- Chart Biotech Consulting, LLC, Erie, CO, United States
Introduction: Development of hemoglobin-based oxygen carriers (HBOCs) for use as temporary blood replacement solutions and treatment of hemorrhagic shock has been hindered because of evidence HBOC infusion increases the risk of myocardial infarction (MI).
Methods: To gain insight into potential toxicity mechanisms, MI incidence from later stage clinical testing of five HBOCs was compared to pharmacokinetic and biochemical parameters to identify correlations suggestive of cause-and-effect hypotheses.
Results: There are positive correlations between MI incidence and HBOC dose, size, intravascular half-life and area under the plasma concentration versus time curve (AUC). Furthermore, MI incidence is positively correlated with initial rates of HBOC autoxidation, oxidation by nitric oxide, and AUCs estimated for these HBOC oxidation products.
Conclusions: These observations imply that increased MI risk after HBOC infusion is due to intravascular reactions which exacerbate oxidative stress.
Introduction
Hemoglobin-based oxygen carriers (HBOCs) have been under development for several decades to improve trauma resuscitation outcomes and provide alternative oxygen transport solutions when blood is not available (Liu et al., 2022). While the efficacy of such solutions has been demonstrated in preclinical studies and human patients, regulatory approval has yet to be obtained in most countries due to concerns about serious adverse events (Estep, 2019). Such concerns were crystallized by a meta-analysis demonstrating increased risk of mortality and myocardial infarction (MI) after HBOC infusion (Natanson et al., 2008).
To develop hypotheses as to potential mechanism(s) of MI risk enhancement, the preclinical literature on HBOC toxicity and clinical data collected during later stage testing were reviewed. Both in vitro and in vivo experiments suggest that HBOCs may increase toxicity by exacerbating oxidative stress in a synergistic fashion with other insults (Alayash, 2019), particularly with respect to the endothelium (Biro, 2012). There is also an extensive clinical literature implicating oxidative stress as a risk factor for MI (Wang and Kang, 2020).
No single clinical trial performed with HBOCs was adequately powered to assess differences in serious adverse events on the order of a few percent. Thus, aggregation of data is required to identify significant correlations (Natanson et al., 2008). In the present analysis, the ratio of MI incidence in treated versus control patients was calculated from published clinical data for four crosslinked and/or polymerized HBOCs (CP HBOCs), HemAssist™, Hemolink™, Hemopure® and Polyheme™, and one polyethylene derivatized hemoglobin HBOC (PEG HBOC), Hemospan® (also denoted as MP-4) (Przybelski et al., 1999; Sloan et al., 1999; Garrioch et al., 1999; Lamy et al., 2000; Schubert et al., 2002; Kerner et al., 2003; Bloomfield et al., 2004; Schubert et al., 2003; Cheng, 2001; Hill et al., 2002; Cheng et al., 2002; Greenburg and Kim, 2004; Jahr et al., 2008; Kasper et al., 1996; Kasper et al., 1998; Standl et al., 1998; LaMuraglia et al., 2000; Levy et al., 2002; Sprung et al., 2002; Hemelrijck et al., 2014; Gould et al., 1998; Northfield Laboratories, 2017; Moore et al., 2009; Olofsson et al., 2006; Olofsson et al., 2008; Olofsson et al., 2011; Van der Linden et al., 2011), and compared to a variety of parameters (Meng et al., 2018) to characterize the pharmacokinetics and toxicodynamics with respect to MI. All of the evaluated HBOCs use mammalian tetrameric hemoglobin as the oxygen transporting component, four human and one (Hemopure) bovine (Table 1). Due to their similarities with respect to the structure of the hemoglobin starting material, size of chemical modification reagents, rates of reaction with nitric oxide (NO), and oxygen binding characteristics, the CP HBOCs were analyzed as a subgroup. Hemospan is neither crosslinked nor polymerized. In addition, to better define correlations with specific reaction products, a mathematical model of the evolution of total, reduced, autoxidized and nitic oxide oxidized HBOC species was developed to identify the most important reactions in the etiology of MI risk enhancement.
Methods
Calculation of MI incidence ratio
After an extensive literature search, MI data were tabulated from all of the randomized, controlled Phase II and III clinical trials (RCTs) of HBOCs used in the treatment of surgical blood loss or trauma resuscitation wherein the total patients in each of the treated and control groups exceeded 200. The five HBOCs meeting these criteria are noted in the Introduction. While adjudicated MI data were reported in one clinical trial (Moore et al., 2009), only the MI incidences as assessed by the physicians directly treating patients were used in this analysis for consistency of comparison. The MI ratio for each HBOC was calculated by dividing the MI incidence rate in treated patients by the incidence rate in controls (Table 1). This procedure was used to adjust for the facts that differing numbers of patients were enrolled in treated and control groups and the variation of MI incidence between different control patient populations was significant.
Calculation of average HBOC dose and size
Average HBOC doses ([Hb]0, g/kg) were calculated as number weighted averages of the average doses utilized in RCTs with a particular HBOC. When doses were not reported as g/kg, they were estimated by division of the average total dose by average patient weight. If average patient weights were not reported, they were estimated using continent/country specific averages (North America 80.7 kg, Europe 70.8 kg South Africa 73.0 kg) (Walpole et al., 2012; WorldData.info., 2023). The average dose of Polyheme in a trial using repetitive stepwise hemodilution was corrected for the estimated product loss due to blood removal after the first HBOC dose was administered. Average doses in g/kg were converted to heme concentration (mM/L) by multiplying by the conversion factor 1.47, which assumes a plasma volume of 42 mL/kg. Average molecular size (radius of gyration, Rg) was based on published values (Vandegriff et al., 1997; Vandegriff et al., 2003) or estimated by assuming Rg is equivalent to a linear right circular cylinder composed of the number of hemoglobin tetramers equivalent to the average molecular weight listed in Table 1. This method was selected amongst several evaluated because it accurately reproduced the measured Rg for Hemolink (5.0 nm calculated versus 4.9 measured).
Calculation of estimated AUC
Assuming that HBOC plasma clearance is adequately described as a single exponential decay (Olofsson et al., 2006; Olofsson et al., 2008; Estep, 2019; Carmichael et al., 2000; Swan et al., 1995; O’Hare et al., 2001; Hughes et al., 1996), integration leads to the expression:
where T1/2 is the circulatory half-life. The functional dependence of T1/2 on [Hb]0 was estimated for each HBOC (Table 2; Figure 1) as the best linear fit to published T1/2 versus dose data (Standl et al., 1998; Olofsson et al., 2006; Olofsson et al., 2008; Carmichael et al., 2000; Przybelski et al., 1996; Swan et al., 1995; O’Hare et al., 2001; Hughes et al., 1995; Hughes et al., 1996). The resulting equation was substituted into (Equation 1) to yield an estimated AUC as a quadratic function of [Hb]0 of the form:
where A and B are coefficients derived from the best linear fit to the T1/2 versus dose plots. Due to the lack of T1/2 versus dose data for Polyheme, the corresponding dependence for Hemopure was utilized, since Hemopure is the most similar HBOC of those evaluated to Polyheme with respect to overall structure.
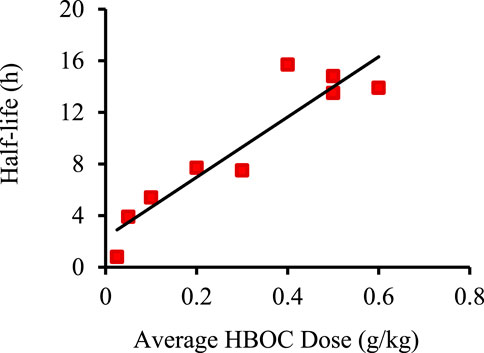
Figure 1. Example of best linear fit of HBOC plasma half-life versus dose using data for Hemolink. Data taken from (Carmichael et al., 2000). Linear best fit was determined using the Excel data analysis package.
Sources and calculations of other HBOC biophysical and biochemical parameters
HBOC biophysical and biochemical parameters were taken from the compendium of Meng and coworkers (Meng et al., 2018; Table 3). Initial HBOC autoxidation reaction rates were estimated by multiplying the autoxidation rate constant (ka) by [Hb]0. The initial rate of hemoglobin reaction with nitric oxide (NO) was calculated by multiplying [Hb]0 with the corresponding reaction rate constant (kN) assuming an initial NO concentration of 1 nM, a value within the range of plasma NO concentrations derived from multiple studies (Hall and Garthwaite, 2009). Quasi steady-state NO concentrations were calculated using an average NO synthesis rate of 1.7 mmoles/day (Hall and Garthwaite, 2009). Assuming that half is secreted into an average of 3 L of plasma, this equates to approximately 0.01 mM/L/h of NO secretion. Due to the rapidity of the hemoglobin reaction with NO, it was assumed that any secreted NO reacts immediately with plasma HBOC. These assumptions lead to the result that the steady state concentration of NO after the administration of each HBOC, ([NO]ss) is given by:
Rate constants for overall HBOC removal at the average administered dose (kT) were calculated as 0.693/T1/2, where T1/2 was calculated as described above. The results of these calculations are shown in Table 3.
Comparison of MI ratio to pharmaceutical, biophysical, and biochemical parameters
The MI ratio for all five HBOCs or the CP HBOCs was graphically compared to various independent variables and evaluated for best fit to linear and quadratic functions utilizing the EXCEL data analysis package (Table 4).
Calculation of expected component AUC values
A mathematical model of the variation in time of the concentrations of reduced, autoxidized, and NO oxidized HBOCs was constructed assuming that autoxidation and overall HBOC removal could be described by first order rate constants and the reaction with NO was equal to the NO secretion rate. The contribution of methemoglobin reduction reactions was assumed to be negligible and the kinetics of overall HBOC removal from circulation was assumed to be the same for reduced and oxidized hemoglobin (Snyder et al., 1987; Vandegriff et al., 2006)). For simplicity, the starting metHBOC concentration was assumed to be zero. These assumptions yield the following rate equations:
where [Hb]r, [Hb]a, and [Hb]N are the concentrations of reduced, autoxidized and NO oxidized HBOC, respectively; ka and kT are the first order rate constants for HBOC autoxidation and overall HBOC removal from plasma, respectively; and R is the rate of NO secretion into plasma.
The solutions to these equations are (Ritger and Rose, 1968):
where K = ka + kT. In integrating these equations to give the AUC for each HBOC, it is noted that Equation 2 will equal zero at a finite time, t0, given by:
Therefore, to calculate the AUC for [Hb]r, Equation 2 was integrated from t = 0 to t = t0 to yield:
Time t0 is also the time at which the generation of autoxidized or NO oxidized HBOC will stop, since there is no more reduced hemoglobin substrate for these reactions. The concentrations of these species at t0, denoted as [Hb(t0)]a and [Hb(t0)]N, respectively, are then assumed to decrease with a first order exponential decay with a kT rate constant. The AUCs for [Hb]a and [Hb]N are therefore given by the integral of Equations (3) and (4) from t = 0 to t = t0 plus the integral of the exponential decay of these HBOC species from t = t0 to infinity:
Note that for R = 0 these equations simplify to:
Comparison of model predictions to AUCs determined from clinical data
To compare model predictions with actual clinical data, total HBOC and metHBOC AUC values were obtained using the data measuring tool in Adobe Acrobat applied to data from a dose escalation study of Hemospan (Olofsson et al., 2006). Model predicted values for these paraments were then calculated utilizing various autoxidation rate constants and NO secretion rates (Table 5). These calculations assumed an initial plasma heme concentration of 0.418 mM/L which was calculated to result from the infusion of the 0.27 g/kg HBOC, a dose used for two of the patient cohorts (n = 4 each) in this trial. These cohorts were chosen for comparison in part because the initial plasma HBOC concentration was closest to that of the 0.35 mM/L average estimated for all of the later stage Hemospan clinical trial data and because the use of two cohorts gives the largest number of total data points. Total AUC predictions were also compared with data published from a HemAssist clinical trial (O’Hare et al., 2001) in the same manner.
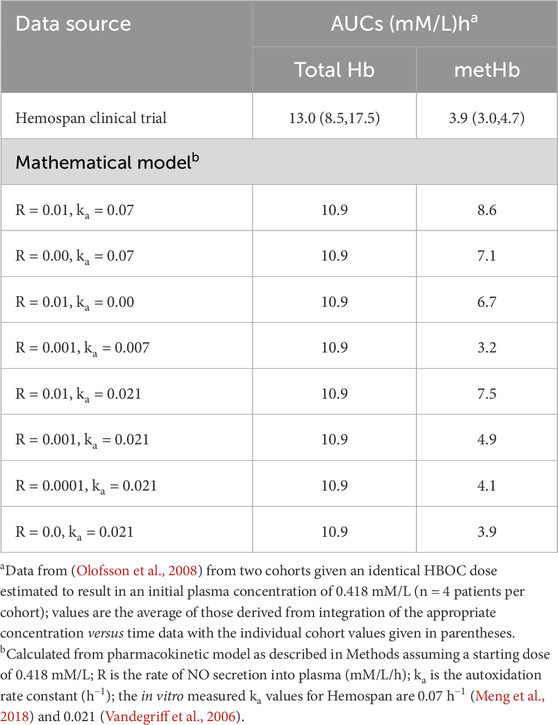
Table 5. Comparison of total and methemoglobin AUCs estimated from Hemospan clinical trial data and calculated using the mathematical model with different assumed values for NO secretion into plasma and autoxidation rate constants.
Comparison of calculated AUCs with MI ratios
Estimated AUCs of reduced, autoxidized, NO oxidized, total, and total oxidized HBOC species were calculated for all five HBOCs at the average doses evaluated in clinical testing, using three combinations of autoxidation rate constants and NO secretion rates (Table 6). These AUC values were then compared to the corresponding HBOC MI ratios (Table 7).
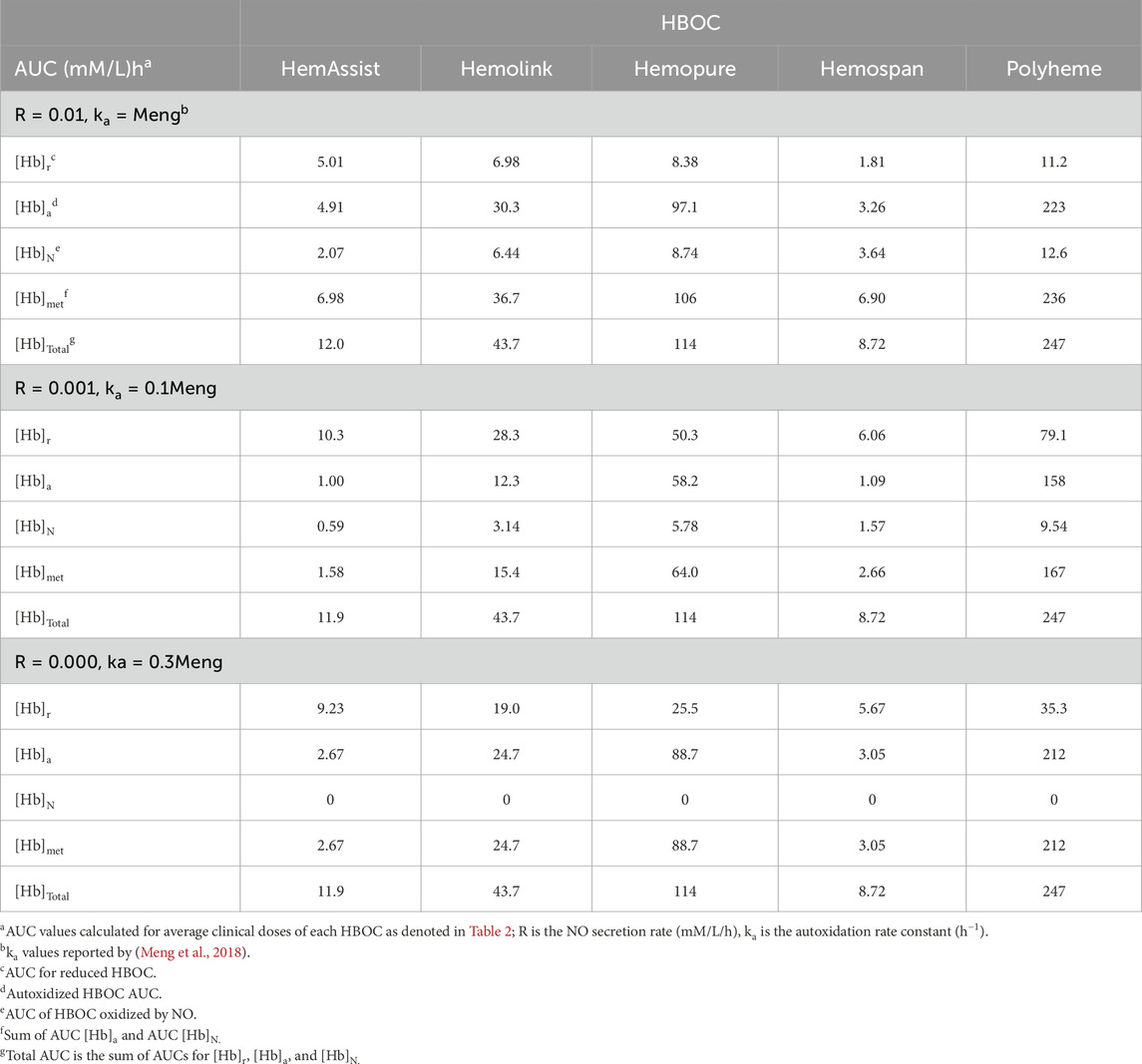
Table 6. AUC values predicted by a mathematical model of HBOC oxidation and removal from plasma for differing autoxidation rate constants and NO secretion rates.
Statistical analysis
Incidence rate means and standard deviations for treated and control patients were calculated along with the 99% confidence intervals using a modified Wald method (Motulsky, 2010). Statistical data with respect to best fits of MI versus various parameters were taken from the EXCEL data analysis package. All p values are two-tailed obtained by doubling the values reported in the EXCEL analysis.
Results
Comparison of MI incidence ratio with HBOC dose, size, plasma half-life and AUC
HBOC properties (Table 1), pharmacokinetic data (Tables 2), and estimated in vivo reaction rates for oxidative reactions and overall HBOC removal from plasma (Table 3) were summarized, and comparisons made between MI incidence ratios and various independent variables by regression analysis (Table 4; Figure 2). There is a significant (R2 = 0.9994, p < 0.05) positive correlation between MI ratio and dose for the four CP HBOCs, with the best fit being a quadratic function. There is also a positive correlation (R2 = 0.9649) between MI and HBOC size. These two correlations confirm the results from a preliminary analysis performed with a smaller, less refined data set (Estep, 2019). Since HBOC size correlates positively with intravascular half-life over the molecular weight range encompassed by the evaluated HBOCs (Berbers et al., 1991; Bleeker et al., 1992; Keipert et al., 1992; Hsia et al., 1992; Conover et al., 1997; Wicks et al., 2003; Estep, 2015; Taguchi et al., 2017), MI incidence was compared with this parameter as well (Table 4; Figure 2C) using estimated HBOC half-lives derived from the best linear fits to published dose versus half-life data. Here again a positive correlation was observed, although this did not reach statistical significance (R2 = 0.9961, p = 0.12). Collectively, these data suggest that the total exposure of blood and endothelium to HBOCs as reflected in the area under the HBOC plasma concentration versus time curve (AUC) is important, since dose and intravascular persistence are the primary determinants of AUC. No AUC data were reported for HBOCs in Phase II or III clinical trials, but this parameter can be estimated as described in Methods as a quadratic function of dose (Table 3). When MI incidence is compared with these AUC values (Table 4; Figure 2D), the correlation for the CP HBOCs (R2 = 0.9999, p = < 0.03) is stronger than with either dose, size or half-life alone. Note also that, by virtue of how the MI ratio is defined, the y intercept should approach 1.0 as dose or AUC approach zero. The y-intercept (1.11) with the AUC versus MI ratio function agrees closely with this expectation. In these comparisons PEG HBOC exhibits a notably higher incidence of MI than CP HBOCs at comparable values of dose or AUC.
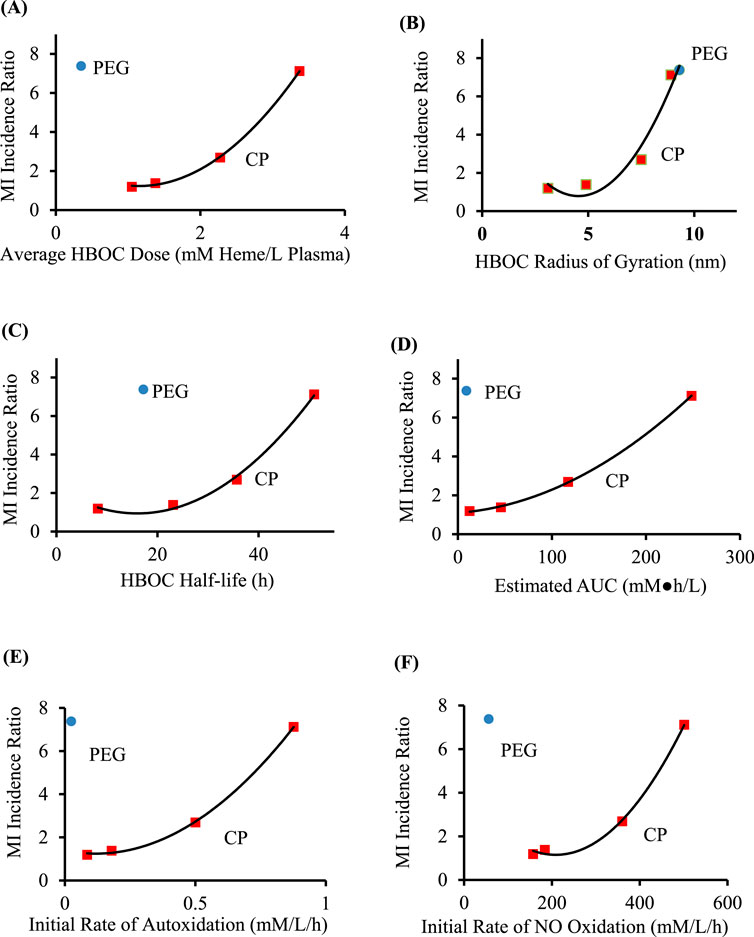
Figure 2. Comparison of MI ratios to average HBOC dose (A), size (B), circulatory half-life (C), estimated AUC (D), initial rate of autoxidation (E), and initial rate of oxidation by NO (F), for crosslinked and polymerized (CP) HBOCs (squares) and Hemospan, a PEG modified HBOC (PEG, circle). The equations of best fit (Table 4) are for the CP HBOCs (n = 4) except for the comparison with average molecular size (n = 5). Data are derived from clinical trials enrolling a total of 206–592 patients in each treated or control group for each HBOC.
Comparison of MI incidence ratio with HBOC biochemical and biophysical properties
To explore potential toxicity mechanisms, MI ratios were compared to several HBOC properties, as well as calculated initial average reaction rates in plasma (Table 4). No significant correlations were observed between the MI ratio and HBOC oxygen half saturation values (P50), or the rate constants for autoxidation, reaction with nitric oxide (NO), or overall HBOC removal from circulation, or the initial rate of overall HBOC removal from circulation. Positive correlations between MI and initial autoxidation and NO oxidation rates were high, although they did not reach statistical significance (Table 4; Figures 2E,F). It is also recognized that the initial oxidation rate by NO would only persist for a short period of time because the millimolar concentration of HBOC would rapidly consume NO, driving this concentration down to sub picomolar levels ([NO]ss, Table 3). At this point the reaction rate of HBOC with NO would be expected to equal the rate of NO secretion into plasma. However, due to the fact that the NO reaction rate constant is approximately nine orders of magnitude greater than the autoxidation or hemoglobin removal rate constants, the reaction rate of HBOC oxidation with NO is still comparable to the rates of autoxidation and HBOC plasma clearance, especially at lower HBOC concentrations (Table 3).
Calculation of AUCs for various HBOC species
By analogy with the concept of AUC for the total HBOC concentration, one can also contemplate assessing the relationship between the MI ratio and the AUCs of the products of the two primary HBOC oxidation reactions, autoxidation and oxidation by reaction with NO. Both reactions generate oxidized hemoglobin (metHBOC), with the former reaction also generating superoxide and the latter resulting in the consumption of NO. While it is recognized that metHBOC formed by these two processes cannot be distinguished experimentally, one can conceptually model the contributions to HBOC oxidation by these two reactions with the sum resulting in the experimentally accessible total metHBOC concentration. Therefore, a mathematical model was constructed to predict the expected evolution of these reaction products with time. Key assumptions were that the primary mechanisms for reduced HBOC disappearance are autoxidation, NO oxidation, and overall HBOC removal, with the last of these acting equally on both reduced and oxidized HBOCs (Snyder et al., 1987; Vandegriff et al., 2006). It was further assumed that no oxidized HBOC (metHBOC) is converted to the reduced form, that doses of HBOC were administered as a bolus, and that the reaction rate of HBOC with NO is equal to the rate of NO secretion into plasma. Equations for the concentration of these HBOCs were integrated to provide estimates of their respective AUCs. Several different assumed values for the autoxidation rate constants and NO secretion rates were explored (Tables 5, 6).
Comparison of model predictions with clinical data
To assess the veracity of the mathematical model, predicted AUC values were compared to those calculated from published pharmacokinetic data for Hemospan (Olofsson et al., 2008), as this is the only data set of which the author is aware in which plasma concentrations of both total and metHBOC were reported in detail. Model calculations assumed an initial plasma heme concentration of 0.418 mM/L, corresponding to the dose administered to two cohorts in this dose-response study (Table 5). The integrated clinical data yield AUCs of 8.48 and 17.5 mM•h/L for the two cohorts, a difference which probably reflects the inherent biological variability in these small (n = 4) sample sizes. The predicted total AUC of 10.9 mM•h/L from the mathematical model is between the two values measured from these cohorts, and similar to their average (13.0 mM•h/L), suggesting reasonable agreement. However, the degree of total oxidation predicted by the mathematical model (8.6 mM•h/L) utilizing the data of Meng et al. (Meng et al., 2018) is significantly greater than that observed in the clinical data (3.0 and 4.7 mM•h/L). One possibility is that the difference is a result of lower in vivo oxidation rates compared with those measured in vitro. To explore this possibility, expected values for total oxidized HBOC were calculated assuming that either the autoxidation rate constant or the NO secretion rate were zero. Although the total predicted oxidized HBOC AUC was reduced, in neither case was it reduced to the value measured in vivo (Table 5). Only when both the autoxidation rate constant and the NO secretion rate were simultaneously reduced by a factor of ten did the predicted total metHBOC AUC (3.2 mM•h/L) agree within experimental error with clinical observations. However, other combinations of reductions in autoxidation rates and NO secretion rates are also possible, and the developers of Hemospan reported an autoxidation rate constant approximately 0.3 that of Meng and coworkers (Vandegriff et al., 2006). When this rate constant was combined with the assumption of negligible NO secretion into plasma, good agreement was obtained with the observed total methemoglobin AUC for Hemospan (3.9 mM•h/L for both calculated and measured average values).
The only other clinical data set containing a sufficient number of observations to perform an AUC integration with confidence was a report of the total plasma hemoglobin concentration of HemAssist (O’Hare et al., 2001). The total AUC value predicted from the model (22 mM•h/L) is similar to, but somewhat higher than, that estimated from the clinical data (16 mM•h/L). The absence of sufficient plasma metHBOC values from this and other reported clinical data preclude further comparisons.
Comparison of MI values with predicted AUCs
In light of the results of comparison of the model predictions with Hemospan clinical data, estimated AUC values for the different HBOC components were calculated using three different assumptions for the autoxidation rate constants and NO secretion rates (Tables 6 and 7). The total AUC values predicted by this model are virtually identical to those predicted by the simpler method based on a quadratic function of dose as described in Methods (compare AUC values in Tables 3, 6). The total AUC also does not change when the assumed NO secretion rate and/or the autoxidation rate constants are multiplied by the same factor (Table 6), but the AUC values for differing HBOC components (i.e., reduced, autoxidized, and NO oxidized) do.
Good correlations between the MI ratios and estimated AUCs are obtained for reduced, autoxidized, NO oxidized, total oxidized and total HBOC for both scenarios in which a finite NO secretion rate is assumed, and for each of the AUCs save that for total NO oxidized hemoglobin in the scenario in which NO secretion is assumed to be zero (Table 7, Figure 3). However, taking into account y-intercept values as well as the correlation coefficients and p-values, best agreement is observed with quadratic fits between the MI ratios and autoxidized, total methemoglobin, and total HBOC AUCs.
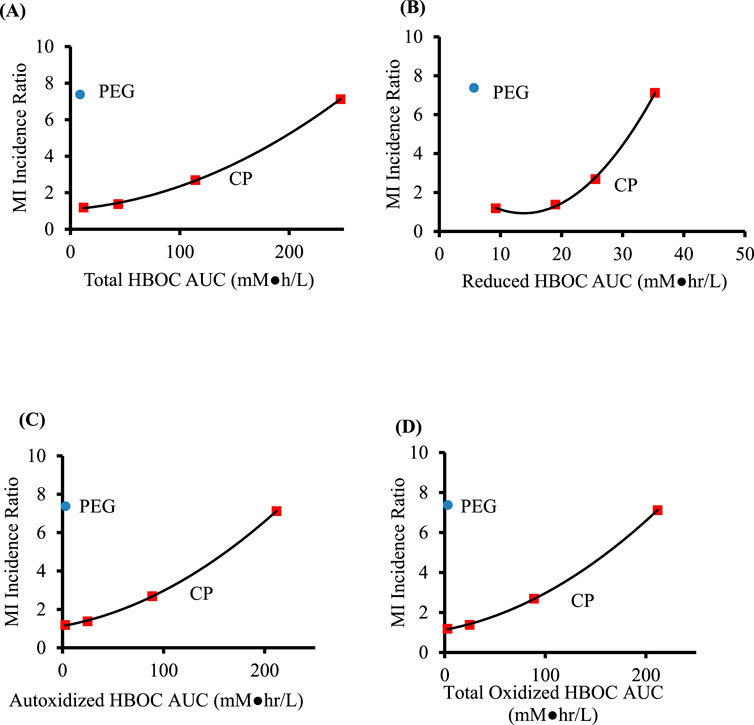
Figure 3. Comparison of AUC values predicted from a mathematical model of HBOC pharmacokinetics for crosslinked and polymerized (CP) HBOCs (squares) and Hemospan, a PEG modified HBOC (PEG, circle). Calculations assumed autoxidation rate constants 0.3 that reported by (Meng et al., 2018) and a zero NO secretion rate. Specific AUCs are for total (A), reduced (B), autoxidized (C), and total oxidized (D) HBOCs. The adjusted coefficients of determination, p values, and y intercepts for equations of best fit for the CP HBOCs are given in Table 7. Data are derived from clinical trials enrolling a total of 206–592 patients in each treated or control group for each HBOC.
Discussion
A narrative has been promoted in the HBOC literature that toxicity is primarily a consequence of the extravasation of lower molecular weight hemoglobins and their consumption of NO in the interstitial space, thereby causing vasoconstriction (Gould et al., 1998). While there is significant experimental support for this process as a primary mechanism for HBOC induced hypertension (Olson et al., 2004), this narrative ignores the potential adverse consequences of intravascular interactions. It also implies that HBOCs of larger size should be less toxic; however, the opposite is observed with respect to MI. Furthermore, infusion of HBOCs into rats, dogs, cats, or pigs resulted in no decrease in coronary blood flow (Sharma et al., 1994; Kingma et al., 2002; Ulatowski et al., 1996; Mongan et al., 2009), even in the face of substantial overall increases in systemic vascular resistance (Sharma et al., 1994), nor were coronary artery dimensions decreased in human cardiac patients after HBOC infusion (Collins et al., 1993; Serruys et al., 2008; Meliga et al., 2008). This implies that other mechanisms are important in increasing MI risk and that these are intravascular in nature.
The fact MI incidence exhibits a quadratic dependence with respect to HBOC dose motivated exploration of why this should be the case. This ultimately led to the realization this is at least in part a consequence of the fact that HBOC AUCs also exhibit a quadratic dose dependence, since increasing dose increases the circulatory half-life as well as the initial value of plasma HBOC concentration. Indeed, the correlation of MI ratio with AUC is stronger than that with either dose or size, suggesting that AUC is the better basis for the interpretation of biological effects.
HBOCs exacerbate oxidative stress in a variety of cellular, tissue and whole animal models with an important first step being an autoxidative process in which bound oxygen dissociates as superoxide, leaving the hemoglobin in the oxidized (methemoglobin) form (Alayash, 2019). Both superoxide and methemoglobin may participate in oxidative stress reactions, some of which are known to cause endothelial dysfunction (D’Agnillo, 2013) and increased vaso-occlusion, with accelerated heme loss from methemoglobin identified as a major contributing factor (Belcher et al., 2014). The oxidation of HBOCs in plasma to methemoglobin has been directly observed in clinical trials (Sprung et al., 2002; Olofsson et al., 2006; Olofsson et al., 2008; O’Hare et al., 2001). While humans may have some capacity to reduce plasma methemoglobin (Vandegriff et al., 2006; McGown et al., 1990), this capacity is limited and may be overwhelmed in patients with pre-existing endothelial dysfunction at higher HBOC doses (Biro, 2012; D’Agnillo, 2013). Oxidative stress is also variable among patients, with an extensive literature correlating such stress with increased MI risk (Wang and Kang, 2020; Liguori et al., 2018). In light of this, it is not surprising the incidence of MI is highly correlated with the initial rate of CP HBOC autoxidation as well as the estimated autoxidation AUC. Indeed, the correlation between HBOC infusion, oxidative events and enhanced MI incidence may be the most rigorous proof to date of the hypothesis that oxidative stress increases MI risk.
Methemoglobin is also formed when hemoglobin reacts with NO, with conversion of the latter to nitrate (D’Agnillo, 2013). Comparing the amount of metHBOC generated by autoxidation versus NO oxidation as reflected in their estimated relative AUC values suggests the former is more important at higher HBOC doses, but the latter can make a significant contribution at lower doses (Table 6). What cannot be directly compared in this analysis is the relative contribution of oxidative stress resulting from metHBOC formation and the consequences of profound NO depletion. For example, NO consumption is known to activate platelets (Radomski et al., 1987) and could contribute to endothelial dysfunction (Biro, 2012; D’Agnillo, 2013). Ideally, both the autoxidation and NO reaction rate constants should be reduced to minimize HBOC toxicity.
In comparing MI risk enhancement between CP HBOCs and Hemospan at comparable doses, the latter increases risk to a greater extent. This may be a consequence of increased tetramer dissociation in PEG HBOCs (Caccia et al., 2009) which can in turn accelerate autoxidation (Zhang et al., 1991). However, the in vitro measured autoxidation rate constant for Hemospan is actually slightly lower than that of the CP HBOCs (Meng et al., 2018). A more likely possibility is that PEG modification of hemoglobin leads to an increased rate of heme loss, which can in turn exacerbate vaso-occlusion (Belcher et al., 2014). Meng et al. reported that the fast phase of heme loss from Hemospan was greater than that of CP HBOCs and approximately 1.4 -fold greater than that of unmodified human hemoglobin (Meng et al., 2018). Vandegriff and coworkers reported that the fast phase of heme loss from Hemospan was fivefold greater than that of unmodified hemoglobin (Vandegriff et al., 2006). Free heme has been increasingly implicated as contributing to endothelial dysfunction and MI risk (Guo et al., 2022). Thus, although PEG modification has proven useful in improving the therapeutic index of other proteins, it may not be helpful in improving the desired characteristics of HBOCs.
Some may be disconcerted by the fact that multiple combinations of autoxidation rate constants and NO secretion rates result in AUC values that demonstrate a strong correlation to the MI ratios (Tables 5–7). However, this reflects the mathematical reality that multiplication of any set of independent variables by the same constant will not affect the correlation coefficient due to the fact that the independent variables appear in both the numerator and denominator to the same power. Thus, correlations do not in themselves necessarily reflect the relative absolute contributions of different variables to the observed dependent variable. This can be better defined by comparison of the absolute values of AUC to actual clinical data as was done with respect to Hemospan. Unfortunately, this is the only HBOC clinical data set which has a sufficient number of plasma metHBOC data points in order to do so. For this reason, correlations of various AUC values with MI ratios were assessed for a range of autoxidation and NO secretion rates, encompassing the specific values that give good agreement with clinical data for Hemospan. The fact that there were strong correlations between AUC values and MI incidence in all of these scenarios implies that these correlations are robust in this sense.
The analysis presented in this publication is limited by several factors. None of the clinical trials from which data were derived were specifically designed to explore possible mechanisms by which HBOCs may increase MI risk, in part because this was not identified in preclinical studies or individual clinical trials as a product related adverse event prior to 2008. It was only when data are aggregated that this became apparent (Natanson et al., 2008). In addition, the diagnosis of MI is not necessarily straightforward in the presence of HBOCs because hemoglobin can interfere with MI diagnostic troponin assays (Estep, 2019), although several HBOC developers have considered such interference (Ma et al., 1997; Olofsson et al., 2011). The exact criteria used for MI diagnosis are also not always well described, and may therefore differ, which could contribute to between study variability. On the other hand, it is reasonable to assume that the same criteria are used in assessing treated and control patients within a study, and common authorship across a number of the studies should increase consistency. Finally, the analyses presented in this publication required the estimation, interpretation or extrapolation of a variety of parameters. The need for data aggregation also results in only four or five data points for each comparative analysis. As a consequence, the observations presented in this publication should be considered as hypothesis generating rather than definitive.
Collectively, the analyses presented in this communication, when viewed in light of an extensive literature on HBOC safety, suggests that efforts to minimize hemoglobin oxidative reactions should result in less toxic formulations (Bian and Chang, 2015). In addition, further exploration of the manner in which HBOCs impact patient oxidation/reduction status and endothelial dysfunction could usefully improve patient selection criteria. Such efforts may ultimately enable the identification of patient subpopulations at low risk for HBOC side effects.
Data availability statement
The raw data supporting the conclusions of this article will be made available by the authors, without undue reservation.
Ethics statement
Ethical approval was not required for the study involving humans in accordance with the local legislation and institutional requirements. Written informed consent to participate in this study was not required from the participants or the participants’ legal guardians/next of kin in accordance with the national legislation and the institutional requirements.
Author contributions
TE: Conceptualization, Data curation, Formal Analysis, Investigation, Methodology, Writing – original draft, Writing – review and editing.
Funding
The author(s) declare that no financial support was received for the research and/or publication of this article.
Acknowledgments
The author expresses appreciation to emeritus professor John Olson of Rice University for helpful comments after a review of a preliminary version of this manuscript. Preliminary results of this study were presented at the International Society of Blood Substitutes 19th symposium on 17 November 2024.
Conflict of interest
The author is the sole employee of the biotech consulting firm Chart Biotech Consulting, LLC. The author is also serving on the External Advisory Board of the DARPA Fieldable Solutions for Hemorrhage with bio-Artificial Products (FSHARP) program and as a consultant to 20BLOC, Inc., which is developing a new HBOC formulation. The author also previously consulted with Omniox in which he retains a financial interest. None of these entities contributed technically, conceptually or financially to the research described in this publication.
Generative AI statement
The author(s) declare that no Generative AI was used in the creation of this manuscript.
Publisher’s note
All claims expressed in this article are solely those of the authors and do not necessarily represent those of their affiliated organizations, or those of the publisher, the editors and the reviewers. Any product that may be evaluated in this article, or claim that may be made by its manufacturer, is not guaranteed or endorsed by the publisher.
References
Alayash A. I. (2019). Mechanisms of toxicity and modulation of hemoglobin-based oxygen carriers. Shock 52 (1S Suppl. 1), 41–49. doi:10.1097/SHK.0000000000001044
Author Anonymous (2006). Northfield laboratories releases summary observations from its elective surgery trial (news release). Evanston, IL: Northfield laboratories Inc. Available online at: http://phx.corporate-ir.net/phoenix.zhtml?c=91374&p=irol-newsArticle_print&ID=1005951&highlight= (Accessed December 16, 2017).
Belcher J. D., Chen C., Nguyen J., Milbauer L., Abdulla F., Alayash A. I., et al. (2014). Heme triggers TLR4 signaling leading to endothelial cell activation and vaso-occlusion in murine sickle cell disease. Blood 123, 377–390. doi:10.1182/blood-2013-04-495887
Berbers G. A. M., Bleeker W. K., Stekkinger P., Agterberg J., Rigter G., Bakker J. C. (1991). Biophysical characteristics of hemoglobin intramolecularly cross-linked and polymerized. J. Lab. Clin. Med. 117, 157–165.
Bian Y., Chang T. M. S. (2015). A novel nanobiotherapeutic poly-[hemoglobin-superoxide dismutase-catalase-carbonic anhydrase] with no cardiac toxicity for the resuscitation of a rat model with 90 minutes of sustained severe hemorrhagic shock with loss of 2/3 blood volume. Artif. Cells Nanomed. Biotechnol. 43, 1–9. doi:10.3109/21691401.2014.964554
Biro G. P. (2012). Adverse HBOC-endothelial dysfunction synergism: a possible contributor to adverse clinical outcomes? Curr. Drug. Discov. Technol. 9, 194–203. doi:10.2174/157016312802650733
Bleeker W. K., Berbers A. M., Boer P. J. D., Agterberg J., Rigter G., Bakker J. C. (1992). Effect of polymerization on clearance and degradation of free hemoglobin. Biomater. Artif. Cells Immobil. Biotechnol. 20, 747–750. doi:10.3109/10731199209119713
Bloomfield E. L., Rady M. Y., Esfandiari S. (2004). A prospective trial of diaspirin cross-linked hemoglobin solution in patients after elective repair of abdominal aortic aneurysm. Mil. Med. 169, 546–550. doi:10.7205/milmed.169.7.546
Caccia D., Ronda L., Frassi R., Perrella M., Del Favero E., Bruno S., et al. (2009). PEGylation promotes hemoglobin tetramer dissociation. Bioconjug Chem. 20, 1356–1366. doi:10.1021/bc900130f
Carmichael F. J. L., Ali A. C. Y., Campbell J. A., Langlois S. F., Biro G. P., Willan A. R., et al. (2000). A phase I study of oxidized raffinose cross-linked human hemoglobin. Crit. Care Med. 28, 2283–2292. doi:10.1097/00003246-200007000-00017
Cheng D. C. H. (2001). Safety and efficacy of o-raffinose cross-linked human hemoglobin (Hemolink™) in cardiac surgery. Can. J. Anaesth. 44, S41–S48.
Cheng D. C. H., Mazer C. D., Martineau R., Ralph-Edwards A., Karski J., Robblee J., et al. (2002). A phase II dose-response study of hemoglobin raffimer (Hemolink) in elective coronary artery bypass surgery. J. Thorac. Cardiovasc. Surg. 127, 79–86. doi:10.1016/j.jtcvs.2003.08.024
Collins P., Burman J., Chung H.-I., Fox K. (1993). Hemoglobin inhibits endothelium-dependent relaxation to acetylcholine in human coronary arteries in vivo. Circulation 87, 80–85. doi:10.1161/01.cir.87.1.80
Conover C. D., Linberg R., Gilbert C. W., Shum K. L., Shorr R. G. L. (1997). Effect of polyethylene glycol conjugated bovine hemoglobin in both top-load and exchange transfusion rat models. Artif. Organs 21, 1066–1075. doi:10.1111/j.1525-1594.1997.tb00444.x
D’Agnillo F. (2013). “Redox activity of cell-free hemoglobin: implications for vascular oxidative stress and endothelial dysfunction,” in Hemoglobin-based oxygen carriers as red cell substitutes. Editors H. W. Kim, and A. G. Greenburg (Berlin: Springer), 665–682.
Estep T. N. (2015). Pharmacokinetics and mechanisms of plasma removal of hemoglobin-based oxygen carriers. Artif. Cells. Nanomed. Biotechnol. 43, 203–215. doi:10.3109/21691401.2015.1047501
Estep T. N. (2019). Haemoglobin-based oxygen carriers and myocardial infarction. Artif. Cells Nanomed. Biotechnol. 47, 593–601. doi:10.1080/21691401.2019.1573181
Estep T. N. (2019). Issues in the development of hemoglobin based oxygen carriers. Semin. Hematol. 5, 257–261. doi:10.3109/21691401.2015.1047501
Garrioch M. A., McClure J. H., Wildsmith A. W. (1999). Haemodynamic effects of diaspirin crosslinked haemoglobin (DCLHb) given before abdominal aortic aneurysm surgery. Br. J. Anaesth. 83, 702–707. doi:10.1093/bja/83.5.702
Gould S. A., Moore E. M., Hoyt D. B., Burch J. M., Haenel J. B., Garcia J., et al. (1998). The first randomized trial of human polymerized hemoglobin as a blood substitute in acute trauma and emergent surgery. J. Am. Coll. Surg. 187, 113–122. doi:10.1016/s1072-7515(98)00095-7
Greenburg A. G., Kim H. W.Hemolink Study Group (2004). Use of an oxygen therapeutic as an adjunct to intraoperative autologous donation to reduce transfusion requirements in patients undergoing coronary artery bypass graft surgery. J. Am. Coll. Surg. 198, 373–385. doi:10.1016/j.jamcollsurg.2003.11.020
Guo Y., Zhao H., Lin Z., Ye T., Xu D., Zeng Q. (2022). Heme in cardiovascular diseases: a ubiquitous dangerous molecule worthy of vigilance. Front. Cell Dev. Biol. 9, 781839. doi:10.3389/fcell.2021.781839
Hall C. N., Garthwaite J. (2009). Nitric Oxide., What is the real physiological NO concentration in vivo? Nitric Oxide. 21, 92–103. doi:10.1016/j.niox.2009.07.002
Hemelrijck J. V., Levien L. J., Veeckman L., Pitman A., Zafirelis Z., Standl T. (2014). A safety and efficacy evaluation of hemoglobin-based oxygen carrier HBOC-201 in a randomized, multicenter red blood cell controlled trial in noncardiac surgery patients. Anesth. Analg. 119, 766–776. doi:10.1213/ANE.0000000000000305
Hill S. E., Gottschalk I., Grichnik K. (2002). Safety and preliminary efficacy of hemoglobin raffimer for patients undergoing coronary artery bypass surgery. J. Cardiothorac. Vasc. Anesth. 16, 695–702. doi:10.1053/jcan.2002.128416
Hsia J. C., Song D. L., Er S. S., Wong L. T., Keipert P. E., Gomez C. L., et al. (1992). Pharmacokinetics studies in the rat on a o-raffinose polymerized human hemoglobin. Biomater. Artif. Cells Immobil. Biotechnol. 202, 587–595. doi:10.3109/10731199209119687
Hughes G. S., Antal E. J., Locker P. K., Francom S. F., Adams W. J., Jacobs E. E. (1996). Physiology and pharmacokinetics of a novel hemoglobin-based oxygen carrier in humans. Crit. Care Med. 24, 756–764. doi:10.1097/00003246-199605000-00006
Hughes G. S., Francom S. F., Antal E. J., Adams W. J., Locker P. K., Yancey E. P., et al. (1995). Hematologic effects of a novel hemoglobin-based oxygen carrier in normal male and female subjects. J. Lab. Clin. Med. 126, 444–451.
Jahr J. S., Mackenzie C., Pearce L. B., Pitman A., Greenberg A. G. (2008). HBOC-201 as an alternative to blood transfusion: efficacy and safety evaluation in a multicenter Phase III trial in elective orthopedic surgery. J. Trauma 64, 1484–1497. doi:10.1097/TA.0b013e318173a93f
Kasper S. M., Grüne F., Walter M., Amr N., Erasmi H., Buzello W. (1998). The effects of increased doses of bovine hemoglobin on hemodynamics and oxygen transport in patients undergoing preoperative hemodilution for elective abdominal aortic surgery. Anesth. Analg. 87, 284–291. doi:10.1097/00000539-199808000-00009
Kasper S. M., Walter M., Grüne F., Bischoff A., Erasmi H., Buzello W. (1996). Effects of a hemoglobin-based oxygen carrier (HBOC-201) on hemodynamics and oxygen transport in patients undergoing preoperative hemodilution for elective abdominal aortic surgery. Anesth. Analg. 83, 912–927. doi:10.1097/00000539-199611000-00006
Keipert P. E., Gomez C. L., Gonzales A., Macdonald V. W., Winslow R. M. (1992). The role of the kidneys in the excretion of chemically modified hemoglobins. Biomater. Artif. Cells Immobil. Biotechnol. 20, 737–745. doi:10.3109/10731199209119712
Kerner T., Ahlers O., Veit S., Riou B., Saunders M., Pison U., et al. (2003). DCL-Hb for trauma patients with severe hemorrhagic shock: the European “on-scene” multicenter study. Intensive Care Med. 29, 378–385. doi:10.1007/s00134-002-1622-x
Kingma J. G., Sandhu R., Hamelin N. D., Gendron D., Trudel Y., Bosa M., et al. (2002). The effects of hemodilution with Hemolink upon hemodynamics and blood flow distribution in anesthetized dogs. Immobil. Biotechnol. 30, 137–154. doi:10.1081/bio-120003194
LaMuraglia G. M., O’Hare P. J., Baker W. H., Naslund T. C., Norris E. J., Jolly K., et al. (2000). The reduction of the allogenic transfusion requirement in aortic surgery with a hemoglobin-based solution. J. Vasc. Surg. 31, 299–308. doi:10.1016/s0741-5214(00)90161-7
Lamy M. L., Daily E. K., Brichant J.-F., Larbuisson R. P., Demeyere R. H., Vandermeersch E. A., et al. (2000). Randomized trial of diaspirin cross-linked hemoglobin solution as an alternative to blood transfusion after cardiac surgery. The DCLHb Cardiac Surgery Trial Collaborative Group. Anesthesiology 92, 646–656. doi:10.1097/00000542-200003000-00007
Levy J. H., Goodnough L. T., Greilich P. E., Parr G. V. S., Stewart R. W., Gratz I., et al. (2002). Polymerized bovine hemoglobin solution as a replacement for allogeneic red blood cell transfusion after cardiac surgery: results of a randomized, double-blind trial. J. Thorac. Cardiovasc. Surg. 124, 35–42. doi:10.1067/mtc.2002.121505
Liguori I., Russo G., Curcio F., Bulli G., Aran L., Della-Morte D., et al. (2018). Oxidative stress, aging, and diseases. Clin. Interv. Aging 13, 757–772. doi:10.2147/CIA.S158513
H. Liu, A. D. Kaye, and J. S. Jahr (2022). Blood substitutes and oxygen biotherapeutics (Charm, Switzerland: Springer).
Ma Z., Monk T. G., Goodnough L. T., McCellan A., Gawryl M., Clark T., et al. (1997). Effect of hemoglobin- and perflubron-based oxygen carriers on common clinical laboratory tests. Clin. Chem. 43, 1732–1737. doi:10.1093/clinchem/43.9.1732
McGown E. L., Lyons M. F., Marini M. A., Zegna A. (1990). Reduction of extracellular methemoglobin by erythrocytes. Biochim. Biophys. Acta 1036, 202–206. doi:10.1016/0304-4165(90)90035-u
Meliga E., Vranckx P., Regar E., Kint P.-P., Duncker D. J., Serruys P. W. (2008). Proof-of-concept trial to evaluate haemoglobin based oxygen therapeutics in elective percutaneous coronary revascularisation. Rationale, protocol design and haemodynamic results. EuroIntervention 4, 99–107. doi:10.4244/eijv4i1a17
Meng F., Kassa T., Jana S., Wood F., Zhang X., Jia Y., et al. (2018). Comprehensive biochemical and biophysical characterization of hemoglobin-based oxygen carrier therapeutics: all HBOCs are not created equally. Bioconjug. Chem. 29, 1560–1575. doi:10.1021/acs.bioconjchem.8b00093
Mongan P. D., Moon-Massat P. F., Rentko V., Mihok S., Dragovich A., Sharma P. (2009). Regional blood flow after serial normovolemic exchange transfusion with HBOC-201 (Hemopure) in anesthetized swine. J. Trauma 67, 51–60. doi:10.1097/TA.0b013e3181838030
Moore E. E., Moore F. A., Fabian T. C., Bernard A. C., Fulda G. J., Hoyt D. B., et al. (2009). Human polymerized hemoglobin for the treatment of hemorrhagic shock when blood is unavailable: the USA multicenter trial. J. Am. Coll. Surg. 208, 1–13. doi:10.1016/j.jamcollsurg.2008.09.023
Motulsky H. (2010). “Confidence intervals of a proportion,” in Intuitive biostatistics. Editor H. Motulsky (New York: Oxford University Press), 25–37.
Natanson C., Kern S., Lurie P., Banks S. M., Wolfe S. M. (2008). Cell-free hemoglobin-based blood substitutes and risk of myocardial infarction and death. JAMA 299, 2303–2312. doi:10.1001/jama.299.19.jrv80007
O’Hare J. F., Colburn W. A., Tetzlaff J. E., Novick A. C., Angermeier K. W., Schubert A. (2001). Hemoglobin and methemoglobin concentrations after large-dose infusions of diaspirin cross-linked hemoglobin. Anesth. Analg. 92, 44–48. doi:10.1097/00000539-200101000-00009
Olofsson C., Nygärd E. B., Ponzer S., Fagrell B., Przybelski R., Keipert P. E., et al. (2008). A randomized, single-blind, increasing dose safety trial of an oxygen-carrying plasma expander (Hemospan®) administered to orthopedic surgery patients with spinal anaesthesia. Transfus. Med. 18, 28–39. doi:10.1111/j.1365-3148.2007.00811.x
Olofsson C., Torbjörn A., Johansson T., Larsson S., Nellgärd P., Ponzer S., et al. (2006). A multicenter clinical study of the safety and activity of maleimide-polyethylene glycol-modified hemoglobin (Hemospan®) in patients undergoing major orthopedic surgery. Anesthesiology 105, 1153–1163. doi:10.1097/00000542-200612000-00015
Olofsson C. I., Górecki A. Z., Dirksen R., Kofranek I., Majewski J. A., Mazurkiewicz T., et al. (2011). Evaluation of MP4OX for prevention of perioperative hypotension in patients undergoing primary hip arthroplasty with spinal anesthesia: a randomized, double-blind, multicenter study. Anesthesiology 114, 1048–1063. doi:10.1097/ALN.0b013e318215e198
Olson J. S., Foley E. W., Rogge C., Tsai A.-L., Doyle M. P., Lemon D. D. (2004). NO scavenging and the hypertensive effect of hemoglobin-based blood substitutes. Free Radic. Biol. Med. 36, 685–697. doi:10.1016/j.freeradbiomed.2003.11.030
Przybelski R. J., Daily E. K., Kisicki J. C., Mattia-Goldbery C., Bounds M. A., Colburn W. A. (1996). Phase I study of the safety and pharmacologic effects of diaspirin cross-linked hemoglobin solution. Crit. Care Med. 24, 1993–2000. doi:10.1097/00003246-199612000-00011
Przybelski R. J., Daily E. K., Micheels J., Sloan E., Mols P., Corne L., et al. (1999). A safety assessment of diaspirin cross-linked hemoglobin (DCLHb) in the treatment of hemorrhagic, hypovolemic shock. Prehosp. Disaster. Med. 14, 47–60. doi:10.1017/s1049023x00027722
Radomski M. W., Palmer R. M., Moncada S. (1987). Endogenous nitric oxide inhibits human platelet adhesion to vascular endothelium. Lancet 2, 1057–1058. doi:10.1016/s0140-6736(87)91481-4
Schubert A., O’Hare J. F., Przybelski R. J., Tetzlaff J. E., Marks K. E., Mascha E., et al. (2002). Effect of diaspirin crosslinked hemoglobin (DCLHb HemAssist™) during high blood loss surgery on selected indices of organ function. Artif. Cells. Blood. Substit. Immobil. Biotechnol. 30, 259–283. doi:10.1081/bio-120006118
Schubert A., Przybelski R. J., Eidt J. F., Lasky L. C., Marks K. E., Karafa M., et al. (2003). Diaspirin-crosslinked hemoglobin reduces blood transfusion in noncardiac surgery: a multicenter, randomized, controlled, double-blinded trial. Anesth. Analg. 97, 323–332. doi:10.1213/01.ANE.0000068888.02977.DA
Serruys P. W., Vranckx P., Slagboom T., Regar E., Meliga E., de Winter R. J., et al. (2008). Haemodynamic effects, safety, and tolerability of haemoglobin-based oxygen carrier-201 in patients undergoing PCI for CAD. EuroIntervention 3, 600–609. doi:10.4244/eijv3i5a108
Sharma A. C., Rebello S., Gulati A. (1994). Regional circulatory and systemic hemodynamic effects of diaspirin cross-linked hemoglobin in the rat. Artif. Cells Blood Substit. Immobil. Biotechnol. 22, 593–602. doi:10.3109/10731199409117888
Sloan E. P., Koenigsberg M., Gens D., Cipolle M., Runge J., Mallory M. N., et al. (1999). Diaspirin cross-linked hemoglobin (DCLHb) in the treatment of severe traumatic hemorrhagic shock: a randomized controlled efficacy trial. JAMA 282, 1857–1864. doi:10.1001/jama.282.19.1857
Snyder S. R., Welty E. V., Walder R. Y., Williams L. A., Walder J. A. (1987). HbXL99 alpha: a hemoglobin derivative that is cross-linked between the alpha subunits is useful as a blood substitute. Proc. Natl. Acad. Sci. USA. 84, 7280–7284. doi:10.1073/pnas.84.20.7280
Sprung J., Kindscher J. D., Wahr J. A., Levy J. H., Monk T. G., Moritz M. W., et al. (2002). The use of bovine hemoglobin glutamer-250 (Hemopure®) in surgical patients: results of a multicenter, randomized, single-blinded trial. Anesth. Analg. 94, 799–808. doi:10.1097/00000539-200204000-00006
Standl T., Burmeister M.-A., Horn E.-P., Wilhelm S., Knoefel W. T., Schulte am Esch J. (1998). Bovine haemoglobin-based oxygen carrier for patients undergoing haemodilution before liver resection. Br. J. Anaesth. 80, 189–194. doi:10.1093/bja/80.2.189
Swan S. K., Halstenson C. E., Collins A. J., Colburn W. A., Blue J., Przybelski R. J. (1995). Pharmacologic profile of diaspirin cross-linked hemoglobin in hemodialysis patients. Am. J. Kidney Dis. 26, 918–923. doi:10.1016/0272-6386(95)90056-x
Taguchi K., Yamasaki K., Maruyama T., Obtiri M. (2017). Comparison of the pharmacokinetics properties of hemoglobin-based oxygen carriers. J. Funct. Biomater. 8, 1–18. doi:10.3390/jfb8010011
Ulatowski J. A., Nishikawa T., Matheson-Urbaitis B., Bucci E., Traystman R. J., Koehler R. C. (1996). Regional blood flow alterations after bovine fumaryl beta beta-crosslinked hemoglobin transfusion and nitric oxide synthase inhibition. Crit. Care Med. 24, 558–565. doi:10.1097/00003246-199604000-00003
Vandegriff K. D., Malavalli A., Minn C., Jiang E., Lohman J., Young M. A., et al. (2006). Oxidation and haem loss kinetics of poly(ethylene glycol)-conjugated haemoglobin (MP4): dissociation between in vitro and in vivo oxidation rates. Biochem. J. 399, 463–471. doi:10.1042/BJ20060809
Vandegriff K. D., Malavalli A., Woodridge J., Lohman J., Winslow R. M. (2003). MP4, a new nonvasoactive PEG-Hb conjugate. Transfusion 43, 509–516. doi:10.1046/j.1537-2995.2003.00341.x
Vandegriff K. D., McCarthy M., Rohlfs R. J., Winslow R. M. (1997). Colloid osmotic properties of modified hemoglobins: chemically cross-linked versus polyethylene glycol surface-conjugated. Biophys. Chem. 69, 23–30. doi:10.1016/s0301-4622(97)00079-3
Van der Linden P., Gazdzik T. S., Jahoda D., Heylen R. J., Skowronski J. C., Pellar D., et al. (2011). A double-blind, randomized, multicenter study of MP4OX for treatment of perioperative hypotension in patients undergoing primary hip arthroplasty under spinal anesthesia. Anesth. Analg. 112, 759–773. doi:10.1213/ANE.0b013e31820c7b5f
Walpole S. C., Prieto Merino D., Edwards P., Cleland J., Stevens G., Roberts I., et al. (2012). The weight of nations: an estimation of adult human biomass. BMC Public Health (Internet) 12, 439. doi:10.1186/1471-2458-12-439
Wang W., Kang P. M. (2020). Oxidative stress and antioxidant treatments in cardiovascular diseases. Antioxidants 9, 1292–1317. doi:10.3390/antiox9121292
Wicks D., Wong L. T., Sandhu R., Stewart R. K., Biro G. P. (2002). The intravascular persistence and methemoglobin formation of Hemolink (hemoglobin raffimer) in dogs. Immobil. Biotechnol. 31, 1–17. doi:10.1081/bio-120018000
WorldData (1995). Average height and weight by country. Available online at: http://www.worlddata.info/average-bodyheight.php (Accessed February 2, 2023).
Keywords: hemoglobin-based oxygen carriers, HBOC, myocardial infarction, oxidative stress, nitric oxide, hemoglobin autoxidation, pharmacokinetics, AUC
Citation: Estep TN (2025) Hemoglobin-based oxygen carriers, oxidative stress and myocardial infarction. Front. Physiol. 16:1551932. doi: 10.3389/fphys.2025.1551932
Received: 26 December 2024; Accepted: 11 March 2025;
Published: 15 April 2025.
Edited by:
Dario A. Vitturi, University of Alabama at Birmingham, United StatesReviewed by:
Leif Bulow, Lund University, SwedenAndre Palmer, The Ohio State University, United States
Copyright © 2025 Estep. This is an open-access article distributed under the terms of the Creative Commons Attribution License (CC BY). The use, distribution or reproduction in other forums is permitted, provided the original author(s) and the copyright owner(s) are credited and that the original publication in this journal is cited, in accordance with accepted academic practice. No use, distribution or reproduction is permitted which does not comply with these terms.
*Correspondence: Timothy N. Estep, Y2hhcnRiaW90ZWNoQGFvbC5jb20=