- Department of Physiology, School of Medical Sciences, New Zealand National Eye Centre, University of Auckland, Auckland, New Zealand
UV light is known to cause damage to biomolecules in living tissue. Tissues of the eye that play highly specialised roles in forming our sense of sight are uniquely exposed to light of all wavelengths. While these tissues have evolved protective mechanisms to resist damage from UV wavelengths, prolonged exposure is thought to lead to pathological changes. In the lens, UV light exposure is a risk factor for the development of cataract, which is a condition that is characterised by opacity that impairs its function as a focusing element in the eye. Cataract can affect spatially distinct regions of the lens. Age-related nuclear cataract is the most prevalent form of cataract and is strongly associated with oxidative stress and a decrease in the antioxidant capacity of the central lens region. Since UV light can generate reactive oxygen species to induce oxidative stress, its effects on lens structure, transparency, and biochemistry have been extensively investigated in animal models in order to better understand human cataract aetiology. A review of the different light exposure models and the advances in mechanistic understanding gained from these models is presented.
1 Introduction
1.1 The cataract epidemic
Our sense of sight is critically dependent on the ability of the ocular lens to maintain its transparent and refractive properties over many decades of life. Failure to maintain lens transparency results in opacification of the lens due to the scattering of transmitted light rays. Lens opacification, or cataracts, are the leading cause of vision impairment and blindness worldwide (1), accounting for around half of all forms of vision loss (2). While cataract is a multi-factorial pathology, with genetics, increasing age, diabetes, and environmental factors such as exposure to cigarette smoking (3) and alcohol use (4) all contributing to its development, exposure to sunlight (UV radiation) is also a major risk factor (5–7), which can exacerbate different types of cataract.
Cortical cataract, the second most prevalent form of cataract, occurs earlier than age-related nuclear (ARN) cataract (8–10), and progresses slowly before manifesting as tissue damage in the outer cortex of the lens (Figure 1A) (11). In contrast, ARN cataract in the human lens (Figure 1B) occurs when the intrinsic repair and protection mechanisms that exist to mitigate the effects of oxidative stress slowly deteriorate or become ineffective (12). Under oxidative stress conditions, thiol groups of proteins are easily oxidised to form protein mixed disulfides with oxidised glutathione (PSSG), cysteine (PSSC), and eventually, protein:protein cross-links (PSSP) (12). This accumulated damage can change protein structure and function, and leads to protein aggregation and insolubilisation (13, 14), which causes the light scattering that is characteristic of ARN cataract. Posterior subcapsular cataracts (Figure 1C) are characterised by dysplasia of the equatorial epithelial cells (15). On their own, they are relatively uncommon (16), and are generally associated with other types of opacities, especially in those aged >80 years old (17).
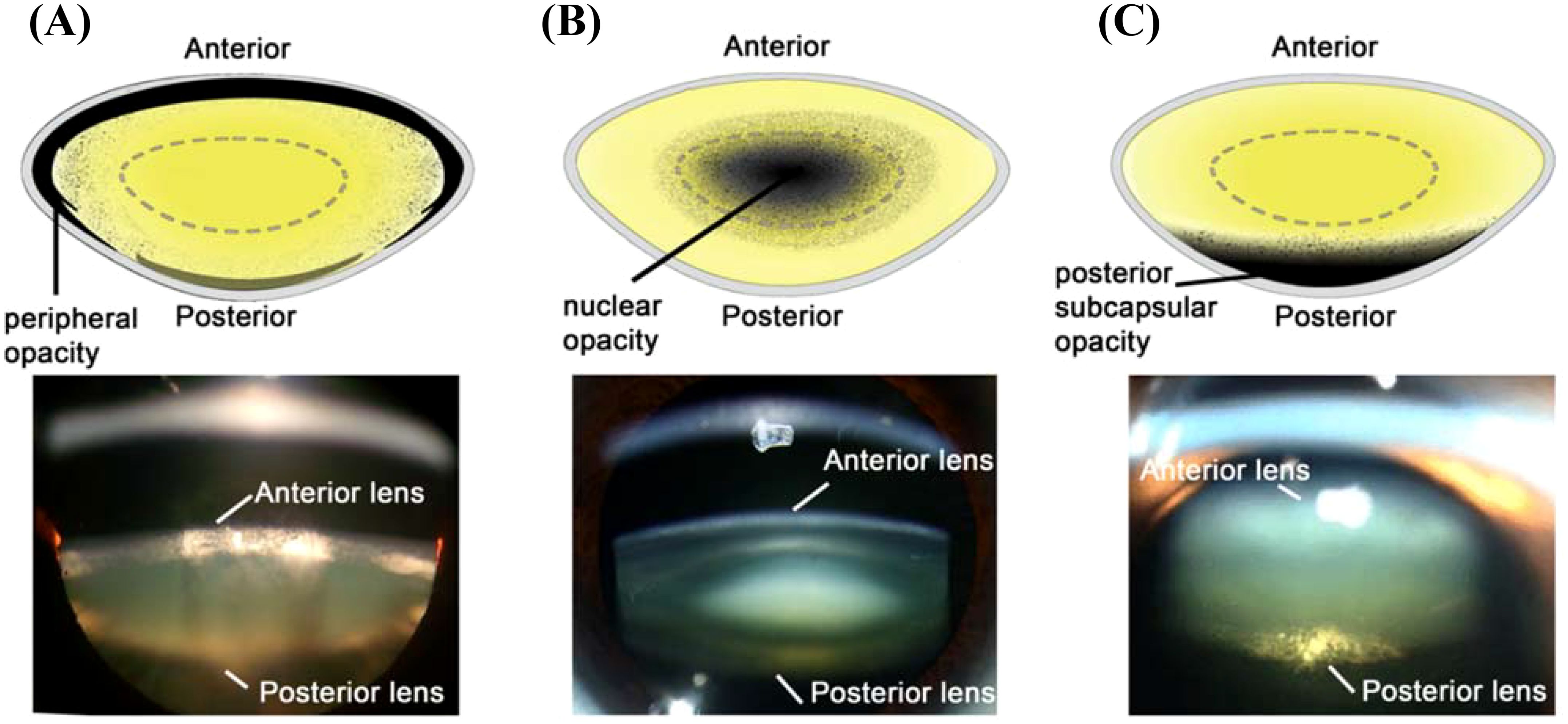
Figure 1. Schematic diagrams (Top) and Scheimpflug slit-lamp photographic (bottom) images of the three main types of cataracts. (A) cortical cataract, from Uspal NG, Schapiro ES (2011). Cataracts as the initial manifestation of type 1 diabetes mellitus. Paediatric Emergency Care. 27 (2): 132–4. (B) Nuclear cataract, from Ophthalmic Atlas Images by EyeRounds.org. (C) Posterior subcapsular cataract, from Chaudhary M, Shah DN, Chaudhary, RP (2017). Scleritis and Takayasu’s disease. Nepalese Journal of Ophthalmology (18): 170–174. Reproduced with permission from MDPI under Creative Commons Attribution (CC BY 4.0).
Currently, the only treatment for human cataract is surgical removal of the opaque lens and implantation of an intra-ocular lens (IOL). Cataract surgery is one of the most commonly performed elective surgical procedures in developed countries (18) and is highly successful. The main outcomes include a marked improvement in visual acuity, decreased risk of falls, and improved quality of life (19, 20). In economically developed countries, cataract blindness in the community is rare, yet across developing countries with low rates of cataract surgery, blindness from unoperated cataract is common (21). Cataract surgery is a substantial cost to global health systems. For example, in the USA, approximately 3 million surgeries are performed each year, with an estimated cost of >$3.4 billion in annual Medicare spending (19, 22). In developing countries, costs associated with cataract surgery can be prohibitive (23, 24). Hence there is a need to develop more cost-effective therapeutic alternatives to cataract surgery to delay, prevent, or reverse cataract formation (25).
Unfortunately, investigating the causes and mechanisms of human cataract formation and the ongoing effort to develop non-surgical anti-cataract therapies has associated difficulties. The use of post-mortem human donor tissue suffers from an inconsistent supply of cataractous lenses (26), as well as variable post-mortem delays between death and tissue processing (27). In addition, lenses obtained from human donors have significant biochemical variability. For example, the lifestyle, genetics, underlying diseases, and causes of death of individual donors will all contribute to this variability, and therefore the consistency of subsequent analysis. Finally, whole, cataractous lenses are now less readily available (28) due to the arrival of the extracapsular cataract surgical extraction (ECCE) method (29), where the nucleus and cortex are now emulsified and removed, leaving the capsule behind that can then be used to hold the IOL implant.
As a consequence of these challenges to utilising human tissue in cataract research, animal models have been used to investigate the underlying mechanisms of cataract formation (26) following a range of cataractous insults. While animal models of cataract aim to recapitulate the characteristics seen in human cataract that take many decades to develop, they are often induced in a laboratory environment over a relatively short time period. Lens parameters that are typically monitored in these models include transparency and morphological changes (that induce light scattering), biochemical changes (such as antioxidant depletion and pigmentation), and biomechanical changes (such as stiffening of the lens) that only manifest as cataract in later in life (30–32).
Animal models that mimic the distinctly different cataract phenotypes observed in ARN cataract (27) and diabetic cortical cataract (33) have previously been reviewed. In this review, animal models used to determine the mechanisms of lens cataract formation following exposure to UV radiation are presented and evaluated. We will first review the evidence for the cataractous effects of UV radiation in humans, and the intrinsic properties that the human eye has to protect against cataract formation. This will provide a contextualisation of the animal models used to study the role of UV exposure in cataract formation.
1.2 UV light in human cataract formation: exposure, epidemiology, and effects of aging
UV radiation is a known toxin to biological tissues and is classified as a carcinogen (34). The sun produces UV radiation in the UV-A, -B, and -C ranges. Approximately 97% of the wavelengths of radiation that pass through the atmosphere and reach Earth are UV-A (λ = 315-400nm), while ~3% is UV-B (λ = 280-315nm) (35, 36). Solar UV-C (λ = 200-280nm) is blocked by the Earth’s atmosphere (35) and UV-C wavelengths are produced in only a few settings on Earth, such as Arc welding.
Three main types of tissue damage can result from light exposure. While photothermal and photomechanical damage typically result from exposure to the upper end of visible and infrared light wavelengths, photochemical damage is the result of exposure to wavelengths in the UV and visible light range (37). Photochemical damage is further divided into three types. Ablation is utilised extensively in ophthalmology, where high energy wavelengths under 200 nm remove or shape ocular tissue structures. In contrast, both photo-oxidative damage and photosensitised reactions are the result of UV-A and UV-B exposure, typically as a result of long exposure times (37).
Several mechanisms have evolved to protect the eye from the phototoxic effects of UV radiation. For example, the cornea absorbs the majority of incoming UV-B light and a small amount of UV-A (38–41). However, the age of the eye has an impact on UV light penetration and consequently the amount of UV light entering the eye and reaching the lens increases with age (38). Once adulthood is reached, it is assumed that the retina is no longer exposed to UV radiation, due to the decreasing transmission properties of the lens (42). The lens absorbs most of the incoming UV-A, and the small amount of UV-B radiation that is not absorbed by the cornea (Table 1) (39, 40).
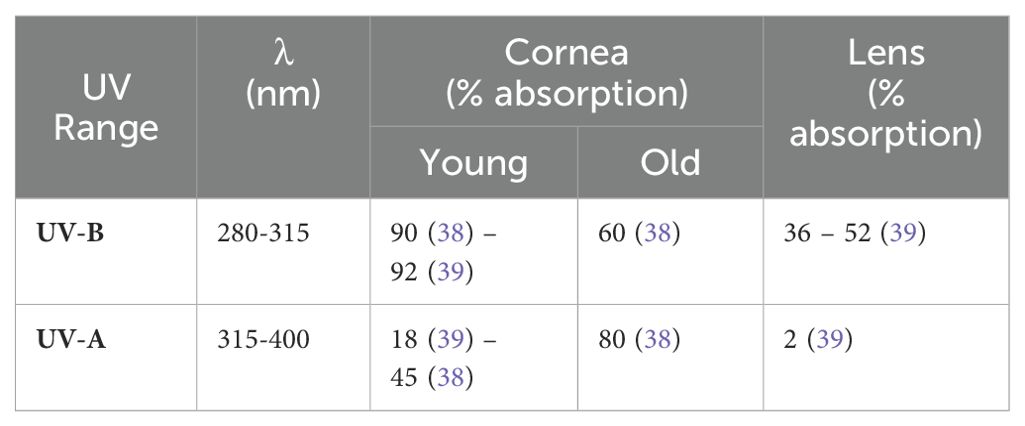
Table 1. Corneal absorbance of incoming UV light as a function of age, and lenticular absorbance of incoming light.
Considerable epidemiological evidence shows the harmful effects of different UV wavelengths of light on the lens. The World Health Organization estimates that cataracts in up to 20% of the people who become blind annually may be caused or enhanced by sun exposure (43). Generally, UV-B light has been associated with an increased risk of cortical cataracts (Figure 1A) and subcapsular cataract (Figure 1C) (44–46), but there is less evidence for the effects of UV-B exposure on nuclear cataracts in humans (47, 48). This is possibly due to its limited depth of penetration into the lens in humans (49), monkeys (50), and rats (51). Although once dismissed as a risk factor for cataract, UV-A has since been associated with nuclear cataract formation (Figure 1B) (52, 53), with UV-A light shown to penetrate deep into the lens nucleus of guinea pigs (44).
Epidemiological studies have shown that higher rates of cataract are observed in populations that spend more time outdoors (54) or in the sun (55), in rural as opposed to urban living (56–58), and other specific geospatial relationships (17, 47, 48, 57, 59–73). For example, higher exposure to sunlight significantly increases the risk of age-related cataract, with a slight increased risk of cortical cataract, but no risk effect on nuclear or posterior subcapsular cataract (74). This higher exposure to sunlight can be from reflection of UV from different surfaces in the environment, with snowfields and/or increased altitude (75) having the most reflection, and forest the least (76). Interestingly, prevalence of the type of cataract appears to change with global location. Sasaki and colleagues showed that cortical opacification was more prevalent in Iceland and Japan, while nuclear cataract was more prevalent amongst Singaporeans (77). Furthermore, variations in populations within Japan show an increased prevalence for nuclear cataract formation in Okinawa due to high UV exposure (78).
Brunescence, the process of progressive pigmentation of the aging human lens which turns a young, colourless lens increasingly yellow, brown and even black, has been specifically linked to UV exposure (53). Moreover, brunescent cataracts are particularly prevalent in populations living in tropical regions of the world due to their higher exposure to solar radiation (76, 79, 80). Several of the chromophores and fluorophores (81, 82) responsible for lens colouration have been isolated and identified, including advanced glycation end products (83–86), and tryptophan oxidation products (87–89). Interestingly, some of these tryptophan metabolites are beneficial in young lenses where they play an important role in the intrinsic UV protection mechanism of the eye but become detrimental to the lens following chronic exposure to UV.
1.3 Lens UV exposure protection mechanisms
The young lens contains several tryptophan metabolites, which act as UV filter compounds that absorb light in the 300 to 400 nm wavelength range (90, 91). Approximately 95% of the light that enters the lens is absorbed by these compounds, with the remaining 5% being absorbed by tryptophan residues on proteins (92). UV filters also decrease chromatic aberration, thus enhancing visual acuity (93), and aid in protecting the retina from induced photo-oxidative damage (92). Synthesis of UV filters occurs between late pregnancy and birth, with some filters detectable in lenses five months post-natal (94). There are two main types of filters: primary and secondary filters. In young lenses, the ratio of primary to secondary is approximately 10:1, but this decreases with age to 2:1 (91).
When found in their free form, both primary and secondary filters are photochemically inert, and act to dissipate UV energy (95) without the production of harmful radicals (93, 96, 97), that could induce oxidative stress (97) (Figure 2). Photo-oxidative damage occurs when incident light reacts with a tissue chromophore such as a UV filter, which then attains an excited state. Reactive oxygen species (ROS) are generated through interaction of the excited state chromophore with a variety of substrates, which go on to oxidatively damage biomolecules (37). In contrast, photosensitisation reactions occur when oxygen and a photosensitiser molecule absorb the UV to produce hydrogen peroxide (H2O2). This can either be detoxified by the action of glutathione peroxidase, or go on to form the hydroxyl radical, which can damage a range of biomolecules, including DNA, proteins, and lipids (37).
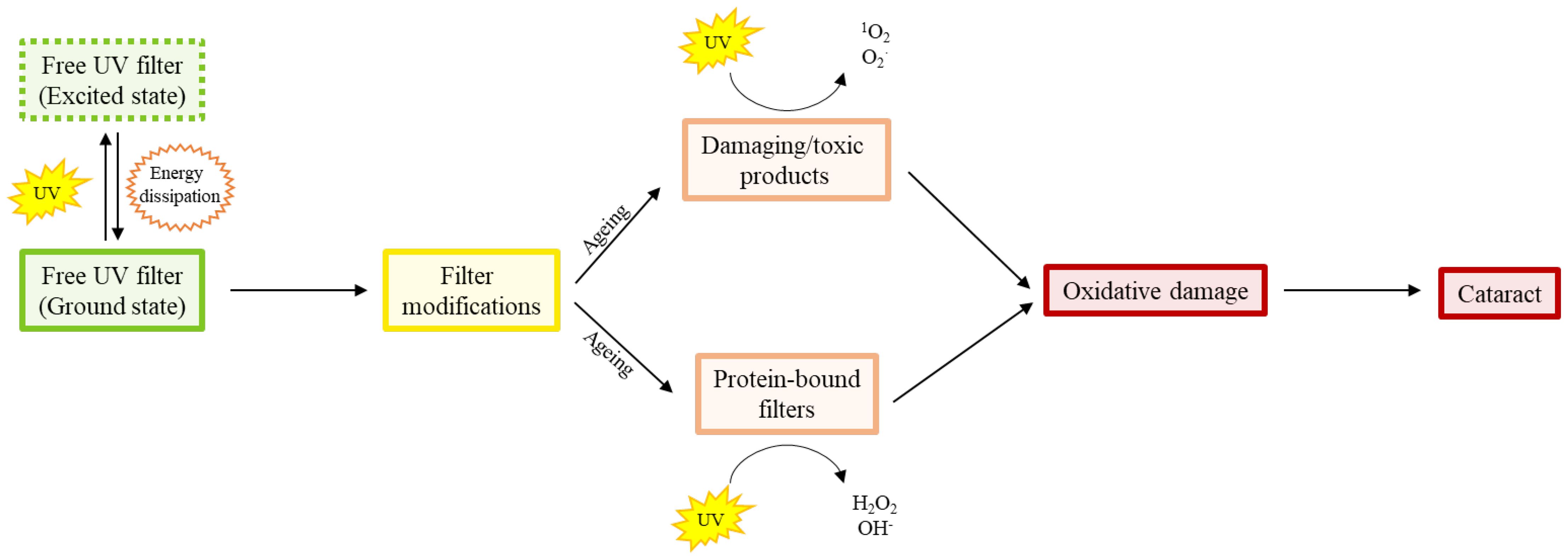
Figure 2. Diagram showing the age-related shift in proportion of free human UV filters to modified free and protein bound filters that produce oxidatively damaging species. The free (i.e. not bound to proteins) filters absorb UV light and dissipate the UV energy efficiently. However, with increasing age, there is conversion of filters to different compounds which produce singlet oxygen and superoxide radicals, and the binding of filters to proteins which produce peroxide and hydroxyl radicals, in response to UV light. It is this age-dependent accumulation of oxidative damage that is thought to be responsible for the initiation of ARN cataract.
While the young lens contains high levels of glutathione (GSH) to protect it from oxidative stress through direct neutralisation of ROS, the age-related decline in this key antioxidant makes the lens vulnerable to cataract formation. This is due to the high concentration of cell membranes in the lens, which make it vulnerable to damage from free radical-mediated lipid peroxidation (37), its high protein concentration which can form irreversible protein-protein cross-links (12), and a variety of naturally occurring small molecules, such as UV filter molecules. While UV filters are highly efficient at dissipating energy, modifications to the filters, and the binding of filters to proteins within the lens, can change their ability to quench UV radiation (95), and instead act as photosensitisers in the aging human lens (98). These filters and their modifications are discussed herein, and their classification summarised (Table 2).
The primary UV filters in the human lens are kynurenine (kyn), 3-hydroxykynurenine (3OHK), and 3-hydroxykynurenine O-β-D-glucoside (3OHKG) (99–102). One of the intermediates in the formation of kyn from tryptophan metabolism, N-formyl-kynurenine (NFK), differs from other tryptophan metabolites in its photophysical properties, in that it acts as a photosensitiser to produce singlet oxygen and superoxide (103, 104). In the presence of oxygen, NFK is synthesised enzymatically by indoleamine 2,3-dioxygenase (IDO), which has been found in human lenses (105), or through tryptophan photolysis following in vitro exposure to UV light (106). NFK has been shown to bind to crystallin proteins under oxidative stress in vitro (107), and during exposure to sunlight (108), suggesting that in the absence of UV filters, it could be a key mediator of UV light induced damage in the lens. 3OHKG is the most abundant filter (109), and is formed via glycosylation of 3OHK (110). Kyn, 3OHK and 3OHKG are found prominently in young lenses (102), but decline at a rate of ~12% per decade, with kyn and 3OHK being nearly undetectable in 80 year old lenses (110).
The amino acid side chain of primary filters is unstable, and is thought to be able to spontaneously deaminate, to form an α-β-ketoalkene (109, 111–113), which is also highly unstable (94). The primary filters are able to form GSH adducts, whereby a molecule of GSH scavenges the deaminated filter, potentially protecting lens proteins from covalent binding of filters (111, 114). NAD(P)H has also been identified as a protective agent, scavenging the deaminated filters (109). High concentrations of GSH, such as those in young lenses, can protect the lens in two ways: by scavenging filter deamination products, and promoting the decomposition of kyn-protein adducts. GSH-conjugated UV filters increase with a corresponding decrease in free GSH, and therefore may contribute to a decreased capacity for nuclear GSH to protect lens proteins from cross-linking and insolubilisation (110). In addition to glutathionylation, all three primary filter compounds can also undergo cyclisation to form 3OHKG-yellow, kyn-yellow, and 3OHK-yellow respectively, although this is thought to be a slow process (109). Enzymatic modification of kyn can result in the formation of kynurenic acid, which acts as a photosensitiser and produces reactive oxygen species (104).
The secondary filters 4-(2-amino-3-hydroxyphenyl)-4-oxobutanoic acid O-β-D-glucoside (AHBG) and glutathionyl-3-hydroxykynurenine O-β-D-glucoside (GSH-3OHKG) are found predominantly in the lens nucleus (98, 110, 115, 116). The α-β-ketoalkene formed through primary filter deamination undergoes reduction to form AHBG (94), binds to GSH to create GSH-3OHKG (117), or free cysteine (118), and can bind to proteins through lysine, cysteine and histidine (119). 3OHK can also form 3-hydroxyanthranilic acid (3OAA), through the enzyme kynureninase (102). This molecule is also photochemically inert and inhibits the crosslinking of crystallins within the lens (104). High levels of GSH should prevent the autooxidation of 3OAA, but with falling GSH levels in aging lens, autooxidation can occur, producing H2O2 that can damage crystallins (120). For secondary filters, AHBG can undergo additional glycosylation to create 4-(2-amino-3-hydroxyphenyl)-4-oxobutanoic acid O-β-D-di-glucoside (AHBGD), but neither of these filters can bind to lens proteins. This is because neither compound is able to undergo deamination, in contrast to the other filters (121).
With increasing age, the levels of free UV filters decrease markedly (110), to the point where protein-bound UV filters and free UV filters are equal in concentration in the centre of normal lenses (122). UV filters, however, are present in cataractous tissue at higher concentrations than aged-matched controls (123). Deamination of the UV filters appears to be more pronounced in the nuclear region of the lens (110). This, in combination with the age-related decrease in nuclear GSH (124), would make the nuclear region more susceptible to the covalent linkage of UV filters to crystallin proteins.
In addition to binding to proteins, UV filters also create some damaging products. Xanthurenic acid (XA) is proposed to be one of the damaging products created through filter modification, although there are conflicting findings on whether or not XA is present in normal human lenses (103). However, it is present in cataractous lenses (125), with its glucoside (XAG), being present in brunescent cataracts (95, 126). XA could be formed enzymatically within the lens, from 3OHK, or through oxidation of 3OHK-yellow (127, 128), or through 3OHKG (95, 129). In addition to its glucoside, XA can be oxidised to form oxo-xanthurenic acid (OXA) and subsequently dioxo-xanthurenic acid (DOXA). DOXA may induce oxidative stress by generating oxygen free radicals, and also denature proteins through the crosslinking of crystallin proteins within the lens (128).
It is hypothesised that instead of protecting the lens from oxidative damage, the protein-bound UV filters may initiate oxidative damage, or act as an oxidant (130), resulting in the formation of proteins with altered physical and chemical properties (96, 98). These alterations include cross-linking, oxidation, fragmentation, peroxide formation, amino acid isomerisation, unfolding, and alterations to protein solubility (96–98, 131–134). The coloration or brunescence seen in the cataractous lenses is thought to be a result of accumulated oxidative reactions involving protein bound UV filters (121).
In summary, it has been shown that the human lens has developed a collection of filters to absorb the UV-A and UV-B light that passes through the cornea and penetrates into the different regions of the lens. UV light causes the degradation of these filters, with GSH preventing some of these damaged filters from binding to proteins. With age, the amount of UV light reaching the lens increases as the UV filtering capacity of the cornea declines. This increase in the incidence of UV light, plus a reduction in the efficacy of the filters and a parallel age-related decrease in the GSH availability in the lens, produces oxidative stress that leads to cataract formation. In the next sections, we review what we have learnt about the effects of UV light on lens transparency from a variety of different animal models and critically assess whether these models accurately model the effects of UV exposure seen in the human lens.
1.4 The use of animal models to mimic UV-induced cataract in humans
To understand how UV radiation induces lens cataract, a considerable number of studies have exposed animal lenses, either in vivo or ex vivo, to UV light (Supplementary Table 1). For in vivo models, sub-threshold doses can be applied over many days as cumulative, chronic doses, whereas ex vivo models are subject to tissue degradation, and therefore often use acute, super-threshold doses. While in vivo models can better mimic the processes that occur in a whole system than an ex vivo lens, this comes at added time and financial cost. In addition, the penetration of UV light through the cornea changes depending on the animal model used. Hence, ex vivo models that use the lens alone must also consider that the dose given to the lens may be different to what the lens would experience in vivo, due to the lack of protection from the cornea. While both in vivo and ex vivo models can be used to assess recovery of lens tissue post-exposure, ex vivo models are again constrained by tissue degradation and time post-mortem. Despite these limitations, ex vivo models can be exposed to large doses of UV without concerns for animal welfare. In addition, lenses from larger animals, such as pigs and cows, can often be obtained as a by-product from abattoirs and are more cost effective than tissue derived from smaller laboratory animals. The downside of this, however, is that the exact age and other potential confounding factors such as disease, sex, and post-mortem time is less precise than small laboratory animals sourced in-house.
Despite the above factors, both in vivo and ex vivo models have been very effective in elucidating the mechanisms underlying cataract initiation and progression following UV exposure (Table 3). However, the relevance of the chosen animal model to the level of exposure and cataract development in the human lens is often not critically assessed. In each section of this review, we have assessed the relative merits of the existing animal models of UV cataract and have assigned the models to one of two categories: 1) Nocturnal animal models where “non-environmental” UV exposure serves as an oxidative stress that compromises lens transparency, and 2) UV light exposure in crepuscular and diurnal animals that could act as more relevant models that mimic the effects of UV light on cataract development in humans. While many of these animal studies investigated alterations in gene expression (135–140) and DNA damage (141, 142) upon irradiation, in this review we focus on the morphological, biochemical, metabolic, and protein changes that characterise the cataract phenotype induced as a result of UV-A or UV-B exposure.
2 Nocturnal animal models of UV as an oxidative stress
Due to their size and ease of housing, mice and rats have proven to be popular choices for the development of models of UV cataract formation. However, the most widely used rodent animal models are nocturnal and not naturally exposed to the high levels of UV light experienced by diurnal animals. Moreover, rodent models are often exposed to UV light at much higher doses than diurnal animals experience environmentally in order to shorten the experimental time course required for the development of cataract. Due to their low natural exposure to UV radiation nocturnal animals do not express the same system of UV filters seen in the human lens. Therefore, the same radiation energy dissipation that occurs in the human lens does not occur in mice and rat lenses that do not have UV filters.
2.1 Mice
Mice have been used as models for many types of cataract (see (27)). Important differences between the mouse and human lens include a different distribution of β-crystallins (143) and crystallin proteins that are modified differently (144). Critically, however, mice see in the ultraviolet range (145), and thus their lenses contain no UV filters to absorb UV radiation (32). Despite these differences, mice have been used to study the effects of both UV-A and UV-B radiation on lens protein content, as well as the morphological and biochemical status of UV-exposed lens. Murine tissue has also been used to assess the efficacy of external agents, such as caffeine and ascorbate, in preventing UV cataract in vivo (146, 147).
2.1.1 Cataract phenotypes induced by UV light exposure
To establish the relative toxicity of UV light exposure to lenses, mice have been exposed to UV-A or UV-B for up to 39 weeks. UV-A exposure was found to be weakly cataractogenic when compared to UV-B in albino mice in vivo (148, 149). In vivo exposure of mice to UV-B has not only induced subcapsular cataract, but also cortical and nuclear cataract (150). Further development of this model showed that when only one eye was exposed to UV-B, the non-exposed eye suffered intraocular inflammation and an increase in lens light scattering also, perhaps due to a co-cataractogenic inflammatory response (151).
2.1.2 Effects of UV light on metabolism, antioxidant pathways and protein function
Changes to lenticular protein concentration of albino mice in response to UV-A has been investigated in vivo (152, 153). Following long-term exposure to UV-A (up to 87 weeks), insoluble protein levels rose to 46% higher than controls (153), which is similar to the accumulation of insoluble proteins observed in ARN cataracts in humans (124, 154). Subcapsular and cortical opacities were observed between 30 and 50 weeks, after which anterior cortical cloudiness was observed. While the cataract phenotype observed here was different to that observed in humans, these results confirmed that long-term in vivo exposure to UV-A light leads to cataract in an albino mouse model.
Morphological and biochemical alterations produced as a result of in vivo exposure of mice to UV-B radiation have been investigated (155). Within two days of exposure, the mice had developed anterior subcapsular cataracts, similar to results from another study (156), with the onset of morphological changes beginning at 24 hours post-exposure. Importantly, older mice showed more prominent macroscopic changes compared to younger mice, and GSH depletion was more prominent in the older lenses than the younger lenses, again reflecting changes observed in human lenses. Glyceraldehyde-3-phosphate dehydrogenase (G3PD) inactivation was more exaggerated in the older lenses, diminishing ATP production and having a direct impact on lens transparency.
In addition, the enzymes thioltransferase (TTase) and thioredoxin (Trx) were upregulated following UV exposure, likely providing oxidative damage repair in the younger mice. Trx has been shown to play an important role in defending against UV-A light in cultured human epithelial cells (157). The decrease in G3PD function, which can be restored by dethiolation of TTase, was thought to be a result of suppressed enzyme activity by UV exposure, rather than a direct effect on protein expression levels. While there are differences in how deep UV will penetrate into the mouse eye versus the human eye, the same age-related deterioration in enzyme function is seen in humans (158), suggesting that a similar response to UV-B exposure may occur in the human lens.
In summary, while prolonged UV-A exposure to mice in vivo induces a variety of cataract morphologies that differ to those observed in humans, these studies showed that elevated protein insolubility and impaired enzyme function are caused by UV-B irradiation. In addition, older lens tissue appears to have a reduced capacity for repair compared to younger lens tissue.
2.2 Rats
Rats are one of the most commonly used laboratory animals. However in comparison to human lenses, rat lenses have different protein distributions (159). Relative to body size, rats have larger lenses and thinner corneas than humans (49, 160). Rat corneas attenuate less UV-B and more UV-A radiation than the human cornea (49). Rat lenses also do not accommodate, due to poorly developed ciliary muscles (161). In addition, rat lenses transmit almost all incoming UV-A, which can damage the rat retina (162), suggesting that rats lack UV-A absorbing compounds (32). Despite these fundamental difference to human lenses, numerous studies have investigated the effects of UV light exposure on lens morphology and biochemistry.
2.2.1 Cataract phenotypes induced by UV light exposure
Acute exposure of rat lenses in vivo to both UV-A and UV-B cause a variety of cataract phenotypes (163, 164). Results from these studies have suggested that the lens epithelium exhibits an ability for regenerative repair, which is not observed in cortical fibre cells. Dose accumulation of UV-B radiation was assessed in a chronic exposure rat lens model (160). Lenses that were exposed to UV irradiation developed cataracts on the anterior surface. In addition, the anterior lens opacities intensified in all exposure period groups with the increasing cumulative dose. However, the sensitivity of the lens to UV-B radiation decreased with the number of days during which the dose was accumulated, suggesting that repeated exposure to UV-B decreases the lenses ability to recover and repair damage (160).
2.2.2 Effects of UV light on metabolism, antioxidant pathways and protein function
The localised cell swelling induced in the anterior surface of the rat lens induced by in vitro UV-A exposure appears to be due to an effect of UV-A on Na+/K+ ATPase activity, which decreased in both the lens epithelium and cortex (164). Low Na+/K+ ATPase activity may also underly cataract formation following exposure to UV-B, where lactate dehydrogenase (LDH) activity, and therefore ATP production, was lower predominantly in the anterior lens regions (epithelium and outer cortex), which was consistent with the pattern of exposure (51). While the decrease was relatively small (20%), this suggested a role for decreased ATPase activity in UV-B-induced cataract formation. However, this mechanism is yet to be confirmed in human lenses exposed to UV-B.
Changes in the metabolic profile of lenses exposed to UV-B radiation may also be anticipated if ATP production is affected as shown by Löfgren and Söderberg (51). Decreases in phenylalanine, GSH, and succinate, have been detected, potentially due to their leakage from the lens following membrane damage from UV irradiation (165). Metabolite levels can be restored following UV-B exposure, although the timeframe of metabolite decrease and restoration can vary (166). It would be interesting to apply this approach to study UV-B exposure in human lenses, and whether similar changes to metabolites and LDH are observed, whether metabolite levels can be restored, and whether this exposure would produce similar cataract phenotypes to those observed in the rat.
The effect of UV-B irradiation on lens glycolysis has been investigated in Sprague-Dawley rats (167). Lactate (produced by LDH) is an end product of anaerobic glycolysis and is often used as an indicator for activity of the glycolytic pathway (168). Lactate production was reduced initially, however, six hours after exposure, the lactate level in the exposed lenses was greater than contralateral lenses (167).
In vitro, irradiation of both intact and homogenised rat lenses has shown decreased activity of enzymes involved in the major metabolic pathways. For example, hexokinase, G6PD, aldose reductase, and Na+/K+ ATPase showed decreased activity of up to 57% compared to the control lenses, although UV-B exposure did not result in cataract formation (169). Interestingly, physiologically relevant levels of antioxidants (vitamins C and E, and β-carotene) that were added to the lens incubation medium during irradiation prevented the perturbation of enzymatic activity detected in UV-B-exposed lenses in a concentration dependent manner (169), suggesting that the damage to enzymes was through an oxidative stress mechanism. While enzyme activity changes may be involved in UV-B-induced lens opacity, the therapeutic potential of antioxidant supplementation for human lenses remains unclear since it has already been shown that both vitamin C and E have little to no effect on the prevention of human ARN cataracts when consumed as a dietary supplement (170–172).
Interestingly, albino rats are more sensitive to UV-B radiation than pigmented rats in vivo (173), possibly due to differences in corneal, aqueous humour, or iris transmission of light. The same trend is also seen with in vitro irradiation of lenses extracted from albino and pigmented rats (174). However, it is difficult to say whether the pigmented or albino rat is more suitable as a model for UV cataract.
In summary, rat models have shown that both UV-A and UV-B can impact cation homeostasis through Na+/K+ ATPase, and both ranges of wavelengths can create anterior subcapsular cataracts in the rat lens. The rat lens has been shown to be most susceptible to UV-B at 300 nm (175), with most of these models employing this wavelength. UV-B increases light scattering, and also decreases water soluble metabolites, enzyme activities, and cellular respiration.
3 Crepuscular animal models of human age-related cataract
Crepuscular animals are active during twilight hours of dawn and dusk. The UV index at twilight is approximately 200 µJ cm-2 (176), and therefore considerably less than the average 2 J cm-2 experienced during the day. However, the dose of UV that these animals would be exposed to in their natural environment is more than nocturnal animals and more similar to humans. Thus, crepuscular animals have been used as animal models of human age-related cataract. Crepuscular animals can be used in vivo as they are relatively small and easy to keep, and ex vivo tissue is readily available.
3.1 Guinea pigs
Guinea pigs have previously been used for models of ARN cataracts (177–180), and galactose-induced cataract (181, 182). Due to high levels of a UV-A chromophore in the lens (122) that mimics that of the human UV filter, a similar pattern of lens GSH distribution with lower levels of GSH in the nucleus relative to the cortex (183), and a similar age-dependent pattern of nuclear disulfide formation (184), it is proposed that guinea pigs are the best non-primate model currently available for the study of UV-A exposure and cataract (32).
However, while there are many benefits to the use of guinea pigs as a model of human UV exposure, it is also important to consider inconsistencies between humans and guinea pigs. For example, there is conflicting evidence for the formation of brunescence in the guinea pig lens (185, 186), unlike the characteristic time dependent increase in colouration observed in the human lens (187).
3.1.1 Cataract phenotypes induced by UV light
Chronic exposure of guinea pigs to a low level of UV-A light in vivo produces protein aggregation and cataract in the centre of the lens (188). In contrast, the anterior lens cortex showed no difference between UV-A exposed and control guinea pigs, perhaps due to the higher metabolic activity, and therefore antioxidant capacity of the lens cortex. The mechanism of nuclear cataract formation in the guinea pig nucleus may be due to the binding of the UV-A chromophore NADPH to zeta crystallin. This is proposed to mimic the binding of kynurenine to crystallin proteins in the human lens (122). NADPH is known to cause the formation of superoxide ions (189), and H2O2 (190), when it absorbs UV-A (see Figure 2). Interestingly, the guinea pig is over 10-fold more tolerant to UV-B than pigmented rabbits, rats, and mouse (191). Very high doses of UV-B are required to produce cortical cataracts in the anterior subcapsular region of the lens in vivo. The exact mechanism for this enhanced protection is unclear, although perhaps it is because the guinea pig lens contains more ascorbate than rat lenses, with levels similar to the human lens (191), as well as high levels of free NADPH (44, 192) and zeta-crystallins (193).
3.1.2 Effects of UV light on metabolism, antioxidant pathways and protein function
Interestingly, UV-A light produced deleterious effects on the nucleus of guinea pig lenses, when compared to age-matched controls (44). There was an increase in light scattering, distention of intracellular spaces, a decrease in GSH, increased lipid peroxidation, and a loss of water-soluble proteins in the lens nucleus. Results from UV-A exposure of guinea pig lenses in vivo strongly support the role of oxidative stress in cataract formation following UV exposure. For example, lenses show up to a 50% reduction in free sulfhydryl, with a concomitant 100% increase in disulphide formation (185). This is possibly due to the formation of protein mixed disulphides (44), or reduction in activity of glutathione reductase, such as that suggested from studies of squirrel lenses (194). The guinea pig has also been used to test if ascorbate delivered by the diet (195–197) can protect against photooxidative damage to the lens induced by UV exposure. While this work showed promise, more recent investigations showed that ascorbate does not prevent human ARN cataract formation when consumed as a dietary supplement (170, 171).
In summary, in vivo guinea pig models show that UV-A can penetrate deep into the lens nucleus and cause dense nuclear opacification, as well as brunescence of lens tissue. In addition, ROS may be generated, which may contribute to changes to the lens after exposure that are characteristic of oxidative stress. Nuclear opacification, protein aggregation, loss of free GSH, and increased levels of disulphides within UV-A exposed lenses show how potentially damaging UV-A radiation can be in vivo. Guinea pigs appear to be quite tolerant to UV-B radiation, with very high doses required to produce cataracts.
3.2 Rabbits
Rabbits have previously been used as ex vivo models for ARN cataract formation (198) and oxygen-induced protein changes (199, 200). Cultured rabbit lens epithelial cells have also been used to investigate the effects of UV-A and/or UV-B (201–206), as well as the efficacy of UV blocking contact lenses (207, 208). While rabbit lenses lack kynurenine-based UV filters (32), they contain high levels of NADH and NADPH (207) which absorb UV-A light and may therefore act as a human UV filter analogue. Rabbit lenses are also more similar in size and sphericity to human lenses than other commonly used rodent lenses and have been used in both ex vivo and in vivo studies.
3.2.1 Cataract phenotypes induced by UV light
The rabbit cornea absorbs radiation completely at wavelengths at, and below 290 nm (209), and therefore the lens is more susceptible to damage at wavelengths greater than 290 nm. UV-A irradiation of rabbit lenses (210) produced opacification, potentially due to the tight bundling of actin filaments, or other morphological effects indicating cell cytotoxicity, including the breakdown of plasma membranes. Relatively low exposures of UV-B can produce anterior subcapsular lenticular opacities in vivo, although these opacities are not permanent and resolve within three months (209).
After in vivo irradiation of rabbit lenses, the lenses exhibit a pale yellow colour, although the reason for this colouration is unclear (207). Given that human lenses show colouration with aging, this is an interesting finding and suggests the potential of the rabbit lens for investigating the effects of UV light on lens colouration, despite differences in UV absorbing compounds.
3.2.2 Effects of UV light on metabolism, antioxidant pathways and protein function
Changes to the metabolic profile of in vivo albino rabbit lenses with either a single dose, or repeated exposures adding up to a single dose, of UV-B has been investigated (211). Interestingly, repeated exposure to a small dose had more of an impact on the lenticular metabolic profile than a single dose (equal to cumulative repeated dose) did, demonstrating the cumulative effect of repeated UV-B irradiations. No lenticular opacification was reported, therefore, combined with the results of Pitts (209), it is unclear whether UV-B irradiation produces permanent cataracts in rabbit lenses in vivo. In contrast, exposure of ex vivo albino rabbit lenses to UV-B does produce lens opacification. This appears to confirm that impairment of Na+/K+ ATPase function is a common mechanism for UV-induced lens cataract (212), while highlighting that in vivo and ex vivo exposure systems can produce conflicting results.
Cultured lenses from rabbits four to five weeks old are not sensitive to UV-A irradiation alone (213), which contradicts previous findings (210), although the age of the rabbit may influence the extent of UV-A sensitivity. However, a combination of UV-A and UV-B has been shown to be more damaging to the cultured rabbit lens than UV-A alone (213). In vivo exposure to combinations of UV-A and UV-B using a variety of protocols have not consistently produced changes to rabbit lens metabolites (211, 214), proteins or malondialdehyde as a marker of oxidative stress (215). Taken together it appears that the rabbit lens in vivo is relatively well protected from UV-induced damage.
4 Diurnal animal models of human age-related cataract
Diurnal animals are active during the day, and sleep at night and therefore more closely resemble the activity of the average human than nocturnal and crepuscular animals. Moreover, UV filters have been identified in diurnal animal lenses. Like humans, primate lenses have been found to contain 3OHKG (216–218). 3OHKG is the main absorbing species in young primate lenses, and both UV-A and UV-B have been shown to penetrate, and be absorbed by, the young lens nucleus (219). Primate lenses have similar optical and biometric properties to human lenses (220), but tissue can be difficult to obtain. Primate lenses have been used to investigate changes in UV absorption and transmission with age (219, 221), and effects of UV radiation on the cornea (222). However, to our knowledge, primate lenses have not been used experimentally to assess the impact of UV radiation on the lens, and thus will not be discussed further. Grey squirrels (223, 224), thirteen-lined ground squirrels (225, 226), cows (227), fish (228–230), and other mammalian lenses (231), have been shown to contain tryptophan metabolites or UV sensitive pigments. While smaller diurnal animals (e.g. squirrels) can be studied in vivo, most diurnal animal models generally use ex vivo tissue.
4.1 Cows
Bovine lenses are thought to express limited UV filter compounds, such as 3OHK (227), but share a similar predominance of α-crystallin (232, 233). Bovine lenses also do not undergo significant accommodation (234). There are UV models that utilise isolated bovine proteins (235–238), and epithelial cell cultures (239), however they will not be discussed.
4.1.1 Effect of UV light on cataract phenotype
Daily exposure of ex vivo bovine lenses to UV-A results in no significant changes to transmittance or focal length, when compared to controls (240). Mild subcapsular opacity is also observed. When ex vivo bovine lenses were exposed to UV-B, the results show that weekly doses of UV-B prevented lens repair, but these changes should not be considered to be cumulative, since the damage did not worsen with subsequent doses (240).
The effect of varying low-level UV-B exposure on light scattering and lens focal length ex vivo has also been investigated (241). Although not statistically significant, the second lowest dose (0.06 J cm-2) appeared to have the greatest impact on the measurement parameters. Slight anterior subcapsular opacities became apparent as soon as two hours after irradiation, but in most cases, the damage cleared and only a very small area of damage remained on the anterior surface of the lens. Most of the exposed lenses showed measurable increases in light scatter and focal length but were able to recover. Thus, low doses of UV-B radiation do not permanently damage ex vivo bovine lenses, similar to findings in rabbit lenses (209).
4.1.2 Effects of UV light on metabolism, antioxidant pathways and protein function
The effect of UV-A irradiation and subsequent recovery on the biochemical and optical properties of ex vivo bovine eyes has been investigated (242). The activity of hexokinase, catalase, and G6PD enzymes is perturbed by UV-A in a dose-dependent manner. Hexokinase appears to be the most sensitive to UV-A exposure, similar to the observation for rat lens hexokinase in response to UV-B radiation (243). In addition, the activity of Na+/K+ ATPase in lens epithelial cells is impaired in response to UV-A exposure (244). However, repair mechanisms exist within the bovine lens that remain intact ex vivo, which were able to repair damage done to optical quality and Na+/K+ ATPase activity in the central region of the lens epithelium.
The effect of UV-A irradiation on the chaperone-like properties of α-crystallin has been investigated in bovine lenses, showing differences between the response of α-crystallin from young, and old lenses (245). In comparison to young lenses, α-crystallin from old lenses had a decreased ability to inhibit protein denaturation in vitro. There was an increase in the molecular weight of α-crystallin fractions, and a loss of tryptophan fluorescence which barely recovered following irradiation. This suggested that older lens proteins are more susceptible to damage from irradiation, which has also been observed in mice exposed to UV-B (155). This is perhaps due to a cumulative effect of UV-A radiation, and potentially similar to the UV-B effects observed in rats (160), and rabbits (211). When the lenses began to recover (indicated by focal length repair), chaperone-like activity recovered and tryptophan fluorescence increased predominantly in young lenses, suggesting that conformational changes to α-crystallin which had occurred during irradiation had resolved.
In summary, UV-A irradiation can cause anterior subcapsular opacities in the bovine lens and older lens proteins appear to be more susceptible to UV-A damage. Permanent damage to lenticular enzyme activities can occur with sufficiently high doses of UV-A and may be implicated in UV-A induced cataract. UV-B can induce small subcapsular opacities in the bovine lens, although low doses do not appear to permanently damage the lens. However, lens repair may be prevented with repeated UV-B exposure.
4.2 Pigs
In comparison to humans, pigs have a similar lens protein concentration (32, 246), lens shape (247, 248), light transmission (249), and protein content (250). While pig lenses are thought not to accommodate (251), and differences in the UV filter composition of pigs and humans exist (252), some inferences on human lens response to UV radiation may be made from porcine studies. While the impact of UV-A light on protein isolates (253, 254), and lens tissue sections (255) have been investigated, we will only focus on changes induced in ex vivo organ cultured lenses.
4.2.1 Cataract phenotypes induced by UV light
The effectiveness of different wavelengths of light has been assessed using ex vivo porcine lenses (256). Mid-range UV-B (295 nm) was 25 times more effective than tail-end UV-B (315 nm) radiation at producing anterior subcapsular lesions. To assess for the ability of porcine lenses to recover from exposure to UV, lenses were exposed to five times UV-B threshold exposure, resulting in the appearance of the lens sutures, suggesting the radiation had inflicted permanent damage to the lens. At two times threshold for UV-A, there was no full recovery of the lens, confirming that UV-A is cataractogenic in the porcine lens. This study concluded that the most damaging wavelengths are 270 to 315 nm, due to the low dosages required to produce visible damage. Without the protection provided by the cornea in vivo, the UV-B radiation was able to have a substantial impact on the lens tissue. Wavelengths shorter than 285 nm would be expected to be more damaging, since shorter wavelength photons are also higher energy (257), but this was not the case.
In addition, the same group utilised ex vivo porcine lenses to investigate the effect of a combination exposure of both UV-A and UV-B radiation (252). This model demonstrated the synergistic effects of low, subsolar UV-A and UV-B, with significant inhibition of cellular metabolic activity and no indication of recovery. Some recovery of plasma membrane damage was observed; however, optical quality did not recover in the study period. UV-A radiation alone required high doses (λ = 365 nm, 86 J/cm2) to produce significant decreases in cellular and optical integrity, in accordance with the previous study (256).
In summary, there are numerous similarities between porcine and human lenses, making them a more popular animal model of choice in recent UV exposure investigations. High doses of UV-A are required to produce anterior subcapsular opacities in porcine lenses, compared to the lower doses of UV-B required to produce the same phenotype. However, a combination of UV-A and UV-B incurs significant damage to the cellular metabolic activity and optical quality of the lens. Further experiments using porcine lenses could monitor changes to the cellular systems that are known to be involved in UV-induced damage which have been established in other models, and to investigate whether porcine lenses would become brunescent with age and/or UV exposure.
4.3 Squirrels
Squirrel lenses share several features in common with humans, suggesting they may be a good model animal for understanding the effects of UV exposure on lens transparency. For example, they contain UV filters (226) which have a similar structure and concentration to those found in humans (225), and display brunescence (224, 225). Squirrels have similar levels of GSH in the nucleus as a young human lens (225), but the total GSH is approximately twice that of humans (258). While cultured squirrel lens epithelial cells have been used to study the use of vitamin E as a protective agent against UV-induced damage (202, 205), only in vivo and ex vivo experiments that utilised whole squirrel lenses will be discussed here.
4.3.1 Cataract phenotypes induced by UV light
The effects of ambient exposure to UV-A have been investigated in grey squirrels in vivo (194). Following chronic UV-A (λ = 365nm), well defined lens opacities (cortical and subcapsular) were observed, and histological analysis showed swelling of the superficial cortical fibre cells and some degenerating fibres post UV-A exposure. Anterior opacities that increase in severity with exposure time have also been observed using ex vivo squirrel lenses (259). The type of cataract formed from exposure to UV-B is not documented, but UV-B exposed lenses have been used for biochemical analyses (260).
4.3.2 Effects of UV light on metabolism, antioxidant pathways and protein function
UV-A exposure was associated with an increase in crosslinking and degradation of crystallin proteins, and small changes in the levels of soluble crystallin proteins (194). A major loss of GSH in the outer and inner cortex was detected, while levels in the nucleus remained the same, which is opposite to the pattern seen in the aging human lens (183). Although apparent in the lens cortex, this study showed that chronic exposure of UV-A light can induce cataract formation.
Furthermore, UV-A exposure causes significant damage to phosphorous metabolites, such as ATP, in the ex vivo squirrel lens (259). Changes to ATP levels appear to scale with dose, whereby a lower dose causes a smaller decrease in ATP. Crystallin proteins have been shown to undergo crosslinking when exposed to UV-A in the squirrel lens (261). In vivo, increases in proteins with greater molecular weights occurred in the outer layers of the lens, but not the nucleus (261). This is similar to the pattern of altered lens protein distribution in cataractous human lenses (262). Crosslinking of soluble crystallins was seen in both in vivo and ex vivo exposed lenses. A link was also made between squirrel lens pigment and protein crosslinking, indicating that lens pigment stimulates the photosensitised crosslinking of lens proteins in vitro (261) which may provide some insight into the protein changes that occur during human cataract formation.
Indoleamine 2,3-dioxygenase (IDO) is an antioxidant enzyme, and the first-rate limiting enzyme of tryptophan catabolism. Exposure of squirrel lenses to UV-B led to an increase in IDO activity within the lens, and thus an increase in tryptophan metabolites (i.e. kyn and 3OHK) (260). Irradiation also led to increased lipid peroxidation and a decrease in GSH, suggesting UV-B had caused oxidative stress within the lens. Long durations of UV-B exposure had a small but suppressive effect on the activity of superoxide dismutase (SOD), an antioxidant protein that reduces intracellular levels of superoxide radicals. Human lenses rely on IDO for the formation of UV filters (105, 263, 264), and these findings in squirrel lenses may have parallels in human lenses.
In summary, squirrel models have been used to show the damaging effect UV-A has on proteins, initiating crosslinking and degradation. Although it is unclear if UV-B induces opacification of squirrel lenses, it does cause oxidative stress, shown through increasing lipid peroxidation and a reduction in antioxidant.
5 Conclusions
The paucity and significant biochemical variability of human lens tissue necessitates the use of animal tissue to model and characterise the effect that UV light has on tissue transparency and its role in cataract formation. While conclusions drawn from animal studies cannot always be directly translated to human cataract due to the morphological and biochemical differences between species, animal models have revealed several changes that take place in lenses exposed to different wavelengths of UV light with both UV-A and UV-B light appearing to play a role in cataract formation, albeit by different mechanisms. It remains clear that the same wavelength and dose of UV-A or UV-B can produce an array of different biochemical and metabolic changes, as well as cataract phenotypes, and is dependent on the animal model used. Similarly, different cataract phenotypes can result from the same underlying mechanism. While mice and rats are convenient laboratory animal models, the fact that they are nocturnal animals that normally experience completely different UV exposure levels to humans means that the role of UV light exposure in cataract development in rodents must be carefully interpreted with respect to cataract formation in the human lens. Nevertheless, these models have helped to identify oxidative stress via photooxidation, and photosensitisation as major factors involved in UV cataract development. Specifically, the Na/K ATPase activity is impaired in several UV exposure models, which is likely to impair the specialised transport system known to maintain lens tissue transparency (265).
Although crepuscular animals experience higher levels of UV radiation than nocturnal animals, they still do not experience the same levels of exposure as humans. In addition, both classes of animal lack the same range of UV filters found in the lens. However, in both types of animals the application of “non-environmental” UV exposures to these laboratory animals does provide information on UV-A and UV-B as cataractous stressors that can differentially activate oxidative defence pathways in different regions of the lens that normally act to maintain lens structure and function. Guinea pigs, however, appear to recapitulate many of the characteristics of UV cataract in humans, including lens brunescence.
Diurnal animals share more similarities with humans than nocturnal or crepuscular animals with respect to UV exposure levels, the presence of UV filters, and the characteristic brunescence of lens tissue at least in the case of the squirrel lens (225). This suggests that mechanisms of UV damage observed in squirrel lenses could be directly applicable to UV cataract formation in humans. However, not all laboratories have access to these animals.
In contrast, porcine and bovine lenses are more readily available and easily utilised in organ culture experiments. The use of these diurnal ex vivo animal models has shown that UV-A radiation has the potential to be more harmful than UV-B radiation, possibly due to the absorption of this longer wavelength energy in deeper lens cell layers that inactivates enzymatic activity involved in the protection against oxidative stress. They have also shown that in younger lenses at low doses this UV induced damage can be repaired; but that older lenses are more susceptible to UV damage and showed impaired recovery compared to young lenses receiving the same dose. The lack of evidence surrounding yellowing of the lens tissue with age (or UV exposure) in these larger animals, however, means that while they are useful for understanding potential changes to proteins resulting from photooxidation and photosensitisation, they lack a key characteristic of human UV cataract.
Despite this substantial body of work, gaps in our understanding of the extent of the impact that exposure to UV light has on the lens remain. To close this gap continued development of UV light exposure models that utilise diurnal animals, especially guinea pig and squirrel lenses, will further enhance our understanding of the role that UV light exposure plays in the development of human lens opacities. In addition, models that combine stressors, for example oxidative and photooxidative stress, may prove useful to further investigate human cataract development.
Author contributions
EM: Writing – original draft, Writing – review & editing. PD: Writing – review & editing. AG: Writing – review & editing.
Funding
The author(s) declare financial support was received for the research, authorship, and/or publication of this article. The authors acknowledge support from the Health Research Council of New Zealand.
Conflict of interest
The authors declare that the research was conducted in the absence of any commercial or financial relationships that could be construed as a potential conflict of interest.
Publisher’s note
All claims expressed in this article are solely those of the authors and do not necessarily represent those of their affiliated organizations, or those of the publisher, the editors and the reviewers. Any product that may be evaluated in this article, or claim that may be made by its manufacturer, is not guaranteed or endorsed by the publisher.
Supplementary material
The Supplementary Material for this article can be found online at: https://www.frontiersin.org/articles/10.3389/fopht.2024.1414483/full#supplementary-material
References
1. Pascolini D, Mariotti S. Global estimates of visual impairment: 2010. Br J Ophthalmol. (2012) 96:614–8. doi: 10.1136/bjophthalmol-2011-300539
2. Bourne RRA, Stevens GA, White RA, Smith JL, Flaxman SR, Price H, et al. Causes of vision loss worldwide, 1990-2010: a systematic analysis. Lancet. (2013) 1:339–49. doi: 10.1016/S2214-109X(13)70113-X
3. Liu Y-C, Wilkins M, Kim T, Malyugin B, Mehta JS. Cataracts. Lancet. (2017) 390:600–12. doi: 10.1016/S0140-6736(17)30544-5
5. Hollows F, Moran D. Cataract - the ultraviolet risk factor. Lancet. (1981) 2:1249–50. doi: 10.1016/S0140-6736(81)91490-2
6. Taylor HR. Ultraviolet radiation and the eye: an epidemiologic study. Trans Am Ophthalmol Soc. (1989) 87:802–53.
7. Wittenberg S. Solar radiation and the eye: a review of knowledge relevant to eye care. Am J Opto Physiol Opt. (1985) 63:676–89. doi: 10.1097/00006324-198608000-00012
8. Javadi M-A, Zarei-Ghanavati S. Cataracts in diabetic patients: A review article. J Ophthal Vision Res. (2008) 3:62–5.
9. Klein BEK, Klein R, Moss SE. Incidence of cataract surgery in the Wisconsin epidemiologic study of diabetic retinopathy. Am J Ophthalmol. (1995) 119:295–300. doi: 10.1016/S0002-9394(14)71170-5
10. Klein BEK, Klein R, Moss SE. Prevalence of cataracts in a population-based study of persons with diabetes mellitus. Ophthalmology. (1985) 92:1191–6. doi: 10.1016/S0161-6420(85)33877-0
11. Chan AWH, Ho Y-S, Chung SK, Chung SSM. Synergistic effect of osmotic and oxidative stress in slow-developing cataract formation. Exp Eye Res. (2008) 87:454–61. doi: 10.1016/j.exer.2008.08.001
12. Lou MF. Redox regulation in the lens. Prog Retinal Eye Res. (2003) 22:657–82. doi: 10.1016/S1350-9462(03)00050-8
13. Lim JC, Vaghefi E, Li B, Nye-Wood MG, Donaldson PJ. Characterization of the effects of hyperbaric oxygen on the biochemical and optical properties of the bovine lens. Invest Opthalmol Visual Sci. (2016) 57:1961–73. doi: 10.1167/iovs.16-19142
14. Lou MF. Glutathione and glutaredoxin in redox regulation and cell signalling of the lens. Antioxidants. (2022) 11:1973–2003. doi: 10.3390/antiox11101973
15. Vasavada AR, Mamidipudi PR, Sharma PS. Morphology of and visual performance with posterior subcapsular cataract. J Cataract Refractive Surge. (2004) 30:2097–104. doi: 10.1016/j.jcrs.2004.02.076
16. Shun-Shin GA, Brown NAP, Bron AJ, Sparrow JM. Dynamic nature of posterior subcapsular cataract. Br J Ophthalmol. (1989) 73:522–7. doi: 10.1136/bjo.73.7.522
17. Delcourt C, Cristol J-P, Tessier F, Leger CL, Michel F, Papoz L, et al. Risk factors for cortical, nuclear, and posterior subcapsular cataracts. POLA Study Am J Epidemiol. (2000) 151:497–504. doi: 10.1093/oxfordjournals.aje.a010235
18. OECD. Health Care Utilisation: Surgical procedures : Stat technology; OECD Stat (2023). Available online at: https://stats.oecd.org/index.aspx?queryid=30167. (AccessedJune 19, 2023).
19. Brown GC, Brown MM, Menezes A, Busbee BG, Lieske HB, Lieske PA. Cataract surgery cost utility revisited in 2012. Am Acad Ophthalmol. (2013) 120:2367–76. doi: 10.1016/j.ophtha.2013.04.030
20. Heemraz BS, Lee CN, Hysi PG, Jones CA, Hammond CJ, Mahroo OA. Changes in quality of life shortly after routine cataract surgery. Can J Ophthalmol. (2016) 51:282–7. doi: 10.1016/j.jcjo.2016.02.004
21. Wang W, Yan W, Fotis K, Prasad NM, Lansingh VCL, Taylor HR, et al. Cataract surgical rate and socioeconomics: A global study. Invest Ophthalmol Visual Sci. (2017) 57:5872–81. doi: 10.1167/iovs.16-19894
22. Schein OD, Cassard SD, Tielsch JM, Gower EW. Cataract surgery among medicare beneficiaries. Ophthal Epidemiol. (2012) 19:257–64. doi: 10.3109/09286586.2012.698692
23. Kessy JP, Lewallen S. Poverty as a barrier to accessing cataract surgery: a study from Tanzania. Br J Ophthalmol. (2007) 91:1114–6. doi: 10.1136/bjo.2006.112474
24. Nirmalan PK. Utilisation of eye care services in rural south India: the Aravind Comprehensive Eye Survey. Br J Ophthalmol. (2004) 88:1237–41. doi: 10.1136/bjo.2004.042606
25. Semmens JB, Li J, Morlet N, Ng J. Trends in cataract surgery and postoperative endophthalmitis in Western Australia (1980–1998): the Endophthalmitis Population Study of Western Australia. Clin Exp Ophthalmol. (2003) 31:312–219. doi: 10.1046/j.1442-9071.2003.00647.x
26. Zigler JS. Animal models for the study of maturity-onset and hereditary cataract. Exp Eye Res. (1990) 50:651–7. doi: 10.1016/0014-4835(90)90109-8
27. Lim JC, Umapathy A, Donaldson PJ. Tools to fight the cataract epidemic: A review of experimental animal models that mimic age related nuclear cataract. Exp Eye Res. (2016) 145:432–43. doi: 10.1016/j.exer.2015.09.007
28. Duncan G, Wormstone IM, Davies PD. The aging human lens: structure, growth, and physiological behaviour. Br J Ophthalmol. (1997) 81:818–23. doi: 10.1136/bjo.81.10.818
29. Apple DJ, Solomon KD, Tetz MR, Assia EI, Holland EY, Legler UFC, et al. Posterior capsule opacification. Survey Ophthalmol. (1992) 37:73–116. doi: 10.1016/0039-6257(92)90073-3
30. Bron AJ, Vrensen GFJM, Koretz J, Maraini G, Harding JJ. The ageing lens. Ophthalmologica. (2000) 214:86–104. doi: 10.1159/000027475
31. Michael R, Bron AJ. The ageing lens and cataract: a model of normal and pathological ageing. Philos Trans R Soc B. (2011) 366:1728–292. doi: 10.1098/rstb.2010.0300
32. Truscott RJ. Age-related nuclear cataract-oxidation is the key. Exp Eye Res. (2005) 80:709–25. doi: 10.1016/j.exer.2004.12.007
33. Lim JC, Vorontsova I, Martis RM, Donaldson PJ. Animal Models in Cataract Research. In: Animal Models for the Study of Human Disease (2017) United Kingdom: Elsevier, Academic Press. p. 103–16.
34. Organization WH. World report on vision. Switzerland: World Health Organisation (2019), ISBN: ISBN 978-92-4-151657-0. Contract No.: Licence: CC BY-NC-SA 3.0 IGO.
35. Frederick JE, Snell HE, Haywood EK. Solar ultraviolet radiation at the Earth's surface. Photochem Photobiol. (1989) 50:443–50. doi: 10.1111/j.1751-1097.1989.tb05548.x
36. Nunez M, Forgan B, Roy C. Estimating ultraviolet radiation at the Earth's surface. Int J Biometerol. (1994) 38:5–17. doi: 10.1007/BF01241798
37. Ivanov IV, Mappes T, Schaupp P, Lappe C, Wahl S. Ultraviolet radiation oxidative stress affects eye health. J Biophoton. (2018) 11:e201700377. doi: 10.1002/jbio.201700377
38. Mallet JD, Rochette PJ. Wavelength-dependent ultraviolet induction of cyclobutane pyrimidine dimers in the human cornea. Photochem Photobiol Sci. (2013) 12:1310. doi: 10.1039/c3pp25408a
39. Boettner EA, Reimer Wolter J. Transmission of the ocular media. Invest Ophthalmol Visual Sci. (1962) 1:776–83.
40. Organization WH. The Effects of Solar UV Radiation on the Eye. Switzerland: World Health Organisation (1993). Report No.: Who/PBL/EHG/94.1.
41. Kamari F, Hallaj S, Dorosti F, Alinezhad F, Taleschian-Tabrizi N, Farhadi F, et al. Phototoxicity of environmental radiations in human lens: revisiting the pathogenesis of UV-induced cataract. Graefe's Arch Clin Exp Ophthalmol. (2019) 257:2065–77. doi: 10.1007/s00417-019-04390-3
42. Saßmannshausen M, Ach T. Influence of ultraviolet radiation exposure to the retina. Ophthalmol. (2022) 119:240–7. doi: 10.1007/s00347-021-01506-1
43. Murray CJL, Lopez AD, Organisation WH, Bank W, Health HSoP. The Global burden of disease: a comprehensive assessment of mortality and disability from diseases, injuries, and risk factors in 1990 and projected to 2020. Boston, MA, USA: Harvard School of Public Health (1996).
44. Giblin FJ, Leverenz VR, Padgaonkar VA, Unakar NJ, Dang L, Lin L-R, et al. UVA light in vivo reaches the nucleus of the Guinea pig lens and produces deleterious, oxidative effects. Exp Eye Res. (2002) 75:445–58. doi: 10.1006/exer.2002.2039
45. Beebe DC, Holekamp NM, Shui Y-B. Oxidative damage and the prevention of age-related cataracts. Ophthal Res. (2010) 44:155–65. doi: 10.1159/000316481
46. McCarty CA, Taylor HR. A review of the epidemiologic evidence linking ultraviolet radiation and cataracts. Develop Ophthalmol. (2002) 35:21–31. doi: 10.1159/000060807
47. Dolin PJ. Ultraviolet radiation and cataract: a review of the epidemiological evidence. Br J Ophthalmol. (1994) 78:478–82. doi: 10.1136/bjo.78.6.478
48. Taylor HR, West SK, Rosenthal FS, Muñoz B, Newland HS, Abbey H, et al. Effect of ultraviolet radiation on cataract formation. New Engl J Med. (1988) 319:1429–33. doi: 10.1056/NEJM198812013192201
49. Dillon JP, Zheng L, Merriam JC, Gaillard ER. The optical properties of the anterior segment of the eye: implications for cortical cataract. Exp Eye Res. (1999) 68:785–95. doi: 10.1006/exer.1999.0687
50. Maher EF. Transmission and absorption coefficients for ocular media of the Rhesus Monkey. Brooks Air Force Base, San Antonio, Texas: USAF School of Aerospace Medicine (1978). Contract No.: Report SAM-TR-78-32.
51. Löfgren S, Söderberg PG. Lens lactate dehydrogenase inactivation after UV-B irradiation: an in vivo measure of UVR-B penetration. Invest Ophthalmol Visual Sci. (2001) 42:1833–6.
52. Neale RE, Purdie JL, Hirst LW, Green AC. Sun exposure as a risk factor for nuclear cataract. Int J Biochem Cell Biol. (2003) 35:1500–4. doi: 10.1097/01.ede.0000086881.84657.98
53. Zigman S, Datiles M, Torczynski E. Sunlight and human cataract. Assoc Res Vision Ophthalmol. (1979) 18:462–7.
54. Sasaki K, Sasaki H, Kojima M, Shui YB, Hockwin O, Jonasson F, et al. Epidemiological studies on UV-related cataract in climatically different countries. J Epidemiol. (1999) 9:33–8. doi: 10.2188/jea.9.6sup_33
55. Rosmini F, Stazi MA, Milton RC, Sperduto RD, Pasquini P, Maraini G, et al. A dose response effect between a sunlight index and age related cataracts annals of epidemiology. Annals of Epidemiology. (1994) 4:266–70. doi: 10.1016/1047-2797(94)90081-7
56. Hiller R, Giacometti L, Yuen K. Sunlight and cataract: an epidemiologic investigation. Am J Epidemiol. (1977) 105:450–9. doi: 10.1093/oxfordjournals.aje.a112404
57. Hiller R, Sperduto RD, Ederer F. EPIDEMIOLOGIC ASSOCIATIONS WITH CATARACT IN THE 1971–1972 NATIONAL HEALTH AND NUTRITION EXAMINATION SURVEY1. Am J Epidemiol. (1983) 118:239–49. doi: 10.1093/oxfordjournals.aje.a113631
58. Said M-E, Goldstein H, Korra A, El-Kashlan K. Prevalence and causes of blindness in urban and rural areas of Egypt. Public Health Rep. (1970) 85:587–99. doi: 10.2307/4593913
59. Schein OD, West SK, Munoz B, Vitale S, Maguire M, Taylor HR, et al. Cortical lenticular opacification: distribution and location in a longitudinal study. Invest Ophthalmol Visual Sci. (1994) 35:263–366.
60. Brilliant LB, Grasset NC, Pokhrel RP, Kolstad A, Lepkowski JM, Brilliant G,E, et al. Associations among cataract prevalence, sunlight hours, and altitude in the Himalayas. Am J Epidemiol. (1983) 118:250–64. doi: 10.1093/oxfordjournals.aje.a113632
61. Hu T-s, Lao Y-x. An epidemiologic survey of senile cataract in China. In: Sasaki K, Hockwin O, Leske MC, editors. Cataract Epidemiology: International Meeting. Noto/Japan: S. Karger AG (1987).
62. Hu T-S. Age-related cataract in the Tibet eye study. Arch Ophthalmol. (1989) 107:666. doi: 10.1001/archopht.1989.01070010684027
63. Miyashita H, Hatsusaka N, Shibuya E, Mita N, Yamazaki M, Shibata T, et al. Association between ultraviolet radiation exposure dose and cataract in Han people living in China and Taiwan: A cross-sectional study. PloS One. (2019) 14:e0215338. doi: 10.1371/journal.pone.0215338
64. Hatsusaka N, Yamamoto N, Miyashita H, Shibuya E, Mita N, Yamazaki M, et al. Association among pterygium, cataracts, and cumulative ocular ultraviolet exposure: A cross-sectional study in Han people in China and Taiwan. PloS One. (2021) 16:1–15. doi: 10.1371/journal.pone.0253093
65. Garzon-Chavez DR, Quentin E, Harrison SL, Parisi AV, Butler HJ, Downs NJ. The geospatial relationship of pterygium and senile cataract with ambient solar ultraviolet in tropical Ecuador. Photochem Photobiol Sci. (2018) 17:1075–83. doi: 10.1039/c8pp00023a
67. Cruickshanks KJ, Klein BE, Klein R. Ultraviolet light exposure and lens opacities: the Beaver Dam Eye Study. Am J Public Health. (1992) 82:1658–62. doi: 10.2105/AJPH.82.12.1658
68. Prokofyeva E, Wegener A, Zrenner E. Cataract prevalence and prevention in Europe: a literature review. Acta Ophthalmol. (2013) 91:395–405. doi: 10.1111/j.1755-3768.2012.02444.x
69. Sasaki H, Jonasson F, Kojima M, Katoh N, Ono M, Takahashi N, et al. The Reykjavik eye study – prevalence of lens opacification with reference to identical Japanese studies. Ophthalmologica. (2000) 214:412–20. doi: 10.1159/000027535
70. West SK, Duncan DD, Muñoz B, Rubin GS, Fried LP, Bandeen-Roche K, et al. Sunlight exposure and risk of lens opacities in a population-based study. JAMA. (1998) 280:714. doi: 10.1001/jama.280.8.714
71. Javitt JC, Taylor HR. Cataract and latitude. Doc Ophthalmol. (1995) 88:307–25. doi: 10.1007/BF01203684
72. Collman GW, Shore DL, Shy CM, Checkoway H, Luria AS. Sunlight and other risk factors for cataracts: an epidemiologic study. Am J Public Health. (1988) 78:1459–62. doi: 10.2105/AJPH.78.11.1459
73. Tang Y, Ji Y, Ye X, Wang X, Cai L, Xu J. The association of outdoor activity and age-related cataract in a rural population of Taizhou eye study: phase 1 report. Public Library Sci. (2015) 10:1–13. doi: 10.1371/journal.pone.0135870
74. Li X, Cao X, Yu Y, Bao Y. Correlation of sunlight exposure and different morphological types of age-related cataract. BioMed Res Int. (2021) 2021:10. doi: 10.1155/2021/8748463
75. Goldsmith RI, Rothhammer F, Schull WJ. The multinational Andean genetic and health program: III. Ophthalmic disease and disability among the Aymara. Bull Pan Am Health Organ. (1979) 13:58–65. doi: 10665.2/27562
76. Sliney DH. Physical factors in cataractogenesis: ambient ultraviolet radiation and temperature. Invest Ophthalmol Visual Sci. (1986) 27:781–90.
77. Sasaki K, Sasaki H, Jonasson F, Kojima M, Cheng HM. Racial differences of lens transparency properties with aging and prevalence of age-related cataract applying a WHO classification system. Ophthal Res. (2004) 36:332–40. doi: 10.1159/000081636
78. Sasaki K, Ono M, Aoki K, Katou N, Morine M, Nakaizumi H, et al. Cataract epidemiology survey in the three climatically different areas in Japan–prevalence of cataracts and types of lens opacification. Nippon Ganka Gakksi Zasshi. (1995) 99:204–11.
79. Mohan M, Sperduto RD, Angra SK, Milton RC, Mathur RL, Underwood BA, et al. India-US case-control study of age-related cataracts. Arch Ophthalmol. (1989) 107:670–6. doi: 10.1001/archopht.1989.01070010688028
80. Halpern P, Dave JV, Braslau N. Sea-level solar radiation in the biologically active spectrum. Science. (1974) 186:1204–8. doi: 10.1126/science.186.4170.1204
81. Manabe S, Wada O, Urban RC. A fluorescent carcinogen, 2-amino-1-methyl-6-phenylimidazo[4,5-b]pyridine (PhlP) in human lens. Exp Eye Res. (1993) 57:319–24. doi: 10.1006/exer.1993.1130
82. Ranjan M, Beedu SR. Spectroscopic and biochemical correlations during the course of human lens aging. BMC Ophthalmol. (2006) 6. doi: 10.1186/1471-2415-6-10
83. Cheng R, Lin B, Lee K-W, Ortwerth BJ. Similarity of the yellow chromophores isolated from human cataracts with those from ascorbic acid-modified calf lens proteins: Evidence for ascorbic acid glycation during cataract formation. Biochem Biophys Acta. (2001) 1537:14–26. doi: 10.1016/S0925-4439(01)00051-5
84. Ahmed MU, Thorpe SR, Baynes JW. Identification of N(ϵ)-carboxymethyllysine as a degradation product of fructoselysine in glycated protein. J Biol Chem. (1986) 261:4889–94. doi: 10.1016/S0021-9258(19)89188-3
85. Nagaraj RH, Monnier VM. Isolation and characterization of a blue fluorophore from human eye lens crystallins: In vitro formation from Maillard reaction with ascorbate and ribose. Biochem Biophys Acta. (1992) 1116:34–42. doi: 10.1016/0304-4165(92)90125-E
86. Tessier F, Obrenovich M, Monnier VM. Structure and mechanism of formation of human lens fluorophore LM-1. J Biol Chem. (1999) 274:20796–804. doi: 10.1074/jbc.274.30.20796
87. Manabe S, Wada O. Carcinogenic tryptophan pyrolysis products in human lens. Exp Eye Res. (1989) 48:351–3. doi: 10.1016/S0014-4835(89)80004-1
88. Dillon J, Spector A, Nakanishi K. Identification of β carbolines isolated from fluorescent human lens proteins. Nature. (1976) 259:422–3. doi: 10.1038/259422a0
89. Spector A, Roy D, Stauffer J. Isolation and characterization of an age-dependednt polypeptide from human lens with non-tryptophan fluorescence. Exp Eye Res. (1975) 21:9–24. doi: 10.1016/0014-4835(75)90053-6
90. Truscott RJW. Human cataract: the mechanisms responsible; light and butterfly eyes. Int J Biochem Cell Biol. (2003) 35:1500–4. doi: 10.1016/S1357-2725(03)00145-6
91. Tsentalovich YP, Sherin PS, Kopylova LV, Cherepanov IV, Grilj J, Vauthey E. Photochemical properties of UV filter molecules of the human eye. Invest Opthalmol Visual Sci. (2011) 52:7687. doi: 10.1167/iovs.11-8120
92. Dillon J, Atherton SJ. Time resolved spectroscopic studies on the intact human lens. Photochem Photobiol. (1990) 51:465–8. doi: 10.1111/j.1751-1097.1990.tb01738.x
93. van Heyningen R. Photo-oxidation of lens proteins by sunlight in the presence of fluorescent derivatives of kynurenine, isolated from the human lens. Exp Eye Res. (1973) 17:137–47. doi: 10.1016/0014-4835(73)90203-0
94. Bova LM, Wood AM, Jamie JF, Truscott RJW. UV filter compounds in human lenses: the origin of 4-(2-amino-3-hydroxyphenyl)-4-oxobutanoic acid O-β-d-glucoside. Invest Opthalmol Visual Sci. (1999) 40:3237–44.
95. Thiagarajan G, Shirao E, Ando K, Inoue A, Balasubramanian D. Role of xanthurenic acid 8-O-β-d-glucoside, a novel fluorophore that accumulates in the brunescent human eye lens. Photochem Photobiol. (2002) 76:368. doi: 10.1562/0031-8655(2002)076<0368:ROXAOD>2.0.CO;2
96. Parker NR, Jamie JF, Davies MJ, Truscott RJW. Protein-bound kynurenine is a photosensitizer of oxidative damage. Free Radical Biol Med. (2004) 37:1479–89. doi: 10.1016/j.freeradbiomed.2004.07.015
97. Tweeddale HJ, Hawkins CL, Janmie JF, Truscott RJW, Davies MJ. Cross-linking of lens crystallin proteins induced by tryptophan metabolites and metal ions: implications for cataract development. Free Radical Res. (2016) 50:1116–30. doi: 10.1080/10715762.2016.1210802
98. Mizdrak J, Hains PG, Truscott RJW, Jamie JF, Davies MJ. Tryptophan-derived ultraviolet filter compounds covalently bound to lens proteins are photosensitizers of oxidative damage. Free Radical Biol Med. (2008) 44:1108–19. doi: 10.1016/j.freeradbiomed.2007.12.003
99. Taylor LM, Aquilina JA, Willis RH, Jamie JF, Truscott RJW. Identification of a new human lens UV filter compound. FEBS Letters. (2001) 509:6–10. doi: 10.1016/S0014-5793(01)03102-7
100. van Heyningen R. Experimental studies on cataract. Invest Ophthalmol Vis Sci. (1976) 15:685–97.
101. van Heyningen R. What happens to the human lens in cataract. Sci American. (1975) 233:70–83. doi: 10.1038/scientificamerican1275-70
102. Wood AM, Truscott RJW. UV filters in human lenses: tryptophan catabolism. Exp Eye Res. (1993) 56:317–25. doi: 10.1006/exer.1993.1041
103. Hains PG, Gao L, Truscott RJW. The photosensitiser xanthurenic acid is not present in normal human lenses. Exp Eye Res. (2003) 77:547–53. doi: 10.1016/S0014-4835(03)00194-5
104. Luthra M, Balasubramanian D. 3-hydroxykynurenine and 3-hydroxyanthranilic acid may act as endogenous antioxidants in the eye lens. Exp Eye Res. (1992) 55:641–3. doi: 10.1016/S0014-4835(05)80177-0
105. Takikawa O, Littlejohn TK, Truscott RJW. Indoleamine 2,3-dioxygenase in the human lens, the first enzyme in the synthesis of UV filters. Exp Eye Res. (2001) 72:271–7. doi: 10.1006/exer.2000.0951
106. Borkman RF, Hibbard LB, Dillon J. The photolysis of tryptophan with 337.1 nm laser radiation. Photochem Photobiol. (1986) 43:13–9. doi: 10.1111/j.1751-1097.1986.tb05585.x
107. Finley EL, Dillon J, Crouch RK, Schey KL. Identification of tryptophan oxidation products in bovine α-crystallin. Protein Sci. (1998) 7:2391–7. doi: 10.1002/pro.5560071116
108. Pirie A. Formation of N-formylkynurenine in proteins from lens and other sources by exposure to sunlight. Biochem J. (1971) 125:203–8. doi: 10.1042/bj1250203
109. Taylor LM, Aquilina JA, Jamie JF, Truscott RJW. UV filter instability: consequences for the human lens. Exp Eye Res. (2002) 75:165–75. doi: 10.1006/exer.2002.2012
110. Bova LM, Sweeney MHJ, Jamie JF, Truscott RJW. Major changes in human ocular UV protection with age. Invest Ophthalmol Visual Sci. (2001) 42:200–5.
111. Taylor LM, Aquilina JA, Jamie JF, Truscott RJW. Glutathione and NADH, but not Ascorbate, Protect Lens Proteins from Modification by UV Filters. Exp Eye Res. (2002) 74:503–11. doi: 10.1006/exer.2001.1165
112. Tsentalovich YP, Snytnikova OA, Forbes MDE, Chernyak EI, Morozov SV. Photochemical and thermal reactivity of kynurenine. Exp Eye Res. (2006) 83:1439–45. doi: 10.1016/j.exer.2006.07.022
113. Tsentalovich YP, Snytnikova OA, Sagdeev RZ. Photochemical and thermal reactions of kynurenines. Russian Chem Rev. (2008) 77:189–97. doi: 10.1070/RC2008v077n09ABEH003880
114. Stutchbury GM, Truscott RJW. The modification o proteins by 3-hydroxykynurenine. Exp Eye Res. (1993) 57:149–55. doi: 10.1006/exer.1993.1110
115. Demarais NJ, Donaldson PJ, Grey AC. Age-related spatial differences of human lens UV filters revealed by negative ion mode MALDI imaging mass spectrometry. Exp Eye Res. (2019) 184:146–51. doi: 10.1016/j.exer.2019.04.016
116. Tamara SO, Yanshole LV, Yanshole VV, Fursova AZ, Stepakov DA, Novoselov VP, et al. Spatial distribution of metabolites in the human lens. Exp Eye Res. (2016) 143:68–74. doi: 10.1016/j.exer.2015.10.015
117. Garner B, Vazquez S, Griffith R, Lindner RA, Carver JA, Truscott RJW. Identification of glutathionyl-3-hydroxykynurenine glucoside as a novel fluorophore associated with aging of the human lens. J Biol Chem. (1999) 274:20847–54. doi: 10.1074/jbc.274.30.20847
118. Hains PG, Mizdrak J, Streete IM, Jamie JF, Truscott RJW. Identification of the new UV filter compound cysteine-L-3-hydroxykynurenine O-B-D-glucoside in human lenses. FEBS Letters. (2006) 580:5071–6. doi: 10.1016/j.febslet.2006.08.026
119. Korlimbinis A, Truscott RJW. Identification of 3-hydroxykynurenine bound to proteins in the human lens. A possible role in age-related nuclear cataract†. Biochemistry. (2006) 45:1950–60. doi: 10.1021/bi051744y
120. Truscott RJ, Martin F. The reaction of proteins with 3-hydroxyanthranilic acid as a possible model for senile nuclear cataract in man. Exp Eye Res. (1989) 49:927–40. doi: 10.1016/S0014-4835(89)80017-X
121. Hood BD, Garner B, Truscott RJW. Human lens coloration and aging. J Biol Chem. (1999) 274:32547–50. doi: 10.1074/jbc.274.46.32547
122. Korlimbinis A, Aquilina JA, Truscott RJ. Protein-bound and free UV filters in cataract lenses. concent UV filters is much lower than normal lenses Exp Eye Res. (2007) 85:21–225. doi: 10.1016/j.exer.2007.04.004
123. Streete IM, Jamie JF, Truscott RJW. Lenticular levels of amino acids and free UV filters differ significantly between normals and cataract patients. Invest Opthalmol Visual Sci. (2004) 45:4091. doi: 10.1167/iovs.04-0178
124. Truscott RJW, Augusteyn RC. The state of sulphydryl groups in normal and cataractous human lenses. Exp Eye Res. (1977) 25:139–48. doi: 10.1016/0014-4835(77)90126-9
125. Roberts JE, Wishart JF, Martinez L, Chignell CF. Photochemical studies on xanthurenic acid. Photochem Photobiol. (2000) 72:467–71. doi: 10.1562/0031-8655(2000)072<0467:PSOXA>2.0.CO;2
126. Shirao E, Ando K, Inoue A, Shirao Y, Balasubramanian D. Identification of a novel fluorophore, xanthurenic acid 8-O-D-glucoside in human brunescent cataract. Exp Eye Res. (2001) 73:421–31. doi: 10.1006/exer.2001.1051
127. Hains PG, Truscott RJ. Proteomic analysis of the oxidation of cysteine residues in human age-related nuclear cataract lenses. Biochim Biophys Acta. (2008) 1784:1959–64. doi: 10.1016/j.bbapap.2008.07.016
128. Malina HZ, Martin XD. Xanthurenic acid derivative formation in the lens is responsible for senile cataract in humans. Graefe's Arch Clin Exp Ophthalmol. (1996) 234:723–30. doi: 10.1007/BF00189352
129. Roberts JE, Finley EL, Patat SA, Schey KL. Photooxidation of lens proteins with zanthurenic acid: A putative chromophore for cataractogenesis. Photochem Photobiol. (2001) 74:740–4. doi: 10.1562/0031-8655(2001)074<0740:POLPWX>2.0.CO;2
130. Linetsky M, Raghavan CT, Johar K, Fan X, Monnier VM, Vasavada AR, et al. UVA light-excited kynurenines oxidize ascorbate and modify lens proteins through the formation of advanced glycation end products. Implic Hum lens Aging cataract form J Biol Chem. (2014) 289:17111–23. doi: 10.1074/jbc.M114.554410
131. Garner MH, Spector A. Selective oxidation of cysteine and methionine in normal and senile cataractous lenses. Proc Natl Acad Sci USA. (1980) 77:1274–7. doi: 10.1073/pnas.77.3.1274
132. Spector A, Wang G-M, Wang R-H, Li W-C, Kleiman NJ. A brief photochemically induced oxidative insult causes irreversible lens damage and cataract II. Mech action Exp Eye Res. (1995) 60:483–93. doi: 10.1016/S0014-4835(05)80063-6
133. Finley EL, Busman M, Dillon J, Crouch RK, Schey KL. Identification of photooxidation sites in bovine α-crystallin. Photochem Photobiol. (1997) 66:635–41. doi: 10.1111/j.1751-1097.1997.tb03200.x
134. Korlimbinis A, Hains PG, Truscott RJW, Aquilina JA. 3-hydroxykynurenine oxidizes alpha-crystallin: potential role in cataractogenesis. Biochemistry. (2006) 45:1852–60. doi: 10.1021/bi051737+
135. Breadsell RO, Wegener A, Breipohl W, Hirst LW. UV-B radiation-induced cataract in the royal college of surgeons rat. Ophthal Res. (1994) 26:84–9. doi: 10.1159/000267522
136. Chauss D, Brennan LA, Bakina O, Kantorow M. Integrin aV5-mediated Removal of Apoptotic Cell Debris bythe Eye Lens and Its Inhibition by UV Light Exposure. J Biol Chem. (2015) 290:30253–66. doi: 10.1074/jbc.M115.688390
137. Lv J, Xing Y. Effects of UV on apoptotic factors in lens epithelial cells of an animal model. Exp Ther Med. (2018) 16:2309–12. doi: 10.3892/etm
138. Xiang J-W, Xiao Y, Gan Y, Chen H, Liu Y, Wang L, et al. Glucose oxidase- and UVA-induced changes in the expression patterns of seven de-sumoylation enzymes (SENPs) are associated with cataract development. Curr Mol Med. (2019) 19:48–53. doi: 10.2174/1566524019666190311094313
139. Jiang Y, Fu R, Zhao J, Wu D, Qiao G, Li R. Effects of ELL-associated factor 2 on ultraviolet radiation-induced cataract formation in mice. Mol Med Rep. (2015) 12:6605–11. doi: 10.3892/mmr.2015.4281
140. Ayala M, Strid H, Jacobsson U, Söderberg PG. p53 expression and apoptosis in the lens after ultraviolet radiation exposure. Invest Opthalmol Visual Sci. (2007) 48:4187. doi: 10.1167/iovs.06-0660
141. Michael R, Vrensen GFJM, van Marle J, Gan L, Söderberg PG. Apoptosis in the rat lens after in vivo threshold dose ultraviolet irradiation. Invest Ophthalmol Visual Sci. (1998) 39:2681–7.
142. Li W-C, Spector A. Lens epithelial cell apoptosis is an early event in the development of UVB-induced cataract. Free Radical Biol Med. (1995) 20:301–11. doi: 10.1016/0891-5849(96)02050-3
143. Ueda Y, Duncan MK, David LL. Lens proteomics: the accumulation of crystallin modifications in the mouse lens with age. Invest Ophthalmol Visual Sci. (2002) 43:205–15.
144. Bloemendal H, De Jong W, Jaenicke R, Lubsen NH, Slingsby C, Tardieu A. Ageing and vision: structure, stability and function of lens crystallins. Prog Biophys Mol Biol. (2004) 86:407–85. doi: 10.1016/j.pbiomolbio.2003.11.012
145. Gouras P, Ekesten B. Why do mice have ultra-violet vision? Exp Eye Res. (2004) 79:887–92. doi: 10.1016/j.exer.2004.06.031
146. Varma SD, Hegde KR, Kovtun S. UV-B-induced damage to the lens in vitro prevention by caffeine. J Ocular Pharmacol Ther. (2008) 24:439–44. doi: 10.1089/jop.2008.0035
147. Varma SD, Kovtun S, Hegde KR. Role of ultraviolet irradiation and oxidative stress in cataract formation—Medical prevention by nutritional antioxidants and metabolic agonists. Eye Contact Lens: Sci Clin Pract. (2011) 37:233–45. doi: 10.1097/ICL.0b013e31821ec4f2
148. Jose JG. Posterior cataract induction by UV-B radiation in albino mice. Exp Eye Res. (1986) 42:11–20. doi: 10.1016/0014-4835(86)90013-8
149. Jose JG, Pitts DG. Wavelength dependency of cataracts in albino mice following chronic exposure. Exp Eye Res. (1985) 41:545–63. doi: 10.1016/S0014-4835(85)80011-7
150. Meyer LM, Söderberg P, Dong X, Wegener A. UVR-B induced cataract development in C57 mice. Exp Eye Res. (2005) 81:389–94. doi: 10.1016/j.exer.2005.02.009
151. Meyer LM, Löfgren S, Holz FG, Wegener A, Söderberg P. Bilateral cataract induced by unilateral UVR-B exposure - evidence for an inflammatory response. Acta Ophthalmol. (2013) 91:236–42. doi: 10.1111/j.1755-3768.2012.02384.x
152. Zigman S, Schultz J, Yulo T. Possible roles of near UV light in the cataractous process. Exp Eye Res. (1973) 15:201–8. doi: 10.1016/0014-4835(73)90120-6
153. Zigman S, Yulo T, Schultz J. Cataract induction in mice exposed to near UV light. Ophthal Res. (1974) 6:259–70. doi: 10.1159/000264710
154. Pirie A. Color and solubility of the proteins of human cataracts. Invest Ophthalmol Visual Sci. (1968) 7:634–50.
155. Zhang J, Yan H, Löfgren S, Tian X, Lou MF. Ultraviolet radiation–induced cataract in mice: the effect of age and the potential biochemical mechanism. Invest Opthalmol Visual Sci. (2012) 53:7276. doi: 10.1167/iovs.12-10482
156. Meyer LM, Löfgren S, Ho Y-S, Lou MF, Wegener A, Holz F, et al. Absence of glutaredoxin1 increases lens susceptibility to oxidative stress induced by UVR-B. Exp Eye Res. (2009) 89:833–9. doi: 10.1016/j.exer.2009.07.020
157. Padgaonkar VA, Leverenz VR, Bhat AV, Pelliccia SE, Giblin FJ. Thioredoxin Reductase Activity may be More Important than GSH Level in Protecting Human Lens Epithelial Cells against UVA Light. Photochem Photobiol. (2015) 91:387–96. doi: 10.1111/php.12404
158. Xing K-Y, Lou MF. Effect of age on the thioltransferase (Glutaredoxin) and thioredoxin systems in the human lens. Invest Ophthalmol Visual Sci. (2010) 51:6598. doi: 10.1167/iovs.10-5672
159. Ramaekers F, Dodemont H, Vorstenbosch P, Bloemendal H. Classification of rat lens crystallins and identification of proteins encoded by rat lens mRNA. Eur J Biochem. (1982) 128:503–8. doi: 10.1111/j.1432-1033.1982.tb06993.x
160. Galichanin K, Löfgren S, Söderberg PG. Cataract after Repeated Daily in vivo Exposure to Ultraviolet Radiation. Health Phys. (2014) 107:523–9. doi: 10.1097/HP.0000000000000152
161. Levere TE. The primary visual system of the rat: A primer of its anatomy. Physiol Psychol. (1978) 6:142–69. doi: 10.3758/BF03326707
162. Gorgels TG, van Norren D. Ultraviolet and green light cause different types of damage in rat retina. Invest Ophthalmol Visual Sci. (1995) 36:851–63.
163. Galichanin K, Löfgren S, Bergmanson J, Söderberg PG. Evolution of damage in the lens after in vivo close to threshold exposure to UV-B radiation: Cytomorphological study of apoptosis. Exp Eye Res. (2010) 91:369–77. doi: 10.1016/j.exer.2010.06.009
164. Torriglia A, Zigman S. The effect of near-UV light on Na-K-ATPase of the rat lens. Curr Eye Res. (1988) 7:539–48. doi: 10.3109/02713688809031809
165. Risa Ø, Sæther O, Löfgren S, Söderberg PG, Krane J, Midelfart A. Metabolic changes in rat lens after in vivo exposure to ultraviolet irradiation: measurements by high resolution MAS1H NMR spectroscopy. Invest Opthalmol Visual Sci. (2004) 45:1916. doi: 10.1167/iovs.03-1292
166. Risa Ø, Sæther O, Kakar M, Mody VC, Löfgren S, Söderberg PG, et al. Time dependency of metabolic changes in rat lens after in vivo UVB irradiation analysed by HR-MAS 1H NMR spectroscopy. Exp Eye Res. (2005) 81:407–24. doi: 10.1016/j.exer.2005.02.012
167. Löfgren S, Söderberg PG. Rat lens glycolysis after in vivo exposure to narrow band UV or blue light radiation. J Photochem Photobiol B: Biol. (1995) 30:145–51. doi: 10.1016/1011-1344(95)07183-3
168. Hightower KR, Harrison SE. The influence of calcium on glucose metabolism in the rabbit lens. Invest Ophthalmol Visual Sci. (1987) 28:1433–6.
169. Reddy B, Bhat S. Protection against UVB inactivation (in vitro) of rat lens enzymes by natural antioxidants. Mol Cell Biochem. (1999) 194:41–5. doi: 10.1023/a:1006966318403
170. Christen WG, Glynn RJ, Sesso HD, Kurth T, MacFadyen J, Bubes V, et al. Age-related cataract in a randomized trial of vitamins E and C in men. Arch Ophthalmol. (2010) 128:1397. doi: 10.1001/archophthalmol.2010.266
171. Mathew MC, Ervin A-M, Tao J, Davis RM. Antioxidant vitamin supplementation for preventing and slowing the progression of age-related cataract. Cochrane Database Systema Rev. (2012) 6:1–62. doi: 10.1002/14651858
172. Lim JC, Caballero Arredondo M, Braakhuis AJ, Donaldson PJ. Vitamin C and the lens: new insights into delaying the onset of cataract. Nutrients. (2020) 12:3142. doi: 10.3390/nu12103142
173. Löfgren S, Michael R, Söderberg P. Impact of iris pigment and pupil size in ultraviolet radiation cataract in rat. Acta Ophthalmol. (2010) 90:44–8. doi: 10.1111/j.1755-3768.2010.01871.x
174. Löfgren S. Lenses from Brown-Norway pigmented rats are more tolerant to in vitro ultraviolet irradiation than lenses from Fischer-344 albino rats. Acta Ophthalmol. (2012) 90:179–83. doi: 10.1111/j.1755-3768.2010.01903.x
175. Merriam JC, Löfgren S, Michael R, Söderberg PG, Dillon JP, Zheng L, et al. An action spectrum for UV-B radiation and the rat lens. Invest Ophthalmol Visual Sci. (2000) 41:2642–7.
176. Negelspach DC, Kaladchibachi S, Fernandez F. The circadian activity rhythm is reset by nanowatt pulses of ultraviolet light. Proc R Soc B: Biol Sci. (2018) 285:20181288. doi: 10.1098/rspb.2018.1288
177. Borchman D, Giblin FJ, Leverenz VR, Reddy VN, Lin L-R, Yappert MC, et al. Impact of aging and hyperbaric oxygen in vivo on Guinea pig lens lipids and nuclear light scatter. Invest Ophthalmol Visual sci. (2000) 41:3061–73.
178. Giblin FJ, Anderson DM, Han J, Rose KL, Wang Z, Schey KL. Acceleration of age-induced proteolysis in the Guinea pig lens nucleus by in vivo exposure to hyperbaric oxygen: a mass spectrometry analysis. Exp Eye Res. (2021) 210:1–8. doi: 10.1016/j.exer.2021.108697
179. Giblin FJ, Padgaonkar VA, Leverenz VR, Lin L-R, Lou MF, Unakar NJ, et al. Nuclear light scattering, disulfide formation and membrane damage in lenses of older Guinea pigs treated with hyperbaric oxygen. Exp Eye Res. (1995) 60:219–35. doi: 10.1016/S0014-4835(05)80105-8
180. Padgaonkar VA, Leverenz VR, Rinke A, Reddy VN, Giblin FJ. Hyperbaric oxygen in vivo accelerates the loss of cytoskeletal proteins and MIP26 in Guinea pig lens nucleus. Exp Eye Res. (1999) 68:493–505. doi: 10.1006/exer.1998.0630
181. Kosegarten DC, Maher TJ. Use of Guinea pigs as model to study galactose-induced cataract formation. J Pharma Sci. (1978) 67:1478–9. doi: 10.1002/jps.2600671045
182. Yokoyama T, Sasaki H, Giblin FJ, Reddy VN. A physiological level of ascorbate inhibits galactose cataract in Guinea pigs by decreasing polyol accumulation in the lens epithelium: a dehydroascorbate-linked mechanism. Exp Eye Res. (1994) 58:207–18. doi: 10.1006/exer.1994.1009
183. Giblin FJ. Glutathione: A vital lens antioxidant. J Ocular Pharmacol Ther. (2000) 16:121–35. doi: 10.1089/jop.2000.16.121
184. Yu N-T, DeNagel DC, Pruett PL, Kuck JFR. Disulfide bond formation in the eye lens. Proc Natl Acad Sci. (1985) 82:7965–8. doi: 10.1073/pnas.82.23.7965
185. Barron BC, Yu N-T, Kuck JFRJ. Raman spectroscopic evaluation of aging and long-wave UV exposure in the Guinea pig lens: A possible model for human aging. Exp Eye Res. (1988) 46:249–58. doi: 10.1016/S0014-4835(88)80082-4
186. Bergbauer KL, Kuck JFR, Su KC, Yu N-T. Use of a UV-Blocking contact lens in evaluation of UV-induced damage to the Guinea pig lens. Int Contact Lens Clinic. (1991) 18:182–7. doi: 10.1016/0892-8967(91)90005-K
187. Truscott RJW, Augusteyn RC. Changes in human lens proteins during nuclear cataract formation. Exp Eye Res. (1977) 24:159–70. doi: 10.1016/0014-4835(77)90256-1
188. Simpanya MF, Ansari RR, Leverenz VR, Giblin FJ. Measurement of lens protein aggregation in vivo using dynamic light scattering in a Guinea pig/UVA model for nuclear cataract. Photochem Photobiol. (2008) 84:1589–95. doi: 10.1111/j.1751-1097.2008.00390.x
189. Cunningham ML, Johnson JS, Giovanazzi SM, Peak MJ. Photosensitized production of superoxide anion by monochromatic (290-405 nm) ultraviolet irradiation of NADH and NADPH coenzymes. Photochem Photobiol. (1985) 42:125–8. doi: 10.1111/j.1751-1097.1985.tb01549.x
190. Czochralska B, Kawczynski W, Bartosz G, Shugar D. Oxidation of excited-state NADH and NAD dimer in aqueous medium involvement of O2 as a mediator in the presence of oxygen. Biochem Biophys Acta. (1984) 801:403–9. doi: 10.1016/0304-4165(84)90145-4
191. Mody VC, Kakar M, Söderberg PG, Löfgren S. High lenticular tolerance to ultraviolet radiation-B by pigmented Guinea pig; application of a safety limit strategy for UVR induced cataract. Acta Ophthalmol. (2012) 90:226–30. doi: 10.1111/j.1755-3768.2010.01931.x
192. Rao CM, Zigler JS. Levels of reduced pyridine nucleotides and lens photodamage. Photochem Photobiol. (1992) 56:523–8. doi: 10.1111/j.1751-1097.1992.tb02196.x
193. Rao PV, Gonzalez P, Persson B, Jörnvall H, Garland D, Zigler JS. Guinea pig and bovine ζ-crystallins have distinct functional characteristics highlighting replacements in otherwise similar structures. Biochemistry. (1997) 36:5353–62. doi: 10.1021/bi9622985
194. Zigman S, Paxhia T, McDaniel T, Lou MF, Yu N-T. Effect of chronic near-ultraviolet radiation on the gray squirrel lens in vivo. Invest Ophthalmol Visual Sci. (1991) 32:1723–32.
195. Blondin J, Baragi V, Schwartz E, Sadowski JA, Taylor A. Delay of UV induced eye lens protein damage in Guinea pigs by dietary ascorbate. J Free Radicals Biol Med. (1986) 2:275–81. doi: 10.1016/S0748-5514(86)80010-1
196. Malik A, Kojima M, Sasaki K. Morphological and biochemical changes in lenses of Guinea pigs after vitamin-C-deficient diet and UV-B radiation. Ophthal Res. (1995) 27:189–96. doi: 10.1159/000267704
197. Reddy VN, Giblin FJ, Lin L-R, Chakrapani B. The effect of aqueous humor ascorbate on ultraviolet B-induced DNA damage in lens epithelium. Invest Ophthalmol Visual Sci. (1998) 39:344–50.
198. Giblin FJ, Schrimscher L, Chakrapani B, Reddy VN. Exposure of rabbit lens to hyperbaric oxygen in vitro: regional effects on GSH level. Invest Ophthalmol Visual Sci. (1988) 29:1312–9.
199. Padgaonkar VA, Giblin FJ, Reddy VN. Disulfide cross-linking of urea-insoluble proteins in rabbit lenses treated with hyperbaric oxygen. Exp Eye Res. (1989) 49:887–99. doi: 10.1016/s0014-4835(89)80047-8
200. Padgaonkar VA, Giblin FJ, Reddan JR, Dziedzic DC. Hyperbaric oxygen inhibits the growth of cultured rabbit lens epithelial cells without affecting glutathione level. Exp Eye Res. (1993) 56:443–52. doi: 10.1006/exer.1993.1057
201. Rogers CS, Chan L-M, Sims YS, Byrd KD, Hinton DL, Twining SS. The effects of sub solar levels of UV-A and UV-B on rabbit corneal and lens epithelial cells. Exp Eye Res. (2004) 78:1007–14. doi: 10.1016/j.exer.2003.12.011
202. Zigman S, McDaniel T, Schultz JB, Reddan J, Meydani M. Damage to cultured lens epithelial cells of squirrels and rabbits by UV-A (99.9%) plus UV-B (0.1%) radiation and alpha tocopherol protection. Mol Cell Biochem. (1995) 143:35–46. doi: 10.1007/bf00925924
203. Sidjanin D, Grdina D, Woloschak GE. UV-induced changes in cell cycle and gene expression within rabbit lens epithelial cells. Photochem Photobiol. (1996) 63:79–85. doi: 10.1111/j.1751-1097.1996.tb02995.x
204. Sidjanin D, Zigman S, Reddan J. DNA damage and repair in rabbit lens epithelial cells following UVA radiation. Curr Eye Res. (1993) 12:773–81. doi: 10.3109/02713689309020382
205. Zigman S, Reddan J, Schultz JB, McDaniel T. Structural and functional changes in catalase induced by near-UV radiation. Photochem Photobiol. (1996) 63:818–24. doi: 10.1111/j.1751-1097.1996.tb09637.x
206. Andley UP, Lewis RM, Reddan JR, Kochevar IE. Action spectrum for cytotoxicity in the UVA- and UVB-wavelength region in cultured lens epithelial cells. Invest Ophthalmol Visual Sci. (1994) 35:367–73.
207. Giblin FJ, Lin L-R, Simpanya MF, Leverenz VR, Fick CE. A Class I UV-blocking (senofilcon A) soft contact lens prevents UVA-induced yellow fluorescence and NADH loss in the rabbit lens nucleus in vivo. Exp Eye Res. (2012) 102:17–27. doi: 10.1016/j.exer.2012.06.007
208. Chandler HL, Reuter KS, Sinnott LT, Nichols JJ. Prevention of UV-induced damage to the anterior segment using class I UV-absorbing hydrogel contact lenses. Invest Ophthalmol Visual Sci. (2010) 51:172–8. doi: 10.1167/iovs.09-3996
209. Pitts DG, Cullen AP, Hacker PD. Ocular effects of ultraviolet radiation from 295 to 365 nm. Invest Ophthalmol Visual Sci. (1977) 16:932–9.
210. Rafferty NS, Zigman S, McDaniel T, Scholz DL. Near-UV radiation disrupts filamentous actin in lens epithelial cells. Cell Motil Cytoskeleton. (1993) 26:40–8. doi: 10.1002/cm.970260105
211. Fris M, Čejková J, Midelfart A. The effect of single and repeated UVB radiation on rabbit lens. Graefe's Arch Clin Exp Ophthalmol. (2008) 246:551–8. doi: 10.1007/s00417-007-0747-6
212. Hightower KR, McCready J. Physiological effects of UVB irradiation on cultured rabbit lens. Invest Ophthalmol Visual Sci. (1992) 33:1783–7.
213. Hightower K, McCready J. Comparative effect of UVA and UVB on cultured rabbit lens. Photochem Photobiol. (1993) 58:827–30. doi: 10.1111/j.1751-1097.1993.tb04978.x
214. Tessem M-B, Midelfart A, Čejková J, Bathen TF. Effect of UVA and UVB irradiation on the metabolic profile of rabbit cornea and lens analysed by HR-MAS 1H NMR spectroscopy. Ophthal Res. (2006) 38:105–14. doi: 10.1159/000090511
215. Čejka Č, Pláteník J, Buchal R, Guryca V, Širc J, Vejražka M, et al. Effect of two different UVA doses on the rabbit cornea and lens. Photochem Photobiol. (2009) 85:794–800. doi: 10.1111/j.1751-1097.2008.00478.x
216. van Heyningen R. Fluorescent glucoside in the human lens. Nature. (1971) 230:393–4. doi: 10.1038/230393a0
217. van Heyningen R. Fluorescent derivatives of 3-hydroxy-L-kynurenine in the lens of man, the baboon and the grey squirrel. Biochem J. (1971) 123:30P–1P. doi: 10.1042/bj1230030P
218. Manthey MK, Jamie JF, Truscott RJW. Synthesis of human ultraviolet filter compounds: O-β-d-glucopyranosides of 3-hydroxykynurenine and 2-amino-3-hydroxy-γ-oxobenzenebutanoic acid. J Organ Chem. (1999) 64:3930–3. doi: 10.1021/jo982321n
219. Gaillard ER, Zheng L, Merriam JC, Dillon JP. Age-related changes in the absorption characteristics of the primate lens. Invest Ophthalmol Visual Sci. (2000) 41:1454–9.
220. Borja D, Manns F, Ho A, Ziebarth NM, Acosta AC, Arrieta-Quintero E, et al. Refractive power and biometric properties of the nonhuman primate isolated crystalline lens. Invest Ophthalmol Visual Sci. (2010) 51:2118–25. doi: 10.1167/iovs.09-3905
221. Gaillard ER, Merriam JC, Zheng L, Dillon J. Transmission of light to the young primate retina: possible implicationsfor the formation of lipofuscin. Photochem Photobiol. (2011) 87:18–21. doi: 10.1111/j.1751-1097.2010.00837.x
222. Pitts DG, Bergmanson JPG, Chu LWF. Ultrastructural analysis of corneal exposure to UV radiation. Acta Ophthalmol. (1987) 65:263–73. doi: 10.1111/j.1755-3768.1987.tb08504.x
223. Collier R, Zigman S. The grey squirrel lens protects the retina from near-UV radiation damage. Prog Clin Biol Res. (1987) 247:571–85.
224. Zigman S, Paxhia T. The nature and properties of squirrel lens yellow pigment. Exp Eye Res. (1988) 47:819–24. doi: 10.1016/0014-4835(88)90065-6
225. Hains PG, Simpanya MF, Giblin F, Truscott RJW. UV filters in the lens of the thirteen lined ground squirrel (Spermophilus tridecemlineatus). Exp Eye Res. (2006) 82:730–7. doi: 10.1016/j.exer.2005.09.014
226. Lyons B, Karuso P, Jamie JF, Simpanya MF, Giblin FJ, Truscott RJW. Characterisation of a novel UV filter in the lens of the thirteen-lined ground squirrel (Ictidomys tridecemlineatus). Exp Eye Res. (2014) 121:114–20. doi: 10.1016/j.exer.2014.01.022
227. Malina HZ, Martin XD. Deamination of 3-hydroxykynurenine in bovine lenses: a possible mechanism of cataract formation in general. Graefe's Arch Clin Exp Ophthalmol. (1995) 233:38–44. doi: 10.1007/BF00177784
228. Thorpe A, Truscott RJW, Douglas RH. Kynurenine identified as the short-wave absorbing lens pigment in the deep-sea fish stylephorus chordatus. Exp Eye Res. (1992) 55:53–7. doi: 10.1016/0014-4835(92)90091-6
229. Truscott RJ, Carver JA, Thorpe A, Douglas RH. Identification of 3-hydroxykynurenine as the lens pigment in the gourami Trichogaster trichopterus. Exp Eye Res. (1992) 54:1015–7. doi: 10.1016/0014-4835(92)90167-Q
230. Zigman S. Special features of the lens relative to the environment. Lens Eye Toxic Res. (1989) 6:807–21.
231. Douglas RH, Jeffery G. The spectral transmission of ocular media suggests ultraviolet sensitivity is widespread among mammals. Proc R Soc B: Biol Sci. (2014) 281:20132995. doi: 10.1098/rspb.2013.2995
232. Grey AC, Schey KL. Distribution of bovine and rabbit lens α-crystallin products by MALDI imaging mass spectrometry. Mol Vision. (2008) 14:171–9.
233. Selivanova OM, Galzitskaya OV. Structural and functional peculiarities of α-crystallin. Biology. (2020) 9:85. doi: 10.3390/biology9040085
234. Marussich L, Manns F, Nankivil D, Maceo Heilman B, Yao Y, Arrieta-Quintero E, et al. Measurement of crystalline lens volume during accommodation in a lens stretcher. Invest Ophthalmol Visual Sci. (2015) 56:4239–48. doi: 10.1167/iovs.15-17050
235. Hott JL, Borkman RF. Concentration dependence of transmission losses in UV-laser irradiated bovine α-, βH-,βL- and γ-crystallin solutions. Photochem Photobiol. (1993) 57:312–7. doi: 10.1111/j.1751-1097.1993.tb02293.x
236. Krivandin AV, Muranov KO, Yakovlev FY, Poliansky NB, Wasserman LA, Ostrovsky MA. Resistance of α-crystallin quaternary structure to UV irradiation. Biochem (Moscow). (2009) 74:633–42. doi: 10.1134/S0006297909060078
237. Ostrovsky MA, Sergeev YV, Atkinson DB, Soustov LV, Hejtmancik JF. Comparison of ultraviolet induced photo-kinetics for lens-derived and recombinant β-crystallins. Mol Vision. (2002) 8:72–8.
238. Chelnokov E, Soustov L, Sapogova N, Ostrovsky M, Bityurin N. Nonreciprocal XeCl laser-induced aggregation of β -crystallins in water solution. Opt Express. (2008) 16:18798. doi: 10.1364/OE.16.018798
239. Kleiman NJ, Wang R-R, Spector A. Ultraviolet induced DNA damage and repair in bovine lens epithelial cells. Curr Eye Res. (1990) 9:1185–93. doi: 10.3109/02713689009003475
240. Stuart DD, Cullen AP, Sivak JG, Doughty MJ. Optical effects of UV-A and UV-B radiation on the cultured bovine lens. Curr Eye Res. (1994) 13:371–6. doi: 10.3109/02713689409167301
241. Stuart DD, Sivak JG, Cullen AP, Weerheim JA, Monteith CA. UV-B radiation and the optical properties of cultured bovine lenses. Curr Eye Res. (1991) 10:177–84. doi: 10.3109/02713689109001746
242. Dovrat A, Weinreb O. Recovery of lens optics and epitehlial enzymes after ultraviolet A radiation. Invest Ophthalmol Visual Sci. (1995) 36:2417–24.
243. Reddy B, Bhat S. UVB irradiation alters the activities and kinetic properties of the enzymes of energy metabolism in rat lens during aging. J Photochem Photobiol B: Biol. (1998) 42:40–6. doi: 10.1016/S1011-1344(97)00114-0
244. Dovrat A, Weinreb O. Effects of UV-A radiation on lens epithelial NaK-ATPase in organ culture. Invest Ophthalmol Visual Sci. (1999) 40:1616–20.
245. Weinreb O, Adrianus M, van Boekel M, Dovrat A, Bloemendal H. Effect of UV-A light on the chaperone-like properties of young and old lens α-crystallin. Invest Ophthalmol Visual Sci. (2000) 41:191–8.
246. van Heyningen R. The human lens III some observations on the post-mortem lens. Exp Eye Res. (1972) 13:155–60. doi: 10.1016/0014-4835(72)90028-0
247. Evans JW. Anatomy and histology of the eye and orbit in domestic animals. Arch Neurol. (1961) 5:693–. doi: 10.1001/archneur.1961.00450180115017
248. Olsen TW, Sanderson S, Feng X, Hubbard WC. Porcine sclera: thickness and suface area. Invest Ophthalmol Visual Sci. (2002) 43:2529–32.
249. Artigas CN, López-Murcia M-M, Felipe A, Desco C, Artigas J-M. Spectral transmission of the pig lens: effect of ultraviolet A+B radiation. J Francais d'Ophtalmol. (2014) 37:773–9. doi: 10.1016/j.jfo.2014.06.006
250. Keenan J, Orr DF, Pierscionek BK. Patterns of crystallin distribution in porcine eye lenses. Mol Vision. (2008) 14:1245–53.
251. Heys KR, Friedrich MG, Truscott RJW. Presbyopia and heat: changes associated with aging of the human lens suggest a functional role for the small heat shock protein, α-crystallin, in maintaining lens flexibility. Aging Cell. (2007) 6:807–15. doi: 10.1111/j.1474-9726.2007.00342.x
252. Oriowo OM, Cullen AP, Sivak JG. Impairment of eye lens cell physiology and optics by broadband ultraviolet A-ultraviolet B radiation. Photochem Photobiol. (2002) 76:361–7. doi: 10.1562/0031-8655(2002)076<0361:IOELCP>2.0.CO;2
253. Lee J-S, Liao J-H, Wu S-H, Chiou S-H. a-crystallin acting as a molecular chaperonin against photodamage by UV irradiation. J Protein Chem. (1997) 16:283–9. doi: 10.1023/A:1026305025816
254. Honisch C, Donadello V, Hussain R, Peterle D, De Filippis V, Arrigoni G, et al. Application of circular dichroism and fluorescence spectroscopies to assess photostability of water-soluble porcine lens proteins. ACS Omega. (2020) 5:4293–301. doi: 10.1021/acsomega.9b04234
255. Zhang TO, Alperstein AM, Zanni MT. Amyloid β-sheet secondary structure identified in UV-induced cataracts of porcine lenses using 2D IR spectroscopy. J Mol Biol. (2017) 429:1705–21. doi: 10.1016/j.jmb.2017.04.014
256. Oriowo OM, Cullen AP, Chou BR, Sivak JG. Action spectrum and recovery for in vitro UV-induced cataract using whole lenses. Invest Ophthalmol Visual Sci. (2001) 42:2596–602.
257. Yuan D, Liu Q. Photon energy and photon behaviour discussions. Energy Rep. (2022) 8:22–42. doi: 10.1016/j.egyr.2021.11.034
258. Lou MF, Dickerson JE. Protein-thiol mixed disulfides in human lens. Exp Eye Res. (1992) 55:889–96. doi: 10.1016/0014-4835(92)90015-K
259. Thomas DM, Papadopoulou O, Mahendroo PP, Zigman S. Phosphorous-31 NMR study of the effects of UV on squirrel lenses. Exp Eye Res. (1993) 57:59–65. doi: 10.1006/exer.1993.1099
260. Nagalaxmi V, Praveen KM, Sashidhar R, Turlapati NR. UV-B exposure increases the activity of indoleamine 2, 3-dioxygenase (Ido) and alters the levels of tryptophan metabolites in Indian ground squirrel (Funambulus palmarum) lens. J Diabetic Complic Med. (2015) 1:6. doi: 10.4172/2475-3211.1000102
261. Zigman S, Paxhia T, Waldron W. Effects of near-UV radiation on the protein of the grey squirrel lens. Curr Eye Res. (1988) 7:531–7. doi: 10.3109/02713688809031808
262. Horwitz J, Neuhaus R, Dockstader J. Analysis of microdissected cataractous human lenses. Invest Ophthalmol Visual Sci. (1981) 21:616–9.
263. Takikawa O, Truscott RJW, Fukao M, Miwa S. Age-Related Nuclear Cataract and Indoleamine 2,3-Dioxygenase-Initiated Tryptophan Metabolism in the Human Lens. Boston, MA, USA: Springer US (2003) p. 277–85.
264. Malina HZ, Martin XD. Indoleamine 2,3-dioxygenase: Antioxidant enzyme in the human eye. Graefe's Arch Clin Exp Ophthalmol. (1996) 234:457–62. doi: 10.1007/BF02539413
265. Donaldson PJ, Grey AC, Maceo Heilman B, Lim JC, Vaghefi E. The physiological optics of the lens. Prog Retinal Eye Res. (2016) 56:1–24. doi: 10.1016/j.preteyeres.2016.09.002
266. Wood AM, Truscott RJW. Ultraviolet filter compounds in human lenses: 3-hydroxykynurenine glucoside formation. Vision Res. (1994) 34:1369–74. doi: 10.1016/0042-6989(94)90135-X
267. Tsentalovich YP, Verkhovod T, Yanshole VV, Kiryutin A, Yanshole LV, Fursova AZ, et al. Metabololmic composition of normal aged and cataractous human lenses. Exp Eye Res. (2015) 164:15–23. doi: 10.1016/j.exer.2015.03.008
268. Snytnikova OA, Fursova AZ, Chernyak EI, Vasiliev VG, Morozov SV, Kolosova NG, et al. Deaminated UV filter 3-hydroxykynurenine O-B-D-glucoside is found in cataractous human lenses. Exp Eye Res. (2008) 86:951–6. doi: 10.1016/j.exer.2008.03.013
269. Mizdrak J, Hains PG, Kalinowski D, Truscott RJW, Davies MJ, Jamie JF. Novel human lens metabolites from normal and cataractous human lenses. Tetrahedron. (2007) 63:4990–9. doi: 10.1016/j.tet.2007.03.133
Keywords: lens, UV light, cataract, UV-A, UV-B, oxidative stress, UV filter
Citation: MacFarlane ER, Donaldson PJ and Grey AC (2024) UV light and the ocular lens: a review of exposure models and resulting biomolecular changes. Front. Ophthalmol. 4:1414483. doi: 10.3389/fopht.2024.1414483
Received: 09 April 2024; Accepted: 12 August 2024;
Published: 05 September 2024.
Edited by:
Barbara Pierscionek, Anglia Ruskin University, United KingdomReviewed by:
Michael Wormstone, The University of Nottingham Ningbo, ChinaJohn I. Clark, University of Washington, United States
Copyright © 2024 MacFarlane, Donaldson and Grey. This is an open-access article distributed under the terms of the Creative Commons Attribution License (CC BY). The use, distribution or reproduction in other forums is permitted, provided the original author(s) and the copyright owner(s) are credited and that the original publication in this journal is cited, in accordance with accepted academic practice. No use, distribution or reproduction is permitted which does not comply with these terms.
*Correspondence: Angus C. Grey, YWMuZ3JleUBhdWNrbGFuZC5hYy5ueg==