- School of Optometry and Vision Science Program, Indiana University, Bloomington, IN, United States
The eye lens is a transparent, ellipsoid tissue in the anterior chamber that is required for the fine focusing of light onto the retina to transmit a clear image. The focusing function of the lens is tied to tissue transparency, refractive index, and biomechanical properties. The stiffness and elasticity or resilience of the human lens allows for shape changes during accommodation to focus light from objects near and far. It has long been hypothesized that changes in lens biomechanical properties with age lead to the loss of accommodative ability and the need for reading glasses with age. However, the cellular and molecular mechanisms that influence lens biomechanical properties and/or change with age remain unclear. Studies of lens stiffness and resilience in mouse models with genetic defects or at advanced age inform us of the cytoskeletal, structural, and morphometric parameters that are important for biomechanical stability. In this review, we will explore whether: 1) tissue level changes, including the capsule, lens volume, and nucleus volume, 2) cellular level alterations, including cell packing, suture organization, and complex membrane interdigitations, and 3) molecular scale modifications, including the F-actin and intermediate filament networks, protein modifications, lipids in the cell membrane, and hydrostatic pressure, influence overall lens biomechanical properties.
Introduction
The function of the eye lens to fine-focus light onto the retina depends on tissue transparency, high refractive index, and biomechanical integrity and resilience. In humans, the lens changes shape during a process called accommodation to focus light coming from different distances to transmit a clear image. Accommodation depends on the biomechanical properties of the lens, ciliary muscles, and zonular fibers. Previous work has studied several factors that may be involved in the age-related loss of accommodative amplitude, including ciliary muscle weakening, zonular fiber changes in elasticity, and increased lens stiffness. Studies have shown no age-related loss of zonular fiber elasticity (1, 2), and while there are age-related changes in the ciliary muscles and its connection to the peripheral tissues of the eye (1, 3, 4), the major contributing factor for presbyopia appears to be lens biomechanical properties (5). Age-related changes in lens stiffness and elasticity have been linked to the loss of accommodative amplitude leading to presbyopia and the need for reading glasses (6–17). There are many theories regarding how lens stiffness increases with age, but the cellular and molecular mechanisms that drive these biomechanical changes are just beginning to be understood.
The lens is surrounded by a thin collagenous basement membrane, known as the capsule, and is composed of two cell types, epithelial and fiber cells (Figure 1A). A monolayer of lens epithelial cells covers the anterior hemisphere of the tissue, and most of the lens consists of a large mass of fiber cells (18). Anterior quiescent epithelial cells are cuboidal in shape and cobblestone in cross section. These cells do not normally proliferate and are thought to help maintain the adjacent lens fiber cells. Epithelial cells at the lens equator in the germinative zone proliferate, migrate, and differentiate into fiber cells. The lifelong growth of the lens depends on the continuous and concentric addition of new fiber cell shells (19, 20). The oldest cells of the lens are in the inner portions of the tissue, and no cells are shed from the lens due to the lens capsule. Newly formed fiber cells elongate toward the anterior pole with their apical tips crawling along the apical surface of anterior epithelial cells while their basal tips migrate toward the posterior pole along the posterior lens capsule. When elongating fiber cell tips reach the anterior or posterior pole, the tips detach from the anterior epithelial cells or posterior capsule and contact fiber cell tips that extend from opposing sides of the tissue to form the suture (21–23). The suture solves the geometric problem that the fiber cells tips can never narrow enough to fully reach the point of the anterior and posterior poles (20). Fiber cells are long and skinny cells that are hexagonal in cross section with complex interdigitations forming a 3-dimensional zipper along the cell length (18, 24–27). The patterning of the interdigitations changes during fiber cell maturation and are hypothesized to affect lens biomechanical properties (28, 29). Fiber cells are supported by F-actin and specialized beaded intermediate filament networks, composed of CP49 and filensin (30–32). As fiber cells continue to mature, the cells undergo a process to remove all cellular organelles (18, 33) and are compacted toward the center of the tissue, also known as the lens nucleus, into a region of increased stiffness (6, 14, 18, 24, 34–36).
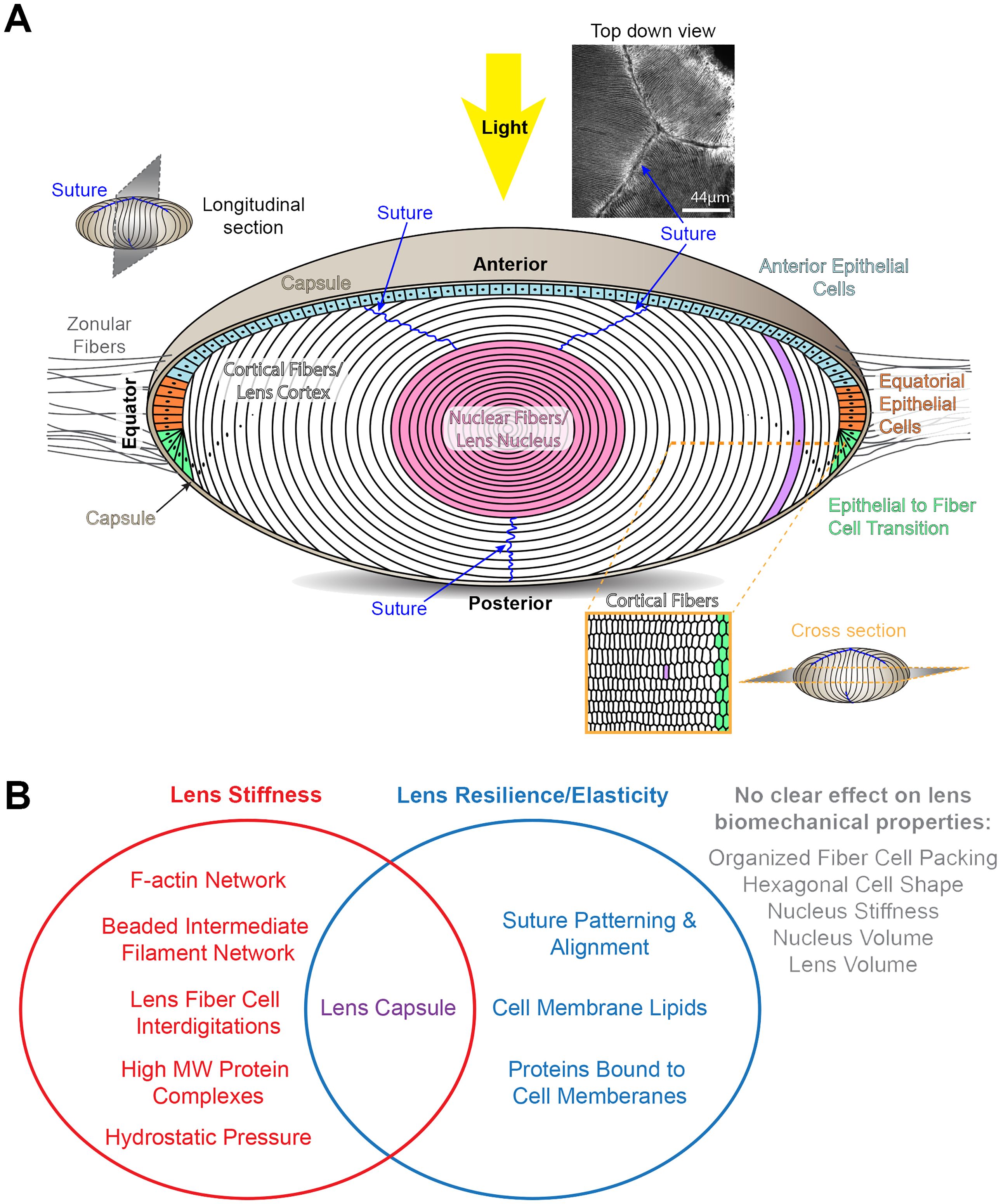
Figure 1 Diagrams of the structure of the lens and factors that influence lens biomechanical properties. (A) The cartoon of the lens shows a longitudinal plane. The lens is surrounded by a basement membrane called the capsule (tan). The lens is suspended in the anterior chamber of the eye by zonular fibers (gray lines) extending from the ciliary body that are anchored into the lens capsule. Light rays enter the lens from the anterior pole. Lens epithelial cells form a monolayer that covers the anterior hemisphere of the lens. The anterior epithelial cells (light blue) are quiescent. Equatorial epithelial cells (orange) proliferate, and one of the daughter cells (green) will start to differentiate into lens fibers cells. The bulk of the lens is made up of fiber cells (white and pink). These long and skinny cells are hexagonal in cross section (lower right cartoon). Newly formed fiber cells will elongate toward the anterior and posterior poles, and the tips of these fiber cells will form the suture (blue lines). A photo of the lens suture stained for F-actin to mark the cell boundaries is shown on the upper right. During fiber cell maturation, there is a process that removes all cellular organelles to prevent light scattering. Fiber cells are highly compacted at the center of the lens, also known as the lens nucleus (pink). Scale bar for suture picture, 44μm. The rest of the cartoons are not drawn to scale. (B) A Venn diagram summary of the tissue, cellular, and molecular factors that influence lens stiffness and/or resilience.
Many methods have been used to determine stiffness and elasticity in human lenses (6, 10, 12–15, 37–40) and animal lenses (34, 41–55). Although animal lenses do not accommodate like human lenses, animal lenses increase in stiffness with age in a similar manner (34, 35, 41, 43–45, 52–54). In addition, mouse models offer the ability to study age-related changes in a relatively shortened period of time with the average lifespan of laboratory mice being 26-29 months (56, 57). Genetic models of knockout, knock-in, and transgenic mice allow the dissection of pathways required for lens homeostasis and biomechanics. It should be noted that murine lenses are nearly spherical in shape compared to human lenses that are ellipsoid in shape, and rodent lenses have much stiffer lens nuclei compared to primate lenses. Structurally, human and mouse lens fibers share similar morphologies, interdigitations, and patterning, but human lenses have more complex sutures (21, 58). While studies of mouse models can offer insights into lens tissue mechanical properties, murine lenses do not accommodate like human lenses.
In this review, we will discuss the various factors that have been hypothesized to affect lens biomechanics and highlight data from human and animal studies that reveal tissue, cellular, and molecular scale changes that influence lens stiffness and resilience or elasticity (Figure 1B).
Tissue organization and integrity
Lens capsule
The capsule surrounding the lens is mainly composed of collagen IV, laminin, and perlecan (59–71), and this basement membrane prevents the shedding of lens cells during development and aging (18). The capsule shapes the lens during accommodation (72). With age, the human lens capsule increases in thickness, stiffens, becoming more brittle (12, 73–77). Additionally, measurements of biomechanical properties of decapsulated human and animal lenses show that removal of the lens capsule causes changes in lens shape (46), a decrease in stiffness (40, 50, 73), and altered viscoelastic properties (46). Based on lens capsule and whole lens stretching studies, in samples from older humans and primates, the stiffness of the lens capsule alone does not determine overall tissue stiffness and, most likely, does not contribute to presbyopia (78). In mouse lenses, the capsule increases in thickness up to 4 months of age, and the continued age-related increase in lens stiffness is not linked to capsule thickness (35). Measurements of human lens stiffness with a spinning method showed larger post-spinning equatorial diameters in decapsulated lenses than intact lenses (40), suggesting that the lens capsule plays a role in resilience of the lens to recover its shape after deformation. From these data, while the lens capsule is important for overall tissue biomechanics and shape, this basement membrane is unlikely to be the major factor in the age-related stiffening of the lens.
Lens volume, nucleus volume, and nucleus stiffness
It has been theorized that increased lens volume, nucleus volume, or nucleus stiffness with age lead to an overall increase in human lens stiffness with age, leading to presbyopia (6, 47, 79–81). In isolated fiber cells from the cortex or nucleus of sheep lenses, atomic force microscopy (AFM) showed that nuclear fibers were stiffer than cortical fibers (55). Our data in aging mice showed that the lens volume increases with age until the volume plateaus at 18 months of age (35). Lens stiffness in aged mice continues to increase up to 30 months of age, and thus, lens volume increases are unlikely to affect lens stiffness in old mice (35). In aged mice, the volume of the lens nucleus increases with age, and there is a large jump in lens nucleus volume between 24 months and 30 months of age without a similarly large jump in lens stiffness at 30 months of age (35). Genetic knockout or knockdown mouse models can lead to opposing changes in lens nucleus volume. Loss of EphA2, a receptor tyrosine kinase, results in a smaller and softer lens nucleus without any changes in overall lens stiffness (82, 83). In contrast, knockdown of tropomyosin 3.5 (Tpm3.5), an F-actin stabilizing protein, resulted in a larger and stiff lens nucleus, but these knockdown lenses were soft at high compressive loads (84). From these data, lens volume, nucleus volume, and nucleus stiffness are unlikely to play significant roles in overall lens stiffness, at least in rodent lenses. In human lenses, an alternate theory proposes that the restriction of cytoplasmic movement in the lens nuclear fibers can lead to increased lens stiffness and decreased accommodation with age (85). This idea is discussed below in the section on lens protein modifications with age.
The relationship between lens resilience or elasticity with lens and nucleus volumes is unclear. Lens resilience, measured by axial diameter recovery in 2- to 24-month-old mice after compression, was ~94-96% of the pre-compression axial diameter (35). Interestingly, in very old mice, lens resilience is nearly 99% (35). This jump in lens resilience is not correlated with lens volume since the lens stops increasing in volume by 18 months of age (35). The increased lens resilience in 30-month-old mice could be related to the significant increase in nucleus volume (35). Alternatively, the lenses from 30-month-old mice are very stiff and do not compress much; thus, these lenses recover more fully after load removal.
Cellular arrangement and packing
Hexagon cell shape and organized fiber cell packing
The correlation between hexagon cell shape and the organized packing of lens fiber cells on lens stiffness is weak. Studies of mouse models with a disturbance of cell membrane-associated proteins, periaxin and ankyrin-B, showed changes in fiber cell shape and decreased lens stiffness (86). These abnormal fibers appear polygonal but differ in width and height compared to the uniform cell size in control lens fibers (86–88). Further work revealed that changes in fiber cell hexagon cell shape and disorganization of fiber cell packing does not affect lens stiffness. Loss of tropomodulin 1 (Tmod1), an actin pointed-end capping protein, in the lens only leads to a mild change in lens stiffness at low compressive loads (41, 89). The loss of EphA2 or mutations in myosin IIA (NMIIA) lead to obvious defects in fiber cell packing and hexagon cell shape, but no changes in lens stiffness (82, 90–92). Further, in aged mice, there is a loss of hexagon cell shape, uniform fiber cell size, and organized fiber cell packing, but lenses from the very old mice are very stiff. Thus, the collective evidence suggests that organized fiber cell packing and hexagon cell shape does not affect lens stiffness. It is likely that decreased levels of cytoskeletal proteins in homozygous periaxin knockout and heterozygous ankyrin-B knockout lenses lead to changes in stiffness rather than just simply a change in cell shape, as discussed below.
In the mutant mouse models described above with disordered lens fibers, resilience is increased in very old mice and in EphA2 knockout lenses. The increased lens resilience in very old mice happens suddenly at 30 months of age, but the hexagonal cell shape changes are apparent by 12 months of age. Thus, in this case, it is unlikely that ordered fiber cell packing affects lens resilience. For the EphA2 knockout lenses, the reason for increased resilience is due to the lens suture changes discussed in detail below.
Lens suture
The lens suture structure differs between non-accommodating and accommodating species. Typically, in non-accommodating mouse lenses, there are well-aligned Y-shape sutures with 3 branches, and there are a low percentage of wild-type lenses that exhibit sutures with additional branches (42, 82). Each layer of the Y-suture in mouse lenses is overlaid precisely on previously shells of lens fiber cells (42, 82). In contrast, in accommodating species, lens sutures begin as a 3-pronged Y shape and continue to branch with the addition of new layers of lens fibers (21, 58). Studies of the EphA2 and ephrin-A5 knockout mice have revealed the role of the Y-suture in lens resilience. Ephrin-A5 is part of a family of ligand proteins that bind to Eph receptors. Loss of either EphA2 or ephrin-A5 result in misaligned shells of lens fiber cells that have abnormal lens suture branching (82, 93). There are no changes in lens stiffness in either EphA2 or ephrin-A5 knockout lenses, but there is increased lens resilience, and knockout lenses recovered more completely after load removal (82). This change in lens resilience is not related to disorganized fiber cell packing because the ephrin-A5 knockout lenses have increased resilience with normal, organized, and hexagonal lens fiber cells (94, 95). Thus, this data indicates that Y-shaped sutures in mouse lenses constrain the resilience of the tissue. It should be noted that these biomechanical studies compressed the lenses with a high load that resulted in higher than physiological strains on the lens. When control and knockout lenses were compressed to physiological strains, both groups of samples recovered completely after load removal.
Lens fiber cell interdigitations
It has long been hypothesized that the complex interdigitations that create a 3D zipper between fibers cells is required for lens biomechanical properties (28, 29). This elegant pattern of interlocking membrane structures changes during fiber cell differentiation and maturation, and has been characterized extensively by electron microscopy (24–29, 35, 83, 84, 96–107). During lens fiber cell maturation, the loss of cellular organelles is accompanied by the appearance of large interlocking paddle domains between neighboring cells (83, 84, 96–98). In Tmod1 knockout lenses, which are softer at low mechanical loads (41), the large paddle domains do not form between these knockout fiber cells (96). Tmod1 interacts with the spectrin-actin network and is normally associated with β2-spectrin at the fiber cell membrane in the valleys between large paddle domains. Loss of Tmod1 causes dissociation of β2-spectrin from the cell membrane and abnormal distribution of α-actinin, a crosslinking protein for antiparallel actin filaments, along the cell membrane (89). This is the first direct evidence that fiber cell interdigitations influence lens stiffness. There is no change in lens resilience in these knockout lenses, and it remains unknown whether fiber cells interdigitations play a significant role in lens elasticity.
Molecular level alterations
The F-actin network and associated proteins
The F-actin network plays a crucial role in lens fiber cell shape and patterning [reviewed in (30)]. When Tpm3.5 is knocked down in mouse lenses, there is a significant decrease in stiffness at high compressive loads (84). Electron microscopy and single fiber cell staining studies reveal no obvious changes in fiber cell interdigitations, but the decrease in Tpm3.5 at the fiber cell membrane causes a reorganization of the F-actin network. While the protein levels remained unchanged, there was an expansion of the β2-spectrin- and α-actinin-associated F-actin networks at the cell membrane displacing the fimbrin, also known as plastin, bundled F-actin network. The alteration in the type of F-actin network at the fiber cell membrane is correlated with decreased stiffness of the knockdown lens.
Several studies have looked at the effects of actin polymerization, myosin motor domain, and myosin light chain kinase inhibition on lens stiffness. Treatment of chick lenses ex vivo with F-actin and myosin disruptors, including latrunculin, blebbistatin, and ML-7, resulted in softer lenses under whole tissue compression tests, and the effects of treatments were reversible (108). The stiffness changes were accompanied by a decrease in the levels of F-actin and phosphorylated myosin along with dissociation of F-actin and myosin from the basal surface of fiber cells. Presumably, shorter actin filaments or disruption of myosin motor activities leads to the softening of treated lenses. In contrast, AFM studies of sheep lens fibers treated with cytochalasin D, an inhibitor of F-actin polymerization, or nocodazole, an inhibitor of microtubule polymerization, showed no changes in local stiffness of the fiber cells (55). Since the lenses were flash frozen, stored at -80°C, and thawed before cell dissociation with chelating agents, the cells were presumably no longer metabolically active. Thus, the use of cytoskeletal inhibitors in this study may have little effect on these thawed cells.
Interestingly, recent studies of transgenic mice with human mutations in NMIIA showed no change in lens stiffness (91, 92). One mutation was in the motor domain of NMIIA, and two mutations were in the tail domain. It should be noted that the motor domain mutation and one of the tail domain mutations are homozygous embryonic lethal, and therefore, in the heterozygous transgenic mice, compensation by the endogenous wild-type protein may affect the biomechanical testing results.
Reports of softer lenses due to loss of periaxin or decreased levels of ankyrin-B are accompanied by decreased protein levels of β-actin, β2-spectrin, desmoyokin, NrCam, activated myosin, and calcium channels as well as changes in the localization of membrane cytoskeleton proteins in immunostained lens fibers (86, 87). The changes in fiber cell shape and width are likely downstream of the loss of these membrane proteins that are required for normal cell architecture. These data support the notion that lens stiffness depends on a normal F-actin network.
The role of the F-actin network in lens resilience is unclear. While loss of Tmod1 slightly alters lens stiffness at low compressive strains, the loss of Tmod1 and mutations in NMIIA do not affect lens resilience (41, 91, 92). The Tpm3.5 knockdown lenses have decreased lens resilience, but this is likely due to high strains on the softer knockdown lenses that are unable to recover fully after load removal.
Beaded intermediate filaments
Lens fiber cells are supported by a specialized beaded intermediate filament network, composed of two proteins, CP49 (also known as phakinin) and filensin [reviewed in (32)]. Initially, the “beaded” structure of these intermediate filaments was erroneously attributed to the binding of α-crystallin proteins (109, 110). There is a spontaneous mutation in CP49 in the 129 mouse strain that leads to loss of CP49 (111, 112). Deletion of either CP49 or filensin causes complete loss of the beaded intermediate filaments in the lens (112–115). CP49 knockout lenses are softer than control lenses (41, 43). The contribution of CP49 to lens diameter and formation of the lens nucleus is unclear. One report suggests loss of CP49 leads to decreased lens diameters and nucleus diameters (43) while another study shows no difference in lens volumes and nuclear volumes when compared to control lenses (41). Both studies showed that resilience of lenses without CP49 is comparable to control lenses (41, 43). Thus, the loss of beaded intermediate filaments leading to softer lenses without a change in lens elasticity is presumably due to weakening of the fiber cell cytoskeleton. The conflicting reports of lens and nucleus diameter and volume changes in mice with CP49 disruption are not clearly linked to the change in lens stiffness.
Protein modifications and crosslinking
For transparency and high refractive index, lens cells contain an extraordinarily high amount of proteins (~450mg/ml), and 90% of all lens proteins are crystallins (18). Crystallins are divided into three families in humans, α, β, and γ. Since there is little or no protein turnover and no loss or renewal of cells in this tissue, the proteins made during initial embryonic development are present in the nuclear fiber cells at the center of the lens for an entire lifetime. There have been many studies showing post-translational modification, glycation, and aggregation of crystallins with age. Alpha-crystallins, members of the small heat shock protein family, have been shown to have chaperone-like activity and act as sinks to sequester misfolded or unfolded proteins to prevent protein aggregation (116, 117). The other major classes of crystallins belong to the family of β/γ crystallins that function as structural proteins that bind calcium in the lens (116, 118–121). By 35-45 years of age in human lenses, there is significant loss of α-crystallin proteins from the soluble protein fractions in the lens nucleus, and at the same time, there is an increase of insoluble high molecular protein species (7, 122, 123). This change in protein solubility coincides with the typical onset age for presbyopia, and it is hypothesized that the increased size and stiffness of high molecular weight protein species may affect the ability for the lens nucleus to deform during accommodation (124), supporting the theory that the cytoplasm of aged nuclear fibers has restricted movement (85).
During aging, human and mouse lenses have increased levels of advanced glycation end products (AGEs) (125–133). Studies of mouse lenses after thermal stress revealed changes in lens stiffness that were correlated with increased AGEs (132, 134). Treatment of mouse lenses under thermal stress with a plant flavanone, hesperetin, prevents the increase in AGEs and the increase in lens stiffness. However, heating the tissue may cause other types of damage to lead to the change in lens stiffness. In addition to glycation, lysine acylation is a common type of protein modification. In the lens, changes in lysine have been detected as one of the major post-translational protein modifications (135–137). Human and mouse lenses treated in vitro and in vivo with aggrelyte-2 had reduced lens stiffness and increased water-soluble proteins, presumably through protein acetylation and breaking of disulfide bonds (138, 139). Many other post-translational modifications of crystallins have been described in aging lenses, including phosphorylation, deamindation, methylation, racemization, isomerization, lipidation, oxidation, and truncation [reviewed in (140–143)]. Studies have largely linked these protein changes to cataracts. Presumably, any protein modifications that results in disulfide bond formation or crosslinking can lead to changes in lens stiffness, but there have not yet been any direct experiments to address the possible changes in biomechanics due to specific post-translational protein modifications.
The strategy to break disulfide protein bonds to reverse presbyopia has previously been explored through the application of α-lipoic acid to animal and human lenses. There was encouraging data from old animal and in vitro human studies that this agent could soften lenses (144–146). Unfortunately, the formulation of α-lipoic acid UNR844 being developed by Novartis failed in phase 2b clinical trials, and this potential treatment for presbyopia has been abandoned (147).
Lipids in the cell membrane
The lipid content of human lens fiber cell membranes changes with age until about 40-45 years of age (148, 149), coinciding with the onset of presbyopia. Cholesterol content influences cell membrane rigidity, and lens fiber cell membranes have unusually high levels of cholesterol (150–154). High cholesterol contents lead to the formation of cholesterol bilayer domains in lens fiber cell membranes (155). AFM studies suggest that cholesterol content in the fiber cell membranes regulates cell elasticity (156). Age-related changes in the cholesterol species in fiber cell membranes (150) and the binding of α-crystallins to the cell membrane with age (157–163) are thought to lower lens deformability with age (164).
Hydrostatic pressure and osmotic balance
Hydrostatic pressure is maintained in the lens by a network of sodium channels, gap junctions composed of connexins, and water channels composed aquaporins (165). There is a steady increase in hydrostatic pressure in the lens with age (166). Loss of connexin 46 (Cx46 or α3) leads to stiffening of knockout lenses possibly due to protein degradation and nuclear cataracts (48). It is worth noting that heterozygous Cx46 knockout lenses have elevated hydrostatic pressure (165), and therefore, it is also possible that the loss of Cx46 causes increased lens stiffness due to the disruption of the gap junctions’ ability to regulate lens hydrostatic pressure. Water channels, made of aquaporin 0, are abundant in the lens fiber cells and are thought to facilitate intercellular water transport in the lens cortex (167–169). Aqp0 is known to be cleaved during fiber cell differentiation leading to a loss of water channel function, and cleaved Aqp0 is thought to function as cell-cell adhesion molecules in mature fiber cells (170–173). Heterozygous and homozygous Aqp0 knockout lenses demonstrate changes in lens fiber cell shape, enlarged extracellular spaces, and these mutant lenses are softer than control lenses (45). Thus, the loss of Aqp0 in lenses can lead to changes in lens stiffness due to altered hydrostatic pressure and changes in fiber cell structure and stability (45, 174).
The hydrostatic pressure in the lens may be influenced by the ratio of bound vs. free water in the lens. Water is bound to the abundant amount of crystallin proteins in the lens, and during pressure changes, which can occur during accommodation, bound water is released from proteins resulting in an increase of free water in the lens, and thus decreasing the osmotic pressure (175–178). This process is known as syneresis. The ratio of free-to-bound water decreases with age leading to increased bound water and increased internal hydrostatic pressure as accommodative ability decreases (179–181). The change in free water content in the aging lens has been proposed as a possible contributor to lens stiffening with age and cataract formation (182).
Concluding thoughts
Studies of animal and human lenses have revealed that lens stiffness is influenced by the capsule, complex interdigitations between fiber cells, the F-actin and beaded intermediate filament networks, age-related protein modifications, hydrostatic pressure, and osmotic balance. Lens elasticity is linked to the capsule, suture alignment, and the lipid and protein composition of fiber cell membranes. Surprisingly, animal lens studies do not show a clear link between lens volume, nucleus volume, nucleus stiffness, or organized fiber cell packing with overall lens biomechanical properties (Figure 1B). A common factor in regulating lens stiffness and elasticity is the integrity of the capsule. This important basement membrane is required to hold all lens cells together and provides the anchoring point for zonular fibers. While the contribution of the capsule to the development of presbyopia remains unclear, the contribution of various extracellular matrix components of the lens capsule to tissue biomechanics remains to be studied.
Lens fiber cell interdigitations are affected by cytoskeletal protein complexes, and both factors are required for normal lens stiffness. The mechanism for how fiber cell morphology is modulated by cytoskeletal proteins is unknown, and the signaling pathways required for normal fiber cell interdigitation development are still being studied. Protein modifications affect protein-protein interactions that in turn modulate osmotic balance and hydrostatic pressure. Changes in this balance lead to altered lens stiffness, and protein-protein disulfide bonds are now a target for pharmaceutical intervention for preventing, delaying, or even reversing presbyopia.
The patterning of the lens suture affects tissue elasticity likely though a change in the distribution of forces in lenses with branched sutures. These data support the known differences between accommodating and non-accommodating species where accommodating and more elastic lenses have high branched sutures. The age-related change in composition of the lipids and proteins bound to the fiber cell membrane suggests that the fluidity of cell membranes also influences lens resilience. The interconnected nature of tissue, cellular, and molecular level alterations that affect lens biomechanical properties with age suggest that strategies to prevent or reverse presbyopia may need to target multiple factors that influence the stiffening of the lens.
Funding
The author(s) declare financial support was received for the research, authorship, and/or publication of this article. This work was supported by the National Eye Institute Grant R01 EY032056 to CC.
Acknowledgments
The author thanks Michael P. Vu for helpful comments and critical reading of this manuscript.
Conflict of interest
The author declares that the research was conducted in the absence of any commercial or financial relationships that could be construed as a potential conflict of interest.
The author(s) declared that they were an editorial board member of Frontiers, at the time of submission. This had no impact on the peer review process and the final decision.
Publisher’s note
All claims expressed in this article are solely those of the authors and do not necessarily represent those of their affiliated organizations, or those of the publisher, the editors and the reviewers. Any product that may be evaluated in this article, or claim that may be made by its manufacturer, is not guaranteed or endorsed by the publisher.
References
1. van Alphen GW, Graebel WP. Elasticity of tissues involved in accommodation. Vision Res. (1991) 31:1417–38. doi: 10.1016/0042-6989(91)90061-9
2. Fisher RF. The ciliary body in accommodation. Trans Ophthalmol Soc U K (1962). (1986) 105:208–19.
3. Graebel WP, van Alphen GWHM. The elasticity of sclera and choroid of the human eye, and its implications on scleral rigidity and accommodation. J Biomechanical Engineering. (1977) 99:203–8. doi: 10.1115/1.3426291
4. Tamm S, Tamm E, Rohen JW. Age-related changes of the human ciliary muscle. A quantitative morphometric study. Mech Ageing Dev. (1992) 62:209–21. doi: 10.1016/0047-6374(92)90057-K
5. Beers APA, van der Heijde GL. Age-related changes in the accommodation mechanism. Optometry Vision Science. (1996) 73:235–42. doi: 10.1097/00006324-199604000-00004
6. Heys KR, Cram SL, Truscott RJ. Massive increase in the stiffness of the human lens nucleus with age: the basis for presbyopia? Mol Vis. (2004) 10:956–63.
7. Heys KR, Friedrich MG, Truscott RJ. Presbyopia and heat: changes associated with aging of the human lens suggest a functional role for the small heat shock protein, alpha-crystallin, in maintaining lens flexibility. Aging Cell. (2007) 6:807–15. doi: 10.1111/j.1474-9726.2007.00342.x
8. Glasser A, Campbell MC. Biometric, optical and physical changes in the isolated human crystalline lens with age in relation to presbyopia. Vision Res. (1999) 39:1991–2015. doi: 10.1016/S0042-6989(98)00283-1
9. Weeber HA, Eckert G, Soergel F, Meyer CH, Pechhold W, van der Heijde RG. Dynamic mechanical properties of human lenses. Exp Eye Res. (2005) 80:425–34. doi: 10.1016/j.exer.2004.10.010
10. Weeber HA, van der Heijde RG. On the relationship between lens stiffness and accommodative amplitude. Exp Eye Res. (2007) 85:602–7. doi: 10.1016/j.exer.2007.07.012
11. Pierscionek BK. Age-related response of human lenses to stretching forces. Exp Eye Res. (1995) 60:325–32. doi: 10.1016/S0014-4835(05)80114-9
12. Fisher RF. Elastic properties of the human lens. Exp Eye Res. (1971) 11:143. doi: 10.1016/S0014-4835(71)80086-6
13. Burd HJ, Wilde GS, Judge SJ. An improved spinning lens test to determine the stiffness of the human lens. Exp Eye Res. (2011) 92:28–39. doi: 10.1016/j.exer.2010.10.010
14. Pau H, Kranz J. The increasing sclerosis of the human lens with age and its relevance to accommodation and presbyopia. Graefes Arch Clin Exp Ophthalmol. (1991) 229:294–6. doi: 10.1007/BF00167888
15. Schachar RA, Chan RW, Fu M. Viscoelastic properties of fresh human lenses under 40 years of age: implications for the aetiology of presbyopia. Br J Ophthalmol. (2011) 95:1010–3. doi: 10.1136/bjo.2011.202895
16. Wang K, Pierscionek BK. Biomechanics of the human lens and accommodative system: Functional relevance to physiological states. Prog Retin Eye Res. (2019) 71:114–31. doi: 10.1016/j.preteyeres.2018.11.004
17. Wilkes RP, Reilly MA. A pre-tensioned finite element model of ocular accommodation and presbyopia. Int J Adv Eng Sci Appl Mathematics. (2016) 8:25–38. doi: 10.1007/s12572-015-0141-2
18. Lovicu FJ, Robinson ML. Development of the Ocular Lens. Cambridge, England: Cambridge University Press (2011).
19. Kuszak JR. The ultrastructure of epithelial and fiber cells in the crystalline lens. Int Rev Cytol. (1995) 163:305–50. doi: 10.1016/S0074-7696(08)62213-5
20. Bassnett S, Winzenburger PA. Morphometric analysis of fibre cell growth in the developing chicken lens. Exp Eye Res. (2003) 76:291–302. doi: 10.1016/S0014-4835(02)00315-9
21. Kuszak JR. The development of lens sutures. Prog Retinal Eye Res. (1995) 14:567–91. doi: 10.1016/1350-9462(94)00019-C
22. Kuszak JR, Zoltoski RK, Tiedemann CE. Development of lens sutures. Int J Dev Biol. (2004) 48:889–902. doi: 10.1387/ijdb.041880jk
23. Kuszak JR, Mazurkiewicz M, Zoltoski R. Computer modeling of secondary fiber development and growth: I. Nonprimate lenses. Mol Vis. (2006) 12:251–70.
24. Kuwabara T. The maturation of the lens cell: a morphologic study. Exp Eye Res. (1975) 20:427–43. doi: 10.1016/0014-4835(75)90085-8
25. Kistler J, Gilbert K, Brooks HV, Jolly RD, Hopcroft DH, Bullivant S. Membrane interlocking domains in the lens. Invest Ophthalmol Vis Sci. (1986) 27:1527–34.
26. Dickson DH, Crock GW. Interlocking patterns on primate lens fibers. Invest Ophthalmol. (1972) 11:809–15.
27. Willekens B, Vrensen G. The three-dimensional organization of lens fibers in the rhesus monkey. Graefes Arch Clin Exp Ophthalmol. (1982) 219:112–20. doi: 10.1007/BF02152295
28. Biswas SK, Lee JE, Brako L, Jiang JX, Lo WK. Gap junctions are selectively associated with interlocking ball-and-sockets but not protrusions in the lens. Mol Vis. (2010) 16:2328–41.
29. Lo WK, Biswas SK, Brako L, Shiels A, Gu S, Jiang JX. Aquaporin-0 targets interlocking domains to control the integrity and transparency of the eye lens. Invest Ophthalmol Vis Sci. (2014) 55:1202–12. doi: 10.1167/iovs.13-13379
30. Cheng C, Nowak RB, Fowler VM. The lens actin filament cytoskeleton: Diverse structures for complex functions. Exp Eye Res. (2017) 156:58–71. doi: 10.1016/j.exer.2016.03.005
31. Song S, Landsbury A, Dahm R, Liu Y, Zhang Q, Quinlan RA. Functions of the intermediate filament cytoskeleton in the eye lens. J Clin Invest. (2009) 119:1837–48. doi: 10.1172/JCI38277
32. FitzGerald PG. Lens intermediate filaments. Exp Eye Res. (2009) 88:165–72. doi: 10.1016/j.exer.2008.11.007
34. Cheng C, Gokhin DS, Nowak RB, Fowler VM. Sequential application of glass coverslips to assess the compressive stiffness of the mouse lens: strain and morphometric analyses. J Vis Exp. (2016) 111. doi: 10.3791/53986
35. Cheng C, Parreno J, Nowak RB, Biswas SK, Wang K, Hoshino M, et al. Age-related changes in eye lens biomechanics, morphology, refractive index and transparency. Aging (Albany NY). (2019) 11:12497–531. doi: 10.18632/aging.v11i24
36. Al-Ghoul KJ, Nordgren RK, Kuszak AJ, Freel CD, Costello MJ, Kuszak JR. Structural evidence of human nuclear fiber compaction as a function of ageing and cataractogenesis. Exp Eye Res. (2001) 72:199–214. doi: 10.1006/exer.2000.0937
37. Krueger RR, Sun XK, Stroh J, Myers R. Experimental increase in accommodative potential after neodymium: yttrium-aluminum-garnet laser photodisruption of paired cadaver lenses. Ophthalmology. (2001) 108:2122–9. doi: 10.1016/S0161-6420(01)00834-X
38. Glasser A, Campbell MC. Presbyopia and the optical changes in the human crystalline lens with age. Vision Res. (1998) 38:209–29. doi: 10.1016/S0042-6989(97)00102-8
39. Hollman KW, O'Donnell M, Erpelding TN. Mapping elasticity in human lenses using bubble-based acoustic radiation force. Exp Eye Res. (2007) 85:890–3. doi: 10.1016/j.exer.2007.09.006
40. Wilde GS, Burd HJ, Judge SJ. Shear modulus data for the human lens determined from a spinning lens test. Exp Eye Res. (2012) 97:36–48. doi: 10.1016/j.exer.2012.01.011
41. Gokhin DS, Nowak RB, Kim NE, Arnett EE, Chen AC, Sah RL, et al. Tmod1 and CP49 synergize to control the fiber cell geometry, transparency, and mechanical stiffness of the mouse lens. PloS One. (2012) 7:e48734. doi: 10.1371/journal.pone.0048734
42. Parreno J, Cheng C, Nowak RB, Fowler VM. The effects of mechanical strain on mouse eye lens capsule and cellular microstructure. Mol Biol Cell. (2018) 29:1963–74. doi: 10.1091/mbc.E18-01-0035
43. Fudge DS, McCuaig JV, Van Stralen S, Hess JF, Wang H, Mathias RT, et al. Intermediate filaments regulate tissue size and stiffness in the murine lens. Invest Ophthalmol Vis Sci. (2011) 52:3860–7. doi: 10.1167/iovs.10-6231
44. Baradia H, Nikahd N, Glasser A. Mouse lens stiffness measurements. Exp Eye Res. (2010) 91:300–7. doi: 10.1016/j.exer.2010.06.003
45. Sindhu Kumari S, Gupta N, Shiels A, FitzGerald PG, Menon AG, Mathias RT, et al. Role of Aquaporin 0 in lens biomechanics. Biochem Biophys Res Commun. (2015) 462:339–45. doi: 10.1016/j.bbrc.2015.04.138
46. Mekonnen T, Zevallos-Delgado C, Zhang H, Singh M, Aglyamov SR, Larin KV. The lens capsule significantly affects the viscoelastic properties of the lens as quantified by optical coherence elastography. Front Bioeng Biotechnol. (2023) 11:1134086. doi: 10.3389/fbioe.2023.1134086
47. Scarcelli G, Kim P, Yun SH. In vivo measurement of age-related stiffening in the crystalline lens by Brillouin optical microscopy. Biophys J. (2011) 101:1539–45. doi: 10.1016/j.bpj.2011.08.008
48. Stopka W, Libby T, Lin S, Wang E, Xia CH, Gong X. Age-related changes of lens stiffness in wild-type and Cx46 knockout mice. Exp Eye Res. (2021) 212:108777. doi: 10.1016/j.exer.2021.108777
49. Reilly MA, Brian R, Hamilton Paul D, Shen Amy Q, Nathan R. Material characterization of porcine lenticular soluble proteins. Biomacromolecules. (2008) 9:1519–26. doi: 10.1021/bm701229t
50. Reilly M, Ravi N. Microindentation of the young porcine ocular lens. J Biomech Eng. (2009) 131:044502. doi: 10.1115/1.3072891
51. Candia OA, Zamudio AC, Alvarez LJ. Mechanical stretching forces oppose osmotic lens swelling. Exp Eye Res. (2010) 91:472–4. doi: 10.1016/j.exer.2010.06.002
52. Sharma PK, Busscher HJ, Terwee T, Koopmans SA, van Kooten TG. A comparative study on the viscoelastic properties of human and animal lenses. Exp Eye Res. (2011) 93:681–8. doi: 10.1016/j.exer.2011.08.009
53. Zhang X, Wang Q, Lyu Z, Gao X, Zhang P, Lin H, et al. Noninvasive assessment of age-related stiffness of crystalline lenses in a rabbit model using ultrasound elastography. BioMed Eng Online. (2018) 17:75. doi: 10.1186/s12938-018-0509-1
54. Wu C, Han Z, Wang S, Li J, Singh M, Liu CH, et al. Assessing age-related changes in the biomechanical properties of rabbit lens using a coaligned ultrasound and optical coherence elastography system. Invest Ophthalmol Vis Sci. (2015) 56:1292–300. doi: 10.1167/iovs.14-15654
55. Hozic A, Rico F, Colom A, Buzhynskyy N, Scheuring S. Nanomechanical characterization of the stiffness of eye lens cells: a pilot study. Invest Ophthalmol Vis Sci. (2012) 53:2151–6. doi: 10.1167/iovs.11-8676
56. Flurkey K, Currer J, Harrison D. The Mouse in Aging Research. Cambridge, Massachusetts, United States: Academic Press (2007). doi: 10.1016/B978-012369454-6/50074-1
57. Kunstyr I, Leuenberger HG. Gerontological data of C57BL/6J mice. I. Sex differences in survival curves. J Gerontol. (1975) 30:157–62. doi: 10.1093/geronj/30.2.157
58. Koretz JF, Cook CA, Kuszak JR. The zones of discontinuity in the human lens: development and distribution with age. Vision Res. (1994) 34:2955–62. doi: 10.1016/0042-6989(94)90267-4
59. Mohan PS, Spiro RG. Macromolecular organization of basement membranes. Characterization and comparison of glomerular basement membrane and lens capsule components by immunochemical and lectin affinity procedures. J Biol Chem. (1986) 261:4328–36. doi: 10.1016/S0021-9258(17)35665-X
60. Danysh BP, Duncan MK. The lens capsule. Exp Eye Res. (2009) 88:151–64. doi: 10.1016/j.exer.2008.08.002
61. Parmigiani C, McAvoy J. Localisation of laminin and fibronectin during rat lens morphogenesis. Differentiation. (1984) 28:53–61. doi: 10.1111/j.1432-0436.1984.tb00266.x
62. Cummings CF, Hudson BG. Lens capsule as a model to study type IV collagen. Connect Tissue Res. (2014) 55:8–12. doi: 10.3109/03008207.2013.867337
63. Kelley PB, Sado Y, Duncan MK. Collagen IV in the developing lens capsule. Matrix Biol. (2002) 21:415–23. doi: 10.1016/S0945-053X(02)00014-8
64. Barnard K, Burgess SA, Carter DA, Woolley DM. Three-dimensional structure of type IV collagen in the mammalian lens capsule. J Struct Biol. (1992) 108:6–13. doi: 10.1016/1047-8477(92)90002-R
65. Wu W, Tholozan FM, Goldberg MW, Bowen L, Wu J, Quinlan RA. A gradient of matrix-bound FGF-2 and perlecan is available to lens epithelial cells. Exp Eye Res. (2014) 120:10–4. doi: 10.1016/j.exer.2013.12.004
66. Bystrom B, Virtanen I, Rousselle P, Gullberg D, Pedrosa-Domellof F. Distribution of laminins in the developing human eye. Invest Ophthalmol Vis Sci. (2006) 47:777–85. doi: 10.1167/iovs.05-0367
67. Cammarata PR, Cantu-Crouch D, Oakford L, Morrill A. Macromolecular organization of bovine lens capsule. Tissue Cell. (1986) 18:83–97. doi: 10.1016/0040-8166(86)90009-1
68. Lee J, Gross JM. Laminin beta1 and gamma1 containing laminins are essential for basement membrane integrity in the zebrafish eye. Invest Ophthalmol Vis Sci. (2007) 48:2483–90. doi: 10.1167/iovs.06-1211
69. Kohno T, Sorgente N, Ishibashi T, Goodnight R, Ryan SJ. Immunofluorescent studies of fibronectin and laminin in the human eye. Invest Ophthalmol Vis Sci. (1987) 28:506–14.
70. Silver PH, Wakely J. The initial stage in the development of the lens capsule in chick and mouse embryos. Exp Eye Res. (1974) 19:73–7. doi: 10.1016/0014-4835(74)90074-8
71. DeDreu J, Walker JL, Menko AS. Dynamics of the lens basement membrane capsule and its interaction with connective tissue-like extracapsular matrix proteins. Matrix Biol. (2021) 96:18–46. doi: 10.1016/j.matbio.2020.12.005
73. Fisher RF. Elastic constants of the human lens capsule. J Physiol. (1969) 201:1–19. doi: 10.1113/jphysiol.1969.sp008739
74. Fisher RF, Pettet BE. The postnatal growth of the capsule of the human crystalline lens. J Anat. (1972) 112:207–14.
75. Krag S, Olsen T, Andreassen TT. Biomechanical characteristics of the human anterior lens capsule in relation to age. Invest Ophthalmol Vis Sci. (1997) 38:357–63.
76. Krag S, Andreassen TT. Mechanical properties of the human posterior lens capsule. Invest Ophthalmol Vis Sci. (2003) 44:691–6. doi: 10.1167/iovs.02-0096
77. Krag S, Andreassen TT. Mechanical properties of the human lens capsule. Prog Retin Eye Res. (2003) 22:749–67. doi: 10.1016/S1350-9462(03)00063-6
78. Ziebarth NM, Borja D, Arrieta E, Aly M, Manns F, Dortonne I, et al. Role of the lens capsule on the mechanical accommodative response in a lens stretcher. Invest Ophthalmol Vis Sci. (2008) 49:4490–6. doi: 10.1167/iovs.07-1647
79. Zhou H, Yan H, Yan W, Wang X, Li Q. In vivo ultraosound elastographic evaluation of the age-related change of human lens nuclear stiffness. BMC Ophthalmol. (2020) 20:135. doi: 10.1186/s12886-020-01404-1
80. Hermans E, Dubbelman M, van der Heijde R, Heethaar R. The shape of the human lens nucleus with accommodation. J Vis. (2007) 7:16 1–0. doi: 10.1167/7.10.16
81. Fisher RF, Pettet BE. Presbyopia and the water content of the human crystalline lens. J Physiol. (1973) 234:443–7. doi: 10.1113/jphysiol.1973.sp010353
82. Cheng C. EphA2 and ephrin-A5 guide eye lens suture alignment and influence whole lens resilience. Invest Ophthalmol Vis Sci. (2021) 62:3. doi: 10.1167/iovs.62.15.3
83. Cheng C, Wang K, Hoshino M, Uesugi K, Yagi N, Pierscionek B. EphA2 affects development of the eye lens nucleus and the gradient of refractive index. Invest Ophthalmol Vis Sci. (2022) 63:2. doi: 10.1167/iovs.63.1.2
84. Cheng C, Nowak RB, Amadeo MB, Biswas SK, Lo WK, Fowler VM. Tropomyosin 3.5 protects the F-actin networks required for tissue biomechanical properties. J Cell Sci. (2018) 131. doi: 10.1242/jcs.222042
85. Koretz JF, Handelman GH, Brown NP. Analysis of human crystalline lens curvature as a function of accommodative state and age. Vision Res. (1984) 24:1141–51. doi: 10.1016/0042-6989(84)90168-8
86. Maddala R, Walters M, Brophy PJ, Bennett V, Rao PV. Ankyrin-B directs membrane tethering of periaxin and is required for maintenance of lens fiber cell hexagonal shape and mechanics. Am J Physiol Cell Physiol. (2016) 310:C115–26. doi: 10.1152/ajpcell.00111.2015
87. Maddala R, Skiba NP, Lalane R 3rd, Sherman DL, Brophy PJ, Rao PV. Periaxin is required for hexagonal geometry and membrane organization of mature lens fibers. Dev Biol. (2011) 357:179–90. doi: 10.1016/j.ydbio.2011.06.036
88. Rao PV, Maddala R. Ankyrin-B in lens architecture and biomechanics: Just not tethering but more. Bioarchitecture. (2016) 6:39–45. doi: 10.1080/19490992.2016.1156284
89. Nowak RB, Fowler VM. Tropomodulin 1 constrains fiber cell geometry during elongation and maturation in the lens cortex. J Histochem Cytochem. (2012) 60:414–27. doi: 10.1369/0022155412440881
90. Cheng C, Ansari MM, Cooper JA, Gong X. EphA2 and Src regulate equatorial cell morphogenesis during lens development. Development. (2013) 140:4237–45. doi: 10.1242/dev.100727
91. Islam ST, Cheng C, Parreno J, Fowler VM. Nonmuscle myosin IIA regulates the precise alignment of hexagonal eye lens epithelial cells during fiber cell formation and differentiation. Invest Ophthalmol Vis Sci. (2023) 64:20. doi: 10.1167/iovs.64.4.20
92. Islam ST, Cheheltani S, Cheng C, Fowler VM. Disease-related non-muscle myosin IIA D1424N rod domain mutation, but not R702C motor domain mutation, disrupts mouse ocular lens fiber cell alignment and hexagonal packing. Cytoskeleton (Hoboken). (2024) 1–17. doi: 10.1002/cm.21853
93. Zhou Y, Shiels A. Epha2 and Efna5 participate in lens cell pattern-formation. Differentiation. (2018) 102:1–9. doi: 10.1016/j.diff.2018.05.002
94. Cheng C, Gong X. Diverse roles of Eph/ephrin signaling in the mouse lens. PloS One. (2011) 6:e28147. doi: 10.1371/journal.pone.0028147
95. Cheng C, Fowler VM, Gong X. EphA2 and ephrin-A5 are not a receptor-ligand pair in the ocular lens. Exp Eye Res. (2017) 162:9–17. doi: 10.1016/j.exer.2017.06.016
96. Cheng C, Nowak RB, Biswas SK, Lo WK, FitzGerald PG, Fowler VM. Tropomodulin 1 regulation of actin is required for the formation of large paddle protrusions between mature lens fiber cells. Invest Ophthalmol Vis Sci. (2016) 57:4084–99. doi: 10.1167/iovs.16-19949
97. Vu MP, Cheng C. Preparation and immunofluorescence staining of bundles and single fiber cells from the cortex and nucleus of the eye lens. J Vis Exp. (2023) 196. doi: 10.3791/65638
98. Blankenship T, Bradshaw L, Shibata B, Fitzgerald P. Structural specializations emerging late in mouse lens fiber cell differentiation. Invest Ophthalmol Vis Sci. (2007) 48:3269–76. doi: 10.1167/iovs.07-0109
99. Willekens B, Vrensen G. The three-dimensional organization of lens fibers in the rabbit. A scanning electron microscopic reinvestigation. Albrecht Von Graefes Arch Klin Exp Ophthalmol. (1981) 216:275–89. doi: 10.1007/BF00455035
100. Kuszak J, Alcala J, Maisel H. The surface morphology of embryonic and adult chick lens-fiber cells. Am J Anat. (1980) 159:395–410. doi: 10.1002/aja.1001590406
101. Willekens B, Vrensen G. Lens fiber organization in four avian species: a scanning electron microscopic study. Tissue Cell. (1985) 17:359–77. doi: 10.1016/0040-8166(85)90055-2
102. Lo WK, Harding CV. Square arrays and their role in ridge formation in human lens fibers. J Ultrastruct Res. (1984) 86:228–45. doi: 10.1016/S0022-5320(84)90103-5
103. Lo WK, Reese TS. Multiple structural types of gap junctions in mouse lens. J Cell Sci. (1993) 106:227–35. doi: 10.1242/jcs.106.1.227
104. Harding CV, Susan S, Murphy H. Scanning electron microscopy of the adult rabbit lens. Ophthalmic Res. (2009) 8:443–55. doi: 10.1159/000264853
105. Leeson TS. Lens of the rat eye: an electron microscope and freeze-etch study. Exp Eye Res. (1971) 11:78–82. doi: 10.1016/S0014-4835(71)80067-2
106. Zhou CJ, Lo WK. Association of clathrin, AP-2 adaptor and actin cytoskeleton with developing interlocking membrane domains of lens fibre cells. Exp Eye Res. (2003) 77:423–32. doi: 10.1016/S0014-4835(03)00171-4
107. Kuszak JR, Macsai MS, Rae JL. Stereo scanning electron microscopy of the crystalline lens. Scan Electron Microsc. (1983) 1983:1415–26.
108. Won GJ, Fudge DS, Choh V. The effects of actomyosin disruptors on the mechanical integrity of the avian crystalline lens. Mol Vis. (2015) 21:98–109.
109. Ireland M, Maisel H. A cytoskeletal protein unique to lens fiber cell differentiation. Exp Eye Res. (1984) 38:637–45. doi: 10.1016/0014-4835(84)90182-9
110. Bloemendal H, Berbers GA, De Jong WW, Ramaekers FC, Vermorken AJ, Dunia I, et al. Interaction of crystallins with the cytoskeletal-plasma membrane complex of the bovine lens. Ciba Found Symp. (1984) 106:177–90. doi: 10.1002/9780470720875.ch10
111. Alizadeh A, Clark J, Seeberger T, Hess J, Blankenship T, FitzGerald PG. Characterization of a mutation in the lens-specific CP49 in the 129 strain of mouse. Invest Ophthalmol Vis Sci. (2004) 45:884–91. doi: 10.1167/iovs.03-0677
112. Sandilands A, Wang X, Hutcheson AM, James J, Prescott AR, Wegener A, et al. Bfsp2 mutation found in mouse 129 strains causes the loss of CP49' and induces vimentin-dependent changes in the lens fibre cell cytoskeleton. Exp Eye Res. (2004) 78:875–89. doi: 10.1016/j.exer.2003.09.028
113. Alizadeh A, Clark JI, Seeberger T, Hess J, Blankenship T, Spicer A, et al. Targeted genomic deletion of the lens-specific intermediate filament protein CP49. Invest Ophthalmol Vis Sci. (2002) 43:3722–7. doi: 10.1167/iovs.03-0677
114. Alizadeh A, Clark J, Seeberger T, Hess J, Blankenship T, FitzGerald PG. Targeted deletion of the lens fiber cell-specific intermediate filament protein filensin. Invest Ophthalmol Vis Sci. (2003) 44:5252–8. doi: 10.1167/iovs.03-0224
115. Sandilands A, Prescott AR, Wegener A, Zoltoski RK, Hutcheson AM, Masaki S, et al. Knockout of the intermediate filament protein CP49 destabilises the lens fibre cell cytoskeleton and decreases lens optical quality, but does not induce cataract. Exp Eye Res. (2003) 76:385–91. doi: 10.1016/S0014-4835(02)00330-5
116. Bloemendal H, de Jong W, Jaenicke R, Lubsen NH, Slingsby C, Tardieu A. Ageing and vision: structure, stability and function of lens crystallins. Prog Biophys Mol Biol. (2004) 86:407–85. doi: 10.1016/j.pbiomolbio.2003.11.012
118. Rajini B, Shridas P, Sundari CS, Muralidhar D, Chandani S, Thomas F, et al. Calcium binding properties of gamma-crystallin: calcium ion binds at the Greek key beta gamma-crystallin fold. J Biol Chem. (2001) 276:38464–71. doi: 10.1074/jbc.M102164200
119. Srivastava SS, Mishra A, Krishnan B, Sharma Y. Ca2+-binding motif of betagamma-crystallins. J Biol Chem. (2014) 289:10958–66. doi: 10.1074/jbc.O113.539569
120. Jobby MK, Sharma Y. Calcium-binding to lens betaB2- and betaA3-crystallins suggests that all beta-crystallins are calcium-binding proteins. FEBS J. (2007) 274:4135–47. doi: 10.1111/j.1742-4658.2007.05941.x
121. Aravind P, Mishra A, Suman SK, Jobby MK, Sankaranarayanan R, Sharma Y. The betagamma-crystallin superfamily contains a universal motif for binding calcium. Biochemistry. (2009) 48:12180–90. doi: 10.1021/bi9017076
122. Pierscionek BK, Chan DY, Ennis JP, Smith G, Augusteyn RC. Nondestructive method of constructing three-dimensional gradient index models for crystalline lenses: I. Theory and experiment. Am J Optom Physiol Opt. (1988) 65:481–91. doi: 10.1097/00006324-198806000-00008
123. McFall-Ngai MJ, Ding LL, Takemoto LJ, Horwitz J. Spatial and temporal mapping of the age-related changes in human lens crystallins. Exp Eye Res. (1985) 41:745–58. doi: 10.1016/0014-4835(85)90183-6
124. Bron AJ, Vrensen GF, Koretz J, Maraini G, Harding JJ. The ageing lens. Ophthalmologica. (2000) 214:86–104. doi: 10.1159/000027475
125. Smuda M, Henning C, Raghavan CT, Johar K, Vasavada AR, Nagaraj RH, et al. Comprehensive analysis of maillard protein modifications in human lenses: effect of age and cataract. Biochemistry. (2015) 54:2500–7. doi: 10.1021/bi5013194
126. Biemel KM, Friedl DA, Lederer MO. Identification and quantification of major maillard cross-links in human serum albumin and lens protein. Evidence for glucosepane as the dominant compound. J Biol Chem. (2002) 277:24907–15. doi: 10.1074/jbc.M202681200
127. Cheng R, Feng Q, Argirov OK, Ortwerth BJ. K2P–a novel cross-link from human lens protein. Ann N Y Acad Sci. (2005) 1043:184–94. doi: 10.1196/annals.1333.023
128. Lyons TJ, Silvestri G, Dunn JA, Dyer DG, Baynes JW. Role of glycation in modification of lens crystallins in diabetic and nondiabetic senile cataracts. Diabetes. (1991) 40:1010–5. doi: 10.2337/diab.40.8.1010
129. Nagaraj RH, Sell DR, Prabhakaram M, Ortwerth BJ, Monnier VM. High correlation between pentosidine protein crosslinks and pigmentation implicates ascorbate oxidation in human lens senescence and cataractogenesis. Proc Natl Acad Sci U.S.A. (1991) 88:10257–61. doi: 10.1073/pnas.88.22.10257
130. Tessier F, Obrenovich M, Monnier VM. Structure and mechanism of formation of human lens fluorophore LM-1. Relationship to vesperlysine A and the advanced Maillard reaction in aging, diabetes, and cataractogenesis. J Biol Chem. (1999) 274:20796–804. doi: 10.1074/jbc.274.30.20796
131. Nagaraj RH, Linetsky M, Stitt AW. The pathogenic role of Maillard reaction in the aging eye. Amino Acids. (2012) 42:1205–20. doi: 10.1007/s00726-010-0778-x
132. Doki Y, Nakazawa Y, Morishita N, Endo S, Nagai N, Yamamoto N, et al. Hesperetin treatment attenuates glycation of lens proteins and advanced−glycation end products generation. Mol Med Rep. (2023) 27. doi: 10.3892/mmr
133. Rankenberg J, Rakete S, Wagner BD, Patnaik JL, Henning C, Lynch A, et al. Advanced glycation end products in human diabetic lens capsules. Exp Eye Res. (2021) 210:108704. doi: 10.1016/j.exer.2021.108704
134. Nandi SK, Nahomi RB, Rankenberg J, Glomb MA, Nagaraj RH. Glycation-mediated inter-protein cross-linking is promoted by chaperone-client complexes of alpha-crystallin: Implications for lens aging and presbyopia. J Biol Chem. (2020) 295:5701–16. doi: 10.1074/jbc.RA120.012604
135. Nagaraj RH, Nahomi RB, Shanthakumar S, Linetsky M, Padmanabha S, Pasupuleti N, et al. Acetylation of alphaA-crystallin in the human lens: effects on structure and chaperone function. Biochim Biophys Acta. (2012) 1822:120–9. doi: 10.1016/j.bbadis.2011.11.011
136. Nahomi RB, Nandi SK, Rakete S, Michel C, Fritz KS, Nagaraj RH. Lysine malonylation and propionylation are prevalent in human lens proteins. Exp Eye Res. (2020) 190:107864. doi: 10.1016/j.exer.2019.107864
137. Nandi SK, Rakete S, Nahomi RB, Michel C, Dunbar A, Fritz KS, et al. Succinylation is a gain-of-function modification in human lens alphaB-crystallin. Biochemistry. (2019) 58:1260–74. doi: 10.1021/acs.biochem.8b01053
138. Panja S, Nahomi RB, Rankenberg J, Michel CR, Gaikwad H, Nam MH, et al. Aggrelyte-2 promotes protein solubility and decreases lens stiffness through lysine acetylation and disulfide reduction: Implications for treating presbyopia. Aging Cell. (2023) 22:e13797. doi: 10.1111/acel.13797
139. Panja S, Nam M-H, Gaikwad H, Rankenberg J, Nagaraj RH. Topical ocular application of aggrelyte-2A reduces lens stiffness in mice. Front Ophthalmol. (2023) 3. doi: 10.3389/fopht.2023.1274825
140. Schey KL, Wang Z, Friedrich MG, Garland DL, Truscott RJW. Spatiotemporal changes in the human lens proteome: Critical insights into long-lived proteins. Prog Retin Eye Res. (2020) 76:100802. doi: 10.1016/j.preteyeres.2019.100802
141. Sharma KK, Santhoshkumar P. Lens aging: effects of crystallins. Biochim Biophys Acta. (2009) 1790:1095–108. doi: 10.1016/j.bbagen.2009.05.008
142. Ray NJ. Biophysical chemistry of the ageing eye lens. Biophys Rev. (2015) 7:353–68. doi: 10.1007/s12551-015-0176-4
143. Quinlan RA, Clark JI. Insights into the biochemical and biophysical mechanisms mediating the longevity of the transparent optics of the eye lens. J Biol Chem. (2022) 298:102537. doi: 10.1016/j.jbc.2022.102537
144. Garner WH, Garner MH. Protein disulfide levels and lens elasticity modulation: applications for presbyopia. Invest Ophthalmol Vis Sci. (2016) 57:2851–63. doi: 10.1167/iovs.15-18413
145. Korenfeld MS, Robertson SM, Stein JM, Evans DG, Rauchman SH, Sall KN, et al. Topical lipoic acid choline ester eye drop for improvement of near visual acuity in subjects with presbyopia: a safety and preliminary efficacy trial. Eye (Lond). (2021) 35:3292–301. doi: 10.1038/s41433-020-01391-z
146. Zhang H, Singh M, Nair A, Larin KV, Aglyamov SR. Elasticity changes in the crystalline lens during oxidative damage and the antioxidant effect of alpha-lipoic acid measured by optical coherence elastography. Photonics. (2021) 8:207. doi: 10.3390/photonics8060207
147. Novartis maintains growth momentum and confirms FY’22 Group guidance(2022). Available online at: https://www.novartis.com/news/media-releases/novartis-maintains-growth-momentum-and-confirms-fy22-group-guidance.
148. Ostrin LA, Glasser A. Accommodation measurements in a prepresbyopic and presbyopic population. J Cataract Refract Surg. (2004) 30:1435–44. doi: 10.1016/j.jcrs.2003.12.045
149. Weale RA. Evolution, age and ocular focus. Mech Ageing Dev. (1990) 53:85–9. doi: 10.1016/0047-6374(90)90036-F
150. Duindam JJ, Vrensen GF, Otto C, Greve J. Aging affects the conformation of cholesterol in the human eye lens. Ophthalmic Res. (1996) 28 Suppl 1:86–91. doi: 10.1159/000267978
151. Li LK, So L, Spector A. Age-dependent changes in the distribution and concentration of human lens cholesterol and phospholipids. Biochim Biophys Acta. (1987) 917:112–20. doi: 10.1016/0005-2760(87)90291-8
152. Mainali L, Raguz M, O'Brien WJ, Subczynski WK. Changes in the properties and organization of human lens lipid membranes occurring with age. Curr Eye Res. (2017) 42:721–31. doi: 10.1080/02713683.2016.1231325
153. Truscott RJ. Age-related nuclear cataract: a lens transport problem. Ophthalmic Res. (2000) 32:185–94. doi: 10.1159/000055612
154. Widomska J, Subczynski WK. Why is very high cholesterol content beneficial for the eye lens but negative for other organs? Nutrients. (2019) 11. doi: 10.3390/nu11051083
155. Subczynski WK, Raguz M, Widomska J, Mainali L, Konovalov A. Functions of cholesterol and the cholesterol bilayer domain specific to the fiber-cell plasma membrane of the eye lens. J Membr Biol. (2012) 245:51–68. doi: 10.1007/s00232-011-9412-4
156. Khadka NK, Mortimer MF, Marosvari M, Timsina R, Mainali L. Membrane elasticity modulated by cholesterol in model of porcine eye lens-lipid membrane. Exp Eye Res. (2022) 220:109131. doi: 10.1016/j.exer.2022.109131
157. Maraini G, Fasella P. Reversible binding of soluble lens proteins to lens fibre ghosts. Exp Eye Res. (1970) 10:133–9. doi: 10.1016/S0014-4835(70)80019-7
158. Bracchi PG, Carta F, Fasella P, Maraini G. Selective binding of aged alpha-crystallin to lens fibre ghosts. Exp Eye Res. (1971) 12:151–4. doi: 10.1016/0014-4835(71)90140-0
159. Broekhuyse RM, Kuhlmann ED. Lens membranes. IV. Preparative isolation and characterization of membranes and various membrane proteins from calf lens. Exp Eye Res. (1978) 26:305–20. doi: 10.1016/0014-4835(78)90077-5
160. Friedrich MG, Truscott RJ. Large-scale binding of alpha-crystallin to cell membranes of aged normal human lenses: a phenomenon that can be induced by mild thermal stress. Invest Ophthalmol Vis Sci. (2010) 51:5145–52. doi: 10.1167/iovs.10-5261
161. Timsina R, Mainali L. Association of alpha-crystallin with fiber cell plasma membrane of the eye lens accompanied by light scattering and cataract formation. Membranes (Basel). (2021) 11. doi: 10.3390/membranes11060447
162. Timsina R, Trossi-Torres G, O'Dell M, Khadka NK, Mainali L. Cholesterol and cholesterol bilayer domains inhibit binding of alpha-crystallin to the membranes made of the major phospholipids of eye lens fiber cell plasma membranes. Exp Eye Res. (2021) 206:108544. doi: 10.1016/j.exer.2021.108544
163. Timsina R, Khadka NK, Maldonado D, Mainali L. Interaction of alpha-crystallin with four major phospholipids of eye lens membranes. Exp Eye Res. (2021) 202:108337. doi: 10.1016/j.exer.2020.108337
164. Khadka NK, Timsina R, Mainali L. An AFM Approach Applied in a Study of alpha-Crystallin Membrane Association: New Insights into Lens Hardening and Presbyopia Development. Membranes (Basel). (2022) 12. doi: 10.3390/membranes12050522
165. Gao J, Sun X, Moore LC, White TW, Brink PR, Mathias RT. Lens intracellular hydrostatic pressure is generated by the circulation of sodium and modulated by gap junction coupling. J Gen Physiol. (2011) 137:507–20. doi: 10.1085/jgp.201010538
166. Gao J, Wang H, Sun X, Varadaraj K, Li L, White TW, et al. The effects of age on lens transport. Invest Ophthalmol Vis Sci. (2013) 54:7174–87. doi: 10.1167/iovs.13-12593
167. Grey AC, Li L, Jacobs MD, Schey KL, Donaldson PJ. Differentiation-dependent modification and subcellular distribution of aquaporin-0 suggests multiple functional roles in the rat lens. Differentiation. (2009) 77:70–83. doi: 10.1016/j.diff.2008.09.003
168. Petrova RS, Webb KF, Vaghefi E, Walker K, Schey KL, Donaldson PJ. Dynamic functional contribution of the water channel AQP5 to the water permeability of peripheral lens fiber cells. Am J Physiol Cell Physiol. (2018) 314:C191–201. doi: 10.1152/ajpcell.00214.2017
169. Schey KL, Gletten RB, O'Neale CVT, Wang Z, Petrova RS, Donaldson PJ. Lens aquaporins in health and disease: location is everything! Front Physiol. (2022) 13:882550. doi: 10.1152/ajpcell.00214.2017
170. Michea LF, Andrinolo D, Ceppi H, Lagos N. Biochemical evidence for adhesion-promoting role of major intrinsic protein isolated from both normal and cataractous human lenses. Exp Eye Res. (1995) 61:293–301. doi: 10.1016/S0014-4835(05)80124-1
171. Zampighi GA, Hall JE, Ehring GR, Simon SA. The structural organization and protein composition of lens fiber junctions. J Cell Biol. (1989) 108:2255–75. doi: 10.1083/jcb.108.6.2255
172. Liu J, Xu J, Gu S, Nicholson BJ, Jiang JX. Aquaporin 0 enhances gap junction coupling via its cell adhesion function and interaction with connexin 50. J Cell Sci. (2011) 124:198–206. doi: 10.1242/jcs.072652
173. Palanivelu DV, Kozono DE, Engel A, Suda K, Lustig A, Agre P, et al. Co-axial association of recombinant eye lens aquaporin-0 observed in loosely packed 3D crystals. J Mol Biol. (2006) 355:605–11. doi: 10.1016/j.jmb.2005.10.032
174. Al-Ghoul KJ, Kirk T, Kuszak AJ, Zoltoski RK, Shiels A, Kuszak JR. Lens structure in MIP-deficient mice. Anat Rec A Discovery Mol Cell Evol Biol. (2003) 273:714–30. doi: 10.1002/ar.a.10080
175. Bettelheim FA. Syneretic response to pressure in ocular lens. J Theor Biol. (1999) 197:277–80. doi: 10.1006/jtbi.1998.0855
176. Bettelheim FA, Lizak MJ, Zigler JS Jr. Syneretic response of aging normal human lens to pressure. Invest Ophthalmol Vis Sci. (2003) 44:258–63. doi: 10.1167/iovs.02-0422
177. Bettelheim FA, Zigler JS Jr. Pressure-induced syneretic response in rhesus monkey lenses. Invest Ophthalmol Vis Sci. (1999) 40:1285–8.
178. Lizak MJ, Zigler JS Jr., Bettelheim FA. Syneretic response to incremental pressures in calf lenses. Curr Eye Res. (2005) 30:21–5. doi: 10.1080/02713680490894216
179. Heys KR, Friedrich MG, Truscott RJ. Free and bound water in normal and cataractous human lenses. Invest Ophthalmol Vis Sci. (2008) 49:1991–7. doi: 10.1167/iovs.07-1151
180. Lie AL, Pan X, White TW, Vaghefi E, Donaldson PJ. Age-dependent changes in total and free water content of in vivo human lenses measured by magnetic resonance imaging. Invest Ophthalmol Vis Sci. (2021) 62:33. doi: 10.1167/iovs.62.9.33
181. Lie AL, Pan X, Vaghefi E, White TW, Donaldson PJ. Alterations in lens free water distribution are associated with shape deformation in accommodation. Ophthalmol Sci. (2024) 4:100404. doi: 10.1016/j.xops.2023.100404
Keywords: actin, Eph, ephrin, CP49, interdigitations, capsule, suture
Citation: Cheng C (2024) Tissue, cellular, and molecular level determinants for eye lens stiffness and elasticity. Front. Ophthalmol. 4:1456474. doi: 10.3389/fopht.2024.1456474
Received: 28 June 2024; Accepted: 26 July 2024;
Published: 08 August 2024.
Edited by:
Ram H. Nagaraj, University of Colorado Denver, United StatesReviewed by:
Janice Walker, Thomas Jefferson University, United StatesXingjun Fan, Augusta University, United States
Copyright © 2024 Cheng. This is an open-access article distributed under the terms of the Creative Commons Attribution License (CC BY). The use, distribution or reproduction in other forums is permitted, provided the original author(s) and the copyright owner(s) are credited and that the original publication in this journal is cited, in accordance with accepted academic practice. No use, distribution or reproduction is permitted which does not comply with these terms.
*Correspondence: Catherine Cheng, Y2tjaGVuZ0BpdS5lZHU=