- 1Institute of Plant and Microbial Biology, Academia Sinica, Taipei, Taiwan
- 2Molecular and Biological Agricultural Sciences Program, Taiwan International Graduate Program, National Chung-Hsing University and Academia Sinica, Taipei, Taiwan
- 3Graduate Institute of Biotechnology, National Chung-Hsing University, Taichung, Taiwan
- 4Aquaculture Research and Development Division, Department of Agriculture-National Fisheries Research and Development Institute (DA-NFRDI), Manila, Philippines
- 5Institute of Biological Chemistry, Academia Sinica, Taipei, Taiwan
- 6Biotechnology Center, National Chung-Hsing University, Taichung, Taiwan
The type VI secretion system (T6SS) assembles into a contractile nanomachine to inject effectors across bacterial membranes for secretion. The Agrobacterium tumefaciens species complex is a group of soil inhabitants and phytopathogens that deploys T6SS as an antibacterial weapon against bacterial competitors at both inter-species and intra-species levels. The A. tumefaciens strain 1D1609 genome encodes one main T6SS gene cluster and four vrgG genes (i.e., vgrGa-d), each encoding a spike protein as an effector carrier. A previous study reported that vgrGa-associated gene 2, named v2a, encodes a His-Me finger nuclease toxin (also named HNH/ENDO VII nuclease), contributing to DNase-mediated antibacterial activity. However, the functions and roles of other putative effectors remain unknown. In this study, we identified vgrGc-associated gene 2 (v2c) that encodes another His-Me finger nuclease but with a distinct Serine Histidine Histidine (SHH) motif that differs from the AHH motif of V2a. We demonstrated that the ectopic expression of V2c caused growth inhibition, plasmid DNA degradation, and cell elongation in Escherichia coli using DNAse activity assay and fluorescence microscopy. The cognate immunity protein, V3c, neutralizes the DNase activity and rescues the phenotypes of growth inhibition and cell elongation. Ectopic expression of V2c DNase-inactive variants retains the cell elongation phenotype, while V2a induces cell elongation in a DNase-mediated manner. We also showed that the amino acids of conserved SHH and HNH motifs are responsible for the V2c DNase activity in vivo and in vitro. Notably, V2c also mediated the DNA degradation and cell elongation of the target cell in the context of interbacterial competition. Importantly, V2a and V2c exhibit different capacities against different bacterial species and function synergistically to exert stronger antibacterial activity against the soft rot phytopathogen, Dickeya dadantii.
Introduction
The type VI secretion system (T6SS) is a nanomachine used by many Gram-negative bacteria for antagonism or pathogenesis by injecting toxins into target bacterial or eukaryotic cells (Hachani et al., 2016; Hood et al., 2017; Coulthurst, 2019). T6SS is composed of a membrane complex in connection to a baseplate, which is the docking site for the polymerization of a tube surrounded by a contractile sheath (Wang et al., 2019). The tube is a puncturing device stacked of a Hcp protein hexamer tipped with the trimeric valine–glycine repeat protein G (VgrG) spike and a PAAR repeat-containing protein, which sharpens the tip (Shneider et al., 2013). When the sheath contracts, the puncturing device carrying effectors is propelled toward the target cell to deliver effectors. After firing, the sheath disassembles, and the subunits of the sheath are recycled to build another machine (Basler et al., 2012).
In terms of delivery, the T6SS effectors can be classified as cargo or specialized effectors. The cargo effectors interact non-covalently with Hcp, VgrG, or PAAR, while in specialized effectors, the effector domain is covalently fused to Hcp, VgrG, or PAAR (Cianfanelli et al., 2016). Functionally, most of the identified effectors mediate antibacterial activities (e.g., nuclease, peptidoglycan hydrolase, lipase, phospholipase, NAD (P) + −glycohydrolase, and ADP-ribosyltransferase) by targeting conserved cellular structures such as nucleic acids, peptidoglycan, and the inner membrane, as well as specific metabolites or proteins (Lien and Lai, 2017; Cherrak et al., 2019).
To date, the characterized T6SS nuclease effectors include members of the HNH, N-Tox, Tox-Rease, and PoNe superfamilies and were shown to target DNA substrates without specificity (Ma et al., 2014, 2017; Fitzsimons et al., 2018; Pissaridou et al., 2018; Fridman et al., 2020; Pei et al., 2020; Santos et al., 2020). Thus, the expression of these T6SS nuclease effectors causes DNA degradation in vitro and/or in vivo. Interestingly, the majority of T6SS DNase effectors belong to the HNH/ENDO VII nuclease superfamily, also known as the His-Me finger endonuclease superfamily, consisting of 38 distinct families (Jablonska et al., 2017; Wu et al., 2020). These nuclease superfamily proteins harbor three conserved His-Asp-His within an approximately 30-amino acid domains formed by a β1 hairpin followed by an α-helix to form a ββα-metal topology for DNA binding and hydrolysis (Jablonska et al., 2017; Wu et al., 2020). Several T6SS effectors belonging to this HNH/His-Me superfamily, including Tse7 of Pseudomonas aeruginosa as Tox-GHH2 (Pissaridou et al., 2018) and Tse1 of Aeromonas dhakensis (Pei et al., 2020), V2a of Agrobacterium tumefaciens as Tox-AHH (Santos et al., 2020), and two Tox-SHH family effectors, Tke4 of Pseudomonas putida (Bernal et al., 2017) and Txe4 of the fish pathogen Pseudomonas plecoglossicida (Li et al., 2022). Amino acid substitution experiments on Tse7, V2a, and Tse1 have indicated that this conserved catalytic site [A/G] HH is required for nuclease activity and toxicity (Pissaridou et al., 2018; Pei et al., 2020; Santos et al., 2020). However, the impacts of SHH and HNH motifs of the Tox-SHH family effectors on nuclease activity and T6SS toxicity have not been demonstrated.
Agrobacterium tumefaciens is a Gram-negative Alphaproteobacteria belonging to the Rhizobiaceae family. It is an economically important pathogen that causes crown gall disease and a gene delivery tool with the capability of transforming plant cells and fungi (Hwang et al., 2017). The T6SS main gene cluster is highly conserved in the genome of the A. tumefaciens genomospecies complex (Wu et al., 2019, 2021; Chou et al., 2022). Besides the main cluster consisting of two divergent operons, imp (impaired in nitrogen fixation) encode core structural and regulatory components and hcp operon for effectors and effector delivery components (e.g., Hcp, VgrG, and PAAR). Most A. tumefaciens strains also encode additional vgrG operons encoding effector-immunity (EI) gene pairs. The A. tumefaciens strain 1D1609 encodes four vgrG genes (vgrGa, vgrGb, vgrGc, and vgrGd) with each vgrG module associated with three to four associated genes, named as vgrG-associated genes 1–4 in cluster a/b/c/d (Santos et al., 2020). Each vgrG gene is genetically linked to a conserved chaperone/adaptor gene and different known or putative EI pairs, including three effectors with a conserved N-terminal PAAR-like DUF4150 domain but distinct C-terminal effector domains (V2a, V2c, and V2d) (Santos et al., 2020; Supplementary Figure S1). For the vgrGb module, V2b does not encode an effector domain but downstream two genes encode a putative ADP-ribosylating enzyme (V3b) and a Rhs-linked effector domain (V4b), followed by putative immunity. A previous study by deleting single or multiple EI pairs suggested that vgrGa-associated effector V2a, a DNase effector harboring the C-terminal Tox-AHH domain, appears to be the major antibacterial toxin, but the vgrGd-associated effector V2d also contributes to full antibacterial activity for 1D1609 against E. coli prey (Santos et al., 2020). The biochemical and biological functions of other putative effectors encoded in the other three orphan vgrG modules remain uncharacterized.
In this study, we discovered that the vgrGc-associated effector V2c is a Tox-SHH DNase toxin and explored the rationale for having two T6SS DNase toxins in A. tumefaciens strain 1D1609. We show that the expression of V2c causes growth inhibition, plasmid DNA degradation, and cell elongation, and that these phenotypes are neutralized by the co-expression of the cognate immunity protein, V3c. We also show that the SHH motif is responsible for the nuclease activity of V2c. Importantly, V2a AHH nuclease and V2c SHH nuclease exert different antibacterial capacities against specific bacterial competitors and function synergistically to exert stronger antibacterial activity against the soft rot phytopathogen, Dickeya dadantii. Harboring two classes of His-Me finger nuclease effectors with potential target-specific toxicity may grant 1D1609 versatile antibacterial weapons in facing different bacterial competitors in the microbial community.
Materials and methods
Bacterial strains and growth conditions
Bacterial strains and plasmids are listed in Supplementary Table S1. E. coli strains were grown in Luria-Bertani (LB) medium at 37°C, A. tumefaciens strains in 523 medium at 25°C, and D. dadantii 3937 in LB medium at 30°C. Antibiotics were added when necessary: for E. coli, 30 μg/mL of gentamicin and 250 μg/mL of streptomycin and for D. dadantii and A. tumefaciens, 50 μg/mL of gentamicin.
Molecular cloning
Plasmid pJN105 was used for the expression of toxin genes, and the pTrc200 plasmid was used for the expression of immunity genes. PCR was performed with KAPA HiFi Hot Start DNA Polymerase (Roche, Switzerland) using the genomic DNA of A. tumefaciens 1D1609. The primers used in the study are listed in Supplementary Table S2. Site-directed mutagenesis was performed using either DpnI or Gibson Assembly (New England BioLabs, USA), ligated using T4 ligase (Takara), and transformed into chemically competent E. coli DH10B. Plasmid DNA was isolated using the QIAprep Miniprep Kit (Qiagen, Germany). All constructs were confirmed by sequencing. The in-frame deletion mutants in A. tumefaciens were generated using a suicide plasmid via double crossing over by electroporation or by conjugation, as described previously (Santos et al., 2020).
Bioinformatic analysis
The orthologs of V2c were analyzed using amino acid sequences after the DUF4150 domain via BLASTP search. Multiple sequence alignment was performed using MUltiple Sequence Comparison by Log-Expectation (MUSCLE) (Edgar, 2004). Full-length sequences of the V2c orthologs were analyzed to obtain domain architectures from the Pfam database (Mistry et al., 2021). The E-value threshold was set at 10−5. HHpred (Soding et al., 2005) and Swiss Model (Waterhouse et al., 2018) were used for homology-based protein structure prediction. Protein structure was predicted by AlphaFold (Jumper et al., 2021; Varadi et al., 2022) from the UniProt database. Secondary structure prediction was performed using default settings in PSIPRED 4.0 Protein Structure Prediction Server/DMPfold 1.0 Fast Mode (Buchan and Jones, 2019; Greener et al., 2019).
Growth inhibition assay
The growth inhibition assay was performed as described previously (Ma et al., 2014). In brief, overnight cultures of the E. coli DH10B strain with empty vectors or the derivatives were adjusted to an OD600 of 0.1 in LB medium. A measure of 1 mM isopropyl β-D-1-thiogalactopyranoside (IPTG, Amresco) was used to induce the expression of the putative immunity protein. After 1 h of IPTG induction, L-arabinose (Ara, Sigma-Aldrich) was added to the final concentration of 0.2% to induce the expression of the toxin. Cell growth was recorded every hour at OD600. Empty vectors were used as controls. After 8 h, cells were harvested to quantify the number of colony-forming units (log10 CFU/mL) by automatic diluter and plater, easySpiral Dilute (Interscience, France).
Plasmid DNA degradation analysis in Escherichia coli cells
In vivo plasmid DNA degradation analysis was performed as described previously (Ma et al., 2014). In brief, overnight cultures of the E. coli DH10B strain with empty vectors or the derivatives grown in LB broth were harvested and adjusted to OD600 of approximately 0.3 in LB medium. E. coli cultures were induced with 1 mM IPTG at 0 h for v3c expressed from the pTrc200 plasmid, followed by L-arabinose (0.2%, final concentration) induction at 1 h to induce v2c from the pJN105 plasmid, and cultured for 2 h. Equal amounts of cells were used for plasmid DNA extraction, and an equal volume of extracted DNA was resolved in agarose gel, followed by ethidium bromide staining, and visualized using Gel Doc XR+ UV Gel Documentation Molecular Imager Universal Hood II (Bio-Rad, USA).
Cloning, expression, and purification of V2c and mutants
For the in vitro DNase activity assay, the effector gene v2c was cloned in the pET28a plasmid to generate N-terminal His-tagged V2c, and the immunity gene v3c was constructed in the pTrc200 plasmid. Site-directed mutagenesis was performed by using overlap extension PCR. The plasmid for expressing effector protein, v2c or its variants, and the plasmid for expressing immunity protein, v3c, were transformed into E. coli BL21 (DE3) for IPTG-induced co-expression. The bacterial culture was incubated at 37°C until OD600 reached 0.5 and then 0.1 mM IPTG was added, followed by 5-h expression at 37°C. Pellets were collected, sonicated, and expressed proteins were purified using Ni Sepharose 6 Fast Flow histidine-tagged protein purification resin (Cytiva, Germany). Briefly, cells were lysed in extraction buffer (50 mM Tris–Cl, pH7.5, 0.1 M NaCl, 20 mM imidazole), and proteins were eluted by using an elution buffer with up to 250 mM imidazole in 50 mM Tris–Cl, pH7.5, 0.1 M NaCl.
In vitro DNase activity assay
A 10-μL reaction mixture containing 200 ng of pTrc200HA plasmid DNA and 1× CutSmart Buffer (New England BioLabs, USA) with partially purified V2c or its variants normalized by the major V2c protein band was incubated at 37°C for 1 h. The digestion of DNA was determined by agarose gel electrophoresis and visualized by SYBR Safe DNA gel stain (Invitrogen, USA). The reaction samples of V2c or its variants were scaled up for Western blotting analysis. An anti-V2c antibody was generated in rabbits against two V2c peptides, PFYWDFPNSQVGRD and KRIAMLRGDPTRYD (indicated in Supplementary Figure S2), by Yao-Hong Biotechnology Inc. (Taiwan).
Western blotting analysis
A Western blotting analysis was performed as described previously (Lai and Kado, 1998). In general, proteins were resolved by SDS-PAGE and transferred onto a PVDF membrane by using a transfer apparatus (Bio-Rad, USA). The membrane was probed with primary antibodies against V2c (1:4000), Hcp (1:2500), TssB (1:4000), and GFP (1:1000), followed by incubation with a horseradish peroxidase-conjugated anti-rabbit secondary antibody (ChemiChem) (1:25000) and visualized with the ECL system (Perkin-Elmer Life Science, Boston, USA).
Fluorescence microscopy and image analysis
Escherichia coli DH10B carrying the empty vectors or the derivatives were grown overnight in LB broth at 37°C. For the expression of the effector gene alone, overnight culture was diluted to OD600 reached 0.2 and sub-cultured for 1.5 h prior to induction by 0.2% L-arabinose for 1 h. For the co-expression of effector and putative immunity genes, overnight culture was diluted to OD600 reached 0.1 and sub-cultured for 1 h prior to induction by 0.2% L-arabinose and 1 mM IPTG for 3–4 h. Cultures were harvested and resuspended with 30 μL of PBS with 0.5% Tween 20 (PBST). Three μL of cells were placed on a 2% agarose pad and imaged. To check the DNA degradation, the same protocol was followed, and live cells were stained with 2 μg/mL of Hoechst (Life Technologies, USA) and 1 μg/mL of FM4-64FX, a fixable analog of FM 4–64 (Invitrogen, USA) for 5 min and washed with PBS to remove the excess dye.
For the co-culture of D. dadantii ∆imp-GFP (S65T) with various 1D1609 EI pair mutants, strains were mixed at 1:10 ratio on Agrobacterium kill-triggering (AK) agar and the AK medium (3 g K2HPO4, 1 g NaH2PO4, 1 g NH4Cl, 0.15 g KCl, 9.76 g MES in 900 mL of ddH20, pH 5.5) (Yu et al., 2020) was solidified by 2% (w/v) agar. Cells from 16-h co-culture at 25°C were concentrated to an OD600 of 10 and spotted on a 2% agarose pad for imaging.
Imaging was performed using an inverted fluorescent microscope (IX81, Olympus Japan) with the objective lens UPLSAPO 100XO (Olympus, Tokyo, Japan), DAPI (49,000; Chroma), GFP (49,002; Chroma), and Cy3 (49,005; Chroma) filter sets and a CCD camera (ORCA-R2; Hamamatsu, Japan). The images were acquired using Olympus cellSens Dimension software.
All the image analysis was performed using the Fiji analyze particles tool (Schindelin et al., 2012). The micrograph of FM 4–64 fluorescence was used to segment surfaces (i.e., individual bacterial cells) with background subtraction and thresholding. Feret diameter was used. The cell length (μm) was determined from the cumulative counts of three random frames per sample. For the quantification of cell degradation, the Hoechst intensity was normalized using the formula intensity/unit area of cells, where intensity is major × minor × 3.14 (π).
Interbacterial competition assay
Bacterial competitions were carried out as described previously (Wu et al., 2019) with modifications. Briefly, an overnight culture of A. tumefaciens and D. dadantii 3937 ∆imp was mixed at a 10:1 attacker-to-prey ratio and spotted on AK agar plates (Yu et al., 2020). The prey, A. tumefaciens C58 or D. dadantii 3937 ∆imp, was transformed with pRL662-GFP (S65T) for gentamicin selection or microscopy imaging. After 18 h of incubation at 25°C, the co-cultured cells were collected, serially diluted, and plated on LB agar containing gentamicin to quantify surviving prey cells by counting CFU.
Statistical analysis
The statistical analyses were performed using GraphPad Prism version 6.01 for Windows, GraphPad Software, La Jolla, California, USA.1 The number of technical replicates and independent biological replicates, p-values, and statistical tests performed are indicated in the figure legends. The mean of independent replicates was compared using the one-way ANOVA followed by Tukey’s multiple comparisons tests2 for statistical analysis. The error bars indicate the standard error of the mean (SEM).
Results
V2c is a DNase belonging to the Tox-SHH clade of His-Me finger superfamily
In 1D1609, vgrGa-associated effector V2a appears to be the major antibacterial toxin against Escherichia coli prey (Santos et al., 2020). V2c is a putative PAAR (DUF4150)-linked specialized effector (Figure 1A), but the presence or absence of v2c did not affect the antibacterial activity of 1D1609 against E. coli prey (Santos et al., 2020). Aside from the N-terminal PAAR domain, V2c does not show any sequence similarity to V2a (Supplementary Figure S2). BLAST and CDD searches did not identify a known or conserved domain at its C-terminal region. However, multiple sequence alignments of V2c and V2c orthologs revealed conserved SHH and HNH motifs at the C-terminal region (Figure 1B). These V2c orthologs all harbor the C-terminal Tox-SHH domain, but their N-terminal region can be fused to other known T6SS domains, such as Found in the type sIX effector (FIX), the DUF4150/PAAR-like domain, and the Rhs core domain (Jana et al., 2019; Figure 1C). Using the protein homology detection program HHpred (Soding et al., 2005), we found that the C-terminal region of V2c encodes two parallel β-strands connected by an α-helix, which is a signature structure in His-Me finger nuclease (Wu et al., 2020). Consistent with the result of secondary structure prediction, 3D structure prediction using AlphaFold (Jumper et al., 2021; Varadi et al., 2022; Figure 1D) shows that this domain consists of two antiparallel β-strands connected with an Ω loop with a His (H384) at the C-terminus of the first β-strand and followed by an α-helix, featuring a HNH motif of His-Me finger endonuclease (Jablonska et al., 2017; Wu et al., 2020). These suggest that V2c belongs to the Tox-SHH (Pfam PH15652) clade of the His-Me finger superfamily.
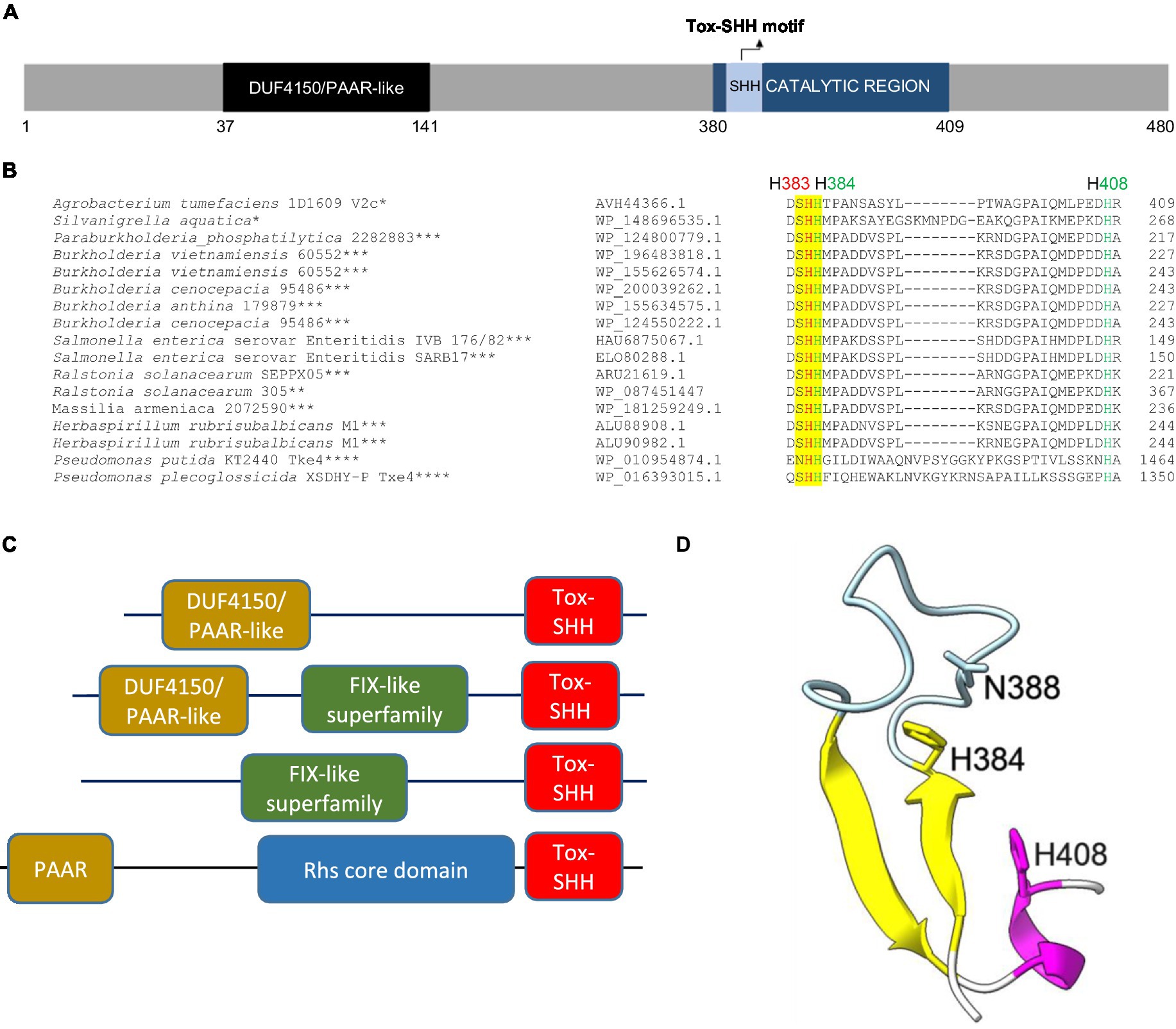
Figure 1. V2c is a PAAR-linked specialized effector containing a C-terminal Tox-SHH motif. (A) A schematic diagram of V2c protein showing the N-terminal DUF4150 PAAR region and the catalytic region (amino acids 380–409), which covers the Tox-SHH motif. (B) Partial sequence alignment of orthologs of V2c from BLASTP. The strain name and accession number are indicated on the left. SHH motif is highlighted in yellow, with H383 (indicated in red) as the metal ion coordinating residue, and H384 and H408 (indicated in green) of the HNH motif for catalysis and metal ion binding, respectively, are targeted for mutagenesis. The star corresponds to the specific domains shown in panel (C): *DUF4150/PAAR-like, **DUF4150/PAAR-like + FIX-like, ***FIX-like superfamily, ****PAAR + Rhs core domain. (C) Domain architectures of V2c orthologs and Tox-SHH-containing proteins found in this study. Full-length sequences were analyzed, and the domains were from the Pfam database with E value threshold set at 10−5. (D) A cartoon model of V2c (residues 380–409). The structure of V2c predicted by AlphaFold indicates V2c that consists of a His-Me finger domain with two antiparallel β-strands (yellow) connected with an Ω loop (light blue), a histidine (H384) at the C-terminus of the first β-strand and is followed by an α-helix (magenta). The confidence score of this region is higher than 90, indicating high structure accuracy.
To determine the antibacterial and DNase activities of V2c, we expressed it using an arabinose-inducible promoter to determine if V2c is a bacterial toxin functioning as a nuclease. Growth inhibition measured by optical density was observed when v2c is expressed by arabinose induction in E. coli, and this can be neutralized by the co-expression of its putative cognate immunity, v3c, as compared to the vector control (Figure 2A). Furthermore, the colony-forming unit (CFU) count shows the reduced CFU of v2c-expressed cells, and higher CFU could be recovered with the co-expression of v3c (Figure 2B). In addition, the plasmid degradation assay showed that the expression of v2c upon arabinose induction resulted in the degradation of plasmid DNA in E. coli, and this is abolished when v3c is co-expressed (Figure 2C). The data indicated that V2c exhibits DNase activity in vivo.
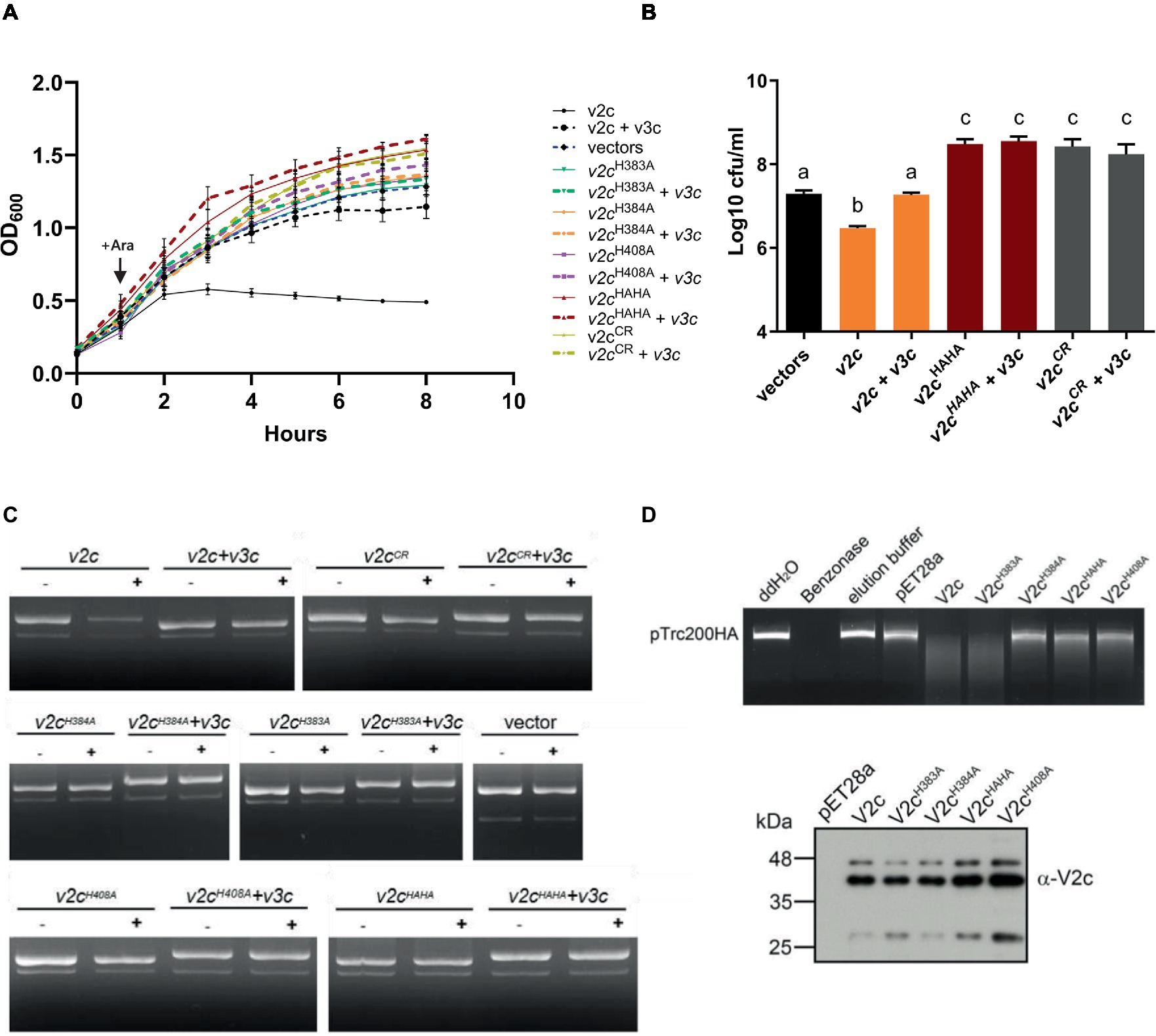
Figure 2. V2c exhibits growth inhibition and DNase activity in vivo and in vitro. (A) Growth inhibition analysis of expression of v2c and its variants with or without v3c in E. coli DH10B. E. coli cultures were induced with 1 mM IPTG at 0 h for v3c expressed from the pTrc200 plasmid, followed by L-arabinose (Ara) induction at 1 h to induce v2c from the pJN105 plasmid. Cell growth was recorded every hour at OD600. Strain expressing empty vectors (vectors) were used as a control. (B) Number of colony-forming units (log10 CFU/mL) quantified after growth inhibition assay at 8 h. Data in panel (A,B) represent mean ± SEM of four independent experiments (n = 4), each averaged with three technical repeats. Different letters above the bar indicate statistically different groups of strains (p < 0.05) determined by Tukey’s HSD test. (C) Plasmid degradation assay performed by E. coli DH10B cells harboring pTrc200 and pJN105 plasmids (vector) or the derivatives induced with (+) or without (−) Ara for 2 h. Plasmid DNA was extracted, and the degradation pattern was observed in agarose gel. (D) In vitro DNase activity assay performed by using pTrc200HA plasmid DNA as DNA substrate and incubated with purified V2c or its variants. Nuclease-free water (ddH2O), protein elution buffer (elution buffer), and eluate from empty vector expression (pET28a) served as negative controls, while Benzonase nuclease served as a positive control. The digestion of the DNA substrate was determined by agarose gel electrophoresis. The reaction samples of V2c or its variants were detected by Western blotting using an anti-V2c antibody (α-V2c). The results of panel (C,D) are representative of three independent experiments.
We further investigated whether the SHH and HNH motifs are involved in DNase activity. The His-Me finger superfamily has a strictly conserved His residue and catalytic metal ion, which are both essential for nucleic acid hydrolysis. Based on this premise, we define a region spanning amino acid residues 380–409 as a His-Me finger domain core region (CR). Based on the alignment, H384 is the catalytic His and H408 is for metal ion binding, while H383 may function as a second residue for metal ion coordination. To verify their roles in antibacterial and DNase activity, a series of deletions as well as single and double amino acid substitution variants were generated. Strikingly, no growth inhibition or plasmid degradation were observed in any of the mutants expressing the CR deletion (V2cCR), single (V2cH383A, V2cH384A, and V2cH408A), or double (V2cHAHA substitution in both H383 and H384) variants (Figures 2A,C,D). Higher CFU was also recovered from E. coli culture expressing these mutants alone or with v3c (Figure 2B). To further confirm the DNase activity of V2c, each V2c and its variants were fused with 6x-His and purified for in vitro DNase activity assay. The results showed that the purified V2c fraction is able to degrade DNA but not the eluate from E. coli expressing the vector (pET28a) (Figure 2D). Consistent with the in vivo plasmid degradation assay, no activity could be detected from V2cH384A, V2cH408A, or V2cHAHA variants. However, the purified V2cH383A fraction exhibited DNase activity by degrading the plasmid DNA substrate. The Western blotting analysis of the reaction samples shows whether V2c and its variants used for the assay were at similar protein levels. Taken together, the results of in vivo and in vitro DNA degradation assays show that the conserved H384 and H408 in the HNH motif are crucial for V2c DNase activity, whereas H383 in the SHH motif appears to be less critical for in vitro but may be required for in vivo nuclease activity.
Ectopic expression of V2c caused DNA degradation and cell elongation
We next examined the cell morphology and DNA integrity of E. coli cells expressing v2c and the two variants (v2cCR and v2cHAHA) that are defective in DNase activity and toxicity. Cell morphology was observed by phase contrast, while the bacterial cell membrane and DNA were stained by FM 4–64 and Hoechst, respectively, under the fluorescence microscope. Hoechst signals are significantly reduced when v2c is expressed by arabinose induction in E. coli, and this can be rescued by the co-expression of its cognate immunity v3c shown in the images and quantification (Figures 3A,B). Hoechst signals are diminished only in E. coli cells producing v2c but not v2cHAHA, v2cCR, and vector control, suggesting that cellular DNA degradation is caused by V2c DNase activity, which can be neutralized by its immunity protein V3c. In addition, we also observed cell elongation in E. coli expressing v2c, and co-expression of v3c rescues the cell elongation phenotype (Figures 3A,C). However, E. coli cells expressing v2cCR and v2cHAHA, as well as other single amino acid substitution variants (v2cH383A, v2cH384A, and v2cH408A), do not abolish the elongated cell phenotype (Figures 3A,C; Supplementary Figures S3A,B).
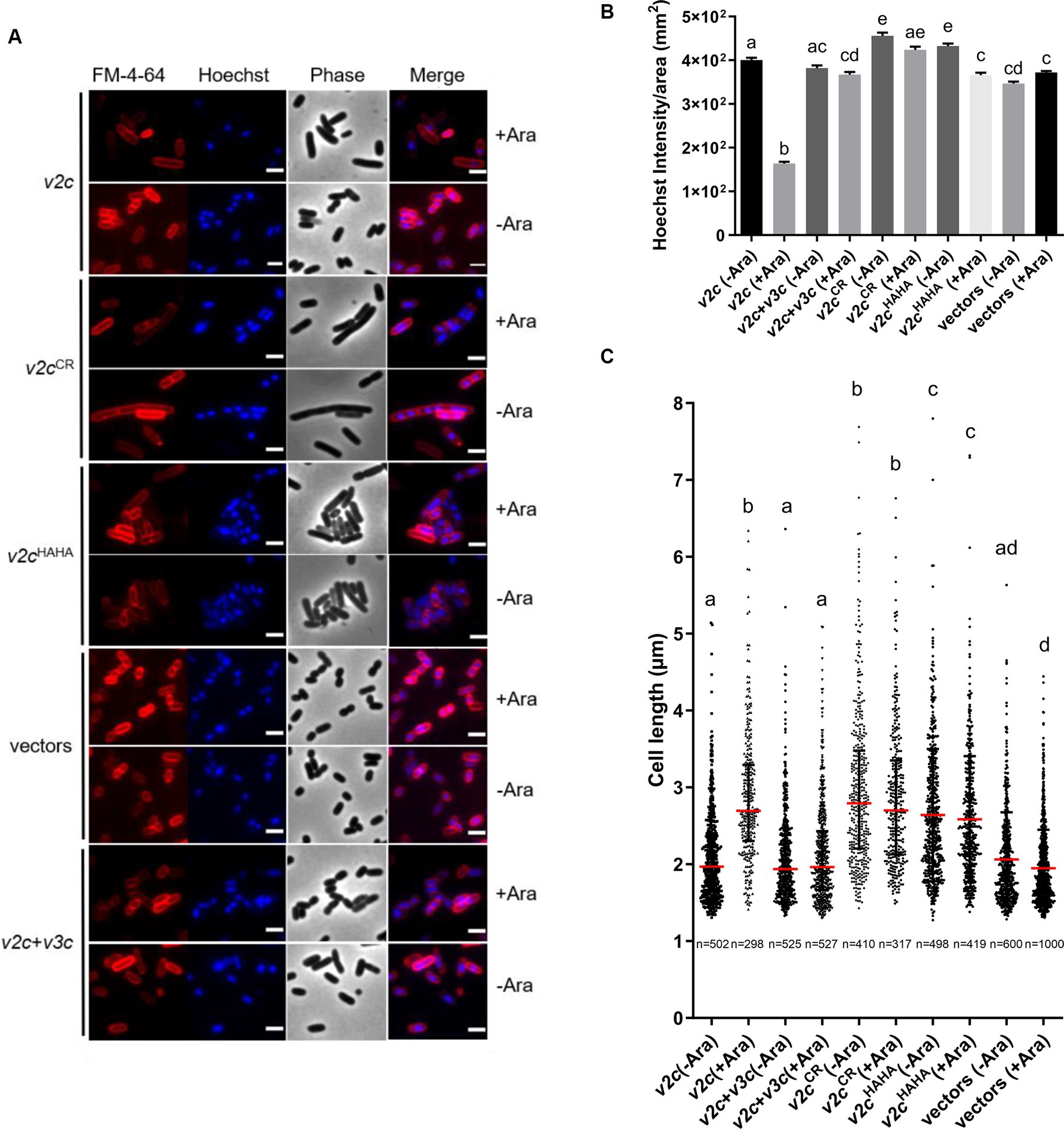
Figure 3. V2c Tox-SHH DNase exhibits the cell elongation phenotype. (A) Images of E. coli DH10B cells harboring vector(s) and the derivatives expressing v2c or its variants in the presence or absence of its cognate immunity gene v3c with (+Ara) and without (-Ara) Arabinose induction stained with FM 4–64 (red) and Hoechst stain (blue). The micrographs from left to right are FM 4–64, Hoechst, phase contrast, and a merged image of FM 4–64 and Hoechst stains. Scale bar, 2 μm. Representative images of two independent experiments are shown. (B) Normalized Hoechst intensity (intensity/unit area of cells) and (C) cell length (μm) as measured from the indicated number of cells (n) per treatment. The graph shows a combined count from three frames of a representative result of two independent experiments. The red line in panel (C) shows the median with an interquartile range. Statistics were performed with the mean ± SEM of three frames. Different letters above the bar indicate statistically different groups of strains (p < 0.05) determined by Tukey’s HSD test.
Ectopic expression of V2a also caused cell elongation phenotype
A previous study showed that V2a is a Tox-AHH nuclease that serves as the major antibacterial weapon in 1D1609 (Santos et al., 2020). H385 and H386 are two of the predicted His residues in the AHH motif of V2a, corresponding to H383 and H384 in the SHH motif of V2c. The structure predicted by AlphaFold indicates that V2a also features a His-Me finger, having two α-helices with histidine residues (H430 and H456) followed by the β-strands (Figure 4A). Previous findings demonstrated that the expression of v2a but not v2aH385A caused growth inhibition and plasmid degradation in E. coli (Santos et al., 2020). To gather further insight into the V2a AHH motif in the His-Me finger domain, we evaluated the growth and morphology of arabinose-induced v2a mutants. The results showed that the expression of v2a caused a reduction in Hoechst-stained DNA signals and resulted in cell elongation (Figures 4B–D). The expression of v2aH385A has similar levels of Hoechst-stained DNA signals and cell length as compared to the vector control. These results suggest that the cell elongation phenotype observed was caused by V2a DNase activity, whereas V2c shows DNase activity-independent cell elongation. We also determined the toxicity of another putative effector V4b of 1D1609, a PAAR (DUF4150)-linked specialized effector but harboring an Rhs domain with structural similarity to the insecticidal toxin (Santos et al., 2020; Supplementary Figure S4A). Expression of v4b caused growth inhibition in E. coli, in which the growth inhibition can be rescued by the co-expression of its putative cognate immunity v5b to a level similar to vector control (Supplementary Figure S4B). However, no DNA degradation or cell elongation could be observed (Supplementary Figures S4C–F). These results show that V4b is a bacterial toxin and V5b is the cognate immunity protein, suggesting that the cell elongation phenotype may be specific to the toxicity of nuclease effectors.
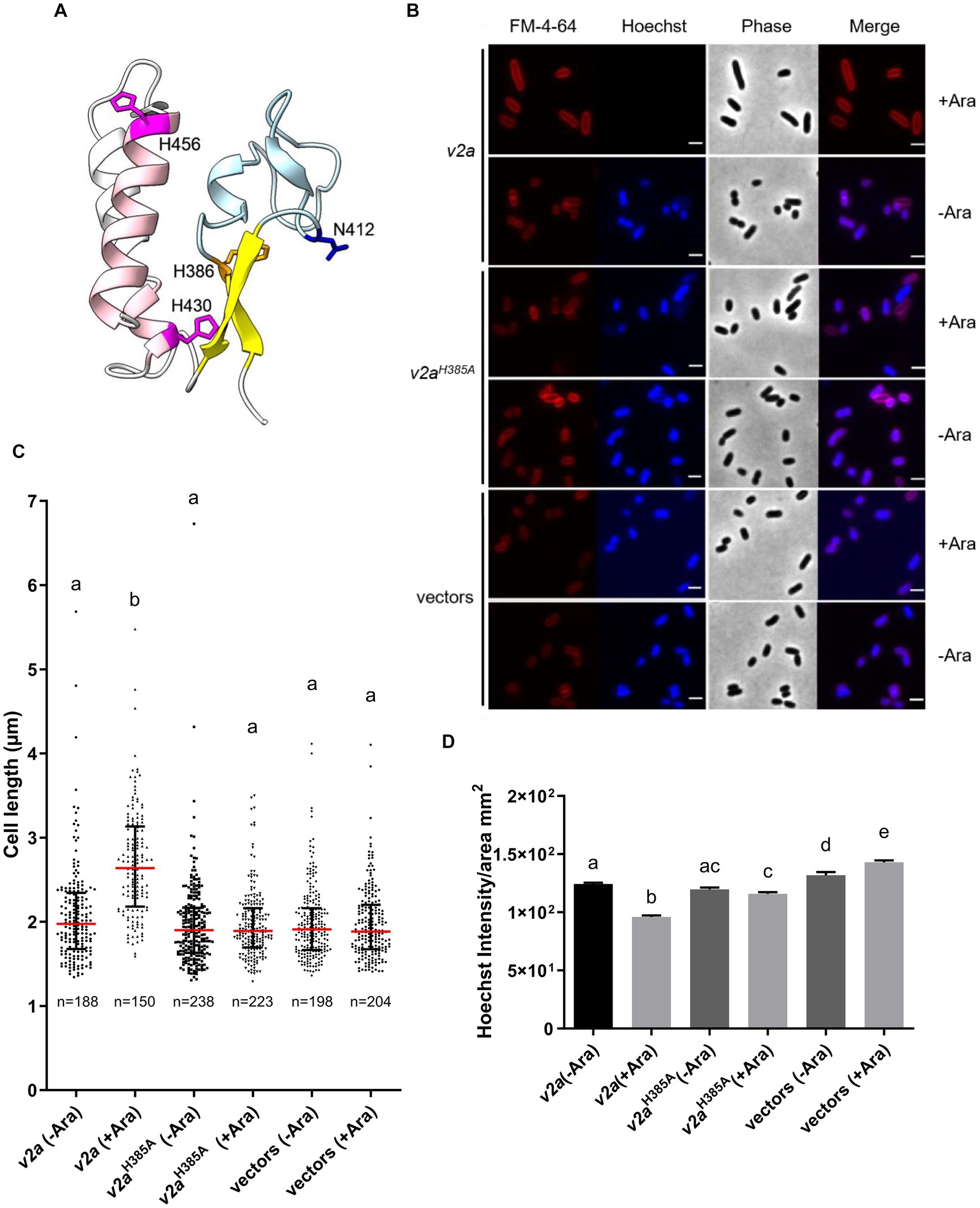
Figure 4. V2a Tox-AHH DNase exhibits the cell elongation phenotype. (A) A cartoon model of V2a (residues 380–470). The structure of V2a predicted by AlphaFold indicates V2a consisting of a His-Me finger domain with its signature antiparallel β-strands (yellow) connected with an Ω loop (light blue) with a histidine (H386, orange) at the C-terminus of the first β-strand. Two α-helices (pink) with histidine residues (H430, H456 magenta) followed the β-strands. N412 is found in the loop between the β-strands, and H430 is in the first α-helix (E429–G430). The predicted local-distance difference tests (pLDDTs) of the His-Me domains of V2a range from confident to very high; most of the pLDDTs of the residues are >90. (B) Morphological analysis of E. coli DH10B cells harboring vector(s) or its derivatives expressing v2a, catalytic site (H385A) mutant without (-Ara) or with (+Ara) arabinose induction. Cells were stained with FM 4–64 (red) and Hoechst stain (blue). The micrographs from left to right are FM 4–64, Hoechst, phase contrast, and a merged image of the two fluorescent images. Scale bar, 2 μm. (C) Cell length (μm) and (D) Hoechst intensity in different treatments as determined from a combined count of three random frames of a representative result; the number of cells (n) per sample is indicated. The graph shows a combined count from three frames of a representative result of two independent experiments. The red line shows the median with an interquartile range. Statistics were performed with the mean ± SEM of three frames. Different letters above the bar indicate statistically different groups of strains (p < 0.05) determined by Tukey’s HSD test.
V2c nuclease exhibits antibacterial activity at both intra-species and inter-species competition and functions synergistically with V2a against the soft rot phytopathogen, Dickeya dadantii
To correlate the DNA degradation and cell elongation phenotypes observed by the ectopic expression of v2c in E. coli in a more biologically relevant context, we further performed the interbacterial competition assay. We first selected A. tumefaciens strain C58, which possesses incompatible EI pairs with 1D1609 and has previously been demonstrated to be susceptible to 1D1609 T6SS killing (Wu et al., 2019), for intra-species competition. Each of the 1D1609 mutants lacking multiple EI pairs was co-cultured with a C58 strain harboring pRL-GFP (S65T) prey in vitro on AK agar (Yu et al., 2020) and the number of viable C58 prey cells (log10 CFU/mL) was quantified. Similar to the previous killing assay using E. coli prey (Santos et al., 2020), we detected approximately 2 log of reduced prey cell survival by the 1D1609 WT attacker as compared to the ∆4EI mutant with the deletion of all four EI pairs (Figure 5A). Other double (∆EIbd) or triple deletion mutants (∆EIabd, ∆EIbcd) exhibited compromised but detectable antibacterial activity against C58, indicating that v2a or v2c alone is sufficient to exhibit antibacterial activity against C58.
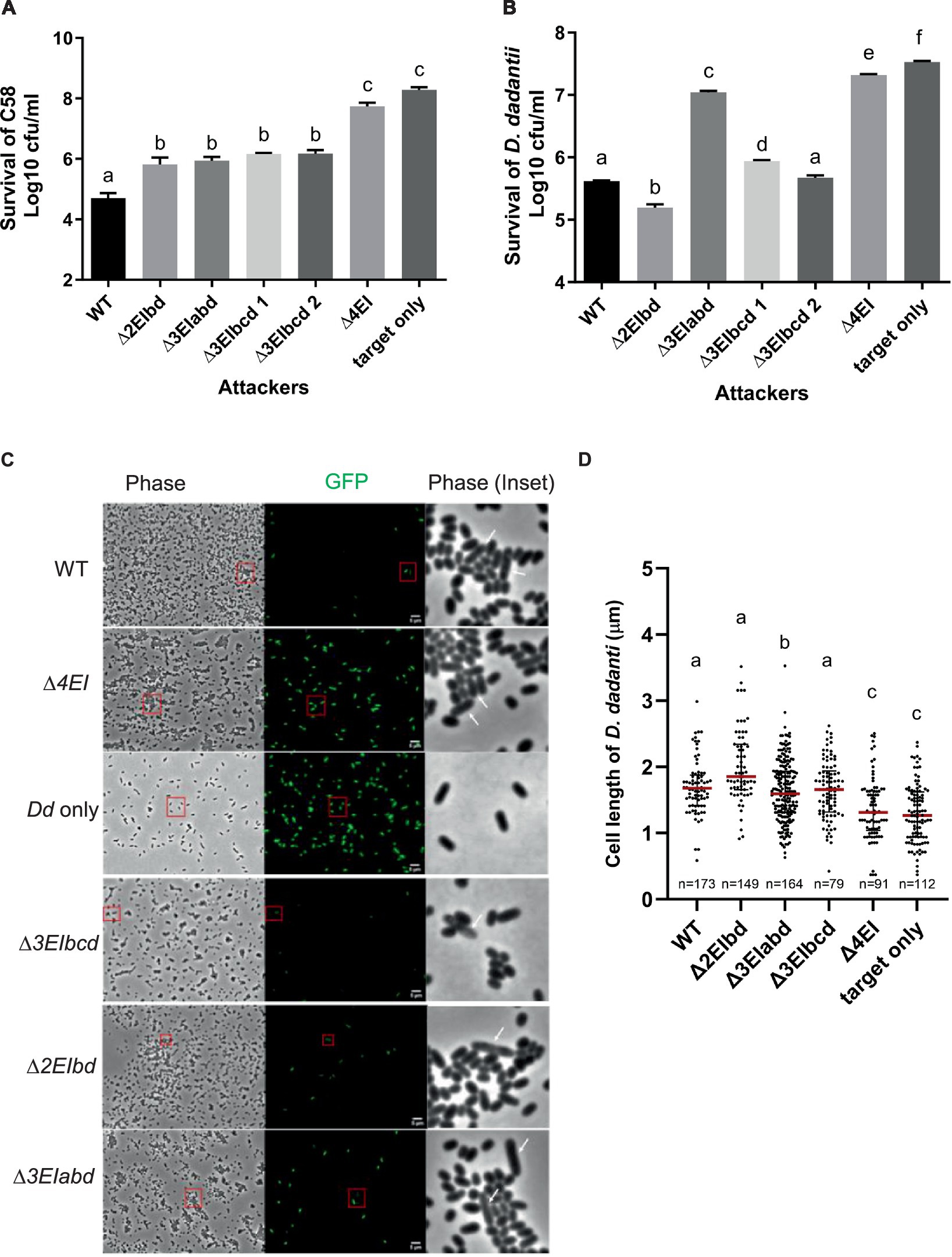
Figure 5. V2a and V2c exhibit different toxicity against A. tumefaciens and D. dadantii. (A) Intra-species competition between A. tumefaciens 1D1609 attacker and prey C58. (B–D) Inter-species competition between A. tumefaciens 1D1609 attacker and prey D. dadantii 3937 ∆imp. The plasmid pRL-GFP(S65T) plasmid (GmR) was transformed into prey cells, which were mixed at a 1:10 ratio with each of the attacker strains, 1D1609 WT, or various EI mutant strains as indicated. After co-culture on AK agar plates, cells were collected, serially diluted, and plated on the LB agar plate containing Gm for CFU counting of surviving prey cells (B). Data are mean ± SEM (n = 6 biological repeats from three independent experiments). For imaging, co-cultured D. dadantii Δimp-GFP(S65T) and 1D1609 EI pair mutants were concentrated and spotted on a 2% agarose pad for fluorescence micrographs (C). D. dadantii Δimp-GFP(S65T) indicated as Dd only is included as a control. The inset in phase contrast shows a magnified image of cells in the red box. White arrows point to D. dadantii cells. Scale bar, 2 μm. (D) Cell length after competition between A. tumefaciens 1D1609 EI mutants and D. dadantii 3937 ∆imp-GFP(S65T). Cell length of GFP fluorescent D. dadantii cells was measured using the Fiji analyze particles tool. Cell length (μm) in different treatments as determined from a combined count of at least 10 random frames of two independent experiments. The red line shows the median with an interquartile range. Statistics were performed with the mean ± SEM of total counts. Different letters above the bar indicate statistically different groups of strains (p < 0.05) determined by Tukey’s HSD test.
Intrigued by the observation that v2c could exhibit antibacterial activity to the A. tumefaciens C58 prey but no significant effect against E. coli prey (Santos et al., 2020), we reasoned that E. coli may not be a physiologically relevant prey for A. tumefaciens although v2c is able to cause toxicity when directly expressed in E. coli (Figures 2A,B). We then selected D. dadantii as the prey cells for inter-species competition because it is a phytopathogen but also a close relative of E. coli, both belonging to Enterobacteriaceae. To avoid potential counterattack by D. dadantii T6SS (Koskiniemi et al., 2013), a T6SS-deficient mutant, ∆imp with deletion of imp operon, harboring pRL-GFP (S65T) (D. dadantii ∆imp-GFP (S65T)) was used as a prey. CFU counting of D. dadantii ∆imp-GFP (S65T) prey cells after co-culture show approximately 1.5 log of reduced prey cell survival by 1D1609 WT attacker, as compared to the ∆4EI mutant (Figure 5B). The presence of v2a only (∆3EIbcd) exhibited comparable antibacterial activity as the WT, while v2c only (∆3EIabd) showed weak but detectable antibacterial activity as compared to ∆4EI, indicating that v2c itself is able to kill D. dadantii but with much weaker activity than v2a. Strikingly, 1D1609 expressing both v2a and v2c (∆2EIbd) could result in stronger killing effects than WT. The use of pRL-GFP (S65T) with constitutive expression of GFP also allowed us to observe the D. dadantii ∆imp-GFP (S65T) prey cells under the fluorescence microscope after co-culture. We observed very few cells expressing GFP when competing with WT or ∆3EIbcd expressing v2a only. The lysed and elongated D. dadantii cells were also detected when co-cultured with 1D1609 with either strains expressing v2a only (∆3EIbcd), v2c only (∆3EIabd), or both ∆3EIbd (Figure 5C). We further quantified the cell length of GFP-expressing D. dadantii cells. The results show that the majority of cells were elongated after 18 h of co-culture with 1D1609 expressing either v2a (∆3EIbcd) or v2c (∆3EIabd), or a combination of both (∆3EIbd), as compared to those D. dadantii cells alone or co-incubated with ∆4EI (Figure 5D).
Taken together, these results suggest that V2c nuclease exhibits antibacterial activity at both intra-species and inter-species competitions but at different capacities. While V2a and V2c exert similar antibacterial activity against A. tumefaciens C58, V2c only exhibits weak antibacterial activity but functions synergistically with V2a when competing with D. dadantii prey, resulting in elongated and lysed cells.
Discussion
His-Me finger nucleases are a large and diverse superfamily of nucleases; however, only a limited number of T6SS effectors have been identified as His-Me finger nucleases. In the present study, we reported that A. tumefaciens strain 1D1609 encodes two His-Me finger superfamily DNases, V2a and V2c, belonging to the Tox-AHH and Tox-SHH families, respectively, exhibiting different capacities for specific bacterial competitors. It is well established that the effector encoded in the vgrG module is delivered by its cognate VgrG carrier via T6SS (Hachani et al., 2014; Whitney et al., 2014; Bondage et al., 2016; Flaugnatti et al., 2016). Together with our previous studies that 1D1609 with deletion of four EI pairs or four vgrG genes completely loses the T6SS-mediated antibacterial activity (Santos et al., 2020), we concluded that the V2a and V2c are T6SS effectors, although direct demonstration is yet to be determined.
His-Me finger nucleases are one-metal-ion-dependent nucleases, primarily dependent on Mg2+ or, in some cases, on Zn2+. The only invariant residue in His-Me endonuclease is the catalytic His located at the end of the β1 strand (first H in the HNH motif, H384 for V2c, and H386 for V2a, Figures 1D, 4A; Supplementary Figure S2), which functions as the general base for the hydrolysis of phosphodiester bonds. The other conserved His (last H in the HNH motif, H408 for V2c and H430 for V2a) and Asn/Asp/Glu/His located before the invariant His (H383 for V2c and H384 for V2a) are involved in coordinating the single metal ion. Indeed, our data show that H383, H384, and H408 of V2c and H385 and H386 of V2a are required for exerting DNase activity in vivo (Figure 2C; Santos et al., 2020). However, different from the loss of DNase activity in vivo and in vitro for both V2cH384A and V2cH3408A, the V2cH383A variant retains the DNase activity by an in vitro nuclease activity assay (Figure 2D), which indicates the requirement of H383 in coordinating metal ion binding for DNase activity may be subject to environmental factors.
His-Me finger encodes a small structural motif, so it is usually associated with other domain architectures that could play additional functions. In addition, its three-element structural motif is unable to provide enough of a hydrophobic core for stability (Jablonska et al., 2017). Thus, many proteins containing the His-Me finger employ a variety of additional structural elements or domains for stabilization and/or specificity. V2c and its orthologs commonly found in Rhizobiaceae are not included in the reported 77 sequences with conserved core regions of the His-Me finger superfamily (Jablonska et al., 2017). Our evidence further suggests that V2c may represent a new branch of the Tox-SHH toxin family (Pfam PF15652), which includes proteins with additional domain architectures (Figure 1C). These additional domain(s) are conserved regions found in many bacterial toxin proteins and may facilitate the secretion of nuclease and affect its toxicity to the competing cell. While both V2c and V2a harbor the conserved N-terminal DUF4150 PAAR-like domain, they share low sequence similarity in the C-terminal His-Me finger domain (Supplementary Figure S2), in which the protein structures predicted by AlphaFold reveal the structural difference between V2c and V2a (Figures 1C, 4A). This discrepancy may contribute to the difference on toxicity strength and the role of DNase on cell elongation. Alternatively, V2a and V2c may have the same mechanism to bind and hydrolyze DNA for toxicity, and the difference in the phenotype is caused by another domain outside the His-Me finger fold.
Cell elongation could still be observed in the E. coli strain carrying different DNase-defective v2c variants, even in the absence of arabinose (Figures 3A,C; Supplementary Figure S3A). Several studies reported that the leaky expression of T6SS toxin driven by the arabinose-inducible PBAD promoter resulted in toxicity in the absence of arabinose (Jana et al., 2019; Fridman et al., 2020). Thus, we speculated that the leaky expression of these v2c variants is sufficient to induce the cell elongation phenotype. The data suggest that another domain outside the SHH catalytic region in V2c is responsible for the cell elongation phenotype, which is different from the nuclease activity-dependent filamentation observed previously (Yu and Lai, 2017; Pissaridou et al., 2018) and V2a (Figure 4), suggesting that V2c-induced cell elongation is independent of nuclease activity. The proposed biological role of bacterial filamentation is mainly related to the stress response to thrive for survival (Justice et al., 2008). One of the conditions that lead to filamentation is DNA damage. When the DNA is damaged, the SOS response is induced, and cells may delay the cell cycle and inhibit cell division until DNA repair and replication are complete. Although V2c catalytic site variants lose activity for DNA cleavage, they may still bind to DNA and induce cell elongation. Furthermore, cell division arrest could also be caused by non-nuclease effectors. For example, a T6SS ADP-ribosyltransferase effector Tre1 in Serratia proteamaculans was reported to inactivate bacterial cell division by modifying arginine on FtsZ to block polymerization (Ting et al., 2018). A future study by domain dissection of V2c is needed to identify the domain causing cell elongation.
While HNH/His-Me T6SS nucleases are the most prevalent nuclease toxins identified in T6SS, most bacterial genomes only encode one type of HNH/His-Me nuclease effector, including Tse7 of P. aeruginosa (Pissaridou et al., 2018), Tse1 of A. dhakensis (Pei et al., 2020), and Tke4 of P. putida (Bernal et al., 2017). In addition to A. tumefaciens 1D1609 encoding Tox-AHH (V2a) and Tox-SHH (V2c) nucleases, the use of multiple HNH/His-Me nucleases as T6SS antibacterial weapons has been identified in the fish pathogen P. plecoglossicida (Li et al., 2022). P. plecoglossicida T6SS-2 mediates interbacterial competition and encodes four putative effectors, all of which contain C-terminal toxin domains belonging to the HNH/His-Me superfamily but with distinct classes (Txe1 with a dipeptide HH motif, Txe2 as Tox-AHH, Txe3 with HNHc, and Txe4 as Tox-SHH) (Li et al., 2022). Among them, Txe1, Txe2, and Txe4 exhibit in vitro nuclease activity and toxicity when expressed in E. coli. However, only Txe1 and Txe4 contribute to interbacterial activity against E. coli, with Txe1 as the predominant toxin, in which its C-terminal conserved dipeptide HH motif is required for nuclease activity and toxicity to E. coli. Txe4 is also required for full interbacterial competition, but the role of the SHH motif in DNase and toxicity has not been determined. Together with our findings, it is possible that having multiple distinct or the same classes of nuclease effectors may provide versatile toxicity when competing with different preys, in which some toxins may only exhibit activity against specific preys.
The strain 1D1609 is unique in the A. tumefaciens species complex with multiple VgrG spikes carrying different antibacterial effectors (Santos et al., 2020). Our findings that v2a and v2c exhibit different capacities against different preys support previous studies that some T6SS toxins can only exert toxicity in specific bacterial species (LaCourse et al., 2018; Wu et al., 2021). From the observation of the synergism of V2a and V2c against D. dadantii, harboring multiple T6SS effectors in 1D1609 may provide an advantage for the strain to maintain growth competitiveness in different niches and environments.
Data availability statement
The original contributions presented in the study are included in the article/Supplementary material, further inquiries can be directed to the corresponding author.
Author contributions
MS: Conceptualization, Investigation, Writing – original draft, Writing – review & editing, Methodology. KP: Investigation, Writing – review & editing, Methodology. P-YH: Investigation, Methodology, Writing – review & editing. Y-WC: Investigation, Methodology, Writing – review & editing. L-KS: Investigation, Methodology, Writing – review & editing. Y-LS: Funding acquisition, Investigation, Methodology, Resources, Supervision, Writing – review & editing. E-ML: Conceptualization, Funding acquisition, Investigation, Project administration, Resources, Supervision, Writing – original draft, Writing – review & editing.
Funding
The author(s) declare financial support was received for the research, authorship, and/or publication of this article. This work was supported by grants from the National Science and Technology Council (NSTC) of Taiwan (107-2311-B-001-019-MY3) and the Academia Sinica Investigator Award (AS-IA-107-L01) to E-ML and the NSTC grant (110-2311-B001-011) to Y-LS. Y-WC was supported by the postdoctoral fellowship (NSTC 110-2811-B-001-504). The funders had no role in study design, data collection and interpretation, or the decision to submit the work for publication.
Acknowledgments
The authors acknowledge Ching-Hong Yang (Department of Biological Sciences, University of Wisconsin, Milwaukee) for providing Dickeya dadantii 3937 strains, which were imported under permit 106-B-003 issued by the Council of Agriculture of Taiwan. The authors would like to thank the former and current Lai lab members for their help and fruitful discussion throughout this study, as well as See-Yeun Ting and Mao-Sen Liu for critically reading the manuscript and their valuable comments. The authors also thank Venus Marie Gaela and Thomas Boudier for their guidance and help in processing the images using Fiji and the Sanger DNA sequencing service provided by the Genomic Technology Core located at the Institute of Plant and Microbial Biology, Academia Sinica.
Conflict of interest
The authors declare that the research was conducted in the absence of any commercial or financial relationships that could be construed as a potential conflict of interest.
Publisher’s note
All claims expressed in this article are solely those of the authors and do not necessarily represent those of their affiliated organizations, or those of the publisher, the editors and the reviewers. Any product that may be evaluated in this article, or claim that may be made by its manufacturer, is not guaranteed or endorsed by the publisher.
Supplementary material
The Supplementary material for this article can be found online at: https://www.frontiersin.org/articles/10.3389/fmicb.2024.1351590/full#supplementary-material
Footnotes
References
Basler, M., Pilhofer, M., Henderson, G. P., Jensen, G. J., and Mekalanos, J. J. (2012). Type VI secretion requires a dynamic contractile phage tail-like structure. Nature 483, 182–186. doi: 10.1038/nature10846
Bernal, P., Allsopp, L. P., Filloux, A., and Llamas, M. A. (2017). The Pseudomonas putida T6SS is a plant warden against phytopathogens. ISME J. 11, 972–987. doi: 10.1038/ismej.2016.169
Bondage, D. D., Lin, J. S., Ma, L. S., Kuo, C. H., and Lai, E. M. (2016). VgrG C terminus confers the type VI effector transport specificity and is required for binding with PAAR and adaptor-effector complex. Proc. Natl. Acad. Sci. U. S. A. 113, E3931–E3940. doi: 10.1073/pnas.1600428113
Buchan, D. W. A., and Jones, D. T. (2019). The PSIPRED protein analysis workbench: 20 years on. Nucleic Acids Res. 47, W402–W407. doi: 10.1093/nar/gkz297
Cherrak, Y., Flaugnatti, N., Durand, E., Journet, L., and Cascales, E. (2019). Structure and activity of the type VI secretion system. Microbiol. Spectr. 7. doi: 10.1128/microbiolspec.PSIB-0031-2019
Chou, L., Lin, Y. C., Haryono, M., Santos, M. N. M., Cho, S. T., Weisberg, A. J., et al. (2022). Modular evolution of secretion systems and virulence plasmids in a bacterial species complex. BMC Biol. 20:16. doi: 10.1186/s12915-021-01221-y
Cianfanelli, F. R., Monlezun, L., and Coulthurst, S. J. (2016). Aim, load, fire: the type VI secretion system, a bacterial Nanoweapon. Trends Microbiol. 24, 51–62. doi: 10.1016/j.tim.2015.10.005
Coulthurst, S. (2019). The type VI secretion system: a versatile bacterial weapon. Microbiology 165, 503–515. doi: 10.1099/mic.0.000789
Edgar, R. C. (2004). MUSCLE: multiple sequence alignment with high accuracy and high throughput. Nucleic Acids Res. 32, 1792–1797. doi: 10.1093/nar/gkh340
Fitzsimons, T. C., Lewis, J. M., Wright, A., Kleifeld, O., Schittenhelm, R. B., Powell, D., et al. (2018). Identification of novel Acinetobacter baumannii type VI secretion system antibacterial effector and immunity pairs. Infect. Immun. 86:e00297-18. doi: 10.1128/IAI.00297-18
Flaugnatti, N., Le, T. T., Canaan, S., Aschtgen, M. S., Nguyen, V. S., Blangy, S., et al. (2016). A phospholipase A1 antibacterial type VI secretion effector interacts directly with the C-terminal domain of the VgrG spike protein for delivery. Mol. Microbiol. 99, 1099–1118. doi: 10.1111/mmi.13292
Fridman, C. M., Keppel, K., Gerlic, M., Bosis, E., and Salomon, D. (2020). A comparative genomics methodology reveals a widespread family of membrane-disrupting T6SS effectors. Nat. Commun. 11:1085. doi: 10.1038/s41467-020-14951-4
Greener, J. G., Kandathil, S. M., and Jones, D. T. (2019). Deep learning extends de novo protein modelling coverage of genomes using iteratively predicted structural constraints. Nat. Commun. 10:3977. doi: 10.1038/s41467-019-11994-0
Hachani, A., Allsopp, L. P., Oduko, Y., and Filloux, A. (2014). The VgrG proteins are "a la carte" delivery systems for bacterial type VI effectors. J. Biol. Chem. 289, 17872–17884. doi: 10.1074/jbc.M114.563429
Hachani, A., Wood, T. E., and Filloux, A. (2016). Type VI secretion and anti-host effectors. Curr. Opin. Microbiol. 29, 81–93. doi: 10.1016/j.mib.2015.11.006
Hood, R. D., Peterson, S. B., and Mougous, J. D. (2017). From striking out to striking gold: discovering that type VI secretion targets bacteria. Cell Host Microbe 21, 286–289. doi: 10.1016/j.chom.2017.02.001
Hwang, H. H., Yu, M., and Lai, E. M. (2017). Agrobacterium-mediated plant transformation: biology and applications. Am. Soc. Plant Biol. 15:e0186. doi: 10.1199/tab.0186
Jablonska, J., Matelska, D., Steczkiewicz, K., and Ginalski, K. (2017). Systematic classification of the his-me finger superfamily. Nucleic Acids Res. 45, 11479–11494. doi: 10.1093/nar/gkx924
Jana, B., Fridman, C. M., Bosis, E., and Salomon, D. (2019). A modular effector with a DNase domain and a marker for T6SS substrates. Nat. Commun. 10:3595. doi: 10.1038/s41467-019-11546-6
Jumper, J., Evans, R., Pritzel, A., Green, T., Figurnov, M., Ronneberger, O., et al. (2021). Highly accurate protein structure prediction with AlphaFold. Nature 596, 583–589. doi: 10.1038/s41586-021-03819-2
Justice, S. S., Hunstad, D. A., Cegelski, L., and Hultgren, S. J. (2008). Morphological plasticity as a bacterial survival strategy. Nat. Rev. Microbiol. 6, 162–168. doi: 10.1038/nrmicro1820
Koskiniemi, S., Lamoureux, J. G., Nikolakakis, K. C., T'Kint de Roodenbeke, C., Kaplan, M. D., Low, D. A., et al. (2013). Rhs proteins from diverse bacteria mediate intercellular competition. Proc. Natl. Acad. Sci. USA 110, 7032–7037. doi: 10.1073/pnas.1300627110
LaCourse, K. D., Peterson, S. B., Kulasekara, H. D., Radey, M. C., Kim, J., and Mougous, J. D. (2018). Conditional toxicity and synergy drive diversity among antibacterial effectors. Nat. Microbiol. 3, 440–446. doi: 10.1038/s41564-018-0113-y
Lai, E. M., and Kado, C. I. (1998). Processed VirB2 is the major subunit of the promiscuous pilus of Agrobacterium tumefaciens. J. Bacteriol. 180, 2711–2717. doi: 10.1128/JB.180.10.2711-2717.1998
Li, Y., Yan, X., and Tao, Z. (2022). Two type VI secretion DNase effectors are utilized for interbacterial competition in the fish pathogen Pseudomonas plecoglossicida. Front. Microbiol. 13:869278. doi: 10.3389/fmicb.2022.869278
Lien, Y. W., and Lai, E. M. (2017). Type VI secretion effectors: methodologies and biology. Front. Cell. Infect. Microbiol. 7:254. doi: 10.3389/fcimb.2017.00254
Ma, L. S., Hachani, A., Lin, J. S., Filloux, A., and Lai, E. M. (2014). Agrobacterium tumefaciens deploys a superfamily of type VI secretion DNase effectors as weapons for interbacterial competition in planta. Cell Host Microbe 16, 94–104. doi: 10.1016/j.chom.2014.06.002
Ma, J., Pan, Z., Huang, J., Sun, M., Lu, C., and Yao, H. (2017). The Hcp proteins fused with diverse extended-toxin domains represent a novel pattern of antibacterial effectors in type VI secretion systems. Virulence 8, 1189–1202. doi: 10.1080/21505594.2017.1279374
Mistry, J., Chuguransky, S., Williams, L., Qureshi, M., Salazar, G. A., Sonnhammer, E. L. L., et al. (2021). Pfam: the protein families database in 2021. Nucleic Acids Res. 49, D412–D419. doi: 10.1093/nar/gkaa913
Pei, T. T., Li, H., Liang, X., Wang, Z. H., Liu, G., Wu, L. L., et al. (2020). Intramolecular chaperone-mediated secretion of an Rhs effector toxin by a type VI secretion system. Nat. Commun. 11:1865. doi: 10.1038/s41467-020-15774-z
Pissaridou, P., Allsopp, L. P., Wettstadt, S., Howard, S. A., Mavridou, D. A. I., and Filloux, A. (2018). The Pseudomonas aeruginosa T6SS-VgrG1b spike is topped by a PAAR protein eliciting DNA damage to bacterial competitors. Proc. Natl. Acad. Sci. U. S. A. 115, 12519–12524. doi: 10.1073/pnas.1814181115
Santos, M. N. M., Cho, S. T., Wu, C. F., Chang, C. J., Kuo, C. H., and Lai, E. M. (2020). Redundancy and specificity of type VI secretion vgrG loci in antibacterial activity of Agrobacterium tumefaciens 1D1609 strain. Front. Microbiol. 10:3004. doi: 10.3389/fmicb.2019.03004
Schindelin, J., Arganda-Carreras, I., Frise, E., Kaynig, V., Longair, M., Pietzsch, T., et al. (2012). Fiji: an open-source platform for biological-image analysis. Nat. Methods 9, 676–682. doi: 10.1038/nmeth.2019
Shneider, M. M., Buth, S. A., Ho, B. T., Basler, M., Mekalanos, J. J., and Leiman, P. G. (2013). PAAR-repeat proteins sharpen and diversify the type VI secretion system spike. Nature 500, 350–353. doi: 10.1038/nature12453
Soding, J., Biegert, A., and Lupas, A. N. (2005). The HHpred interactive server for protein homology detection and structure prediction. Nucleic Acids Res. 33, W244–W248. doi: 10.1093/nar/gki408
Ting, S. Y., Bosch, D. E., Mangiameli, S. M., Radey, M. C., Huang, S., Park, Y. J., et al. (2018). Bifunctional immunity proteins protect bacteria against FtsZ-targeting ADP-Ribosylating toxins. Cell 175, 1380–1392.e14. doi: 10.1016/j.cell.2018.09.037
Varadi, M., Anyango, S., Deshpande, M., Nair, S., Natassia, C., Yordanova, G., et al. (2022). AlphaFold protein structure database: massively expanding the structural coverage of protein-sequence space with high-accuracy models. Nucleic Acids Res. 50, D439–D444. doi: 10.1093/nar/gkab1061
Wang, J., Brodmann, M., and Basler, M. (2019). Assembly and subcellular localization of bacterial type VI secretion systems. Ann. Rev. Microbiol. 73, 621–638. doi: 10.1146/annurev-micro-020518-115420
Waterhouse, A., Bertoni, M., Bienert, S., Studer, G., Tauriello, G., Gumienny, R., et al. (2018). SWISS-MODEL: homology modelling of protein structures and complexes. Nucleic Acids Res. 46, W296–W303. doi: 10.1093/nar/gky427
Whitney, J. C., Beck, C. M., Goo, Y. A., Russell, A. B., Harding, B., De Leon, J. A., et al. (2014). Genetically distinct pathways guide effector export through the type VI secretion system. Mol. Microbiol. 92, 529–542. doi: 10.1111/mmi.12571
Wu, C. C., Lin, J. L. J., and Yuan, H. S. (2020). Structures, mechanisms, and functions of His-Me finger nucleases. Trends Biochem. Sci. 45, 935–946. doi: 10.1016/j.tibs.2020.07.002
Wu, C. F., Santos, M. N. M., Cho, S. T., Chang, H. H., Tsai, Y. M., Smith, D. A., et al. (2019). Plant-pathogenic Agrobacterium tumefaciens strains have diverse type VI effector-immunity pairs and vary in in-planta competitiveness. Mol Plant Microbe Int. 32, 961–971. doi: 10.1094/MPMI-01-19-0021-R
Wu, C. F., Weisberg, A. J., Davis, E. W. 2nd, Chou, L., Khan, S., Lai, E. M., et al. (2021). Diversification of the type VI secretion system in Agrobacteria. MBio 12:e0192721. doi: 10.1128/mBio.01927-21
Yu, M., and Lai, E. M. (2017). Warfare between host immunity and bacterial weapons. Cell Host Microbe 21, 3–4. doi: 10.1016/j.chom.2016.12.012
Keywords: type VI secretion system (T6SS), interbacterial antagonism, bacterial toxin, nuclease, Agrobacterium
Citation: Santos MNM, Pintor KL, Hsieh P-Y, Cheung Y-W, Sung L-K, Shih Y-L and Lai E-M (2024) Agrobacteria deploy two classes of His-Me finger superfamily nuclease effectors exerting different antibacterial capacities against specific bacterial competitors. Front. Microbiol. 15:1351590. doi: 10.3389/fmicb.2024.1351590
Edited by:
Peter Graumann, University of Marburg, GermanyReviewed by:
Pratick Khara, University of Texas Health Science Center at Houston, United StatesRonghao Chen, The Ohio State University, United States
Copyright © 2024 Santos, Pintor, Hsieh, Cheung, Sung, Shih and Lai. This is an open-access article distributed under the terms of the Creative Commons Attribution License (CC BY). The use, distribution or reproduction in other forums is permitted, provided the original author(s) and the copyright owner(s) are credited and that the original publication in this journal is cited, in accordance with accepted academic practice. No use, distribution or reproduction is permitted which does not comply with these terms.
*Correspondence: Erh-Min Lai, emlai@gate.sinica.edu.tw
†Present addresses
Katherine L. Pintor, Department of Ecophysiology, Max Planck Institute for Terrestrial Microbiology, Marburg, Germany
Pei-Yu Hsieh, Department of Pharmacology, University of Cambridge, Cambridge, United Kingdom
‡ORCID: Mary Nia M. Santos, https://orcid.org/0000-0001-6544-0339
Katherine L. Pintor, https://orcid.org/0000-0001-9137-4726
Pei-Yu Hsieh, https://orcid.org/0000-0002-7263-1691
Yee-Wai Cheung, https://orcid.org/0000-0002-1421-4419
Li-Kang Sung, https://orcid.org/0000-0002-7078-8003
Yu-Ling Shih, https://orcid.org/0000-0002-8232-2569
Erh-Min Lai, https://orcid.org/0000-0003-3630-8683