- 1Division of Pulmonary and Critical Care Medicine and Department of Medicine, Stanford University, Stanford, CA, United States
- 2Medical and Molecular Genetics Institute (INGEMM), IdiPaz, Hospital Universitario La Paz, Madrid, Spain
- 3CIBERER, Centro de Investigación en Red de Enfermedades Raras, Instituto de Salud Carlos III, Madrid, Spain
- 4Pulmonary Hypertension Unit, Department of Cardiology, Hospital Universitario Doce de Octubre, Madrid, Spain
- 5Centro de Investigación Biomedica en Red en Enfermedades Cardiovasculares, Instituto de Salud Carlos III (CIBERCV), Madrid, Spain
- 6Intellectual Disability, TeleHealth, Autism and Congenital Anomalies (ITHACA), European Reference Network on Rare Congenital Malformations and Rare Intellectual Disability, Brussels, Belgium
Background: Pulmonary arterial hypertension (PAH) is a rare disease characterized by pulmonary vascular remodeling and right heart failure. Specific genetic variants increase the incidence of PAH in carriers with a family history of PAH, those who suffer from certain medical conditions, and even those with no apparent risk factors. Inflammation and immune dysregulation are related to vascular remodeling in PAH, but whether genetic susceptibility modifies the PAH immune response is unclear. TNIP2 and TRAF2 encode for immunomodulatory proteins that regulate NF-κB activation, a transcription factor complex associated with inflammation and vascular remodeling in PAH.
Methods: Two unrelated families with PAH cases underwent whole-exome sequencing (WES). A custom pipeline for variant prioritization was carried out to obtain candidate variants. To determine the impact of TNIP2 and TRAF2 in cell proliferation, we performed an MTS [3-(4,5-dimethylthiazol-2-yl)-5-(3-carboxymethoxyphenyl)-2-(4-sulfophenyl)-2H-tetrazolium] assay on healthy lung pericytes transfected with siRNA specific for each gene. To measure the effect of loss of TNIP2 and TRAF2 on NF-kappa-beta (NF-κB) activity, we measured levels of Phospho-p65-NF-κB in siRNA-transfected pericytes using western immunoblotting.
Results: We discovered a novel missense variant in the TNIP2 gene in two affected individuals from the same family. The two patients had a complex form of PAH with interatrial communication and scleroderma. In the second family, WES of the proband with PAH and primary biliary cirrhosis revealed a de novo protein-truncating variant in the TRAF2. The knockdown of TNIP2 and TRAF2 increased NF-κB activity in healthy lung pericytes, which correlated with a significant increase in proliferation over 24 h.
Conclusions: We have identified two rare novel variants in TNIP2 and TRAF2 using WES. We speculate that loss of function in these genes promotes pulmonary vascular remodeling by allowing overactivation of the NF-κB signaling activity. Our findings support a role for WES in helping identify novel genetic variants associated with dysfunctional immune response in PAH.
Introduction
Pulmonary arterial hypertension (PAH) is a rare disorder associated with progressive elevation of pulmonary pressures that, if untreated, leads to right heart failure and death (1, 2). The major pathological features of PAH are obliterative vasculopathy and progressive loss of distal pulmonary microvessels that are unresponsive to available therapies (3, 4). While the cause of PAH remains incompletely understood, pathogenic variants in the bone morphogenetic protein receptor 2 (BMPR2) have been associated with both familial (60%) and sporadic PAH (20–25%) (5, 6). Studies have now shown that BMPR2 is responsible for ensuring appropriate vascular repair in response to injury and loss of function variants are associated with impaired angiogenesis, as well as endothelial and smooth muscle cell proliferation within the vessel wall (7–9). Given the low penetrance of BMPR2 variants, it is likely that additional genetic modifiers are required for the development of PAH. Besides genetic predisposition, immune dysregulation and inflammation have been established as risk factors for PAH development (10, 11). Recent work in this area has led to identifying immune subtypes within PAH, which may explain phenotypic differences among patients (12). Understanding the interaction between genetics and immunity could provide an avenue for revealing the basis of PAH susceptibility and might offer opportunities for novel therapeutics that could change the natural history of this disease.
Whole-exome sequencing (WES) is a valuable tool for discovering novel pathogenic variants in the protein-coding regions and the genome's intron-exon boundaries. The use of WES to analyze probands and family members (i.e., trios and quartets) has led to the identification of novel disease genes associated with multiple genetic disorders, including PAH (13–16). In this study, we employed WES to detect novel pathogenic variants in a patient trio and a quartet that had previously tested negative for known PAH pathogenic variants using our customized parallel sequencing PAH gene platform (17). We report the discovery of potentially pathogenic variants in TNIP2 and TRAF2, two genes associated with inflammation and immunity whose role in PAH has not been previously documented. Through the use of patient-derived cells and in vitro gene knockdown studies, we show that TNIP2 and TRAF2 act by suppressing the activation of NF-κB, a transcription complex that controls the expression of inflammatory cytokines and genes associated with cell proliferation in PAH (18–21). We conclude that TNIP2 and TRAF2 pathogenic variants could increase PAH susceptibility through their capacity to alter cellular immune responses and drive abnormal cellular proliferation in the pulmonary vasculature.
Materials and Methods
Patient Studies
All patients involved in this study gave their informed consent to participate. Samples were obtained from the Spanish PAH registries (REHAP and REHIPED), and the ethical committee approved the project of scientific research of the Hospital Universitario La Paz, Madrid (PI-1210, PI-3996). PAH was confirmed in all patients by right heart catheterization (RHC) following the recommendations of the 6th World Symposium and the European Society of Cardiology (1, 2, 22). Screening of family members was carried out by performing a family pedigree of all individual index cases of PAH. In those with a clinical suspicion of heritable PAH, evaluation of medical history, physical examination, laboratory tests, 12-lead electrocardiogram, and transthoracic echocardiography of all family members was done to select possible affected members and RHC candidates.
Whole Exome Sequencing
The workflow used for WES analysis is summarized in Supplementary Table 1. Library preparation was carried by Agilent SureSelectTM (v. 6.0), all exons kit followed by sequencing in a NovaSeq Sequencer (Illumina, USA). The exomes were analyzed by Saphetor Varsome software (23) and VarSeq (Golden Helix, USA) to prioritize variants in the probands with segregation patterns consistent with potential disease pathogenesis. Copy number variant (CNV) analysis was carried out through VarSeq to detect large rearrangements (deletions, insertions, and Indels of more than 1 Kb). Segregation analysis and validation of the variants detected in the WES was performed by classical Sanger sequencing. Screening for pathogenic variants in well-known PAH genes was carried out through a custom gene panel described previously (17).
Variant prioritization was carried out by a custom algorithm (see Supplementary Table 1). The first step was to filter out variants not meeting the standard quality requirements for depth, genotype quality, and variant allele frequency, followed by segregation analysis based on the variant's suggested pattern of inheritance. All variants with a population frequency above 1% were filtered out, and the pathogenicity of the remaining variants was assessed using several bioinformatic tools included in the dbNSFP database (24) plus the computation of the CADD score, which predict the deleteriousness of variants throughout the human genome (25). The variant analysis was prioritized according to predicted pathogenicity. Predicted impact of variants on candidate gene function and role in PAH pathogenesis was carried out using in silico analysis via STRING and literature review, respectively.
Pericyte Isolation and Culture
Pericytes were obtained from human donor lung tissue samples and lineage was confirmed by FACS, and western immunoblotting as previously described (26–28). 3G5 (ATCC CRL-1814) hybridoma cells were cultured in 1X DMEM with 10% FBS. Culture medium was collected and concentrated by centrifugal filter Amicon Ultra-15 (Millipore). 3G5 IgM was isolated using the IgM Purification Kit (Pierce), and concentration was determined using the Lowry Method. The day before the extraction, 40 μl of rat anti-mouse IgM magnetic beads (Invitrogen 11039D) were incubated with 10 μg of 3G5 IgM overnight. The next day, fresh lung tissue was washed with 1X HBSS twice and digested in a 10 ml 1X HBSS solution containing 10 mg Collagenase (Sigma-Aldrich, USA), 1 mg dispase, and 75 μg DNase for 15–30 min at 37 degrees on an orbital shaker at vigorous speed (150 rpm). An equivalent amount of ice-cold pericyte medium (ScienCell, USA) containing 2% FBS was added immediately after digestion. The suspension was passed through a 100 μm mesh filter (BD Falcon, USA) to remove debris and undigested fibrous tissue and washed once with 1X PBS. The cell pellet was resuspended in 1 ml 1X PBS containing 0.2% FBS and 40 μl of antibody-coated magnetic beads and gently rotated in 4 degrees for 45 min. Cells were used between Passage 8–10.
Western Immunoblotting
Cells were washed three times with ice-cold 1x PBS, and lysates were prepared by adding lysis buffer (1X radio-immunoprecipitation assay buffer, 1 mMol/L phenyl-methylsulfonyl fluoride, and 1x phosphatase inhibitor) and vortexed for 10 s before centrifugation. Supernatants were transferred to fresh microcentrifuge tubes and stored at −20°C. The protein concentration was determined by Pierce BCA assay (Thermo Scientific Product #23227, Rockford, Illinois). Equal amounts of protein were loaded onto each lane of a 4–12% Bis-Tris gel (Life Technologies) and subjected to SDS-PAGE electrophoresis under reducing conditions. After blotting, polyvinylidene difluoride (PVDF) membranes were blocked for 1 h in blocking buffer (5% milk powder in 0.1% PBS/Tween 20) and incubated with primary antibodies overnight at 4°C, followed the next day by incubation with horseradish peroxidase-conjugated secondary antibodies. Bands were visualized using the ECL kit (Thermo Fisher).
RNA Interference
To achieve gene knockdown, 2 μmol/L silencing RNA (siRNA) of ABIN2 (encoded by TNIP2) (L-014328-01, Dharmacon, Lafayette, CO), TRAF2 (L-005198-00, Dharmacon, Lafayette, CO), or non-targeting siRNA control transfected into healthy pulmonary pericytes. Transfection was performed using a Nucleofector II (Program U-025; Lonza) with the Basic Smooth Muscle Cell Nucleofection kit (Lonza). All experiments were performed 48 h after electroporation.
MTS Proliferation Assay
Cells were seeded in eight replicates on collagen-coated 96-well plates (5.0 × 103 cells per well) (Nunc, Rochester, NY) and cultured in complete medium overnight at 37°C and 5% CO2. The next day, reduced serum media and MTS reagent were added to each well for 1 h in accordance with the manufacturer's instructions. MTS absorbance was measured at 490 nm.
Statistical Analysis
The number of samples studied per experiment is indicated in the figure legends. Values from multiple experiments are expressed as mean +/– SEM. Statistical significance was determined using the Mann-Whitney test. A value of P < 0.05 was considered significant.
Results
Discovery of the TNIP2 Variant
The pedigree of the first family is shown in Figure 1A. The index case was a 63-year-old female with a history of Ostium Secundum atrial septal defect (ASD), which was surgically corrected in 1994, and severe restrictive lung disease caused by thoracic deformities and diaphragmatic paralysis. The patient was diagnosed with PAH when she presented with exertional dyspnea and chest pain. Despite treatment with sildenafil and ambrisentan, the patient died 4 years after diagnosis from progressive right heart failure. Her 45-year-old daughter was diagnosed in 2015 with PAH associated with limited scleroderma and remains clinically stable with triple therapy of tadalafil, bosentan, and intravenous epoprostenol. The clinical characteristics of the two affected patients are summarized in Table 1. The remaining family members were screened and demonstrated no signs and symptoms of PAH to date. Given the familial predisposition, we conducted a genetic screen of the patients and family members using an in-house custom panel (HAPv2.1) (17), which was negative for mutations in 21 genes associated with PAH. As a next step, we conducted WES on the two patients and four unaffected family members, followed by segregation analysis (Figure 1A).
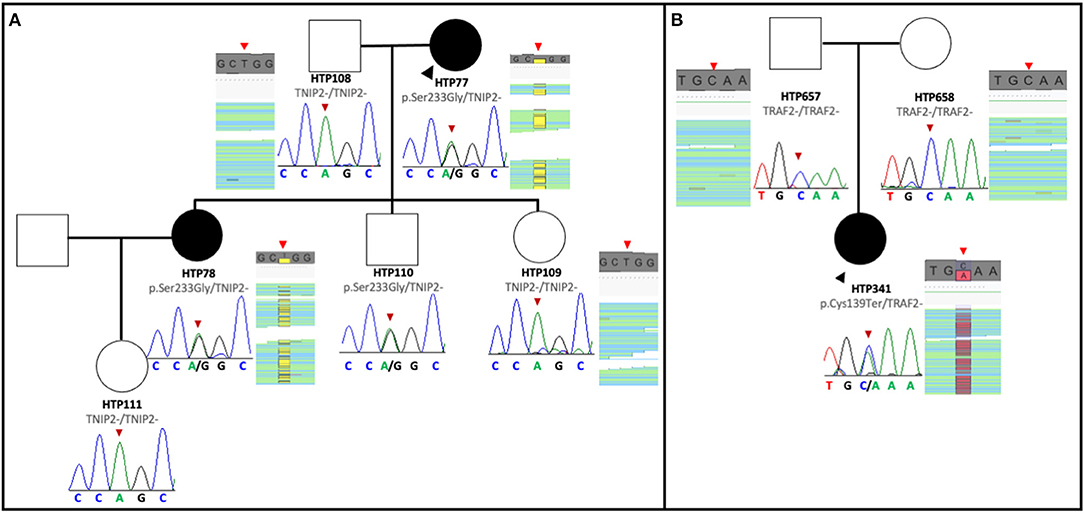
Figure 1. Pedigree of the families with TNIP2 and TRAF2 variants. (A) The TNIP2 variant was found in two affected family members and an unaffected sibling of the proband. Sanger sequencing segregation in all available members is presented, with BAM capture from the IGV software showing the vertical read coverage at the specific position (red arrows). (B) Pedigree of the family with the TRAF2 variant. The nonsense variant was detected only in the proband and confirmed with Sanger sequencing of the variant. BAM capture from the IGV software showing the vertical read coverage at the specific position (red arrows).
Analysis of WES data confirmed the absence of mutations in PAH associated genes. However, we discovered that both PAH patients were carriers of a novel missense variant in TNFAIP3 Interacting Protein 2 (TNIP2). This gene encodes for the protein ABIN2, which acts as an inhibitor of the NF-κB signaling pathway (29). The variant is predicted to change an adenine to a cytosine base at position 697 (NM_024309.4:c.697A>C), which results in a switch from serine to glycine at position 233 [p.(Ser233Gly)] of the peptide chain, located between the two ubiquitin-binding domains of the protein. This novel TNIP2 variant was predicted to be damaging based on the estimates obtained via dbSNFP and would likely result in loss of protein function (Table 2). Additionally, this variant is absent from several control population databases (gnomAD exomes, gnomAD genomes, Kaviar, Beacon, Bravo, ESP, 1000G phase III). Interestingly, one of the unaffected family members was also found to be a carrier of the same TNIP2 variant and is currently undergoing clinical surveillance in our clinic.
Discovery of the TRAF2 Variant
The pedigree of the second family is shown in Figure 1B. The index case was a 27-year-old female that presented to the clinic in 2015 complaining of dizziness and was subsequently diagnosed with idiopathic PAH. Following initiation of tadalafil and ambrisentan, the patient developed elevated liver function enzymes, which prompted ambrisentan cessation. During the workup for elevation in liver enzymes, the patient was diagnosed with primary biliary cirrhosis, and the PAH subtype was revised to portopulmonary hypertension. Intravenous epoprostenol was temporarily initiated to stabilize the patient, and treatment was later switched to triple oral therapy with tadalafil, macitentan, and selexipag (Table 1). At the time of writing, the patient remains clinically stable.
As part of the clinical evaluation, we applied the custom gene panel HAPv.2.1 to screen the patient and her unaffected parents but found no evidence of pathogenic variants. However, WES analysis detected a novel pathogenic variant in the Tumor Necrosis Factor Receptor-Associated Factor (TRAF2). This gene encodes for a protein involved in the TNF-dependent activation of NF-κB (30). The variant is predicted to change cysteine to adenosine in position 417 (NM_021138.4:c.417C>A), introducing a premature stop codon and resulting in a truncated TRAF2 transcript. Analysis of the parents' exomes showed that the variant is de novo in the proband. This variant is also absent from the control population databases (gnomAD exomes, gnomAD genomes, Kaviar, Beacon, Bravo, ESP, 1000G phase III), and the majority of the pathogenic in silico tools from the dbSNFP suggested a damaging effect for this variant (Table 2).
TNIP2 and TRAF2 Interact With Pathways Associated With PAH
To date, no studies have shown evidence of TNIP2 and TRAF2 involvement in the pathogenesis of PAH. To determine whether these genes could potentially be interacting with components of know PAH-associated pathways, we carried out an in silico protein-protein screen using STRING (Figure 2A). This tool illustrates protein-protein interactions based on function and previously described associations (31). Our STRING analysis showed that TNIP2 and TRAF2 indirectly interacted with the transforming growth factor-β (TGF-β) family genes via CAV1, a known risk gene for hereditary PAH (13). Notably, BMPR2, the gene most commonly implicated in hereditary and idiopathic PAH, is part of the TGF-β family identified within the STRING cluster. As expected, we found strong associations between TNIP2 and TRAF2 with proteins involved in the NF-κB pathway (Figures 2B,C). Of the proteins associated with familial PAH, only CAV1 directly interacts with TRAF2 based on in vitro studies demonstrating that these proteins form a cytoplasmic protein-protein complex that modulates NF-κB activation in response to TNF (32, 33).
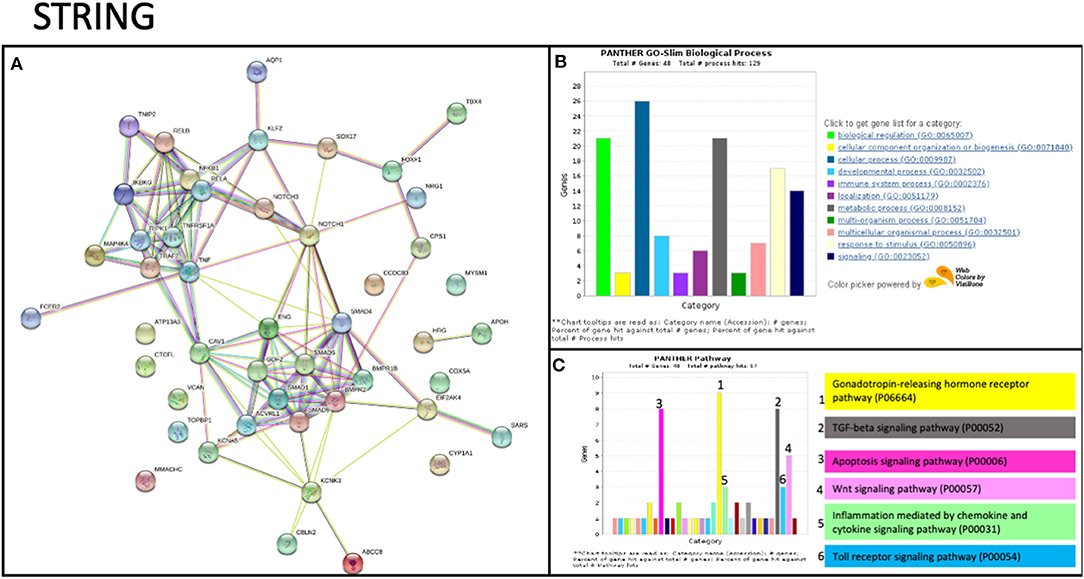
Figure 2. STRING analysis of TRAF2 and TNIP2 related to previously associated genes to PAH. (A) Protein network showing the possible interactions between all genes associated with PAH. This graph includes genes previously associated with PAH and candidate genes described in case reports/series. The strength of evidence for the relation between TRAF2 and CAV1 comes from published experimental/biochemical data and association in curated databases (see Methods). (B,C) Gene enrichment analysis, including biological processes (B) and interaction pathways (C) in which the STRING analysis genes are involved. Several genes are involved in the immune system process and participate in inflammation mediated by chemokine and cytokine signaling.
Loss of TNIP2 and TRAF2 Result in Increased Pericyte Proliferation and Activation of NF-κB
TNIP2 and TRAF2 are part of a gene network responsible for regulating the NF-κB pathway's signaling activity. The NF-κB proteins exist in the cytoplasm in an inactive state and can be rapidly activated in response to harmful stimuli such as oxidative stress, bacterial lipopolysaccharides, environmental toxins, and inflammation. Once activated, the NF-κB protein complex translocates to the nucleus and binds to target genes involved in cell proliferation, survival, and the production of cytokines that regulate innate and adaptive immune responses (34). Inappropriate NF-κB activation is associated with a wide range of diseases, including cancer, autoimmune conditions such as scleroderma, and PAH (35). Given that TNIP2 and TRAF2 serve to regulate NF-κB activity, we hypothesized that the loss of these proteins would lead to NF-κB overactivation.
To evaluate the biological consequences of deleterious variants affecting TNIP2 and TRAF2, gene silencing via siRNA transfection in human-derived pulmonary pericytes was performed. We chose to study pericytes because these cells have been shown to contribute to progressive small vessel loss and muscularization through hyperproliferation and inflammatory cytokine production (26, 27, 36). A query of our recently published pericyte RNA-seq analysis confirmed that both TNIP2 and TRAF2 are expressed in healthy and PAH pericytes, although no significant differences in expression were observed (26). Transfection of siTNIP2 and siTRAF2 resulted in >50% reduction in corresponding protein levels (Figure 3A). To determine whether TNIP2 and TRAF2 knockdown led to inappropriate activation of NF-κB, we used western immunoblotting to probe whole cell lysates for phospho-p65-NF-κB, the active form of p-65-NF-κB that becomes activated to trigger transcription of NF-κB target genes (34, 35). Stimulation with lipopolysaccharide (LPS, a known activator of NF-κB) triggered an increase in phospho-p65-NF-κB and was used as a positive control (37). Compared to baseline controls and cells transfected with a non-specific siRNA (siCtl), both siTNIP2 and siTRAF2 pericytes demonstrated a significantly increased phospho-p65-NF-κB of similar magnitude as that observed with LPS stimulation (Figure 3B). To assess the effect of TNIP2 and TRAF2 knockdown on pericyte proliferation, we used an MTS assay to quantify cell numbers under different experimental conditions over 24 h. Compared to the controls, both siTNIP2 and siTRAF2 pericytes demonstrated significantly increased cell proliferation (Figure 3C). Interestingly, the degree of proliferation observed in both siTNIP2 and siTRAF2 pericytes was similar to that of healthy pericytes stimulated with LPS.

Figure 3. Phospho- to Total-p65-NF-κB determination and MTS Assay in lung pericytes transfected with siTNIP2 and siTRAF2. (A,B) Representative western immunoblots demonstrating knockdown efficiency of TNIP2 and TRAF2 in healthy lung pericytes transfected with the corresponding siRNA (A), and the fold change of phosphorylated to total p65-NF-κB (B). (C) MTS assay of healthy lung pericytes transfected with siControl (siCtl), siTNIP2, and siTRAF2 under different experimental conditions. The data in the graphs are the average of three independent experiments.
Discussion
In this study, we utilized a WES custom gene prioritization method optimized to identify potentially pathogenic variants in PAH patients and family members to elucidate possible genetic mechanisms influencing disease development. This approach enabled the discovery of novel variants in TRAF2 and TNIP2, two genes involved in regulating the NF-κB pathway. We demonstrated that loss of TNIP2 and TRAF2 results in inappropriate NF-κB activation and increased proliferation of healthy lung pericytes through the use of siRNA-based knockdown. A graphical abstract is included in Figure 4. To our knowledge, ours is the first report to document a potential link between TNIP2/TRAF2 loss of function and PAH in humans.
Since the description of BMPR2, the increasing prevalence of genetic screening has shown that pathogenic variants in this gene are present in ~60% of hereditary PAH cases and 20-25% of idiopathic cases. BMPR2, as well as other genes (i.e., KDR, SOX17, TBX4), may also be involved in the development of associated forms of PAH (APAH) (38–41). Multiple groups have applied custom panels, whole-exome sequencing (WES), or even whole-genome sequencing to elucidate these mechanisms (42), which include variation in gene regulatory elements in addition to the protein-coding sequences themselves (43). Whole-genome sequencing in more extensive and more inclusive cohorts will be vital for uncovering intronic and regulatory genetic sequences that may impact PAH development in patients with unrevealing results upon initial gene panel screening. In many pulmonary hypertension centers, however, genetic analysis is not frequently offered in patients with PAH associated with other medical conditions (e.g., connective tissue diseases, congenital heart diseases), limiting the data available in this population. This practice will be essential to address future clinical guidelines to deepen our understanding of genetic variation in APAH.
As part of the clinical evaluation, our group routinely performs genetic screening for patients diagnosed with idiopathic, heritable, and associated PAH forms with suspected genetic components. We have developed a custom NGS panel of genes that is updated yearly to reflect published risk genes in PAH and recently reported our experience applying it in 300 patients, some of whom suffered from APAH (17). In all patients with inconclusive results on the gene panel, we move to WES, preferably including parents and other proband relatives, to screen for new risk genes. In recent years, multiple publications have demonstrated the role of genetic variants in the development of many PH subtypes, some of which were previously not known to have a genetic cause. For example, variants in the endoglin gene are linked with PAH associated with connective tissue disease (44), polymorphisms in estrogen-related genes may impact the risk of portopulmonary hypertension (45), and SOX17 have been implicated in PAH associated with congenital heart disease (41). Also, variants in CAV1, TRPM8, and BNP have been linked with PH secondary to chronic obstructive pulmonary disease (46–48). These findings underscore the importance of expanding our genetic sequencing application to PH populations not traditionally thought to have a genetic predisposition.
In the present study, our WES data suggest an association between TNIP2 and TRAF2 variants with the development of APAH. By analyzing exome data of individuals affected with PAH compared to healthy family members, we focused on genes that correlate with phenotypic differences. After our prioritization analysis, we selected the variants in TNIP2 and TRAF2 based on the criteria described in Supplementary Table 1. These two variants were absent in control population databases, and the majority of the in silico pathogenic tools suggest a damaging effect for these variants. Both variants are highly conserved through evolution, highlighting these residues' importance in the affected protein's function. Importantly, these two genes have plausible mechanistic significance as regulators of the NF-κB pathway, previously associated with PAH (49–53). As is the case with the discovery of all novel variants, consideration of pseudocontrol human population gene frequency, conservation in orthologs through evolution, pathogenicity prediction, and biological context was necessary to establish how TNIP2 and TRAF2 were involved in PAH.
The family genetic analysis showed that the variant in TNIP2 is present in both affected individuals and one unaffected member. We suspect two possible explanations for this observation. First, the variant may have incomplete penetrance or cause variable age of disease onset, allowing some carriers to be unaffected for some or all of their lives. Secondly, it may be necessary for an additional disease process to trigger the development of PAH in those who carry this variant. The high-impact nonsense TRAF2 variant identified in a separate family was only seen in the affected proband, suggesting a de novo event or germinal mosaicism of the parents. Because there was no previous evidence linking pathogenic variants in TNIP2 and TRAF2 with PAH, we performed in silico analysis to search for relationships between these genes and others implicated in PAH pathogenesis. A study of the protein network by STRING showed that TRAF2 interacts with CAV1, a gene linked to hereditary PAH (54). In addition to STRING, there are numerous methods to identify critical gene-gene interactions and visualize pathways affected by genes of interest. Available tools include GSEA from the Broad Institute for gene list analysis and EnrichmentMap to visualize relationships between distinct pathways (55). We believe that investigators should implement these algorithms as part of ongoing genetic studies in PAH.
Inflammation has garnered increasing interest in PAH in recent years (56). Immune phenotypes characterized by differing signatures of inflammatory cytokines offer prognostic and potentially therapeutic significance (12). Antiinflammatory agents such as anakinra and tocilizumab are currently being investigated in PAH clinical trials (57, 58). NF-κB is known to regulate the expression of a wide array of inflammatory genes, and both human and animal studies indicate that NF-κB activity is increased in PAH. Core processes in PAH pathophysiology, such as aberrant pulmonary artery wall cell proliferation, apoptosis, and arterial obliteration, are attenuated with NF-κB inhibition (50). The TNIP2 gene codes for ABIN2 (A20 binding inhibitor of NF-κB activation-2), a cytosolic protein that interacts with several membrane receptors (CD40, TNF receptor 1, and toll-like receptor 4) to suppress downstream activation of the NF-κB pathway (29, 59–61). TRAF2 belongs to a family of adaptor proteins associated with TNF receptors 1 and 2 to modulate TNF stimulation response in immune cells. Prior work supports TRAF2 as an essential mediator of cell survival and apoptosis through its capacity to activate or inhibit NF-κB, depending on the cell type (62, 63). Also, TRAF2 is involved in a membrane complex that can recruit caspases to promote apoptosis independent of the NF-κB pathway (64). Lastly, some authors have noted common hyperproliferative mechanisms driving PAH pathogenesis and cancer development (65), and pathogenic variants in TRAF proteins have been previously described in cancer (66). Specifically, TRAF2 has suggested roles as an oncogene in epithelial cancer, osteotropic breast cancer and colon cancer (67–69), mainly through the NF-kB pathway. As evidenced by our in vitro studies, decreased expression of TNIP2 and TRAF2 can increase NF-κB activation in human pericytes. More importantly, pericytes demonstrated a change in proliferative activity under these conditions. These data support our hypothesis that pathogenic variants in TNIP2 and TRAF2 result in dysregulated NF-κB activation and could drive abnormal cell proliferation in PAH, as illustrated by the response of lung pericytes transfected with the corresponding siRNAs (Figure 3). However, a manifestation of clinically detectable PAH may require the presence of predisposing conditions (a “second-hit”), such as primary biliary cirrhosis or scleroderma, as seen in our subjects.
Our study has several limitations. First, whole-exome data was only available for a limited number of probands and their relatives, therefore limiting our power to detect novel variants potentially associated with PAH. Secondly, the technology used for our genetic analysis variants does not allow identification of variants outside of exons or exon-intron boundaries, which may hold essential regulatory elements. Additionally, our cell studies used pericytes from human donors, not from the subjects analyzed in our study. These donors do not have predisposing inflammatory or cardiac conditions, which we believe in having been essential contributors to PAH development in the probands we analyzed. However, our data support the ongoing investigation of NF-κB as a potential therapeutic target in PAH and show that our genomics-to-bench approach can be useful for expanding our view of PAH genetics.
Conclusion
Identifying genetic biomarkers related to PAH holds the potential for a tremendous impact on patient care and the development of personalized treatments. We suggest that WES be considered for patients with associated forms of PAH that do not have causative variants identified with targeted gene panels, especially in cases with family history supportive of inherited disease. Genetic sequencing of family members with and without the disease, when feasible, will provide additional power to link genotype, phenotype, and endotype in PAH. Understanding the genetic underpinnings that predispose individuals to immune dysregulation in the pulmonary vascular wall will be paramount for advancing targeted therapies for this deadly disease. Variants in TRAF2 and TNIP2 may contribute to hyperproliferation and increased NF-κB activation in some individuals with PAH, however further studies are needed to describe the possible relationship of these genes with aberrant immune activity.
Data Availability Statement
The datasets presented in this study can be found in online repositories. The names of the repository/repositories and accession number(s) can be found below: NCBI BioProject, accession no: PRJNA702898 (ACCESSION PRIVATE).
Ethics Statement
All patients involved in this study gave their informed consent to participate. Samples were obtained from the Spanish PAH registries (REHAP and REHIPED), and the Ethical Committee approved the project of scientific research of the Hospital Universitario La Paz, Madrid (PI-1210, PI-3996).
Author Contributions
JT and VDJP designed and supervised the work. NG, PA, and JT performed all genetic studied and NGS analysis. SP, DC, SA, HP, and AC performed all the functional and in vitro studies. ACU, NO, PE, and PL reviewed all the clinical features of the patients. JN performed the microarray analysis. JT, VDJP, SP, NG, and DC wrote the manuscript. All authors contributed to the article and approved the submitted version.
Funding
VDJP was supported by NIH NHLBI R01HL134776 and R01 HL139664. This project was also supported by FEDER ISCIII Grant: PI18/01233, and by an unrestricted grant from the Spanish Foundation Against PAH (FCHP) (https://www.fchp.es/). AC holds a research-training contract Rio Hortega (CM20/00164) from the Spanish Ministry of Science and Innovation (Instituto de Salud Carlos III), Grant from FEDER (Federación Española de Enfermedades Raras; https://enfermedades-raras.org/).
Conflict of Interest
The authors declare that the research was conducted in the absence of any commercial or financial relationships that could be construed as a potential conflict of interest.
Acknowledgments
We would like to thank the National registries of patients with PAH, REHAP, and the REHIPED.
Supplementary Material
The Supplementary Material for this article can be found online at: https://www.frontiersin.org/articles/10.3389/fmed.2021.625763/full#supplementary-material
References
1. Galie N, Humbert M, Vachiery JL, Gibbs S, Lang I, Torbicki A, et al. 2015 ESC/ERS guidelines for the diagnosis and treatment of pulmonary hypertension. Rev Espanola de Cardiol. (2016) 69:177. doi: 10.1016/j.recesp.2016.01.002
2. Condon DF, Nickel NP, Anderson R, Mirza S, de Jesus Perez VA. The 6th world symposium on pulmonary hypertension: what's old is new. F1000Res. (2019) 8:F1000 Faculty Rev-888. doi: 10.12688/f1000research.18811.1
3. Rabinovitch M. Molecular pathogenesis of pulmonary arterial hypertension. J Clin Investig. (2012) 122:4306–13. doi: 10.1172/JCI60658
4. de Jesus Perez VA, Yuan K, Orcholski ME, Sawada H, Zhao M, Li CG, et al. Loss of adenomatous poliposis coli-alpha3 integrin interaction promotes endothelial apoptosis in mice and humans. Circul Res. (2012) 111:1551–64. doi: 10.1161/CIRCRESAHA.112.267849
5. Evans JD, Girerd B, Montani D, Wang XJ, Galie N, Austin ED, et al. BMPR2 mutations and survival in pulmonary arterial hypertension: an individual participant data meta-analysis. Lancet Respir Med. (2016) 4:129–37. doi: 10.1016/S2213-2600(15)00544-5
6. Machado RD, Eickelberg O, Elliott CG, Geraci MW, Hanaoka M, Loyd JE, et al. Genetics and genomics of pulmonary arterial hypertension. J Am College Cardiol. (2009) 54:S32–42. doi: 10.1016/j.jacc.2009.04.015
7. Cai J, Pardali E, Sanchez-Duffhues G, ten Dijke P. BMP signaling in vascular diseases. FEBS Lett. (2012) 586:1993–2002. doi: 10.1016/j.febslet.2012.04.030
8. Dyer LA, Pi X, Patterson C. The role of BMPs in endothelial cell function and dysfunction. Trends Endocrinol Metabol TEM. (2014) 25:472–80. doi: 10.1016/j.tem.2014.05.003
9. Tada Y, Majka S, Carr M, Harral J, Crona D, Kuriyama T, et al. Molecular effects of loss of BMPR2 signaling in smooth muscle in a transgenic mouse model of PAH. Am J Physiol Lung Cell Mol Physiol. (2007) 292:L1556–63. doi: 10.1152/ajplung.00305.2006
10. NMorrell W, Aldred MA, Chung WK, Elliott CG, Nichols WC, Soubrier F, et al. Genetics and genomics of pulmonary arterial hypertension. Eur Respir J. (2018) 53:1801899. doi: 10.1183/13993003.01899-2018
11. Zhu N, Pauciulo MW, Welch CL, Lutz KA, Coleman AW, Gonzaga-Jauregui C, et al. Novel risk genes and mechanisms implicated by exome sequencing of 2572 individuals with pulmonary arterial hypertension. Genome Med. (2019) 11:69. doi: 10.1186/s13073-019-0685-z
12. Sweatt AJ, Hedlin HK, Balasubramanian V, Hsi A, Blum LK, Robinson WH, et al. Discovery of distinct immune phenotypes using machine learning in pulmonary arterial hypertension. Circulat Res. (2019) 124:904–19. doi: 10.1161/CIRCRESAHA.118.313911
13. Austin ED, Ma L, LeDuc C, Berman Rosenzweig E, Borczuk A, Phillips JA, et al. Whole exome sequencing to identify a novel gene (caveolin-1) associated with human pulmonary arterial hypertension. Circ Cardiovasc Genet. (2012) 5:336–43. doi: 10.1161/CIRCGENETICS.111.961888
14. de Jesus Perez VA, Yuan K, Lyuksyutova MA, Dewey F, Orcholski ME, Shuffle EM, et al. Whole-exome sequencing reveals TopBP1 as a novel gene in idiopathic pulmonary arterial hypertension. Am J Respir Crit Care Med. (2014) 189:1260–72. doi: 10.1164/rccm.201310-1749OC
15. Eyries M, Montani D, Girerd B, Perret C, Leroy A, Lonjou C, et al. EIF2AK4 mutations cause pulmonary veno-occlusive disease, a recessive form of pulmonary hypertension. Nat Genet. (2014) 46:65–9. doi: 10.1038/ng.2844
16. Ma L, Roman-Campos D, Austin ED, Eyries M, Sampson SK, Soubrier F, et al. A novel channelopathy in pulmonary arterial hypertension. N Engl J Med. (2013) 369:351–61. doi: 10.1056/NEJMoa1211097
17. Castano JAT, Hernandez-Gonzalez I, Gallego N, Perez-Olivares C, Ochoa Parra N, Arias P, et al. Customized massive parallel sequencing panel for diagnosis of pulmonary arterial hypertension. Genes. (2020) 11:1158. doi: 10.3390/genes11101158
18. Fan J, Fan X, Guang H, Shan X, Tian Q, Zhang F, et al. Upregulation of miR-335-3p by NF-kappaB transcriptional regulation contributes to the induction of pulmonary arterial hypertension via APJ during hypoxia. Int J Biol Sci. (2020) 16:515–28. doi: 10.7150/ijbs.34517
19. Li Y, Yang L, Dong L, Yang ZW, Zhang J, Zhang SL, et al. Crosstalk between the Akt/mTORC1 and NF-kappaB signaling pathways promotes hypoxia-induced pulmonary hypertension by increasing DPP4 expression in PASMCs. Acta Pharmacol Sin. (2019) 40:1322–33. doi: 10.1038/s41401-019-0272-2
20. Liu WY, Wang L, Lai YF. Hepcidin protects pulmonary artery hypertension in rats by activating NF-kappaB/TNF-alpha pathway. Eur Rev Med Pharmacol Sci. (2019) 23:7573–81. doi: 10.26355/eurrev_201909_18878
21. Woods M, Wood EG, Bardswell SC, Bishop-Bailey D, Barker S, Wort SJ, et al. Warner, role for nuclear factor-kappaB and signal transducer and activator of transcription 1/interferon regulatory factor-1 in cytokine-induced endothelin-1 release in human vascular smooth muscle cells. Mol Pharmacol. (2003) 64:923–31. doi: 10.1124/mol.64.4.923
22. Thomas CA, Anderson RJ, Condon DF, de Jesus Perez VA. Diagnosis and management of pulmonary hypertension in the modern era: insights from the 6th world symposium. Pulm Ther. (2020) 6:9–22. doi: 10.1007/s41030-019-00105-5
23. Kopanos C, Tsiolkas V, Kouris A, Chapple CE, Albarca Aguilera M, Meyer R, et al. VarSome: the human genomic variant search engine. Bioinformatics. (2019) 35:1978–80. doi: 10.1093/bioinformatics/bty897
24. Liu X, Wu C, Li C, Boerwinkle E. dbNSFP v3.0: a one-stop database of functional predictions and annotations for human nonsynonymous and splice-site SNVs. Hum Mutat. (2016) 37:235–41. doi: 10.1002/humu.22932
25. Rentzsch P, Witten D, Cooper GM, Shendure J, Kircher M. CADD: predicting the deleteriousness of variants throughout the human genome. Nucleic Acids Res. (2019) 47:D886–94. doi: 10.1093/nar/gky1016
26. Yuan K, Liu Y, Zhang Y, Nathan A, Tian W, Yu J, et al. Mural cell SDF1 signaling is associated with the pathogenesis of pulmonary arterial hypertension. Am J Respir Cell Mol Biol. (2020) 62:747–59. doi: 10.1165/rcmb.2019-0401OC
27. Yuan K, Orcholski ME, Panaroni C, Shuffle EM, Huang NF, Jiang X, et al. Activation of the Wnt/planar cell polarity pathway is required for pericyte recruitment during pulmonary angiogenesis. Am J Pathol. (2015) 185:69–84. doi: 10.1016/j.ajpath.2014.09.013
28. Zehendner CM, Valasarajan C, Werner A, Boeckel JN, Bischoff FC, John D, et al. Long noncoding RNA TYKRIL plays a role in pulmonary hypertension via the p53-mediated regulation of PDGFRbeta. Am J Respir Crit Care Med. (2020) 202:1445–57. doi: 10.1164/rccm.201910-2041OC
29. Banks CA, Boanca G, Lee ZT, Eubanks CG, Hattem GL, Peak A, et al. TNIP2 is a hub protein in the NF-kappaB network with both protein and RNA mediated interactions. Mol Cell Proteomics. (2016) 15:3435–49. doi: 10.1074/mcp.M116.060509
30. Pomerantz JL, Baltimore D. NF-kappaB activation by a signaling complex containing TRAF2, TANK and TBK1, a novel IKK-related kinase. EMBO J. (1999) 18:6694–704. doi: 10.1093/emboj/18.23.6694
31. Szklarczyk D, Gable AL, Lyon D, Junge A, Wyder S, Huerta-Cepas J, et al. STRING v11: protein-protein association networks with increased coverage, supporting functional discovery in genome-wide experimental datasets. Nucleic Acids Res. (2019) 47:D607–13. doi: 10.1093/nar/gky1131
32. Feng X, Gaeta ML, Madge LA, Yang JH, Bradley JR, Pober JS. Caveolin-1 associates with TRAF2 to form a complex that is recruited to tumor necrosis factor receptors. J Biol Chem. (2001) 276:8341–9. doi: 10.1074/jbc.M007116200
33. Legler DF, Micheau O, Doucey MA, Tschopp J, Bron C. Recruitment of TNF receptor 1 to lipid rafts is essential for TNFalpha-mediated NF-kappaB activation. Immunity. (2003) 18:655–64. doi: 10.1016/S1074-7613(03)00092-X
34. Liu T, Zhang L, Joo D, Sun SC. NF-kappaB signaling in inflammation. Signal Transduct Target Ther. (2017) 2:17023. doi: 10.1038/sigtrans.2017.23
35. Karin M. NF-kappaB as a critical link between inflammation and cancer. Cold Spring Harb Perspect Biol. (2009) 1:a000141. doi: 10.1101/cshperspect.a000141
36. Ricard N, Tu L, Le Hiress M, Huertas A, Phan C, Thuillet R, et al. Increased pericyte coverage mediated by endothelial-derived fibroblast growth factor-2 and interleukin-6 is a source of smooth muscle-like cells in pulmonary hypertension. Circulation. (2014) 129:1586–97. doi: 10.1161/CIRCULATIONAHA.113.007469
37. Guijarro-Munoz I, Compte M, Alvarez-Cienfuegos A, Alvarez-Vallina L, Sanz L. Lipopolysaccharide activates Toll-like receptor 4 (TLR4)-mediated NF-kappaB signaling pathway and proinflammatory response in human pericytes. J Biol Chem. (2014) 289:2457–68. doi: 10.1074/jbc.M113.521161
38. Roberts KE, McElroy JJ, Wong WP, Yen E, Widlitz A, Barst RJ, et al. BMPR2 mutations in pulmonary arterial hypertension with congenital heart disease. Eur Respir J. (2004) 24:371–4. doi: 10.1183/09031936.04.00018604
39. Liu D, Liu QQ, Guan LH, Jiang X, Zhou DX, Beghetti M, et al. BMPR2 mutation is a potential predisposing genetic risk factor for congenital heart disease associated pulmonary vascular disease. Int J Cardiol. (2016) 211:132–6. doi: 10.1016/j.ijcard.2016.02.150
40. Eyries M, Montani D, Girerd B, Favrolt N, Riou M, Faivre L, et al. Familial pulmonary arterial hypertension by KDR heterozygous loss of function. Eur Respir J. (2020) 55:1902165. doi: 10.1183/13993003.02165-2019
41. Zhu N, Welch CL, Wang J, Allen PM, Gonzaga-Jauregui C, Ma L, et al. Rare variants in SOX17 are associated with pulmonary arterial hypertension with congenital heart disease. Genome Med. (2018) 10:56. doi: 10.1186/s13073-018-0566-x
42. Graf S, Haimel M, Bleda M, Hadinnapola C, Southgate L, Li W, et al. Morrell, identification of rare sequence variation underlying heritable pulmonary arterial hypertension. Nat Commun. (2018) 9:1416. doi: 10.1038/s41467-018-03672-4
43. Rhodes CJ, Batai K, Bleda M, Haimel M, Southgate L, Germain M, et al. Genetic determinants of risk in pulmonary arterial hypertension: international genome-wide association studies and meta-analysis. Lancet Respir Med. (2019) 7:227–38. doi: 10.1016/S2213-2600(18)30409-0
44. Wipff J, Kahan A, Hachulla E, Sibilia J, Cabane J, Meyer O, et al. Association between an endoglin gene polymorphism and systemic sclerosis-related pulmonary arterial hypertension. Rheumatology. (2007) 46:622–5. doi: 10.1093/rheumatology/kel378
45. Al-Naamani N, Krowka MJ, Forde KA, Krok KL, Feng R, Heresi GA, et al. Pulmonary vascular complications of liver disease study, estrogen signaling and portopulmonary hypertension: the pulmonary vascular complications of liver disease study (PVCLD2). Hepatology. (2020) 73:726–37. doi: 10.1002/hep.31314
46. Xiong M, Wang J, Guo M, Zhou Q, Lu W. TRPM8 genetic variations associated with COPD risk in the Chinese Han population. Int J Chron Obstruct Pulmon Dis. (2016) 11:2563–71. doi: 10.2147/COPD.S109026
47. Li M, Cai W, Chen Y, Dong L. The CAV1 gene 3' Untranslated region single nucleotide polymorphisms are associated with the risk of pulmonary hypertension in Chinese Han chronic obstructive pulmonary patients. Genet Test Mol Biomarkers. (2019) 23:634–43. doi: 10.1089/gtmb.2019.0053
48. Jin G, Chen Z, Zhang J, Song J, Shi J, Zhou B. Association of brain natriuretic peptide gene polymorphisms with chronic obstructive pulmonary disease complicated with pulmonary hypertension and its mechanism. Biosci Rep. (2018) 38:BSR20180905. doi: 10.1042/BSR20180905
49. Hosokawa S, Haraguchi G, Sasaki A, Arai H, Muto S, Itai A, et al. Pathophysiological roles of nuclear factor kappaB (NF-kB) in pulmonary arterial hypertension: effects of synthetic selective NF-kB inhibitor IMD-0354. Cardiovasc Res. (2013) 99:35–43. doi: 10.1093/cvr/cvt105
50. Farkas D, Alhussaini AA, Kraskauskas D, Kraskauskiene V, Cool CD, Nicolls MR, et al. Nuclear factor kappaB inhibition reduces lung vascular lumen obliteration in severe pulmonary hypertension in rats. Am J Respir Cell Mol Biol. (2014) 51:413–25. doi: 10.1165/rcmb.2013-0355OC
51. Price LC, Caramori G, Perros F, Meng C, Gambaryan N, Dorfmuller P, et al. Nuclear factor κ-B is activated in the pulmonary vessels of patients with end-stage idiopathic pulmonary arterial hypertension. PLoS ONE. (2013) 10:e75415. doi: 10.1371/journal.pone.0075415
52. Sawada H, Mitani Y, Maruyama J, Jiang BH, Ikeyama Y, Dida FA, et al. A nuclear factor-kappaB inhibitor pyrrolidine dithiocarbamate ameliorates pulmonary hypertension in rats. Chest. (2007) 4:1265–74. doi: 10.1378/chest.06-2243
53. Pang Y, Liang MT, Gong Y, Yang Y, Bu PL, Zhang M, et al. HGF reduces disease severity and inflammation by attenuating the NF-κB signaling in a rat model of pulmonary artery hypertension. Inflammation. (2018) 3:924–31. doi: 10.1007/s10753-018-0747-1
54. Han B, Copeland CA, Kawano Y, Rosenzweig EB, Austin ED, Shahmirzadi L, et al. Characterization of a caveolin-1 mutation associated with both pulmonary arterial hypertension and congenital generalized lipodystrophy. Traffic. (2016) 17:1297–312. doi: 10.1111/tra.12452
55. Grimes T, Potter SS, Datta S. Integrating gene regulatory pathways into differential network analysis of gene expression data. Sci Rep. (2019) 9:5479. doi: 10.1038/s41598-019-41918-3
56. Rabinovitch M, Guignabert C, Humbert M, Nicolls MR. Inflammation and immunity in the pathogenesis of pulmonary arterial hypertension. Circulat Res. (2014) 115:165–75. doi: 10.1161/CIRCRESAHA.113.301141
57. Trankle CR, Canada JM, Kadariya D, Markley R, De Chazal HM, Pinson J, et al. IL-1 blockade reduces inflammation in pulmonary arterial hypertension and right ventricular failure: a single-arm, open-label, phase IB/II pilot study. Am J Respir Crit Care Med. (2019) 199:381–4. doi: 10.1164/rccm.201809-1631LE
58. Hernandez-Sanchez J, Harlow L, Church C, Gaine S, Knightbridge E, Bunclark K, et al. Clinical trial protocol for TRANSFORM-UK: a therapeutic open-label study of tocilizumab in the treatment of pulmonary arterial hypertension. Pulm Circ. (2018) 8:2045893217735820. doi: 10.1177/2045893217735820
59. Besson B, Sonthonnax F, Duchateau M, Ben Khalifa Y, Larrous F, Eun H, et al. Regulation of NF-kappaB by the p105-ABIN2-TPL2 complex and RelAp43 during rabies virus infection. PLoS Pathog. (2017) 13:e1006697. doi: 10.1371/journal.ppat.1006697
60. Nanda SK, Nagamori T, Windheim M, Amu S, Aviello G, Patterson-Kane J, et al. ABIN2 function is required to suppress DSS-induced colitis by a Tpl2-independent mechanism. J Immunol. (2018) 201:3373–82. doi: 10.4049/jimmunol.1700614
61. Papoutsopoulou S, Symons A, Tharmalingham T, Belich MP, Kaiser F, Kioussis D, et al. ABIN-2 is required for optimal activation of Erk MAP kinase in innate immune responses. Nat Immunol. (2006) 7:606–15. doi: 10.1038/ni1334
62. Etemadi N, Chopin M, Anderton H, Tanzer MC, Rickard JA, Abeysekera W, et al. TRAF2 regulates TNF and NF-kappaB signalling to suppress apoptosis and skin inflammation independently of Sphingosine kinase 1. Elife. (2015) 4:e10592. doi: 10.7554/eLife.10592.018
63. Grech AP, Amesbury M, Chan T, Gardam S, Basten A, Brink R. TRAF2 differentially regulates the canonical and noncanonical pathways of NF-kappaB activation in mature B cells. Immunity. (2004) 21:629–42. doi: 10.1016/j.immuni.2004.09.011
64. Van Quickelberghe E, De Sutter D, van Loo G, Eyckerman S, Gevaert K. A protein-protein interaction map of the TNF-induced NF-kappaB signal transduction pathway. Sci Data. (2018) 5:180289. doi: 10.1038/sdata.2018.289
65. Boucherat O, Vitry G, Trinh I, Paulin R, Provencher S, Bonnet S. The cancer theory of pulmonary arterial hypertension. Pulm Circ. (2017) 2:285–99. doi: 10.1177/2045893217701438
66. Zhu S, Jin J, Gokhale S, Lu AM, Shan H, Feng J, et al. Genetic alterations of TRAF proteins in human cancers. Front Immunol. (2018) 9:2111. doi: 10.3389/fimmu.2018.02111
67. Shen RR, Zhou AY, Kim E, O'Connell JT, Hagerstrand D, Beroukhim R, et al. TRAF2 is an NF-κB-activating oncogene in epithelial cancers. Oncogene. (2015) 2:209–16. doi: 10.1038/onc.2013.543
68. Peramuhendige P, Marino S, Bishop RT, de Ridder D, Khogeer A, Baldini I, et al. TRAF2 in osteotropic breast cancer cells enhances skeletal tumour growth and promotes osteolysis. Sci Rep. (2018) 1:39. doi: 10.1038/s41598-017-18327-5
69. Peng C, Zhu F, Wen W, Yao K, Li S, Zykova T, et al. Tumor necrosis factor receptor-associated factor family protein 2 is a key mediator of the epidermal growth factor-induced ribosomal S6 kinase 2/cAMP-responsive element-binding protein/Fos protein signaling pathway. J Biol Chem. (2012) 287:25881–92. doi: 10.1074/jbc.M112.359521
Keywords: pulmonary arterial hypertension, NF-κB, inflammation, massive paralleled sequencing, TNIP2, TRAF2
Citation: Pienkos S, Gallego N, Condon DF, Cruz-Utrilla A, Ochoa N, Nevado J, Arias P, Agarwal S, Patel H, Chakraborty A, Lapunzina P, Escribano P, Tenorio-Castaño J and de Jesús Pérez VA (2021) Novel TNIP2 and TRAF2 Variants Are Implicated in the Pathogenesis of Pulmonary Arterial Hypertension. Front. Med. 8:625763. doi: 10.3389/fmed.2021.625763
Received: 03 November 2020; Accepted: 23 March 2021;
Published: 30 April 2021.
Edited by:
Silvia Ulrich, University Hospital Zürich, SwitzerlandReviewed by:
Vasile Foris, Medical University of Graz, AustriaGabriele Grunig, New York University, United States
Copyright © 2021 Pienkos, Gallego, Condon, Cruz-Utrilla, Ochoa, Nevado, Arias, Agarwal, Patel, Chakraborty, Lapunzina, Escribano, Tenorio-Castaño and de Jesús Pérez. This is an open-access article distributed under the terms of the Creative Commons Attribution License (CC BY). The use, distribution or reproduction in other forums is permitted, provided the original author(s) and the copyright owner(s) are credited and that the original publication in this journal is cited, in accordance with accepted academic practice. No use, distribution or reproduction is permitted which does not comply with these terms.
*Correspondence: Vinicio A. de Jesús Pérez, dmRlamVzdXNAc3RhbmZvcmQuZWR1; Jair Tenorio-Castaño, amFpcmEudGVub3Jpb0BzYWx1ZC5tYWRyaWQub3Jn
†These authors have contributed equally to this work