Cotter TG. Fractalkine-CX3CR1 signaling is critical for progesterone-mediated neuroprotection in the retina. Sci Rep (2017) 7:43067. doi: 10.1038/srep43067
169. Arroba AI, Álvarez-Lindo N, van Rooijen N, de la Rosa EJ. Microglia-mediated IGF-I neuroprotection in the rd10 mouse model of retinitis pigmentosa. Invest Ophthalmol Vis Sci (2011) 52(12):9124–30. doi: 10.1167/iovs.11-7736
170. Wang SK, Xue Y, Cepko CL. Microglia modulation by TGF-β1 protects cones in mouse models of retinal degeneration. J Clin Invest (2020) 130(8):4360–9. doi: 10.1172/JCI136160
171. Zhao L, Zabel MK, Wang X, Ma W, Shah P, Fariss RN, et al. Microglial phagocytosis of living photoreceptors contributes to inherited retinal degeneration. EMBO Mol Med (2015) 7(9):1179–97. doi: 10.15252/emmm.201505298
172. He J, Zhao C, Dai J, Weng CH, Bian BSJ, Gong Y, et al. Microglia mediate synaptic material clearance at the early stage of rats with retinitis pigmentosa. Front Immunol (2019) 10(APR):912. doi: 10.3389/fimmu.2019.00912
173. Silverman SM, Ma W, Wang X, Zhao L, Wong WT. C3- and CR3-dependent microglial clearance protects photoreceptors in retinitis pigmentosa. J Exp Med (2019) 216(8):1925–43. doi: 10.1084/jem.20190009
174. Funatsu J, Murakami Y, Shimokawa S, Nakatake S, Fujiwara K, Okita A, et al. Circulating inflammatory monocytes oppose microglia and contribute to cone cell death in retinitis pigmentosa Vol. 1. Nelson KE, editor. PNAS Nexus (2022) 1(1):pgac003. doi: 10.1093/pnasnexus/pgac003
175. He J, Fu Y, Ge L, Dai J, Fang Y, Li Y, et al. Disease-associated microglial activation prevents photoreceptor degeneration by suppressing the accumulation of cell debris and neutrophils in degenerating rat retinas. Theranostics (2022) 12(6):2687–706. doi: 10.7150/thno.67954
176. Okunuki Y, Mukai R, Pearsall EA, Klokman G, Husain D, Park DH, et al. Microglia inhibit photoreceptor cell death and regulate immune cell infiltration in response to retinal detachment. Proc Natl Acad Sci USA (2018) 115(27):E6264–73. doi: 10.1073/pnas.1719601115
177. Todd L, Palazzo I, Suarez L, Liu X, Volkov L, Hoang TV, et al. Reactive microglia and IL1β/IL-1R1-signaling mediate neuroprotection in excitotoxin-damaged mouse retina. J Neuroinflamm (2019) 16(1):118. doi: 10.1186/s12974-019-1505-5
178. Delyfer MN, Forster V, Neveux N, Picaud S, Léveillard T, Sahel JA. Evidence for glutamate-mediated excitotoxic mechanisms during photoreceptor degeneration in the rd1 mouse retina. Mol Vis (2005) 11:688–96.
179. Bourne RRA, Steinmetz JD, Saylan M, Mersha AM, Weldemariam AH, Wondmeneh TG, et al. Causes of blindness and vision impairment in 2020 and trends over 30 years, and prevalence of avoidable blindness in relation to VISION 2020: The right to sight: An analysis for the global burden of disease study. Lancet Glob Health (2021) 9(2):e144–60. doi: 10.1016/S2214-109X(20)30489-7
181. Jonas JB, Aung T, Bourne RR, Bron AM, Ritch R, Panda-Jonas S. Glaucoma. Lancet (2017) 390(10108):2183–93. doi: 10.1016/S0140-6736(17)31469-1
182. Storgaard L, Tran TL, Freiberg JC, Hauser AS, Kolko M. Glaucoma clinical research: Trends in treatment strategies and drug development. Front Med (2021) 8:1492. doi: 10.3389/fmed.2021.733080
183. Howell GR, Macalinao DG, Sousa GL, Walden M, Soto I, Kneeland SC, et al. Molecular clustering identifies complement and endothelin induction as early events in a mouse model of glaucoma. J Clin Invest (2011) 121(4):1429–44. doi: 10.1172/JCI44646
184. Qu J, Jakobs TC. The time course of gene expression during reactive gliosis in the optic nerve. PloS One (2013) 8(6):e67094. doi: 10.1371/journal.pone.0067094
185. Tezel G. Molecular regulation of neuroinflammation in glaucoma: Current knowledge and the ongoing search for new treatment targets. Prog Retin Eye Res (2022) 87:100998. doi: 10.1016/j.preteyeres.2021.100998
186. Tezel G, Chauhan BC, LeBlanc RP, Wax MB. Immunohistochemical assessment of the glial mitogen-activated protein kinase activation in glaucoma. Invest Ophthalmol Vis Sci (2003) 44(7):3025–33. doi: 10.1167/iovs.02-1136
187. Howell GR, Soto I, Zhu X, Ryan M, Macalinao DG, Sousa GL, et al. Radiation treatment inhibits monocyte entry into the optic nerve head and prevents neuronal damage in a mouse model of glaucoma. J Clin Invest (2012) 122(4):1246–61. doi: 10.1172/JCI61135
188. Anderson MG, Libby RT, Gould DB, Smith RS, John SWM. High-dose radiation with bone marrow transfer prevents neurodegeneration in an inherited glaucoma. Proc Natl Acad Sci USA (2005) 102(12):4566–71. doi: 10.1073/pnas.0407357102
189. Guo Y, Cepurna WO, Dyck JA, Doser TA, Johnson EC, Morrison JC. Retinal cell responses to elevated intraocular pressure: A gene array comparison between the whole retina and retinal ganglion cell layer. Invest Ophthalmol Vis Sci (2010) 51(6):3003–18. doi: 10.1167/iovs.09-4663
190. Guo Y, Johnson EC, Cepurna WO, Dyck JA, Doser T, Morrison JC. Early gene expression changes in the retinal ganglion cell layer of a rat glaucoma model. Invest Ophthalmol Vis Sci (2011) 52(3):1460–73. doi: 10.1167/iovs.10-5930
191. Wang X, Tay SSW, Ng YK. An immunohistochemical study of neuronal and glial cell reactions in retinae of rats with experimental glaucoma. Exp Brain Res (2000) 132(4):476–84. doi: 10.1007/s002210000360
192. Hubens WHG, Beckers HJM, Gorgels TGMF, Webers CAB. Increased ratios of complement factors C3a to C3 in aqueous humor and serum mark glaucoma progression. Exp Eye Res (2021) 204:108460. doi: 10.1016/j.exer.2021.108460
193. Reinehr S, Gomes SC, Gassel CJ, Asaad MA, Stute G, Schargus M, et al. Intravitreal therapy against the complement factor C5 prevents retinal degeneration in an experimental autoimmune glaucoma model. Front Pharmacol (2019) 10:1381. doi: 10.3389/fphar.2019.01381
194. Bosco A, Anderson SR, Breen KT, Romero CO, Steele MR, Chiodo VA, et al. Complement C3-targeted gene therapy restricts onset and progression of neurodegeneration in chronic mouse glaucoma. Mol Ther (2018) 26(10):2379–96. doi: 10.1016/j.ymthe.2018.08.017
195. Kumari R, Astafurov K, Genis A, Danias J. Differential effects of C1qa ablation on glaucomatous damage in two sexes in DBA/2NNia mice. PloS One (2015) 10(11):e0142199. doi: 10.1371/journal.pone.0142199
196. Harder JM, Braine CE, Williams PA, Zhu X, MacNicoll KH, Sousa GL, et al. Early immune responses are independent of RGC dysfunction in glaucoma with complement component C3 being protective. Proc Natl Acad Sci USA (2017) 114(19):E3839–48. doi: 10.1073/pnas.1608769114
197. Howell GR, MacNicoll KH, Braine CE, Soto I, Macalinao DG, Sousa GL, et al. Combinatorial targeting of early pathways profoundly inhibits neurodegeneration in a mouse model of glaucoma. Neurobiol Dis (2014) 71:44–52. doi: 10.1016/j.nbd.2014.07.016
198. Tezel G, Yang X, Luo C, Kain AD, Powell DW, Kuehn MH, et al. Oxidative stress and the regulation of complement activation in human glaucoma. Invest Ophthalmol Vis Sci (2010) 51(10):5071–82. doi: 10.1167/iovs.10-5289
199. Kuehn MH, Kim CY, Ostojic J, Bellin M, Alward WLM, Stone EM, et al. Retinal synthesis and deposition of complement components induced by ocular hypertension. Exp Eye Res (2006) 83(3):620–8. doi: 10.1016/j.exer.2006.03.002
200. Yang X, Hondur G, Li M, Cai J, Klein JB, Kuehn MH, et al. Proteomics analysis of molecular risk factors in the ocular hypertensive human retina. Invest Ophthalmol Vis Sci (2015) 56(10):5816–30. doi: 10.1167/iovs.15-17294
201. Ahmed F, Brown KM, Stephan DA, Morrison JC, Johnson EC, Tomarev SI. Microarray analysis of changes in mRNA levels in the rat retina after experimental elevation of intraocular pressure. Invest Ophthalmol Vis Sci (2004) 45(4):1247–58. doi: 10.1167/iovs.03-1123
202. Miyahara T, Kikuchi T, Akimoto M, Kurokawa T, Shibuki H, Yoshimura N. Gene microarray analysis of experimental glaucomatous retina from cynomologous monkey. Invest Ophthalmol Vis Sci (2003) 44(10):4347–56. doi: 10.1167/iovs.02-1032
203. Johnson EC, Jia L, Cepurna WO, Doser TA, Morrison JC. Global changes in optic nerve head gene expression after exposure to elevated intraocular pressure in a rat glaucoma model. Invest Ophthalmol Vis Sci (2007) 48(7):3161–77. doi: 10.1167/iovs.06-1282
204. Panagis L, Zhao X, Ge Y, Ren L, Mittag TW, Danias J. Gene expression changes in areas of focal loss of retinal ganglion cells in the retina of DBA/2J mice. Invest Ophthalmol Vis Sci (2010) 51(4):2024–34. doi: 10.1167/iovs.09-3560
205. Doudevski I, Rostagno A, Cowman M, Liebmann J, Ritch R, Ghiso J. Clusterin and complement activation in exfoliation glaucoma. Invest Ophthalmol Vis Sci (2014) 55(4):2491–9. doi: 10.1167/iovs.13-12941
206. Ovodenko B, Rostagno A, Neubert TA, Shetty V, Thomas S, Yang A, et al. Proteomic analysis of exfoliation deposits. Invest Ophthalmol Vis Sci (2007) 48(4):1447–57. doi: 10.1167/iovs.06-0411
207. Stasi K, Nagel D, Yang X, Wang RF, Ren L, Podos SM, et al. Complement component 1Q (C1Q) upregulation in retina of murine, primate, and human glaucomatous eyes. Invest Ophthalmol Vis Sci (2006) 47(3):1024–9. doi: 10.1167/iovs.05-0830
208. Lam TT, Kwong JMK, Tso MOM. Early glial responses after acute elevated intraocular pressure in rats. Invest Ophthalmol Vis Sci (2003) 44(2):638–45. doi: 10.1167/iovs.02-0255
209. Williams PA, Braine CE, Kizhatil K, Foxworth NE, Tolman NG, Harder JM, et al. Inhibition of monocyte-like cell extravasation protects from neurodegeneration in DBA/2J glaucoma. Mol Neurodegeneration (2019) 14(1):1–23. doi: 10.1186/s13024-018-0303-3
210. Howell GR, Soto I, Ryan M, Graham LC, Smith RS, John SWM. Deficiency of complement component 5 ameliorates glaucoma in DBA/2J mice. J Neuroinflamm (2013) 10(1):1–8. doi: 10.1186/1742-2094-10-76
211. Williams PA, Tribble JR, Pepper KW, Cross SD, Morgan BP, Morgan JE, et al. Inhibition of the classical pathway of the complement cascade prevents early dendritic and synaptic degeneration in glaucoma. Mol Neurodegener (2016) 11(1):1–13. doi: 10.1186/s13024-016-0091-6
212. Neufeld AH. Microglia in the optic nerve head and the region of parapapillary chorioretinal atrophy in glaucoma. Arch Ophthalmol (1999) 117(8):1050–6. doi: 10.1001/archopht.117.8.1050
213. Yuan L, Neufeld AH. Activated microglia in the human glaucomatous optic nerve head. J Neurosci Res (2001) 64(5):523–32. doi: 10.1002/jnr.1104
214. Taylor S, Calder CJ, Albon J, Erichsen JT, Boulton ME, Morgan JE. Involvement of the CD200 receptor complex in microglia activation in experimental glaucoma. Exp Eye Res (2011) 92(5):338–43. doi: 10.1016/j.exer.2011.01.012
215. Bosco A, Steele MR, Vetter ML. Early microglia activation in a mouse model of chronic glaucoma. J Comp Neurol (2011) 519(4):599–620. doi: 10.1002/cne.22516
216. Thanos S, Pavlidis C, Mey J, Thiel HJ. Specific transcellular staining of microglia in the adult rat after traumatic degeneration of carbocyanine-filled retinal ganglion cells. Exp Eye Res (1992) 55(1):101–17. doi: 10.1016/0014-4835(92)90098-D
217. Schuetz E, Thanos S. Neuro-glial interactions in the adult rat retina after reaxotomy of ganglion cells: examination of neuron survival and phagocytic microglia using fluorescent tracers. Brain Res Bull (2004) 62(5):391–6. doi: 10.1016/j.brainresbull.2003.10.008
218. Ramírez AI, de Hoz R, Fernández-Albarral JA, Salobrar-Garcia E, Rojas B, Valiente-Soriano FJ, et al. Time course of bilateral microglial activation in a mouse model of laser-induced glaucoma. Sci Rep (2020) 10(1):1–17. doi: 10.1038/s41598-020-61848-9
219. de Hoz R, Ramírez AI, González-Martín R, Ajoy D, Rojas B, Salobrar-Garcia E, et al. Bilateral early activation of retinal microglial cells in a mouse model of unilateral laser-induced experimental ocular hypertension. Exp Eye Res (2018) 171:12–29. doi: 10.1016/j.exer.2018.03.006
220. Rojas B, Gallego BI, Ramírez AI, Salazar JJ, de Hoz R, Valiente-Soriano FJ, et al. Microglia in mouse retina contralateral to experimental glaucoma exhibit multiple signs of activation in all retinal layers. J Neuroinflamm (2014) 11(1):1–24. doi: 10.1186/1742-2094-11-133
221. De Hoz R, Gallego BI, Ramírez AI, Rojas B, Salazar JJ, Valiente-Soriano FJ, et al. Rod-like microglia are restricted to eyes with laser-induced ocular hypertension but absent from the microglial changes in the contralateral untreated eye. PloS One (2013) 8(12):e83733. doi: 10.1371/journal.pone.0083733
222. Chen M, Hombrebueno JR, Luo C, Penalva R, Zhao J, Colhoun L, et al. Age- and light-dependent development of localised retinal atrophy in CCL2-/-CX3CR1GFP/GFP mice. PloS One (2013) 8(4):e61381. doi: 10.1371/journal.pone.0061381
223. Gallego BI, Salazar JJ, de Hoz R, Rojas B, Ramírez AI, Salinas-Navarro M, et al. IOP induces upregulation of GFAP and MHC-II and microglia reactivity in mice retina contralateral to experimental glaucoma. J Neuroinflamm (2012) 9(1):1–18. doi: 10.1186/1742-2094-9-92
224. Tribble JR, Harder JM, Williams PA, John SWM. Ocular hypertension suppresses homeostatic gene expression in optic nerve head microglia of DBA/2 J mice. Mol Brain (2020) 13(1):1–14. doi: 10.1186/s13041-020-00603-7
225. Frade JM, Mellado M, del Real G, Gutierrez-Ramos JC, Lind P, Martinez AC. Characterization of the CCR2 chemokine receptor: functional CCR2 receptor expression in b cells. J Immunol (1997) 159(11):5576–84. doi: 10.4049/jimmunol.159.11.5576
226. Komiya H, Takeuchi H, Ogawa Y, Hatooka Y, Takahashi K, Katsumoto A, et al. CCR2 is localized in microglia and neurons, as well as infiltrating monocytes, in the lumbar spinal cord of ALS mice. Mol Brain (2020) 13(1):1–4. doi: 10.1186/s13041-020-00607-3
227. Williams PA, Braine CE, Foxworth NE, Cochran KE, John SWM. GlyCAM1 negatively regulates monocyte entry into the optic nerve head and contributes to radiation-based protection in glaucoma. J Neuroinflamm (2017) 14(1):1–12. doi: 10.1186/s12974-017-0868-8
228. Chen X, Lei F, Zhou C, Chodosh J, Wang L, Huang Y, et al. Glaucoma after ocular surgery or trauma: The role of infiltrating monocytes and their response to cytokine inhibitors. Am J Pathol (2020) 190(10):2056–66. doi: 10.1016/j.ajpath.2020.07.006
229. Chiu K, Yeung SC, So KF, Chang RCC. Modulation of morphological changes of microglia and neuroprotection by monocyte chemoattractant protein-1 in experimental glaucoma. Cell Mol Immunol (2010) 7(1):61–8. doi: 10.1038/cmi.2009.110
230. Wong WL, Su X, Li X, Cheung CMG, Klein R, Cheng CY, et al. Global prevalence of age-related macular degeneration and disease burden projection for 2020 and 2040: A systematic review and meta-analysis. Lancet Glob Health (2014) 2(2):e106–16. doi: 10.1016/S2214-109X(13)70145-1
231. Deng Y, Qiao L, Du M, Qu C, Wan L, Li J, et al. Age-related macular degeneration: Epidemiology, genetics, pathophysiology, diagnosis, and targeted therapy. Genes Dis (2022) 9:62–79. doi: 10.1016/j.gendis.2021.02.009
232. Ambati J, Atkinson JP, Gelfand BD. Immunology of age-related macular degeneration. Nat Rev Immunol (2013) 13(6):438–51. doi: 10.1038/nri3459
233. Ferris FL, Wilkinson CP, Bird A, Chakravarthy U, Chew E, Csaky K, et al. Clinical classification of age-related macular degeneration. Ophthalmology (2013) 120(4):844–51. doi: 10.1016/j.ophtha.2012.10.036
234. Nozaki M, Raisler BJ, Sakurai E, Sarma JV, Barnum SR, Lambris JD, et al. Drusen complement components C3a and C5a promote choroidal neovascularization. Proc Natl Acad Sci (2006) 103(7):2328–33. doi: 10.1073/pnas.0408835103
235. Schick T, Steinhauer M, Aslanidis A, Altay L, Karlstetter M, Langmann T, et al. Local complement activation in aqueous humor in patients with age-related macular degeneration. Eye (2017) 31(5):810–3. doi: 10.1038/eye.2016.328
236. Johnson LV, Leitner WP, Staples MK, Anderson DH. Complement activation and inflammatory processes in drusen formation and age related macular degeneration. Exp Eye Res (2001) 73(6):887–96. doi: 10.1006/exer.2001.1094
237. Mullins RF, Russell SR, Anderson DH, Hageman GS. Drusen associated with aging and age-related macular degeneration contain proteins common to extracellular deposits associated with atherosclerosis, elastosis, amyloidosis, and dense deposit disease. FASEB J (2000) 14(7):835–46. doi: 10.1096/fasebj.14.7.835
238. Natoli R, Fernando N, Jiao H, Racic T, Madigan M, Barnett NL, et al. Retinal macrophages synthesize C3 and activate complement in AMD and in models of focal retinal degeneration. Invest Ophthalmol Vis Sci (2017) 58(7):2977–90. doi: 10.1167/iovs.17-21672
239. Ma W, Coon S, Zhao L, Fariss RN, Wong WT. A2E accumulation influences retinal microglial activation and complement regulation. Neurobiol Aging (2013) 34(3):943–60. doi: 10.1016/j.neurobiolaging.2012.06.010
240. Ambati J, Anand A, Fernandez S, Sakurai E, Lynn BC, Kuziel WA, et al. An animal model of age-related macular degeneration in senescent ccl-2- or ccr-2-deficient mice. Nat Med (2003) 9(11):1390–7. doi: 10.1038/nm950
241. Balser C, Wolf A, Herb M, Langmann T. Co-Inhibition of PGF and VEGF blocks their expression in mononuclear phagocytes and limits neovascularization and leakage in the murine retina. J Neuroinflamm (2019) 16(1):1–12. doi: 10.1186/s12974-019-1419-2
242. Tsutsumi C, Sonoda KH, Egashira K, Qiao H, Hisatomi T, Nakao S, et al. The critical role of ocular-infiltrating macrophages in the development of choroidal neovascularization. J Leukoc Biol (2003) 74(1):25–32. doi: 10.1189/jlb.0902436
243. Krause TA, Alex AF, Engel DR, Kurts C, Eter N. VEGF-production by CCR2-dependent macrophages contributes to laser-induced choroidal neovascularization. PloS One (2014) 9(4):e94313. doi: 10.1371/journal.pone.0094313
244. Tisi A, Parete G, Flati V, Maccarone R. Up-regulation of pro-angiogenic pathways and induction of neovascularization by an acute retinal light damage. Sci Rep (2020) 10(1):1–14. doi: 10.1038/s41598-020-63449-y
245. Itaya M, Sakurai E, Nozaki M, Yamada K, Yamasaki S, Asai K, et al. Upregulation of VEGF in murine retina via monocyte recruitment after retinal scatter laser photocoagulation. Invest Ophthalmol Vis Sci (2007) 48(12):5677–83. doi: 10.1167/iovs.07-0156
246. Marneros AG. NLRP3 inflammasome blockade inhibits VEGF-a-induced age-related macular degeneration. Cell Rep (2013) 4(5):945–58. doi: 10.1016/j.celrep.2013.08.002
247. Nguyen CL, Oh LJ, Wong E, Wei J, Chilov M. Anti-vascular endothelial growth factor for neovascular age-related macular degeneration: A meta-analysis of randomized controlled trials. BMC Ophthalmol (2018) 18(1):1–15. doi: 10.1186/s12886-018-0785-3
248. Hadziahmetovic M, Malek G. Age-related macular degeneration revisited: From pathology and cellular stress to potential therapies. Front Cell Dev Biol (2021) 8:1872. doi: 10.3389/fcell.2020.612812
249. Gupta N, Brown KE, Milam AH. Activated microglia in human retinitis pigmentosa, late-onset retinal degeneration, and age-related macular degeneration. Exp Eye Res (2003) 76(4):463–71. doi: 10.1016/S0014-4835(02)00332-9
250. Lad EM, Cousins SW, Van Arnam JS, Proia AD. Abundance of infiltrating CD163+ cells in the retina of postmortem eyes with dry and neovascular age-related macular degeneration. Graefe’s Arch Clin Exp Ophthalmol (2015) 253(11):1941–5. doi: 10.1007/s00417-015-3094-z
251. Wang M, Wang X, Zhao L, Ma W, Rodriguez IR, Fariss RN, et al. Macroglia-microglia interactions via TSPO signaling regulates microglial activation in the mouse retina. J Neurosci (2014) 34(10):3793–806. doi: 10.1523/JNEUROSCI.3153-13.2014
252. Crespo-Garcia S, Reichhart N, Hernandez-Matas C, Zabulis X, Kociok N, Brockmann C, et al. In vivo analysis of the time and spatial activation pattern of microglia in the retina following laser-induced choroidal neovascularization. Exp Eye Res (2015) 139:13–21. doi: 10.1016/j.exer.2015.07.012
253. Eter N, Engel DR, Meyer L, Helb HM, Roth F, Maurer J, et al. In vivo visualization of dendritic cells, macrophages, and microglial cells responding to laser-induced damage in the fundus of the eye. Invest Ophthalmol Vis Sci (2008) 49(8):3649–58. doi: 10.1167/iovs.07-1322
254. Ma W, Silverman SM, Zhao L, Villasmil R, Campos MM, Amaral J, et al. Absence of TGFβ signaling in retinal microglia induces retinal degeneration and exacerbates choroidal neovascularization. Elife (2019) 8. doi: 10.7554/eLife.42049
255. Sennlaub F, Auvynet C, Calippe B, Lavalette S, Poupel L, Hu SJ, et al. CCR2+ monocytes infiltrate atrophic lesions in age-related macular disease and mediate photoreceptor degeneration in experimental subretinal inflammation in Cx3cr1 deficient mice. EMBO Mol Med (2013) 5(11):1775–93. doi: 10.1002/emmm.201302692
256. Zhou Y, Yoshida S, Kubo Y, Yoshimura T, Kobayashi Y, Nakama T, et al. Different distributions of M1 and M2 macrophages in a mouse model of laser-induced choroidal neovascularization. Mol Med Rep (2017) 15(6):3949–56. doi: 10.3892/mmr.2017.6491
257. Chu XK, Wang Y, Ardeljan D, Tuo J, Chan CC. Controversial view of a genetically altered mouse model of focal retinal degeneration. Bioengineered (2013) 4(3):130–5. doi: 10.4161/bioe.22949
258. Caicedo A, Espinosa-Heidmann DG, Piña Y, Hernandez EP, Cousins SW. Blood-derived macrophages infiltrate the retina and activate Muller glial cells under experimental choroidal neovascularization. Exp Eye Res (2005) 81(1):38–47. doi: 10.1016/j.exer.2005.01.013
259. Combadière C, Feumi C, Raoul W, Keller N, Rodéro M, Pézard A, et al. CX3CR1-dependent subretinal microglia cell accumulation is associated with cardinal features of age-related macular degeneration. J Clin Invest (2007) 117(10):2920–8. doi: 10.1172/JCI31692
260. Chen M, Forrester JV, Xu H. Dysregulation in retinal para-inflammation and age-related retinal degeneration in CCL2 or CCR2 deficient mice. PloS One (2011) 6(8):e22818. doi: 10.1371/journal.pone.0022818
261. Luhmann UFO, Robbie S, Munro PMG, Barker SE, Duran Y, Luong V, et al. The drusenlike phenotype in aging Ccl2-knockout mice is caused by an accelerated accumulation of swollen autofluorescent subretinal macrophages. Invest Ophthalmol Vis Sci (2009) 50(12):5934–43. doi: 10.1167/iovs.09-3462
262. Raoul W, Auvynet C, Camelo S, Guillonneau X, Feumi C, Combadière C, et al. CCL2/CCR2 and CX3CL1/CX3CR1 chemokine axes and their possible involvement in age-related macular degeneration. J Neuroinflamm (2010) 7:1–7. doi: 10.1186/1742-2094-7-87
263. Tuo J, Bojanowski CM, Zhou M, Shen D, Ross RJ, Rosenberg KL, et al. Murine Ccl2/Cx3cr1 deficiency results in retinal lesions mimicking human age-related macular degeneration. Invest Ophthalmol Vis Sci (2007) 48(8):3827–36. doi: 10.1167/iovs.07-0051
264. Zhang R, Wang LY, Wang YF, Wu CR, Lei CL, Wang MX, et al. Associations between the T280M and V249I SNPs in CX3CR1 and the risk of age-related macular degeneration. Invest Ophthalmol Vis Sci (2015) 56(9):5590–8. doi: 10.1167/iovs.15-16830
265. Tuo J, Smith BC, Bojanowski CM, Meleth AD, Gery I, Csaky KG, et al. The involvement of sequence variation and expression of CX3CR1 in the pathogenesis of age-related macular degeneration. FASEB J (2004) 18(11):1297–9. doi: 10.1096/fj.04-1862fje
266. Ma B, Dang G, Yang S, Duan L, Zhang Y. CX3CR1 polymorphisms and the risk of age-related macular degeneration. Int J Clin Exp Pathol (2015) 8(8):9592–6.
267. Chan CC, Tuo J, Bojanowski CM, Csaky KG, Green WR. Detection of CX3CR1 single nucleotide polymorphism and expression on archived eyes with age-related macular degeneration. Histol Histopathol (2005) 20(3):857–63. doi: 10.14670/HH-20.857
268. Despriet DDG, Bergen AAB, Merriam JE, Zernant J, Barile GR, Smith RT, et al. Comprehensive analysis of the candidate genes CCL2, CCR2, and TLR4 in age-related macular degeneration. Invest Ophthalmol Vis Sci (2008) 49(1):364–71. doi: 10.1167/iovs.07-0656
269. Wolf A, Herb M, Schramm M, Langmann T. The TSPO-NOX1 axis controls phagocyte-triggered pathological angiogenesis in the eye. Nat Commun (2020) 11(1):2709. doi: 10.1038/s41467-020-16400-8
270. Toma C, De Cillà S, Palumbo A, Garhwal DP, Grossini E. Oxidative and nitrosative stress in age-related macular degeneration: A review of their role in different stages of disease. Antioxidants (2021) 10(5):653. doi: 10.3390/antiox10050653
271. Cheng SY, Cipi J, Ma S, Hafler BP, Kanadia RN, Brush RS, et al. Altered photoreceptor metabolism in mouse causes late stage age-related macular degeneration-like pathologies. Proc Natl Acad Sci USA (2020) 117(23):13094–104. doi: 10.1073/pnas.2000339117
272. Hollyfield JG, Bonilha VL, Rayborn ME, Yang X, Shadrach KG, Lu L, et al. Oxidative damage-induced inflammation initiates age-related macular degeneration. Nat Med (2008) 14(2):194–8. doi: 10.1038/nm1709
273. Luhmann UFO, Carvalho LS, Robbie SJ, Cowing JA, Duran Y, Munro PMG, et al. Ccl2, Cx3cr1 and Ccl2/Cx3cr1 chemokine deficiencies are not sufficient to cause age-related retinal degeneration. Exp Eye Res (2013) 107:80–7. doi: 10.1016/j.exer.2012.11.015
274. Chinnery HR, McLenachan S, Humphries T, Kezic JM, Chen X, Ruitenberg MJ, et al. Accumulation of murine subretinal macrophages: effects of age, pigmentation and CX3CR1. Neurobiol Aging (2012) 33(8):1769–76. doi: 10.1016/j.neurobiolaging.2011.03.010
275. Mattapallil MJ, Wawrousek EF, Chan CC, Zhao H, Roychoudhury J, Ferguson TA, et al. The Rd8 mutation of the Crb1 gene is present in vendor lines of C57BL/6N mice and embryonic stem cells, and confounds ocular induced mutant phenotypes. Invest Ophthalmol Vis Sci (2012) 53(6):2921–7. doi: 10.1167/iovs.12-9662
276. Vessey KA, Greferath U, Jobling AI, Phipps JA, Ho T, Waugh M, et al. Ccl2/Cx3cr1 knockout mice have inner retinal dysfunction but are not an accelerated model of AMD. Invest Ophthalmol Vis Sci (2012) 53(12):7833–46. doi: 10.1167/iovs.12-10650
277. Sahu B, Chavali VRM, Alapati A, Suk J, Bartsch DU, Jablonski MM, et al. Presence of rd8 mutation does not alter the ocular phenotype of late-onset retinal degeneration mouse model. Mol Vis (2015) 21:273.
278. Yu C, Roubeix C, Sennlaub F, Saban DR. Microglia versus monocytes: Distinct roles in degenerative diseases of the retina. Trends Neurosci (2020) 43:433–49. doi: 10.1016/j.tins.2020.03.012
279. Lückoff A, Caramoy A, Scholz R, Prinz M, Kalinke U, Langmann T. Interferon-beta signaling in retinal mononuclear phagocytes attenuates pathological neovascularization. EMBO Mol Med (2016) 8(6):670–8. doi: 10.15252/emmm.201505994
280. Tosi GM, Caldi E, Neri G, Nuti E, Marigliani D, Baiocchi S, et al. HTRA1 and TGF-β1 concentrations in the aqueous humor of patients with neovascular age-related macular degeneration. Invest Ophthalmol Vis Sci (2017) 58(1):162–7. doi: 10.1167/iovs.16-20922
281. Tosi GM, Neri G, Caldi E, Fusco F, Bacci T, Tarantello A, et al. TGF-β concentrations and activity are down-regulated in the aqueous humor of patients with neovascular age-related macular degeneration. Sci Rep (2018) 8(1):1–9. doi: 10.1038/s41598-018-26442-0
282. Tosi GM, Orlandini M, Galvagni F. The controversial role of tgf-β in neovascular age-related macular degeneration pathogenesis. Int J Mol Sci (2018) 19:3363. doi: 10.3390/ijms19113363
283. Bai Y, Liang S, Yu W, Zhao M, Huang L, Zhao M, et al. Semaphorin 3A blocks the formation of pathologic choroidal neovascularization induced by transforming growth factor beta. Mol Vis (2014) 20(March):1258–70.
284. Wang X, Ma W, Han S, Meng Z, Zhao L, Yin Y, et al. TGF-β participates choroid neovascularization through Smad2/3-VEGF/TNF-α signaling in mice with laser-induced wet age-related macular degeneration. Sci Rep (2017) 7(1):1–13. doi: 10.1038/s41598-017-10124-4
285. Schlecht A, Leimbeck SV, Jägle H, Feuchtinger A, Tamm ER, Braunger BM. Deletion of endothelial transforming growth factor–β signaling leads to choroidal neovascularization. Am J Pathol (2017) 187(11):2570–89. doi: 10.1016/j.ajpath.2017.06.018
286. Wang K, Li H, Sun R, Liu C, Luo Y, Fu S, et al. Emerging roles of transforming growth factor β signaling in wet age-related macular degeneration. Acta Biochim Biophys Sin (Shanghai) (2019) 51(1):1–8. doi: 10.1093/abbs/gmy145
287. Madeira MH, Rashid K, Ambrósio AF, Santiago AR, Langmann T. Blockade of microglial adenosine A2A receptor impacts inflammatory mechanisms, reduces ARPE-19 cell dysfunction and prevents photoreceptor loss in vitro. Sci Rep (2018) 8(1):1–15. doi: 10.1038/s41598-018-20733-2
288. Li Y, Schön C, Chen CC, Yang Z, Liegl R, Murenu E, et al. TPC2 promotes choroidal angiogenesis and inflammation in a mouse model of neovascular age-related macular degeneration. Life Sci Alliance (2021) 4(8). doi: 10.26508/lsa.202101047
289. Wooff Y, Man SM, Aggio-Bruce R, Natoli R, Fernando N. IL-1 family members mediate cell death, inflammation and angiogenesis in retinal degenerative diseases. Front Immunol (2019) 10:1618. doi: 10.3389/fimmu.2019.01618
290. Eandi CM, Messance HC, Augustin S, Dominguez E, Lavalette S, Forster V, et al. Subretinal mononuclear phagocytes induce cone segment loss via IL-1β. Elife (2016) 5(JULY). doi: 10.7554/eLife.16490
291. Antonetti DA, Klein R, Gardner TW. Diabetic retinopathy. New Engl J Med (2012) 366(13):1227–39. doi: 10.1056/NEJMra1005073
292. Barber AJ, Lieth E, Khin SA, Antonetti DA, Buchanan AG, Gardner TW. Neural apoptosis in the retina during experimental and human diabetes: Early onset and effect of insulin. J Clin Invest (1998) 102(4):783–91. doi: 10.1172/JCI2425
293. Garcia-Ramírez M, Hernández C, Villarroel M, Canals F, Alonso MA, Fortuny R, et al. Interphotoreceptor retinoid-binding protein (IRBP) is downregulated at early stages of diabetic retinopathy. Diabetologia (2009) 52(12):2633–41. doi: 10.1007/s00125-009-1548-8
294. Carrasco E, Hernández C, Miralles A, Huguet P, Farrés J, Simó R. Lower somatostatin expression is an early event in diabetic retinopathy and is associated with retinal neurodegeneration. Diabetes Care (2007) 30(11):2902–8. doi: 10.2337/dc07-0332
295. Frydkjaer-Olsen U, Hansen RS, Peto T, Grauslund J. Structural neurodegeneration correlates with early diabetic retinopathy. Int Ophthalmol (2017) 38(4):1621–6. doi: 10.1007/s10792-017-0632-1
296. Garcia-Martin E, Cipres M, Melchor I, Gil-Arribas L, Vilades E, Polo V, et al. Neurodegeneration in patients with type 2 diabetes mellitus without diabetic retinopathy. J Ophthalmol (2019) 2019:1825819. doi: 10.1155/2019/1825819
297. Sohn EH, van Dijk HW, Jiao C, Kok PHB, Jeong W, Demirkaya N, et al. Retinal neurodegeneration may precede microvascular changes characteristic of diabetic retinopathy in diabetes mellitus. Proc Natl Acad Sci USA (2016) 113(19):E2655–64. doi: 10.1073/pnas.1522014113
298. Duh EJ, Sun JK, Stitt AW. Diabetic retinopathy: Current understanding, mechanisms, and treatment strategies. JCI Insight Am Soc Clin Invest (2017) 2. doi: 10.1172/jci.insight.93751
299. Terao R, Kaneko H. Lipid signaling in ocular neovascularization. Int J Mol Sci (2020) 21:1–22. doi: 10.3390/ijms21134758
300. Kinuthia UM, Wolf A, Langmann T. Microglia and inflammatory responses in diabetic retinopathy. Front Immunol (2020) 11:564077/full. doi: 10.3389/fimmu.2020.564077/full
301. Lv K, Ying H, Hu G, Hu J, Jian Q, Zhang F. Integrated multi-omics reveals the activated retinal microglia with intracellular metabolic reprogramming contributes to inflammation in STZ-induced early diabetic retinopathy. Front Immunol (2022) 13:942768. doi: 10.3389/fimmu.2022.942768
302. Zhang R, Huang C, Chen Y, Li T, Pang L. Single-cell transcriptomic analysis revealing changes in retinal cell subpopulation levels and the pathways involved in diabetic retinopathy. Ann Transl Med (2022) 10(10):562–2. doi: 10.21037/atm-22-1546
303. Sun L, Wang R, Hu G, Liu H, Lv K, Duan Y, et al. Single cell RNA sequencing (scRNA-seq) deciphering pathological alterations in streptozotocin-induced diabetic retinas. Exp Eye Res (2021) 210:108718. doi: 10.1016/j.exer.2021.108718
304. Zeng XX, Ng YK, Ling EA. Neuronal and microglial response in the retina of streptozotocin-induced diabetic rats. Vis Neurosci (2000) 17(3):463–71. doi: 10.1017/S0952523800173122
305. Chen X, Zhou H, Gong Y, Wei S, Zhang M. Early spatiotemporal characterization of microglial activation in the retinas of rats with streptozotocin-induced diabetes. Graefe’s Arch Clin Exp Ophthalmol (2015) 253(4):519–25. doi: 10.1007/s00417-014-2727-y
306. McVicar CM, Hamilton R, Colhoun LM, Gardiner TA, Brines M, Cerami A, et al. Intervention with an erythropoietin-derived peptide protects against neuroglial and vascular degeneration during diabetic retinopathy. Diabetes (2011) 60(11):2995–3005. doi: 10.2337/db11-0026
307. Park YG, Lee JY, Kim C, Park YH. Early microglial changes associated with diabetic retinopathy in rats with streptozotocin-induced diabetes. J Diabetes Res (2021) 2021:4920937. doi: 10.1155/2021/4920937
308. Jiang M, Xie H, Zhang C, Wang T, Tian H, Lu L, et al. Enhancing fractalkine/CX3CR1 signalling pathway can reduce neuroinflammation by attenuating microglia activation in experimental diabetic retinopathy. J Cell Mol Med (2022) 26(4):1229–44. doi: 10.1111/jcmm.17179
309. Zeng HY, Green WR, Tso MOM. Microglial activation in human diabetic retinopathy. Arch Ophthalmol (2008) 126(2):227–32. doi: 10.1001/archophthalmol.2007.65
310. Ong JX, Nesper PL, Fawzi AA, Wang JM, Lavine JA. Macrophage-like cell density is increased in proliferative diabetic retinopathy characterized by optical coherence tomography angiography. Invest Ophthalmol Vis Sci (2021) 62(10):2–2. doi: 10.1167/iovs.62.10.2
311. Gaucher D, Chiappore JA, Pâques M, Simonutti M, Boitard C, Sahel JA, et al. Microglial changes occur without neural cell death in diabetic retinopathy. Vision Res (2007) 47(5):612–23. doi: 10.1016/j.visres.2006.11.017
312. Arroba AI, Alcalde-Estevez E, García-Ramírez M, Cazzoni D, de la Villa P, Sánchez-Fernández EM, et al. Modulation of microglia polarization dynamics during diabetic retinopathy in db/db mice. Biochim Biophys Acta Mol Basis Dis (2016) 1862(9):1663–74. doi: 10.1016/j.bbadis.2016.05.024
313. Shahulhameed S, Vishwakarma S, Chhablani J, Tyagi M, Pappuru RR, Jakati S, et al. A systematic investigation on complement pathway activation in diabetic retinopathy. Front Immunol (2020) 11:154. doi: 10.3389/fimmu.2020.00154
314. Mandava N, Tirado-Gonzalez V, Geiger MD, Patnaik JL, Frazer-Abel A, Lynch AM, et al. Complement activation in the vitreous of patients with proliferative diabetic retinopathy. Invest Ophthalmol Vis Sci (2020) 61(11):39. doi: 10.1167/iovs.61.11.39
315. Huang C, Fisher KP, Hammer SS, Navitskaya S, Blanchard GJ, Busik JV. Plasma exosomes contribute to microvascular damage in diabetic retinopathy by activating the classical complement pathway. Diabetes (2018) 67(8):1639–49. doi: 10.2337/db17-1587
316. Scott IU, Campochiaro PA, Newman NJ, Biousse V. Retinal vascular occlusions. Lancet (2020) 396(10266):1927–40. doi: 10.1016/S0140-6736(20)31559-2
317. Palmhof M, Frank V, Rappard P, Kortenhorn E, Demuth J, Biert N, et al. From ganglion cell to photoreceptor layer: Timeline of deterioration in a rat ischemia/reperfusion model. Front Cell Neurosci (2019) 13:174. doi: 10.3389/fncel.2019.00174
318. Palmhof M, Wagner N, Nagel C, Biert N, Stute G, Dick HB, et al. Retinal ischemia triggers early microglia activation in the optic nerve followed by neurofilament degeneration. Exp Eye Res (2020) 198:108133. doi: 10.1016/j.exer.2020.108133
319. Kaur C, Foulds WS, Ling EA. Hypoxia-ischemia and retinal ganglion cell damage. Clin Ophthalmol (2008) 2(4):879–89. doi: 10.2147/OPTH.S3361
320. Adachi M, Takahashi K, Nishikawa M, Miki H, Uyama M. High intraocular pressure-induced ischemia and reperfusion injury in the optic nerve and retina in rats. Graefe’s Arch Clin Exp Ophthalmol (1996) 234:7. doi: 10.1007/BF02539411
321. Zhang C, Lam TT, Tso MO. Heterogeneous populations of microglia/macrophages in the retina and their activation after retinal ischemia and reperfusion injury. Exp Eye Res (2005) 81(6):700–9. doi: 10.1016/j.exer.2005.04.008
322. Madeira MH, Boia R, Elvas F, Martins T, Cunha RA, Ambrósio AF, et al. Selective A2A receptor antagonist prevents microglia-mediated neuroinflammation and protects retinal ganglion cells from high intraocular pressure-induced transient ischemic injury. Trans Res (2016) 169:112–28. doi: 10.1016/j.trsl.2015.11.005
323. Zheng G-Y, Zhang C, Li Z-G. Early activation of caspase-1 after retinal ischemia and reperfusion injury in mice. Chin Med J (2004) 117(05):717–21. doi: 10.3760/cma.j.issn.0366-6999.2004.05.118
324. Ahmed A, Wang LL, Abdelmaksoud S, Aboelgheit A, Saeed S, Zhang CL. Minocycline modulates microglia polarization in ischemia-reperfusion model of retinal degeneration and induces neuroprotection. Sci Rep (2017) 7(1):1–16. doi: 10.1038/s41598-017-14450-5
325. Ebneter A, Kokona D, Jovanovic J, Zinkernagel MS. Dramatic effect of oral CSF-1R kinase inhibitor on retinal microglia revealed by In vivo scanning laser ophthalmoscopy. Transl Vis Sci Technol (2017) 6(2):10–0. doi: 10.1167/tvst.6.2.10
326. Ebneter A, Kokona D, Schneider N, Zinkernagel MS. Microglia activation and recruitment of circulating macrophages during ischemic experimental branch retinal vein occlusion. Invest Ophthalmol Vis Sci (2017) 58(2):944–53. doi: 10.1167/iovs.16-20474
327. Jovanovic J, Liu X, Kokona D, Zinkernagel MS, Ebneter A. Inhibition of inflammatory cells delays retinal degeneration in experimental retinal vein occlusion in mice. Glia (2020) 68(3):574–88. doi: 10.1002/glia.23739
328. Boia R, Elvas F, Madeira MH, Aires ID, Rodrigues-Neves AC, Tralhão P, et al. Treatment with A2A receptor antagonist KW6002 and caffeine intake regulate microglia reactivity and protect retina against transient ischemic damage. Cell Death Dis (2017) 8(10):e3065. doi: 10.1038/cddis.2017.451
329. Matejuk A, Ransohoff RM. Crosstalk between astrocytes and microglia: An overview. Front Immunol (2020) 11:1416. doi: 10.3389/fimmu.2020.01416
330. Jha MK, Jo M, Kim JH, Suk K. Microglia-astrocyte crosstalk: An intimate molecular conversation. Neuroscientist (2019) 25(3):227–40. doi: 10.1177/1073858418783959?url_ver=Z39.88-2003
331. Wang M, Wong WT. Microglia-müller cell interactions in the retina. Adv Exp Med Biol (2014) 801:333–8. doi: 10.1007/978-1-4614-3209-8_42
332. Vecino E, Kwok JCF, Vecino E, Kwok JCF. The extracellular matrix in the nervous system: The good and the bad aspects. Composition Funct Extracell Matrix Hum Body (2016). doi: 10.5772/62527
333. Ventura-Antunes A, Herculano-Houzel S. Energy supply per neuron is constrained by capillary density in the mouse brain. Front Integr Neurosci (2022) 16:106. doi: 10.3389/fnint.2022.760887
334. Zhi Z, Chao JR, Wietecha T, Hudkins KL, Alpers CE, Wang RK. Noninvasive imaging of retinal morphology and microvasculature in obese mice using optical coherence tomography and optical microangiography. Invest Ophthalmol Vis Sci (2014) 55(2):1024–30. doi: 10.1167/iovs.13-12864
335. Lavia C, Mecê P, Nassisi M, Bonnin S, Marie-Louise J, Couturier A, et al. Retinal capillary plexus pattern and density from fovea to periphery measured in healthy eyes with swept-source optical coherence tomography angiography. Sci Rep (2020) 10:1. doi: 10.1038/s41598-020-58359-y
336. Antonovaite N, Hulshof LA, Hol EM, Wadman WJ, Iannuzzi D. Viscoelastic mapping of mouse brain tissue: Relation to structure and age. J Mech Behav BioMed Mater (2021) 113:104159. doi: 10.1016/j.jmbbm.2020.104159
337. Lu YB, Franze K, Seifert G, Steinhäuser C, Kirchhoff F, Wolburg H, et al. Viscoelastic properties of individual glial cells and neurons in the CNS. Proc Natl Acad Sci USA (2006) 103(47):17759–64. doi: 10.1073/pnas.0606150103
Keywords: microglia, retina, immune system, homeostasis, disease
Citation: Murenu E, Gerhardt M-J, Biel M and Michalakis S (2022) More than meets the eye: The role of microglia in healthy and diseased retina. Front. Immunol. 13:1006897. doi: 10.3389/fimmu.2022.1006897
Received: 29 July 2022; Accepted: 11 November 2022;
Published: 29 November 2022.
Edited by:
Inderjeet Kaur, L V Prasad Eye Institute, IndiaReviewed by:
Linlu Tian, Medical College of Wisconsin, United StatesWenxin Ma, National Eye Institute (NIH), United States
António Francisco Ambrósio, University of Coimbra, Portugal
Copyright © 2022 Murenu, Gerhardt, Biel and Michalakis. This is an open-access article distributed under the terms of the Creative Commons Attribution License (CC BY). The use, distribution or reproduction in other forums is permitted, provided the original author(s) and the copyright owner(s) are credited and that the original publication in this journal is cited, in accordance with accepted academic practice. No use, distribution or reproduction is permitted which does not comply with these terms.
*Correspondence: Elisa Murenu, elisa.murenu@cup.uni-muenchen.de; murenu.elisa@gmail.com; Stylianos Michalakis, michalakis@lmu.de