- 1State Key Laboratory for Managing Biotic and Chemical Threats to the Quality and Safety of Agro-Products, Institute of Agro-Product Safety and Nutrition, Zhejiang Academy of Agricultural Sciences, Hangzhou, China
- 2College of Plant Science and Technology, Huazhong Agricultural University, Wuhan, China
- 3Department of Plant Pathology, College of Agriculture, University of Sargodha, Sargodha, Pakistan
- 4Department of Plant Pathology, Faculty of Agriculture and Environment, The Islamia University of Bahawalpur, Bahawalpur, Pakistan
- 5Plant Cytogenetics and Molecular Biology Group, Institute of Biology, Biotechnology and Environmental Protection, Faculty of Natural Sciences, University of Silesia in Katowice, Katowice, Poland
- 6Department of Biotechnology Engineering, Institute of Engineering and Technology, Bundelkhand University, Jhansi, India
- 7Institute of Food Science and Technology, Chinese Academy of Agricultural Sciences, Beijing, China
- 8Fitosanidad-Fitopatología, Colegio de Postgraduados, Montecillo, Mexico
- 9Department of Plant Production, Faculty of Agronomy, Universidad de Concepción, Chillán, Chile
Rhizoctonia solani is a pathogen that causes considerable harm to plants worldwide. In the absence of hosts, R. solani survives in the soil by forming sclerotia, and management methods, such as cultivar breeding, crop rotations, and fungicide sprays, are insufficient and/or inefficient in controlling R. solani. One of the most challenging problems facing agriculture in the twenty-first century besides with the impact of global warming. Environmentally friendly techniques of crop production and improved agricultural practices are essential for long-term food security. Trichoderma spp. could serve as an excellent example of a model fungus to enhance crop productivity in a sustainable way. Among biocontrol mechanisms, mycoparasitism, competition, and antibiosis are the fundamental mechanisms by which Trichoderma spp. defend against R. solani, thereby preventing or obstructing its proliferation. Additionally, Trichoderma spp. induce a mixed induced systemic resistance (ISR) or systemic acquired resistance (SAR) in plants against R. solani, known as Trichoderma-ISR. Stimulation of every biocontrol mechanism involves Trichoderma spp. genes responsible for encoding secondary metabolites, siderophores, signaling molecules, enzymes for cell wall degradation, and plant growth regulators. Rhizoctonia solani biological control through genes of Trichoderma spp. is summarized in this paper. It also gives information on the Trichoderma-ISR in plants against R. solani. Nonetheless, fast-paced current research on Trichoderma spp. is required to properly utilize their true potential against diseases caused by R. solani.
Introduction
Trichoderma spp. (teleomorph: Hypocrea) is a saprotrophic fungus. They may survive in a variety of settings, including soil, wood, bark, other fungus, and many more, demonstrating their adaptability and opportunistic potential (Jaklitsch, 2009; Brotman et al., 2010; Druzhinina et al., 2011; Oszust et al., 2020; Vinale and Sivasithamparam, 2020; Yu et al., 2022). Rhizoctonia solani J.G. Kühn [teleomorph: Thanatephorus cucumeris (A.B. Frank) Donk], is a soil-borne pathogen with a necrotrophic lifestyle that lives in soil by developing a resistant survival structure known as sclerotia (Mayo et al., 2015). This fungus is a species complex that causes significant harm to numerous economically important agricultural, horticultural, pasture crops, turf grasses, and forest and fruit trees worldwide (Ajayi-Oyetunde and Bradley, 2018). It caused sheath blight in corn (Li et al., 1998) and rice (Abbas et al., 2021), seed, stem, collar, root, and hypocotyl rot and damping off in soybean, tomatoes, eggplant, pepper, lettuce, and zinnia (Ohkura et al., 2009) and stem canker and black scurf in potatoes (Das et al., 2014). In addition, the fungus caused root, stem, root, crown, and hypocotyl rot and blights of legumes and cotton (Nerey et al., 2010), grey leaf spot, and brown patch of turf grasses (Dong et al., 2008). Geographical distribution of R. solani has been shown in Figure 1. Chemical fungicides are widely used to control this disease, as no resistance resources have yet been discovered in available rice germplasm. Moreover, the species complex of R. solani is composed of various 14 anastomosis groups (AGs; AG1-13 and AG-B1), having wide genetic diversity, broad host compatibility, and ability to survive from one crop season to the next by forming dormant sclerotia made disease control even more difficult (Patil and Solanki, 2016; Abbas et al., 2021). Furthermore, agriculture confronts enormous challenges in providing enough food in a sustainable manner for an ever-increasing worldwide population while simultaneously dealing with unpredictable global environmental changes. As a result, there is an increased demand for ecologically friendly solutions that may assist plants in performing well in a range of conditions. In this regard, Trichoderma spp. might serve as a model fungus for sustaining agricultural output (Abdel-lateif, 2017; Singh et al., 2021). Many studies have indicated that biological management with the genus Trichoderma effectively controls R. solani (Solanki et al., 2011; Kashyap et al., 2020). For instance, in several countries, T. harzianum and T. asperellum were employed to prevent damping off, root, and crown rots (Herrera et al., 2020; Khadka and Miller, 2021). Similarly, there is a widespread use of T. harzianum to suppress black scurf, sheath blight, and stem canker in potatoes throughout the world (Wilson et al., 2008; de França et al., 2015; Naeimi et al., 2020). Trichoderma spp. can parasitize and compete with R. solani for nutrients, rhizosphere, and root colonization (Guzmán-Guzmán et al., 2017; Vinale and Sivasithamparam, 2020; Segreto et al., 2021; Yu et al., 2022). They can also compete for seed exudates, which stimulate the development of R. solani propagules in the soil (Nawrocka et al., 2018). Furthermore, Trichoderma spp. are prolific makers of secondary metabolites, such as peptaibols, pyrones, non-ribosomal peptides (NRPs), polyketides and terpenoids, and siderophores, when grown with R. solani (Manganiello et al., 2018; Halifu et al., 2020). Furthermore, they block or degrade pectinases and other enzymes required for R. solani development (Kullnig et al., 2000; Halifu et al., 2020). Furthermore, by colonizing the rhizospheres of plants, they drive plant development and defense responses against R. solani (Nawrocka et al., 2018; Zhang and Zhuang, 2020). Furthermore, Trichoderma spp. can activate plant se mechanisms, resulting in ISR, SAR, and, according to a new research, Trichoderma-induced systemic resistance (TISR; Leonetti et al., 2017). Application of Trichoderma spp. led to activation of defense signaling involving SA and/or JA/ET pathways against R. solani, hence increasing plant resistance. Recent research has produced a wealth of knowledge on the discovery and cloning of several genes involved in mycoparasitism, resistance, antibiosis, and competition induction in plants against R. solani (Druzhinina et al., 2011; Rahimi Tamandegani et al., 2020; Zhang and Zhuang, 2020). Numerous reviews explain the role of Trichoderma spp. genes against many plant pathogens (Harman et al., 2004; Zeilinger and Omann, 2007; Druzhinina et al., 2011; Nawrocka and Małolepsza, 2013; Daguerre et al., 2014; Sarma et al., 2014; Contreras-Cornejo et al., 2016; Guzmán-Guzmán et al., 2019). This article examines Trichoderma spp. genes that encode proteins linked with antibacterial action against R. solani and resistance induction in plants. The function of Trichoderma spp. genes in competition for root colonization, mycoparasitism, antibiosis, rhizosphere and nutrients, and stimulation of plant defensive mechanisms against R. solani is depicted in Figure 2. The following parts have been included in the review: (1) Trichoderma spp. biology, (2) genes involved in mycoparasitism, (3) genes involved in competition, (4) induced resistance in various plants against R. solani, and (5) resistance to R. solani in plants is mediated by biochemical changes associated to Trichoderma-induced defensive responses.
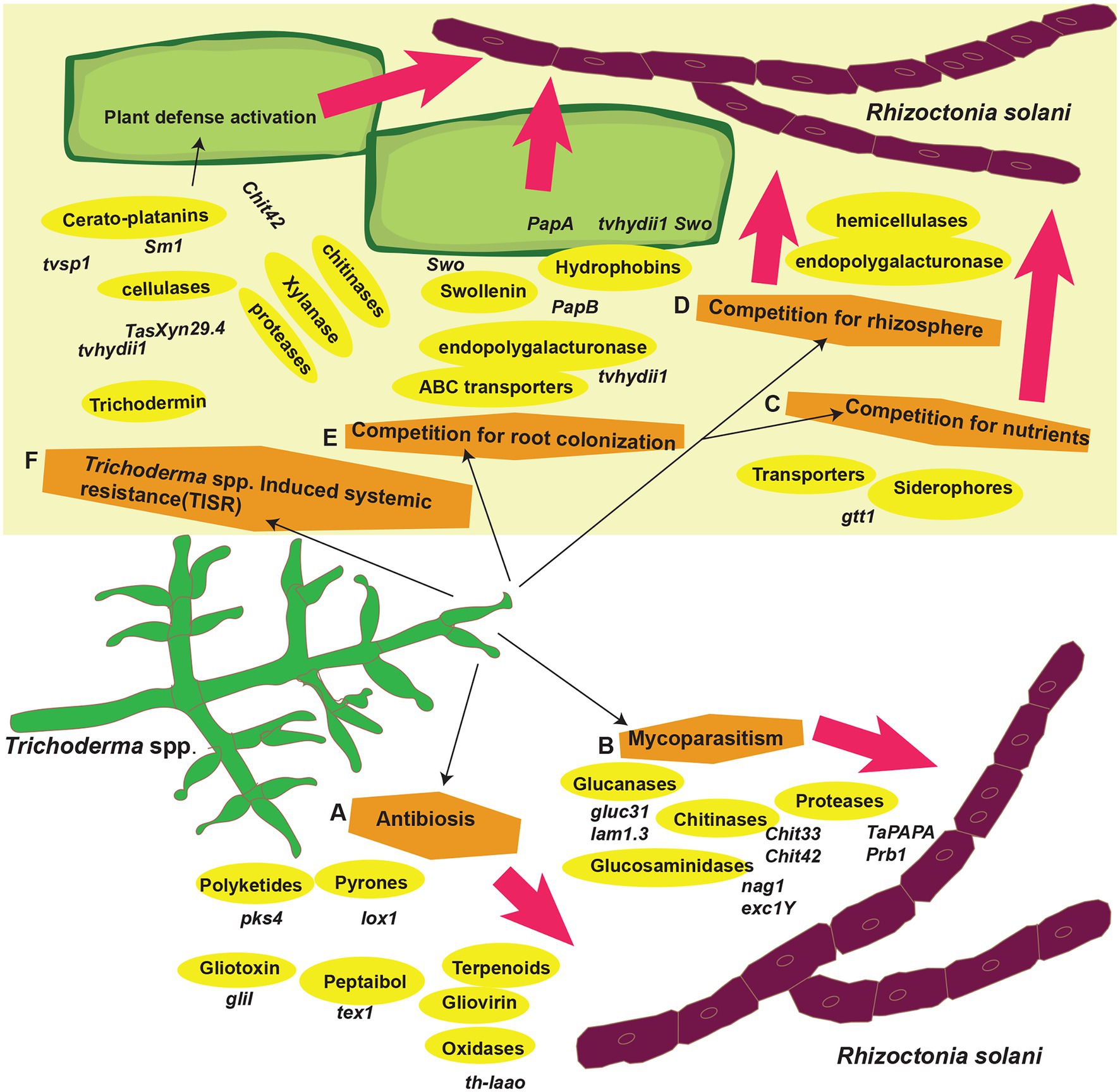
Figure 2. Trichoderma spp. biocontrol mechanisms against R. solani. (A) Trichoderma spp. genes in antibiosis, (B) mycoparasitism, (C–E) competition for root colonization, rhizosphere and nutrients, and (F) induced systemic resistance.
Biology of Trichoderma spp.
Trichoderma spp. exist in two separate morphological and physiological stages. Hypocrea is the sexual (teleomorphic) stage, while Trichoderma is the asexual (anamorphic or mitosporic) stage (Rahimi Tamandegani et al., 2020; Zhang and Zhuang, 2020). Trichoderma spp. that can no longer reproduce sexually are known as “agamospecies,” but sexual forms make up the majority of the genus’ genetic diversity. There is an average of 101–103 culturable Trichoderma spp. per gram of temperate and tropical soils (Harman et al., 2004; Zeilinger and Omann, 2007; Druzhinina et al., 2011; Nawrocka and Małolepsza, 2013; Daguerre et al., 2014; Sarma et al., 2014; Morán-Diez et al., 2021). These fungi also colonize plant materials, such as wood and herbaceous plants, where the sexual genus Hypocrea is most commonly seen. The majority of biocontrol strains lack a documented sexual stage (Druzhinina et al., 2011; Daguerre et al., 2014). Asexual fungi are clonal, frequently heterokaryotic individuals and communities that most likely evolved separately during the asexual stage (Contreras-Cornejo et al., 2016). They have a lot of genetic diversity and can make various commercial and ecologically valuable goods. They are prolific extracellular protein makers, well known for producing enzymes that digest cellulose and chitin (Harman et al., 2004; Druzhinina et al., 2011; Daguerre et al., 2014). Distinct strains, for example, produce over 100 different compounds with recognized antibiotic properties. Trichoderma spp. has long been known for suppressing plant disease and promoting plant growth and development. In horticulture, they are getting increasingly popular because of their “rhizosphere competence” and can colonize and develop near plant roots. Much of the known biology of these fungi and many of their uses have only lately been documented. These fungi’s biology is being considerably revised because many new species are being recognized. Most of the distinctive species in the genus Trichoderma were difficult to distinguish morphologically. As a result, a polyphasic approach is utilized to discover the characteristics of a novel species by combining the results of numerous techniques, such as molecular, morphological, genomic, and physiological study (Badaluddin et al., 2018). For example, one of the most common genetic methods for identifying Trichoderma spp. is multi-gene phylogeny. Currently, the combination of multi-gene phylogeny and morphological features is employed to identify the species level description of Trichoderma. Both morphological and molecular analysis employing genes including rpb2, cal, act, tef1, and ITS were used to identify Trichoderma spp. strains. The many functional groups within Trichoderma spp. that are important for secondary metabolite production were also identified utilizing a combination of novel genomic approaches and physiological activities (Zeilinger et al., 2016; Ali et al., 2021). These integrated methodologies contributed to the need to identify Trichoderma strains as biological control agents (Mahr, 2021). Furthermore, genomic investigations of Trichoderma spp. have highlighted the fungal kingdom’s genetic variety as well as distinctions in shape, physiology, and ecology (Inglis et al., 2018). Because of advancements in high-throughput sequencing technology, the number of available fungal genome data is quickly increasing. Recently, the genomes of the most common Trichoderma spp. were compared in an attempt to better understand Trichoderma biology (Chung et al., 2021).
Trichoderma spp. Genes Involved in Mycoparasitism
The combination of Trichoderma spp. with other fungi is referred to as necrotrophic hyperparasitism or mycoparasitism. Many studies showed that Trichoderma spp. exhibited mycoparasitic capacity against R. solani (Geremia et al., 1993; De La Cruz et al., 1995; Lorito et al., 1998; Kubicek et al., 2011; Dubey et al., 2021). Trichoderma spp. on the other hand, feeds on fungal biomass; hence, classified as mycotrophic to encompass both saprotrophic and biotrophic feeding methods. Antibiosis, mycoparasitism, nutrient competition, rhizosphere and root colonization, and activation of plant defense mechanisms are all used by Trichoderma spp. to battle pathogenic fungus (Rai et al., 2019; Morán-Diez et al., 2021; Sánchez-Cruz et al., 2021; Segreto et al., 2021; Yu et al., 2022). Trichoderma spp. specifically detect and establish an antagonistic relationship with R. solani. Finally, they kill or control R. solani by genetic reprogramming of their gene expression. These two processes are crucial because they impact the type and degree of the Trichoderma spp. hostile behavior against R. solani (Yu et al., 2022).
Chemotropism and Recognition of Prey
The initial phase in mycoparasitism is Trichoderma spp. detecting or identifying R. solani as prey. Trichoderma spp. recognize the oligopeptide and oligosaccharide compounds produced by R. solani in reaction to hydrolytic enzymes, such as proteases and chitinases (Druzhinina et al., 2011; Sood et al., 2020). A signaling cascade is activated when these molecules attach to receptors on Trichoderma spp. hyphae. This causes transcription factors to be activated, which govern production of secondary metabolite (SM) production and lysis of cell wall (Harman et al., 2004; Druzhinina et al., 2011; Table 1). Many Trichoderma spp. express genes encoding proteases and oligopeptide transporters before and during interaction with R. solani, according to a recent study. The bulk of proteases are subtilisin-like serine proteases, and genes for these enzymes may be found in abundance in expressed sequence tags (ESTs). For example, a review of the ESTs collected at the start of the T. atroviridis-R. solani interaction revealed many genes that produce subtilisin-like serine proteases. The prb1 gene encodes these proteases, and overexpression of these proteases boosted mycoparasitic activity. The action of these proteases on R. solani may result in the release of oligopeptide molecules that bind to receptors on Trichoderma spp. Using T. atroviride EST libraries, a preliminary transcriptome research revealed considerable alterations in T. atroviride gene expression, including changes that resembled a response to nitrogen restriction, lipid metabolism adjustments, and signaling changes (Seidl et al., 2009; Kaur et al., 2021).
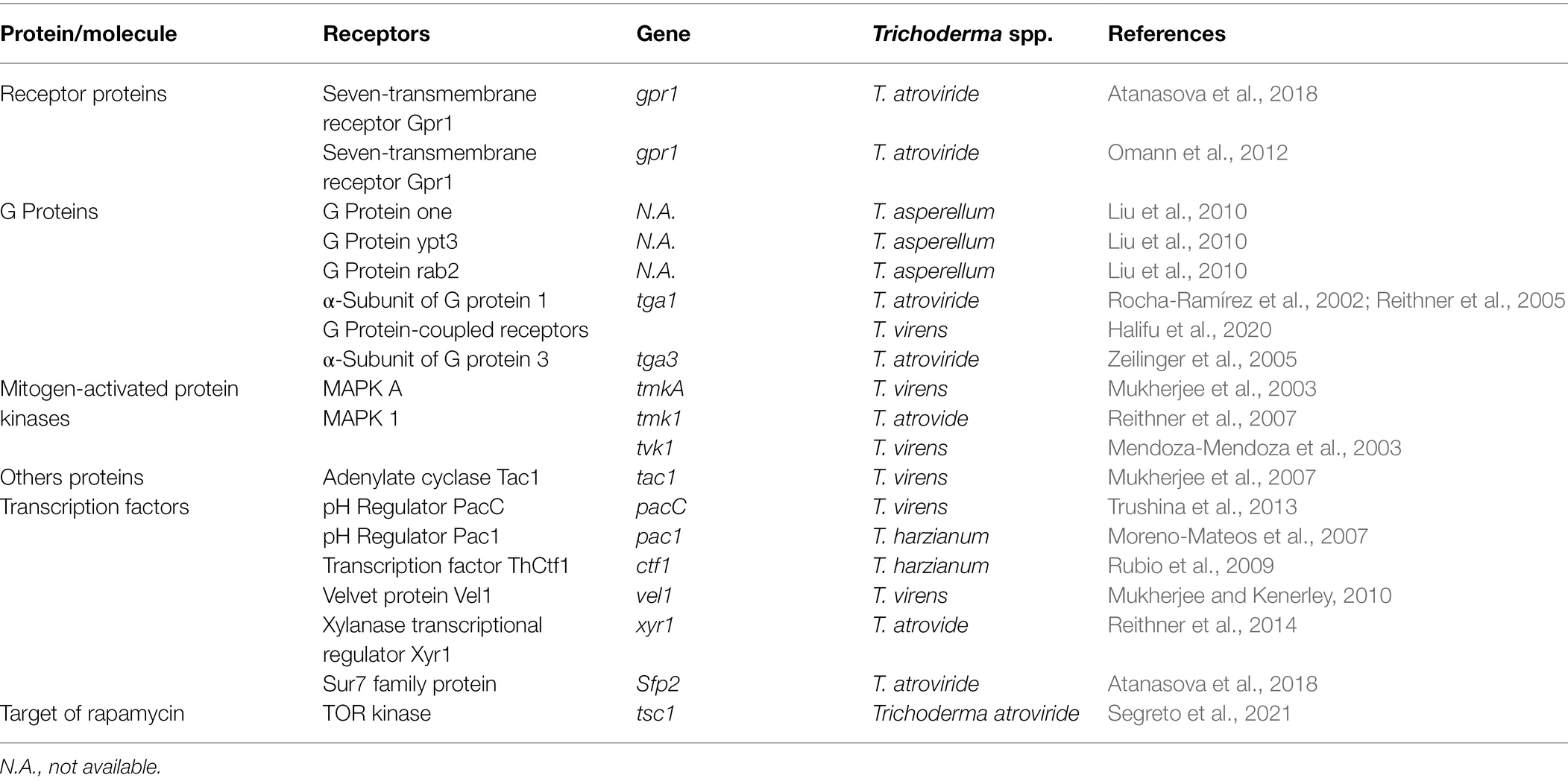
Table 1. Role of Trichoderma spp. genes involved in recognition of R. solani and signal transduction.
G Protein-Coupled Receptors
G protein-coupled receptors (GPCRs) of biocontrol agents act as a sensor for the oligopeptides secreted by plant pathogens (Daguerre et al., 2014). They are the most frequent cell surface receptors for detecting environmental signals at the plasma membrane. These receptors sense ligands, such as nutrients, oligopeptides, sex pheromones, oxylipins. They frequently use heterotrimeric G proteins to connect with downstream signaling pathways including the mitogen-activated protein kinase (MAPK) and cAMP-protein kinase A (PKA) cascades (Table 1; Figure 3). In response to R. solani, several GPCR-encoding genes were expressed in Trichoderma spp. showing that GPCRs play a role in detecting and triggering the mycoparasitic response. The three primary components of heterotrimeric G protein signaling are a GPCR, a heterotrimeric G protein (made up of G and G subunits), and an effector. G protein activation (exchange of GDP for GTP on the G subunit) and dissociation of the GTP-bound subunit from the associated dimer occur as a result of the ligand contact, allowing both units to govern downstream effectors. In fungus, the cAMP-PKA pathway and MAPK cascades are typical effectors of heterotrimeric G protein. Both routes are substantially conserved and linked. Transmembrane G protein-coupled receptor signals are channeled by heterotrimeric G proteins to various intracellular destinations via activating effectors, such as adenylate cyclase or the MAPK cascades (Kaziro et al., 1991; Daguerre et al., 2014; Segreto et al., 2021). Trichoderma spp. require G proteins, GPCRs, and adenylate cyclase receptors to synthesize external cell wall lytic enzymes, release antifungal chemicals, and produce infection structures (Figure 3). Trichoderma hyphae were prevented from connecting to R. solani cell surfaces by inhibiting the gene encoding the T. atroviride seven-transmembrane receptor Gpr1, as well as upregulation of two chitinase genes (nag1 and ech42) and the protease gene prb1. Due to the importance of these genes in mycoparasitism, their downregulation in T. atroviride results in pathogen survival (Omann et al., 2012). Two G protein subunits identified in T. atroviride are Tga1 and Tga3. During direct fight with R. solani, the tga1 mutant completely lost its mycoparasitic activity (Reithner et al., 2011). Infection structure development remained steady, while production of 6-pentyl-pyrone and sesquiterpene-derived antifungal metabolites reduced (Rocha-Ramírez et al., 2002; Reithner et al., 2011). Similarly, when addressed directly, the tga3 mutant was unable to build infection structures or mycoparasitize R. solani (Zeilinger et al., 1999). In fungus, the MAPK pathways are well-known signal transduction systems (Schmoll et al., 2016). Trichoderma’s genome contains genes for three pathogenicity MAPKs: (1) TmKA (also known as Tvk1 and Tmk1), (2) cell integrity kinase (TmkB), and (3) osmoregulatory MAPK (Hog1; Schmoll et al., 2016). TmkA gene mutation in T. virens strain “P” had no effect on biocontrol efficacy against R. solani (Zeilinger et al., 1999; Viterbo et al., 2004; Mukherjee et al., 2011, 2012). However, deletion of the same gene TmkA in the T. virens strain “Q” drastically lowered the Trichoderma’s biocontrol efficacy against R. solani (Mendoza-Mendoza et al., 2003). Trichoderma virens tvk1 mutants secreted more lytic enzymes and were far more efficient in disease control than the wild-type strain (Mukherjee et al., 2003). Trichoderma atroviride tmk1 mutants displayed decreased mycoparasitism activity against R. solani in direct mycoparasite–host interactions, as well as against R. solani specific control of ech42 gene transcription (Reithner et al., 2011). Furthermore, deletion of a Tmk1 homologue in T. atroviridis resulted in decreased mycoparasitic activity against R. solani, as well as increased synthesis of chitinase and other antifungal chemicals (Reithner et al., 2011).
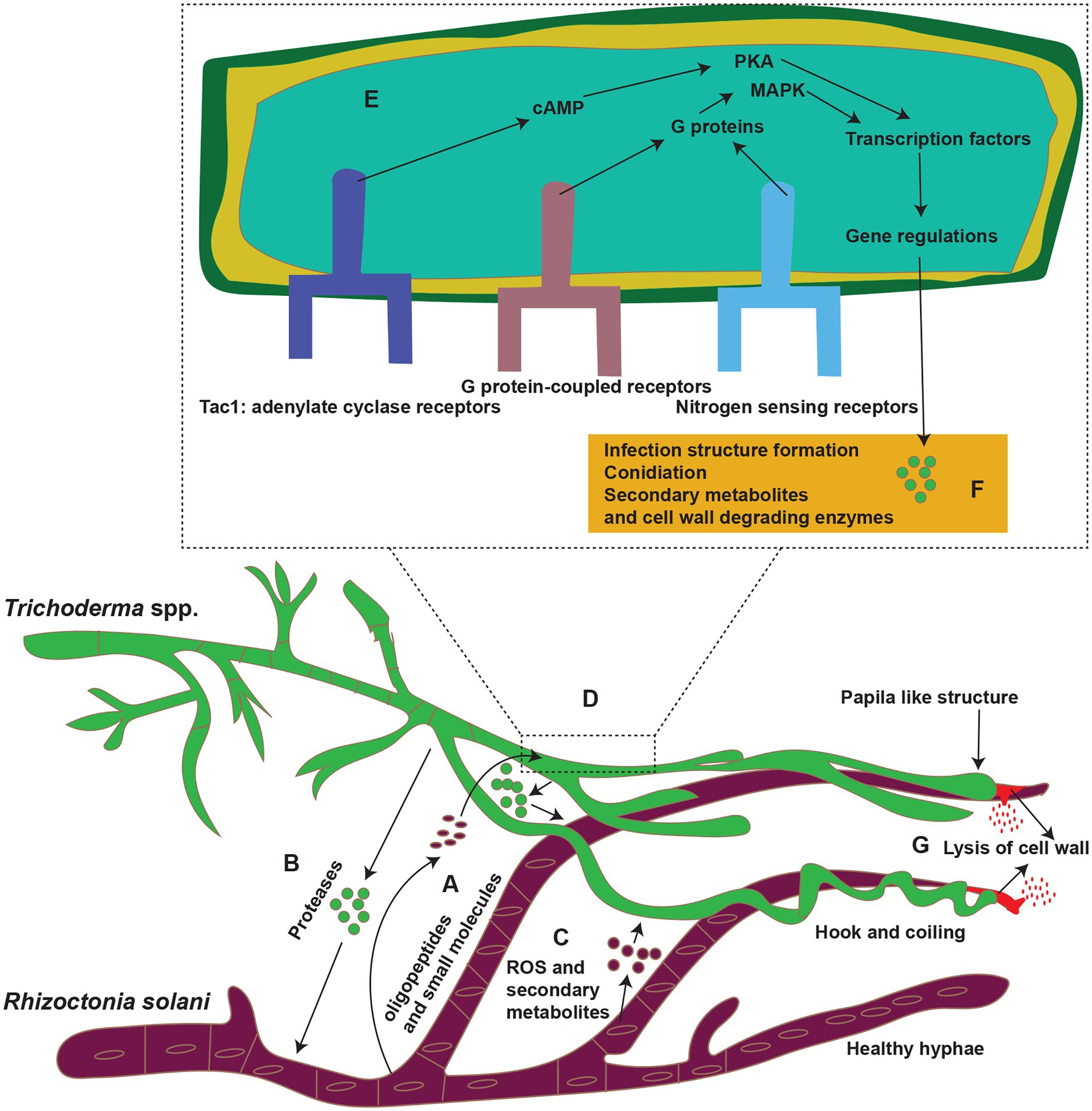
Figure 3. Mycoparasitism; Trichoderma spp. (Green color) parasitize R. solani (purple) in soil. (A–C) Trichoderma spp. recognized R. solani by tiny molecules (oligopeptides and small other molecules); some of these molecules are peptides released by the action of proteases of Trichoderma spp. prior to contact. Also R. solani secrete ROS and secondary metabolites in response to Trichoderma spp. (D) These molecules bind to G protein-coupled receptors (GPCRs; such as Gpr1) or nitrogen-sensing receptors (Target of rapamycin; TOR pathway), or adenylate cyclase receptors on the surface of Trichoderma spp. hyphae. (E) After binding to the receptors, the molecules induce a signaling cascade involving G proteins and mitogen-activated protein kinases (MAPKs) or protein kinases (PKA), which then modulate the activities of transcription factors (TFs) and gene regulations. (F,G) These substances then boost the expression of genes that code for enzymes involved in secondary metabolite production and lysis of the cell wall of R. solani. Reconstructed from Druzhinina et al. (2011).
Cyclic Adenosine Monophosphate Receptors
In addition to, GPCRs, another importance receptors for signaling transduction are Cyclic adenosine monophosphate (cAMP) receptors. They are involved in the growth, condition, development, and biocontrol efficiency in Trichoderma spp. (Brunner et al., 2008; Table 1; Figure 3). Adenylate cyclase converts ATP to cAMP and is present on the inner side of the plasma membrane and at several sites throughout the fungal cell. Adenylate cyclase is triggered by a multitude of signaling molecules that activate G (Gs) protein-coupled receptors that stimulate adenylate cyclase. cAMP activates a cAMP-dependent protein kinase (PKA) that phosphorylates proteins like transcription factors to regulate gene expression (Dickman and Yarden, 1999). Tac1, an adenylate cyclase gene in T. virens, was deleted, which not only removed biocontrol efficacy against R. solani but also lowered secondary metabolite synthesis (Mukherjee et al., 2007).
Target of Rapamycin Proteins
In addition to cAMP and MAPK, the target of rapamycin (TOR) pathway is a critical regulator of Trichoderma spp. cell proliferation in response to nutrient availability (Table 1; Figure 3). In response to a lack of carbon and nitrogen, this pathway is triggered, resulting in anabolic activities and development (Schmoll et al., 2016). The TOR kinase is inhibited by rapamycin, and nutritional deficiency enhances the expression of genes involved in alternate nitrogen absorption. A growing body of evidence suggests that TOR has a role in nitrogen signaling and pathogenicity-related activities in fungal plant diseases. The Trichoderma genomes also encode single TOR kinase-like the fungal plant pathogens. In a recent study, the activity of TOR1, that is, T. atroviride’s solitary and crucial TOR kinase, was suppressed by chemical TOR inhibitors or genetic alteration. TSC2 and TSC1, which are negative regulators of TOR complex 1 (TORC1) in human cells, resulting in altered nitrogen source-dependent growth of T. atroviride, decreased generation of numerous secondary metabolites, and decreased mycoparasitic overgrowth on R. solani (Segreto et al., 2021). Transcription factors (TFs), which regulate gene transcription at the cellular level during antagonism, are currently understudied (Table 1). Specific motifs in the promoters of Trichoderma spp. biocontrol genes may bind transcription factors involved in nitrogen repression, stress responses, and the regulation of plant cell wall-degrading enzymes. There is no indication, however, that they have a role in antifungal activity. For example, T. atroviride’s xylanase transcriptional regular Xyr1 has a role in mycoparasitism and is essential to trigger the plant defense response (Reithner et al., 2014). When xyr1 was eliminated, R. solani competed more effectively. Plant defense responses were similarly delayed during the T. atroviride/Arabidopsis thaliana interaction.
Attachment and Coiling
Trichoderma spp. coil and generate helix-shaped hyphae around R. solani shortly after recognition, and this phenomenon is dependent on lectin recognition from R. solani’s cell wall. Conversely, plant lectins also cause coiling, demonstrating that lectins do not determine specificity in Trichoderma spp. Additionally, coiling is not always associated with mycoparasitism, as some Trichoderma spp. do exhibit this characteristic. Besides, hyphae of Trichoderma spp. become spiral or helical in shape and are considered diagnostic features in some Trichoderma spp. Trichoderma spp. often precede mycoparasitic attack by growing alongside the host hyphae and forming papilla-like structures. At the places where papilla-like structures form, the cell wall is degraded, and the lumen is penetrated. These papilla-like structures are identical to the appressorium of plant pathogenic fungi and those generated. Recent study suggests the presence of essential components of the cAMP and MAP kinase signaling pathways, such as G protein subunits (G), which govern extracellular enzyme synthesis, antibiotic production, and coil formation surrounding R. solani and T. atroviride expressed the G-gene (tga1) under the control of its promoter or the promoter of the proteinase gene (prb1; Reithner et al., 2005). All mutants showed an increase in coiling. Furthermore, T. viride overexpressing tga1 exhibited a greater capacity to outgrow R. solani. Induction of genes encoding ABC efflux transporters, pleiotropic and multidrug resistance transporters, and detoxification mechanisms (such as those encoding ABC efflux transporters and pleiotropic and multidrug resistance transporters) and detoxification mechanisms (such as those encoding ABC efflux transporters and pleiotropic and multidrug resistance transporters) in the presence of R. solani is a distinctive feature of Trichoderma spp. When R. solani develops sclerotia, it signals with radical oxygen species and excretes antifungal chemicals into the environment. Both radical oxygen species and antifungal drugs have been demonstrated to promote Trichoderma spp. stress’s response. The deletion of one of the genes in T. atroviridis that produces an ABC transporter (Abc2) resulted in poor biocontrol of R. solani, demonstrating that detoxification plays a role in mycoparasitism.
Death of the Fungus
Secondary antifungal metabolites, such as NRPs (peptaibols, gliotoxin, gliovirin, etc.), polyketides, isoprenoid-derived metabolites, pyrones, and cell wall-hydrolytic enzymes or degrading enzymes (CWDEs) eventually kill the prey (Table 2; Figure 3). Trichoderma spp. genomes contain many genes for the synthesis of antifungal metabolites and CWDEs. Trichoderma virens, for example, possess the most non-ribosomal peptide synthesis of any plant pathogenic fungus. The cell wall of any fungus is composed of 30% dry weight chitin, -1,3-glucans, -1,3-glucans, and -1,4-glucans. Cellulases, polygalacturonases (PG), chitinases, glucanases, and proteinase are only a few of the CWDEs found in Trichoderma spp.
Chitinases
Several chitinases enzymes are found in Trichoderma spp., and the list of these enzymes is updated continuously as new enzymes and their associated genes are discovered. Trichoderma spp. produce both endo and exochitinases that belong to the glycosyl hydrolase (GH) family. GH is divided into three groups based on amino acid sequence similarity: GH 18, GH 19, and GH 20 (Kim et al., 2002). Endochitinases break chitin into chitotetraose, chitotriose, and diacetylchitobiose at internal locations. Chitobiosidases and N-acetyl-glucosaminidases were classified further into exochitinases. Chitobiosidases are enzymes that catalyze the stepwise release of diacetylchitobiose. Diacetylchitobiose is broken into N-acetylglucosamine monomers by N-acetylglucosaminidases (Baek et al., 1999). These chitinases degrade chitin polymers by breaking β-1,4 glycosidic linkages in the hyphae of R. solani. Many chitinase-encoding genes have recently been found and reported, and their antagonistic action against R. solani has been tested (Table 2). Trichoderma harzianum and T. atroviride have the most widely explored chitinolytic system among the Trichoderma spp. The biocontrol activity of T. virens transformants overexpressing Cht42 against R. solani in cotton seedling tests was greatly increased as compared to the wild type, as demonstrated by the results of previous investigations (Baek et al., 1999). When the same gene was expressed in other Trichoderma spp. it resulted in higher biocontrol activity against R. solani than when the wild type was used (Howell, 2003). However, in greenhouse biocontrol testing, the activity of chit42 mutants was identical to that of the wild type (Harman et al., 2004). Limón et al. (2004) identified and reported transformants of the biocontrol agent T. harzianum strain CECT 2413 that overexpressed a 33 kDa chitinase (chit33). Under the guidance of the T. reesei pki constitutive promoter, strain CECT 2413 was co-transformed with the amdS gene and its chit33 gene. The transformants were more effective in inhibiting R. solani growth than the wild type (Limón et al., 2004).
Glucanases
In synergistic cooperation with chitinases and secondary metabolites, glucanases have been demonstrated to reduce spore germination or pathogen growth. Glucans are glucose polysaccharides that act as crosslinks between chitin or chitosan polymers. There are two types of glucans, which are distinguished by the chemical link that exists between the glucose subunits. The stiffness of the cell wall is provided by β-glucans, which are made up of -(1,3)- or -(1,6)-linkages. In contrast, α-glucans are made up of -(1,3)- and/or -(1,4)-linkages and serve as a matrix component. Many glucanases with antagonistic activity against R. solani have been isolated from Trichoderma spp. as shown in Table 2. These enzymes degrade glucan polymers in R. solani hyphae by cleaving β-1,3 glycosidic linkage. When the gene bgn13.1 was overexpressed in T. harzianum, it resulted in the greatest suppression of R. solani infection. A higher level of antagonistic activity was seen in the case of the oomycete, P. citrophthora, which has cellulose and glucans as its primary cell wall components, compared to the R. solani, which has chitin and glucan as its primary cell wall components. Many 1,6-glucanases have also been isolated from Trichoderma spp. and have demonstrated antagonistic activity, either alone or in conjunction with chitinases (da Silva Aires et al., 2012). It has recently been shown that T. harzianum strain ALL42 contains a gene that encodes an endo-1,3-glucanase that is related to the GH16 family, and that this gene is involved in the metabolism of glucans. The lack of the gluc31 gene had no effect on the in vivo mycoparasitism capacity of mutant T. harzianum ALL42 against R. solani; however, the removal of the gluc31 gene appeared to have an impact on the structure of the cell wall of T. harzianum ALL42 (Suriani Ribeiro et al., 2019).
Proteases
There are several varieties of fungal proteases (also known as fungal peptidases or proteolytic enzymes) that help in the lysis of cell walls (Mata-Essayag et al., 2001; Haggag et al., 2006). They accelerate the peptide bond breakage in other proteins. Fungal proteases are peptide hydrolases or peptidases that belong to a large number of enzymes that may be divided into endopeptidases and exopeptidases. Several investigations have shown that Trichoderma spp. exopeptidases contribute in the biocontrol of R. solani (Table 2). In addition to breaking down the host cell wall, fungal proteases may function as proteolytic inactivators of pathogen enzymes involved in plant infection (Suárez et al., 2005). Table 2 shows the mycoparasitic protease genes of Trichoderma spp. that have been cloned so far. They also encode aspartic and serine proteases that function in the same way as subtilisin, chymotrypsin/elastase, and trypsin (Pozo et al., 2004; Yang, 2017). Prb1 from T. harzianum IMI 206040 has been shown to play an essential role in biological control, and prb1 transformants increased the biocontrol effectiveness of Trichoderma strains against R. solani by up to fivefold (Flores et al., 1997; Herrera-Estrella, 1997; Cortes et al., 1998; Goldman and Goldman, 1998). Trichoderma harzianum’s protease pra1 has a preference for fungal cell walls. T. virens extracellular serine protease gene (tvsp1) was cloned, and its overexpression dramatically enhanced cotton seedling protection against R. solani (Pozo et al., 2004). Another study found that cold-tolerant T. harzianum strains produced chitinases, glucosidases, trypsin-like, and chymotrypsin-like proteases that were active at low temperatures (Antal et al., 2000; Szekeres et al., 2004). Furthermore, it was shown that mutants obtained by UV irradiation produced substantially more proteases. Some of these mutants have been found to be effective R. solani antagonists. According to a recent RNA sequencing study, 20 genes associated with mycoparasitism, including extracellular proteases, oligopeptide transporters, GPCRs, chitinases, glucanases, and proteases, were found to be upregulated during the antagonistic process between T. virens ZT05 and R. solani (Halifu et al., 2020).
Trichoderma spp. Genes Involved in the Antibiosis
Antibiosis is the antagonism of R. solani caused by the toxicity of secondary metabolites generated by Trichoderma spp. In Trichoderma spp. several genes involved in secondary metabolite synthesis have been discovered (Cardoza et al., 2007; Ruocco et al., 2009; Vinale and Sivasithamparam, 2020). As indicated in Table 3, these genes encode secondary metabolites, such as pyrones, polyketides, peptaibols, gliotoxin, gliovirin, terpenoids, and other chemicals. Depending on the chemical and the target location, varying amounts of these compounds are poisonous to R. solani (Malmierca et al., 2013; Rahimi Tamandegani et al., 2020).
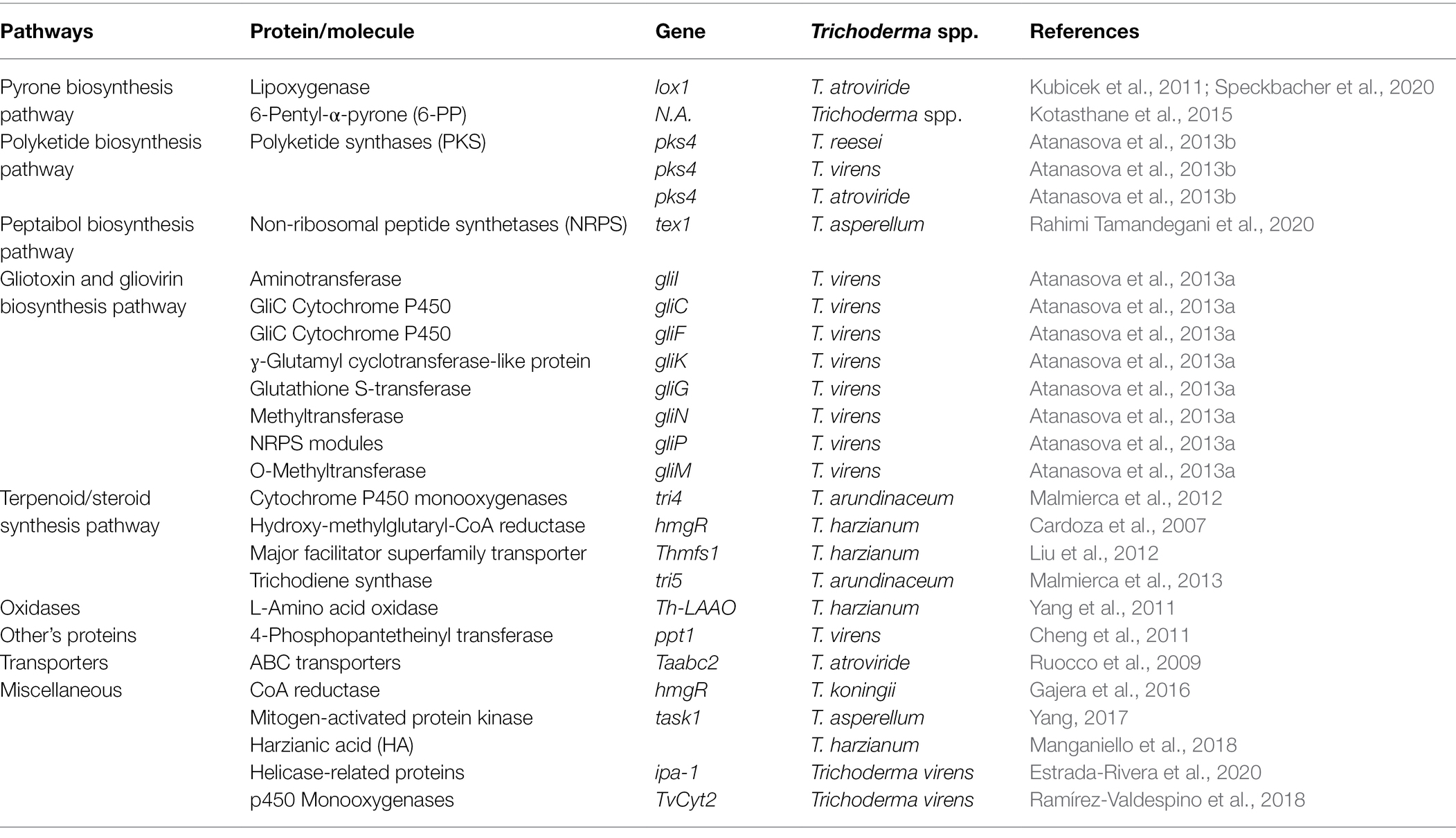
Table 3. Role of Trichoderma spp. genes involved in the antagonism and synthesis of secondary metabolites deleterious to R. solani.
Pyrones
Pyrones are a good example of secondary metabolites produced by Trichoderma spp. that have strong biocontrol activity against R. solani. They have numerous antagonistic activities against R. solani were isolated from several Trichoderma spp. For example, a characteristic aromatic odor resembling coconut was observed in a T. harzianum and T. viride due to 6-pentyl-α-pyrone (6-PP). Pyrones with significant antifungal action against R. solani were observed as one of the paramount secondary metabolites produced by Trichoderma spp. (Kotasthane et al., 2015).
Polyketides and Non-ribosomal Peptides
Polyketides
Polyketides (PKs) are a large collection of carbon-skeletoned compounds that include polyphenols, macrolides, polyenes, enediynes, and polyethers. Their synthesis is based on the regulated assembly of acetate and propionate, notwithstanding their structural and functional diversity. T. reesei, T. atroviride, and T. virens have all been shown to have PKs genes with antimicrobial action against R. solani. Two T. atroviride PKS genes were expressed during the encounter with R. solani, indicating a possible role in mycoparasitism (Mukherjee et al., 2012). Furthermore, deletion of the PKs4 gene in T. reesei altered the regulation of other PKs-encoding genes and lowered antagonistic activity against R. solani (Atanasova et al., 2013a). According to recent comparative genomics research, T. virens and T. atroviride have a considerable number of non-ribosomal peptide (NRP) and polyketides synthases genes, with T. virens having more NRPs than any other filamentous fungus investigated thus far (Niu et al., 2020).
Non-ribosomal Peptides
Non-ribosomal peptides are produced by Trichoderma spp. without the involvement of ribosomes or messenger RNAs by multidomain mega-enzymes called non-ribosomal peptide synthetases (NRPSs). NRPs perform a variety of biological functions, including iron uptake, antibacterial, and antifungal activity. Peptaibols synthesis against R. solani is attributed to NRPSs, which construct a variety of compounds from a variety of precursors, including non-proteinogenic amino acids and hydroxy or carboxyl acids (Mukherjee et al., 2011, 2012). However, NRPSs genes from additional biological control agents have yet to be characterized.
Peptaibols
Peptaibols are antibacterial, antifungal, and antiviral short-chain linear polypeptides (Mukherjee et al., 2011). Trichoderma spp. secrete peptaibols in a combination of isoforms with over 300 sequences discovered so far (Szekeres et al., 2004). Peptaibols biological activity stems from their membrane-modifying abilities, ability to make holes in lipid membranes, and proclivity to establish systemic resistance in plants against plant diseases, such as R. solani (Vey et al., 2001). So far, one NRPS generated by the tex1 gene has been identified as playing a role in peptaibols synthesis in Trichoderma spp. in response to R. solani (Table 3; Rahimi Tamandegani et al., 2020).
Gliotoxins
Gliotoxins are mycotoxins that contain sulfur have antimicrobial, antiviral, and immunomodulatory activities (Vey et al., 2001). The cell wall-degrading enzymes of Trichoderma spp. augment their antifungal activity synergistically (Lorito et al., 1998). Several genes of T. virens that encode gliotoxins, such as gliI, gliC, gliF, and gliG, appear to be important in antifungal action against R. solani (Atanasova et al., 2013a,b). However, these genes transcription is regulated by a number of factors, including pH, temperature, culture medium composition, and aeration (McDonagh et al., 2008; Table 3).
Terpenes
Terpenes are natural compounds having the formula (C5H8). There are various classes of terpenes and classification is based on the number of carbon (C) atoms as; C15; sesquiterpenes, C5; hemiterpenes, C20; diterpenes, C10; monoterpenes, C30; triterpenes, C40; tetraterpenes, C25; sesquiterpenes, or polyterpenes (Daguerre et al., 2014). In Trichoderma spp. a gene hmgR that codes for hydroxy-methylglutaryl-coenzyme has been identified. An enzyme reductase (HMGR) converts hydroxy-methylglutaryl-coenzyme into mevalonate. Mevalonate is than required for the formation of terpene compounds, such as terpene cyclases, trichodermin, triterpene viridin, and trichothecenes (Figures 2, 3). Some of these compounds, such as trichothecenes and trichodermin produced by Trichoderma spp., have antifungal activity against R. solani. Other terpenes compounds, such as triterpene ergosterol, are required for cell membrane fluidity. Trichoderma harzianum antifungal activity was diminished when hmgR was largely silenced, demonstrating that terpenoid chemicals are important in antagonism (Cardoza et al., 2007; Table 3). Harzianum A is an example of trichothecene, which inhibits fungal plant infections, such as R. solani, and induces genes involved in plant defense. Harzianum A synthesis is regulated by the tri gene cluster, which was recently identified in T. arundinaceum (Cardoza et al., 2007; Malmierca et al., 2013). When the genes tri5 and tri4 were disrupted, the generation of Harzianum A ceased, and the biocontrol activity of the transformants against R. solani was diminished.
Oxidases
Oxidases are crucial to Trichoderma spp.’ antagonistic activities against R. solani. During oxidation of glucose by oxidases, hydrogen peroxide (H2O2) is produced. Hydrogen peroxide inhibits sclerotia and subsequent hyphal growth of fungi when glucose is present. T. harzianum ETS 323 extracellular proteins recently yielded a new L-amino oxidase (Th-LAAO; Yang et al., 2011). In the presence of Th-LAAO, hyphal lysis of R. solani was seen in vitro. The efficiency of fungal antagonism against soil-borne pathogens is based not only on the production and release of antimicrobial components but also on antagonistic fungi’s capacity to protect themselves against toxins. To defend themselves from toxins generated by infections or themselves, biocontrol agents have many genes that encode ABC transporters and detoxifying enzymes. T. atroviride Taabc2 deletion mutants, for example, were less resistant to fungal inhibitory compounds, including their own, and performed less well in defending tomato plants against R. solani assaults (Ruocco et al., 2009).
Trichoderma spp. Genes Involved in Competition
Competition for Nutrients
Microorganisms require nutrients to survive, so competition for nutrient constraints and the colonization of plant tissues results in pathogens control (Sarma et al., 2014). When resources are few, microorganisms with the same ecological niche and physiological requirements struggle for nourishment (de Boer et al., 2003). Trichoderma spp., compete with R. solani for nutrients, mainly carbon (Sivan and Harman, 1991; Sarrocco et al., 2009). Comparatively to other fungi, they are better at mobilizing and absorbing soil nutrients (Sood et al., 2020). Moreover, in comparison to other fungi, they have a remarkable ability for ATP through the sugars metabolism including cellulose, hemicelluloses, glucans, and chitin (Oszust et al., 2020). Biomass components, mainly cellulose and hemicelluloses, are thought to be significant determinants of biocontrol fungi’s antagonistic activity. They are intended to be part of the saprophytic lifestyle and plant pathogen competition. Trichoderma spp., undoubtedly the most investigated fungal biocontrol agent, contain bulk of the genes encoding biomass-degrading hydrolytic enzymes found so far. Proteases, cellulases, hemicelluloses and amylases, are biological substrates degrading hydrolytic enzymes. As a result, Trichoderma spp. aid in carbon recycling. Trichoderma spp. and R. solani’s competitive capacity for cellulose utilization on wheat straw was assessed in a prior research (Sarrocco et al., 2009). Because wheat straw is the primarily source of cellulose and hemicelluloses, cellulolytic activity levels measured as mechanism in the straw possession competition. Fungus competition may also be influenced by the prompt uptake of nitrogen and carbon molecules that are either naturally present or released in the soil. Overexpression has been linked to the absorption of nutrients produced through the destruction of pathogenic fungi’s fungal cell walls, as well as direct nutrients competition in the soil, according to many transcriptome investigations. So far, only Gtt1, a high-affinity glucose transporter discovered in T. harzianum, has been examined, and its mRNA level rose in response to R. solani (Delgado-Jarana et al., 2003; Table 4). Competition for micronutrients can also arise in the soil. The most well-known example is competition for iron, which is essential for fungal pathogen development and pathogenicity. Trichoderma spp. secrete a number of siderophores that chelate iron and alter its availability to other bacteria. T. harzianum produced the most siderophores and had potent antifungal properties. A paucity of iron in the environment causes siderophore development and iron competition. Trichoderma spp. biocontrol ability against R. solani is influenced by iron competition (Table 4). As compared to R. solani and Trichoderma spp. can more effectively access the limited amounts of iron available. A peptide synthetase gene, Psy1, has been discovered. Psy1 disruptants generated normal levels of gliotoxin but struggled to grow in low-iron environments, indicating that Psy1 is involved in siderophore synthesis (Wilhite et al., 2001). Harzianic acid is a siderophore released by T. harzianum that promotes plant development while also acting as an antifungal against R. solani (Vinale et al., 2013).

Table 4. Role of Trichoderma spp. genes involved in competition for nutrients and root colonization against R. solani.
Competition for Rhizosphere
Ahmad and Baker (1987) coined the term rhizosphere competence for Trichoderma spp. which they described as the capacity of these fungi to grow and operate in the growing rhizosphere. The rhizosphere is a common ecological habitat for Trichoderma spp. and it provides saprotrophy and biotrophy possibilities on plant root exudates. Mucigel is a slimy gel-like capsule that covers plant root terminals (Oszust et al., 2020; Vinale and Sivasithamparam, 2020; Yu et al., 2022). The outermost cells of the root cap expel highly hydrated polysaccharides, such as pectins and hemicelluloses (arabinoxylans and rhamnogalacturonans). Trichoderma spp. produce hemicellulases (hemicellulolytic) and cellulases (cellulolytic) to utilize polysaccharides more effectively than R. solani secreted by plant root tips (Guzmán-Guzmán et al., 2017). For example, an endopolygalacturonase expressing gene, is required for the effective establishment of T. harzianum in the tomato rhizosphere, and this gene is also useful in root colonization and the induction of plant defenses. Plants also excrete saccharides, such as monosaccharides, disaccharides, and sucrose, which offer essential carbon substrate for Trichoderma spp. rhizosphere establishment (Oszust et al., 2020). Trichoderma spp. have also genes that encode intracellular invertases; for example, sucrose permease takes up sucrose before being hydrolyzed. Trichoderma spp. also have particular sucrose transporter and have biochemical properties similar to plant sucrose transporters (Sood et al., 2020). Sucrose is actively transmitted from plant to fungus, according to these shards of evidence. Furthermore, several Trichoderma spp. express a large number of important solute transporters, the functions of which to acquire additional root exudates are unclear (Zhang and Zhuang, 2020). In conclusion, the presence of pathogens and root-derived nutrients may have been significant attractants for Trichoderma spp. to establish themselves in the rhizosphere and create relationships with plant roots.
Competition for Colonization of Intercellular Root Spaces
The ability to recognize and cling to roots, penetrate the plant, and endure toxic compounds released by the plant in reaction to invasion is necessary for root colonization. Trichoderma spp. colonize the intercellular spaces of the first or second layer of root cells (Brotman et al., 2008). The hyphal and conidial cell walls contain several proteins that aid Trichoderma spp. in attaching to the roots via appressorium-like structures (Steindorff et al., 2012; Table 4). Enzymes, such as cellulase, hemicellulase, and protease, are subsequently secreted and employed to enter the roots (Viterbo et al., 2004). Trichoderma spp. proliferation is further aided by the highly hydrated polysaccharides of the root-secreted mucigel layer, as well as the mono- and disaccharides discharged into the rhizosphere by plant roots. According to research, root colonization, defense mechanism coordination, and leaf photosynthetic rate enhancement are all facilitated by plant-derived sucrose. To obtain root exudates, Trichoderma spp. have a variety of transporters/carriers, such as permease/intracellular invertase system and a di/tripeptide transporter. Hydrophobins are tiny proteins secreted by Trichoderma spp. that have recently been discovered. These proteins aid Trichoderma spp. in the attachment of fungal roots. These proteins feature a unique domain with eight cysteine residues in conserved positions. Based on their hydropathy patterns and solubility, hydrophobins were initially classified as class I and II. Furthermore, phytopathogenic fungi also rely on them to attach to the surface of the host plant (Talbot et al., 1996). Recently, a gene tvhydii1 that belong to class II hydrophobin, was isolated and characterized from T. virens (Guzmán-Guzmán et al., 2017). Overexpression of the gene tvhydii1 increases T. virens’ antagonistic activity against R. solani as well as the colonization of plant roots. Furthermore, deletion of tvhydii1 reduces antagonistic activity against R. solani and plant root colonization. Trichoderma spp. also encode expansin-like proteins, such as Swollenin TasSwo, which loosen, expand, or disrupt plant cell wall elements including cellulose and hemicellulose. Although their exact mechanism of action is unclear, it is thought that expansin-like proteins break into the crevices generated by interlacing microfibers in the cell wall, causing a conformational shift that causes the cell wall to expand, assisting root colonization. Plant CWDEs are also engaged in active root colonization, in addition to expansin and hydrophobin proteins. Many plants showed the strengthening of epidermal and cortical cell walls, as well as the deposition of considerable quantities of callose and cellulose, 72 h following root colonization. Callose-enriched cell walls limit Trichoderma spp. to the epidermis and cortical intercellular gaps, preventing Trichoderma spp. from entering the vascular stele. Antimicrobial chemicals are also synthesized and accumulated by plants in response to Trichoderma spp. invasion. To a large extent, the ability to colonize plant roots is determined by the strain’s ability to withstand environmental stresses. Several ATP-binding cassette (ABC) transporter genes have been discovered and described in Trichoderma spp., and they have been linked to the transfer of a variety of substrates, including phytotoxins, mating factors, antibiotics, pesticides, and heavy metals. The rapid degradation of phenolic compounds exuded by plants and the suppression of phytoalexin production, as detected in Trichoderma spp. is due to ABC transport systems (Cheng et al., 2011). ABC transport systems are key factors in the multiple interactions established by Trichoderma spp. with other microbes in a potentially toxic or antagonistic environment. Trichoderma spp. has been shown to contribute to biocontrol through small cysteine-rich proteins. These small proteins bind to chitin of plants and fungi and prevent Trichoderma spp. from chitinases (Stergiopoulos and de Wit, 2009).
Trichoderma spp. Induced Resistance in Different Plants Against Rhizoctonia solani
Trichoderma-Induced Systemic Resistance
Depending on the pathosystem, plant defense responses are usually triggered by activation of a complex signal transduction network that includes salicylic acid (SA), jasmonic acid (JA), either with or without ethylene (ET), and abscisic acid (ABA) as important plant immunity regulators. Checker et al. (2018), SA-mediated signaling pathways in plants result in systemic acquired resistance (SAR) against biotrophic and hemibiotrophic diseases. JA/ET-mediated signaling pathways, on the other hand, result in induced systemic resistance (ISR) in plants against necrotrophic diseases (Pieterse et al., 2014). According to a recent research, Trichoderma spp. induce a hybrid ISR/SAR type of resistance in plants against fungal pathogens including R. solani known as Trichoderma-induced systemic resistance (TISR; Leonetti et al., 2017; Figure 4). Hence, resistance in plants is increased by Trichoderma spp. against R. solani, which can be activated through signaling pathways that involve both SA and JA/ET-mediated signal transduction. Even still, scientists disagree on the method by which Trichoderma spp. activate defensive responses and the sort of resistance they induce in plants. Furthermore, there are significant gaps and differences when it comes to explaining the crosstalk of signaling molecules involved in Trichoderma-induced defense responses in plants. Other chemicals that may reduce or increase the synthesis and activity of signaling molecules must also be considered in order to better comprehend the crucial interactions between JA, SA, ET, and their derivatives in the intricate signaling network (Figure 4).
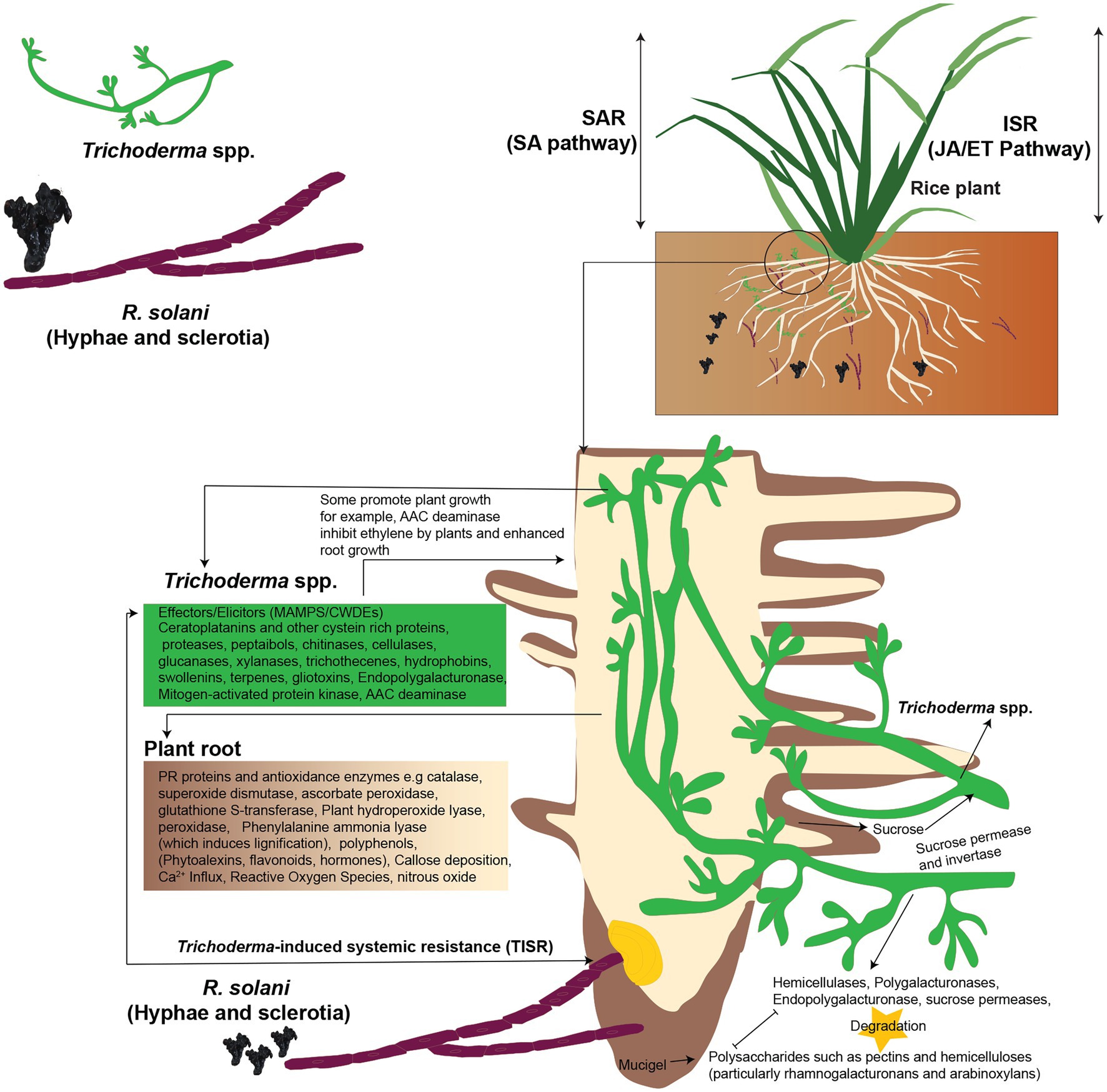
Figure 4. Trichoderma-induced systemic resistance (TISR) in plants against R. solani. Both SA and JA/ET-mediated signal transduction pathways may trigger defensive responses against R. solani, boosting plant resistance. Trichoderma spp. release enzymes to degrade plant polysaccharides, colonize the roots, and take sucrose as a carbon source by using sucrose permease and invertase enzymes. Trichoderma spp. produce elicitors, such as MAMPs to induce TISR in the plants; plants synthesize hydroperoxide lyase, peroxidase, and phenylalanine ammonia-lyase (which induces lignification) and deposit callose. Trichoderma MAMPs, such as xylanase, elicits plant defense responses against R. solani. The 1-aminocyclopropane-1-carboxylic acid (AAC) deaminase inhibits ethylene formation by the plant, and this leads to enhanced root growth, and this is due to the formation of hormones. Besides, Trichoderma spp. attach to plants roots by producing hydrophobins and swollenin. Reconstructed from Druzhinina et al. (2011).
Microorganism-Associated Molecular Patterns
Like fungi and mammals, plants activate possible defense systems in response to the presence of other species. This is best appreciated in the context of pathogens that induce a two-branched inborn immune response. PAMP-triggered immunity (PTI) is the first stage in which plants use pattern recognition receptors to recognize and respond to pathogen-associated molecular patterns (PAMPs) or microorganism-associated molecular patterns (MAMPs). Plants respond to pathogen virulence factors in the second step known as effector-triggered immunity (ETI). MAMPs are molecular signatures that are extremely conserved, such as fungi’s chitin and xylanase and oomycetes’ heptaglucan. After PRR activation, changes in ion fluxes across the plasma membrane, the oxidative burst (production of nitric oxide and reactive oxygen species), activation of MAPK cascades, and callose deposition are all downstream defensive activation events. Many breakthroughs have been made in identifying the pathways involved in this resistance; in many cases, SA or JA, when combined with ET, ROS, or NO, causes a cascade of processes that culminate in the synthesis of a range of metabolites and proteins with varied roles (Bellin et al., 2013). ABA, auxins, gibberellins, cytokinins, and brassinosteroids have also been demonstrated to play essential roles in recent studies (Pieterse et al., 2009). Although there appears to be crosstalk or competition between the pathways, different stresses stimulate distinct routes (Durrant and Dong, 2004). SAR confers long-term disease resistance by accumulating genes that produce pathogenesis-related (PR) proteins against pathogens that are either biotrophic or hemibiotrophic (Pieterse et al., 2009). However, rather than directly initiating PR gene transcription, ISR enhances plants’ defenses against a future onslaught by necrotrophic pathogen like R. solani. Overall, the JA/ET and SA pathways are thought to be mutually special. However, other investigations have found a synergistic effect between these routes. According to recent advances, Trichoderma spp. simultaneously induces plant SAR-related genes as well as ISR-related genes during plant root colonization, providing protection against R. solani with various lifestyles (Salas-Marina et al., 2011). Recent research has shown that Trichoderma spp. may cause biochemical and molecular alterations in SAR, which are mostly linked to the production of PR proteins, such as PR1, PR5, and PR2 (Zhang and Zhuang, 2020). The activation of the mutually antagonistic SA and JA pathways by Trichoderma spp. results in a loss of plant ecological fitness, a process known as crosstalk (Van Oosten et al., 2008). Trichoderma–plant interactions take place predominantly in the rhizosphere, where resistance development is driven by the interchange of microbial and plant elicitors required for organism-to-organism interactions. Depending on the involved elicitors created by Trichoderma hyphae, the interaction of these molecules with plant receptors may influence Trichoderma adhesion and identification, and hence the induction of resistance in plants. Secondary metabolites produced by Trichoderma spp., such as proteins with enzymatic activity, as well as Trichoderma spp. and plant cell wall components, are all implicated in the development of plant resistance. Pathogen defenses have been activated by plant components, such as pectins, phospholipids, and saccharides. Some Trichoderma elicitors, include proteins produced by avirulence genes and MAMPs, which are slow-evolving molecules. Trichoderma spp. secrete low molecular weights (6–42 kDa) enzymes or peptides which act as elicitors, such as serine xylanases, proteases, chitinases, cellulases, or glucanases. Other Trichoderma spp. compounds which act as elicitors are indole compounds, fatty acids, lipids and their derivatives (glycosphingolipids etc.), saccharides (polysaccharides or oligosaccharides) and chitin or chitin-like compounds (Djonović et al., 2007; da Silva Aires et al., 2012; Tables 4 and 5).
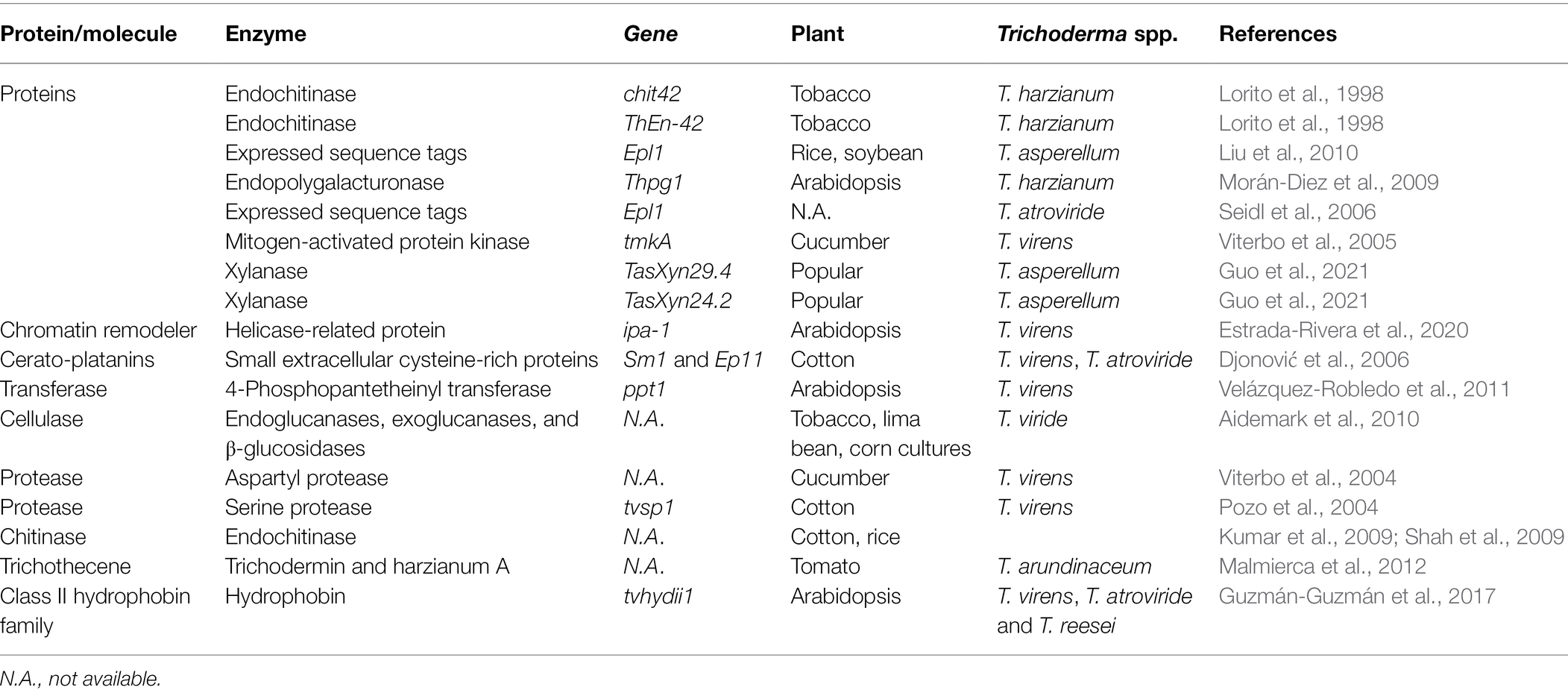
Table 5. Role of Trichoderma spp. genes which act as elicitors in resistance induction in different plants against R. solani.
Cerato-Platanins, Hydrophobins, Swollenins, and Expansins
Small proteins like cerato-platanins, hydrophobins, swollenins, and expansins are vital between Trichoderma spp. and plants. Host presence stimulates secretion of these proteins by Trichoderma spp. These proteins are also involved in mycoparasitism and induction of plants resistance against pathogens. For example, Cerato-platanins (Sm1/Epl1), which are tiny secreted proteins with four cysteines linked together by two disulfide linkages are necessary for T. atroviride and T. virens mediated cotton resistance to R. solani. They trigger systemic and local resistance in plants for R. solani (Djonović et al., 2006; Seidl et al., 2009). Hydrophobins are a group of other small proteins which are secreted from the Trichoderma spp. cell wall. They help Trichoderma spp. to adhere to the root surface. Local defense in plants is stimulated by Swollenin, expansin-like proteins with a cellulose-binding domain (Guzmán-Guzmán et al., 2017; Table 5). Swollenin disrupt plant cell walls’ crystalline cellulose structure and aid in Trichoderma spp. colonization of plants roots (Saloheimo et al., 2002). Root colonization by T. asperellum stimulates the production of swollenin to induce local defense in plant (Brotman et al., 2008). In the rhizosphere, Trichoderma spp. use swollenin to establish themselves by increase root surface area. Plant protein for expansion of root and root hair cell wall is expansins which has sequence similarity to Swollenin (Guo et al., 2011).
Xylanases
Glycosyl Hydrolase Family 11 bacteria secrete xylanases, which are essential CWDEs (GH11). These CWDEs have the ability to degrade xylan, a key component of plant cell walls. Plants can detect the loss of cell wall integrity when xylanases begin to breakdown the plant cell wall and trigger the defensive signaling system. Recently, xylanases (TasXyn29.4 and TasXyn24.2) were discovered as Trichoderma spp. MAMPs that induce plant defensive responses in Popular. The production of these T. asperellum xylanases in plants induced plant defense responses against R. solani attacks mediated by ethylene and H2O2 (Guo et al., 2021).
Chitinases
Chit42, a T. harzianum chitinase improved resistance of tobacco to R. solani. This show the importance of chitinases in the activation of resistance in plants (Lorito et al., 1998).
Proteases
Trichoderma spp. produce considerable amounts of proteases. T. harzianum, T. asperellum, and T. virens have aspartyl and serine proteases that are involved in both mycoparasitism and symbiotic relationship between Trichoderma spp. and plants, besides, enhancing defensive capability against R. solani (Viterbo et al., 2005). Additionally, these enzymes assist Trichoderma spp. in colonization of plants roots as well as the production of secondary metabolites such PR proteins and phytoalexins against R. solani (Viterbo et al., 2005).
Peptaibols and Trichothecenes
Another notable group of Trichoderma spp. secondary metabolites are peptaibols (peptaibiotic). The name peptaibols comes from a combination of the terms PEPTide, AIB, and alcohOLs, which are the distinguishing characteristics of peptaibols. Peptaibols are characterized by large quantities of non-standard amino acids (especially a-aminoisobutyric acid), 2-amino alcohol and a C-terminal 1. Peptaibols are not produced by ribosomes. Instead, multidomain enzymes on huge non-ribosomal peptide synthetase (NRPS) complexes produce them (Mukherjee et al., 2012). These peptaibols are also important elicitors produced mainly by T. atroviride and T. virens and are implicated in the development of pathogen resistance in plants (Mukherjee et al., 2012). Trichothecenes are also produced by Trichoderma spp., that is, Harzianum A and trichodermin which act as elicitors for plant resistance to R. solani. A tri4 gene mutation, for example, reduced antifungal action against R. solani as well as the ability to regulate the production of tomato plant defense-related genes from the SA and JA pathways (Malmierca et al., 2012). Furthermore, plant development and plant defense mechanisms are also induced by oxygen heterocyclic compounds (OHC), such as polyketides, harzianolides, harzianopyridone, and pyrones (esters), peptides (gliovirin and gliotoxin), non-polar chemicals (terpenoids and steroids), and anthraquinone pigments. However, plant resistance through Trichoderma spp. by reduction of VOCs, that is, ketones and aldehydes remain questionable.
Role of MAMPs, Reactive Oxygen and Nitrogen Species, Transcription Factors, Defense-Related Genes and Enzymes
Trichoderma spp. Release MAMPs for Molecular Recognition by Receptors of Plants
PRRs recognize MAMPs released by Trichoderma spp. By signaling molecules within the plants, these MAMPs contribute to the signal cascade. Trichoderma MAMPs transiently promote Ca2+ and H+ influx and K+ and Cl− ejection in response to suitable plant receptors. Variations in the plasma membrane potential (Vm) occur often because of ion imbalances and changes in the channel activity (Liu et al., 2010). Plant cells undergo fast ROS, RNS and pH changes during depolarization. In addition to changes in ion absorption, Trichoderma spp. releases organic acids (gluconic or citric acid) into the soil, which lowers the soil pH. ROS and RNS are released during cell wall expansion and lignification. Furthermore, cell wall peroxidases and calcium channels become active. Many secondary metabolites and signaling molecules, including as H2O2 and NO, as well as SA and its derivatives, and JA/ET are generated and accumulated in response to Trichoderma spp. (Harman et al., 2004). Trichoderma spp. MAMPS and other effector molecules bind to PRRs and intracellular receptors in plants, triggering MTI (MAMPS-triggered) and ETI (effector-triggered) immunity. This interaction between Trichoderma spp. and plants produces ROS and RNS, which act as signaling molecules and initiate a defensive response in plants by synthesizing antifungal molecules, such as phytoalexins, VOCs (volatile organic compounds), PRs proteins, such as CWDEs, and so on. Trichoderma spp. has a local and systemic action that involves a signaling cascade and activation, as well as the accumulation of antimicrobial compounds and enzymes, such as polyphenol oxidase, peroxidase, lipoxygenase and PAL. PR proteins, terpenoids, phytoalexins (rishitin, phytosterol, lubimin, coumarin, resveratrol, solavetivone, and others), and antioxidants (glutathione, ascorbic acid, and others) are produced. Plants respond to fungal invasion by producing and concentrating defensive molecules, such as phytoalexins, aglycones, flavonoids, phenolic byproducts, terpenoids, and other antimicrobial substances. Trichoderma spp. on the other hand, are typically resistant to plant defense compounds and colonize plant root due to presence of ABC transport systems. Recent studies show that plants employ ROS and RNS as messengers to control the interplay between secondary messengers, MAPKs, and hormones, which are crucial in the induction of plant resistance SAR (Sami et al., 2018). An enzyme system known as the MAPKs kinase pathway may be activated by extracellular Trichoderma MAMPs, when they connect with plant receptors. The first step in triggering MPKKK kinase activity is a ligand–receptor contact. In the next step, MPK kinase is phosphorylated by MPKKKs, which in turn activates MPKK kinases, which phosphorylate MPKs (Sami et al., 2018).
Reactive Oxygen and Nitrogen Species
In oxidative signaling, reactive oxygen (RO) and reactive nitrogen (RN) species interact with multiple hormonal signaling pathways. When plants were challenged with necrotrophic fungi, such as R. solani, among all RO and RN species, H2O2 and nitric oxide (NO) were shown to be quickly produced and were considered primary defensive activators. The processes by which NO may influence defensive signaling cascades were well investigated. S-nitrosylation and tyrosine-rich group nitration have emerged as significant NO-dependent protein regulatory mechanisms. Protein cysteine-rich thiol groups react with NO to create S-nitrosothiols so-called “reversible S-nitrosylation of proteins.” S-nitrosylation plays an important role in defensive responses, as shown by its effects on the SA signaling protein NPR1 and the ROS-generating NADPH oxidase complex AtRBOHD. NO has been shown to work with ROS and SA to establish SAR in plants (Sami et al., 2018). NO is also linked to other resistance-inducing signaling pathways, such as JA and ET. NO via S-nitrosylation has been shown in recent research to be one of the key regulators of SA-dependent systemic defensive responses in T. atroviride-treated plants. SA play an important part in the systemic defense responses elicited by T. atroviride in cucumber plants, protecting them from R. solani. SA or increasing the quantity of H2O2 or NO could promote the synthesis of active NPR1 proteins, which regulate the expression of genes that code for plant defense proteins. NPR1, which resides as an oligomer in the cytoplasm and is held together by intermolecular disulfide bonds, is susceptible to changes in redox status. By modifying the state of cell reduction, accumulated SA, H2O2 and NO might diminish disulfide bonds, leading NPR1 to degrade into monomers, which, when transferred into a nucleus to induce TISR by activating pathogenesis-related (PR) genes (Nawrocka et al., 2019). The mitogenic kinase pathways are also activated, resulting in the activation of transcription factors against R. solani (Nawrocka et al., 2019).
Transcription Factors, Defense-Related Genes, and Enzymes
Transcription Factors and Pathogenesis-Related Genes
Transcription factors modulate the expression of certain genes required for many key physiological activities and stress responses, acting as regulators of gene transcription. The WRKY transcription factor family has been connected to abiotic stress, growth, and development in addition to plant–microbe interactions. As discussed before, SAR often results in increased levels of SA and coordinated activation of PR genes, such as PR5, PR2, and PR1 involving one or more signaling molecules, that transmit an elevated immune response against R. solani. For example, when bean plants were exposed to T. velutinum in the absence of R. solani, WRKY33 gene expression increased considerably whereas PR1 expression decreased. However, when beans plants were just exposed to R. solani, WRKY33 gene expression was reduced whereas PR1 gene expression remained unaffected. Furthermore, when bean plants were exposed to T. velutinum and R. solani, the genes WRKY33 and PR1 were both downregulated (Mayo et al., 2016). Furthermore, T. asperelloides colonization of A. thaliana roots triggered a rapid increase in expression of WRKY transcription factors, which suppressed SA signaling and triggered JA pathway responses against R. solani. These findings imply that WRKY proteins, which are well-known PR gene activators, play a key role in chromatin modifications that enhance gene expression (Mayo et al., 2016). PR gene expression, including enzymes, such as cellulases, glucanases, and chitinases, is engaged in direct control of R. solani and plant biochemical barrier reinforcement (Heflish et al., 2021). In another study, the interaction of bean plants with R. solani resulted in the downregulation of seven defense-related genes, including chitinases (CH5b, CH1), PR1, PR2, PR3, PR4, and PAL, as a mechanism to overcome the plant defense response, allowing the infection process to progress within the plant. Ergosterol is a sterol present in the fungal membrane that, although being classified as a MAMP by the plant, causes a sequence of events that result in the activation of defense-related genes. Squalene (polyunsaturated terpene) is a precursor in the biosynthesis of ergosterol. In one study, higher ergosterol and squalene synthesis by Trichoderma spp. resulted in the activation of defense-related genes in bean plants against R. solani (Mayo et al., 2015).
Defense-Related Genes and Enzymes
Many studies have shown that plants treated with Trichoderma spp. boosted the activity of defense-related enzymes, such as peroxidase, chitinase, peroxidase, -1, 3-glucanase, phenylpropanoids, polyphenol oxidase, superoxide dismutase, chitinase, and phenylalanine ammonia-lyase (PAL). When cotton seeds were treated with T. virens, for example, peroxidase activity was increased in the roots of treated cotton plants (Howell, 2003). Furthermore, by boosting ROS scavenging enzymes, Trichoderma spp. contribute to plant resistance to R. solani. Trichoderma harzianum, for example, increases the activities of ascorbate peroxidase (APX), guaiacol peroxidase (GPX), superoxide dismutase (SOD), and catalase (CAT) in tomato plants against R. solani (Youssef et al., 2016). Trichoderma spp. also produce lytic enzymes, such as chitinase and β-1,3-glucanase, which break down R. solani chitin and β-1,3-glucan components. During an R. solani attack, for example, a bean chitinase promoter is substantially activated in transgenic tobacco plants (Roby et al., 1990). The protection against R. solani given by the expression of certain chitinase genes from Trichoderma spp. or other plants is remarkable. Defense-related gene expression and chitinase enzyme activity, for example, confer high resistance to R. solani infection in transgenic cotton plants expressing an endochitinase gene from T. virens (Kumar et al., 2009). Cellulases from Trichoderma spp. have also been shown to produce ISR in plants via the ET or JA pathways. Furthermore, T. viride and T. harzianum with biocontrol capacity to protect rice plants against R. solani exhibited negative morphological and physiological changes in the pathogen hyphae, such as swelling, knotting, crumpling, flattening, shriveling, bursting, and cytoplasm leakage. Furthermore, the increase of defense-related enzymes has been noted (Singh et al., 2016). Trichoderma virens promoted ISR in tomato plants by activating defense enzymes, such as GPX, syringaldazine peroxidase (SPX), and PAL against R. solani in another study. As a result, the buildup of secondary metabolites, such as phenols and H2O2, was increased, but lipid peroxidation was reduced in the leaves (Małolepsza et al., 2017). As a result, Trichoderma spp. treatment of plants produced disease resistance against R. solani by reprogramming the pathways and cascades involved in several defense-related activities (Zeilinger and Atanasova, 2020). The conclusion is that TISR is a complex occurrence, and the current findings do not reflect a thorough grasp of the processes and reactions to R. solani.
Conclusion and Future Perspectives
To date, the best biocontrol agents described against R. solani are Trichoderma spp. Antagonism of R. solani is linked to a variety of Trichoderma spp. genes. As previously mentioned, antagonism is dependent on a number of genes for sensing, signaling, antibiosis, and mycoparasitism. In addition, many Trichoderma spp. genes are involved in competition and systemic resistance induction in plants for R. solani. In Figures 2–4, we described the Trichoderma spp. genes involved in antagonism of R. solani, what happens to R. solani when it is parasitized by Trichoderma spp. and how Trichoderma spp. induce resistance against R. solani. As many Trichoderma spp. genes are involved in the antagonism of R. solani, making the molecular mechanisms underlying the antagonistic effects more complicated. Hence, to completely comprehend the effect of Trichoderma spp. genes against R solani, additional studies are required. In conclusion, Trichoderma spp. possess a diverse set of genes that produce secondary compounds which can parasitize and antagonize R. solani. Systemic resistance against R. solani by Trichoderma spp. largely because of variety of metabolites produced against it. Trichoderma spp. also have a diverse set of effectors and elicitors recognized by plant receptors to activate signaling and gene regulation, which serves as the foundation for Trichoderma spp. to develop R. solani defense responses, as shown in Table 5. More research into the molecular, physiological, and biochemical underpinnings of Trichoderma spp. activity as multifunctional biocontrol agents is required to fully comprehend the impact of Trichoderma spp. on plants and their practical utility in plant protection against R. solani. The chemical nature of a number of secondary metabolites generated by Trichoderma spp. against R. solani is still unknown. Furthermore, there is a need to understand the molecular communication between Trichoderma spp. and plants in the presence of R. solani. Due to their precision, sensitivity, and specificity, efficient and sophisticated Next-generation sequencing technologies are currently used in studies involving Trichoderma spp. and R. solani. They will hopefully fill a gap in Trichoderma spp. biocontrol studies against R. solani when combined with other methods, such as metabolomics, metagenomics, proteomics, and bioinformatics.
Author Contributions
AA and MM: writing-original draft and figure preparations. MAS, QS, MKS, SS, BKK, MCZR, SH, and EAM-E: collecting literatures, software, tables preparations, and editing. HZ, YI, and LZ: supervision, project administration, resources, and funding acquisition. All authors have read and agreed to the published version of the manuscript. All authors listed have made a substantial, direct and intellectual contribution to the work and approved it for publication.
Funding
This work was supported by the China Postdoctoral Science Foundation (no: 2014M561669) and the Zhejiang Academy of Agricultural Sciences “high-talent introduction and ongoing training fund” (grant no: 10300000021LL05).
Conflict of Interest
The authors declare that the research was conducted in the absence of any commercial or financial relationships that could be construed as a potential conflict of interest.
Publisher’s Note
All claims expressed in this article are solely those of the authors and do not necessarily represent those of their affiliated organizations, or those of the publisher, the editors and the reviewers. Any product that may be evaluated in this article, or claim that may be made by its manufacturer, is not guaranteed or endorsed by the publisher.
References
Abbas, A., Fu, Y., Qu, Z., Zhao, H., Sun, Y., Lin, Y., et al. (2021). Isolation and evaluation of the biocontrol potential of Talaromyces spp. against rice sheath blight guided by soil microbiome. Environ. Microbiol. 23, 5946–5961. doi: 10.1111/1462-2920.15596
Abdel-lateif, K. S. (2017). Trichoderma as biological control weapon against soil borne plant pathogens. Afr. J. Biotechnol. 16, 2299–2306. doi: 10.5897/ajb2017.16270
Ahmad, J. S., and Baker, R. (1987). Rhizosphere competence of Trichoderma harzianum. Phytopathology 77, 182–189. doi: 10.1094/phyto-77-182
Aidemark, M., Tjellström, H., Sandelius, A. S., Stålbrand, H., Andreasson, E., Rasmusson, A. G., et al. (2010). Trichoderma viride cellulase induces resistance to the antibiotic pore-forming peptide alamethicin associated with changes in the plasma membrane lipid composition of tobacco BY-2 cells. BMC Plant Biol. 10, 1–13. doi: 10.1186/1471-2229-10-274
Ajayi-Oyetunde, O. O., and Bradley, C. A. (2018). Rhizoctonia solani: taxonomy, population biology and management of Rhizoctonia seedling disease of soybean. Plant Pathol. 67, 3–17. doi: 10.1111/ppa.12733
Ali, A., Zeshan, M. A., Mehtab, M., Khursheed, S., Mudasir, M., Abid, M., et al. (2021). A comprehensive note on Trichoderma as a potential biocontrol agent against soil borne fungal pathogens: a review. Plant Prot. 5, 171–196. doi: 10.33804/pp.005.03.3934
Antal, Z., Manczinger, L., Szakacs, G., Tengerdy, R. P., and Ferenczy, L. (2000). Colony growth, in vitro antagonism and secretion of extracellular enzymes in cold-tolerant strains of Trichoderma species. Mycol. Res. 104, 545–549. doi: 10.1017/S0953756299001653
Atanasova, L., Crom, S. L., Gruber, S., Coulpier, F., Seidl-Seiboth, V., Kubicek, C. P., et al. (2013a). Comparative transcriptomics reveals different strategies of Trichoderma mycoparasitism. BMC Genomics 14, 1–15. doi: 10.1186/1471-2164-14-121
Atanasova, L., Gruber, S., Lichius, A., Radebner, T., Abendstein, L., Münsterkötter, M., et al. (2018). The Gpr1-regulated Sur7 family protein Sfp2 is required for hyphal growth and cell wall stability in the mycoparasite Trichoderma atroviride. Sci. Rep. 8, 1–15.
Atanasova, L., Knox, B. P., Kubicek, C. P., Druzhinina, I. S., and Baker, S. E. (2013b). The polyketide synthase gene pks4 of Trichoderma reesei provides pigmentation and stress resistance. Eukaryot. Cell 12, 1499–1508. doi: 10.1128/ec.00103-13
Badaluddin, N. A., Jamaluddin, S. N. T., Ihsam, N. S., Sajili, M. H., Khalit, S. I., and Mohamed, N. A. (2018). Molecular identification of isolated fungi from Kelantan and Terengganu using internal transcriber spacer (ITS) region. J. Agric. Biotechnol. 9, 222–231.
Baek, J.-M., Howell, C. R., and Kenerley, C. M. (1999). The role of an extracellular chitinase from Trichoderma virens Gv29-8 in the biocontrol of Rhizoctonia solani. Curr. Genet. 35, 41–50. doi: 10.1007/s002940050431
Bellin, D., Asai, S., Delledonne, M., and Yoshioka, H. (2013). Nitric oxide as a mediator for defense responses. Mol. Plant-Microbe Interact. 26, 271–277. doi: 10.1094/MPMI-09-12-0214-CR
Brotman, Y., Briff, E., Viterbo, A., and Chet, I. (2008). Role of swollenin, an expansin-like protein from Trichoderma, in plant root colonization. Plant Physiol. 147, 779–789. doi: 10.1104/pp.108.116293
Brunner, K., Omann, M., Pucher, M. E., Delic, M., Lehner, S. M., Domnanich, P., et al. (2008). Trichoderma G protein-coupled receptors: functional characterisation of a cAMP receptor-like protein from Trichoderma atroviride. Curr. Genet. 54, 283–299. doi: 10.1007/s00294-008-0217-7
Brunner, K., Peterbauer, C. K., Mach, R. L., Lorito, M., Zeilinger, S., and Kubicek, C. P. (2003). The Nag1 N-acetylglucosaminidase of Trichoderma atroviride is essential for chitinase induction by chitin and of major relevance to biocontrol. Curr. Genet. 43, 289–295. doi: 10.1007/s00294-003-0399-y
Cardoza, R. E., Hermosa, M. R., Vizcaíno, J. A., González, F., Llobell, A., Monte, E., et al. (2007). Partial silencing of a hydroxy-methylglutaryl-CoA reductase-encoding gene in Trichoderma harzianum CECT 2413 results in a lower level of resistance to lovastatin and lower antifungal activity. Fungal Genet. Biol. 44, 269–283. doi: 10.1016/j.fgb.2006.11.013
Carsolio, C., Benhamou, N., Haran, S., Cortés, C., Gutiérrez, A., Chet, I., et al. (1999). Role of the Trichoderma harzianum endochitinase gene, ech42, in mycoparasitism. Appl. Environ. Microbiol. 65, 929–935. doi: 10.1128/AEM.65.3.929-935.1999
Checker, V. G., Kushwaha, H. R., Kumari, P., and Yadav, S. (2018). “Role of phytohormones in plant defense: signaling and cross talk,” in Molecular Aspects of Plant-Pathogen Interaction (Singapore: Springer), 159–184.
Cheng, C.-H., Yang, C.-A., Liu, S.-Y., Lo, C.-T., Huang, H.-C., Liao, F.-C., et al. (2011). Cloning of a novel L-amino acid oxidase from Trichoderma harzianum ETS 323 and bioactivity analysis of overexpressed L-amino acid oxidase. J. Agric. Food Chem. 59, 9142–9149. doi: 10.1021/jf201598z
Chung, D., Kwon, Y. M., and Yang, Y. (2021). Telomere-to-telomere genome assembly of asparaginase-producing Trichoderma simmonsii. BMC Genomics 22:830. doi: 10.1186/s12864-021-08162-4
Contreras-Cornejo, H. A., Macías-Rodríguez, L., del Val, E., and Larsen, J. (2016). Ecological functions of Trichoderma spp. and their secondary metabolites in the rhizosphere: interactions with plants. FEMS Microbiol. Ecol. 92:fiw036. doi: 10.1093/femsec/fiw036
Cortes, C., Gutierrez, A., Olmedo, V., Inbar, J., Chet, I., and Herrera-Estrella, A. (1998). The expression of genes involved in parasitism by Trichoderma harzianum is triggered by a diffusible factor. Mol. Gen. Genet. MGG 260, 218–225. doi: 10.1007/s004380050889
da Silva Aires, R., Steindorff, A. S., Ramada, M. H. S., de Siqueira, S. J. L., and Ulhoa, C. J. (2012). Biochemical characterization of a 27kDa 1,3-β-d-glucanase from Trichoderma asperellum induced by cell wall of Rhizoctonia solani. Carbohydr. Polym. 87, 1219–1223. doi: 10.1016/j.carbpol.2011.09.001
Daguerre, Y., Siegel, K., Edel-Hermann, V., and Steinberg, C. (2014). Fungal proteins and genes associated with biocontrol mechanisms of soil-borne pathogens: a review. Fungal Biol. Rev. 28, 97–125. doi: 10.1016/j.fbr.2014.11.001
Das, S., Shah, F. A., Butler, R. C., Falloon, R. E., Stewart, A., Raikar, S., et al. (2014). Genetic variability and pathogenicity of Rhizoctonia solani associated with black scurf of potato in New Zealand. Plant Pathol. 63, 651–666. doi: 10.1111/ppa.12139
de Boer, W., Verheggen, P., Klein Gunnewiek, P. J. A., Kowalchuk, G. A., and van Veen, J. A. (2003). Microbial community composition affects soil fungistasis. Appl. Environ. Microbiol. 69, 835–844. doi: 10.1128/AEM.69.2.835-844.2003
de França, S. K. S., Cardoso, A. F., Lustosa, D. C., Ramos, E. M. L. S., de Filippi, M. C. C., and da Silva, G. B. (2015). Biocontrol of sheath blight by Trichoderma asperellum in tropical lowland rice. Agron. Sustain. Dev. 35, 317–324. doi: 10.1007/s13593-014-0244-3
De La Cruz, J., Pintor-Toro, J. A., Benitez, T., Llobell, A., and Romero, L. C. (1995). A novel endo-beta-1, 3-glucanase, BGN13. 1, involved in the mycoparasitism of Trichoderma harzianum. J. Bacteriol. 177, 6937–6945. doi: 10.1128/jb.177.23.6937-6945.1995
De las Mercedes Dana, M., Limón, M. C., Mejías, R., Mach, R. L., Benítez, T., Pintor-Toro, J. A., et al. (2001). Regulation of chitinase 33 (chit33) gene expression in Trichoderma harzianum. Curr. Genet. 38, 335–342. doi: 10.1007/s002940000169
Delgado-Jarana, J., Moreno-Mateos, M. A., and Benítez, T. (2003). Glucose uptake in Trichoderma harzianum: role of gtt1. Eukaryot. Cell 2, 708–717. doi: 10.1128/EC.2.4.708-717.2003
Delgado-Jarana, J., Rincón, A. M., and Benı Tez, T. A. (2002). Aspartyl protease from Trichoderma harzianum CECT 2413: cloning and characterization. Microbiology 148, 1305–1315. doi: 10.1099/00221287-148-5-1305
Dickman, M. B., and Yarden, O. (1999). Serine/threonine protein kinases and phosphatases in filamentious fungi. Fungal Genet. Biol. 26, 99–117. doi: 10.1006/fgbi.1999.1118
Djonović, S., Pozo, M. J., Dangott, L. J., Howell, C. R., and Kenerley, C. M. (2006). Sm1, a proteinaceous elicitor secreted by the biocontrol fungus Trichoderma virens induces plant defense responses and systemic resistance. Mol. Plant-Microbe Interact. 19, 838–853. doi: 10.1094/MPMI-19-0838
Djonović, S., Vittone, G., Mendoza-Herrera, A., and Kenerley, C. M. (2007). Enhanced biocontrol activity of Trichoderma virens transformants constitutively coexpressing β-1, 3-and β-1, 6-glucanase genes. Mol. Plant Pathol. 8, 469–480. doi: 10.1111/j.1364-3703.2007.00407.x
Dong, S., Shew, H. D., Tredway, L. P., Lu, J., Sivamani, E., Miller, E. S., et al. (2008). Expression of the bacteriophage T4 lysozyme gene in tall fescue confers resistance to gray leaf spot and brown patch diseases. Transgenic Res. 17, 47–57. doi: 10.1007/s11248-007-9073-3
Druzhinina, I. S., Seidl-Seiboth, V., Herrera-Estrella, A., Horwitz, B. A., Kenerley, C. M., Monte, E., et al. (2011). Trichoderma: the genomics of opportunistic success. Nat. Rev. Microbiol. 9, 749–759. doi: 10.1038/nrmicro2637
Dubey, M., Jensen, D. F., and Karlsson, M. (2021). Functional characterization of the AGL1 aegerolysin in the mycoparasitic fungus Trichoderma atroviride reveals a role in conidiation and antagonism. Mol. Gen. Genomics. 296, 131–140. doi: 10.1007/s00438-020-01732-3
Dubey, M. K., Ubhayasekera, W., Sandgren, M., Funck Jensen, D., and Karlsson, M. (2012). Disruption of the Eng18B ENGase gene in the fungal biocontrol agent Trichoderma atroviride affects growth, conidiation and antagonistic ability. PLoS One 7:e36152. doi: 10.1371/journal.pone.0036152
Durrant, W. E., and Dong, X. (2004). Systemic acquired resistance. Annu. Rev. Phytopathol. 42, 185–209. doi: 10.1146/annurev.phyto.42.040803.140421
Estrada-Rivera, M., Hernández-Oñate, M. Á., Dautt-Castro, M., Gallardo-Negrete, J. D. J., Rebolledo-Prudencio, O. G., Uresti-Rivera, E. E., et al. (2020). IPA-1 a putative chromatin remodeler/helicase-related protein of Trichoderma virens plays important roles in antibiosis Against Rhizoctonia solani and induction of Arabidopsis systemic disease resistance. Mol. Plant-Microbe Interact. 33, 808–824. doi: 10.1094/MPMI-04-19-0092-R
Flores, A., Chet, I., and Herrera-Estrella, A. (1997). Improved biocontrol activity of Trichoderma harzianum by over-expression of the proteinase-encoding gene prb1. Curr. Genet. 31, 30–37. doi: 10.1007/s002940050173
Gajera, H. P., Hirpara, D. G., Katakpara, Z. A., Patel, S. V., and Golakiya, B. A. (2016). Molecular evolution and phylogenetic analysis of biocontrol genes acquired from SCoT polymorphism of mycoparasitic Trichoderma koningii inhibiting phytopathogen Rhizoctonia solani Kuhn. Infect. Genet. Evol. 45, 383–392. doi: 10.1016/j.meegid.2016.09.026
Geremia, R. A., Goldman, G. H., Jacobs, D., Ardrtes, W., Vila, S. B., Van Montagu, M., et al. (1993). Molecular characterization of the proteinase-encoding gene, prb1, related to mycoparasitism by Trichoderma harzianum. Mol. Microbiol. 8, 603–613. doi: 10.1111/j.1365-2958.1993.tb01604.x
Goldman, M. H. S., and Goldman, G. H. (1998). Trichoderma harzianum transformant has high extracellular alkaline proteinase expression during specific mycoparasitic interactions. Genet. Mol. Biol. 21, 1–6.
Guo, R., Ji, S., Wang, Z., Zhang, H., Wang, Y., and Liu, Z. (2021). Trichoderma asperellum xylanases promote growth and induce resistance in poplar. Microbiol. Res. 248:126767. doi: 10.1016/j.micres.2021.126767
Guo, W., Zhao, J., Li, X., Qin, L., Yan, X., and Liao, H. (2011). A soybean β-expansin gene GmEXPB2 intrinsically involved in root system architecture responses to abiotic stresses. Plant J. 66, 541–552. doi: 10.1111/j.1365-313X.2011.04511.x
Guzmán-Guzmán, P., Alemán-Duarte, M. I., Delaye, L., Herrera-Estrella, A., and Olmedo-Monfil, V. (2017). Identification of effector-like protein in Trichoderma spp. and role of a hydrophobin in the plant-fungus interaction and mycoparasitism. BMC Genet. 18, 1–20. doi: 10.1186/s12863-017-0481-y
Guzmán-Guzmán, P., Porras-Troncoso, M. D., Olmedo-Monfil, V., and Herrera-Estrella, A. (2019). Trichoderma species: versatile plant Symbionts. Phytopathology 109, 6–16. doi: 10.1094/PHYTO-07-18-0218-RVW
Haggag, W. M., Kansoh, A. L., and Aly, A. M. (2006). Proteases from Talaromyces flavus and Trichoderma harzianum: purification, characterization and antifungal activity against brown spot disease on faba bean. Plant Pathol. Bull. 15, 231–239.
Halifu, S., Deng, X., Song, X., Song, R., and Liang, X. (2020). Inhibitory mechanism of Trichoderma virens ZT05 on Rhizoctonia solani. Plan. Theory 9:912. doi: 10.3390/plants9070912
Harman, G. E., Howell, C. R., Viterbo, A., Chet, I., and Lorito, M. (2004). Trichoderma species—opportunistic, avirulent plant symbionts. Nat. Rev. Microbiol. 2, 43–56. doi: 10.1038/nrmicro797
Heflish, A. A., Abdelkhalek, A., Al-Askar, A. A., and Behiry, S. I. (2021). Protective and curative effects of Trichoderma asperelloides Ta41 on tomato root rot caused by Rhizoctonia solani. Agronomy 11, 1162–1166. doi: 10.3390/agronomy11061162
Herrera, W., Valbuena, O., and Pavone-Maniscalco, D. (2020). Formulation of Trichoderma asperellum TV190 for biological control of Rhizoctonia solani on corn seedlings. Egypt. J. Biol. Pest Control 30, 1–8. doi: 10.1186/s41938-020-00246-9
Herrera-Estrella, A. (1997). Improved biocontrol activity of Trichoderma harzianum by over-expression of the proteinase-encoding. Curr. Genet. 31, 30–37. doi: 10.1007/s002940050173
Howell, C. R. (2003). Mechanisms employed by Trichoderma species in the biological control of plant diseases: the history and evolution of current concepts. Plant Dis. 87, 4–10. doi: 10.1094/PDIS.2003.87.1.4
Inglis, P. W., Pappas, M. D. C. R., Resende, L. V., and Grattapaglia, D. (2018). Fast and inexpensive protocols for consistent extraction of high quality DNA and RNA from challenging plant and fungal samples for high-throughput SNP genotyping and sequencing applications. PLoS One 13:e0206085. doi: 10.1371/journal.pone.0206085
Jaklitsch, W. M. (2009). European species of Hypocrea part I. The green-spored species. Stud. Mycol. 63, 1–91. doi: 10.3114/sim.2009.63.01
Kashyap, P. L., Solanki, M. K., Kushwaha, P., Kumar, S., and Srivastava, A. K. (2020). Biocontrol potential of salt-tolerant Trichoderma and Hypocrea isolates for the management of tomato root rot under saline environment. J. Soil Sci. Plant Nutr. 20, 160–176. doi: 10.1007/s42729-019-00114-y
Kaur, R., Kalia, A., Lore, J. S., Kaur, A., Yadav, I., Sharma, P., et al. (2021). Trichoderma sp. endochitinase and β-1,3-glucanase impede Rhizoctonia solani growth independently, and their combined use does not enhance impediment. Plant Pathol. 70, 1388–1396. doi: 10.1111/ppa.13381
Kaziro, Y., Itoh, H., Kozasa, T., Nakafuku, M., and Satoh, T. (1991). Structure and function of signal-transducing GTP-binding proteins. Annu. Rev. Biochem. 60, 349–400. doi: 10.1146/annurev.bi.60.070191.002025
Khadka, R. B., and Miller, S. A. (2021). Synergy of anaerobic soil disinfestation and Trichoderma spp. in Rhizoctonia root rot suppression. Front. Sustain. Food Syst. 5:645–736. doi: 10.3389/fsufs.2021.645736
Kim, D.-J., Baek, J.-M., Uribe, P., Kenerley, C. M., and Cook, D. R. (2002). Cloning and characterization of multiple glycosyl hydrolase genes from Trichoderma virens. Curr. Genet. 40, 374–384. doi: 10.1007/s00294-001-0267-6
Kotasthane, A., Agrawal, T., Kushwah, R., and Rahatkar, O. V. (2015). In-vitro antagonism of Trichoderma spp. against Sclerotium rolfsii and Rhizoctonia solani and their response towards growth of cucumber, bottle gourd and bitter gourd. Eur. J. Plant Pathol. 141, 523–543. doi: 10.1007/s10658-014-0560-0
Kubicek, C. P., Herrera-Estrella, A., Seidl-Seiboth, V., Martinez, D. A., Druzhinina, I. S., Thon, M., et al. (2011). Comparative genome sequence analysis underscores mycoparasitism as the ancestral life style of Trichoderma. Genome Biol. 12, 1–15. doi: 10.1186/gb-2011-12-4-r40
Kullnig, C., Mach, R. L., Lorito, M., and Kubicek, C. P. (2000). Enzyme diffusion from Trichoderma atroviride (= T. harzianum P1) to Rhizoctonia solani is a prerequisite for triggering of Trichoderma ech42 gene expression before mycoparasitic contact. Appl. Environ. Microbiol. 66, 2232–2234. doi: 10.1128/AEM.66.5.2232-2234.2000
Kumar, V., Parkhi, V., Kenerley, C. M., and Rathore, K. S. (2009). Defense-related gene expression and enzyme activities in transgenic cotton plants expressing an endochitinase gene from Trichoderma virens in response to interaction with Rhizoctonia solani. Planta 230, 277–291. doi: 10.1007/s00425-009-0937-z
Leonetti, P., Zonno, M. C., Molinari, S., and Altomare, C. (2017). Induction of SA-signaling pathway and ethylene biosynthesis in Trichoderma harzianum-treated tomato plants after infection of the root-knot nematode Meloidogyne incognita. Plant Cell Rep. 36, 621–631. doi: 10.1007/s00299-017-2109-0
Li, H. R., Wu, B. C., and Yan, S. Q. (1998). Aetiology of Rhizoctonia in sheath blight of maize in Sichuan. Plant Pathol. 47, 16–21. doi: 10.1046/j.1365-3059.1998.00201.x
Lima, L. H. C., Ulhoa, C. J., Fernandes, A. P., and Felix, C. R. (1997). Purification of a chitinase from Trichoderma sp. and its action on Scierotium rolfsii and Rhizoctonia solani cell walls. J. Gen. Appl. Microbiol. 43, 31–37. doi: 10.2323/jgam.43.31
Limón, M. C., Chacón, M. R., Mejías, R., Delgado-Jarana, J., Rincón, A. M., Codón, A. C., et al. (2004). Increased antifungal and chitinase specific activities of Trichoderma harzianum CECT 2413 by addition of a cellulose binding domain. Appl. Microbiol. Biotechnol. 64, 675–685. doi: 10.1007/s00253-003-1538-6
Limón, M. C., Lora, J. M., García, I., de la Cruz, J., Llobell, A., Benítez, T., et al. (1995). Primary structure and expression pattern of the 33-kDa chitinase gene from the mycoparasitic fungus Trichoderma harzianum. Curr. Genet. 28, 478–483. doi: 10.1007/BF00310819
Liu, M., Liu, J., and Wang, W. M. (2012). Isolation and functional analysis of Thmfs1, the first major facilitator superfamily transporter from the biocontrol fungus Trichoderma harzianum. Biotechnol. Lett. 34, 1857–1862. doi: 10.1007/s10529-012-0972-x
Liu, Y., and Yang, Q. (2007). Cloning and heterologous expression of aspartic protease SA76 related to biocontrol in Trichoderma harzianum. FEMS Microbiol. Lett. 277, 173–181. doi: 10.1111/j.1574-6968.2007.00952.x
Liu, Z., Yang, X., Sun, D., Song, J., Chen, G., Juba, O., et al. (2010). Expressed sequence tags-based identification of genes in a biocontrol strain Trichoderma asperellum. Mol. Biol. Rep. 37, 3673–3681. doi: 10.1007/s11033-010-0019-0
Lorito, M., Woo, S. L., Fernandez, I. G., Colucci, G., Harman, G. E., Pintor-Toro, J. A., et al. (1998). Genes from mycoparasitic fungi as a source for improving plant resistance to fungal pathogens. Proc. Natl. Acad. Sci. 95, 7860–7865. doi: 10.1073/pnas.95.14.7860
Mahr, N. A. (2021). Comparative efficacy of ten commercial fungicides for the control of Rhizoctonia solani, the cause of black scurf disease of potato. Plant Prot. 5, 149–156. doi: 10.33804/pp.005.03.3930
Malmierca, M. G., Cardoza, R. E., Alexander, N. J., McCormick, S. P., Collado, I. G., Hermosa, R., et al. (2013). Relevance of trichothecenes in fungal physiology: disruption of tri5 in Trichoderma arundinaceum. Fungal Genet. Biol. 53, 22–33. doi: 10.1016/j.fgb.2013.02.001
Malmierca, M. G., Cardoza, R. E., Alexander, N. J., McCormick, S. P., Hermosa, R., Monte, E., et al. (2012). Involvement of Trichoderma trichothecenes in the biocontrol activity and induction of plant defense-related genes. Appl. Environ. Microbiol. 78, 4856–4868. doi: 10.1128/AEM.00385-12
Małolepsza, U., Nawrocka, J., and Szczech, M. (2017). Trichoderma virens 106 inoculation stimulates defence enzyme activities and enhances phenolic levels in tomato plants leading to lowered Rhizoctonia solani infection. Biocontrol Sci. Tech. 27, 180–199. doi: 10.1080/09583157.2016.1264570
Manganiello, G., Sacco, A., Ercolano, M. R., Vinale, F., Lanzuise, S., Pascale, A., et al. (2018). Modulation of tomato response to Rhizoctonia solani by Trichoderma harzianum and its secondary metabolite harzianic acid. Front. Microbiol. 9:1966. doi: 10.3389/fmicb.2018.01966
Marcello, C. M., Steindorff, A. S., da Silva, S. P., do Nascimento Silva, R., Bataus, L. A. M., and Ulhoa, C. J. (2010). Expression analysis of the exo-β-1, 3-glucanase from the mycoparasitic fungus Trichoderma asperellum. Microbiol. Res. 165, 75–81. doi: 10.1016/j.micres.2008.08.002
Mata-Essayag, S., Magaldi, S., Hartung de Capriles, C., Deibis, L., Verde, G., and Perez, C. (2001). “In vitro” antifungal activity of protease inhibitors. Mycopathologia 152, 135–142. doi: 10.1023/a:1013175706355
Mayo, S., Cominelli, E., Sparvoli, F., González-López, O., Rodríguez-González, A., Gutiérrez, S., et al. (2016). Development of a qPCR strategy to select bean genes involved in plant defense response and regulated by the Trichoderma velutinum–Rhizoctonia solani interaction. Front. Plant Sci. 7:1109. doi: 10.3389/fpls.2016.01109
Mayo, S., Gutierrez, S., Malmierca, M., Lorenzana, A., Campelo, M., Hermosa, R., et al. (2015). Influence of Rhizoctonia solani and Trichoderma spp. in growth of bean (Phaseolus vulgaris L.) and in the induction of plant defense-related genes. Front. Plant Sci. 6:685. doi: 10.3389/fpls.2015.00685
McDonagh, A., Fedorova, N. D., Crabtree, J., Yu, Y., Kim, S., Chen, D., et al. (2008). Sub-telomere directed gene expression during initiation of invasive aspergillosis. PLoS Pathog. 4:e1000154. doi: 10.1371/journal.ppat.1000154
Mendoza-Mendoza, A., Pozo, M. J., Grzegorski, D., Martínez, P., García, J. M., Olmedo-Monfil, V., et al. (2003). Enhanced biocontrol activity of Trichoderma through inactivation of a mitogen-activated protein kinase. Proc. Natl. Acad. Sci. 100, 15965–15970. doi: 10.1073/pnas.2136716100
Morán-Diez, E., Hermosa, R., Ambrosino, P., Cardoza, R. E., Gutiérrez, S., Lorito, M., et al. (2009). The ThPG1 endopolygalacturonase is required for the Trichoderma harzianum–plant beneficial interaction. Mol. Plant-Microbe Interact. 22, 1021–1031. doi: 10.1094/MPMI-22-8-1021
Morán-Diez, M. E., Martínez de Alba, Á. E., Rubio, M. B., Hermosa, R., and Monte, E. (2021). Trichoderma and the plant heritable priming responses. J. Fungi 7:318. doi: 10.3390/jof7040318
Moreno-Mateos, M. A., Delgado-Jarana, J., Codon, A. C., and Benítez, T. (2007). pH and Pac1 control development and antifungal activity in Trichoderma harzianum. Fungal Genet. Biol. 44, 1355–1367. doi: 10.1016/j.fgb.2007.07.012
Mukherjee, P. K., Buensanteai, N., Moran-Diez, M. E., Druzhinina, I. S., and Kenerley, C. M. (2012). Functional analysis of non-ribosomal peptide synthetases (NRPSs) in Trichoderma virens reveals a polyketide synthase (PKS)/NRPS hybrid enzyme involved in the induced systemic resistance response in maize. Microbiology 158, 155–165. doi: 10.1099/mic.0.052159-0
Mukherjee, P. K., and Kenerley, C. M. (2010). Regulation of morphogenesis and biocontrol properties in Trichoderma virens by a VELVET protein, Vel1. Appl. Environ. Microbiol. 76, 2345–2352. doi: 10.1128/AEM.02391-09
Mukherjee, P. K., Latha, J., Hadar, R., and Horwitz, B. A. (2003). TmkA, a mitogen-activated protein kinase of Trichoderma virens, is involved in biocontrol properties and repression of conidiation in the dark. Eukaryot. Cell 2, 446–455. doi: 10.1128/EC.2.3.446-455.2003
Mukherjee, M., Mukherjee, P. K., and Kale, S. P. (2007). cAMP signalling is involved in growth, germination, mycoparasitism and secondary metabolism in Trichoderma virens. Microbiology 153, 1734–1742. doi: 10.1099/mic.0.2007/005702-0
Mukherjee, P. K., Wiest, A., Ruiz, N., Keightley, A., Moran-Diez, M. E., McCluskey, K., et al. (2011). Two classes of new peptaibols are synthesized by a single non-ribosomal peptide synthetase of Trichoderma virens. J. Biol. Chem. 286, 4544–4554. doi: 10.1074/jbc.M110.159723
Naeimi, S., Khosravi, V., Varga, A., Vágvölgyi, C., and Kredics, L. (2020). Screening of organic substrates for solid-state fermentation, viability and bioefficacy of Trichoderma harzianum AS12-2, a biocontrol strain Against Rice sheath blight disease. Agronomy 10, 1–15. doi: 10.3390/agronomy10091258
Nawrocka, J., Gromek, A., and Małolepsza, U. (2019). Nitric oxide as a beneficial signaling molecule in Trichoderma atroviride TRS25-induced systemic defense responses of cucumber plants Against Rhizoctonia solani. Front. Plant Sci. 10:421. doi: 10.3389/fpls.2019.00421
Nawrocka, J., and Małolepsza, U. (2013). Diversity in plant systemic resistance induced by Trichoderma. Biol. Control 67, 149–156. doi: 10.1016/j.biocontrol.2013.07.005
Nawrocka, J., Małolepsza, U., Szymczak, K., and Szczech, M. (2018). Involvement of metabolic components, volatile compounds, PR proteins, and mechanical strengthening in multilayer protection of cucumber plants against Rhizoctonia solani activated by Trichoderma atroviride TRS25. Protoplasma 255, 359–373. doi: 10.1007/s00709-017-1157-1
Nerey, Y., Pannecoucque, J., Hernandez, H. P., Diaz, M., Espinosa, R., De Vos, S., et al. (2010). Rhizoctonia spp. causing root and hypocotyl rot in Phaseolus vulgaris in Cuba. J. Phytopathol. 158, 236–243. doi: 10.1111/j.1439-0434.2009.01609.x
Niu, X., Thaochan, N., and Hu, Q. (2020). Diversity of linear non-ribosomal peptide in biocontrol fungi. J. Fungi 6:61. doi: 10.3390/jof6020061
Noronha, E. F., Kipnis, A., Junqueira-Kipnis, A. P., and Ulhoa, C. J. (2000). Regulation of 36-kDa β-1, 3-glucanase synthesis in Trichoderma harzianum. FEMS Microbiol. Lett. 188, 19–22. doi: 10.1111/j.1574-6968.2000.tb09162.x
Ohkura, M., Abawi, G. S., Smart, C. D., and Hodge, K. T. (2009). Diversity and aggressiveness of Rhizoctonia solani and Rhizoctonia-like fungi on vegetables in New York. Plant Dis. 93, 615–624. doi: 10.1094/PDIS-93-6-0615
Omann, M. R., Lehner, S., Rodríguez, C. E., Brunner, K., and Zeilinger, S. (2012). The seven-transmembrane receptor Gpr1 governs processes relevant for the antagonistic interaction of Trichoderma atroviride with its host. Microbiology 158:107. doi: 10.1099/mic.0.052035-0
Oszust, K., Cybulska, J., and Frąc, M. (2020). How do Trichoderma genus fungi win a nutritional competition battle against soft fruit pathogens? A report on niche overlap nutritional potentiates. Int. J. Mol. Sci. 21:4235. doi: 10.3390/ijms21124235
Patil, H. J., and Solanki, M. K. (2016). “Molecular prospecting: advancement in diagnosis and control of Rhizoctonia solani diseases in plants,” in Current Trends in Plant Disease Diagnostics and Management Practices. eds. V. K. Gupta and M. G. Tuohy (Cham: Springer), 165–185.
Pieterse, C. M. J., Leon-Reyes, A., Van der Ent, S., and Van Wees, S. C. M. (2009). Networking by small-molecule hormones in plant immunity. Nat. Chem. Biol. 5, 308–316. doi: 10.1038/nchembio.164
Pieterse, C. M. J., Zamioudis, C., Berendsen, R. L., Weller, D. M., Van Wees, S. C. M., and Bakker, P. A. H. M. (2014). Induced systemic resistance by beneficial microbes. Annu. Rev. Phytopathol. 52, 347–375. doi: 10.1146/annurev-phyto-082712-102340
Pozo, M. A. J., Baek, J.-M., García, J. M., and Kenerley, C. M. (2004). Functional analysis of tvsp1, a serine protease-encoding gene in the biocontrol agent Trichoderma virens. Fungal Genet. Biol. 41, 336–348. doi: 10.1016/j.fgb.2003.11.002
Rahimi Tamandegani, P., Marik, T., Zafari, D., Balázs, D., Vágvölgyi, C., Szekeres, A., et al. (2020). Changes in peptaibol production of Trichoderma species during in vitro antagonistic interactions with fungal plant pathogens. Biomol. Ther. 10:730.
Rai, S., Solanki, M. K., Solanki, A. C., and Surapathrudu, K. (2019). “Biocontrol potential of Trichoderma spp.: current understandings and future outlooks on molecular techniques,” in Plant Health Under Biotic Stress (Singapore: Springer), 129–160.
Ramírez-Valdespino, C. A., Porras-Troncoso, M. D., Corrales-Escobosa, A. R., Wrobel, K., Martínez-Hernández, P., and Olmedo-Monfil, V. (2018). Functional characterization of TvCyt2, a member of the p450 monooxygenases from Trichoderma virens relevant during the association with plants and mycoparasitism. Mol. Plant-Microbe Interact. 31, 289–298. doi: 10.1094/MPMI-01-17-0015-R
Reithner, B., Brunner, K., Schuhmacher, R., Peissl, I., Seidl, V., Krska, R., et al. (2005). The G protein α subunit Tga1 of Trichoderma atroviride is involved in chitinase formation and differential production of antifungal metabolites. Fungal Genet. Biol. 42, 749–760. doi: 10.1016/j.fgb.2005.04.009
Reithner, B., Ibarra-Laclette, E., Mach Robert, L., and Herrera-Estrella, A. (2011). Identification of Mycoparasitism-related genes in Trichoderma atroviride. Appl. Environ. Microbiol. 77, 4361–4370. doi: 10.1128/AEM.00129-11
Reithner, B., Mach-Aigner, A. R., Herrera-Estrella, A., and Mach, R. L. (2014). The transcriptional regulator Xyr1 of Trichoderma atroviride supports the induction of systemic resistance in plants. Appl. Environ. Microbiol. 80, 5274–5281.
Reithner, B., Schuhmacher, R., Stoppacher, N., Pucher, M., Brunner, K., and Zeilinger, S. (2007). Signaling via the Trichoderma atroviride mitogen-activated protein kinase Tmk1 differentially affects mycoparasitism and plant protection. Fungal Genet. Biol. 44, 1123–1133. doi: 10.1016/j.fgb.2007.04.001
Roby, D., Broglie, K., Cressman, R., Biddle, P., Chet, I. L., and Broglie, R. (1990). Activation of a bean chitinase promoter in transgenic tobacco plants by phytopathogenic fungi. Plant Cell 2, 999–1007. doi: 10.2307/3869239
Rocha-Ramírez, V., Omero, C., Chet, I., Horwitz, B. A., and Herrera-Estrella, A. (2002). Trichoderma atroviride G-protein α-subunit gene tga1 is involved in mycoparasitic coiling and conidiation. Eukaryot. Cell 1, 594–605. doi: 10.1128/EC.1.4.594-605.2002
Rubio, M. B., Hermosa, R., Reino, J. L., Collado, I. G., and Monte, E. (2009). Thctf1 transcription factor of Trichoderma harzianum is involved in 6-pentyl-2H-pyran-2-one production and antifungal activity. Fungal Genet. Biol. 46, 17–27. doi: 10.1016/j.fgb.2008.10.008
Ruocco, M., Lanzuise, S., Vinale, F., Marra, R., Turrà, D., Woo, S. L., et al. (2009). Identification of a new biocontrol gene in Trichoderma atroviride: the role of an ABC transporter membrane pump in the interaction with different plant-pathogenic fungi. Mol. Plant-Microbe Interact. 22, 291–301. doi: 10.1094/MPMI-22-3-0291
Salas-Marina, M. A., Silva-Flores, M. A., Uresti-Rivera, E. E., Castro-Longoria, E., Herrera-Estrella, A., and Casas-Flores, S. (2011). Colonization of Arabidopsis roots by Trichoderma atroviride promotes growth and enhances systemic disease resistance through jasmonic acid/ethylene and salicylic acid pathways. Eur. J. Plant Pathol. 131, 15–26. doi: 10.1007/s10658-011-9782-6
Saloheimo, M., Paloheimo, M., Hakola, S., Pere, J., Swanson, B., Nyyssönen, E., et al. (2002). Swollenin, a Trichoderma reesei protein with sequence similarity to the plant expansins, exhibits disruption activity on cellulosic materials. Eur. J. Biochem. 269, 4202–4211. doi: 10.1046/j.1432-1033.2002.03095.x
Sami, F., Faizan, M., Faraz, A., Siddiqui, H., Yusuf, M., and Hayat, S. (2018). Nitric oxide-mediated integrative alterations in plant metabolism to confer abiotic stress tolerance, NO crosstalk with phytohormones and NO-mediated post translational modifications in modulating diverse plant stress. Nitric Oxide 73, 22–38. doi: 10.1016/j.niox.2017.12.005
Sánchez-Cruz, R., Mehta, R., Atriztán-Hernández, K., Martínez-Villamil, O., del Rayo Sánchez-Carbente, M., Sánchez-Reyes, A., et al. (2021). Effects on Capsicum annuum plants colonized with Trichoderma atroviride P. karst strains genetically modified in Taswo1, a gene coding for a protein with Expansin-like activity. Plan. Theory 10:1919.
Sarma, B. K., Yadav, S. K., Patel, J. S., and Singh, H. B. (2014). Molecular mechanisms of interactions of Trichoderma with other fungal species. Open Mycol. J. 8, 140–147. doi: 10.2174/1874437001408010140
Sarrocco, S., Guidi, L., Fambrini, S., Degl'Innocenti, E., and Vannacci, G. (2009). Competition for cellulose exploitation between Rhizoctonia solani and two Trichoderma isolates in the decomposition of wheat straw. J. Plant Pathol. 91, 331–338. doi: 10.4454/jpp.v91i2.962
Schmoll, M., Dattenböck, C., Carreras-Villaseñor, N., Mendoza-Mendoza, A., Tisch, D., Alemán, M. I., et al. (2016). The genomes of three uneven siblings: footprints of the lifestyles of three Trichoderma species. Microbiol. Mol. Biol. Rev. 80, 205–327. doi: 10.1128/MMBR.00040-15
Segreto, R., Bazafkan, H., Millinger, J., Schenk, M., Atanasova, L., Doppler, M., et al. (2021). The TOR kinase pathway is relevant for nitrogen signaling and antagonism of the mycoparasite Trichoderma atroviride. PLoS One 16:e0262180. doi: 10.1371/journal.pone.0262180
Seidl, V., Marchetti, M., Schandl, R., Allmaier, G., and Kubicek, C. P. (2006). Epl1, the major secreted protein of Hypocrea atroviridis on glucose, is a member of a strongly conserved protein family comprising plant defense response elicitors. FEBS J. 273, 4346–4359. doi: 10.1111/j.1742-4658.2006.05435.x
Seidl, V., Song, L., Lindquist, E., Gruber, S., Koptchinskiy, A., Zeilinger, S., et al. (2009). Transcriptomic response of the mycoparasitic fungus Trichoderma atroviride to the presence of a fungal prey. BMC Genomics 10, 1–13.
Shah, J. M., Raghupathy, V., and Veluthambi, K. (2009). Enhanced sheath blight resistance in transgenic rice expressing an endochitinase gene from Trichoderma virens. Biotechnol. Lett. 31, 239–244. doi: 10.1007/s10529-008-9856-5
Sharma, R., and Bhat, S. (2011). Molecular cloning of endochitinase 33 (ECH33) gene from Trichoderma harzanium. Afr. J. Biotechnol. 10, 12156–12163.
Singh, R. K., Jatav, H. S., Lakpale, R., Khan, M., Rajput, V. D., and Minkina, T. (2021). Hydrogel-based trichoderma formulation effects on different varieties of rice under rainfed condition of indo-gangetic plains. Environ. Dev. Sustain. 24, 7035–7056. doi: 10.1007/s10668-021-01738-w
Singh, U. B., Malviya, D., Singh, S., Pradhan, J. K., Singh, B. P., Roy, M., et al. (2016). Bio-protective microbial agents from rhizosphere eco-systems trigger plant defense responses provide protection against sheath blight disease in rice (Oryza sativa L.). Microbiol. Res. 192, 300–312. doi: 10.1016/j.micres.2016.08.007
Sivan, A., and Harman, G. E. (1991). Improved rhizosphere competence in a protoplast fusion progeny of Trichoderma harzianum. Microbiology 137, 23–29. doi: 10.1099/00221287-137-1-23
Solanki, M. K., Singh, N., Singh, R. K., Singh, P., Srivastava, A. K., Kumar, S., et al. (2011). Plant defense activation and management of tomato root rot by a chitin-fortified Trichoderma/Hypocrea formulation. Phytoparasitica 39, 471–481. doi: 10.1007/s12600-011-0188-y
Sood, M., Kapoor, D., Kumar, V., Sheteiwy, M. S., Ramakrishnan, M., Landi, M., et al. (2020). Trichoderma: the “secrets” of a multitalented biocontrol agent. Plants 9:762. doi: 10.3390/plants9060762
Speckbacher, V., Ruzsanyi, V., Martinez-Medina, A., Hinterdobler, W., Doppler, M., Schreiner, U., et al. (2020). The Lipoxygenase Lox1 is involved in light- and injury-response, Conidiation, and volatile organic compound biosynthesis in the Mycoparasitic fungus Trichoderma atroviride. Front. Microbiol. 11:2004. doi: 10.3389/fmicb.2020.02004
Steindorff, A. S., do Nascimento Silva, R., Coelho, A. S. G., Nagata, T., Noronha, E. F., and Ulhoa, C. J. (2012). Trichoderma harzianum expressed sequence tags for identification of genes with putative roles in mycoparasitism against Fusarium solani. Biol. Control 61, 134–140. doi: 10.1016/j.biocontrol.2012.01.014
Stergiopoulos, I., and de Wit, P. J. G. M. (2009). Fungal effector proteins. Annu. Rev. Phytopathol. 47, 233–263. doi: 10.1146/annurev.phyto.112408.132637
Suárez, M. B., Sanz, L., Chamorro, M. I., Rey, M., González, F. J., Llobell, A., et al. (2005). Proteomic analysis of secreted proteins from Trichoderma harzianum: identification of a fungal cell wall-induced aspartic protease. Fungal Genet. Biol. 42, 924–934. doi: 10.1016/j.fgb.2005.08.002
Suriani Ribeiro, M., Graciano de Paula, R., Raquel Voltan, A., de Castro, R. G., Carraro, C. B., José de Assis, L., et al. (2019). Endo-β-1,3-glucanase (GH16 family) from Trichoderma harzianum participates in Cell Wall biogenesis but is not essential for antagonism Against plant pathogens. Biomol. Ther. 9:781. doi: 10.3390/biom9120781
Szekeres, A., Kredics, L., Antal, Z., Kevei, F., and Manczinger, L. (2004). Isolation and characterization of protease overproducing mutants of Trichoderma harzianum. FEMS Microbiol. Lett. 233, 215–222. doi: 10.1111/j.1574-6968.2004.tb09485.x
Talbot, N. J., Kershaw, M. J., Wakley, G. E., De Vries, O. M. H., Wessels, J. G. H., and Hamer, J. E. (1996). MPG1 encodes a fungal hydrophobin involved in surface interactions during infection-related development of Magnaporthe grisea. Plant Cell 8, 985–999. doi: 10.2307/3870210
Trushina, N., Levin, M., Mukherjee, P. K., and Horwitz, B. A. (2013). PacC and pH–dependent transcriptome of the mycotrophic fungus Trichoderma virens. BMC Genomics 14, 1–21. doi: 10.1186/1471-2164-14-138
Van Oosten, V. R., Bodenhausen, N., Reymond, P., Van Pelt, J. A., Van Loon, L. C., Dicke, M., et al. (2008). Differential effectiveness of microbially induced resistance against herbivorous insects in Arabidopsis. Mol. Plant-Microbe Interact. 21, 919–930. doi: 10.1094/MPMI-21-7-0919
Velázquez-Robledo, R., Contreras-Cornejo, H. A., Macias-Rodriguez, L., Hernández-Morales, A., Aguirre, J., Casas-Flores, S., et al. (2011). Role of the 4-phosphopantetheinyl transferase of Trichoderma virens in secondary metabolism and induction of plant defense responses. Mol. Plant-Microbe Interact. 24, 1459–1471. doi: 10.1094/MPMI-02-11-0045
Vey, A., Hoagland, R. E., and Butt, T. M. (2001). “Chapter 12: Toxic metabolites of fungal biocontrol agents,” in Fungi as Biocontrol Agents: Progress, Problems and Potential. eds. T. M. Butt, C. Jackson, and Magan N. 311.
Vinale, F., Nigro, M., Sivasithamparam, K., Flematti, G., Ghisalberti, E. L., Ruocco, M., et al. (2013). Harzianic acid: a novel siderophore from Trichoderma harzianum. FEMS Microbiol. Lett. 347, 123–129. doi: 10.1111/1574-6968.12231
Vinale, F., and Sivasithamparam, K. (2020). Beneficial effects of Trichoderma secondary metabolites on crops. Phytother. Res. 34, 2835–2842. doi: 10.1002/ptr.6728
Viterbo, A., Haran, S., Friesem, D., Ramot, O., and Chet, I. (2001). Antifungal activity of a novel endochitinase gene (chit36) from Trichoderma harzianum Rifai TM. FEMS Microbiol. Lett. 200, 169–174. doi: 10.1111/j.1574-6968.2001.tb10710.x
Viterbo, A., Harel, M., and Chet, I. (2004). Isolation of two aspartyl proteases from Trichoderma asperellum expressed during colonization of cucumber roots. FEMS Microbiol. Lett. 238, 151–158. doi: 10.1016/j.femsle.2004.07.028
Viterbo, A., Harel, M., Horwitz, B. A., Chet, I., and Mukherjee, P. K. (2005). Trichoderma mitogen-activated protein kinase signaling is involved in induction of plant systemic resistance. Appl. Environ. Microbiol. 71, 6241–6246. doi: 10.1128/AEM.71.10.6241-6246.2005
Viterbo, A., Ramot, O., Chernin, L., and Chet, I. (2002). Significance of lytic enzymes from Trichoderma spp. in the biocontrol of fungal plant pathogens. Antonie Van Leeuwenhoek 81, 549–556. doi: 10.1023/A:1020553421740
Wilhite, S. E., Lumsden, R. D., and Straney, D. C. (2001). Peptide synthetase gene in Trichoderma virens. Appl. Environ. Microbiol. 67, 5055–5062. doi: 10.1128/AEM.67.11.5055-5062.2001
Wilson, P. S., Ketola, E. O., Ahvenniemi, P. M., Lehtonen, M. J., and Valkonen, J. P. T. (2008). Dynamics of soilborne Rhizoctonia solani in the presence of Trichoderma harzianum: effects on stem canker, black scurf and progeny tubers of potato. Plant Pathol. 57, 152–161. doi: 10.1111/j.1365-3059.2007.01706.x
Yang, P. (2017). The gene task1 is involved in morphological development, mycoparasitism and antibiosis of Trichoderma asperellum. Biocontrol Sci. Tech. 27, 620–635. doi: 10.1080/09583157.2017.1318824
Yang, C.-A., Cheng, C.-H., Lo, C.-T., Liu, S.-Y., Lee, J.-W., and Peng, K.-C. (2011). A novel L-amino acid oxidase from Trichoderma harzianum ETS 323 associated with antagonism of Rhizoctonia solani. J. Agric. Food Chem. 59, 4519–4526. doi: 10.1021/jf104603w
Yang, X., Cong, H., Song, J., and Zhang, J. (2013). Heterologous expression of an aspartic protease gene from biocontrol fungus Trichoderma asperellum in Pichia pastoris. World J. Microbiol. Biotechnol. 29, 2087–2094. doi: 10.1007/s11274-013-1373-6
Youssef, S. A., Tartoura, K. A., and Abdelraouf, G. A. (2016). Evaluation of Trichoderma harzianum and Serratia proteamaculans effect on disease suppression, stimulation of ROS-scavenging enzymes and improving tomato growth infected by Rhizoctonia solani. Biol. Control 100, 79–86. doi: 10.1016/j.biocontrol.2016.06.001
Yu, Y., Gui, Y., Li, Z., Jiang, C., Guo, J., and Niu, D. (2022). Induced systemic resistance for improving plant immunity by beneficial microbes. Annu. Rev. Phytopathol. 11, 1–19. doi: 10.3390/plants11030386
Zeilinger, S., and Atanasova, L. (2020). “Sensing and regulation of mycoparasitism-relevant processes in Trichoderma,” in New and Future Developments in Microbial Biotechnology and Bioengineering Recent Developments in Trichoderma Research. ed. V. K. Gupta (Elsevier), 39–55.
Zeilinger, S., Galhaup, C., Payer, K., Woo, S. L., Mach, R. L., Fekete, C., et al. (1999). Chitinase gene expression during Mycoparasitic interaction of Trichoderma harzianum with its host. Fungal Genet. Biol. 26, 131–140.
Zeilinger, S., Gruber, S., Bansal, R., and Mukherjee, P. K. (2016). Secondary metabolism in Trichoderma–chemistry meets genomics. Fungal Biol. Rev. 30, 74–90. doi: 10.1016/j.fbr.2016.05.001
Zeilinger, S., and Omann, M. (2007). Trichoderma biocontrol: signal transduction pathways involved in host sensing and mycoparasitism. Gene Regul. Syst. Biol. 1, 227–234. doi: 10.4137/grsb.s397
Zeilinger, S., Reithner, B., Scala, V., Peissl, I., Lorito, M., and Mach, R. L. (2005). Signal transduction by Tga3, a novel G protein α subunit of Trichoderma atroviride. Appl. Environ. Microbiol. 71, 1591–1597. doi: 10.1128/AEM.71.3.1591-1597.2005
Keywords: Trichoderma spp., genes, R. solani, antibiosis, competition, mycoparasitism, induced systemic resistance
Citation: Abbas A, Mubeen M, Zheng H, Sohail MA, Shakeel Q, Solanki MK, Iftikhar Y, Sharma S, Kashyap BK, Hussain S, del Carmen Zuñiga Romano M, Moya-Elizondo EA and Zhou L (2022) Trichoderma spp. Genes Involved in the Biocontrol Activity Against Rhizoctonia solani. Front. Microbiol. 13:884469. doi: 10.3389/fmicb.2022.884469
Edited by:
Shekhar Jain, Mandsaur University, IndiaReviewed by:
Tariq Mukhtar, Pir Mehr Ali Shah Arid Agriculture University, PakistanVishnu D. Rajput, Southern Federal University, Russia
Copyright © 2022 Abbas, Mubeen, Zheng, Sohail, Shakeel, Solanki, Iftikhar, Sharma, Kashyap, Hussain, del Carmen Zuñiga Romano, Moya-Elizondo and Zhou. This is an open-access article distributed under the terms of the Creative Commons Attribution License (CC BY). The use, distribution or reproduction in other forums is permitted, provided the original author(s) and the copyright owner(s) are credited and that the original publication in this journal is cited, in accordance with accepted academic practice. No use, distribution or reproduction is permitted which does not comply with these terms.
*Correspondence: Yasir Iftikhar, yasir.iftikhar@uos.edu.pk; Lei Zhou, zhoul@zaas.ac.cn