- 1Department of Neurological Surgery, Feinberg School of Medicine, Northwestern University, Chicago, IL, United States
- 2Department of Pediatrics, Ann and Robert H. Lurie Children’s Hospital of Chicago, Chicago, IL, United States
- 3Department of Pathology, Feinberg School of Medicine, Northwestern University, Chicago, IL, United States
- 4Lurie Cancer Center, Lou and Jean Malnati Brain Tumor Institute, Chicago, IL, United States
Glioblastoma (GBM) accounts for approximately half of all malignant brain tumors, and it remains lethal with a five-year survival of less than 10%. Despite the immense advancements in the field, it has managed to evade even the most promising therapeutics: immunotherapies. The main reason is the highly spatiotemporally heterogeneous and immunosuppressive GBM tumor microenvironment (TME). Accounting for this complex interplay of TME-driven immunosuppression is key to developing effective therapeutics. This review will explore the immunomodulatory role of the extracellular matrix (ECM) by establishing its contribution to the TME as a key mediator of immune responses in GBM. This relationship will help us elucidate therapeutic targets that can be leveraged to develop and deliver more effective immunotherapies.
Introduction
Glioblastoma (GBM) accounts for approximately half of all malignant central nervous system tumors. It is one of the most aggressive malignancies, having a median overall survival of only 15 months and a five-year survival of less than 10% (1). Despite the immense advancements in the field of oncology, the standard of care treatment has not improved significantly in several decades (2, 3). The median progression-free survival after treatment is little more than 6 months (4).
Immunotherapy leverages the body’s own immune responses to specifically target and eradicate tumors. They generally fall within five categories: cancer vaccines, cytokine therapies, adoptive cell transfer, immune checkpoint inhibitors, and oncolytic therapies. Many of these therapies are becoming the additional pillar of cancer treatment by supplementing surgery, radiotherapy, chemotherapy, and targeted therapy (5). However, GBM has not benefitted from these advancements; immunotherapies such as dendritic cell vaccines, chimeric antigen receptor T cell therapy, and several checkpoint inhibitors have not delivered the expected outcomes, as they fail to show a clear survival benefit in clinical trials with high inter-patient variability (6–10). The failure of these therapies is attributed to the highly spatiotemporally heterogeneous and immunosuppressive TME (11). The TME comprises cellular and non-cellular components such as neurons, glial cells, immune cells, and extracellular matrix components (ECM). Of these components, the ECM of GBM and its interaction with immune cells is still clouded in mystery.
Around 20% of the adult brain volume is attributed to the ECM (12). The ECM of the central nervous system is unique from the rest of the body as it is predominantly non-fibrous and contains specialized forms of ECM termed perineuronal nets, as well as the meningeal and vascular basement membranes (13). The perineuronal nets vary in composition with age and location within different brain areas but are characterized by condensed regions of glycosaminoglycan-rich structures surrounding fast-spiking neurons (14). The vascular basement membrane comprises laminins, collagen IV, fibronectin, and proteoglycans essential to maintaining the blood-brain barrier (15). The brain parenchyma is composed of glycosaminoglycans like hyaluronic acid, proteoglycans, glycoproteins, and low amounts of fibrous proteins such as laminins and collagens which are synthesized by neurons and glial cells (16).
The GBM extracellular matrix is characterized by an increased overall density and tension with aberrant expression of ECM components such as hyaluronic acid (HA), tenascin-C, fibronectin, proteoglycans, and matricellular proteins (17, 18). The higher expression of extracellular molecules like tenascin-c and fibronectin predict poor prognosis in the GBM (19–21). A recent correlative study identified that there is a specific collagen signature (COL1A1, COL1A2, COL3A1, COL4A1, COL4A2, and COL5A2) that is associated with poor overall survival and increased immunosuppression (22).
The role of ECM in tumor immunity
Besides their role as structural components, the ECM also exert profound immunomodulatory effects in solid tumors. While this topic is only recently being studied in GBM, there is a wealth of studies in other tumor types that may inform us of its role in GBM. The role of ECM in immunosuppression has been extensively explored in pancreatic ductal adenocarcinoma (PDAC) tumors. The dense and fibrotic ECM in pancreatic tumors is characterized by an abundance of collagens, fibronectin, and hyaluronic acid which create physical barriers that hinder immune cell infiltration into the tumor microenvironment (23). It is thought that this barrier limits the access of cytotoxic T cells and other immune effector cells, reducing their ability to recognize and attack cancer cells.
Another mechanism through which the ECM can hinder or potentiate immunotherapies is its role in leukocyte localization and infiltration. Leukocytes must arrive at the tumor through vasculature and then overcome the blood-brain barrier (24). It is of note that CAFs release vascular endothelial growth factor (VEGF) to target the endothelial membrane and, through aberrant angiogenesis, can mediate the localization of leukocytes in the tumor vasculature (25). Since aberrant neovascularization is another characteristic of the heterogeneous TME that promotes tumorigenesis (26), its modulation by ECM components further emphasizes their importance.
To infiltrate the tumor, leukocytes must cross the endothelial basement membrane. The biochemical composition of these membranes can determine the sites of T cells and monocyte extravasation: laminin α4 defines the sites apt for leukocyte extravasation, in opposition to laminin α5, which inhibits T cell, neutrophil, and monocyte transmigration (27). Afterward, the transmigration across the parenchymal basement membrane is mediated by macrophage-released MMP2 and MMP9 (28). Overall, lymphocytic infiltration occurs due to abnormal vessel formation and decreased adhesion molecules (29). ECM components can aid or hinder anti-tumor immunity by mediating leukocyte infiltration. Regarding the specific components of ECM, below we will discuss their known roles in immunity and GBM, starting with the major producer of ECM, fibroblasts.
CAFs and collagen
Fibroblasts are the cell population responsible for the production of ECM components, particularly collagen (30). As they have historically been found within fibrous tumors such as lung adenocarcinoma and pancreatic ductal adenocarcinoma (PDAC), they have been given the title of cancer-associated fibroblasts (CAFs) (31, 32). In pancreatic cancer, excessive deposition of collagen by CAFs is thought to inhibit CD8+ T cell infiltration into the tumor (33). It was found that PDAC produce unique Collagen 1 (Col1) homotrimers, promoting oncogenic signaling, tumor growth, and immunosuppression, and their deletion enhances overall survival, T cell infiltration, and response to anti-PD-1 immunotherapy, suggesting a potential cancer-specific therapeutic target (34). In lung tumors, those resistant to PD-1/PD-L1 blockade therapy had an increase in collagen levels and a decreased total CD8+ T cell population. This study also found that the blockade of the immune-inhibitory transmembrane receptor LAIR-1, with a high affinity for collagen, sensitized tumors resistant to anti-PD-1 therapy and decreased CD8+ T cell exhaustion (35). These results can potentially be extended to GBM, as Xu et al., also found LAIR-1 expression to be upregulated in GBM (36).
However, this is conflicting evidence to these observations: A seminal study in PDAC tumors identified that depletion of CAFs from pancreatic tumors instead increased malignancy, and promoted immunosuppression, through the increase of regulatory T-cell populations (37). Indeed, a follow-up study specifically depleted collagen production in myofibroblasts which enhanced tumor growth and promoted immunosuppression in murine models of PDAC (38). Therefore, the role of cancer-associated fibroblasts (CAFs) in immunosurveillance remains controversial.
Despite the somewhat conflicting roles of CAFs in immunosuppression in these tumors, there are recent studies indicating that manipulating the ECM can promote immunotherapeutic efficacy. A recent study was able to use collagen overexpression in tumor as a way to increase immunotherapy efficacy (34). In this study, the author’s linked immune checkpoint inhibitor antibodies (anti-CTLA4 and anti- PD-L1) to a collagen-binding domain (CBD) derived from the blood protein von Willebrand factor (VWF) A3 domain. This leveraged the tumor vasculature’s leakiness, exposing the tumor stroma collagen to blood components. The therapy decreased the systemic toxicities observed with standard therapy, increased CD8+ T cell infiltration, and had significant anti-tumoral activity in murine models for skin, colon, and breast cancer.
Recent findings are beginning to uncover the importance of CAFs and the ECM in GBM. CAFs were identified in glioblastoma through spatial transcriptomics and single-cell RNA sequencing experiments that revealed pro-tumoral effects through their interaction with glioblastoma stem cells (39). This same study observed the recruitment of CAFs to the perivascular niche in GBM by glioma stem cells. CAFs also promoted GBM cell survival by conferring them resistance to ferroptosis by upregulating the long noncoding RNA DLEU1 (40). Importantly, CAFs are major producers of the immunosuppressive TGF-ß, providing a poor patient prognosis across most tumors, including GBM (41). Additionally, CAFs can recruit myeloid-derived cells and induce immunosuppressive functions of TAMs (42).
Regarding collagen, while the normal brain tissue expresses minimal amounts of collagen, gliomas overexpress it –including GBM (43). GBM cells synthesize collagen III, IV, V, VI, XVI, and XVII (44–46), and it has been implicated in GBM tumorigenesis. For example, inhibiting Collagen XVI in a human GBM cell line decreased cell invasiveness, and Collagen V and XVII have shown to modulate malignancy through ECM remodeling (47, 48). Furthermore, the ECM crosslinking enzyme lysyl oxidase regulated tumor angiogenesis through the disruption of collagen expression of GBM cells in vivo (49). In GBM, the collagen chaperone protein HSP47 overexpression has been shown to promote tumor formation through the TGF-ß mediated induction of ECM-related genes (50). While the proportion of collagen in GBM matches that of other cancers such as lung and breast, it differs in its decreased organization and more thin fibrillar collagen bundles (51). The characteristics of collagen in GBM seem to play an important role in tumorigenesis and immunosuppression, as GBM patients that had a more organized collagen structure showed higher survival and less tumor invasiveness than those with lesser organized collagen (52).
Hyaluronic acid
Hyaluronic acid affects immune cells differently depending on its polymer size as it binds to varying membrane receptors. For example, macrophages bind HA through CD44 and the receptor for HA-mediated motility (RHAMM) (53, 54). In a three-dimensional matrix containing HA, monocytes were polarized to an M2 immunosuppressive phenotype and showed an increased expression of CD44 and RHAMM (55). Intermediate weight HA, present in tumor culture supernatants from multiple cancer cell lines, including glioma, induced an early activation of macrophages followed by the formation of immunosuppressive M2-like cells are refractory to activating cytokines. Meanwhile, LMWHA and HMWHA had little to no effect (56). This finding was corroborated by Zhang et al., who confirmed this relationship with monocytes from the peripheral blood of patients with solid tumors (57). In mice models, LMW-HA was shown to induce the expression of inflammatory genes on macrophages and act as an adjuvant by enhancing T cell responses through toll-like receptor 2, the same receptor inhibited by HMW-HA (58).
In GBM, HA is abundant and heterogeneously distributed. Infiltrating T cells and CD68+ macrophages exist in areas of low HA content, and this was leveraged to increase immunotherapy efficacy through the introduction of a hyaluronidase (59). In this study, a statistically significant increase in mean survival time was observed when hyaluronidase was added to an oncolytic adenovirus therapy against GBM. The addition of hyaluronidase increased the lymphocytic infiltrates and the number of M1-polarized TAMs in the TME. However, this was accompanied by an increased expression of PD-L1. After the addition of anti-PD1 to the hyaluronidase-including oncolytic virus, the median survival time was double that of the non-hyaluronidase-containing oncolytic virus with anti-PD1. This is a clear example of how the TME component HA can be leveraged to increase the efficacy of cancer therapies.
Fibronectin
Fibronectin is an extracellular matrix protein expressed in blood vessels in normal brain tissue and overexpressed in GBM cells (60). It contains a domain that is almost exclusively expressed in newly formed blood vessels in solid tumors and thus has been identified as a potential for targeted immunotherapies (61). Furthermore, fibronectin-1 can be used to predict muscle-invasive bladder cancer patients’ response to immune checkpoint inhibitors (62).
In the lung tumor stroma, researchers found that the lymphocytes were preferentially located in regions of loose collagen and fibronectin networks (63). Additionally, the lymphocytes migrated along fibronectin fibers parallel to the vessel via a mechanism not mediated by integrin. This study demonstrates that matrix fiber composition and structure can restrict T cells from accessing the tumor cells and even guide migration away from the tumor stroma.
In GBM tumor samples, fibronectin appeared enriched in regions immediately adjacent to areas of necrosis (Figure 1). This protein has been shown to promote GBM growth, invasiveness, and vascularization (64, 65). In vivo, a fibronectin knockdown glioma cell line on mouse models delayed tumor growth through a lag in regulatory T cell recruitment, and conferred survival benefits (66). Further demonstrating the immunomodulatory capacity of the extracellular matrix.
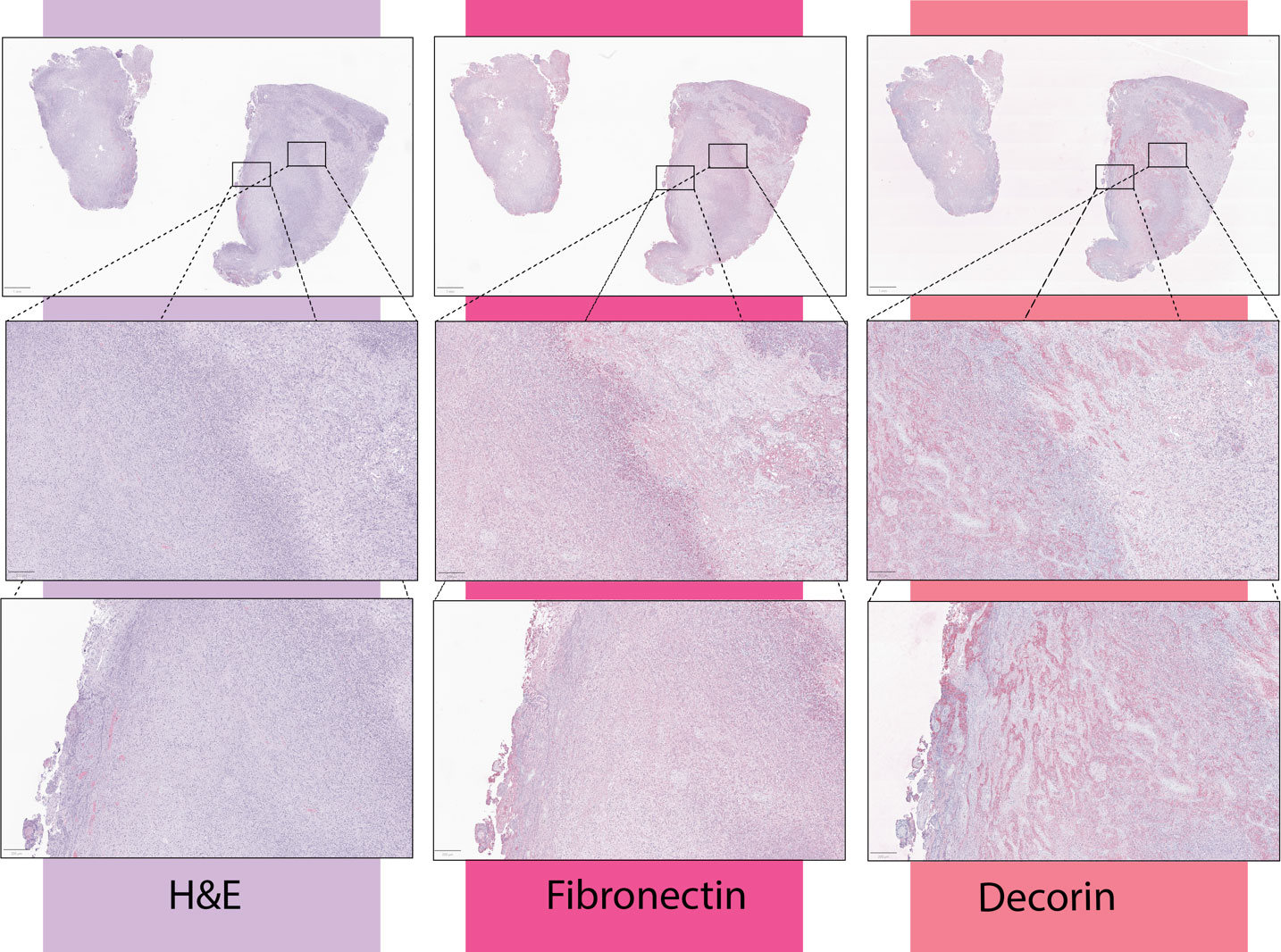
Figure 1 From a cohort of 19 newly-diagnosed GBM patient tumor samples, immunohistochemical stains to fibronectin-1 and decorin were obtained. Per review by a board-certified pathologist, fibronectin was enhanced in areas immediately adjacent to necrosis, while decorin was enhanced within the necrotic core of the tumor. Presented here are three representative samples. Samples were collected by the The Nervous System Tumor Bank at Northwestern University was used to collect all human samples under IRB number STU00202003.
Decorin
Decorin is a small proteoglycan that forms part of the extracellular matrix. It has shown anti-tumorigenic properties, disrupting TGF-ß mediated pathways and activating inflammatory responses through TLR2/4 (67). In vitro, decorin suppressed TGF-ß signaling in glioma cells (68). Inducing decorin expression in oncolytic adenovirus induced cytotoxicity of tumor cells (36, 69). An immunocompetent orthotopic xenograft breast cancer mouse model induced an immune response and decreased anti-inflammatory responses (70). Contrastingly, when decorin was loaded to oncolytic adenoviruses on mesenchymal stem cells, the inhibition of anti-inflammatory cytokines was abolished. As such, it seems that decorin’s role in inflammation depends on the cellular interactions it establishes (71).
In GBM, decorin has been shown to decrease matrix stiffness, enhance immunogenicity, induce autophagy of tumor cells, decrease VEGf expression, and mediate microglia infiltration (72–75). Furthermore, tumors that express decorin have increased infiltration of activated T cells (76). In GBM tumor samples, decorin expression was greatly increased within the necrotic core (Figure 1). While decorin overexpression in GBM has been historically a target for virotherapy (77–79) the role these therapies have on anti-tumor immunity in GBM is unknown.
Conclusion
GBM has provided the field with significant challenges as its immunosuppressive features, aberrant tumor stroma, and spatiotemporal heterogeneity impede effective anti-tumor therapeutics. The ECM is an important immunomodulatory component of the TME that can be harnessed to increase the efficacy of immunotherapies. ECM targets of interest that have shown promise in other tumors or have shown to play a role in GBM include hyaluronic acid, fibronectin, decorin, and collagen. Investigating the localization of fibronectin-1 expression in GBM, showed a denser aggregation of it adjacent to necrotic areas, while decorin expression was highest within the necrotic core (Figure 2). These samples are exemplary of the heterogeneity observed within the tumor that immunotherapies may target, particularly as a mechanism of increasing lymphocytic infiltration in areas currently untouched by them. Understanding the intra-tumoral variation of these various components can clue us in to ways of harnessing the ECM as a powerful adjunctive tool against GBM.
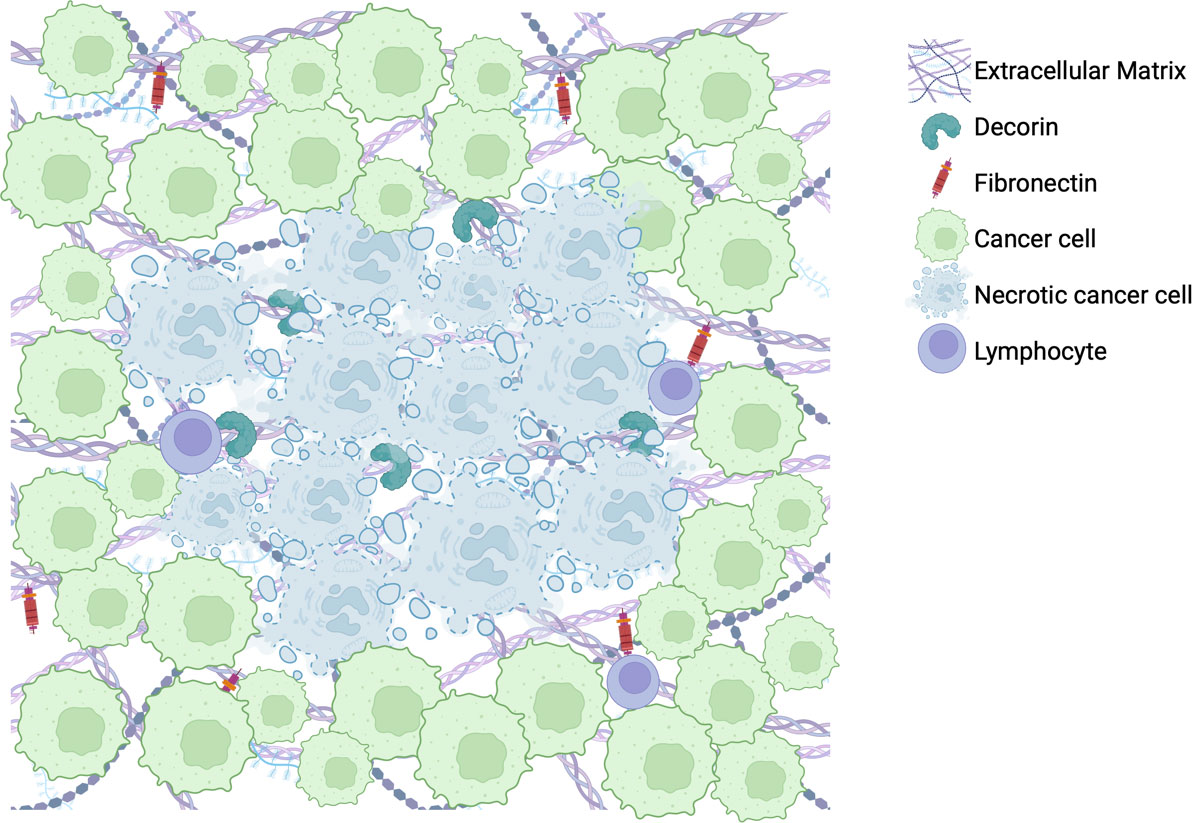
Figure 2 Illustration of representative findings of immunohistochemical stains for fibronectin-1 and decorin within a cohort of 19 newly diagnosed GBM tumor patient samples.
Data availability statement
The raw data supporting the conclusions of this article will be made available by the authors, without undue reservation.
Ethics statement
The studies involving humans were approved by Northwestern University Institutional Review Board. The studies were conducted in accordance with the local legislation and institutional requirements. The human samples used in this study were acquired from The Nervous System Tumor Bank at Northwestern University was used to collect all human samples. Written informed consent for participation was not required from the participants or the participants’ legal guardians/next of kin in accordance with the national legislation and institutional requirements.
Author contributions
JC: Conceptualization, Data curation, Formal Analysis, Investigation, Methodology, Project administration, Software, Validation, Visualization, Writing – original draft. LB: Conceptualization, Data curation, Formal Analysis, Investigation, Methodology, Software, Validation, Visualization, Writing – original draft. JA: Validation, Writing – review & editing. JM: Conceptualization, Data curation, Formal Analysis, Investigation, Methodology, Resources, Software, Supervision, Visualization, Writing – review & editing. CL-C: Conceptualization, Data curation, Formal Analysis, Funding acquisition, Investigation, Methodology, Project administration, Supervision, Validation, Writing – original draft, Writing – review & editing.
Funding
The author(s) declare financial support was received for the research, authorship, and/or publication of this article. CL-C is supported by the National Cancer Institute (NCI, NIH) grants R37CA258426 and P50CA221747, the Cancer Research Institute CLIP grant CRI4896 and the Malnati Brain Tumor Institute. JM is supported by the National Institute of Neurological Disorders and Stroke (NINDS, NIH) grant 1R01NS115955 and the National Cancer Institute (NCI, NIH) grants 1R01CA279686 and P50CA221747.
Acknowledgments
We would like to thank the staff at the Nervous System Tumor Bank (Feinberg School of Medicine, Northwestern University) for managing the interface between the clinic and the laboratory regarding GBM patients’ biological samples. We would also like to acknowledge the Stephen Coffman Trust for their support. Lastly, we are extremely grateful for the patients and their families who consented to donate their biospecimens.
Conflict of interest
The authors declare that the research was conducted in the absence of any commercial or financial relationships that could be construed as a potential conflict of interest.
Publisher’s note
All claims expressed in this article are solely those of the authors and do not necessarily represent those of their affiliated organizations, or those of the publisher, the editors and the reviewers. Any product that may be evaluated in this article, or claim that may be made by its manufacturer, is not guaranteed or endorsed by the publisher.
References
1. Schaff LR, Mellinghoff IK. Glioblastoma and other primary brain Malignancies in adults. JAMA (2023) 329(7):574. doi: 10.1001/jama.2023.0023
2. Rong L, Li N, Zhang Z. Emerging therapies for glioblastoma: current state and future directions. J Exp Clin Cancer Res (2022) 41(1):3. doi: 10.1186/s13046-022-02349-7
3. Stupp R, Mason WP, van den Bent MJ, Weller M, Fisher B, Taphoorn MJ, et al. Radiotherapy plus concomitant and adjuvant temozolomide for glioblastoma. N Engl J Med (2005) 352(10):987–96. doi: 10.1056/NEJMoa043330
4. Stupp R, Taillibert S, Kanner A, Read W, Steinberg DM, Lhermitte B, et al. Effect of tumor-treating fields plus maintenance temozolomide vs maintenance temozolomide alone on survival in patients with glioblastoma: A randomized clinical trial. JAMA (2017) 318(23):2306–16. doi: 10.1001/jama.2017.18718
5. Kumar AR, Devan AR, Nair B, Vinod BS, Nath LR. Harnessing the immune system against cancer: current immunotherapy approaches and therapeutic targets. Mol Biol Rep (2021) 48(12):8075–95. doi: 10.1007/s11033-021-06752-9
6. Datsi A, Sorg RV. Dendritic cell vaccination of glioblastoma: road to success or dead end. Front Immunol (2021) 12:770390. doi: 10.3389/fimmu.2021.770390
7. Gedeon PC, Champion CD, Rhodin KE, Woroniecka K, Kemeny HR, Bramall AN, et al. Checkpoint inhibitor immunotherapy for glioblastoma: current progress, challenges and future outlook. Expert Rev Clin Pharmacol (2020) 13(10):1147–58. doi: 10.1080/17512433.2020.1817737
8. Lim M, Xia Y, Bettegowda C, Weller M. Current state of immunotherapy for glioblastoma. Nat Rev Clin Oncol (2018) 15(7):422–42. doi: 10.1038/s41571-018-0003-5
9. Wahyuhadi J, Immadoel Haq IB, Arifianto MR, Sulistyono B, Meizikri R, Rosada A, et al. Active immunotherapy for glioblastoma treatment: A systematic review and meta-analysis. Cancer Control (2022) 29:107327482210794. doi: 10.1177/10732748221079474
10. Wu W, Klockow JL, Zhang M, Lafortune F, Chang E, Jin L, et al. Glioblastoma multiforme (GBM): An overview of current therapies and mechanisms of resistance. Pharmacol Res (2021) 171:105780. doi: 10.1016/j.phrs.2021.105780
11. Bikfalvi A, da Costa CA, Avril T, Barnier JV, Bauchet L, Brisson L, et al. Challenges in glioblastoma research: focus on the tumor microenvironment. Trends Cancer (2023) 9(1):9–27. doi: 10.1016/j.trecan.2022.09.005
12. Syková E, Nicholson C. Diffusion in brain extracellular space. Physiol Rev (2008) 88(4):1277–340. doi: 10.1152/physrev.00027.2007
13. Soles A, Selimovic A, Sbrocco K, Ghannoum F, Hamel K, Moncada EL, et al. Extracellular matrix regulation in physiology and in brain disease. Int J Mol Sci (2023) 24(8):7049. doi: 10.3390/ijms24087049
14. Testa D, Prochiantz A, Di Nardo AA. Perineuronal nets in brain physiology and disease. Semin Cell Dev Biol (2019) 89:125–35. doi: 10.1016/j.semcdb.2018.09.011
15. Reed MJ, Damodarasamy M, Banks WA. The extracellular matrix of the blood–brain barrier: structural and functional roles in health, aging, and Alzheimer’s disease. Tissue Barriers (2019) 7(4):1651157. doi: 10.1080/21688370.2019.1651157
16. Song I, Dityatev A. Crosstalk between glia, extracellular matrix and neurons. Brain Res Bulletin (2018) 136:101–8. doi: 10.1016/j.brainresbull.2017.03.003
17. Kai F, Drain AP, Weaver VM. The extracellular matrix modulates the metastatic journey. Dev Cell (2019) 49(3):332–46. doi: 10.1016/j.devcel.2019.03.026
18. Mohiuddin E, Wakimoto H. Extracellular matrix in glioblastoma: opportunities for emerging therapeutic approaches. Am J Cancer Res (2021) 11(8):3742–54.
19. Colman H, Zhang L, Sulman EP, McDonald JM, Shooshtari NL, Rivera A, et al. A multigene predictor of outcome in glioblastoma. Neuro Oncol (2010) 12(1):49–57. doi: 10.1093/neuonc/nop007
20. Tsidulko AY, Kazanskaya GM, Volkov AM, Suhovskih AV, Kiselev RS, Kobozev VV, et al. Chondroitin sulfate content and decorin expression in glioblastoma are associated with proliferative activity of glioma cells and disease prognosis. Cell Tissue Res (2020) 379(1):147–55. doi: 10.1007/s00441-019-03127-2
21. Wu S, Liu C, Wei X, Nong W-X, Lin L-N, Li F, et al. High expression of fibronectin 1 predicts a poor prognosis in glioblastoma. Curr Med Science (2022) 42(5):1055–65. doi: 10.1007/s11596-022-2638-9
22. Yin W, Zhu H, Tan J, Xin Z, Zhou Q, Cao Y, et al. Identification of collagen genes related to immune infiltration and epithelial-mesenchymal transition in glioma. Cancer Cell Int (2021) 21(1):276. doi: 10.1186/s12935-021-01982-0
23. Jiang H, Hegde S, DeNardo DG. Tumor-associated fibrosis as a regulator of tumor immunity and response to immunotherapy. Cancer Immunol Immunother (2017) 66(8):1037–48. doi: 10.1007/s00262-017-2003-1
24. Erickson MA, Banks WA. Neuroimmune axes of the blood–brain barriers and blood–brain interfaces: bases for physiological regulation, disease states, and pharmacological interventions. Pharmacol Rev (2018) 70(2):278–314. doi: 10.1124/pr.117.014647
25. Song E, Mao T, Dong H, Boisserand LSB, Antila S, Bosenberg M, et al. VEGF-C-driven lymphatic drainage enables immunosurveillance of brain tumours. Nature (2020) 577(7792):689–94. doi: 10.1038/s41586-019-1912-x
26. Mosteiro A, Pedrosa L, Ferres A, Diao D, Sierra A, Gonzalez JJ. The vascular microenvironment in glioblastoma: A comprehensive review. Biomedicines (2022) 10(6):3–4. doi: 10.3390/biomedicines10061285
27. Congdon KL, Sanchez-Perez LA, Sampson JH. Effective effectors: How T cells access and infiltrate the central nervous system. Pharmacol Ther (2019) 197:52–60. doi: 10.1016/j.pharmthera.2018.12.007
28. Sorokin L. The impact of the extracellular matrix on inflammation. Nat Rev Immunol (2010) 10(10):712–23. doi: 10.1038/nri2852
29. Peranzoni E, Rivas-Caicedo A, Bougherara H, Salmon H, Donnadieu E. Positive and negative influence of the matrix architecture on antitumor immune surveillance. Cell Mol Life Sci (2013) 70(23):4431–48. doi: 10.1007/s00018-013-1339-8
30. Hinz B, Phan SH, Thannickal VJ, Prunotto M, Desmoulière A, Varga J, et al. Recent developments in myofibroblast biology: paradigms for connective tissue remodeling. Am J Pathol (2012) 180(4):1340–55. doi: 10.1016/j.ajpath.2012.02.004
31. Olumi AF, Grossfeld GD, Hayward SW, Carroll PR, Tlsty TD, Cunha GR. Carcinoma-associated fibroblasts direct tumor progression of initiated human prostatic epithelium. Cancer Res (1999) 59(19):5002–11. doi: 10.1186/bcr138
32. Sahai E, Astsaturov I, Cukierman E, DeNardo DG, Egeblad M, Evans RM, et al. A framework for advancing our understanding of cancer-associated fibroblasts. Nat Rev Cancer (2020) 20(3):174–86. doi: 10.1038/s41568-019-0238-1
33. Hartmann N, Giese NA, Giese T, Poschke I, Offringa R, Werner J, et al. Prevailing role of contact guidance in intrastromal T-cell trapping in human pancreatic cancer. Clin Cancer Res (2014) 20:3422 – 33. doi: 10.1158/1078-0432.CCR-13-2972
34. Ishihara J, Ishihara A, Sasaki K, Lee SS, Williford JM, Yasui M, et al. Targeted antibody and cytokine cancer immunotherapies through collagen affinity. Sci Transl Med (2019) 11(487):eaau3259. doi: 10.1126/scitranslmed.aau3259
35. Peng DH, Rodriguez BL, Diao L, Chen L, Wang J, Byers LA, et al. Collagen promotes anti-PD-1/PD-L1 resistance in cancer through LAIR1-dependent CD8+ T cell exhaustion. Nat Commun (2020) 11(1). doi: 10.1038/s41467-020-18298-8
36. Xu L, Wang S, Li J, Li J, Li B. Cancer immunotherapy based on blocking immune suppression mediated by an immune modulator LAIR-1. OncoImmunol (2020) 9(1):1740477. doi: 10.1080/2162402X.2020.1740477
37. Özdemir BC, Pentcheva-Hoang T, Carstens JL, Zheng X, Wu CC, Simpson TR, et al. Depletion of carcinoma-associated fibroblasts and fibrosis induces immunosuppression and accelerates pancreas cancer with reduced survival. Cancer Cell (2014) 25(6):719–34. doi: 10.1016/j.ccr.2014.04.005
38. Chen Y, Kim J, Yang S, Wang H, Wu CJ, Sugimoto H, et al. Type I collagen deletion in αSMA(+) myofibroblasts augments immune suppression and accelerates progression of pancreatic cancer. Cancer Cell (2021) 39(4):548–65.e6. doi: 10.1016/j.ccell.2021.02.007
39. Jain S, Rick JW, Joshi RS, Beniwal A, Spatz J, Gill S, et al. Single-cell RNA sequencing and spatial transcriptomics reveal cancer-associated fibroblasts in glioblastoma with protumoral effects. J Clin Invest (2023) 133(5). doi: 10.1172/JCI147087
40. Zhao J, Yang S, Lv C, Liu Y. Cancer-associated fibroblasts suppressed ferroptosis in glioblastoma via upregulating lncRNA DLEU1. Am J Physiol Cell Physiol (2023) 324(5):C1039–c52. doi: 10.1152/ajpcell.00454.2022
41. Kalluri R. The biology and function of fibroblasts in cancer. Nat Rev Cancer (2016) 16(9):582–98. doi: 10.1038/nrc.2016.73
42. Turley SJ, Cremasco V, Astarita JL. Immunological hallmarks of stromal cells in the tumour microenvironment. Nat Rev Immunol (2015) 15(11):669–82. doi: 10.1038/nri3902
43. Ogawa K, Oguchi M, Nakashima Y, Yamabe H. Distribution of collagen type IV in brain tumors: an immunohistochemical study. J Neurooncol (1989) 7(4):357–66. doi: 10.1007/BF02147093
44. Bauer R, Ratzinger S, Wales L, Bosserhoff A, Senner V, Grifka J, et al. Inhibition of collagen XVI expression reduces glioma cell invasiveness. Cell Physiol Biochem (2011) 27(3-4):217–26. doi: 10.1159/000327947
45. Dong H, Luo L, Hong S, Siu H, Xiao Y, Jin L, et al. Integrated analysis of mutations, miRNA and mRNA expression in glioblastoma. BMC Syst Biol (2010) 4:163. doi: 10.1186/1752-0509-4-163
46. Han J, Daniel JC, Lieska N, Pappas GD. Immunofluorescence and biochemical studies of the type VI collagen expression by human glioblastoma cells in vitro. Neurological Res (1994) 16(5):370–5. doi: 10.1080/01616412.1994.11740256
47. Tsai H-F, Chang Y-C, Li C-H, Chan M-H, Chen C-L, Tsai W-C, et al. Type V collagen alpha 1 chain promotes the Malignancy of glioblastoma through PPRC1-ESM1 axis activation and extracellular matrix remodeling. Cell Death Discovery (2021) 7(1):313. doi: 10.1038/s41420-021-00661-3
48. Yan X, Zhang C, Liang T, Yang F, Wang H, Wu F, et al. A PTEN-COL17A1 fusion gene and its novel regulatory role in Collagen XVII expression and GBM Malignance. Oncotarget (2017) 8(49):85794–803. doi: 10.18632/oncotarget.20526
49. Mammoto T, Jiang A, Jiang E, Panigrahy D, Kieran MW, Mammoto A. Role of collagen matrix in tumor angiogenesis and glioblastoma multiforme progression. Am J Pathol (2013) 183(4):1293–305. doi: 10.1016/j.ajpath.2013.06.026
50. Jiang X, Zhou T, Wang Z, Qi B, Xia H. HSP47 promotes glioblastoma stemlike cell survival by modulating tumor microenvironment extracellular matrix through TGF-β Pathway. ACS Chem Neurosci (2017) 8(1):128–34. doi: 10.1021/acschemneuro.6b00253
51. Widodo SS, Dinevska M, Cuzcano L, Papanicolaou M, Cox TR, Stylli SS, et al. Spatial analysis of the metastatic brain tumor immune and extracellular matrix microenvironment. Adv Cancer Biol - Metastasis (2023) 7:100096. doi: 10.1016/j.adcanc.2023.100096
52. Pointer KB, Clark PA, Schroeder AB, Salamat MS, Eliceiri KW, Kuo JS. Association of collagen architecture with glioblastoma patient survival. J Neurosurg (2017) 126(6):1812–21. doi: 10.3171/2016.6.JNS152797
53. Erickson M, Stern R. Chain gangs: new aspects of hyaluronan metabolism. Biochem Res Int (2012) 2012:893947. doi: 10.1155/2012/893947
54. Tavianatou AG, Caon I, Franchi M, Piperigkou Z, Galesso D, Karamanos NK. Hyaluronan: molecular size-dependent signaling and biological functions in inflammation and cancer. FEBS J (2019) 286(15):2883–908. doi: 10.1111/febs.14777
55. Kim H, Cha J, Jang M, Kim P. Hyaluronic acid-based extracellular matrix triggers spontaneous M2-like polarity of monocyte/macrophage. Biomater Science (2019) 7(6):2264–71. doi: 10.1039/C9BM00155G
56. Kuang D-M, Wu Y, Chen N, Cheng J, Zhuang S-M, Zheng L. Tumor-derived hyaluronan induces formation of immunosuppressive macrophages through transient early activation of monocytes. Blood (2007) 110(2):587–95. doi: 10.1182/blood-2007-01-068031
57. Zhang B, Du Y, He Y, Liu Y, Zhang G, Yang C, et al. INT-HA induces M2-like macrophage differentiation of human monocytes via TLR4-miR-935 pathway. Cancer Immunol Immunother (2019) 68(2):189–200. doi: 10.1007/s00262-018-2261-6
58. Scheibner KA, Lutz MA, Boodoo S, Fenton MJ, Powell JD, Horton MR. Hyaluronan fragments act as an endogenous danger signal by engaging TLR2. J Immunol (2006) 177(2):1272–81. doi: 10.4049/jimmunol.177.2.1272
59. Kiyokawa J, Kawamura Y, Ghouse SM, Acar S, Barcin E, Martinez-Quintanilla J, et al. Modification of extracellular matrix enhances oncolytic adenovirus immunotherapy in glioblastoma. Clin Cancer Res (2021) 27(3):889–902. doi: 10.1158/1078-0432.CCR-20-2400
60. Dzikowski L, Mirzaei R, Sarkar S, Kumar M, Bose P, Bellail A, et al. Fibrinogen in the glioblastoma microenvironment contributes to the invasiveness of brain tumor-initiating cells. Brain Pathol (2021) 31(5). doi: 10.1111/bpa.12947
61. Lieverse RIY, Marcus D, van der Wiel AMA, Van Limbergen EJ, Theys J, Yaromina A, et al. Human fibronectin extra domain B as a biomarker for targeted therapy in cancer. Mol Oncol (2020) 14(7):1555–68. doi: 10.1002/1878-0261.12705
62. Zhang X, Liu H, Zhang J, Wang Z, Yang S, Liu D, et al. Fibronectin-1: A predictive immunotherapy response biomarker for muscle−Invasive bladder cancer. Arch Esp Urol (2023) 76(1):70–83. doi: 10.56434/j.arch.esp.urol.20237601.7
63. Salmon H, Franciszkiewicz K, Damotte D, Dieu-Nosjean M-C, Validire P, Trautmann A, et al. Matrix architecture defines the preferential localization and migration of T cells into the stroma of human lung tumors. J Clin Invest (2012) 122(3):899–910. doi: 10.1172/JCI45817
64. Serres E, Debarbieux F, Stanchi F, Maggiorella L, Grall D, Turchi L, et al. Fibronectin expression in glioblastomas promotes cell cohesion, collective invasion of basement membrane in vitro and orthotopic tumor growth in mice. Oncogene (2014) 33(26):3451–62. doi: 10.1038/onc.2013.305
65. Yu S, Yu X, Sun L, Zheng Y, Chen L, Xu H, et al. GBP2 enhances glioblastoma invasion through Stat3/fibronectin pathway. Oncogene (2020) 39(27):5042–55. doi: 10.1038/s41388-020-1348-7
66. Sengupta S, Nandi S, Hindi ES, Wainwright DA, Han Y, Lesniak MS. Short hairpin RNA-mediated fibronectin knockdown delays tumor growth in a mouse glioma model. Neoplasia (2010) 12(10):837–47. doi: 10.1593/neo.10662
67. Xie C, Mondal DK, Ulas M, Neill T, Iozzo RV. Oncosuppressive roles of decorin through regulation of multiple receptors and diverse signaling pathways. Am J Physiol Cell Physiol (2022) 322(3):C554–C66. doi: 10.1152/ajpcell.00016.2022
68. Yao T, Zhang CG, Gong MT, Zhang M, Wang L, Ding W. Decorin-mediated inhibition of the migration of U87MG glioma cells involves activation of autophagy and suppression of TGF-β signaling. FEBS Open Bio (2016) 6(7):707–19. doi: 10.1002/2211-5463.12076
69. Yang Y, Xu W, Neill T, Hu Z, Wang CH, Xiao X, et al. Systemic delivery of an oncolytic adenovirus expressing decorin for the treatment of breast cancer bone metastases. Hum Gene Ther (2015) 26(12):813–25. doi: 10.1089/hum.2015.098
70. Zhao J, Chen AX, Gartrell RD, Silverman AM, Aparicio L, Chu T, et al. Immune and genomic correlates of response to anti-PD-1 immunotherapy in glioblastoma. Nat Med (2019) 25(3):462–9. doi: 10.1038/s41591-019-0349-y
71. Zhang Y, Liu C, Wang T, Kong F, Zhang H, Yi J, et al. Therapeutic effects of mesenchymal stem cells loaded with oncolytic adenovirus carrying decorin on a breast cancer lung metastatic mouse model. Mol Ther - Oncolytics (2022) 24:486–96. doi: 10.1016/j.omto.2022.01.007
72. Engel S, Isenmann S, Ständer M, Rieger J, Bähr M, Weller M. Inhibition of experimental rat glioma growth by decorin gene transfer is associated with decreased microglial infiltration. J Neuroimmunol (1999) 99(1):13–8. doi: 10.1016/S0165-5728(99)00062-4
73. Jia Y, Feng Q, Tang B, Luo X, Yang Q, Yang H, et al. Decorin Suppresses Invasion and EMT Phenotype of Glioma by Inducing Autophagy via c-Met/Akt/mTOR Axis. Front Oncol (2021) 11. doi: 10.3389/fonc.2021.659353
74. Münz C, Naumann U, Grimmel C, Rammensee H-G, Weller M. TGF-β-independent induction of immunogenicity by decorin gene transfer in human Malignant glioma cells. Eur J Immunol (1999) 29(3):1032–40. doi: 10.1002/(SICI)1521-4141(199903)29:03<1032::AID-IMMU1032>3.0.CO;2-W
75. Patel KS, Yao J, Raymond C, Yong W, Everson R, Liau LM, et al. Decorin expression is associated with predictive diffusion MR phenotypes of anti-VEGF efficacy in glioblastoma. Sci Rep (2020) 10(1). doi: 10.1038/s41598-020-71799-w
76. Ständer M, Naumann U, Dumitrescu L, Heneka M, Löschmann P, Gulbins E, et al. Decorin gene transfer-mediated suppression of TGF-β synthesis abrogates experimental Malignant glioma growth in vivo. Gene Ther (1998) 5(9):1187–94. doi: 10.1038/sj.gt.3300709
77. Biglari A, Bataille D, Naumann U, Weller M, Zirger J, Castro MG, et al. Effects of ectopic decorin in modulating intracranial glioma progression in vivo, in a rat syngeneic model. Cancer Gene Ther (2004) 11(11):721–32. doi: 10.1038/sj.cgt.7700783
78. Ma HI, Hueng DY, Shui HA, Han JM, Wang CH, Lai YH, et al. Intratumoral decorin gene delivery by AAV vector inhibits brain glioblastomas and prolongs survival of animals by inducing cell differentiation. Int J Mol Sci (2014) 15(3):4393–414. doi: 10.3390/ijms15034393
Keywords: glioblastoma, extracellular matrix, tumor microenvironment, immunotherapy, cancer-associated fibroblasts, fibronectin, decorin, collagen
Citation: Collado J, Boland L, Ahrendsen JT, Miska J and Lee-Chang C (2024) Understanding the glioblastoma tumor microenvironment: leveraging the extracellular matrix to increase immunotherapy efficacy. Front. Immunol. 15:1336476. doi: 10.3389/fimmu.2024.1336476
Received: 10 November 2023; Accepted: 24 January 2024;
Published: 06 February 2024.
Edited by:
Jose R. Pineda, University of the Basque Country, SpainReviewed by:
Sadhak Sengupta, Triumvira Immunologics, Inc., United StatesCopyright © 2024 Collado, Boland, Ahrendsen, Miska and Lee-Chang. This is an open-access article distributed under the terms of the Creative Commons Attribution License (CC BY). The use, distribution or reproduction in other forums is permitted, provided the original author(s) and the copyright owner(s) are credited and that the original publication in this journal is cited, in accordance with accepted academic practice. No use, distribution or reproduction is permitted which does not comply with these terms.
*Correspondence: Jason Miska, Jason.miska@northwestern.edu; Catalina Lee-Chang, catalina.leechang@northwestern.edu