- 1Phantom-g, CICECO-Aveiro Institute of Materials, Physics Department, University of Aveiro, Aveiro, Portugal
- 2Department of Molecular Sciences and Nanosystems, Ca’ Foscari University of Venice, Venice, Italy
The design of molecular materials suitable for disparate fields could lead to new advances in engineering applications. In this work, a series of Ln3+-doped BiF3 sub-microparticles were synthesized through microwave-assisted synthesis. The effects of doping are evaluated from the structural and morphological viewpoint. In general, increasing the Ln3+ concentration the octahedral habitus is distorted to a spheric one, and some aggregates are visible without any differences in the crystalline phase. The optical response of the samples confirms that the BiF3 materials are suitable hosts for the luminescence of the tested trivalent lanthanide (Ln3+) ions (Ln = Eu, Tb, Tm, Ho, Er, Yb). A Yb3+/Er3+ co-doped sample is presented as an illustrative example of all-photonic molecular logic operations and primary luminescent thermometry.
Introduction
The development of synthesis routes allowing the design of materials with virtually on-demand properties (e.g., size, shape, functionality) is pivotal for cutting-edge applications in disparate research areas. It is well known that the morphology and size of the materials can impact their physical and chemical properties (Piana et al., 2005; Alivisatos, 1996; Xia et al., 2003), making engineered materials suitable for a wide range of applications. Trivalent lanthanide (Ln3+)-bearing materials have been widely investigated due to their distinctive high quantum yield (>50% in the visible spectral range, when incorporated in complexes), long-lived excited states (>1 ms), narrow emission bands (<10 nm), rendering versatility, and photostability, when incorporated in inorganic or organic-inorganic hosts (Bünzli, 2015). Ln3+-based materials attracted huge scientific scrutiny during the last 25 years for many optical applications from the development of solid-state lasers (Kuriki et al., 2002), lightning (Bai et al., 2014), displays (Chen et al., 2013), and, in the last 10–15 years to luminescence nanothermometry (Jaque and Vetrone, 2012; Brites et al., 2019).
A relatively recent and sagacious application for Ln3+-doped materials is molecular logic, which aims at developing molecular counterparts for current electronic logic systems (De Silva et al., 1993). Any physical and/or chemical change caused by an external stimulus (logic inputs) leading to a physical and/or chemical alteration of the material (logic output), may be interpreted as a transfer function connecting the logic inputs and outputs. Several materials have been designed to mimic the operation of conventional Boolean logic gates, demonstrating basic arithmetic functions and memory units (Erbas-Cakmak et al., 2018; Andréasson and Pischel, 2021; Nicoli et al., 2021). Converting the response of the luminescent material to the logical values 0 and 1, diverse logic gates can be defined. Molecular logic operations comprising chemical species as logic inputs and modifications on the photophysical properties (e.g., emission intensity, absorbance) as the output are those reported most often. Nevertheless, physical stimuli (e.g., light, temperature, pressure, magnetic field) may play the role of logic inputs, with many advantages such as no-contamination, reuse of the device, easy reconfiguration, and reprogramming (Andreasson et al., 2011; Brites et al., 2019; Zanella et al., 2022).
Irrespectively of the foreseen applications, to render tailorable photoluminescence response of the Ln3+-doped materials, the host material should be precisely designed. Ideally, the host material would not interfere with the luminescence processes, therefore, host materials with low phonon energy are desirable. To date, fluorides, such as NaYF4 based materials have been widely investigated as luminescent host materials and remarkable results have been reported. NaYF4 has been used as a host in different contexts such as solar cells (Kumar et al., 2020), biodetection (Yi et al., 2004), detecting fingerprints (Xie et al., 2015), and display (Deng et al., 2015). As an alternative to the well-known NaYF4, Bi-based matrices are an appealing candidate to host Ln3+ ions. In particular, bismuth-based fluoride nanoparticles have been proposed for multi-imaging and phosphor applications (Back et al., 2019), while bismuth oxyhalides of general formula BiOX (X = F, Cl, Br, I) have been studied due to their chemical stability, high refractive index and photocatalytic properties (Wang et al., 2020b). Furthermore, bismuth-based compounds have been exploited in different fields such as luminescent thermometry (Back et al., 2020b; Casagrande et al., 2020), water splitting (Kato et al., 2017), fuel cells (Sanna et al., 2015), UV filtering (Zaccariello et al., 2019) and cosmetics production (Zaccariello et al., 2017). There are several synthetic routes to obtain sub-microparticles and nanoparticles of Bi-based systems with different shapes and morphology. One of these is the microwave-assisted synthesis, developed in the 80’s (Gedye et al., 1986), that exploits microwave radiation with frequencies between 0.3 and 300 GHz as the heating source (Nüchter et al., 2004). As the microwave energy is not enough to break the chemical bonds, it increases the Brownian motion and consequently the temperature. During the reaction, the heat is produced by the collisions of the particles, or by the electric dipole of molecules. Several advantages are ascribed to this synthetic method such as the high chemical yield, reproducibility and homogeneity of the synthesis, possible application in green chemistry, and contactless with the heating source (Dąbrowska et al., 2018). Therefore, sub-micro Bi-based fluoride and oxyfluoride particles can be easily synthesized through the microwave approach, with the possibility to obtain a high yield in powder (Escudero et al., 2014).
Here, we present the synthesis, structural and photophysical characterization of Ln3+ -doped (Ln = Yb, Er, Tm, Eu, Tb) bismuth fluoride (BiF3) sub-micro particles prepared through a microwave-assisted synthesis, demonstrating that several Ln3+ ions can be efficiently hosted. The Er3+-Yb3+ co-doped sample was further explored for molecular logics and luminescent thermometry applications as it presents different emission profiles depending on the power density of the excitation laser. These results open a new path for all-photonic reprogrammable materials that are simultaneously primary thermometers, dismissing the need for recurrent thermal calibrations, and thus enabling the in-situ real-time monitoring of the temperature.
Experimental details
Chemical and materials
Bismuth nitrate pentahydrate (Bi(NO3)3?5H2O, Sigma ≥99.99%), sodium tetrafluoroborate (NaBF4, Across Organic, >97%) were used as bismuth and fluoride sources, respectively. Europium (III) nitrate pentahydrate (Eu(NO3)3·5H2O, Sigma Aldrich, 99.9%), terbium (III) nitrate pentahydrate (Tb(NO3)3∙5H2O, Sigma Aldrich, ≥99.9%), ytterbium (III) nitrate pentahydrate (Yb(NO3)3∙5H2O, Sigma Aldrich, ≥99.9%), erbium (III) nitrate pentahydrate (Er(NO3)3∙5H2O, Sigma Aldrich, ≥99.9%), thulium (III) nitrate pentahydrate (Tm(NO3)3∙5H2O, Sigma Aldrich, ≥99.9%), terbium (III) nitrate pentahydrate (Tb(NO3)3∙5H2O, Sigma Aldrich, ≥99.9%), holmium (III) nitrate pentahydrate (Ho(NO3)3∙5H2O, Sigma Aldrich, ≥99.9%) were used as Ln3+ precursors. Diethylene glycol (DEG, Sigma Aldrich, ≥99.0%), and MilliQ water were used as solvents. Ethanol (C2H5OH, VWR Chemicals 100%) was used in the washing process.
Synthesis of the sub-micro particles
The experimental protocol already reported elsewhere (Escudero et al., 2014) was adopted to produce oxyfluoride and fluoride bismuth materials. In short, pentahydrate bismuth nitrate, Bi(NO3)3∙5H2O, is dissolved in 24 ml of diethylene glycol at 348 K for 10 minutes under stirring and then the solution is cooled to room temperature. In the doping procedure, Ln3+ cations are dissolved in diethylene glycol together with the Bi precursor, with sodium tetrafluoroborate, NaBF4 in 6 ml of MilliQ water. These two solutions are then mixed under stirring and the resulting solution is placed in a Teflon-lined autoclave. The reaction occurs in a microwave digestion system (Ethos Plus Microwave, Milestone) at 393 K for 30 s, at 1000 W power. Afterward, the autoclave is quenched in an ice bath for 15 min. The opalescent solution obtained is separated by centrifugation (6,000 rpm) and the white precipitate is washed twice in ethanol and once in MilliQ water. The sample is dried overnight at room temperature and then characterized. The stoichiometry among reagents, temperature, and reaction time have been previously arranged to obtain octahedral sub-micro particles, as detailed in Supplementary Table S1.
Characterization
Structural and morphological characterization: X-ray powder diffraction (XRPD) measurements were performed on a diffractometer (Philips X’Pert) with a goniometer in the Bragg−Brentano geometry (PW 1319), connected to a highly stabilized X-ray source (Nickel-filtered Cu-Kα radiation), a focusing graphite monochromator, and a proportional counter with a pulse height discriminator. A step-by-step technique with collection times of 10 s per step (steps of 0.05° in 2θ units) was employed.
The size, morphology, and EDX analysis of the sub-micro particles were carried out by a field emission scanning electron microscope (FE-SEM, Carl Zeiss Sigma VP) equipped with a microanalysis detector (Bruker Quantax 200). The EDX spectra were collected at 4–5 kV for all the samples. A second SEM equipment (JEOL JSM-5600LV) with a microanalysis system (OXFORD-Link Isis series 300) was used to perform the morphological analysis.
Photoluminescence characterization: The emission spectra of BiF3:Ln3+ (Ln = Eu, Tb, Yb, Er) were recorded with a modular double grating excitation spectrofluorometer with an emission monochromator (TRIAX 320, Fluorolog-3, Horiba Scientific) coupled to a photomultiplier (R928 Hamamatsu) operating in the visible spectral range, using a front face configuration. A 450 W Xe arc lamp was used as the excitation source for the Eu3+-Tb3+ single-doped and co-doped samples. Both recorded emission and excitation spectra were corrected with the spectrofluorimeter optical spectral response and the spectral distribution of the lamp intensity using a photodiode reference detector, respectively. For recording the temperature-dependent upconversion spectra of BiF3:Yb3+/Er3+ sample, a continuous-wave 980 nm laser diode (BrixX 980-1000 HD, Omicron Laser) focused through a NIR optical lens (Thorlabs, 12 cm focal distance) was used as the excitation source. The temperature was controlled using a temperature controller (IES-RD31) equipped with a Kapton thermofoil heater (Minco) mounted on a Cu-holder. The temperature was recorded using a thermocouple (Barnant 100 model 600-2820) with an accuracy of 0.1 K, accordingly to the manufacturer.
The emission spectra of Yb-Ln3+ (Ln = Tm, Ho) were acquired at Ca’ Foscari University of Venice in the 250–1,050 nm range using a portable spectrometer (QE65 Pro, Ocean Optics), and a continuous-wave laser diode peaking at 980 nm (CNI MDL-III-980) as the excitation source. The laser diode output power was 2 W, distributed on a 5 × 8 mm2 spot, corresponding to a mean power density of 5 W cm−2.
Luminescence thermometry
The temperature (T) was accessed by the intensity ratio
where
and
where δΔ/Δ is the relative uncertainty in Δ estimated here for each temperature using the signal to noise ratio of the corresponding recorded emission spectrum.
Results and discussion
Synthesis, structure and morphology of the Ln3+-doped BiF3 particles
The mechanism of formation of the sub-micro particles is not fully understood since the microwave-assisted reaction takes place very quickly (Escudero et al., 2014). Figures 1A–F shows the surface morphology of BiF3 sub-micro particles Ln3+-doped and undoped obtained by microwave synthesis. It is assumed that spheres are initially formed which then evolve into octahedral shapes (Figures 1A–D), with an average size between 300 and 600 nm (Supplementary Figures S1A,B). As reported in the literature (Escudero et al., 2014), octahedral sub-micro particles are formed in a short time (30 s) as soon as the temperature reaches the set maximum (393 K). Smaller dimensions could be obtained using different molar ratios among reagents. For example, using a higher NaBF4 concentration (Supplementary Table S1), resulted in a smaller size, around 130–150 nm (Supplementary Figure S2A). The decrease in the average size of the octahedral sub-micro particles is probably due to the largest number of nuclei involved during the reaction process.
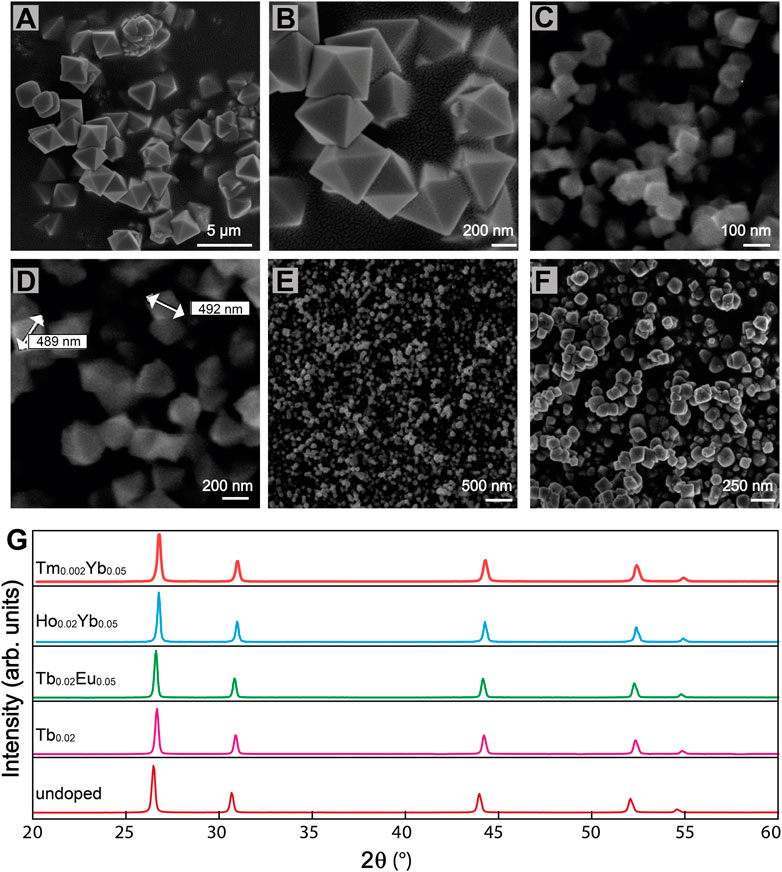
FIGURE 1. SEM micrographs of BiF3 sub-micro particles (A) (B) Eu0.05 doped, (C) (D) undoped, (E) Er0.02 doped, (F) Er0.01Yb0.05 co-doped. (G) XRD patterns of BiF3 sample undoped and Ln3+ single and co-doped.
On one hand, for samples with a low concentration of dopants octahedral is the predominant shape (Figure 1E), on the other hand, with a higher concentration of dopants, the micrographs revealed agglomerated granules (Figure 1F). We observed that when the amount of Ln3+ ions is higher than 5.0 atomic %, the thermodynamic equilibrium is altered and particles with different shapes are obtained (Supplementary Figure S2B).
Figure 1G suggests all the synthesized samples exhibit the same XRD pattern, attributable to α-BiF3 cubic structure (ISCD#2452 card). The adopted microwave-assisted synthesis route can determine the incorporation of the oxygen into the α-BiF3 structure that leads to the formation of BiOyF3−2y solid solutions (Escudero et al., 2014), and thus, diverse solid solutions based on the cubic fluorite, orthorhombic, monoclinic, rhombohedral, and hexagonal structures have been reported so far (Bervas et al., 2006).
The elemental mapping energy performed through X-ray (EDX) confirms the homogeneous distribution of elements in the Ln3+ doped particles. The elemental mapping of BiF3 confirmed that all the elements were finely dispersed over the entire analyzed area (Supplementary Figure S4).
Photoluminescence characterization
It is widely known that the luminescence intensity of Ln3+ ions is sensitive to the concentration of the dopants. Looking forward to studying the optical properties of the material, we synthesized BiF3 particles incorporating different concentrations of Ln3+ ions. Figure 2 displays a series of Ln3+ single-doped and co-doped samples. Figures 2A–C shows the emission spectra for Eu3+ and Tb+3 single-doped and co-doped samples under 370 nm excitation. The narrow lines (FWHM <10 nm) are ascribed to the Tb3+ (5D4→7F3-6) and Eu3+ (5D0→7F0-4) transitions. The Tb3+ single-doped samples are normalized to the peak intensity of 5D4→7F4 transition for comparison. The Eu3+ single-doped and Tb3+ co-doped samples instead are normalized to the peak intensity of 5D0→7F1 transition. From the Eu3+ samples, the relative intensity of the 5D1→7F0,3 and 5D0→7F1,2 decreases as the Eu3+ concentration increases. One important transition is the Eu3+ 5D0→7F1 one (centered at 591 nm), a magnetic dipole transition (MD), allowed by the selection rules. The 5D0→7F1 transition is usually less intense than the 5D0→7F2 one (Binnemans, 2015). For the BiF3 host, however, the MD transition is most intense due to the Eu3+ cations occupying crystallographic sites with an inversion center, which is compatible with the replacement of Bi3+ by Eu3+ cations (Escudero et al., 2014). Moreover, emission bands originating from the 5D1 emitting level are clearly observed at low temperatures for the sample doped with a Eu3+ concentration of 5 mol% due to the suppression of non-radiative processes responsible for the thermal quenching at room temperature. We stress the presence of 5D1→7F0 transition that usually is not allowed for Eu3+ in centrosymmetric sites, in line with previous reports in other matrices with similar local symmetry (Tanner, 2013). The above-described Eu3+ transitions are usually observed in matrices with very low phonon energy, that are very convenient as hosts. In the Eu3+/Tb3+ co-doped samples, there is evidence of a Tb3+-to-Eu3+ energy transfer as signed by the intra-4f8 transitions lines observed in the Eu3+ excitation spectra (Supplementary Figure S5A), together with the decrease of the emission intensity for the Tb3+ transitions as the Eu3+ concentration increases (Figure 2C).
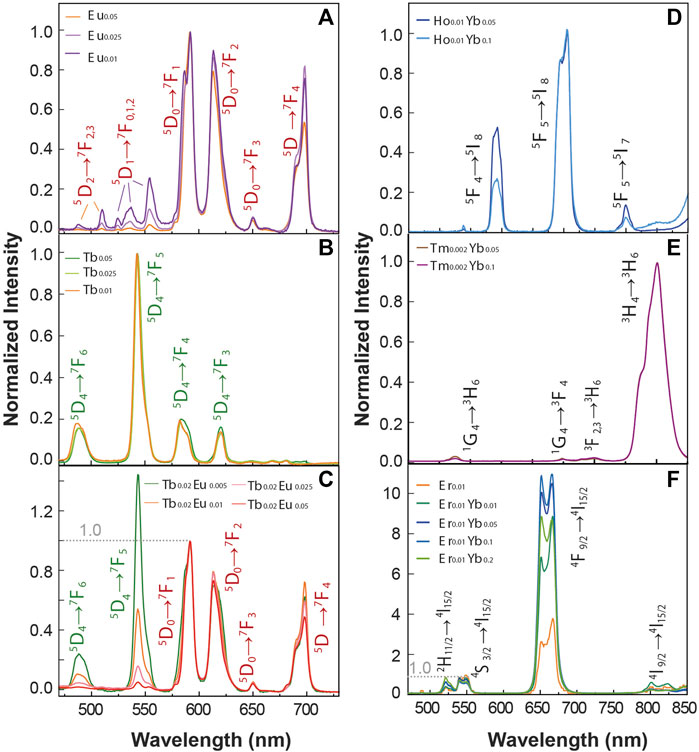
FIGURE 2. Emission spectra of (A) Eu3+single-doped (B) Tb3+ single-doped, and (C) Tb3+-Eu3+ co-doped samples under 370 nm excitation. The transitions assigned the Tb3+ and Eu3+ ions are presented in green and red colors, respectively. Upconversion emission spectra of (D) Yb3+ Tm3+ co-doped, (E) Yb3+ Ho3+ co-doped and (F) Yb3+ Er3+ co-doped and single doped Er3+ samples upon 980 nm excitation.
Figures 2D–F shows the upconversion emission spectra of a series of Ln3+ single-doped and Yb3+-Ln3+ (Er3+, Tm3+, Ho3+) co-doped samples upon 980 nm excitation. The emission Ho3+/Yb3+ co-doped samples (Figure 2D) show the emissions bands of Ho3+ ions in the green, red, and near-infrared spectral ranges, originating from the 5F4→5I8 (∼540 nm), 5F5→5I8 (∼650 nm), and 5F4→5I7 (∼750 nm) transitions, respectively. The emission spectra recorded for the Tm3+/Yb3+ co-doped samples are presented in Figure 2E, and the peaks ascribed to the Tm3+ transitions 1G4→3H6 at 475 nm, 1G4→3F4 (∼650 nm), 3F2,3→3H6 (∼700 nm) and 3H4→3H6 (∼795 nm) are observed. Figure 2F presents the emission spectra of the Er3+/Yb3+ co-doped samples, in which the emission bands ascribed to 2H11/2→4I15/2 (∼520 nm), 4S3/2→4I15/2 (∼550 nm) and 4F9/2→4I15/2 (∼650 nm) transitions are observed. In all the Yb3+-Ln3+ samples, the recorded upconverting emission involves the Yb3+ 2F5/2 level population by ground state absorption, followed by Yb3+-to-acceptor energy transfer mechanisms (Dong et al., 2015). The acceptor ions (Ho3+, Tm3+, Er3+) are first promoted to their respective excited states and then relax originating the observed spectral features. The energy diagram illustrating a possible route for the energy transfer processes is depicted in Supplementary Figure S6.
Exploiting BiF3: Yb3+/Er3+ particles for primary thermometry
Primary thermometers are temperature sensors in which the temperature determination is based on well-grounded physical principles. In 2017, Balabhadra et al. (2017) proposed a straightforward method to predict the temperature calibration curve of any upconverting thermometer based on two thermally coupled electronic levels using the Boltzmann statistics. Here, we selected an Er3+/Yb3+co-doped sample (1% of Er and 5% of Yb) hereafter denominated BiF3:YbEr. Figure 3A displays the temperature-dependent upconversion emission spectra of BiF3:YbEr recorded under 980 nm excitation between 298 and 363 K. Figure 3B shows the thermal dependence of the integrated areas of the Er3+ transitions 2H11/2→4I15/2 and 4S3/2→4I15/2 and corresponding to the IH (510–533 nm) and IS (533–570 nm), respectively. We observe two regimes, depending on the temperature range: for T < 320 K the IH transition remains constant, and IS decreases about 25%, whereas for higher temperatures both IH and IS increase. The parameter that allows the conversion of the integrated areas into temperature is the so-called thermometric parameter, defined as Δ = IH/IS (Figure 3C).
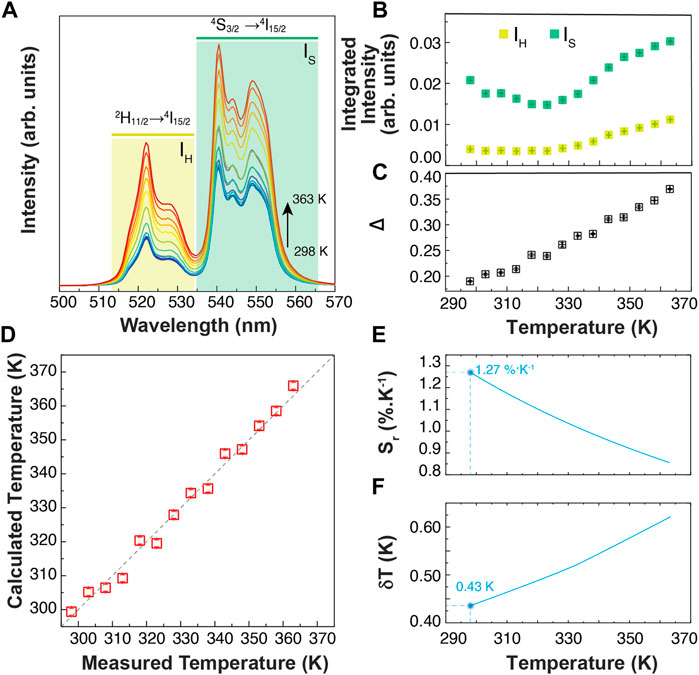
FIGURE 3. (A) Temperature-dependent upconversion emission spectra of BiF3:YbEr upon 980 nm excitation (PD = 5 W cm−2). The shadowed areas correspond to the integration ranges. (B) Temperature dependence of the emission band integrated areas related to the 2H11/2→4I15/2 (IH) and 4S3/2→4I15/2 (IS) Er3+ transitions. The corresponding calibration curve is presented in (C). (D) Comparison between the measured (x-axis) and the calculated trough Eq. 1 (y-axis) temperatures. The interrupted line is a guide to the eyes corresponding to y = x. (E) Relative thermal sensitivity and (F) temperature uncertainty of BiF3:YbEr expressed as Sr obtained through Eq.2 and Eq.3, respectively.
According to the established methodology (S. Balabhadra et al., 2017), the calibration curve can be predicted if the energy separation between the emitting levels (ΔE) and the Δ value in the limit of null excitation power density, Δ0 (at temperature T0, calculus in Supporting Information) can be obtained independently. The ΔE value is calculated by the difference between the barycenter of the emission spectra recorded at room temperature, resulting ΔE = 768 ± 25 cm−1 (calculus in Supporting Information). This value agrees with that reported for Yb3+/Er3+ co-doped systems (Hernández-Rodríguez et al., 2021). The Δ0 value, estimated at room temperature (T0 = 298.0 ± 0.1 K) as the extrapolation for null excitation power densities of the thermometric parameter, is Δ0 = 0.16 ± 0.01.
The maximum Sr value was observed for the lower temperature value tested (298 K) of 1.3 ± 0.1% K−1 and the minimum δT = 0.4 K was estimated at the same temperature (Figure 3E,F). As the temperature increases, Sr decreases reaching 0.9 ± 0.1% K−1 at 365 K. The corresponding thermal uncertainty increases to 0.7 K. We conclude that BiF3:YbEr sub-micro particles exhibited comparable Sr values to those reported for optical thermometers based on Yb3+/Er3+ (Table 1) as expected since the ΔE value obtained here (that is determining the maximum Sr), is comparable to that found in the literature (Hernández-Rodríguez et al., 2021). The validity of Eq. 1 to predict the temperature was verified in the range 295–365 K. Figure 3D presents a comparison between measured (by a thermocouple, uncertainty of 0.1 K) and calculated temperature values in that temperature range. There is a perfect match between calculated and measured temperature values within the uncertainty of both quantities, thus attesting that the studied system is a primary thermometer with temperature determined via Eq. 1. These results sustain that BiF3:YbEr sub-micro particles are primary luminescent thermometers working in a wide range of temperatures.
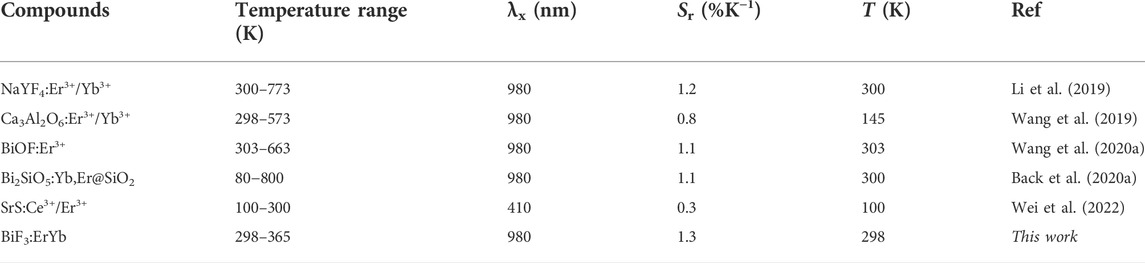
TABLE 1. Working temperature range, excitation wavelength (λx, nm), relative sensitivity (Sr, %K−1) and corresponding temperature value (T) for which it is reported of illustrative Ln3+- based optical temperature sensors involving the ratio of the emission integrated areas of the Er3+ transitions.
Exploiting BiF3: Yb3+/Er3+ particles for molecular logic
The BiF3:YbEr particles present emission features that are dependent on the temperature and on the excitation power density employed. Rationalizing these observations in the context of molecular logic, we exploit external stimuli (e.g., excitation power density, PD, and T) and several logic outputs, considering the modifications induced in the emission spectra (Figures 4A,B). First, we calculate the intensity of some Stark components of the Er3+ transitions 4S3/2, 2H11/2→4I15/2 transition, selecting the peak wavelengths, namely the 522.0 (I1) and 527.0 nm (I2) wavelengths, in the 4S3/2→4I15/2 transition, and the 540.5, 544.0, 549.0 and 552 nm wavelengths (I3 to I6, respectively) in the 2H11/2→ 4I15/2 one. These intensities allow us to define four ratiometric outputs, as R1 = I1/I3; R2 = I1/I2, R3 = I3/I4, and R4 = I5/I6. The ratiometric definition of the logic outputs guarantees that the system is independent of eventual fluctuations in the excitation source intensity and/or electrical drifts of the detection system. The temperature dependence of the intensity ratios is presented in Figures 4C,D for low (5 W·cm−2) and high (111 W cm−2) PD values. As the particles are luminescent primary thermometers, we can determine the actual local temperature upon 980 nm irradiation. The comparison between the measured temperature (set in the temperature controller) and that calculated using Eq. 1 is presented in Figure 4E. As expected, the increase of the PD value induces local heating on the particles independent of the temperature set in the controller, reaching a median value of 0.3° for 5 W cm−2 (comparable to the temperature uncertainty of the thermometer) and 43.3°C for 111 W cm−2 (Figure 4F). These values are comparable to those reported by Bhiri et al. using GdVO4:Yb3+/Er3+ microcrystals [∼50°C at 100 W cm−2, (Bhiri et al., 2022)].
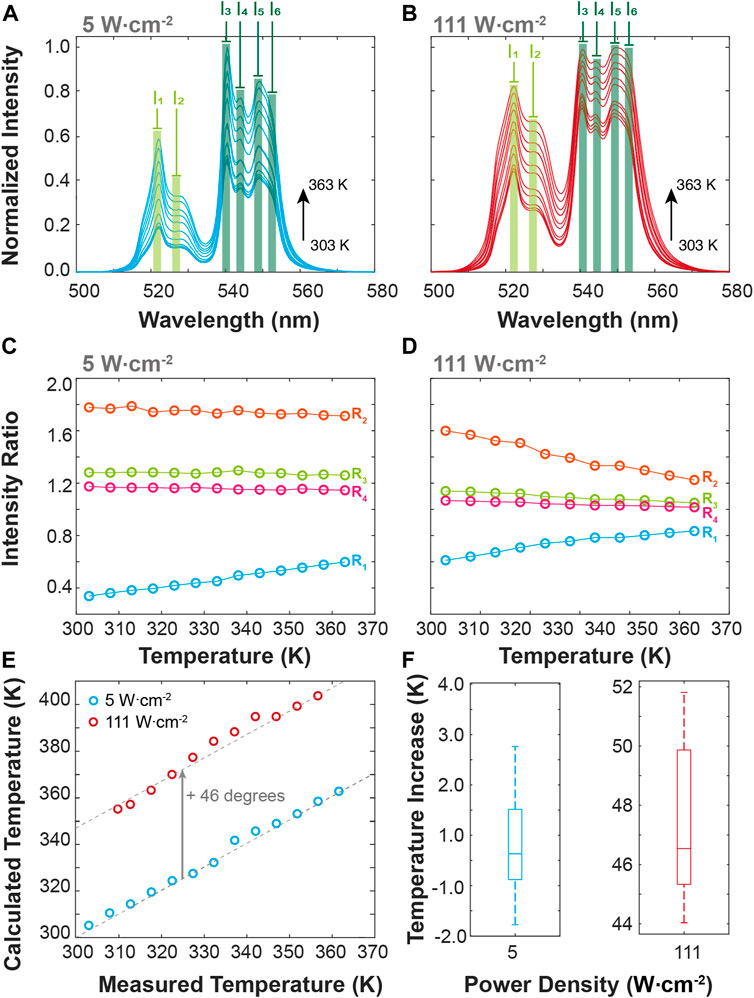
FIGURE 4. Temperature-dependent upconversion emission spectra of BiF3:YbEr upon 980 nm excitation at (A) 5 and (B) 111 W cm−2. (C) Intensities ratio as a function of the temperature calculated from the emission spectra at (C) 5 and (D) 111.1 W cm−2. (E) Comparison between the measured (set in the temperature controller) and the calculated (Eq. 1) temperature values for the 5 and 111 W cm−2. The interrupted lines are guides to the eyes corresponding to y = x (overlapping the blue symbols) and y = x+46.3 (overlapping the red symbols). The corresponding boxplots of the temperature increase are presented in (F).
To demonstrate the application of the BiF3:YbEr particles to molecular logic we represent the dependence of the intensity ratios defined above (R1-R4) on the temperature and the excitation power density, Figure 5A. The excitation radiation was not employed as a logic input being an a priori condition for the activation of the logic gate. The logic inputs are digitalized to 0 and 1 using the room temperature (305 K) set in the temperature controller as 0 and 360 K as 1. For PD the logic input 0 was set to 5 W cm−2 and the logical value 1–111 W cm−2. For each intensity ratio, a threshold value (pointed lines in Figure 5A) was arbitrarily set to get the higher number of distinct logic gates as possible. The corresponding truth tables were then constructed setting the logical values of 1 for the intensity ratios above the thresholds. The ratios R1, R2, and R4 correspond to AND, NAND, and NOR logic gates whereas the ratio R3 is insensitive to the temperature, yielding simply a NOT gate on the logic input PD. This is the first time the same upconversion emission is used to define multiple logic gates, constituting an equivalent molecular chipset as represented in Figure 5B.
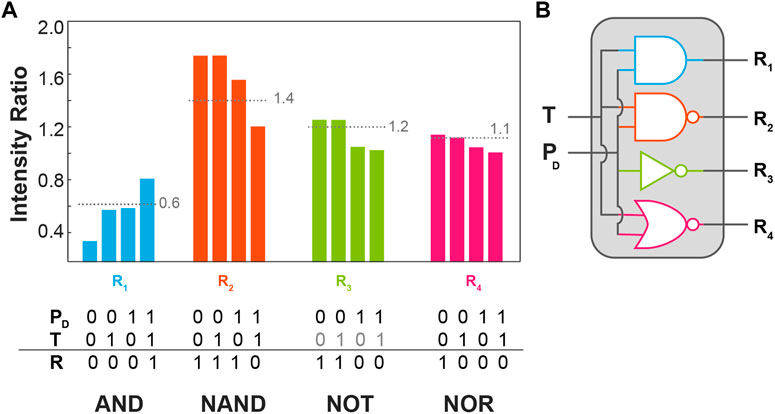
FIGURE 5. (A) Bar chart of the intensity ratios R1-R4 as a function of the temperature input (T) and excitation power density (PD) presenting the corresponding threshold (pointed line) the truth tables in the bottom. (B) Schematic representation of the logic circuitry with the logic gates presented in (A).
Conclusion
BiF3:Ln3+ (Ln = Yb, Er, Tm, Eu, Tb) sub-micro particles were successfully prepared via microwave-assisted synthesis. Monodispersed octahedra sub-micro particles were obtained with Ln3+ doping concentration <5 atomic %, while at higher concentrations of dopants the shape is no longer octahedral and spheres and aggregated are predominant. The crystalline structure of the materials is not altered by the concentration of the dopants. Through a microwave synthesis approach, we obtained samples with high reproducibility and the possibility of a vast field of applications, even on the industrial scale.
All the prepared BiF3:Ln3+ sub-micro particles display a detectable emission, both for downshifting and upconverting emission. We conclude that Ln3+-doped BiF3 sub-micro particles synthesized through the microwave-assisted method are suitable for observing downshifting and upconversion emissions. The matrix has an important role due to the intrinsic low phonon energy that strongly determines the balance between the probabilities of radiative and non-radiative processes such as multiphonon relaxation and cross-relaxation mechanisms. The intrinsic low phonon energy of the BiF3 host is an advantage that allows us to observe transitions that are obscured in other fluoride host matrixes such as NaYF4.
We characterized Er3+-Yb3+ co-doped BiF3 particles under 980 nm excitation for luminescence thermometry and molecular logic. We proved that these particles are luminescent primary thermometers with relative sensitivity up to 1.27% K−1 and temperature uncertainty down to 0.4 K, values in good agreement with those in the literature for Yb3+/Er3+ co-doped upconverting nanoparticles. Moreover, this sample presents emission features that are dependent on the excitation power density and the temperature, and thus, as an illustrative example, we defined a molecular chipset constituted of four independent logic gates (AND, NAND, NOR, and NOT) by analyzing the 2H11/2→4I15/2 and 4S3/2→4I15/2 Er3+ transitions. The next challenge will be to employ these particles to define more complex operations involving more emitting centers and diverse external stimuli, looking forward to yielding logical reconfiguration, thus demonstrating that bismuth-based materials are suitable for molecular computing in all-photonic logical devices.
Data availability statement
The original contributions presented in the study are included in the article/Supplementary Materials, further inquiries can be directed to the corresponding author.
Author contributions
SZ: Original draft and original figures preparation, synthesis of the particles, and photophysical characterization. ET, EM, AT, MB: Conceptualization, supervising the synthesis of the particles, data curation, and validation of the synthesis and structural characterization of the prepared particles. LC, RF, CB: Conceptualization of the luminescent thermometry and molecular logic experiments, methodology on photoluminescence measurements, data curation on photoluminescence for luminescent thermometry and molecular logic, software, validation, writing, reviewing, and editing on the final manuscript and figures.
Funding
This work was developed within the scope of the project CICECO-Aveiro Institute of Materials, UIDB/50011/2020, UIDP/50011/2020 & LA/P/0006/2020, financed by national funds through the FCT/MEC (PIDDAC). Financial support from the project LogicALL (PTDC/CTM-CTM/0298/2020) Portuguese funds through FCT/MCTES, is acknowledged. SZ acknowledges Fundação da Ciência e Tecnologia (Portugal) for a Ph.D. grant (SFRH/BD/144239/2019) financed by Portuguese national funds through the FCT/MEC and when appropriate co-financed by FEDER.
Conflict of interest
The authors declare that the research was conducted in the absence of any commercial or financial relationships that could be construed as a potential conflict of interest.
Publisher’s note
All claims expressed in this article are solely those of the authors and do not necessarily represent those of their affiliated organizations, or those of the publisher, the editors and the reviewers. Any product that may be evaluated in this article, or claim that may be made by its manufacturer, is not guaranteed or endorsed by the publisher.
Supplementary material
The Supplementary Material for this article can be found online at: https://www.frontiersin.org/articles/10.3389/fphot.2022.1010958/full#supplementary-material
References
Alivisatos, A. P. (1996). Semiconductor clusters, nanocrystals, and quantum dots. Science 271, 933–937. doi:10.1126/science.271.5251.933
Andréasson, J., and Pischel, U. (2021). Light-stimulated molecular and supramolecular systems for information processing and beyond. Coord. Chem. Rev. 429, 213695. doi:10.1016/j.ccr.2020.213695
Andreasson, J., Pischel, U., Straight, S. D., Moore, T. A., Moore, A. L., and Gust, D. (2011). All-photonic multifunctional molecular logic device. J. Am. Chem. Soc. 133, 11641–11648. doi:10.1021/ja203456h
Back, M., Casagrande, E., Brondin, C. A., Ambrosi, E., Cristofori, D., Ueda, J., et al. (2020a). Lanthanide-doped Bi2SiO5@SiO2 core–shell upconverting nanoparticles for stable ratiometric optical thermometry. ACS Appl. Nano Mat. 3, 2594–2604. doi:10.1021/acsanm.0c00003
Back, M., Casagrande, E., Trave, E., Cristofori, D., Ambrosi, E., Dallo, F., et al. (2020b). Confined-melting-assisted synthesis of bismuth silicate glass-ceramic nanoparticles: Formation and optical thermometry investigation. ACS Appl. Mat. Interfaces 12, 55195–55204. doi:10.1021/acsami.0c17897
Back, M., Ueda, J., Ambrosi, E., Cassandro, L., Cristofori, D., Ottini, R., et al. (2019). Lanthanide-doped bismuth-based fluoride nanocrystalline particles: Formation, spectroscopic investigation, and chemical stability. Chem. Mat. 31, 8504–8514. doi:10.1021/acs.chemmater.9b03164
Bai, X., Caputo, G., Hao, Z. D., Freitas, V. T., Zhang, J. H., Longo, R. L., et al. (2014). Efficient and tuneable photoluminescent boehmite hybrid nanoplates lacking metal activator centres for single-phase white LEDs. Nat. Commun. 5, 5702. doi:10.1038/ncomms6702
Balabhadra, S., Debasu, M. L., Brites, C. D. S., Ferreira, R. A. S., and Carlos, L. D. (2017). Upconverting nanoparticles working as primary thermometers in different media. J. Phys. Chem. C 121, 13962–13968. doi:10.1021/acs.jpcc.7b04827
Bervas, M., Yakshinskiy, B., Klein, L. C., and Amatucci, G. G. (2006). Soft‐chemistry synthesis and characterization of bismuth oxyfluorides and ammonium bismuth fluorides. J. Am. Ceram. Soc. 89, 645–651. doi:10.1111/j.1551-2916.2005.00721.x
Bhiri, N. M., Dammak, M., Carvajal, J. J., Aguiló, M., Díaz, F., and Pujol, M. C. (2022). Excitation power density dependence of a primary luminescent thermometer based on Er3+, Yb3+: GdVO4 microcrystals operating in the visible. J. Alloys Compd. 921, 166020. doi:10.1016/j.jallcom.2022.166020
Binnemans, K. (2015). Interpretation of europium(III) spectra. Coord. Chem. Rev. 295, 1–45. doi:10.1016/j.ccr.2015.02.015
Brites, C. D. S., Balabhadra, S., and Carlos, L. D. (2019). Lanthanide-based thermometers: At the cutting-edge of luminescence thermometry. Adv. Opt. Mater. 7, 1801239. doi:10.1002/adom.201801239
Brites, C. D. S., Millán, A., and Carlos, L. D. (2017). “Lanthanides in luminescent thermometry,” in Handbook on the Physics and chemistry of Rare Earths. Editors J.-C. G. Bünzli, and V. K. Pecharsky (Amsterdam: Elsevier Science).
Bünzli, J.-C. G. (2015). On the design of highly luminescent lanthanide complexes. Coord. Chem. Rev. 293, 19–47. doi:10.1016/j.ccr.2014.10.013
Casagrande, E., Back, M., Cristofori, D., Ueda, J., Tanabe, S., Palazzolo, S., et al. (2020). Upconversion-mediated Boltzmann thermometry in double-layered Bi 2 SiO5:Yb3+, Tm3+@ SiO2 hollow nanoparticles. J. Mat. Chem. C 8, 7828–7836. doi:10.1039/d0tc01457e
Chen, G., Yang, C., and Prasad, P. N. (2013). Nanophotonics and nanochemistry: Controlling the excitation dynamics for frequency up-and down-conversion in lanthanide-doped nanoparticles. Acc. Chem. Res. 46, 1474–1486. doi:10.1021/ar300270y
Dąbrowska, S., Chudoba, T., Wojnarowicz, J., and Łojkowski, W. (2018). Current trends in the development of microwave reactors for the synthesis of nanomaterials in laboratories and industries: A review. Crystals 8, 379. doi:10.3390/cryst8100379
De Silva, A. P., Gunaratne, N. H. Q., and Mccoy, C. P. (1993). A molecular photoionic AND gate based on fluorescent signalling. Nature 364, 42–44. doi:10.1038/364042a0
Deng, R., Qin, F., Chen, R., Huang, W., Hong, M., and Liu, X. (2015). Temporal full-colour tuning through non-steady-state upconversion. Nat. Nanotechnol. 10, 237–242. doi:10.1038/nnano.2014.317
Dong, H., Sun, L.-D., and Yan, C.-H. (2015). Energy transfer in lanthanide upconversion studies for extended optical applications. Chem. Soc. Rev. 44, 1608–1634. doi:10.1039/c4cs00188e
Erbas-Cakmak, S., Kolemen, S., Sedgwick, A. C., Gunnlaugsson, T., James, T. D., Yoon, J., et al. (2018). Molecular logic gates: The past, present and future. Chem. Soc. Rev. 47, 2228–2248. doi:10.1039/c7cs00491e
Escudero, A., Moretti, E., and Ocaña, M. (2014). Synthesis and luminescence of uniform europium-doped bismuth fluoride and bismuth oxyfluoride particles with different morphologies. CrystEngComm 16, 3274. doi:10.1039/c3ce42462f
Gedye, R., Smith, F., Westaway, K., Ali, H., Baldisera, L., Laberge, L., et al. (1986). The use of microwave ovens for rapid organic synthesis. Tetrahedron Lett. 27, 279–282. doi:10.1016/s0040-4039(00)83996-9
Hernández-Rodríguez, M. A., Kamada, K., Yoshikawa, A., Muñoz-Santiuste, J. E., Casasnovas-Melián, A., Martín, I. R., et al. (2021). 1000 K optical ratiometric thermometer based on Er3+ luminescence in yttrium gallium garnet. J. Alloys Compd. 886, 161188. doi:10.1016/j.jallcom.2021.161188
Jaque, D., and Vetrone, V. (2012). Luminescence nanothermometry. Nanoscale 4, 4301. doi:10.1039/c2nr30764b
Kato, D., Hongo, K., Maezono, R., Higashi, M., Kunioku, H., Yabuuchi, M., et al. (2017). Valence band engineering of layered bismuth oxyhalides toward stable visible-light water splitting: Madelung site potential analysis. J. Am. Chem. Soc. 139, 18725–18731. doi:10.1021/jacs.7b11497
Kumar, D., Sharma, S. K., Verma, S., Sharma, V., and Kumar, V. (2020). A short review on Rare earth doped NaYF4 upconverted nanomaterials for solar cell applications. Mater. Today Proc. 21, 1868–1874. doi:10.1016/j.matpr.2020.01.243
Kuriki, K., Koike, Y., and Okamoto, Y. (2002). Plastic optical fiber lasers and amplifiers containing lanthanide complexes. Chem. Rev. 102, 2347–2356. doi:10.1021/cr010309g
Li, X., Yang, L., Zhu, Y., Zhong, J., and Chen, D. (2019). Upconversion of transparent glass ceramics containing β-NaYF4:Yb3+, Er3+ nanocrystals for optical thermometry. RSC Adv. 9, 7948–7954. doi:10.1039/c9ra01088b
Nicoli, F., Paltrinieri, E., Tranfić Bakić, M., Baroncini, M., Silvi, S., and Credi, A. (2021). Binary logic operations with artificial molecular machines. Coord. Chem. Rev. 428, 213589. doi:10.1016/j.ccr.2020.213589
Nüchter, M., Ondruschka, B., Bonrath, W., and Gum, A. (2004). Microwave assisted synthesis – A critical technology overview. Green Chem. 6, 128–141. doi:10.1039/b310502d
Piana, S., Reyhani, M., and Gale, J. D. (2005). Simulating micrometre-scale crystal growth from solution. Nature 438, 70–73. doi:10.1038/nature04173
Sanna, S., Esposito, V., Andreasen, J. W., Hjelm, J., Zhang, W., Kasama, T., et al. (2015). Enhancement of the chemical stability in confined δ-Bi2O3. Nat. Mat. 14, 500–504. doi:10.1038/nmat4266
Tanner, P. A. (2013). Some misconceptions concerning the electronic spectra of tri-positive europium and cerium. Chem. Soc. Rev. 42, 5090–5101. doi:10.1039/c3cs60033e
Wang, C., Du, P., Li, W., and Luo, L. (2020a). Facile synthesis and photoluminescence performance of Er3+-activated BiOF sub-micro particles for ratiometric thermometers. J. Lumin. 226, 117416. doi:10.1016/j.jlumin.2020.117416
Wang, C., Ran, W., Du, P., Li, W., Luo, L., and Wang, D. (2020b). Enhanced visible light-driven photocatalytic activities and photoluminescence characteristics of BiOF nanoparticles determined via doping engineering. Inorg. Chem. 59, 11801–11813. doi:10.1021/acs.inorgchem.0c01811
Wang, X., Wang, Y., Jin, L., Bu, Y., Yang, X. L., and Yan, X. (2019). Controlling optical temperature detection of Ca3Al2O6: Yb3+, Er3+ phosphors through doping. J. Alloys Compd. 773, 393–400. doi:10.1016/j.jallcom.2018.09.229
Wei, J., Liu, Y., Zhang, M., Zheng, W., Huang, P., Gong, Z., et al. (2022). Blue-LED-excitable NIR-II luminescent lanthanide-doped SrS nanoprobes for ratiometric thermal sensing. Sci. China Mat. 65, 1094–1102. doi:10.1007/s40843-021-1801-8
Xia, Y. N., Yang, P. D., Sun, Y. G., Wu, Y. Y., Mayers, B., Gates, B., et al. (2003). One-dimensional nanostructures: Synthesis, characterization, and applications. Adv. Mat. 15, 353–389. doi:10.1002/adma.200390087
Xie, H.-H., Wen, Q., Huang, H., Sun, T.-Y., Li, P., Li, Y., et al. (2015). Synthesis of bright upconversion submicrocrystals for high-contrast imaging of latent-fingerprints with cyanoacrylate fuming. RSC Adv. 5, 79525–79531. doi:10.1039/c5ra15255k
Yi, G., Lu, H., Zhao, S., Ge, Y., Yang, W., Chen, D., et al. (2004). Synthesis, characterization, and biological application of size-controlled nanocrystalline NaYF4:Yb, Er infrared-to-visible up-conversion phosphors. Nano Lett. 4, 2191–2196. doi:10.1021/nl048680h
Zaccariello, G., Back, M., Benedetti, A., Canton, P., Cattaruzza, E., Onoda, H., et al. (2019). Bismuth titanate-based UV filters embedded mesoporous silica nanoparticles: Role of bismuth concentration in the self-sealing process. J. Colloid Interface Sci. 549, 1–8. doi:10.1016/j.jcis.2019.04.042
Zaccariello, G., Back, M., Zanello, M., Canton, P., Cattaruzza, E., Riello, P., et al. (2017). Formation and controlled growth of bismuth titanate phases into mesoporous silica nanoparticles: An efficient self-sealing nanosystem for UV filtering in cosmetic formulation. ACS Appl. Mat. Interfaces 9, 13252. doi:10.1021/acsami.6b13252
Keywords: trivalent lanthanide, bismuth fluoride, luminescence, primary thermometry, molecular logic
Citation: Zanella S, Trave E, Moretti E, Talon A, Back M, Carlos LD, Ferreira RAS and Brites CDS (2022) Designing Ln3+-doped BiF3 particles for luminescent primary thermometry and molecular logic. Front. Photonics 3:1010958. doi: 10.3389/fphot.2022.1010958
Received: 03 August 2022; Accepted: 14 September 2022;
Published: 29 September 2022.
Edited by:
Wenqin Luo, Huzhou University, ChinaReviewed by:
Renren Deng, Zhejiang University, ChinaWei Zheng, Fujian Institute of Research on the Structure of Matter, Chinese Academy of Sciences (CAS), China
Copyright © 2022 Zanella, Trave, Moretti, Talon, Back, Carlos, Ferreira and Brites. This is an open-access article distributed under the terms of the Creative Commons Attribution License (CC BY). The use, distribution or reproduction in other forums is permitted, provided the original author(s) and the copyright owner(s) are credited and that the original publication in this journal is cited, in accordance with accepted academic practice. No use, distribution or reproduction is permitted which does not comply with these terms.
*Correspondence: Rute A. S. Ferreira, cmZlcnJlaXJhQHVhLnB0; Carlos D. S. Brites, Y2FybG9zLmJyaXRlc0B1YS5wdA==