- 1Institute of Pharmacy, Nirma University, Ahmedabad, Gujarat, India
- 2Institute of Science, Nirma University, Ahmedabad, Gujarat, India
- 3ResearchSat Pvt Ltd., Adelaide, SA, Australia
As human physiology gets altered in the presence of space flight conditions such as microgravity and radiation, microbes also behave differently in space. Many microorganisms have been isolated from space stations and spacecrafts after human space travel. Biofilm formation and biofilm-related fouling specifically in the water recovery system of space stations are known. Microbes have evolved various physiological and genetic adaptations which allow them to grow and develop biofilm in harsh space environments as well. They contaminate the spacecraft environment, its surfaces, water system, and food products and also affect astronauts’ health due to decreased immunity in space. Microbes also develop antibiotic resistance and hence, the choice of antibiotics is a challenging task for treating infection during space travel. There is also a negative impact of the space environment on the stability of antimicrobial agents and many get degraded in the presence of ionizing space radiation. This article describes strategies that may decrease the risk of microbial contamination and associated problems during space expeditions. Various preventive measures are suggested such as use of disinfectants for periodic cleaning, development of antibiotic coated surfaces to kill microbes, development of space-hardy anti-microbial drugs, etc. Multifaceted approach that integrates technological innovation, interdisciplinary collaboration, and robust policy frameworks is essential. Space based microbial research can further help for the innovations in healthcare and environment management on earth.
1 Introduction
The space environment and space stations in low earth orbit (LEO) (at an altitude of 400 km from the earth’s surface) have several stressful conditions such as microgravity (10–3 to 10–6 g gravity), 153°–393 K temperature, 10–7 to 10–4 Pa vacuum pressure as well as 0.2–0.5 kPa CO2 levels (Simoes and Antunes, 2021). On the earth, we are shielded from various radiations by the earth’s atmosphere and magnetosphere, which function like a protective cocoon. It allows only UV-visible rays to penetrate the magnetosphere. Outside this protective cocoon, the space is brimming with ionizing and non-ionizing radiations. The damaging effect of non-ionizing radiation can be prevented using proper shielding. Ionizing radiations are difficult to shield and include electromagnetic rays like gamma rays, X-rays, and particles like electrons, protons, neutrons, and high energy particles. The main sources of space ionizing radiation include Galactic Cosmic Rays (GCR), Solar Cosmic Rays (SCR), and particles trapped by the Earth’s magnetic field. Interaction of ionizing radiation with spaceship construction and shielding material can generate secondary ions like neutrons, proton recoils, gamma rays, and X-rays which can create a complex radiation environment within spacecraft (Chancellor et al., 2014; Bijlani et al., 2021; Bhayani et al., 2022; Urbaniak et al., 2024). Such conditions in space are very different from the environmental conditions on Earth making the situation hostile to the existence of numerous life forms (Merino et al., 2019). Still, the microorganisms carried to space from the Earth via spacecraft or crew members can survive there (Wang et al., 2021; Vaishampayan and Grohmann, 2019). The International Space Station (ISS) is the largest space station currently in low Earth orbit and the outcome of valuable efforts of scientists and engineers from five space agencies representing 15 countries (ISS National Laboratory, 2022; NASA, 2025a) whereas Tiangong is the other space station launched by China (Diaz et al., 2019; Landry et al., 2020; ISS National Laboratory, 2022). Such space stations contain plenty of microorganisms (Wang et al., 2021; Vaishampayan and Grohmann, 2019). During 2015–2018, ISS conducted two investigations designated as Microbial tracking-1 (MT-1) and Microbial tracking-2 (MT-2) for studying the microbial population of ISS. MT-1 conducted three sampling sessions for 14 months between 2015–2016 and collected samples from eight ISS locations. MT-2 continued the study for another 4 flight sessions and collected samples from the same ISS locations during 2017–2018. MT-2 report mentions that the skin associated microbes are dominantly present on the spacecraft surfaces and bacterial species such as Staphylococcus as well as fungal species like Malassezia are the most common (NASA, 2025b; Urbaniak et al., 2022). Moreover, members of the family Enterobacteriaceae, Bacillus spp., Propionibacterium spp., Corynebacterium spp., and Staphylococcus spp. are the common bacteria isolated from air and surface samples as well as from air filters of ISS. Sphingomonas spp. and Methylobacterium spp. were reported in the drinking water of ISS (Wang et al., 2021). Acinetobacter baumannii, Haemophilus influenzae, Klebsiella pneumoniae, Salmonella enterica, Shigella sonnei, Staphylococcus aureus, Yersinia frederiksenii, and Aspergillus lentulus are numerous human pathogens reported in ISS samples (Vaishampayan and Grohmann, 2019). Moreover, recently 5-g positive novel bacterial species were isolated from ISS and among them Paenibacillus vandeheii F6_3S_P_1C was a spore forming bacteria whereas the remaining four were non-spore-forming Actinobacteria (Szydlowski et al., 2024). These microbes are usually non-pathogenic but may become opportunistic pathogens in long-term exposure to radiation and microgravity conditions, causing several types of infections to astronauts as their immunity is weakened during a space mission (Zea et al., 2017; Santomartino et al., 2020; Simoes and Antunes, 2021). Moreover, these microbes disturb the normal functioning of the spacecraft and its equipment by corroding them or by forming a microbial biofilm on surfaces thus creating problems during a space mission. There are several mechanisms adapted by microorganisms which boost their survival in the harsh space environment. Therefore, this review article summarized various factors responsible for microbial survival in space and strategies to mitigate its adverse effect during human space travel.
Microbial contamination risks associated with spacesuits during extravehicular activities (EVAs) are of growing concern, as microorganisms can adhere to suit surfaces, potentially degrading materials and posing health risks to astronauts. Researchers have shown that certain microbes not only survive but can remain metabolically active in space, retaining their ability to degrade materials like cellulose under microgravity and radiation exposure. This raises concerns for the integrity of spacesuit fabrics, which may be susceptible to biodeterioration over time (Shakir et al., 2022). Additionally, the study by Danko et al. (2021) reveals that spacesuits can carry diverse population of microorganisms, including opportunistic pathogens. These microorganisms can be transferred back into the spacecraft after EVAs, increasing the risk of infection, especially in the context of altered immunity during spaceflight. Hence, microbial adhesion on spacesuits is a dual threat, potentially compromising both material durability and astronaut health during long-term missions (Danko et al., 2021).
1.1 Microbial survival in space
The scientific community has witnessed that the space environment causes some adverse effects on microbes such as alteration in cell morphology, cell envelope, cell growth, motility, nutrient uptake potential, carbon source utilization capability, biofilm formation ability, antimicrobial resistance, and others which allow them to grow in space conditions (Su et al., 2021; Aunins et al., 2018).
1.1.1 Microbial survival under microgravity conditions
The exact mechanism behind the mentioned altered cell behavior of microbes in microgravity is not known but it has been hypothesized that the lack of gravity-driven forces such as buoyancy and sedimentations in microgravity are responsible for this (Zea et al., 2016). In microgravity, buoyancy and convection current are completely absent thus leading to low shear and low turbulence (Bijlani et al., 2021). In low shear conditions, the surrounding of the microbial cell is quiescent and the uptake of nutrients by microbial cells from their surrounding is carried out via diffusion. This affects cell metabolism as the movement of molecules in and out of the cell is very limited (reducing mass transfer). The study conducted by Zea and the team in 2016 reported that the microbes have adapted various physiological changes to withstand reduced mass transfer rate. These adaptations are responsible for their survival in space. Microorganisms require energy as well as reduced molecules for performing various activities essential for their survival under space conditions. Thus, carbohydrates metabolism is increased and the amino acids are utilized as a carbon source instead of being used for protein formation to fulfil the energy demand of microbial cells. Altered lipid metabolism leads to the synthesis of various membrane lipids such as lipoprotein and lipopolysaccharide essential for cell-to-cell communication, biofilm formation, virulence, and pathogenicity (Milojevic and Weckwerth, 2020). Altered transport machinery ensures higher uptake of nutrients, efficient removal of waste metabolites as well as elevated signaling of quorum sensing molecules. Thickened cell wall and increase cell density makes them resistant to various stressful conditions (Huang et al., 2018; Taffs et al., 2009). The motility and chemotaxis of microbial cells under space microgravity are enhanced as a low shear effect in microgravity makes the cells’ outer environment quiescent. The flagellar movement of motile cells disturbs the surrounding of the cells ensuring the appropriate distribution of the solutes and molecules essential for cell growth. Electron transport proteins synthesized by microbes play a crucial role in energy management. Induction of the iron uptake mechanism is observed under microgravity. Iron-specific repressor protein named Fur binds with the fur box (GATAATGATAATCATTATC) in the presence of iron as a cofactor. In the absence of iron, it detaches from the fur box allowing the transcription process for the synthesis of siderophores, virulence genes as well as superoxide dismutase (SOD) containing iron and manganese. Various heat shock proteins and chaperon proteins reverse the misfolding of proteins which occurs under space conditions or bind with misfolded proteins and make them available to proteases for degradation. Elevated anti-oxidative stress mechanisms and osmotolerant molecules (compatible solutes like carnitine) also increase microbial survival in space. Microbial spores are known to be resistant to harsh conditions and many spore-forming microbes can survive in space conditions (Milojevic and Weckwerth, 2020). Tellurium is a rare earth metal found in space and is toxic to humans as well as microbes (Vávrová et al., 2021). Microbes have shown enhanced tellurium resistance in space environments which in turn affects other metabolic pathways leading to an increase in oxidative stress response. Figure 1 summarizes the various microbial adaptations under microgravity conditions.
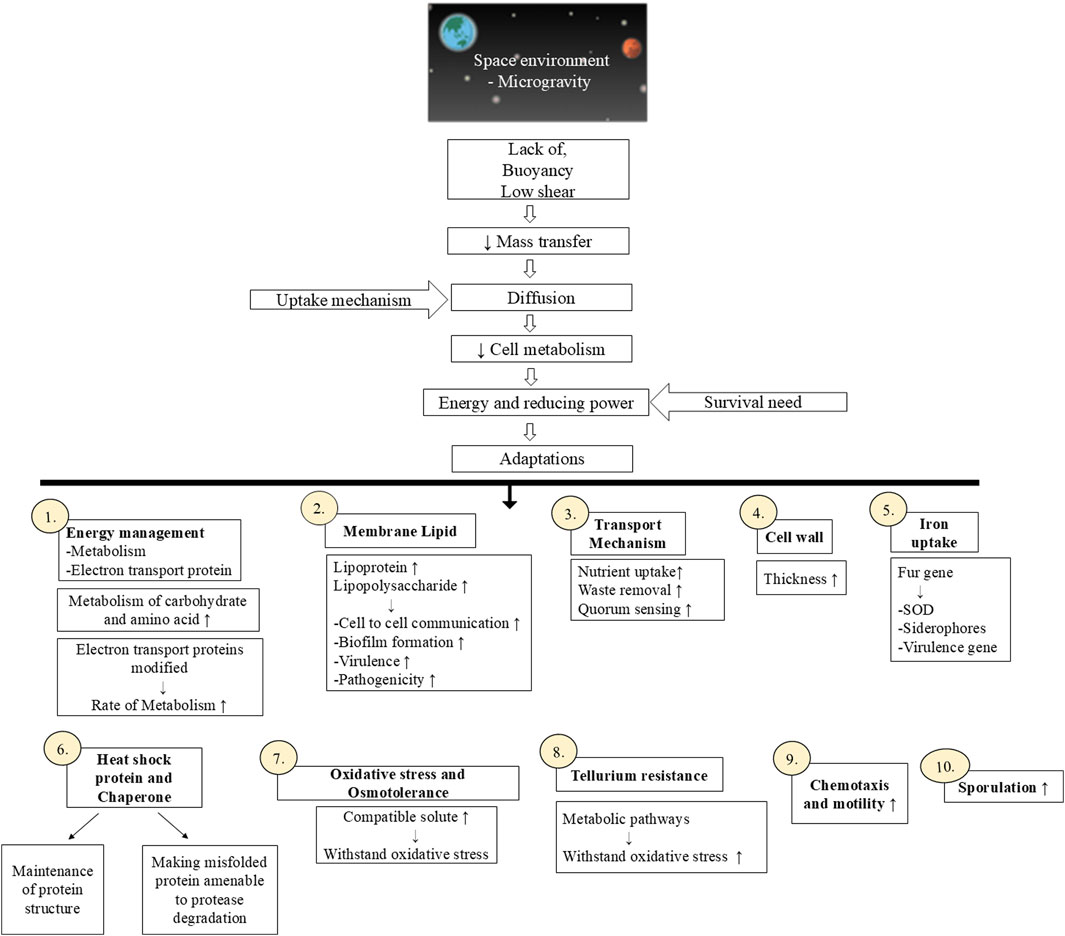
Figure 1. Microbial adaptations in space microgravity condition. [Figure has been made using BioRender (https://biorender.com)].
Stenotrophomonas maltophilia, an opportunistic human pathogen showed altered physiological responses such as increased growth rate, biofilm formation ability as well as increased motility under microgravity conditions in comparison to normal gravity conditions (Su et al., 2021). The 13-fold rise in the growth of Escherichia coli cells was reported in microgravity conditions and the cells were also able to grow in the presence of a minimum inhibitory concentration of gentamicin sulfate (Zea et al., 2017). During long-term space missions, various studies have shown that pathogenic microbes isolated from space stations such as Pseudomonas aeruginosa, A. fumigatus, and Fusarium oxysporum also show changes in gene expression profile and physiology (Bijlani et al., 2021).
1.1.2 Microbial survival in presence of radiation in the space environment
Ionizing radiation present in space can hinders the growth of microbial cells by creating double-stranded breaks in DNA leading to space-induced mutations (Senatore et al., 2018). Moreover, radiation leads to the formation of various reactive oxygen species (ROS) which damage nucleic acid, proteins as well as lipids (Figure 2). Deinococcus radiodurans was the first radiation-resistant microbe isolated from the space atmosphere (10 km away from Earth) by a scientist named Dr. Akihiko Yamagishi from Tokyo University along with the Tanpopo space mission team. In the year 2020, they published that a thick layer surrounding the cells protects them from radiation doses for a longer duration. They kept aggregates of dried D. radiodurans in the exposure panel of ISS for 3 years and from a series of experiments they claimed that the cell aggregates with a thickness of 0.5 mm were able to withstand the exposure to radiation for 3 years. They reported that the D. radiodurans cells in the initial layers died and the cells in the internal layers were alive as they were protected by the thick layer. The DNA damage that occurred is repaired via the nucleotide excision repair mechanism involving uvrA and uvdE genes (Kawaguchi et al., 2020).
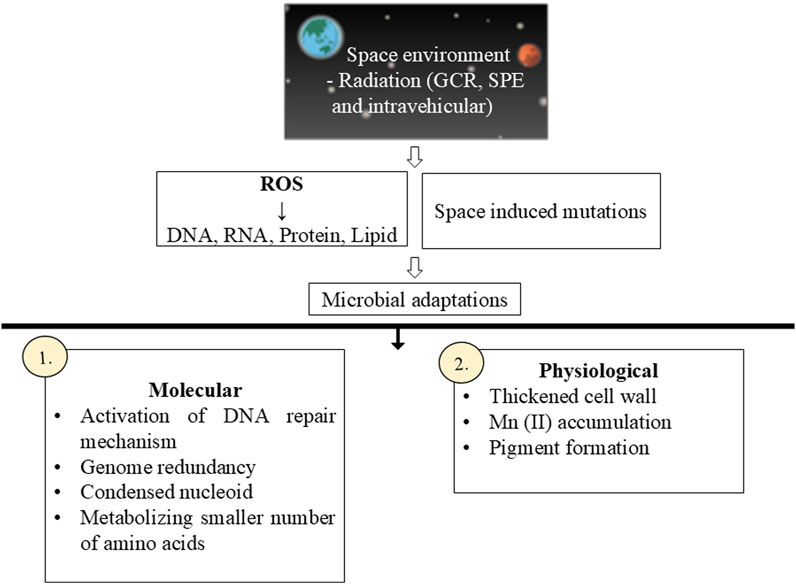
Figure 2. Microbial adaptation to space ionizing radiations. [Figure has been made using BioRender (https://biorender.com)].
Making higher copies of the genome for genome redundancy, condensed nucleoid, alternation in DNA repair mechanism, metabolizing a smaller number of amino acids, accumulating Mn (II), and pigment formation are a few of the various adaptations of bacterial cells which increase their survivability in presence of radiation (Merino et al., 2019). Bacterial cells have evolved various DNA repair mechanisms based on homologous recombination and non-homologous recombination (non-homologous end joining or NHEJ) which support their growth in the presence of radiation (Milojevic and Weckwerth, 2020) (Figure 2). Fungi activate the NHEJ pathway to repair mutations occurring as a result of radiation exposure (Bijlani et al., 2021). Some bacteria also produce a photolyase enzyme which plays an important role in the repair mechanism via photoreactivation (Senatore et al., 2018). The survivability of several bacterial species isolated from space samples has been investigated against UV irradiation and among them all Bacillus pumilus strains were found to be resistant against all three types (UVA, UVA + B, and full spectrum UV) (Newcombe et al., 2005). Rhodospirillum rubrum S1H showed changes at the transcription level when subjected to genomic and proteomic studies after they were exposed to the space environment of ISS (Mastroleo et al., 2009).
Moreover, a spore forming bacteria Bacillus subtilis, a fungus Aureobasidium pullulans and an archaea Methanosarcina mazei S-6T were deposited on cotton wool and exposed in the open space near ISS using a device designated as Test and the obtained data indicates that these microbes were able to survive there for two long years. Research team reports dehydration and lyophilization as the crucial mechanism for their survival (Deshevaya et al., 2024).
1.1.3 Microbial biofilm on spacecraft surfaces and/or cabin parts
A biofilm is the assemblage of microorganisms that grow and stick onto the surfaces in an irreversible manner where microbial cells are enclosed within the matrix composed of extracellular polymeric substances (EPS). EPS is composed of carbohydrates, lipids, proteins, and extracellular DNA and acts as a physical barrier resistant to antibiotics and disinfectants. EPS also helps in providing nutrients to microbial cells and in cell signaling (Landry et al., 2020; Diaz et al., 2019). Biofilm formation is the attribute that is enhanced due to microgravity. Biofilm formation in the water systems and air-duct of spacecraft as well as the food products carried on a mission for crew members are creating major problems (Landry et al., 2020; Vaishampayan and Grohmann, 2019). Biofilm is reported in the pipeline, electrical connectors, equipment, water recycling system, air conditioning, thermal control system, and navigating window of ISS, as seen in Salyut and Mir space stations.
Although biofilm development may be troublesome in any spacecraft system, it is especially crucial when it develops in the Water Recovery System (WRS) and Environmental Control and Life Support System (ECLSS) since these two are vital life-support systems and are responsible for supplying potable water to the crew (Taffs et al., 2009; Marra et al., 2024). WRS has the Urine Processor Assembly and Water Processor Assembly which recycle wastewater from human urine and recover humidity from the spaceflight atmosphere. So, they are continually inoculated with microbes primarily coming from space crew members. There are several phases of the biofilm development process (Figure 3). Bacteria employ a range of extracellular organelles such as fimbriae, flagella, pili, surface-associated polysaccharides or proteins for detecting and connecting to surfaces; initially, their adhesion to the pre-conditioned surface is reversible (Morales and York, 2014). Then the bacteria secrete the anchoring EPS and establish an irreversible attachment (Krumholz et al., 2015). Adsorbed cells on surfaces have the capacity to divide and form microcolonies. EPS keeps the cells close together, which is required for cell-cell communication when the bacteria start to grow. As cells multiply and EPS builds up, the community eventually develops into a three-dimensional structure called a biofilm. Bacteria have better access to nutrients in biofilm (Hornemann et al., 2008). This biofilm formation is regulated via a population density-based cell-to-cell communication process known as quorum sensing (QS) (Figure 1). It involves signaling molecules such as autoinducers (Mangwani et al., 2016; Yeon et al., 2009). Many Gram-negative bacteria utilize autoinducers such as N-acyl homoserine lactones (AHLs) to coordinate gene expression in a population-density-dependent manner (Molina et al., 2003). Microorganisms can synthesize and sense the signaling molecules, form the biofilm, and act efficiently when appropriate population density is achieved (Liao and Li, 2013). It has been reported that biofilm formation in space conditions occurs because of the anhydrobiosis process of microbial cells. Anhydrobiosis is the mechanism in which the organism loses all the water (desiccation), enters into the dormant state, becomes metabolically inactive, survives under extremely dry conditions and restores its vital function after rehydration (Grzyb and Skłodowska, 2022). It has been reported that drying protects the cells from radiation, and reactive oxygen species found in space conditions (Landry et al., 2020).
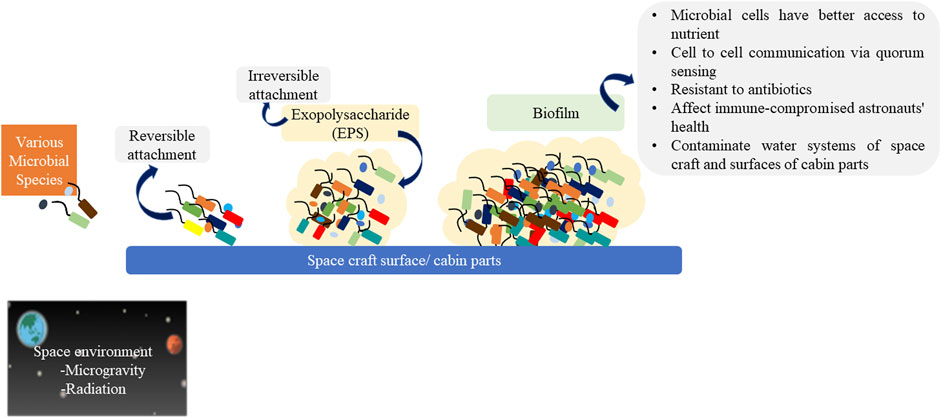
Figure 3. Biofilm formation and its effects in space craft. [Figure has been made using BioRender (https://biorender.com)].
Recently RNA-Seq, cellular proteomics, and metabolomics of “Biological Research in Canisters-23” (BRIC-23) GeneLab spaceflight experiment was conducted to determine the behavior of S. aureus in low earth orbit on ISS. The data specifies that S. aureus exhibited upregulation of RNAIII, the effector of the Accessory Gene Regulator (Agr) quorum sensing system, agr operon gene transcripts, phenol-soluble modulins and secreted proteases which are ultimately responsible for the increase in quorum sensing activity (Hauserman et al., 2024). Staphylococci and Enterococci have been found in the biofilms of spacecraft (Vaishampayan and Grohmann, 2019). Bacillus and coliforms were isolated from waste lines in space stations (Bijlani et al., 2021).
Thus, microorganisms are known to survive under space conditions and it becomes very much necessary to control their growth using antimicrobial medications or surface coating agents otherwise they may obstruct the purpose of space missions.
2 Effect of antimicrobial medicines in the space environment
Space missions can be of a few minutes to as long as 437 days and so far, around 620 crew members have gone for space missions (Cowen et al., 2024). As spaceflight mission length and distance expand, concerns grow regarding the health of crew members. Hence, the exploration of medical capability is given top priority specifically for long-duration planetary missions. The incidences of microbial infections among the crew members on board for the different space missions are managed by using antimicrobial medicines included in medical kits so far. The line of treatment for reported microbial infection during various human space programs is summarized in Table 1. Table 2 presents the list of antimicrobial drugs included in the Shuttle Orbiter Medical System (SOMS) MSMK and, HMS (Barratt and Pool, 2008; Diaz et al., 2024) along with the type of dosage form used, and recommended storage conditions. The use of antimicrobial medicines is an inevitable part of treating microbial infection during space flight and successful human space missions. However, it has been reported that the efficacy of antimicrobial agents is reduced during space travel due to mainly two factors, 1) altered human physiology in microgravity conditions and 2) instability of medicines in the space environment (Blue et al., 2019b).
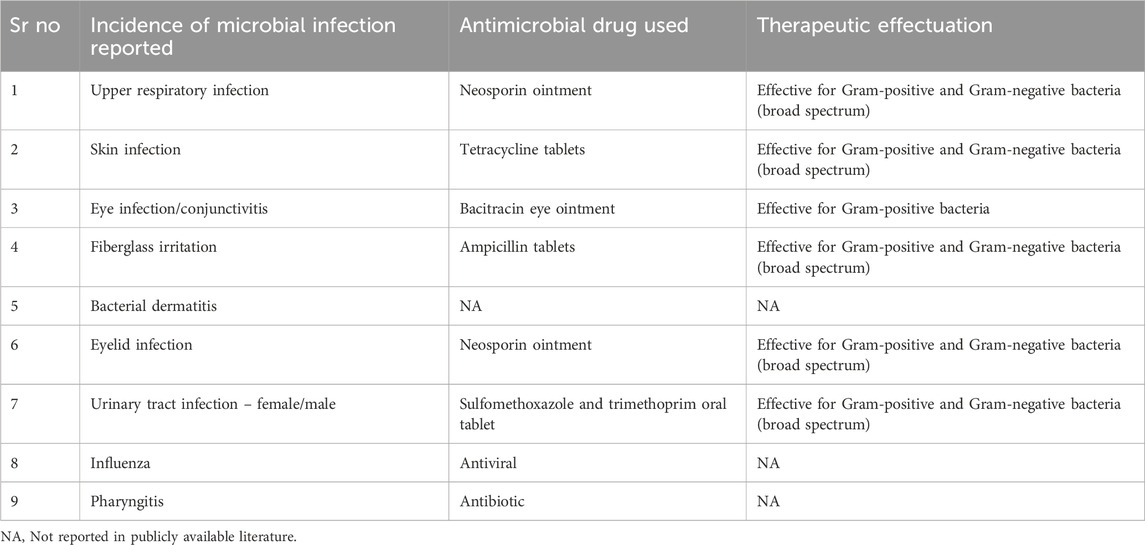
Table 1. Line of treatment for microbial infections occurred to the crew members during the Apollo, Skylab, Space Shuttle Programme, Mir and ISS missions.
2.1 Space flight induced human physiological changes
Humans undergo significant physiological and metabolic changes during spaceflight, which may stand for the altered effect of pharmaceuticals on astronauts during space flight. Limited knowledge is available regarding changes in the Pharmacokinetic and Pharmacodynamics (PK/PD) profile of drugs (i.e., absorption, distribution, metabolism, and excretion) in the human body during spaceflight (Kast et al., 2017; Barchetti et al., 2024). Changes in hepatic blood flow, altered hepatic metabolism due to varied enzyme activity, delayed gastric empty time, and the alteration in renal excretion due to body fluid shift may affect the drug PK/PD profile. Fluid shifts decrease plasma volume, impacting the distribution of hydrophilic drugs with narrow therapeutic indices, such as beta-lactams and aminoglycosides (Hodkinson et al., 2017).
Microgravity-induced alterations in gastrointestinal physiology significantly impact drug absorption. Weakly acidic drugs, such as aspirin, may exhibit increased absorption due to prolonged gastric retention, whereas basic drugs like ciprofloxacin may experience reduced absorption due to delayed gastric emptying. Additionally, drugs susceptible to degradation in acidic environments, may undergo delayed absorption or degradation, potentially reducing their therapeutic efficacy (Wani et al., 2024).
Microgravity also influences drug metabolism by modifying liver enzyme activity, particularly cytochrome P450 isoenzymes, due to reduced hepatic blood flow (Eyal, 2020). Studies under simulated microgravity conditions have demonstrated variability in the metabolism of drugs like acetaminophen and midazolam (Kast et al., 2017). Additionally, microgravity-induced impairments in renal function can reduce the clearance of renally excreted drugs, necessitating careful dose adjustments. Physiological adaptations, including fluid shifts and muscle loss, further affect drug metabolism by altering organ perfusion and function. Nutritional changes during space missions may also influence xenobiotic metabolism (Dello Russo et al., 2022).
The dosage form also plays a critical role in drug absorption under spaceflight conditions. Solid oral formulations, including tablets and capsules, may show delayed or inconsistent absorption due to microgravity-induced changes in gastric motility, whereas liquid and injectable formulations offer more predictable bioavailability. Transdermal and topical drug delivery may be influenced by changes in skin hydration, thickness, and circulation, potentially altering drug absorption rates. Similarly, aerosolized drugs may exhibit reduced pulmonary deposition due to differences in pulmonary ventilation, while extended-release formulations may be affected by variations in intestinal transit time, impacting drug release kinetics (Dello Russo et al., 2022; Reichard et al., 2023).
The pharmacodynamic effects of drugs are equally impacted. Microgravity-induced changes in tissue receptor sensitivity and cellular responses may alter drug efficacy. Antibiotics like ciprofloxacin demonstrate reduced effectiveness against bacterial strains in space, likely due to microbial physiological adaptations. Similarly, sleep-promoting medications, including zolpidem and melatonin, are frequently used to address circadian rhythm disruptions but require dose adjustments to minimize cognitive side effects during missions (Barger et al., 2014).
Most research in this domain relies on observational reports and analog studies, leaving critical gaps in understanding the physiological impacts of microgravity on medication efficacy and safety (Hodkinson et al., 2017). Developing comprehensive models to predict PK/PD changes, expanding ISS-based pharmaceutical studies, and employing advanced monitoring technologies such as wearable biosensors are essential steps forward. Such advancements will enable personalized healthcare approaches for astronauts and ensure medication safety and efficacy during extended spaceflights.
2.2 Impact of the space environment on the stability of pharmaceuticals
Stability is a very important aspect of any pharmaceutical as it is directly related to the shelf-life of a particular drug and to produce the desired efficacy. To be considered stable, the drug must retain its physical and chemical properties over a period of time. The stability of pharmaceuticals is influenced by several variables, including environmental conditions like temperature, humidity, radiation, oxygen, and product-related factors like excipients, manufacturing process, types of dosage form, packaging material, etc. (Diaz et al., 2024). To understand the quality of the drug substance and the drug product under the effect of the aforementioned conditions, pharmaceutical drug products must undergo stability testing before use. According to numerous regulatory requirements set out by organizations like the ICH, WHO, FDA, etc., accelerated and real-time stability tests are typically used to assess the stability of pharmaceutical dosage forms. Light is an important factor causing the degradation of pharmaceuticals which results in a loss of potency and formation of toxic degradation products which leads to adverse effects. So as per the ICH Q1B guideline, ‘Photostability testing of new drug substances and products’, all pharmaceuticals are exposed to the D65 (outdoor daylight) and ID65 (indoor indirect daylight) emission standards using a photostability chamber. However, the radiation environment is different in space compared to Earth (ICH, 1996; ICH, 2003). As stated in Section 1, ionizing radiation can penetrate the space shuttle and create a complex radiation environment inside. Apart from that, high concentration of CO2 in the spacecraft also enhances the diffusion of CO2 through the layers of packaging material and may cause the degradation of the medicines packed inside (Wotring, 2016; Kast et al., 2017, Blue et al., 2019a).
Du et al. (2011) first time reported the impact of the space environment on the physical and chemical stability of antimicrobial formulations along with other medicines. Total of 13 antimicrobial medicines were studied in their solid (10), semisolid (04), and liquid formulations (01). All medicines were part of medical kits (Payloads) stored on the ISS and returned via the space shuttle after an on-orbit duration ranging from 13 to 880 days. The author reported that the difference in the number of unstable formulations between flight and control increased with the length of storage time in space. Table 3 summarizes the key findings of these reports. Amoxycillin/clavulanic acid combination tablets, clotrimazole cream, silver sulfadiazine cream, and levofloxacin tablets were found to be the least stable and degraded after 353 days of exposure before their expiry. The results indicate that different types of radiation have different mechanisms to interact with drugs and can have different impacts (Du et al., 2011, Blue et al., 2019a; Wotring, 2016). Additionally, the Evidence Report, released in 2023 by NASA shows that the pharmaceuticals stored on the ISS exhibit a shorter shelf-life in space than corresponding terrestrial controls; and radiation is thought to be a possible cause. Mehta and Bhayani (2017) also delineated the mechanistic implications of space radiation on the stability of medicines in space.
Kim and Plante, (2015) suggested that the stability of pharmaceuticals in response to exposure to radio sterilization doses is a predictive approach for determining their stability against space radiation during long-term space missions. However, pharmaceutical radio sterilization is performed at doses of 25–50 kGy over a matter of minutes to hours, which far exceed the dose rates and cumulative Mars mission doses. One reported study indicates that clavulanate in a combination with amoxicillin showed remarkably increased degradation to 10 Gy exposure compared to 50 Gy exposure of proton beam (Daniels et al., 2017). So, it is necessary to assess stability at low doses for extended durations to determine the influence of the space environment on medicine (Blue et al., 2019a).
Although many gamma irradiation studies have been conducted on Earth, they often involve excessively high doses and energy levels that do not directly correspond to the space radiation environment (Al-Mohizea et al., 2007). Terrestrial research on pharmaceuticals under simulated space radiation conditions remains limited, despite the availability of high linear energy transfer (LET) radiation sources. Replicating the complex space radiation environment, including its extremely slow dose rate, presents a significant challenge. Additionally, radiation exposure studies are constrained by sample type; for example, proton, neutron, and heavy ion radiation studies are restricted to only solid samples. Conducting real-time stability studies in space is further hindered by logistical constraints, such as high launch costs and storage limitations. Technological and regulatory barriers also pose challenges, as in-flight drug analysis and synthesis technologies are still in development, and quality control measures for space-manufactured drugs lack standardized protocols (Wani et al., 2024).
Research on medication stability beyond labeled expiration dates has demonstrated that many drugs, when stored in their original, unopened packaging, retain potency significantly longer than their labeled shelf life. The Shelf-Life Extension Program (SLEP) has provided evidence supporting extended pharmaceutical stability under controlled terrestrial conditions (Psimadas et al., 2012; Lyon et al., 2006). Notably, azithromycin, fluconazole, levofloxacin, sulfamethoxazole/trimethoprim, and ciprofloxacin have shown spaceflight-induced instability, in contrast to their prolonged stability in terrestrial environments (Antonsen et al., 2017).
2.3 Antimicrobial resistance development in microbes
Antibiotics are primarily used for treating bacterial infections in space but some studies suggest that many of them are becoming less effective against the bacterial strains. Though the exact reason behind this remains uncleared, some gene sequencing studies describes that this happens because of the presence of antimicrobial resistance (AMR) genes in the genome of bacterial strains exposed to space environment (Urbaniak et al., 2018; Madrigal et al., 2022; Singh et al., 2018). Horizontal gene transfer (HGT) is one the leading process for spreading AMR among bacterial communities. Acinetobacter pittii strain IIF1SW-P1 containing two AMR genes-blaOXA-500 and ISAba1 was co-cultured under simulated microgravity (SMG) as well as under normal gravity with four S. aureus strains (IF4SW, IIF6SW, IF7SW, and IIF8SW) which does not contain these AMR genes. Gene blaOXA-500 is located on chromosome and is responsible for providing resistant to oxacillin antibiotic whereas ISAba1 is a transposable element and acts as protomer for blaOXA-500 gene. Results of PCR revealed presence of both the AMR genes in all four strains of S. aureus cultured under SMG condition because of HGT as compared to normal gravity (Urbaniak et al., 2021).
3 Microbial contamination preventive strategies
Microorganisms in space cabins can invade the surfaces of spacecraft, various equipment, and other materials and corrode them as the maximum population is of corrosive bacteria. Bacteria have corroded the aluminum and titanium present on the surface of the inner wall of ISS. Many of the materials of the Mir space station such as tanks, plastic materials, cables, and lighting systems have been damaged by the bacteria. They can even degrade the polymeric material. The antibiotic-resistant microbes in space missions not only affect astronauts’ health but can also damage the spacecraft and its equipment. Therefore, numerous safety measures have been employed to prevent microbial contamination in space flight. Currently, regular monitoring and cleaning of ISS is carried out once a week via environmental microbial control to prevent microbial contamination (Li et al., 2019).
3.1 Sterilization and disinfection
Recent studies demonstrate the effectiveness of several sterilization techniques for spaceship cleaning, including heat sterilization, chemical treatments, and ultraviolet (UV) radiation. The ability of UV-C radiation, which has wavelengths between 200 and 280 nm, to inactivate bacteria by causing damage to their DNA has been thoroughly investigated. Systems for UV sterilization have been developed by NASA, especially for space travel. By preventing harm to delicate parts, these technologies seek to guarantee that spacecraft surfaces are free from contamination by microorganisms. For missions involving the collecting of samples from space or planetary protection, the integration of UV technology is especially important as it ensures the integrity of scientific investigations and prevents the transfer of contaminants between celestial bodies (Hughes et al., 2024).
Disinfectant is the most widely used strategy employed for cleaning surfaces in space flight. Double quaternary ammonium bactericide and hydrogen peroxide-based disinfectants have been used for cleaning the US space segment of the ISS. A mixture of organosilicon quaternary ammonium salt (OQAS), sodium dehydroacetate (SD), and polyhexamethylene biguanidine hydrochloride (PHMB) based disinfectants at the concentration of 0.37 mg/L, 0.7 mg/L, and 0.5 mg/L was found to be effective against four corrosive fungi viz. Cladosporium cladosporioides, Aspergillus niger, Penicillium aurantiogriseum, and Cladosporium herbarum (Li et al., 2019). Table 4 displays the various types of disinfectants used for cleaning space flight.
The Japanese Experiment Module (Kibo) on the ISS employs advanced sterilization technologies and protocols to ensure microbiological safety and control bacterial contamination. Before launch, isopropyl alcohol was used to disinfect Kibo’s surfaces, reducing bacterial counts to below 200 cells/cm2, a critical step in preventing initial contamination. Weekly cleaning with benzalkonium chloride, an effective antimicrobial agent, maintains cleanliness but raises concerns about potential bacterial resistance due to repeated exposure (Ichijo et al., 2016).
There are certain concerns about maintaining low microbial populations in spacecraft environment. While rigorous sanitation minimizes harmful pathogens, it also reduces beneficial microbes that play a critical role in regulating immune responses. It is stated that the reduced presence of “friendly” microbes in the spacecraft environment could disrupt the immune balance, making crew members more susceptible to opportunistic infections and inflammatory responses like respiratory infections, allergic reactions, and persistent skin rashes (Rhys Blakely, 2025; Koehle et al., 2023). However, that remains subjective for spacecraft environments where cleanliness cannot be compromised so as to maintain a healthy balance of friendly microbes. There have been ample evidences for good microbes turning to be opportunistic pathogens in spaceflight conditions, which include microgravity and heightened radiation exposure. Further research would be required in this direction to identify and maintain a suitable and healthy microbial population in spacecraft conditions.
3.2 Antimicrobial coatings
Antimicrobial coatings play a crucial role in spacecraft by mitigating microbial contamination that can degrade materials, threaten astronaut health, and disrupt mission operations. These coatings can be applied to spacecraft surfaces, including walls, ceilings, floors, air filters, ventilation systems, astronaut equipment, and personal items. The two key strategies for antimicrobial coatings in spacecraft are contact-killing coatings and anti-adhesion coatings. Contact-killing coatings actively kill microrganisms upon contact using embedded antimicrobial agents. Anti-adhesion coatings prevent microbial colonization and biofilm formation (Siedenbiedel and Tiller, 2012). The mechanism of microbial coatings used to prevent microbial contamination in spacecraft is shown in Figure 4. Table 5 describes different anti-coating agents used for preventing microbial contamination on space craft surfaces and/or cabin parts.
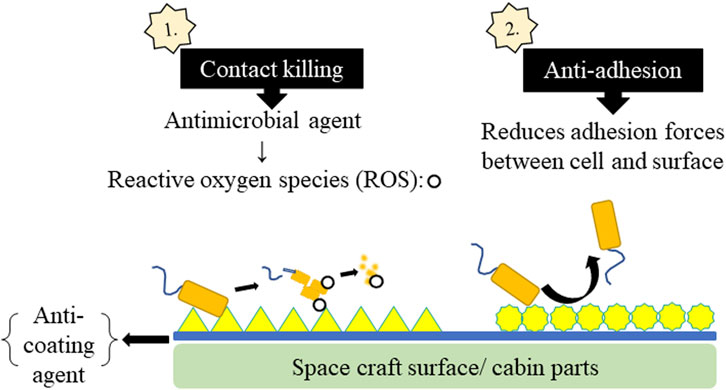
Figure 4. Mechanism of microbial coatings used for prevention of microbial contamination in spacecraft.
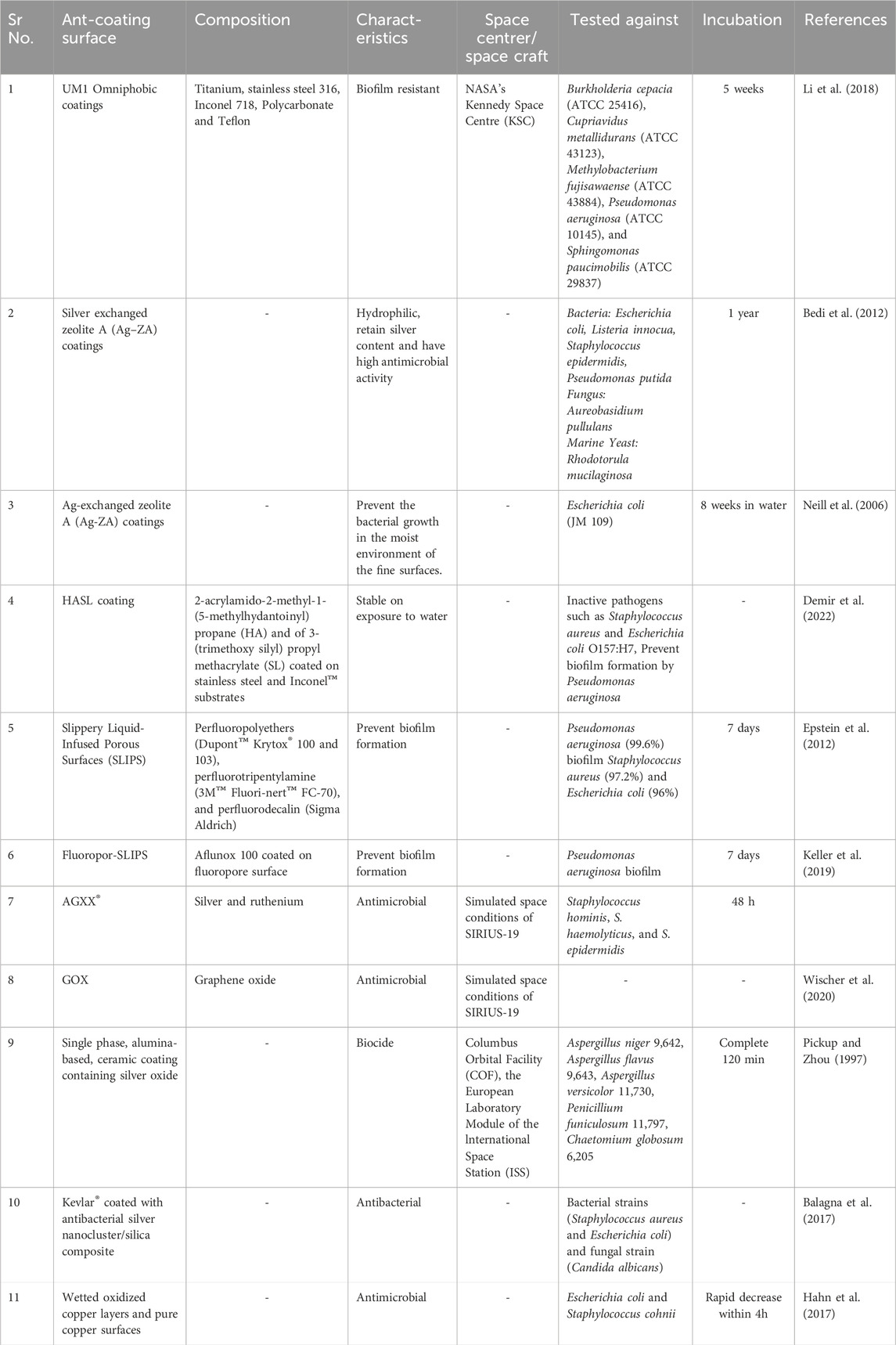
Table 5. Anti-microbial agents used for preventing microbial contamination on space craft surfaces and/or cabin parts.
Contact-killing coatings involve carrier materials that enable the controlled release of antibiotics via diffusion, erosion, or hydrolysis of covalent bonds. Common carrier materials include poly (methacrylic acid) (PMAA), polyacrylic acid (PAA), poly (lactic-co-glycolic acid) (PLGA), hydroxyapatite, polyurethane (PU), hyaluronic acid, and chitosan. Polyelectrolyte multilayers (PEMs), developed through a cost-effective layer-by-layer deposition (LBL) mechanism, trap antimicrobial agents, reducing the risk of antimicrobial resistance by preventing continuous antibiotic exposure. Organic molecules with antimicrobial activity, metal ions, and nanoparticles are commonly used for coating. These agents either physically damage microbes or create oxidative stress to kill them. Presently, nanotechnology-based self-cleaning surfaces are also being explored. Incorporating materials like copper or silver nanoparticles into spacecraft surfaces inhibits microbial growth. Additionally, semiconductors generating reactive oxygen species (ROS) through photoexcitation can eliminate microbes (Campoccia et al., 2013). Titanium dioxide coatings, used by the European Space Agency, 2022, generate ROS upon light exposure and effectively destroy microbial cells. Copper-based coatings disrupt bacterial membranes and DNA, preventing microbial colonization. However, their safety in confined environments like the International Space Station (ISS) must be carefully considered (Agarwalla et al., 2023).
Anti-adhesion coatings prevent microbial attachment by reducing adhesion forces through controlled hydrophobicity and roughness. These surfaces allow bacterial cells to be removed before biofilm formation. Materials such as hydrophobic polymer-based hydrogels, Cu/reduced graphene oxide (GO) inorganic films, mesoporous TiO2, and ZnO-containing fibers exhibit anti-adhesion properties (Ciston et al., 2008). Materials like polytetrafluoroethylene (PTFE) or fluorinated polymers reduces bacterial adhesion by creating a water-repelling surface (Yu et al., 2022). Liquid-Infused Surfaces (LIS), tested aboard the ISS, use nanostructures to create a slippery coating that prevents biofilm buildup (Marra et al., 2024).
Despite their benefits, antimicrobial coatings present challenges related to feasibility, safety, and long-term durability. Feasibility concerns arise from strict ECSS and NASA material restrictions that limit antimicrobial options to silver or copper-based coatings (European Space Agency, 2022; NASA, 2021). The use of Sn, Cd, Zn, Be, Hg, polyvinyl chloride (PVC), and radioactive substances is prohibited for use in space. Ensuring uniform application on intricate surfaces, managing curing conditions, and addressing mass and volume constraints in spacecraft design are further challenges (Mettler et al., 2022). Effective solutions must balance antimicrobial efficacy with regulatory compliance, material compatibility, and engineering constraints.
Another important concern with antimicrobial coatings is safety. Safety considerations include ensuring that volatile byproducts remain within safe exposure limits to protect astronaut health. Long-term exposure to metals like copper or silver can cause respiratory or allergic reactions, and potential leaching may pose contamination risks. Coatings must also meet stringent flammability standards to prevent fire hazards in microgravity, as some organic compounds may release flammable byproducts in oxygen-rich environments (NASA, 2021). Space conditions, including extreme temperatures, UV radiation, and outgassing, can alter coating properties, leading to degradation and potential contamination of spacecraft instruments (ECSS, 2019). Smart coatings capable of detecting microbial presence and activating antimicrobial properties as needed are being explored as a solution to these issues.
Long-term durability of coating is another challenge. Abrasion from astronaut contact, tools, or cleaning procedures can degrade coatings over time (Wang et al., 2021). Additionally, microbial populations may develop resistance, reducing coating effectiveness. Some coatings require reapplication or reactivation (through UV or chemical treatments), which is difficult due to logistical constraints in space missions. Researchers are developing self-replenishing coatings that regenerate antimicrobial activity, reducing the need for reapplication.
Use of antimicrobial coatings in the ISS, SpaceX’s Dragon capsule, and other NASA and ESA spacecraft are successful examples (Zhang et al., 2021).
3.3 Development of space hardy anti-microbial medicines
Depending upon the inherent variances in formulation or the presence of varying excipients, packaging, or other factors, the shelf life of a given medicine may change between manufacturers. About 87% of the pharmaceuticals transported on the ISS had indicated a shelf life of under 24 months. All the medicines are replaced by regular resupply to the ISS before its expiry date to provide efficacious medicines to astronauts. But planetary missions will be too distant for resupply and therefore need to be self-sufficient. Long distance missions will require stable pharmaceutical formulations and advanced packaging technologies to maintain efficacy for up to 3 years or more.
As per the proposed approaches mentioned by Bhayani et al., 2022, a ground-based space radiation analog can be developed using mixed fluencies of various ionizing radiations which will be useful to assess the stability and efficacy of medicines during space missions. Another approach is to alter packaging material for space medicine (Du et al., 2011). Pharmaceutical packaging materials should have radiation-attenuating qualities to avoid radiation-induced medication deterioration (Guetersloh et al., 2006).
Light-sensitive medications are normally packed in light-protective packaging, such as amber-colored bottles, aluminum foils, etc., to protect from sunlight (Nambiar and Yeow, 2012). But high-density polyethylene, can be combined with fillers like boron, tungsten, and other materials, shielding capabilities to be used as packaging material for space medicines (Zeitlin et al., 2005). Apart from that the excipients included in the formulation, are known to exhibit varying degrees of stability (Wotring, 2016).
Various strategies to mitigate pharmaceutical instability in space are cryopreservation, optimized container designs, and the development of space-hardy formulations (Tran et al., 2022; Montesinos et al., 2021; Seoane-Viano et al., 2022). Personalized medicine offers an additional approach by tailoring drug formulations and treatments to an astronaut’s genetic profile, health status, and specific physiological adaptations to spaceflight, thereby improving therapeutic efficacy (Krakos, 2024; Demetzos, 2016). In-flight drug synthesis technologies, such as 3D printing and microfluidic lab-on-a-chip systems, enable on-demand pharmaceutical production, reducing storage challenges and ensuring drug stability (Mu et al., 2022; Wani et al., 2024). Real-time monitoring with advanced biosensors and nanotechnology-based sensors allows continuous assessment of drug potency, detecting subtle formulation changes to maintain efficacy (Kanapskyte et al., 2021; Santa Maria et al., 2023; Nardi et al., 2024; Pavez Loriè et al., 2021; Ledet et al., 2016).
4 Conclusion
Space exploration has presented humanity with unprecedented challenges, including microbial contamination and susceptibility to microbes. Microorganisms can proliferate and form biofilms even in harsh space environments that damage spacecraft systems, contaminate essential resources and jeopardize human health. Because of their adaptability, heightened virulence, and ability to build biofilm, effective countermeasures are desperately needed, particularly for long-duration missions. Addressing these difficulties requires a multifaceted approach that integrates technological innovation, interdisciplinary collaboration, and robust policy frameworks.
Use of disinfectants and sterilization processes helps to reduce microbial load on spacecraft.
Biofilm-resistant polymers, contact-killing coating and antimicrobial coating have potentialTo reduce microbial growth on crucial surfaces. Future research studies on developing smart antimicrobial surfaces and self-cleaning surfaces have transformative potential.
Weak immunity as well as physiological and metabolic changes during space travel impact significantly on efficacy of antimicrobial medicines. Changes in hepatic blood flow, alter hepatic metabolism, delayed gastric empty time, and the alteration in renal excretion may affect the absorption, distribution, metabolism and excretion of antimicrobial drugs in the human body. It can lead to lower treatment efficacy and antimicrobial resistance development in long duration space travel.
To address these issues, future research should prioritize the development of space-tolerant pharmaceuticals with enhanced stability and bioavailability. This includes investigating novel drug formulations, encapsulation techniques using nanocarriers, and radiation-resistant packaging materials to extend drug shelf life and efficacy. Equipping space missions with optimal medical packages including stable, adaptable, and effective therapies can improve the preparedness for microbial diseases. Interdisciplinary collaboration is vital to effectively address these challenges. Scientists from different fields like microbiology, pharmacology, materials science, aerospace engineering, and medicine must collaborate to design robust countermeasures. Innovation will be accelerated by forming global research projects and collaborations with academic institutions, commercial enterprises, and space agencies. To guarantee uniformity during space missions, standardized procedures for pharmaceutical stability testing, contamination control, and microbiological monitoring should be created. To protect astronaut health and mission success, policy recommendations are as important as scientific and technological developments. Space organizations should invest in research on antimicrobial resistance in space, enforce frequent spaceship decontamination procedures, and establish strict guidelines for microbiological contamination. Furthermore, to reduce dependency on Earth-based supplies during long-term missions, policies should also encourage and support the development of in-situ pharmaceutical production technologies, such as bioprinting or microgravity-adapted synthesis. Finally, proactive research paired with breakthrough technologies and interdisciplinary collaboration can guarantee the success and safety of future space expeditions by regulating microbiological contamination as well as pharmacological stability. As mankind pushes the boundaries of space travel, these improvements will not only ensure astronauts’ health but also establish the framework for sustainable human habitation beyond Earth. The lessons learned from space-based microbial and pharmacological research can also drive innovations in healthcare, biotechnology, and environmental management on Earth.
Author contributions
MP: Conceptualization, Investigation, Writing – original draft, Writing – review and editing. PP: Conceptualization, Investigation, Writing – original draft, Writing – review and editing. NM: Investigation, Writing – original draft. ShiP: Investigation, Writing – original draft. ShrP: Investigation, Writing – original draft. AS: Investigation, Writing – original draft. JD: Investigation, Writing – review and editing. RD: Investigation, Writing – review and editing. PM: Conceptualization, Supervision, Validation, Visualization, Writing – review and editing.
Funding
The author(s) declare that no financial support was received for the research and/or publication of this article.
Acknowledgments
The authors are thankful to Institute of Pharmacy and Institute of Science, Nirma University, Ahmedabad, India. Authors are also thankful to ResearchSat Pty Ltd., Australia. Miss Manali Patel is thankful for Research Fellowship provided by National Fellowship for Students with Disabilities, University Grants Commission (NFPWD, UGC), New Delhi, India (File No. NFPWD-202 1-22-GUJ-10597).
Conflict of interest
Authors ShrP, JD, and RD were employed by ResearchSat Pvt Ltd.
The remaining authors declare that the research was conducted in the absence of any commercial or financial relationships that could be construed as a potential conflict of interest.
Generative AI statement
The author(s) declare that no Generative AI was used in the creation of this manuscript.
Publisher’s note
All claims expressed in this article are solely those of the authors and do not necessarily represent those of their affiliated organizations, or those of the publisher, the editors and the reviewers. Any product that may be evaluated in this article, or claim that may be made by its manufacturer, is not guaranteed or endorsed by the publisher.
References
Agarwalla, A., Ahmed, W., Al-Marzouqi, A. H., Rizvi, T. A., Khan, M., and Zaneldin, E. (2023). Characteristics and key features of antimicrobial materials and associated mechanisms for diverse applications. Molecules 28 (24), 8041. doi:10.3390/molecules28248041
Al-Mohizea, A. M., El-Bagory, I. M., Alsarra, I. A., Al-Jenoobi, F. I., and Bayomi, M. A. (2007). Effect of gamma radiation on the physicochemical properties of ciprofloxacin in solid state. J. drug Deliv. Sci. Technol. 17 (3), 211–215. doi:10.1016/S1773-2247(07)50038-9
Antonsen, E., Bayuse, T., Blue, R., Daniels, V., Hailey, M., Hussey, S., et al. (2017). Evidence report: risk of adverse health outcomes and decrements in performance due to in-flight medical conditions human research program exploration medical capabilities element approved for public release. Available online at: https://ntrs.nasa.gov/citations/20170004604.1–80.
Aunins, T. R., Erickson, K. E., Prasad, N., Levy, S. E., Jones, A., Shrestha, S., et al. (2018). Spaceflight modifies Escherichia coli gene expression in response to antibiotic exposure and reveals role of oxidative stress response. Front. Microbiol. 9, 310. doi:10.3389/fmicb.2018.00310
Balagna, C., Irfan, M., Perero, S., Miola, M., Maina, G., Santella, D., et al. (2017). Characterization of antibacterial silver nanocluster/silica composite coating on high performance Kevlar® textile. Surf. Coatings Technol. 321, 438–447. doi:10.1016/j.surfcoat.2017.05.009
Barchetti, K., Derobertmasure, A., Boutouyrie, P., and Sestili, P. (2024). Redefining space pharmacology: bridging knowledge gaps in drug efficacy and safety for deep space missions. Front. Space Technol. 5, 1456614. doi:10.3389/frspt.2024.1456614
Barger, L. K., Flynn-Evans, E. E., Kubey, A., Walsh, L., Ronda, J. M., Wang, W., et al. (2014). Prevalence of sleep deficiency and use of hypnotic drugs in astronauts before, during, and after spaceflight: an observational study. Lancet Neurology 13 (9), 904–912. doi:10.1016/S1474-4422(14)70122-X
M. R. Barratt, and S. L. Pool (2008). Principles of clinical medicine for space flight (Springer Science and Business Media). doi:10.1007/978-1-4939-9889-0
Bedi, R. S., Cai, R., O’Neill, C., Beving, D. E., Foster, S., Guthrie, S., et al. (2012). Hydrophilic and antimicrobial Ag-exchanged zeolite a coatings: a year-long durability study and preliminary evidence for their general microbiocidal efficacy to bacteria, fungus and yeast. Microporous Mesoporous Mater. 151, 352–357. doi:10.1016/j.micromeso.2011.10.012
Bhayani, D., Mehta, P., Patel, M., Naik, H., Nathaniel, T. N., and Khan, S. (2022). Ground-based selected ionizing space radiation effects on stability of APIs and their formulations. J. Pharm. Biomed. Analysis 220, 115019. doi:10.1016/j.jpba.2022.115019
Bijlani, S., Stephens, E., Singh, N. K., Venkateswaran, K., and Wang, C. C. (2021). Advances in space microbiology. Iscience 24 (5), 102395–102419. doi:10.1016/j.isci.2021.102395
Blue, R. S., Bayuse, T. M., Daniels, V. R., Wotring, V. E., Suresh, R., Mulcahy, R. A., et al. (2019a). Supplying a pharmacy for NASA exploration spaceflight: challenges and current understanding. npj Microgravity 5 (1), 14. doi:10.1038/s41526-019-0075-2
Blue, R. S., Chancellor, J. C., Antonsen, E. L., Bayuse, T. M., Daniels, V. R., and Wotring, V. E. (2019b). Limitations in predicting radiation-induced pharmaceutical instability during long-duration spaceflight. npj Microgravity 5 (1), 15. doi:10.1038/s41526-019-0076-1
Campoccia, D., Montanaro, L., and Arciola, C. R. (2013). A review of the biomaterials technologies for infection-resistant surfaces. Biomaterials 34 (34), 8533–8554. doi:10.1016/j.biomaterials.2013.07.089
Chancellor, J. C., Scott, G. B., and Sutton, J. P. (2014). Space radiation: the number one risk to astronaut health beyond low earth orbit. Life 4 (3), 491–510. doi:10.3390/life4030491
Ciston, S., Lueptow, R. M., and Gray, K. A. (2008). Bacterial attachment on reactive ceramic ultrafiltration membranes. J. Membr. Sci. 320 (1-2), 101–107. doi:10.1016/j.memsci.2008.03.065
Cowen, D., Zhang, R., and Komorowski, M. (2024). Infections in long-duration space missions. Lancet Microbe 5, 100875. doi:10.1016/S2666-5247(24)00098-3
Daniels, V. R., Bayuse, T. M., McGuire, K. M., Antonsen, E. L., and Putcha, L. (2017). “Radiation impact on pharmaceutical stability: retrospective data review,” in NASA human research program investigators' workshop (HRP IWS 2018).
Danko, D., Malli Mohan, G. B., Sierra, M. A., Rucker, M., Singh, N. K., Regberg, A. B., et al. (2021). Characterization of spacesuit associated microbial communities and their implications for NASA missions. Front. Microbiol. 12, 608478. doi:10.3389/fmicb.2021.608478
Dello Russo, C., Bandiera, T., Monici, M., Surdo, L., Yip, V. L. M., Wotring, V., et al. (2022). Physiological adaptations affecting drug pharmacokinetics in space: what do we really know? A critical review of the literature. Br. J. Pharmacol. 179 (11), 2538–2557. doi:10.1111/bph.15822
Demetzos, C. (2016). Pharmaceutical nanotechnology: fundamentals and practical applications, doi:10.1007/978-981-10-0791-0
Demir, B., Taylor, A., Broughton, R. M., Huang, T. S., Bozack, M. J., and Worley, S. D. (2022). N-halamine surface coating for mitigation of biofilm and microbial contamination in water systems for space travel. Biofilm 4, 100076. doi:10.1016/j.bioflm.2022.100076
Deshevaya, E. A., Fialkina, S. V., Shubralova, E. V., Tsygankov, O. S., Khamidullina, N. M., Vasilyak, L. M., et al. (2024). Survival of microorganisms during two-year exposure in outer space near the ISS. Sci. Rep. 14 (1), 334. doi:10.1038/s41598-023-49525-z
Diaz, A. M., Li, W., Calle, L. M., and Callahan, M. R. (2019). “Investigation of biofilm formation and control for spacecraft-an early literature review,” in International conference on environmental systems (No. KSC-E-DAA-TN68829).
Diaz, T. E., Ives, E. C., Lazare, D. I., and Buckland, D. M. (2024). Expiration analysis of the International Space Station formulary for exploration mission planning. npj Microgravity 10 (1), 76. doi:10.1038/s41526-024-00414-3
Du, B., Daniels, V. R., Vaksman, Z., Boyd, J. L., Crady, C., and Putcha, L. (2011). Evaluation of physical and chemical changes in pharmaceuticals flown on space missions. AAPS J. 13, 299–308. doi:10.1208/s12248-011-9270-0
Epstein, A. K., Wong, T. S., Belisle, R. A., Boggs, E. M., and Aizenberg, J. (2012). Liquid-infused structured surfaces with exceptional anti-biofouling performance. Proc. Natl. Acad. Sci. 109 (33), 13182–13187. doi:10.1073/pnas.1201973109
European Cooperation for Space Standardization (2019). Space environment (ECSS-E-ST-10-04C Rev.1). European Space Agency, France. Available online at: https://ecss.nl.
Eyal, S. (2020). How do the pharmacokinetics of drugs change in astronauts in space? Expert Opin. drug metabolism and Toxicol. 16 (5), 353–356. doi:10.1080/17425255.2020.1746763
Grzyb, T., and Skłodowska, A. (2022). Introduction to bacterial anhydrobiosis: a general perspective and the mechanisms of desiccation-associated damage. Microorganisms 10 (2), 432. doi:10.3390/microorganisms10020432
Guetersloh, S., Zeitlin, C., Heilbronn, L., Miller, J., Komiyama, T., Fukumura, A., et al. (2006). Polyethylene as a radiation shielding standard in simulated cosmic-ray environments. Nucl. Instrum. Methods Phys. Res. Sect. B Beam Interact. Mater. Atoms 252 (2), 319–332. doi:10.1016/j.nimb.2006.08.019
Hahn, C., Hans, M., Hein, C., Mancinelli, R. L., Mücklich, F., Wirth, R., et al. (2017). Pure and oxidized copper materials as potential antimicrobial surfaces for spaceflight activities. Astrobiology 17 (12), 1183–1191. doi:10.1089/ast.2016.1620
Hauserman, M. R., Ferraro, M. J., Carroll, R. K., and Rice, K. C. (2024). Altered quorum sensing and physiology of Staphylococcus aureus during spaceflight detected by multi-omics data analysis. npj Microgravity 10 (1), 2. doi:10.1038/s41526-023-00343-7
Hodkinson, P. D., Anderton, R. A., Posselt, B. N., and Fong, K. J. (2017). An overview of space medicine. Br. J. Anaesth. 119 (1), i143–i153. doi:10.1093/bja/aex336
Hornemann, J. A., Lysova, A. A., Codd, S. L., Seymour, J. D., Busse, S. C., Stewart, P. S., et al. (2008). Biopolymer and water dynamics in microbial biofilm extracellular polymeric substance. Biomacromolecules 9 (9), 2322–2328. doi:10.1021/bm800269h
Huang, B., Li, D., Huang, Y., and Liu, C. (2018). Effects of spaceflight and simulated microgravity on microbial growth and secondary metabolism. Mil. Med. Res. 5, 18. doi:10.1186/s40779-018-0162-9
Hughes, D. W., Cataldo, G., Pellerano, F. A., Hardwick, T. C., Micalizzi, F., Chambers, V. J., et al. (2024). Lessons learned in designing a proposed ultraviolet sterilization system for space. Aerospace 11 (7), 538. doi:10.3390/aerospace11070538
Ichijo, T., Yamaguchi, N., Tanigaki, F., Shirakawa, M., and Nasu, M. (2016). Four-year bacterial monitoring in the international space station—Japanese experiment Module “Kibo” with culture independent approach. npj Microgravity 2, 16007. doi:10.1038/npjmgrav.2016.7
ICH Q1A(R2) (2003). International Conference on Harmonization (ICH). Guidance for industry: Q1A(R2) stability testing of new drug substances and products. 24.
ISS National Laboratory. Available online at: Issnationallab.org/about/iss-timeline/ (2022). (Accessed October 4, 2022]
Kanapskyte, A., Hawkins, E. M., Liddell, L. C., Bhardwaj, S. R., Gentry, D., and Santa Maria, S. R. (2021). Space biology research and biosensor technologies: past, present, and future. Biosensors 11 (2), 38. doi:10.3390/bios11020038
Kast, J., Yu, Y., Seubert, C. N., Wotring, V. E., and Derendorf, H. (2017). Drugs in space: pharmacokinetics and pharmacodynamics in astronauts. Eur. J. Pharm. Sci. 109, S2–S8. doi:10.1016/j.ejps.2017.05.025
Kawaguchi, Y., Shibuya, M., Kinoshita, I., Yatabe, J., Narumi, I., Shibata, H., et al. (2020). DNA damage and survival time course of deinococcal cell pellets during 3 years of exposure to outer space. Front. Microbiol. 11, 2050. doi:10.3389/fmicb.2020.02050
Keller, N., Bruchmann, J., Sollich, T., Richter, C., Thelen, R., Kotz, F., et al. (2019). Study of biofilm growth on slippery liquid-infused porous surfaces made from fluoropor. ACS Appl. Mater. and interfaces 11 (4), 4480–4487. doi:10.1021/acsami.8b12542
Kim, M., and Plante, I. (2015). An assessment of how radiation incurred during a mars mission could affect food and pharmaceuticals. Wyle Sci. Technol. Eng. Group—NASA Houst. TX, U. S. A. Available online at: https://three.jsc.nasa.gov/articles/Food_and_pharmaceuticals_Paper.pdf
Koehle, A. P., Brumwell, S. L., Seto, E. P., Lynch, A. M., and Urbaniak, C. (2023). Microbial applications for sustainable space exploration beyond low Earth orbit. npj Microgravity 9 (1), 47. doi:10.1038/s41526-023-00285-0
Koenig, D. W., Mishra, S. K., and Pierson, D. L. (1995). Removal of Burkholderia cepacia biofilms with oxidants. Biofouling 9 (1), 51–62. doi:10.1080/08927019509378291
Krakos, A. (2024). Lab-on-chip technologies for space research—current trends and prospects. Microchim. Acta 191 (1), 31. doi:10.1007/s00604-023-06084-4
Krumholz, L. R., Bradstock, P., Sheik, C. S., Diao, Y., Gazioglu, O., Gorby, Y., et al. (2015). Syntrophic growth of Desulfovibrio alaskensis requires genes for H2 and formate metabolism as well as those for flagellum and biofilm formation. Appl. Environ. Microbiol. 81 (7), 2339–2348. doi:10.1128/AEM.03358-14
Landry, K. S., Morey, J. M., Bharat, B., Haney, N. M., and Panesar, S. S. (2020). Biofilms—impacts on human health and its relevance to space travel. Microorganisms 8 (7), 998. doi:10.3390/microorganisms8070998
Ledet, G. A., Biswas, S., Kumar, V. P., Graves, R. A., Mitchner, D. M., Parker, T. M., et al. (2016). Development of orally administered γ-tocotrienol (GT3) nanoemulsion for radioprotection. Int. J. Mol. Sci. 18 (1), 28. doi:10.3390/ijms18010028
Li, L., Fu, Y., and Liu, H. (2019). Development of effective and safe compound disinfectant for space cabins. Acta Astronaut. 159, 480–485. doi:10.1016/j.actaastro.2019.01.037
Li, W., Hummerick, M., Khodadad, C., Buhrow, J., Spencer, L., and Coutts, J. (2018). “Biofilm resistant coatings for space applications,” in 48th international conference on environmental systems. ICES-2018-83, 1–15.
Liao, N. Q., and Li, H. M. (2013). Conceivable bioremediation techniques based on quorum sensing. Appl. Mech. Mater. 295, 39–44. doi:10.4028/www.scientific.net/AMM.295-298.39
Lyon, R. C., Taylor, J. S., Porter, D. A., Prasanna, H. R., and Hussain, A. S. (2006). Stability profiles of drug products extended beyond labeled expiration dates. J. Pharm. Sci. 95, 1549–1560. doi:10.1002/jps.20636
Madrigal, P., Singh, N. K., Wood, J. M., Gaudioso, E., Hernández-del-Olmo, F., Mason, C. E., et al. (2022). Machine learning algorithm to characterize antimicrobial resistance associated with the International Space Station surface microbiome. Microbiome 10 (1), 134. doi:10.1186/s40168-022-01332-w
Mangwani, N., Kumari, S., and Das, S. (2016). Bacterial biofilms and quorum sensing: fidelity in bioremediation technology. Biotechnol. Genet. Eng. Rev. 32 (1-2), 43–73. doi:10.1080/02648725.2016.1196554
Marchin, G. L. (1997). Chemical disinfection under conditions of microgravity. AIP Conf. Proc. 387 (1), 749–754. doi:10.1063/1.52076
Marchin, G. L., Silverstein, J., and Brion, G. M. (1997). Effect of microgravity on Escherichia coli and MS-2 bacteriophage disinfection by iodinated resins. Acta astronaut. 40 (1), 65–68. doi:10.1016/s0094-5765(97)00026-x
Marra, D., Ferraro, R., and Caserta, S. (2024). Biofilm contamination in confined space stations: reduction, coexistence or an opportunity? Front. Mater. 11, 1374666. doi:10.3389/fmats.2024.1374666
Mastroleo, F., Van Houdt, R., Leroy, B., Benotmane, M. A., Janssen, A., Mergeay, M., et al. (2009). Experimental design and environmental parameters affect Rhodospirillum rubrum S1H response to space flight. ISME J. 3 (12), 1402–1419. doi:10.1038/ismej.2009.74
Mehta, P., and Bhayani, D. (2017). Impact of space environment on stability of medicines: challenges and prospects. J. Pharm. Biomed. analysis 136, 111–119. doi:10.1016/j.jpba.2016.12.040
Merino, N., Aronson, H. S., Bojanova, D. P., Feyhl-Buska, J., Wong, M. L., Zhang, S., et al. (2019). Living at the extremes: extremophiles and the limits of life in a planetary context. Front. Microbiol. 10, 780. doi:10.3389/fmicb.2019.00780
Mettler, M. K., Parker, C. W., Venkateswaran, K., and Peyton, B. M. (2022). Antimicrobial coating efficacy for prevention of Pseudomonas aeruginosa biofilm growth on ISS water system materials. Front. Microbiol. 13, 874236. doi:10.3389/fmicb.2022.874236
Milojevic, T., and Weckwerth, W. (2020). Molecular mechanisms of microbial survivability in outer space: a systems biology approach. Front. Microbiol. 11, 923. doi:10.3389/fmicb.2020.00923
Mintsouli, I., Tsiridis, V., Petala, M., Pliatsikas, N., Rebeyre, P., Darakas, E., et al. (2017). Behavior of Ti-6Al-4 V surfaces after exposure to water disinfected with ionic silver. Appl. Surf. Sci. 427, 763–770. doi:10.1016/j.apsusc.2017.08.031
Molina, L., Constantinescu, F., Michel, L., Reimmann, C., Duffy, B., and Défago, G. (2003). Degradation of pathogen quorum-sensing molecules by soil bacteria: a preventive and curative biological control mechanism. FEMS Microbiol. Ecol. 45 (1), 71–81. doi:10.1016/S0168-6496(03)00125-9
Montesinos, C. A., Khalid, R., Cristea, O., Greenberger, J. S., Epperly, M. W., Lemon, J. A., et al. (2021). Space radiation protection countermeasures in microgravity and planetary exploration. Life 11 (8), 829. doi:10.3390/life11080829
Morales, D. K., and York, N. (2014). “Microbial biofilms in human disease,” in Reference Module in biomedical research, 1–11. doi:10.1016/B978-0-12-801238-3.00132-X
Mu, X., He, W., Rivera, V. A. M., De Alba, R. A. D., Newman, D. J., and Zhang, Y. S. (2022). Small tissue chips with big opportunities for space medicine. Life Sci. space Res. 35, 150–157. doi:10.1016/j.lssr.2022.09.002
Nambiar, S., and Yeow, J. T. (2012). Polymer-composite materials for radiation protection. ACS Appl. Mater. and interfaces 4 (11), 5717–5726. doi:10.1021/am300783d
Nardi, L., Davis, N. M., Sansolini, S., Albuquerque, T. B.De, Laarraj, M., Caputo, D., et al. (2024). APHRODITE: a compact lab-on-chip biosensor for the real-time analysis of salivary biomarkers in space missions. Biosensors 14 (2), 72. doi:10.3390/bios14020072
NASA (2025a). International Space Station. Available online at: https://www.nasa.gov/reference/international-space-station/(Accessed March 8, 2025).
NASA (2025b). Monitoring Microorganisms. Available online at: https://www.nasa.gov/missions/station/iss-research/monitoring-microorganisms/(Accessed March 8, 2025).
Neill, C. O, Beving, D. E., Chen, W., and Yan, Y. (2006). Durability of Hydrophilic and Antimicrobial Zeolite Coatings under Water Immersion. AIChE J. 52 (3), 1157–1161. doi:10.1002/aic.10695
Newcombe, D. A., Schuerger, A. C., Benardini, J. N., Dickinson, D., Tanner, R., and Venkateswaran, K. (2005). Survival of spacecraft-associated microorganisms under simulated martian UV irradiation. Appl. Environ. Microbiol. 71 (12), 8147–8156. doi:10.1128/AEM.71.12.8147-8156.2005
Pavez Loriè, E., Baatout, S., Choukér, A., Buchheim, J. I., Baselet, B., Dello Russo, C., et al. (2021). The future of personalized medicine in space: from observations to countermeasures. Front. Bioeng. Biotechnol. 9, 739747. doi:10.3389/fbioe.2021.739747
Pennell, K. L., and Blatchley, E. R. (2004). Complementary disinfection (UV irradiation and iodination) for long-term space missions: preliminary system design. SAE Tech. Pap. doi:10.4271/2004-01-2516
Petala, M., Tsiridis, V., Mintsouli, I., Pliatsikas, N., Spanos, T., Rebeyre, P., et al. (2017). Silver deposition on stainless steel container surfaces in contact with disinfectant silver aqueous solutions. Appl. Surf. Sci. 396, 1067–1075. doi:10.1016/j.apsusc.2016.11.090
Pickup, H., and Zhou, S. J. (1997). Development of a hydrophilic, antimicrobial coating for condensing heat exchangers. SAE Tech. Pap., 972408. doi:10.4271/972408
Psimadas, D., Georgoulias, P., Valotassiou, V., and Loudos, G. (2012). Molecular nanomedicine towards cancer: ¹¹¹In-labeled nanoparticles. J. Pharm. Sci. 101 (7), 2271–2280. doi:10.1002/jps.23146
Pudova, O. G. B., Zharkova, O. G. A., Novikov, S. V., and Tatarinov, V. V. (2021). Development of disinfecting films for repairing surface deterioration of manned spacecraft, caused by microorganisms-biodestructors. AIP Conf. Proc. 2318 (1), 160002. doi:10.1063/5.0036040
Reichard, J. F., Phelps, S. E., Lehnhardt, K. R., Young, M., and Easter, B. D. (2023). The effect of long-term spaceflight on drug potency and the risk of medication failure. npj Microgravity 9 (1), 35. doi:10.1038/s41526-023-00271-6
Rhys Blakely (2025). Space station ‘too clean’ for good of astronauts’ health. Times. Available online at: https://www.thetimes.com/uk/science/article/space-station-too-clean-for-good-of-astronauts-health-5fjdmdrvw?region=global.
Santa Maria, S. R., Marina, D. B., Massaro Tieze, S., Liddell, L. C., and Bhattacharya, S. (2023). BioSentinel: long-term Saccharomyces cerevisiae preservation for a deep space biosensor mission. Astrobiology 23 (6), 617–630. doi:10.1089/ast.2019.2073
Santomartino, R., Waajen, A. C., de Wit, W., Nicholson, N., Parmitano, L., Loudon, C. M., et al. (2020). No effect of microgravity and simulated Mars gravity on final bacterial cell concentrations on the International Space Station: applications to space bioproduction. Front. Microbiol. 11, 579156. doi:10.3389/fmicb.2020.579156
Senatore, G., Mastroleo, F., Leys, N., and Mauriello, G. (2018). Effect of microgravity and space radiation on microbes. Future Microbiol. 13 (7), 831–847. doi:10.2217/fmb-2017-0251
Seoane-Viano, I., Ong, J. J., Basit, A. W., and Goyanes, A. (2022). To infinity and beyond: strategies for fabricating medicines in outer space. Int. J. Pharm. 4, 100121. doi:10.1016/j.ijpx.2022.100121
Shakir, Y., Rcheulishvili, N., Zhang, Y., and Deng, Y. (2022). Effects of short-term space conditions on cellulose degradation ability and biodiversity of microorganisms. Cellulose 29 (9), 5061–5077. doi:10.1007/s10570-022-04574-x
Siedenbiedel, F., and Tiller, J. C. (2012). Antimicrobial polymers in solution and on surfaces: overview and functional principles. Polymers 4 (1), 46–71. doi:10.3390/polym4010046
Simoes, M. F., and Antunes, A. (2021). Microbial pathogenicity in space. Pathogens 10 (4), 450. doi:10.3390/pathogens10040450
Singh, N. K., Wood, J. M., Karouia, F., and Venkateswaran, K. (2018). Succession and persistence of microbial communities and antimicrobial resistance genes associated with International Space Station environmental surfaces. Microbiome 6, 204–223. doi:10.1186/s40168-018-0585-2
Su, X., Guo, Y., Fang, T., Jiang, X., Wang, D., Li, D., et al. (2021). Effects of simulated microgravity on the physiology of Stenotrophomonas maltophilia and multiomic analysis. Front. Microbiol. 12, 701265. doi:10.3389/fmicb.2021.701265
Szydlowski, L. M., Bulbul, A. A., Simpson, A. C., Kaya, D. E., Singh, N. K., Sezerman, U. O., et al. (2024). Adaptation to space conditions of novel bacterial species isolated from the International Space Station revealed by functional gene annotations and comparative genome analysis. Microbiome 12 (1), 190. doi:10.1186/s40168-024-01916-8
Taffs, R., Aston, J. E., Brileya, K., Jay, Z., Klatt, C. G., McGlynn, S., et al. (2009). In silico approaches to study mass and energy flows in microbial consortia: a syntrophic case study. BMC Syst. Biol. 3, 114–116. doi:10.1186/1752-0509-3-114
Tran, Q. D., Tran, V., Toh, L. S., Williams, P. M., Tran, N. N., and Hessel, V. (2022). Space medicines for space health. ACS Med. Chem. Lett. 13 (8), 1231–1247. doi:10.1021/acsmedchemlett.1c00681
Urbaniak, C., Grams, T., Mason, C. E., and Venkateswaran, K. (2021). Simulated microgravity promotes horizontal gene transfer of antimicrobial resistance genes between bacterial genera in the absence of antibiotic selective pressure. Life 11 (9), 960. doi:10.3390/life11090960
Urbaniak, C., Morrison, M. D., Thissen, J. B., Karouia, F., Smith, D. J., Mehta, S., et al. (2022). Microbial Tracking-2, a metagenomics analysis of bacteria and fungi onboard the International Space Station. Microbiome 10 (1), 100. doi:10.1186/s40168-022-01293-0
Urbaniak, C., Tesei, D., and Van Houdt, R. (2024). Editorial: the impact of the space environment on microbial growth and behavior. Front. Microbiol. 15, 1390100. doi:10.3389/fmicb.2024.1390100
Vaishampayan, A., and Grohmann, E. (2019). Multi-resistant biofilm-forming pathogens on the International Space Station. J. Biosci. 44, 125–5. doi:10.1007/s12038-019-9929-8
Vávrová, S., Struhárňanská, E., Turňa, J., and Stuchlík, S. (2021). Tellurium: a rare element with influence on prokaryotic and eukaryotic biological systems. Int. J. Mol. Sci. 22 (11), 5924. doi:10.3390/ijms22115924
Wang, M., Duday, D., Scolan, E., Perbal, S., Prato, M., Lasseur, C., et al. (2021). Antimicrobial surfaces for applications on confined inhabited space stations. Adv. Mater. Interfaces 8 (13), 2100118. doi:10.1002/admi.202100118
Wani, A., Prabhakar, B., and Shende, P. (2024). Strategic aspects of space medicine: a journey from conventional to futuristic requisites. Space Sci. and Technol. 4, 0123. doi:10.34133/space.0123
Wischer, D., Schneider, D., Poehlein, A., Herrmann, F., Oruc, H., Meinhardt, J., et al. (2020). Novel antimicrobial cellulose fleece inhibits growth of human-derived biofilm-forming staphylococci during the SIRIUS19 simulated space mission. Front. Microbiol. 11, 1626. doi:10.3389/fmicb.2020.01626
Wong, W. C., Dudinsky, L. A., Garcia, V. M., Ott, C. M., and Castro, V. A. (2010). Efficacy of various chemical disinfectants on biofilms formed in spacecraft potable water system components. Biofouling 26 (5), 583–586. doi:10.1080/08927014.2010.495772
Wotring, V. E. (2016). Chemical potency and degradation products of medications stored over 550 earth days at the international space station. AAPS J. 18, 210–216. doi:10.1208/s12248-015-9834-5
Yeon, K. M., Cheong, W. S., Oh, H. S., Lee, W. N., Hwang, B. K., Lee, C. H., et al. (2009). Quorum sensing: a new biofouling control paradigm in a membrane bioreactor for advanced wastewater treatment. Environ. Sci. and Technol. 43 (2), 380–385. doi:10.1021/es8019275
Zea, L., Larsen, M., Estante, F., Qvortrup, K., Moeller, R., de Oliveira, S. D., et al. (2017). Phenotypic changes exhibited by E. coli cultured in space. Front. Microbiol. 8, 1598. doi:10.3389/fmicb.2017.01598
Zea, L., Prasad, N., Levy, S. E., Stodieck, L., Jones, A., Shrestha, S., et al. (2016). A molecular genetic basis explaining altered bacterial behavior in space. PloS one 11 (11), e0164359. doi:10.1371/journal.pone.0164359
Zeitlin, C., Guetersloh, S., Heilbronn, L., and Miller, J. (2005). Shielding and fragmentation studies. Radiat. Prot. Dosim. 116 (1-4), 123–124. doi:10.1093/rpd/nci064
Keywords: microbes in space, microgravity, space radiations, antibiotics instability, disinfectant, anti-microbial coating agents
Citation: Patel M, Patel P, Munshi NS, Patel S, Patil S, Srivastva A, Dhanraj J, Duggineni R and Mehta P (2025) Microbial contamination and pharmaceutical stability in space environment: addressing dual challenge with innovative technologies and sustainable practices. Front. Space Technol. 6:1553854. doi: 10.3389/frspt.2025.1553854
Received: 31 December 2024; Accepted: 31 March 2025;
Published: 30 April 2025.
Edited by:
Yasmeen Shakir, Hazara University, PakistanReviewed by:
Talal Jamil Qazi, University of São Paulo, BrazilQiao Wu, Zhongshan Hospital, Fudan University, China
Copyright © 2025 Patel, Patel, Munshi, Patel, Patil, Srivastva, Dhanraj, Duggineni and Mehta. This is an open-access article distributed under the terms of the Creative Commons Attribution License (CC BY). The use, distribution or reproduction in other forums is permitted, provided the original author(s) and the copyright owner(s) are credited and that the original publication in this journal is cited, in accordance with accepted academic practice. No use, distribution or reproduction is permitted which does not comply with these terms.
*Correspondence: Priti Mehta, ZHJwcml0aW1laHRhQG5pcm1hdW5pLmFjLmlu, cGptZWh0YTAxMTFAZ21haWwuY29t
†These authors have contributed equally to this work and share first authorship