- 1Department of Orthodontic Science, Graduate School of Medical and Dental Sciences, Tokyo Medical and Dental University, Tokyo, Japan
- 2Department of Forensic Medicine, Graduate School of Medicine, Tokyo Medical University, Tokyo, Japan
- 3Department of Legal Medicine (Forensic Medicine), Keio University School of Medicine, Tokyo, Japan
- 4Department of Advanced Clinical Science and Therapeutics, The University of Tokyo, Tokyo, Japan
Intermittent hypoxia (IH) recapitulates morphological changes in the maxillofacial bones in children with obstructive sleep apnea (OSA). Recently, we found that IH increased bone mineral density (BMD) in the inter-radicular alveolar bone (reflecting enhanced osteogenesis) in the mandibular first molar (M1) region in the growing rats, but the underlying mechanism remains unknown. In this study, we focused on the hypoxia-inducible factor (HIF) pathway to assess the effect of IH by testing the null hypothesis of no significant differences in the mRNA-expression levels of relevant factors associated with the HIF pathway, between control rats and growing rats with IH. To test the null hypothesis, we investigated how IH enhances mandibular osteogenesis in the alveolar bone proper with respect to HIF-1α and vascular endothelial growth factor (VEGF) in periodontal ligament (PDL) tissues. Seven-week-old male Sprague–Dawley rats were exposed to IH for 3 weeks. The microstructure and BMD in the alveolar bone proper of the distal root of the mandibular M1 were evaluated using micro-computed tomography (micro-CT). Expression of HIF-1α and VEGF mRNA in PDL tissues were measured, whereas osteogenesis was evaluated by measuring mRNA levels for alkaline phosphatase (ALP) and bone morphogenetic protein-2 (BMP-2). The null hypothesis was rejected: we found an increase in the expression of all of these markers after IH exposure. The results provided the first indication that IH enhanced osteogenesis of the mandibular M1 region in association with PDL angiogenesis during growth via HIF-1α in an animal model.
Introduction
Intermittent hypoxia (IH) during sleep has been implicated in the pathogenesis of obstructive sleep apnea (OSA; Noda et al., 1998; Lal et al., 2012), although the role of IH in the growth of children with OSA has not been clarified. In particular, it has been reported that pediatric OSA is frequently associated with impairment in the growth and development of craniofacial and otolaryngological tissues, as well as with neuromuscular diseases (Balbani et al., 2005; Huang and Guilleminault, 2012). Hypertension, cardiac remodeling, and other complications of OSA have been studied using rodent models of IH induced by short cycles of hypoxia–normoxia (Skelly et al., 2012; Maeda et al., 2013; Nagai et al., 2015). Previously, we demonstrated that IH exposure induces a decrease in the volume of the nasal cavity, reflecting impaired development of maxillofacial bones (Kuma et al., 2014). Other researchers showed that IH increased the bone mineral density (BMD) in the inter-radicular alveolar bone in the mandibular first molar (M1) region during growth (Oishi et al., 2015), but the underlying mechanism remains unknown.
Hypoxia is critical to the remodeling and repair of damaged bones via hypoxia-inducible factor (HIF; Maes et al., 2012), the key stimulator of vessel formation and angiogenesis. The gene encoding the prominent angiogenic factor vascular endothelial growth factor (VEGF) is the primary target for HIF-1α (Riddle et al., 2009). A recent study provided evidence supporting the view that HIFs and VEGF play essential roles in coupling between angiogenesis and osteogenesis during bone formation and repair (Schipani et al., 2009).
Alveolar bone proper is covered with collagen fibers in the periodontal ligament (PDL; Shimizu et al., 2014). The PDL is a specialized soft connective tissue that connects the tooth with the alveolar bone socket, thereby promoting the development and maintenance of periodontium (Kaku and Yamauchi, 2014). PDL tissues are comprised of PDL cells (Shimizu et al., 2014), collagen fibers (Kaku and Yamauchi, 2014), blood vessels (Muramoto et al., 2000), nerve elements (Muramoto et al., 2000), extracellular substances (Kaneko et al., 2001), osteoclasts (Kaneko et al., 2001), and osteoblasts (Mayahara et al., 2012), and provides progenitor cells for bone formation and remodeling. Alkaline phosphatase (ALP) and bone morphogenetic protein-2 (BMP-2) are known to induce osteogenesis and the osteogenic transformation of PDL cells (Kuru et al., 1999; Selvig et al., 2002). ALP activity reflects early osteogenic differentiation in the presence of osteoblasts (Kuru et al., 1999). Previous data showed that ALP activation and BMP-2 upregulation in PDL cells induce periodontium osteogenesis in response to growth hormones (Li et al., 2001) or matrix Gla proteins (Li et al., 2012). In addition, mechanical forces such as moderate occlusal stimuli and dissipation of masticatory force are transmitted from the teeth through the PDL to the progenitor cells, thereby promoting bone remodeling in the periodontal tissue (Chen et al., 2005). As such, PDL tissues maintain proper alveolar bone homeostasis via ALP and BMP-2.
Bone remodeling, a complex process by which old bone is continuously replaced by new tissue, is affected by a variety of biochemical and mechanical factors (Hadjidakis and Androulakis, 2006). With regard to signaling pathways, the Wnt (Wang et al., 2014), OPG/RANKL/RANK (Hsu et al., 2006), and HIF pathways (Mamalis and Cochran, 2011) are well-known in the control of bone remodeling. In this study, we specifically focused on the HIF pathway to assess the effect of IH by testing the null hypothesis that no significant differences in the mRNA-expression levels of relevant factors associated with the HIF pathway in PDL occur between control rats and growing rats with IH. To this end, we analyzed the microarchitecture and mineral density of the alveolar bone proper around the mandibular M1 after 3 weeks of IH in growing rats, with reference to HIF1-α, VEGF, ALP, and BMP-2 mRNA expression.
Materials and Methods
Experimental IH Model
Experiments were conducted on twelve 7-week-old male Sprague–Dawley rats randomly divided into two groups. Experimental rats were exposed to IH at a rate of 20 cycles per h (nadir, 4% oxygen; peak, 21% oxygen; 0% carbon dioxide) for 3 weeks (IH group), and control rats breathed room air (C group). The control cage was placed next to the cage equipped with the IH apparatus, and all rats underwent their respective treatments for 8 h per day during the 12-h “lights on” period (Maeda et al., 2013; Nagai et al., 2015). The experiments were conducted while the rats were 7 to 10 weeks of age, when craniofacial bones actively develop (puberty), as documented by studies of craniofacial growth (Spence, 1940) and puberty onset (Cheung et al., 2001) in male rats. All rats were allowed free access to food and water throughout the experimental period, as previously described (Skelly et al., 2012; Maeda et al., 2013; Nagai et al., 2015). Immediately after the IH-exposure period, all rats were anesthetized by a sodium pentobarbital injection and sacrificed. All experimental procedures were performed according to the Guide for the Care and Use of Laboratory Animals published by the US National Institutes of Health (NIH publication 85-23, revised 1996). The animal protocol was approved by the Animal Experimental Committee of Tokyo Medical University (approval number S-26063).
Three-Dimensional Microcomputed Tomography (Micro-CT) Analysis
Changes in the bony microstructure of the alveolar bone proper around roots of the mandibular M1 and in the PDL space were investigated using micro-CT with a desktop X-ray micro-CT system (SMX-100CT; Shimadzu, Kyoto, Japan) with a scanning resolution of 20-μm intervals on individual images. The region of interest (ROI) for structural morphometry was enlarged to a 40-μm radius around the distal root (Figure 1A; Shimizu et al., 2014). Each ROI was analyzed with respect to BMD, bone volume/tissue volume (BV/TV), trabecular thickness (Tb.Th), and trabecular number (Tb.N) using three-dimensional image-analysis software (TRI/3D-BON; Ratoc System Engineering, Tokyo, Japan). Additionally, to evaluate changes from mechanical stimuli in the PDL space, tissue volume (TV) around the distal root of the mandibular M1 was computed (Figure 1B; Shimizu et al., 2014).
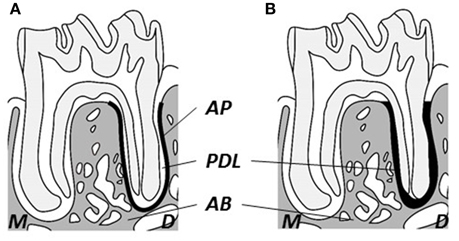
Figure 1. Schematic drawing of observational area of micro-CT. (A) Observational area for alveolar bone proper (heavy black line). (B) Observational area for the periodontal ligament (heavy black area). M, Mesial; D, distal; AB, inter-radicular alveolar bone; AP, alveolar bone proper; PDL, periodontal ligament.
Reverse Transcription Quantitative Real-Time PCR (RT-qPCR) Analysis
PDL tissues were removed from extracted roots of the mandibular M1. Total RNA was isolated from PDL tissues using the PureLinkTM FFPE Total RNA Isolation Kit (Invitrogen, CA, USA) according to instructions provided by the manufacturer (Afanador et al., 2005; Luan et al., 2007). cDNA was synthesized from total RNA with reverse transcription random primers using High-Capacity cDNA Reverse Transcription Kits (Applied Biosystems, Foster City, CA, USA). Quantitative PCR assays were carried out in triplicate for each sample using a 7500 Real-Time PCR System (Applied Biosystems, Foster City, CA, USA). PCR analyses were conducted with gene-specific primers and fluorescently labeled TaqMan probes (Takara Bio, Shiga, Japan). Appropriate primers were chosen for real-time PCR amplification of VEGF (forward primer: 5′-ccttgctgctctacctccac-3′, reverse primer: 5′-ccacttcgtgatgattctgc-3′), HIF-1α (forward primer: 5′-ctaccagaagggcaggatacag-3′, reverse primer: 5′-gca ggcagatgaaataccagtc-3′), ALP (forward primer: 5′-acgtggctaagaatgtcatc-3′, reverse primer: 5′-ctggtaggcgatgtcctta-3′), BMP-2 (forward primer: 5′-tcaagccaaacacaaacagc-3′, reverse primer: 5′-acgtctgaacaatggcatga-3′), and Hprt-1 (forward primer: 5′-cagactttgctttccttgg-3′, reverse primer: 5′-tccactttcgctgatgacac-3′). The thermocycling conditions used were 95°C for 30 s, followed by 40 cycles of 95°C for 5 s and 60°C for 34 s. Gene expression levels were calculated according to the ΔΔCT method of relative quantification. The threshold cycle (Ct) value of the target mRNAs (VEGF, HIF1α, ALP, or BMP-2) was normalized to the Ct values of the internal control (Hprt-1) in the same sample (ΔCt = Cttarget – CtHprt−1), followed by normalization to the control (ΔΔCt = ΔCtIHgroup – ΔCtCgroup). The fold change in expression was calculated as the relative quantification value (RQ; 2−ΔΔCt; Livak and Schmittgen, 2001).
Statistical Analysis
Statistical calculations were performed using statistical analysis software (IBM SPSS Statistics Version 20.0 Chicago, IL, USA). We first examined the normality and variance of the data using the F-test. The control and experimental groups were compared using the Mann–Whitney U-test for nonparametric data, and statistical significance was established at a p level of less than 0.05.
Results
Body Weight Changes in Rats after IH
The median body weight (mean ± standard error) of rats exposed to IH for 3 weeks (277.5 ± 3.8 g) was significantly lower than that of control (C) rats (356.0 ± 9.8 g). Previous reports using this animal model showed that the correlation between the body weight and whole-body growth in this IH model was low (Maeda et al., 2013; Kuma et al., 2014; Oishi et al., 2015).
Three-Dimensional Micro-CT Analysis
We investigated changes in the bony microstructure of the alveolar bone proper around the distal roots of mandibular M1 using micro-CT. Micro-CT images of alveolar bone proper around the distal root of mandibular M1 showed higher bone volume (BV) density in the IH rats than in the C rats (Figure 2). In addition, micro-CT analyses demonstrated significant increases in the BMD, BV/TV, Tb.Th, and Tb.N in the distal root alveolar bone proper of IH rats, compared with those of C rats (Figure 3).
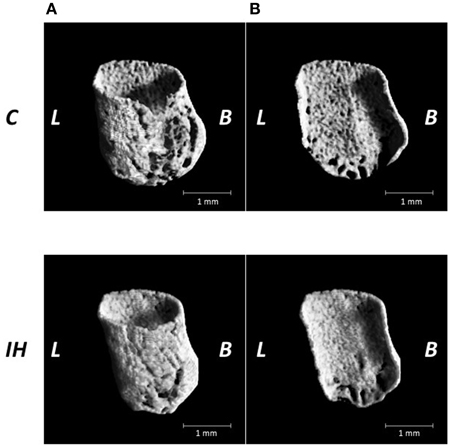
Figure 2. Microarchitecture of the alveolar bone proper of the distal roots in the mandibular first molar region in the C and IH groups. Representative micro-CT images of alveolar bone proper in the distal root of mandibular first molar region (A) and half of mesial surface (B). Scale bar: 1.0 mm. C, control group; IH, IH group; L, lingual; B, buccal.
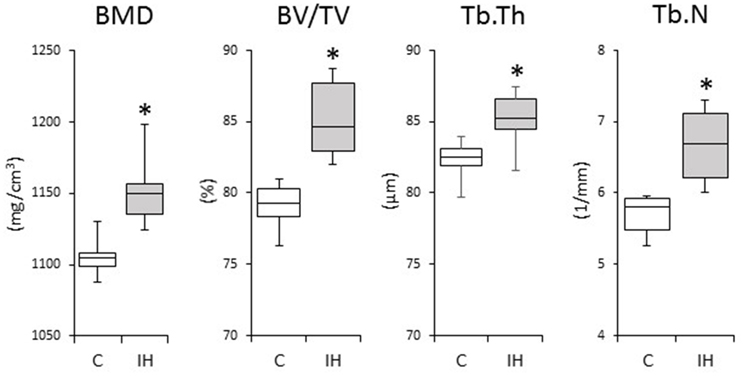
Figure 3. Comparisons of bone morphology between the C and IH groups by micro-CT analysis. Cancellous bone of the alveolar bone proper of the distal roots in the mandibular first molar region was compared between the C and IH groups. Box edges represent the upper and lower quantiles, the middle lines in the boxes represent the medians, and the whiskers represent the maxima and minima. BMD, bone mineral density; BV/TV, bone volume/tissue volume; Tb.Th, trabecular thickness; Tb.N, trabecular number. *p < 0.05 by the Mann–Whitney U-test.
Quantitative Real-Time PCR
We evaluated the relative expression levels of osteogenesis–angiogenesis coupling markers (Figure 4) and osteogenic markers (Figure 5) in PDL tissues by RT-qPCR analysis. IH increased the mRNA levels of HIF-1α and VEGF by 1.71- and 1.97-fold, respectively, in PDL tissues compared with those observed in C rats (Figure 4). Similarly, ALP and BMP-2 mRNA levels were increased 2.75- and 2.57-fold in PDL tissues compared to the corresponding levels in C rats (Figure 5). These changes are not likely to have been mediated by mechanical forces, as IH had no effect on the PDL space surrounding the distal root of the mandibular M1 (Figure 6).
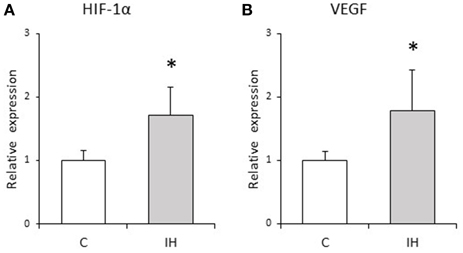
Figure 4. Relative expression levels of osteogenesis–angiogenesis coupling markers. Relative HIF-1α (A) and VEGF (B) expression in the PDL tissues was compared between the C and IH rat groups by RT-qPCR. The mRNA-expression levels measured in C rats were set to a value of 1. Data are shown as the mean ± standard deviation for each group. *p < 0.05 by the Mann–Whitney U-test.
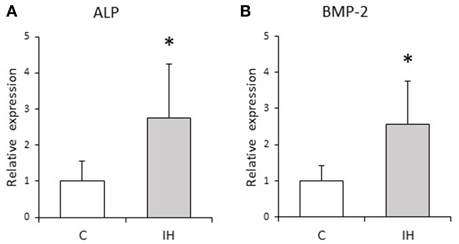
Figure 5. Relative expression levels of osteogenic markers. Relative ALP (A) and BMP-2 (B) expression in the PDL tissues was compared between the C and IH rat groups by RT-qPCR. The mRNA-expression levels measured in C rats were set to a value of 1. Data are shown as the mean ± standard deviation for each group. *p < 0.05 by the Mann–Whitney U-test.
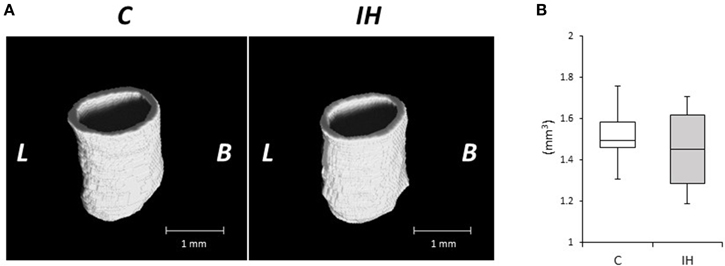
Figure 6. Micro-CT analysis of the periodontal ligament (PDL) space around the distal roots in the mandibular first molar. (A) Three-dimensional reconstructed images of the periodontal ligament (PDL) space. Scale bar: 1.0 mm. C, control group; IH, IH group; L, lingual; B, buccal. (B) Comparison of tissue volume (TV) of the PDL around the distal root of the mandibular first molar. Box edges represent the upper and lower quantiles with the median values shown by the middle line in each box. The whiskers represent the maxima and minima. There was no significant difference in TV in the PDL between the 2 groups. *p < 0.05 by the Mann–Whitney U-test.
Discussion
The null hypothesis that no significant differences would occur in the mRNA-expression levels of some factors associated with the HIF pathway in PDL between control rats and IH rats was rejected: IH enhanced osteogenesis in the mandibular M1 region in association with VEGF, ALP, and BMP-2 gene up-regulation in PDL tissues. These molecules are abundantly expressed in osteoblasts and promote angiogenesis in association with osteogenesis via the HIF-1α pathway (Mamalis and Cochran, 2011).
Previously, we reported that morphological changes occur in craniofacial bone after IH exposure in growing rats. IH suppressed development of the nasal cavity and decreased the size of the mandibular and viscerocranial bones, which could have disturbed nasal breathing (Kuma et al., 2014; Oishi et al., 2015). Oxygen is indispensable for enzymatic reactions to promote tissue development, whereas HIF and VEGF play pivotal survival roles under hypoxic conditions (Maes et al., 2012). In the elderly, it was reported that OSA is associated with an increase in BMD, which suggests that IH can stimulate bone remodeling (Sforza et al., 2013). Moreover, activation of the HIF pathway by hypoxia-mimicking agents prevents bone loss in estrogen-deficient mice, but increases BMD and trabecular microarchitecture (Peng et al., 2014). Data from the present study revealed enhanced BMD and bone development in the alveolar bone proper in the mandibular M1 region after IH exposure (Figures 2, 3). The 3 weeks of IH exposure starting at the age of 7 weeks in rats reflects pre-puberal development (Sengupta, 2013). Given that remodeling of inter-radicular alveolar bone, such as alveolar bone proper, in the mandibular M1 region is promoted by signals from PDL tissues (Kaku and Yamauchi, 2014), we considered that IH induced the peri-M1 changes via enhanced coupling in the signaling pathways for osteogenesis and angiogenesis in the PDL.
HIF-1α, a member of the HIF subfamily, is a ubiquitously expressed transcription factor that regulates cellular adaptation under hypoxia (Liu and Simon, 2004). VEGF is transcriptionally activated by HIF and positively regulates angiogenesis (Miyagawa et al., 2009; Wan et al., 2010). Previous findings have indicated that HIF-1α promotes both angiogenesis and osteogenesis via VEGF upregulation in osteoblasts (Wang et al., 2007). In addition, it was reported that VEGF stimulates the differentiation and chemotactic migration of osteoblastic cells (Hankenson et al., 2015), whereas HIFs and VEGF are involved in skeletal development and bone homeostasis (Wan et al., 2010; Maes et al., 2012). IH stimulates vessel network formation and VEGF production in a highly correlated fashion (Ehsan and George, 2015). The vascular system not only supplies nutrients and oxygen to developing bones, but also delivers critical signals that stimulate mesenchymal cell differentiation toward an osteogenic phenotype, whereas HIF triggers the initiation and promotion of angiogenic-osteogenic cascade events (Mamalis and Cochran, 2011). It was also shown that hypoxia promotes VEGF production in PDL cells (Motohira et al., 2007) and that the PDL plays an important role in tooth eruption (Kaku and Yamauchi, 2014). Consistent with these findings, we found increased HIF-1α and VEGF mRNA expression in peri-M1 PDL tissues after IH exposure (Figure 4), and the lack of change observed in the PDL space (Figure 6) suggests that, not mechanical force, but chemical stimulation related to HIF-1α from PDL tissues induced peri-M1 osteogenesis. Collectively, our findings indicate that HIF-1α mediated VEGF induction in PDL tissues and that VEGF induced peri-M1 osteogenesis.
We evaluated osteogenesis by studying ALP and BMP-2 expression. Given that ALP is a well-known indicator of osteoblastic differentiation, ALP expression indicates the presence of osteoblasts and osteogenic activity (Kuru et al., 1999; Nettelhoff et al., 2016). BMP-2, a member of the transforming growth factor beta superfamily, induces osteogenesis from PDL cells and the regeneration of alveolar bone by promoting osteoblastic differentiation (Selvig et al., 2002). We found significantly higher ALP and BMP-2 expression levels in PDL tissues in rats after IH exposure (Figure 5), which are thought to induce osteogenesis in the alveolar bone proper.
Cephalometric analysis indicated small mandibular sizes and posterior displacements in patients with OSA (Rivlin et al., 1984). Children with OSA present with increased over jet, reduced over bite, narrowed upper dental arches, and shorter lower dental arches (Pirilä-Parkkinen et al., 2009). Oral appliances and mandibular-advancement devices have been used to treat abnormal craniofacial development, such as morphological changes and intermaxillary relations in patients with OSA (Almeida et al., 2006; Hou et al., 2006). It has been suggested that a priori changes in craniofacial bones induce pathogenesis in patients with OSA.
Data from previous reports have indicated that the morphologies of mandibular and dental changes play primary roles in the development of OSA pathophysiology, whereas the present data indicated that the changes of molecular mechanism about PDL tissues in IH rat model. The osteogenesis–angiogenesis coupling phenomenon with HIF-1α and VEGF was involved in the increased BMD observed in developing alveolar bone under IH. Collectively, our data demonstrated for the first time that short periods of IH exposure can enhance peri-M1 abnormally osteogenesis via the HIF-1α-VEGF pathway in growing rats. Similar mechanisms may occur in the craniofacial bones in young children under IH exposure. Our results suggested the necessity of early treatment in children with OSA to maintain normal bone growth. Furthermore, we also demonstrated the usefulness of this IH model in studying the molecular mechanism underlying the morphological changes occurring in craniofacial bones in children with OSA. Although IH has been strongly implicated in OSA pathogenesis, OSA is associated with multifactorial pathogenesis, such as hypercapnia, intrathoracic negative pressure, and sympathetic overactivation (Fletcher, 2001). IH exposure as a single pathogenesis of OSA, a limitation of this study, is that the morphological changes observed in rats may differ somewhat from those that occur in children with OSA; thus, further morphological studies on pediatric OSA are required. Further study is also expected under different experimental settings, involving differences in IH exposure and age.
In conclusion, we demonstrated that IH increases BMD in alveolar bone proper around the roots of the first mandibular molar. Our data suggest the involvement of an osteogenesis–angiogenesis coupling phenomenon with HIF-1α and VEGF in PDL tissues. IH was found to significantly increase BMD and alter bone microstructure, potential risk factors for homeostasis disturbance in alveolar bone proper in growing IH rats. The expression levels of HIF-1α, VEGF, ALP, and BMP-2 transcripts were up-regulated in PDL tissues subjected to IH exposure for 3 weeks. Although the signaling pathway underlying IH-induced changes in the bony microstructure is not yet fully elucidated, these findings improve our current understanding of the molecular mechanisms underlying the impacts of IH on bone homeostasis.
Author Contributions
SO, YS, JH, YK, HM, HN, RU, SK, NS, JS, KY, and TO conceived of and designed the study; SO performed the experiments; SO, YS, JH, and TO analyzed the data; SO, YS, JH, YK, HM, HN, RU, SK, NS, JS, KY, and TO interpreted the results of the experiments; SO and YS prepared the figures; SO drafted the manuscript; YS, JH, JS, and TO edited the manuscript; All authors approved the final version of the manuscript.
Funding
This study was financially supported in part by Grants-in-Aid for Scientific Research (25463170, 26463089) from the Japanese Ministry of Education, Culture, Sports, Science, and Technology (KAKENHI).
Conflict of Interest Statement
The authors declare that the research was conducted in the absence of any commercial or financial relationships that could be construed as a potential conflict of interest.
References
Afanador, E., Yokozeki, M., Oba, Y., Kitase, Y., Takahashi, T., Kudo, A., et al. (2005). Messenger RNA expression of periostin and Twist transiently decrease by occlusal hypofunction in mouse periodontal ligament. Arch. Oral Biol. 50, 1023–1031. doi: 10.1016/j.archoralbio.2005.04.002
Almeida, F. R., Lowe, A. A., Sung, J. O., Tsuiki, S., and Otsuka, R. (2006). Long-term sequellae of oral appliance therapy in obstructive sleep apnea patients: part 1. Cephalometric analysis. Am. J. Orthod. Dentofacial. Orthop. 129, 195–204. doi: 10.1016/j.ajodo.2005.10.001
Balbani, A. P., Weber, S. A., and Montovani, J. C. (2005). Update in obstructive sleep apnea syndrome in children. Braz. J. Otorhinolaryngol. 71, 74–80. doi: 10.1016/S1808-8694(15)31288-X
Chen, C. C., Kanno, Z., and Soma, K. (2005). Occlusal forces promote periodontal healing of transplanted teeth with enhanced nitric oxide synthesis. J. Med. Dent. Sci. 52, 59–64.
Cheung, C. C., Thornton, J. E., Nurani, S. D., Clifton, D. K., and Steiner, R. A. (2001). A reassessment of leptin's role in triggering the onset of puberty in the rat and mouse. Neuroendocrinology 74, 12–21. doi: 10.1159/000054666
Ehsan, S. M., and George, S. C. (2015). Vessel network formation in response to intermittent hypoxia is frequency dependent. J. Biosci. Bioeng. 120, 347–350. doi: 10.1016/j.jbiosc.2015.01.017
Fletcher, E. C. (2001). Invited review: physiological consequences of intermittent hypoxia: systemic blood pressure. J. Appl. Physiol. 90, 1600–1605.
Hadjidakis, D. J., and Androulakis, I. I. (2006). Bone remodeling. Ann. N. Y. Acad. Sci. 1092, 385–396. doi: 10.1196/annals.1365.035
Hankenson, K. D., Gagne, K., and Shaughnessy, M. (2015). Extracellular signaling molecules to promote fracture healing and bone regeneration. Adv. Drug Deliv. Rev. 94, 3–12. doi: 10.1016/j.addr.2015.09.008
Hou, H. M., Sam, K., Hägg, U., Rabie, A. B., Bendeus, M., Yam, L. Y., et al. (2006). Long-term dentofacial changes in Chinese obstructive sleep apnea patients after treatment with a mandibular advancement device. Angle Orthod. 76, 432–440. doi: 10.1043/0003-3219(2006)076[0432:LDCICO]2.0.CO;2
Hsu, Y. H., Niu, T., Terwedow, H. A., Xu, X., Feng, Y., Li, Z., et al. (2006). Variation in genes involved in the RANKL/RANK/OPG bone remodeling pathway are associated with bone mineral density at different skeletal sites in men. Hum. Genet. 118, 568–577. doi: 10.1007/s00439-005-0062-4
Huang, Y. S., and Guilleminault, C. (2012). Pediatric obstructive sleep apnea and the critical role of oral-facial growth: evidences. Front. Neurol. 3:184. doi: 10.3389/fneur.2012.00184
Kaku, M., and Yamauchi, M. (2014). Mechano-regulation of collagen biosynthesis in periodontal ligament. J. Prosthodont. Res. 58, 193–207. doi: 10.1016/j.jpor.2014.08.003
Kaneko, S., Ohashi, K., Soma, K., and Yanagishita, M. (2001). Occlusal hypofunction causes changes of proteoglycan content in the rat periodontal ligament. J. Periodontal. Res. 36, 9–17. doi: 10.1034/j.1600-0765.2001.00607.x
Kuma, Y., Usumi-Fujita, R., Hosomichi, J., Oishi, S., Maeda, H., Nagai, H., et al. (2014). Impairment of nasal airway under intermittent hypoxia during growth period in rats. Arch. Oral Biol. 59, 1139–1145. doi: 10.1016/j.archoralbio.2014.06.005
Kuru, L., Griffiths, G. S., Petrie, A., and Olsen, I. (1999). Alkaline phosphatase activity is upregulated in regenerating human periodontal cells. J. Periodontal. Res. 34, 123–127. doi: 10.1111/j.1600-0765.1999.tb02231.x
Lal, C., Strange, C., and Bachman, D. (2012). Neurocognitive impairment in obstructive sleep apnea. Chest 141, 1601–1610. doi: 10.1378/chest.11-2214
Li, H., Bartold, P. M., Young, W. G., Xiao, Y., and Waters, M. J. (2001). Growth hormone induces bone morphogenetic proteins and bone-related proteins in the developing rat periodontium. J. Bone Miner. Res. 16, 1068–1076. doi: 10.1359/jbmr.2001.16.6.1068
Li, R., Li, X., Zhou, M., Han, N., and Zhang, Q. (2012). Quantitative determination of matrix Gla protein (MGP) and BMP-2 during the osteogenic differentiation of human periodontal ligament cells. Arch. Oral Biol. 57, 1408–1417. doi: 10.1016/j.archoralbio.2012.07.005
Liu, L., and Simon, M. C. (2004). Regulation of transcription and translation by hypoxia. Cancer. Biol. Ther. 3, 492–497. doi: 10.4161/cbt.3.6.1010
Livak, K. J., and Schmittgen, T. D. (2001). Analysis of relative gene expression data using real-time quantitative PCR and the 2(-Delta Delta C(T)) method. Methods 25, 402–408. doi: 10.1006/meth.2001.1262
Luan, X., Ito, Y., Holliday, S., Walker, C., Daniel, J., Galang, T. M., et al. (2007). Extracellular matrix-mediated tissue remodeling following axial movement of teeth. J. Histochem. Cytochem. 55, 127–140. doi: 10.1369/jhc.6A7018.2006
Maeda, H., Nagai, H., Takemura, G., Shintani-Ishida, K., Komatsu, M., Ogura, S., et al. (2013). Intermittent-hypoxia induced autophagy attenuates contractile dysfunction and myocardial injury in rat heart. Biochim. Biophys. Acta 1832, 1159–1166. doi: 10.1016/j.bbadis.2013.02.014
Maes, C., Carmeliet, G., and Schipani, E. (2012). Hypoxia-driven pathways in bone development, regeneration and disease. Nat. Rev. Rheumatol. 8, 358–366. doi: 10.1038/nrrheum.2012.36
Mamalis, A. A., and Cochran, D. L. (2011). The therapeutic potential of oxygen tension manipulation via hypoxia inducible factors and mimicking agents in guided bone regeneration. A review. Arch. Oral. Biol. 56, 1466–1475. doi: 10.1016/j.archoralbio.2011.05.001
Mayahara, K., Yamaguchi, A., Takenouchi, H., Kariya, T., Taguchi, H., and Shimizu, N. (2012). Osteoblasts stimulate osteoclastogenesis via RANKL expression more strongly than periodontal ligament cells do in response to PGE(2). Arch. Oral Biol. 57, 1377–1384. doi: 10.1016/j.archoralbio.2012.07.009
Miyagawa, A., Chiba, M., Hayashi, H., and Igarashi, K. (2009). Compressive force induces VEGF production in periodontal tissues. J. Dent. Res. 88, 752–756. doi: 10.1177/0022034509341637
Motohira, H., Hayashi, J., Tatsumi, J., Tajima, M., Sakagami, H., and Shin, K. (2007). Hypoxia and reoxygenation augment bone-resorbing factor production from human periodontal ligament cells. J. Periodontol. 78, 1803–1809. doi: 10.1902/jop.2007.060519
Muramoto, T., Takano, Y., and Soma, K. (2000). Time-related changes in periodontal mechanoreceptors in rat molars after the loss of occlusal stimuli. Arch. Histol. Cytol. 63, 369–380. doi: 10.1679/aohc.63.369
Nagai, H., Kuwahira, I., Schwenke, D. O., Tsuchimochi, H., Nara, A., Ogura, S., et al. (2015). Pulmonary macrophages attenuate hypoxic pulmonary vasoconstriction via beta3AR/iNOS pathway in rats exposed to chronic intermittent hypoxia. PLoS ONE 10:e0131923. doi: 10.1371/journal.pone.0131923
Nettelhoff, L., Grimm, S., Jacobs, C., Walter, C., Pabst, A. M., Goldschmitt, J., et al. (2016). Influence of mechanical compression on human periodontal ligament fibroblasts and osteoblasts. Clin. Oral Investig. 20, 621–629. doi: 10.1007/s00784-015-1542-0
Noda, A., Ito, R., Okada, T., Yasuma, F., Nakashima, N., and Yokota, M. (1998). Twenty-four-hour ambulatory oxygen desaturation and electrocardiographic recording in obstructive sleep apnea syndrome. Clin. Cardiol. 21, 506–510. doi: 10.1002/clc.4960210710
Oishi, S., Shimizu, Y., Hosomichi, J., Kuma, Y., Nagai, H., Maeda, H., et al. (2015). Intermittent hypoxia induces disturbances in craniofacial growth and defects in craniofacial morphology. Arch. Oral Biol. 61, 115–124. doi: 10.1016/j.archoralbio.2015.10.017
Peng, J., Lai, Z. G., Fang, Z. L., Xing, S., Hui, K., Hao, C., et al. (2014). Dimethyloxalylglycine prevents bone loss in ovariectomized C57BL/6J mice through enhanced angiogenesis and osteogenesis. PLoS ONE 9:e112744. doi: 10.1371/journal.pone.0112744
Pirilä-Parkkinen, K., Pirttiniemi, P., Nieminen, P., Tolonen, U., Pelttari, U., and Löppönen, H. (2009). Dental arch morphology in children with sleep-disordered breathing. Eur. J. Orthod. 31, 160–167. doi: 10.1093/ejo/cjn061
Riddle, R. C., Khatri, R., Schipani, E., and Clemens, T. L. (2009). Role of hypoxia-inducible factor-1alpha in angiogenic-osteogenic coupling. J. Mol. Med. 87, 583–590. doi: 10.1007/s00109-009-0477-9
Rivlin, J., Hoffstein, V., Kalbfleisch, J., McNicholas, W., Zamel, N., and Bryan, A. C. (1984). Upper airway morphology in patients with idiopathic obstructive sleep apnea. Am. Rev. Respir. Dis. 129, 355–360.
Schipani, E., Maes, C., Carmeliet, G., and Semenza, G. L. (2009). Regulation of osteogenesis-angiogenesis coupling by HIFs and VEGF. J. Bone Miner. Res. 24, 1347–1353. doi: 10.1359/jbmr.090602
Selvig, K. A., Sorensen, R. G., Wozney, J. M., and Wikesjö, U. M. (2002). Bone repair following recombinant human bone morphogenetic protein-2 stimulated periodontal regeneration. J. Periodontol. 73, 1020–1029. doi: 10.1902/jop.2002.73.9.1020
Sengupta, P. (2013). The Laboratory rat: relating its age with human's. Int. J. Prev. Med. 4, 624–630.
Sforza, E., Thomas, T., Barthélémy, J. C., Collet, P., and Roche, F. (2013). Obstructive sleep apnea is associated with preserved bone mineral density in healthy elderly subjects. Sleep 36, 1509–1515. doi: 10.5665/sleep.3046
Shimizu, Y., Hosomichi, J., Nakamura, S., and Ono, T. (2014). Micro-computed tomography analysis of changes in the periodontal ligament and alveolar bone proper induced by occlusal hypofunction of rat molars. Korean J. Orthod. 44, 263–267. doi: 10.4041/kjod.2014.44.5.263
Skelly, J. R., Edge, D., Shortt, C. M., Jones, J. F., Bradford, A., and O'Halloran, K. D. (2012). Respiratory control and sternohyoid muscle structure and function in aged male rats: decreased susceptibility to chronic intermittent hypoxia. Respir. Physiol. Neurobiol. 180, 175–182. doi: 10.1016/j.resp.2011.11.004
Spence, J. M. (1940). Method of studying the skull development of the living rat by serial cephalometric roentgenograms. Angle. Orthod. 10, 127–139.
Wan, C., Shao, J., Gilbert, S. R., Riddle, R. C., Long, F., Johnson, R. S., et al. (2010). Role of HIF-1alpha in skeletal development. Ann. N. Y. Acad. Sci. 1192, 322–326. doi: 10.1111/j.1749-6632.2009.05238.x
Wang, Y., Li, Y. P., Paulson, C., Shao, J. Z., Zhang, X., Wu, M., et al. (2014). Wnt and the Wnt signaling pathway in bone development and disease. Front. Biosci. 19, 379–407. doi: 10.2741/4214
Keywords: bone mineral density, hypoxia inducible factor, intermittent hypoxia, periodontal ligament, vascular endothelial growth factor
Citation: Oishi S, Shimizu Y, Hosomichi J, Kuma Y, Maeda H, Nagai H, Usumi-Fujita R, Kaneko S, Shibutani N, Suzuki J-i, Yoshida K-i and Ono T (2016) Intermittent Hypoxia Influences Alveolar Bone Proper Microstructure via Hypoxia-Inducible Factor and VEGF Expression in Periodontal Ligaments of Growing Rats. Front. Physiol. 7:416. doi: 10.3389/fphys.2016.00416
Received: 19 July 2016; Accepted: 05 September 2016;
Published: 16 September 2016.
Edited by:
Thimios Mitsiadis, University of Zurich, SwitzerlandReviewed by:
Harald Osmundsen, University of Oslo, NorwaySupawadee Sukseree, Medical University of Vienna, Austria
Copyright © 2016 Oishi, Shimizu, Hosomichi, Kuma, Maeda, Nagai, Usumi-Fujita, Kaneko, Shibutani, Suzuki, Yoshida and Ono. This is an open-access article distributed under the terms of the Creative Commons Attribution License (CC BY). The use, distribution or reproduction in other forums is permitted, provided the original author(s) or licensor are credited and that the original publication in this journal is cited, in accordance with accepted academic practice. No use, distribution or reproduction is permitted which does not comply with these terms.
*Correspondence: Jun Hosomichi, hosomichi.orts@tmd.ac.jp