- 1Department of Civil and Environmental Engineering, Northwestern University, Evanston, IL, United States
- 2Delta Institute, Chicago, IL, United States
- 3The Nature Conservancy, Chicago, IL, United States
- 4Forest Preserves of Cook County, River Forest, IL, United States
- 5Metropolitan Planning Council, Chicago, IL, United States
- 6OAI, Inc., Chicago, IL, United States
- 7Waterwell, LLC, Chicago, IL, United States
- 8Department of Chemical and Biological Engineering, Northwestern University, Evanston, IL, United States
Climate change is increasing the frequency and severity of extreme precipitation events, requiring new ways of managing stormwater, particularly in urban areas. Nature-based solutions (NBS) have become increasingly popular to provide distributed stormwater storage while supporting urban biodiversity and access to nature. However, long-term monitoring of the hydrological performance of NBS is limited. To date most literature has focused on monitoring methodologies for specific sites and types of NBS, use of remote sensing and modeling for large-scale assessments, or measuring benefits of NBS for urban heat mitigation. More comprehensive and consistent measurement strategies are needed to understand the effects of distributed NBS on urban hydrology at the regional scale, and improve the design, maintenance, and adoption for community-centered stormwater management. To address these gaps, we review available literature on measurement methods, summarize these methods and provide specific recommendations for instrumentation and in situ monitoring of common types and scales of urban NBS. Based on our findings on performance monitoring for individual NBS sites, we extend recommendations for consistent hydrological assessment of distributed NBS at regional scale and the efficacy of NBS in reducing community flooding impacts. These recommendations are particularly applicable for municipalities, researchers and community-based organizations who are now leading the planning and implementation of community-centered NBS systems in many areas.
1 Introduction
By 2050, 68% of the world’s population is expected to live in urban areas, resulting in an additional 2.5 billion urban residents over the next 25 years (United Nations, Department of Economic and Social Affairs, Population Division, 2019). As our cities grow, so does the number of roads, buildings and parking lots, increasing the impervious cover of the land, which in many cities is already greater than 40% (Tabari, 2020). With more impervious surface, stormwater runs more quickly off roads and roofs and into the drainage system, which can become easily overwhelmed, resulting in water quality degradation and flooding. As extreme precipitation events become more frequent due to climate change (Nowak and Greenfield, 2012), urban flooding is expected to increase, especially in under-resourced communities, making stormwater management even more critical.
Nature-based solutions (NBS) have become an increasingly popular means of managing stormwater runoff. NBS are defined by the International Union for Conservation of Nature as measures that “protect, sustainably manage, and restore natural and modified ecosystems” to address environmental challenges and benefit both people and nature (Nature-Based Solutions IUCN, n.d.). A number of different terms are used to describe systems that integrate natural and human-built infrastructure for stormwater management (Environmental Policy Innovation Center, 2024). These include green infrastructure (US EPA, O, 2015b), natural infrastructure (Institute for Resilient Infrastructure Systems, n.d.), natural flood management (University of Reading, n.d.), low impact development (LID) (US EPA, O, 2015a), green stormwater infrastructure (GSI) (Clean Water Education Partnership, 2023), stormwater best management practices (BMPs) (Southwestern Pennsylvania Commission Water Resource Center, n.d.), and sustainable urban drainage systems (SuDS) (British Geological Survey, n.d.). Here we use the term nature-based solutions as an umbrella concept encompassing all approaches that use open land and natural ecosystems to address urban stormwater challenges. NBS capture and absorb stormwater before it enters the drainage system, reducing the burden on gray stormwater infrastructure during heavy rain events and replenishing local groundwater supplies. NBS also provide many other key ecosystem services, including improving air quality, reducing the urban heat island effect, and increasing biodiversity (Chang et al., 2017). We focus specifically on community-centered NBS, which we define as NBS projects led by or in partnership with local communities, non-profit organizations, or community organizations to address local challenges.
Despite its increasingly popular use for stormwater management, limited guidance is available to establish standard methods for monitoring different types of NBS and documenting their effects on local hydrology and stormwater capture. Existing literature has focused mainly on instrumentation and monitoring of a single site with one specific type of NBS and does not consider designs for instrumentation that can be applied across a wide variety of NBS across different scales and regions (Catalano de Sousa et al., 2016; Woznicki et al., 2018; Feldman et al., 2019; Fuentes et al., 2021; Meixner et al., 2021; Xie et al., 2021). Regional assessments to date have used satellite-based data products or other remote sensing methods, and do not include methods for in situ monitoring of NBS (Stewart et al., 2017; Lim and Welty, 2018; Taramelli et al., 2019; Furberg et al., 2020). Prior reviews of the methodologies and frameworks for monitoring NBS have largely focused on quantifying the impact on urban heat mitigation or benefits to urban biodiversity, and not the hydrological benefits (Chen et al., 2016; Bartesaghi Koc et al., 2018; Saaroni et al., 2018).
Measuring the hydrological dynamics of NBS and resulting benefits for flood reduction is critical to improving NBS design, informing maintenance strategies, and encouraging adoption of NBS by both communities and public officials (Ahern, 2007; Geberemariam, 2017; Gordon et al., 2018). However, monitoring of NBS is inconsistent, which makes it difficult to compare effectiveness of alternate NBS strategies and generalize the benefits of NBS to municipal or regional scales (Kerkez et al., 2016; Monteiro et al., 2020; Sun et al., 2020). Consistent methods of measuring the hydrological benefits of NBS are necessary to establish standard metrics and benchmarks for performance (Geberemariam, 2017). Specific guidance for monitoring of community-centered NBS performance is also needed because NBS measurements have been predominantly performed by universities, consultants, or government agencies, but many community organizations and non-profits are now leading the implementation and monitoring of community-scale NBS. To fill these gaps, we review the literature on hydrological monitoring of NBS and derive general recommendations for (1) instrumenting and monitoring different types and scales of community-centered NBS, and (2) use of the resulting data to track long-term performance, inform maintenance strategies, and design regional NBS solutions for climate resilience.
2 Objectives and methods for measuring the performance of nature-based solutions
Soil moisture and groundwater level measurements are important for many types of NBS and are frequently used in monitoring civic infrastructure projects (Fassman-Beck et al., 2013; Kazemi, 2014; Grey et al., 2018; Feldman et al., 2019; Alizadehtazi and Montalto, 2020; Mason et al., 2021). Soil moisture is normally measured using sensors that detect the volumetric water content of the surrounding soil. Soil moisture sensors are frequently used by researchers to assess the performance of green roofs (Versini et al., 2016; Ouellet et al., 2021), rain gardens (Potter, 2023), and urban natural areas (Phillips et al., 2019). While soil water content is normally a small fraction of the total stormwater storage provided by NBS, soil moisture measurements can be used to determine the impact of antecedent soil moisture on the response to rain events and monitor in situ conditions for plant growth (Phillips et al., 2019; Tu et al., 2020; Xie et al., 2021). For broader areal measurements of soil moisture, geophysical techniques such as Electrical Resistivity Tomography (ERT) are often appropriate (Brunet et al., 2010; de Jong et al., 2020). ERT measures electrical resistivity between multiple electrodes spaced across a site (de Jong et al., 2020). As soil conductivity increases with water content, the soil moisture is determined from resistivity measurements using an empirical equation (Brunet et al., 2010). This method is more expensive and labor intensive than point soil sensors, so it is normally used to obtain spatial data across a site on an infrequent basis (e.g., annually or seasonally).
Due to the importance of site hydrogeological conditions, measurements of infiltration rates and soil characteristics provide useful data for both designing NBS and assessing their performance (Schlea et al., 2014; Lewellyn et al., 2016). Infiltration tests (American Society for Testing and Materials, 2020) and soil characterization, such as grain-size distributions, porosity, and soil type, are frequently conducted during site investigation as part of the NBS design process. Time-series measurements of water levels across a site are used to assess (eco)hydrological dynamics. Groundwater levels are frequently measured within a variety of NBS, including rain gardens (Schlea et al., 2014; Mason et al., 2021; Potter, 2023), infiltration trenches (Lewellyn et al., 2016), bioretention cells (Winston et al., 2016), and natural areas (Hernandez Gonzalez et al., 2019). Piezometers are used with a pressure transducer water level sensor (Figure 1), together with soil porosity, to determine the volume of water stored in the subsurface (Potter, 2023). When combined with hydrological modelling, in situ data can potentially be used to understand the hydrologic response of NBS to stormwater and the resulting storage time distributions, though both measurements and modelling hydrologic dynamics between the urban environment and NBS remain challenging (Sharma et al., 2020; Qian et al., 2022). Commonly used models for NBS include groundwater modelling with relatively simple representations of surface water (Sharma et al., 2020; Li et al., 2024). This includes ecohydrological models that use land use, soil type, vegetation, and weather data to represent landscape processes, often in a long-term climate context (Castelli et al., 2017; University of Michigan Graham Sustainability Institute, n.d.); and urban hydrology and hydraulic models that estimate stormwater infiltration, runoff, and flow in both natural and engineered parts of the urban environment (Nanía et al., 2015; Korgaonkar et al., 2018; Mignot and Dewals, 2022).
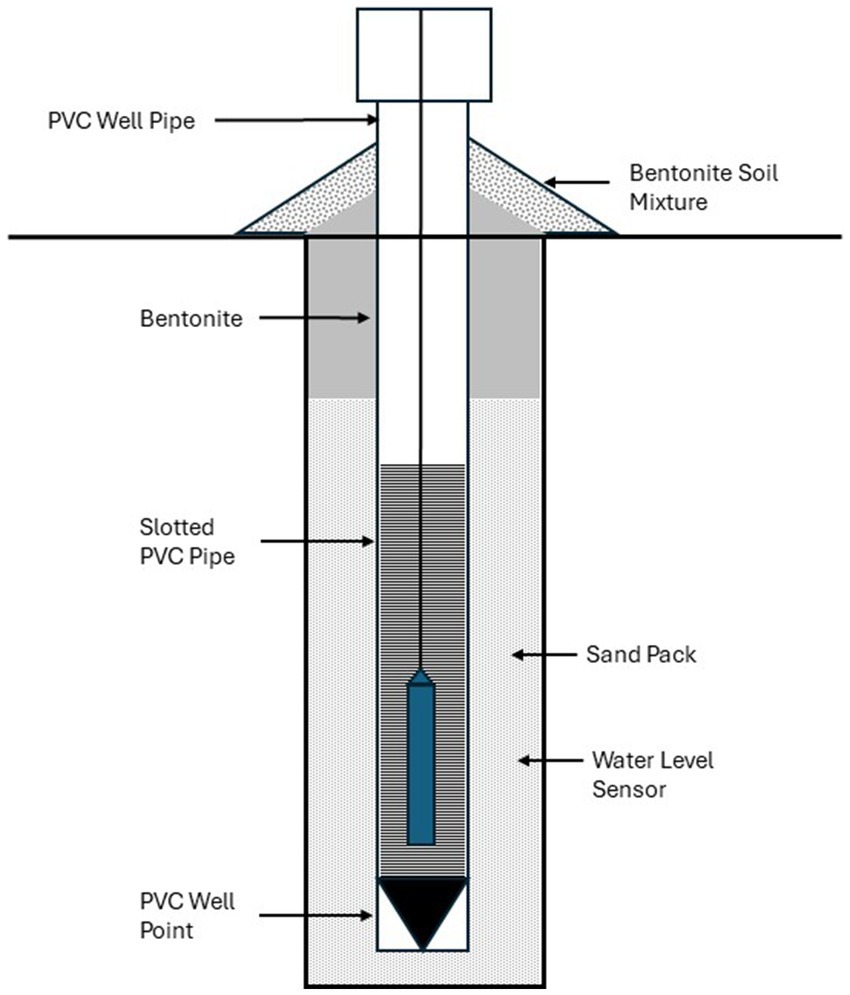
Figure 1. Cross section of typical piezometer with groundwater level sensor. Based on drawings provided by Hey and Associates, Inc (2021).
In engineered NBS designed with a discharge or overflow pipe, outflow is frequently measured using a flow meter (Carson et al., 2013; Versini et al., 2016; Ouellet et al., 2021) or a weir and pressure-transducer water level sensor (Winston et al., 2016). Downstream flow within the stormwater drainage system, such as storm sewers, ditches, and receiving streams and rivers, can also be monitored to assess the efficacy of NBS in reducing urban stormwater impacts (Jarden et al., 2016; Boening-Ulman et al., 2022). However, downstream flow is not often measured in NBS performance evaluation as it requires making measurements over much larger scales and within larger-scale controlled infrastructure that is subject to high forces during flood flows. Consequently, such work is primarily done by stormwater agencies and specialized professional contractors, and not directly by NBS researchers or by communities implementing local NBS solutions.
Precipitation measurements are needed for comparison against soil moisture and water level data to complete water budget calculations and evaluate NBS stormwater performance as a function of storm intensity and antecedent in situ conditions. Precipitation data collected and published from governmental monitoring stations, such as rain gage data collected by the National Weather Service, are often used to estimate water inputs to NBS sites. However, due to the high degree of heterogeneity in precipitation within a city or region, local precipitation is frequently measured at the NBS installation being monitored (Cristiano et al., 2017; Zhuang et al., 2020). Commonly used methods to measure precipitation at the site scale include classic tipping-bucket rain gages and newer optical rain sensors. Tipping bucket rain gages are available heated, which capture precipitation from rain and snow, and unheated, which only capture rainfall. Optical rain sensors are non-contact and detect and measure rainfall via drop size and frequency using infrared light beams (Bartholomew, 2016). To provide a more complete description of local weather and climate, precipitation measurements can be combined with other meteorological measurements, including temperature, relative humidity, wind speed and direction, and solar radiation. These sensors are often used in newer suites of wireless sensors, such as the Wild Sage environmental sensing system (Catlett et al., 2022).
Current conceptual models for NBS hydrology are primarily based on conventional urban stormwater infrastructure design, and monitoring focuses on traditional stormwater metrics such retention, detention, and infiltration (Beauchamp and Adamowski, 2013; Prudencio and Null, 2018; Li et al., 2019; Zhang et al., 2021). However, detention and retention concepts that are based on impermeable constructed infrastructure do not translate well to NBS involving extensive surface-groundwater interactions and long-term ecosystem dynamics. Because there is no consensus on important attributes of NBS hydrologic function, the metrics reported in NBS literature vary quite considerably. In 26 studies evaluating the performance of different types of NBS, 17 different metrics were used. The most common metrics were reduction in peak flow (Khan et al., 2012; Fassman-Beck et al., 2013; McLaughlin et al., 2014; Schlea et al., 2014; Jarden et al., 2016; Winston et al., 2016; Batalini de Macedo et al., 2019); percent of water retained, captured, or infiltrated; (Carson et al., 2013; Fassman-Beck et al., 2013; McLaughlin et al., 2014; Paus et al., 2015; Lewellyn et al., 2016; Feldman et al., 2019; Cook et al., 2021) and infiltration rate (Kazemi, 2014; Lewellyn et al., 2016; Elliott et al., 2018; Mason et al., 2021; Meixner et al., 2021). Other commonly used metrics include storage volume (Stewart et al., 2017; Batalini de Macedo et al., 2019; Xie et al., 2021), runoff depth (Fassman-Beck et al., 2013; Boening-Ulman et al., 2022), and exfiltration rate (Kazemi, 2014; Grey et al., 2018). This variability in reported metrics makes it difficult to compare the hydrology and benefits of different types and scales of NBS. Existing metrics also fail to capture to the full extent of hydrologic processes in NBS, such as large-scale surface-groundwater interactions and long-term water storage. Beyond individual sites, the conceptual focus on retention, detention and infiltration makes it challenging to aggregate hydrologic dynamics across multiple types and locations of NBS and prevents consistent assessments of regional outcomes for urban hydrology and flood reduction. More consistent instrumentation designs are needed to complete full water budgets for NBS, and new metrics are needed to evaluate the performance of NBS systems as regional stormwater solutions.
3 Recommendations for monitoring of nature-based solutions
3.1 Proposed conceptual framework
To develop systematic recommendations for evaluating hydrologic dynamics in NBS, we first develop a conceptual model classifying NBS based on three major factors: the type or form of NBS, the scale, and the degree of naturalness. These three characteristics influence monitoring needs, including sensor type(s), the number of sensors, and their placement within the NBS to accurately determine both site water balances and effectiveness as stormwater infrastructure (Figure 2). The type of NBS strongly influences the type(s) of sensors required. For example, a constructed surface-water wetland will require surface water level sensors but generally not soil moisture sensors, whereas soil moisture sensors will be critical in measuring water storage in a green roof (Versini et al., 2016; Ouellet et al., 2021). The scale of the NBS impacts the number of sensors required. A larger natural green space will require more sensors to assess the benefits than a small park or community garden. The degree of naturalness impacts both the type and configuration of sensors. We define the degree of naturalness as the extent of natural soil and ecosystems within the site relative to the extent of constructed hard infrastructure. For example, permeable pavement is an entirely engineered system constructed from human-made (artificial) materials, whereas a native parkland or forest is considered entirely natural. NBS with intermediate degrees of naturalness include a mixture of natural and engineered elements, for example a green roof, bioretention basin, or restored wetland with engineering control of water levels. NBS that are engineered may have an outflow pipe that will be useful to instrument and may require additional in situ sensors.
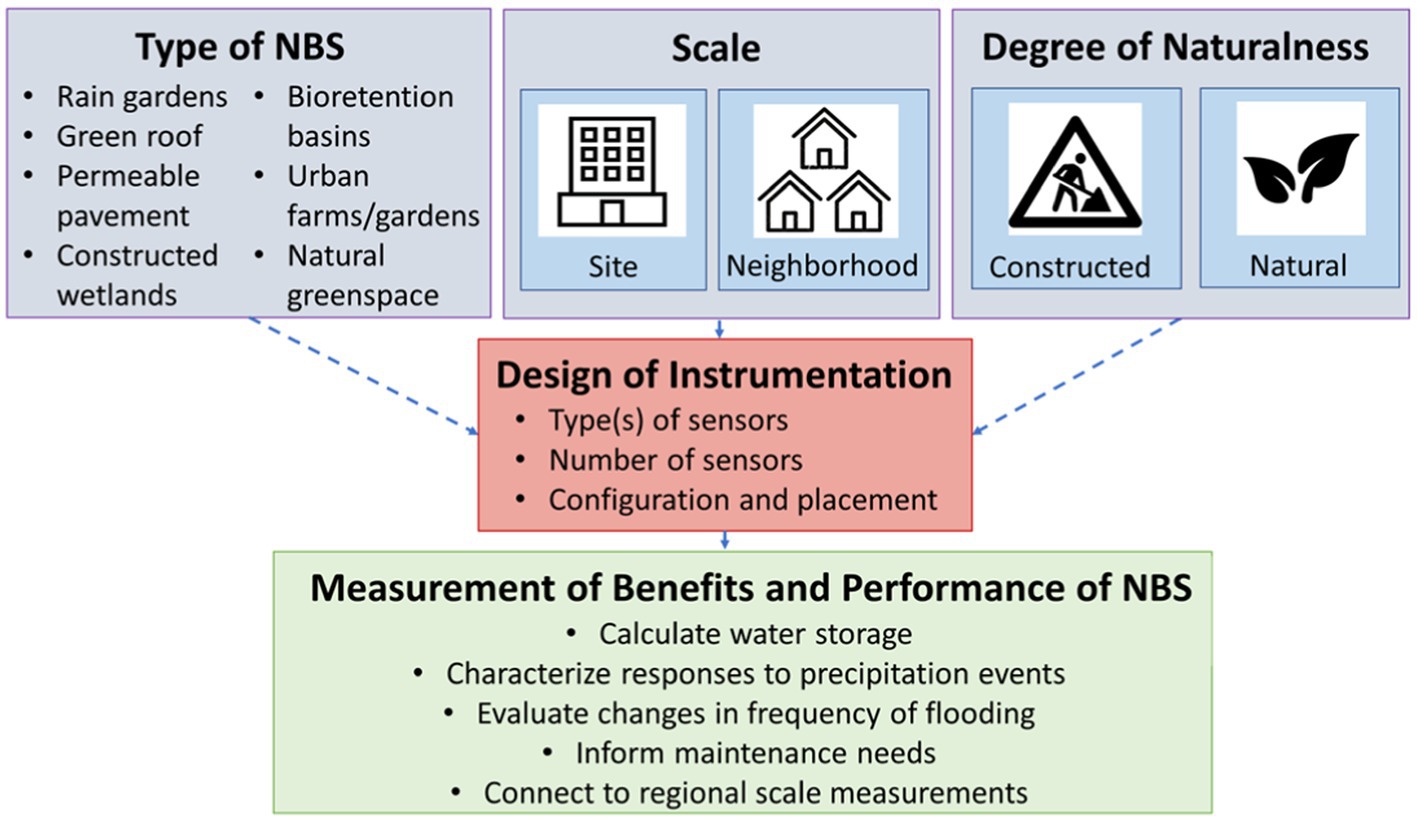
Figure 2. Conceptual framework of the factors influencing instrumentation design for monitoring community-centered NBS performance, including NBS type, scale, and degree of naturalness.
3.2 Instrumentation designs for six common classes of nature-based solutions
Based on the key characteristics of form, scale, and degree of naturalness identified in the conceptual model (Figure 2), we selected six common types of NBS for detailed consideration: bioretention basins, rain gardens, green streetscapes, green roofs, nature preserves, and community green spaces. These selected NBS types span commonly used configurations ranging from small features located within individual residential properties to neighborhood-scale land restoration efforts, large municipal projects, and natural areas. Bioretention basins provide an example of semi-natural NBS with some engineered components that provide both surface and subsurface storage and can range in scale from approximately 5–100 m2 in area, often even larger depending on the contributing catchment area. Rain gardens are similar in design to bioretention basins but tend to include deep-rooted plants and are often at a smaller scale, as they are frequently used in individual-household residential settings. Green streetscapes include several different types of small scale semi-natural NBS that can be implemented individually or, more typically, as part of a larger infrastructure project, e.g., box tree filters along a long stretch of road. Streetscapes include natural vegetation and engineered elements, such as outflow pipes connected to the municipal stormwater system, or may be combined with other infrastructure, such as permeable pavement. Green roofs represent an intermediate scale engineered NBS that provide predominantly subsurface storage and plant transpiration in an engineered soil layer. Nature preserves, including designated natural areas within urban parks, generally have native vegetation and hydrology, and we therefore consider them entirely natural even though the land may be intentionally modified or restored. These areas range in size from 1,500 m2 to over 450,000 m2. Lastly, community greenspaces include a variety of different forms of NBS that are designed to provide amenities for local communities, including community gardens, local parks, and other recreational areas. Many community greenspaces are embedded within highly urban areas and are designed for multiple purposes, including stormwater storage.
Recommended sensor configurations for each class of NBS are shown in Figures 3–8, and recommended types, numbers, and costs of sensors are provided in Table 1. The number of sensors needed within an NBS system is determined mainly based on the size and scale of the NBS being monitored, as well as site heterogeneity and the degree of data resolution needed for analysis of site hydrology, which is determined based on the monitoring objectives.
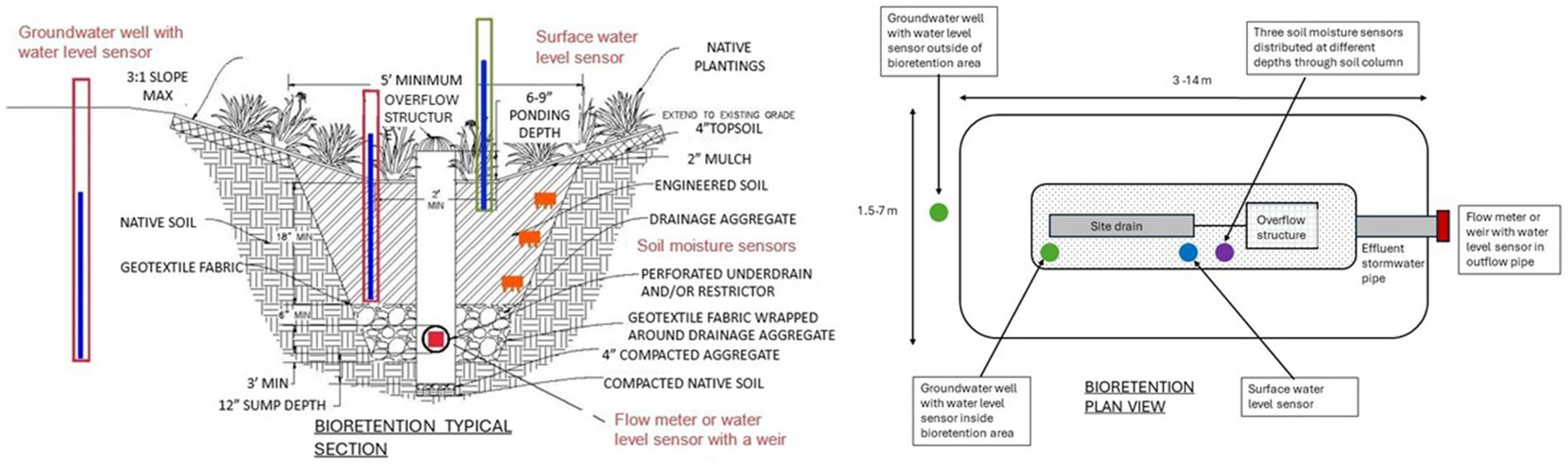
Figure 3. Instrumentation designs for bioswale including soil moisture sensors, piezometers with water level sensors, a surface water level sensor and a flow meter. Bioswale section drawing provided by the Delta Institute (Eskin et al., 2021). Plan view based on drawings by American Wick Drain (n.d.).
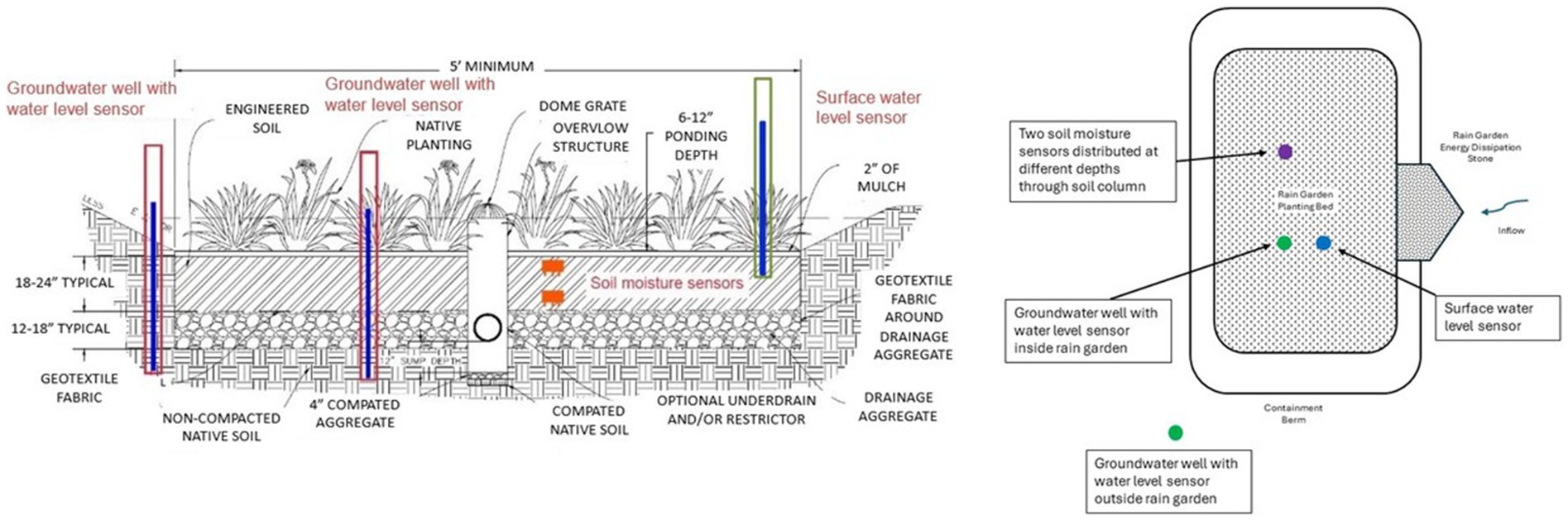
Figure 4. Instrumentation designs for rain garden including soil moisture sensors, a surface water level sensor, and piezometers with water level sensors. Rain garden section drawing provided by the Delta Institute (Eskin et al., 2021). Plan view based on drawings by the Milwaukee Metropolitan Sewerage District and Strand Associates, Inc (Kaminski and Bzdusek, 2017).
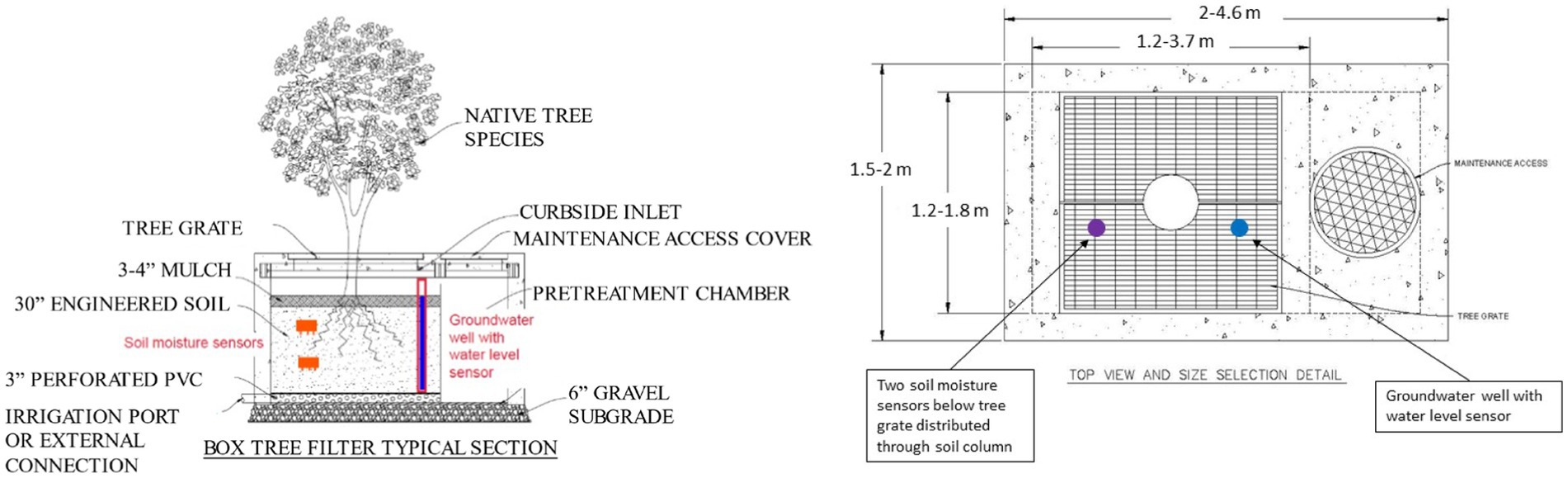
Figure 5. Instrumentation designs for box tree filter in a green streetscape including soil moisture sensors and a piezometer with a water level sensor. Box tree filter section and plan drawings provided by the Delta Institute (Eskin et al., 2021).
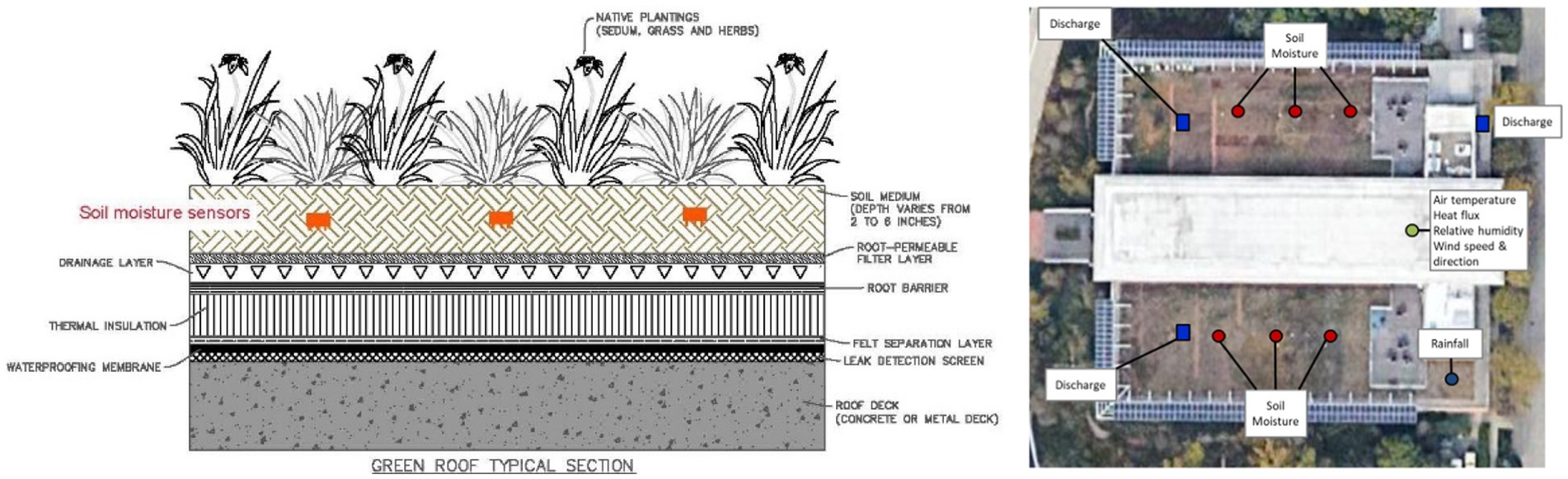
Figure 6. Instrumentation designs for green roof including soil moisture sensors. Instrumentation also includes a flow meter on the drainage outflow pipe. Green roof section drawing provided by the Delta Institute (Eskin et al., 2021).
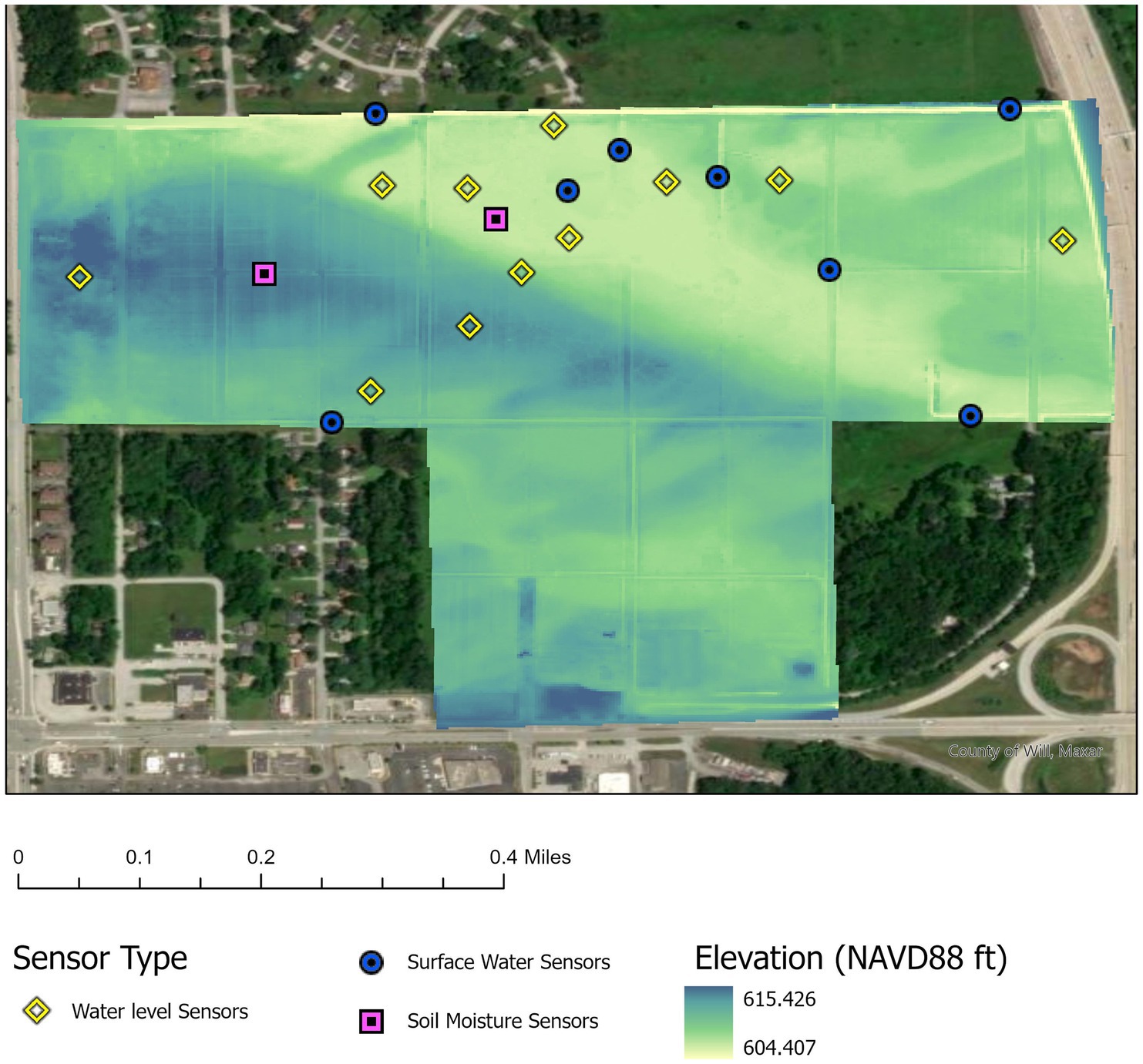
Figure 7. Sensor layout for large natural green space (Gensburg Markham Prairie in Markham, Illinois) including surface water level sensors, piezometers with groundwater level sensors and soil moisture sensors overlayed on a digital elevation model (DEM) derived from 2009 aerial LiDAR Survey (Cook County Board of Commissioners, 2010).
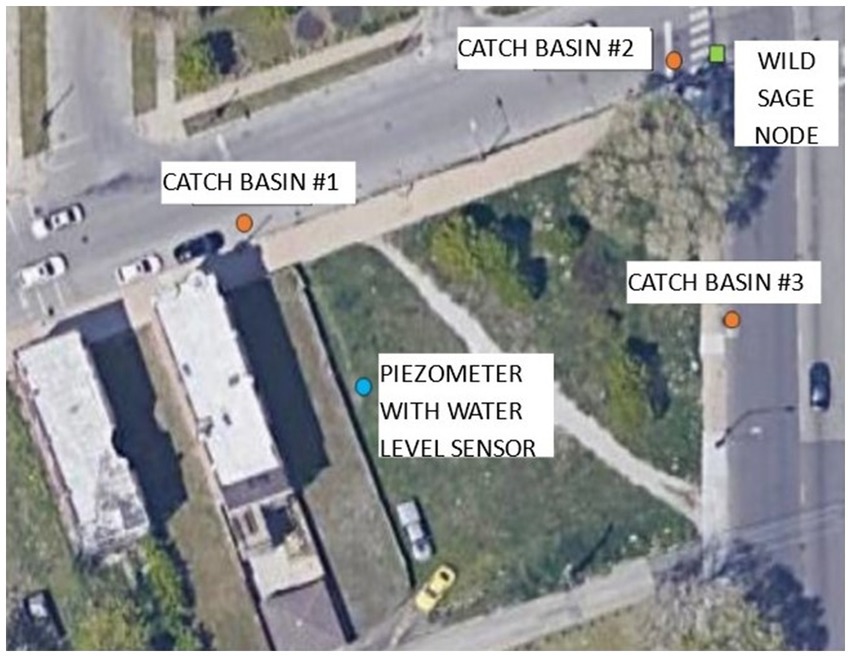
Figure 8. Sensor layout for community green space including groundwater level sensors and catch basin water level sensors. Figure shows aerial view and sensor locations at the Garfield Park Eco-Orchard site in Chicago, IL.
Bioretention basins (Figure 3), and rain gardens (Figure 4) require piezometers with water level sensors, surface water level sensors, and soil moisture sensors to estimate the water storage volume (Khan et al., 2012; Schlea et al., 2014; Paus et al., 2015; Winston et al., 2016; Stewart et al., 2017; Batalini de Macedo et al., 2019; Feldman et al., 2019; Cook et al., 2021; Meixner et al., 2021). The spacing between soil moisture sensors depends on the depth of the system, but in general these should be vertically distributed through the soil column (Stewart et al., 2017; Meixner et al., 2021). In some systems, connectivity between surface water and groundwater has been engineered so that surface and groundwater storage can be measured with one piezometer extending above the ground surface. However, in many cases the presence of an unsaturated zone or buried construction debris limits infiltration and causes a disconnect between groundwater and surface water (Shuster et al., 2014), which necessitates both a piezometer and surface water level sensor to accurately measure total storage volume (Batalini de Macedo et al., 2019). In bioretention basins or rain gardens with a defined inlet, inflow is frequently measured with a weir and water level sensor (Batalini de Macedo et al., 2019; Feldman et al., 2019). For those without a clear inlet point, inflow can be estimated using an overland flow collector (Moruza et al., 2021). In bioretention basins with an outflow or overflow pipe, a flow meter or weir and water level sensor can also be installed to measure outflow when there is interest in determining discharge to the drainage system. In low-lying landscapes, there may be concern that the effectiveness of NBS is limited by a shallow regional groundwater table (Zhang and Chui, 2019). Particularly in flat alluvial landscapes, infiltration from bioretention systems can cause the groundwater table outside the system to rise, decreasing available storage (Thomas and Vogel, 2012; Nemirovsky et al., 2015). Where this is a concern, we recommend installing one or more additional piezometers outside of bioretention basins and rain gardens to monitor the groundwater table. Both pre- and post-construction groundwater monitoring is recommended to understand the limitations imposed by the regional water table and the consequences of NBS on groundwater levels.
In a green streetscape (Figure 5), we recommend installing 1–3 soil moisture sensors distributed vertically through the soil column to measure subsurface stormwater storage within soil pore space (Grey et al., 2018). Where possible, we also recommend installing additional groundwater level sensors to measure the total subsurface water storage (Grey et al., 2018) and/or surface water flow sensors to measure the effects of tree plantings on stormwater discharge. In Table 1, sensor recommendations and cost estimates are provided for both streetscapes at the individual scale (e.g., one box tree filter) and at the city block scale (e.g., ten box tree filters). At the block scale not every box tree filter needs to be instrumented, and our cost estimates are based on instrumenting 50% of box tree filters.
Green roofs (Figure 6) are designed with a shallow substrate and provide storage in soil pore space, in addition to water uptake through plants. Soil moisture sensors are the main instrumentation required (Versini et al., 2016; Ouellet et al., 2021). In addition, many green roofs have outflow pipes that discharge excess water. Non-contact flow meters (i.e., ones that do not block stormwater drainage) or weirs with water level sensors to measure outflow provide useful information for conducting water balances and calculating water storage (Carson et al., 2013; Fassman-Beck et al., 2013; Ouellet et al., 2021).
In nature preserves (Figure 7), a combination of soil moisture sensors, piezometers, and water level sensors are recommended for determining site water balances and estimating hydrologic storage. Since surface-groundwater interactions are important in these systems, surface water level sensors should be used in concert with piezometers to capture both surface and groundwater storage (Gonzalez et al., 2023). Surface water flow measurements will often also be useful to measure discharge to urban waterways (e.g., streams, rivers, engineered drainage ditches) and downstream areas of the watershed. Placement of sensors within a large natural area is much more complex than in small-scale NBS, like a rain garden. Pre-installation modelling of site topography and flow paths is useful to determine hydrological connectivity to the surrounding urban hydrologic connections, such as natural streams and engineered stormwater conveyance ditches, and low points within the site, where pooling of stormwater is anticipated. Local LiDAR data, which is frequently available for public use, and digital elevation models (DEMs) are useful to understand site drainage and inform the optimal placement of sensors to accurately monitor site water balance.
For community greenspaces embedded within highly urbanized areas (Figure 8), on-site piezometers are recommended for monitoring site water balances and stormwater storage (Xie et al., 2021). In addition, flow meters or weirs and water level sensors are useful to document the reduction of stormwater inflow into the urban drainage system (Jarden et al., 2016). In addition, the scale of community greenspaces and NBS often requires coordination between community-based monitoring and governmental infrastructure monitoring. This is an opportunity for community science measurements in collaboration between local residents and government agencies. This is particularly useful for long-term adaptive performance monitoring to inform maintenance. In addition, flow meters or weirs and water level sensors in surrounding drainage infrastructure, like stormwater catch basins, provide data on interactions between the site and drainage infrastructure and can be used to determine the reduction of inflow into the drainage system due to the storage provided by the community greenspace.
In addition to recommendations on the type and number of sensors needed to obtain site water balances, in Table 1 we provide cost estimates for instrumentation of community-centered NBS projects based on the desired data quality. Rain gauges should be located near NBS installations and can be shared by multiple NBS installations in close proximity to one another. Tipping bucket rain gauges costs range from $700 to $900. The lowest cost estimates in Table 1 are for a minimal monitoring strategy with the fewest recommended sensors at the lowest price per sensor, and the highest cost estimates are based on achieving the greatest spatial resolution (largest number of sensors) and data quality (highest price per sensor). The cost estimates are not comprehensive, but rather indicative for typical community-centered NBS projects conducted by non-profit organizations or local governmental agencies in the United States. Larger infrastructure projects and/or more challenging settings for instrument deployment are likely to require substantially larger investments.1
Data provided by the recommended instrumentation designs and monitoring methods is sufficient to conduct a water balance on NBS systems, which provides critical information on both ecohydrologic dynamics in greenspaces and the volume and timing of stormwater storage beyond retention and detention. This comprehensive monitoring approach allows for upscaling from individual site measurements to assess the impact of community-centered NBS on flooding and stormwater management at the catchment and regional scales. This approach is shown in the conceptual framework in Figure 9. Using water budget analysis, researchers can assess how NBS respond to different conditions such as climate, rainfall intensity or shallow groundwater tables (Zhang and Chui, 2019; Hung et al., 2020; Johnson et al., 2022). This can inform adaptive planning and selecting the most appropriate type of NBS for different settings (Kato and Ahern, 2008; Tang et al., 2020). The complexity of water budgets will vary based on the type of green infrastructure. The simplest example is a green roof, where the water input is limited to direct rainfall onto the roof, the hydrologic response time of the system is very short, and outflow can be directly measured at a discharge pipe. For this well-defined case, water mass balance can easily be obtained for a storm as ΔS=P-Q, where S is volume of stormwater stored, P is the total precipitation volume, and Q is the total outflow obtained by integrating the measured discharge hydrograph over time (Versini et al., 2016). The complexity increases when calculating the water budget for a rain garden or bioswale, in which case there is stormwater input from both on-site rainfall and runoff from an off-site contributing area. In this case, we can directly measure the surface and groundwater storage and estimate short-term water balance based on the change in water level measured by surface and groundwater level sensors. In addition, flow meters can provide an estimate of outflow from the system (Fletcher et al., 2021). A longer-term water balance incorporating evapotranspiration and slow groundwater recharge can be estimated by incorporating modeling. However, because these systems respond quickly to precipitation a short-term water balance can provide an estimate of water storage based on direct measurements through the recommended suite of sensors and instrumentation. The complexity further increases in an embedded greenspace. If the system is relatively small and directly connected to a stormwater system, the same methods for rain gardens and bioswales can be used to measure storage and outflow. However, in systems with no clearly defined or engineered inlet or outlet, the total contributing area may vary between storms, making the inflow and outflow very challenging to measure. In these cases, some estimates of short-term storage can be obtained from direct measurements of changes in groundwater and surface water levels from sensors. However, on a larger time scale, modeling is often needed to better understand the longer-term dynamics. Many of the methods for this type of analysis are still under development, but the data provided by groundwater and surface water level measurements can provide a basis for estimating an overall water budget in these systems.
To determine the stormwater storage and other benefits provided by community-centered NBS, baseline data must also be collected for a significant period of time prior to NBS construction to provide points of comparison for a variety of storms of differing intensity, duration, and frequency. Pre-implementation monitoring is useful for both constructed NBS and related activities such as restoration of natural areas. Pre-implementation data collection can range from 3 months before construction begins (Jarden et al., 2016) to more than 2 years (Boening-Ulman et al., 2022). The recommended duration of pre-implementation monitoring will vary based on local climate. Longer pre-implementation periods increase the diversity of storm conditions for comparison with post-installation performance. Our analysis of precipitation data in the Chicago region found that 1 year of pre-implementation data is needed to capture a variety of storm events and obtain a reasonable estimate of the precipitation distribution and site hydrological response (Griffin et al., 2020; Gonzalez et al., 2023). At a minimum, we recommend at least 3 months of pre-implementation data collected during the primary season of concern for high precipitation and flooding. Since sensors used for collecting baseline data can then be deployed within the NBS, no additional sensor costs are associated with collecting baseline data.
4 Discussion
4.1 Benefits and barriers of monitoring nature-based solutions performance
Monitoring NBS performance provides the information necessary to quantify the value of community-centered NBS for stormwater management and flood reduction, improve design, advocate for increased adoption of NBS, and inform the timing and type of maintenance needed (Ahern, 2007; Geberemariam, 2017; Gordon et al., 2018). Comparisons between pre-implementation and post-implementation monitoring data are important to quantify the additional stormwater storage provided by NBS and the resulting reduction in burden placed on the surface water drainage system (U.S. Geological Survey, 2023). These results can then be directly related to the benefits of NBS for reducing flooding in surrounding communities, and the corresponding economic value of these services. Quantitative data on the hydrological performance of NBS can also be used to advocate for additional investment in community-centered NBS from local water management authorities and municipalities. Tangible data are useful to local leaders in communicating the functionality and benefits of NBS to community members, who may not initially support or recognize the benefits of this type of infrastructure (Venkataramanan et al., 2020). In addition, when community members have access to and understand the data and can see the benefits of community-centered NBS, they can become advocates for these types of projects and provide incentives for their local representatives to provide additional support (Ando and Freitas, 2011; Lamond and Everett, 2019).
Long-term monitoring is critical to provide data on the long-term performance of NBS years after implementation and to inform maintenance needs; however, this often presents many challenges in terms of budget and staff availability (Wadzuk et al., 2021). Determining the minimum length of monitoring necessary to achieve project objectives, such as informing monitoring or quantifying the benefits of a community-centered NBS installation, should be discussed in the planning phase to allocate necessary staff time and financial resources. The most challenging aspects of long-term monitoring are deciding who should be responsible for long-term data collection and analysis, allocating staff time for this purpose, and securing budget for replacement of sensor hardware and parts. Municipalities are often understaffed, particularly in disadvantaged communities that are often more susceptible to flooding (Weller, 2023). Nonprofits and community organizations often experience the same challenges in terms of staffing. Therefore, allocating staff time and resources to long-term monitoring is often unfeasible unless resources are allocated as part of the primary NBS construction effort.
These challenges can be addressed through partnerships with local community members and by combining long-term monitoring efforts and maintenance projects. Monitoring data inform the type of maintenance required, which will in turn impact the long-term performance of the NBS (Wadzuk et al., 2021). Citizen science programs provide opportunities for community engagement and education while supporting long-term data collection for both research and operational purposes. Citizen science partnerships involve data collection, performance monitoring, and reporting by local volunteers, who have an incentive to see these sites perform well in the long term. NBS monitoring also presents the opportunity for workforce development (National Academies of Sciences, Engineering, and Medicine, 2018) and creating jobs for monitoring and maintenance of NBS in flood-prone communities. Beyond their value for both hydrologic research and operational performance monitoring, community science programs also provide value for public education and capacity building for maintenance and monitoring in environmental justice communities. These types of partnerships are particularly important for communities to improve climate resilience.
4.2 Data analysis
Data provided by the instrumentation of community-centered NBS will be used differently based on the goals and objectives of the institution or organization leading the monitoring. Municipalities and local non-profits or community organizations may be more interested in using data to inform the maintenance of their systems, whereas researchers may want to better understand how these systems function and inform the design of more efficient NBS.
Monitoring data are useful to inform ongoing operational maintenance, specifically when maintenance is needed and what type of maintenance is necessary. For example, Kazemi, 2014 used water level data to calculate infiltration rates and identify when clogging in a permeable pavement system had occurred. Similarly, the City of Lancaster, Pennsylvania used infiltration rates in permeable pavement to determine when vacuuming of these systems was needed and infiltration rates in bioswales to determine if additional soil testing or amendments were necessary (City of Lancaster, 2019). The early identification of maintenance needs can reduce costs over time, improve long-term performance of NBS, and help inform decision making for allocation of maintenance resources (Wadzuk et al., 2021).
Municipalities may also use monitoring data to track progress toward goals or toward meeting regulatory requirements. For example, the City of Philadelphia, Pennsylvania, uses monitoring data from NBS to assess how their program is reducing combined sewer overflows (CSOs) in the city and inform their long-term control plan for preventing CSOs (Philadelphia Water Department, 2009).
Lastly, monitoring data from multiple locations can be used to expand the assessment of NBS beyond the site scale to the catchment or regional scale. Scaling the analysis of NBS performance is critical to better understand the cumulative impacts on downstream hydrology, inform catchment management, and determine what factors impact changes in performance upon scaling (Golden and Hoghooghi, 2018). Monitoring can also inform community-centered NBS planning at the regional scale and inform decisions on the spatial configurations and network locations of NBS (Weber and Wolf, 2000; Golden and Hoghooghi, 2018; Shi and Qin, 2018; Goodspeed et al., 2022).
4.3 Community-centered impact assessment
Beyond monitoring the stormwater storage provided by community-centered NBS, there is a need for assessment of the actual impacts on community benefits, such as flood reduction and the improved experiences and perceptions of community members. Additional measurement methods are needed for this purpose beyond the instrumentation designs detailed above. One common means of measuring community impact and benefits is through surveys (Baptiste et al., 2015; Kim and Miller, 2019; Miller and Montalto, 2019; Anderson et al., 2021). However, low-income communities and communities of color may be frequently asked to take surveys on similar topics. Therefore, surveys should be prepared with input from community members and should not be unduly long or inaccessible to reduce the burden on community members.
Practitioners and researchers measuring impact should work with stakeholders to identify and prioritize outcome measures that address local concerns. In addition, an assessment of the risks of this type of survey data collection and analysis, including breach of confidentiality or data leaks (University of Oregon, Office of the Vice President for and Research and Innovation, n.d.), should be conducted at the beginning of the project in consultation with the community and relevant Institutional Review Boards, and all risks associated should be adequately communicated to the community beforehand. In cases where community members or community organizations are involved directly in impact assessments, whether recording visual observations or participating in surveys, the effort involved should be recognized and community members should be compensated fairly for their time.
Data management and privacy are concerns among community-centered NBS monitoring projects on both private and public property. Data management should be discussed as part of community engagement early in the planning process. At a minimum, data management, usage and privacy should be discussed with the community before sensors are installed and monitoring begins. Community members may have concerns about the use and protection of data collected near their homes, particularly imagery that may show individuals and the occurrence of flooding, which could impact local property values (Gourevitch et al., 2023). Efforts should be made to balance the protection and security of data with the availability of data for community and professional use. Practitioners engaged in monitoring should make every effort to provide a space for stakeholders to determine outcomes of data collection, including making data collected in communities available to community members in an accessible format. For research purposes, access should be provided to data in raw, processed, and analysed formats.
It is important to be aware of the historical context of data collection and research conduct in the neighborhood and community where monitoring will take place, especially for imagery. Over the past several decades, many local governments have expanded surveillance of public places with the stated goals of deterring crime and enforcing the law (Brown, 2008). However, throughout United States history, surveillance has been used as a means of enforcing structural racism, including enslavement, Jim Crow laws, and segregation, to the harm of millions of people of color (Gellman and Adler-Bell, 2017; Arnett, 2020; Lee and Chin, 2022). As a result, practitioners need to approach monitoring technology with surveillance implications through authentic, responsive engagement with community stakeholders and with an overarching mandate to avoid causing harm.
Community engagement is a key component of selecting the location, type, and scale of community-centered NBS in the area in which it is implemented. However, this is outside the scope of this paper and is discussed by Ferreira et al. (2020) and Mok et al. (2021).
5 Conclusion
Nature-based solutions are a promising technology for addressing urban stormwater management, an issue that will only become more critical in the face of climate change and urbanization. However, there is a need for more systematic collection of consistent performance data using similar monitoring methods. To address this gap, this paper provides a summary of available literature on commonly used methods for monitoring NBS and recommendations for consistent monitoring of community-centered NBS systems that vary in form, scale, and degree of naturalness. In addition, we provide an overview of how this monitoring data can be used to evaluate NBS performance and discuss common barriers to this type of monitoring. The application of the monitoring methodologies presented here would provide consistent performance data, which could be used to quantify the hydrological benefits of community-centered NBS and allow for regional comparisons of the performance of NBS systems. This would support greater adoption of the most appropriate NBS for stormwater management in different urban systems, thereby decreasing flooding and increasing adaptation to the impacts of climate change.
The recommendations presented here are particularly useful for community-based organizations and non-profit organizations who are now leading the planning and implementation of community-centered NBS systems in many areas, but previously had been provided limited resources for how to perform this type of monitoring. In addition, the recommendations presented are also applicable for research institutions analysing the performance of NBS, and government agencies, such as municipal park districts or water and sewer authorities. Cost estimates provided in this paper can be used to develop monitoring budgets, which should be included in the overall NBS project budget to ensure that adequate financial and staff resources are allocated for monitoring and maintenance efforts.
Author contributions
CO’B: Conceptualization, Formal analysis, Visualization, Writing – original draft, Writing – review & editing. MM: Conceptualization, Visualization, Writing – original draft, Writing – review & editing. LC: Visualization, Writing – original draft, Writing – review & editing. JJ: Writing – original draft, Writing – review & editing. JW: Visualization, Writing – original draft, Writing – review & editing. RW: Conceptualization, Writing – original draft, Writing – review & editing. DW-C: Conceptualization, Writing – original draft, Writing – review & editing. AS: Writing – original draft, Writing – review & editing. KR: Writing – original draft, Writing – review & editing. DG: Writing – original draft, Writing – review & editing. WM: Conceptualization, Supervision, Writing – original draft, Writing – review & editing. AP: Conceptualization, Supervision, Writing – original draft, Writing – review & editing.
Funding
The author(s) declare that financial support was received for the research, authorship, and/or publication of this article. This work was funded by Walder Foundation Resilient by Nature (RxN), the National Science Foundation’s SAVUER project (grant no. 184868), and the National Science Foundation’s SAGE project (grant no. 1935984). This material is based in part upon work supported by the United States Department of Energy, Office of Science, Office of Biological and Environmental Research’s Urban Integrated Field Laboratories CROCUS project research activity, under Award no. DE-SC0023364.
Acknowledgments
The authors would like to acknowledge Mike Tomas and the Garfield Park Community Council for their assistance in accessing and monitoring the Garfield Park Eco-Orchard site and Karl Gnaedinger, Emilie Pfeiffer, Shane Tripp, and Martha Lopez-Salazar for their assistance in accessing and monitoring Indian Boundary Prairies. We would also like to acknowledge the Walder Foundation for bringing together multiple organizations engaged in this work to collaborate on this paper.
Conflict of interest
KR was employed by OAI, Inc. DG was employed by Waterwell, LLC.
The remaining authors declare that the research was conducted in the absence of any commercial or financial relationships that could be construed as a potential conflict of interest.
Publisher’s note
All claims expressed in this article are solely those of the authors and do not necessarily represent those of their affiliated organizations, or those of the publisher, the editors and the reviewers. Any product that may be evaluated in this article, or claim that may be made by its manufacturer, is not guaranteed or endorsed by the publisher.
Supplementary material
The Supplementary material for this article can be found online at: https://www.frontiersin.org/articles/10.3389/frwa.2024.1370501/full#supplementary-material
Footnotes
1. ^The costs provided in Table 1 do not include personnel time for data collection, analysis, and sensor maintenance. The time requirements for these tasks can range from a minimum of approximately 70 h annually to over 400 h. This will vary based on the size and proximity of the site, the number and types of sensors at each site, and the extent of analysis required to meet project objectives and evaluate outcomes. Additional cost data are provided in Supplemental material.
References
AECOM, Hey and Associates, Inc (2018). 5th avenue corridor 100% construction documents. Volo, IL: AECOM, Hey and Associates, Inc.
Ahern, J. (2007). Green Infrastructure for Cities the Spatial Dimension. Available at: https://www.semanticscholar.org/paper/Green-Infrastructure-For-Cities-The-Spatial-Ahern/5a5eaa2f3394bf3c8e5eb4400812b2d3c822eb9d.
Alizadehtazi, B., and Montalto, F. A. (2020). Precipitation and soil moisture data in two engineered urban green infrastructure facilities in New York city. Data Brief 32:106225. doi: 10.1016/j.dib.2020.106225
American Society for Testing and Materials (2020). D2487 standard practice for classification of soils for engineering purposes (unified soil classification system). Pennsylvania: American Society for Testing and Materials.
American Wick Drain. (n.d.). Bioretention/bioswale. AWD–American Wick Drain. Available at: https://www.awd-usa.com/drainage-applications/bioretention-bioswale (Accessed August 25, 2023).
Anderson, V., Gough, W. A., and Agic, B. (2021). Nature-based equity: an assessment of the public health impacts of green infrastructure in Ontario Canada. Int. J. Environ. Res. Public Health 18:763. doi: 10.3390/ijerph18115763
Ando, A. W., and Freitas, L. P. C. (2011). Consumer demand for green stormwater management technology in an urban setting: the case of Chicago rain barrels. Water Resour. Res. 47. doi: 10.1029/2011WR011070
Baptiste, A. K., Foley, C., and Smardon, R. (2015). Understanding urban neighborhood differences in willingness to implement green infrastructure measures: a case study of Syracuse, NY. Landsc. Urban Plan. 136, 1–12. doi: 10.1016/j.landurbplan.2014.11.012
Bartesaghi Koc, C., Osmond, P., and Peters, A. (2018). Evaluating the cooling effects of green infrastructure: a systematic review of methods, indicators and data sources. Sol. Energy 166, 486–508. doi: 10.1016/j.solener.2018.03.008
Bartholomew, M. J. (2016). Optical Rain Gauge Instrument Handbook. Available at: https://docslib.org/doc/6415944/optical-rain-gauge-instrument-handbook.
Batalini de Macedo, M., Ambrogi Ferreira do Lago, C., Mendiondo, E. M., and Giacomoni, M. H. (2019). Bioretention performance under different rainfall regimes in subtropical conditions: a case study in São Carlos, Brazil. Adv. Environ. Res. 248:109266. doi: 10.1016/j.jenvman.2019.109266
Beauchamp, P., and Adamowski, J. (2013). An integrated framework for the development of green infrastructure: a literature review. Eur. J. Sustain. Dev. 2, 1–24. doi: 10.14207/ejsd.2013.v2n3p1
Boening-Ulman, K. M., Winston, R. J., Wituszynski, D. M., Smith, J. S., Andrew Tirpak, R., and Martin, J. F. (2022). Hydrologic impacts of sewershed-scale green infrastructure retrofits: outcomes of a four-year paired watershed monitoring study. J. Hydrol. 611:128014. doi: 10.1016/j.jhydrol.2022.128014
British Geological Survey. (n.d.). SuDS. Available at: https://www.bgs.ac.uk/geology-projects/suds/ (Accessed June 4, 2024).
Brown, J. (2008). Pan, tilt, zoom: regulating the use of video surveillance of public places part V: privacy: section a: notes. Berkeley Technol. Law J. 23, 755–782. Available at: https://www.jstor.org/stable/24118339
Brunet, P., Clément, R., and Bouvier, C. (2010). Monitoring soil water content and deficit using electrical resistivity tomography (ERT) – a case study in the Cevennes area, France. J. Hydrol. 380, 146–153. doi: 10.1016/j.jhydrol.2009.10.032
Carson, T. B., Marasco, D. E., Culligan, P. J., and McGillis, W. R. (2013). Hydrological performance of extensive green roofs in New York city: observations and multi-year modeling of three full-scale systems. Environ. Res. Lett. 8:024036. doi: 10.1088/1748-9326/8/2/024036
Castelli, G., Foderi, C., Guzman, B. H., Ossoli, L., Kempff, Y., Bresci, E., et al. (2017). Planting waterscapes: green infrastructures, landscape and hydrological modeling for the future of Santa Cruz de la sierra, Bolivia. Forests 8:437. doi: 10.3390/f8110437
Catalano de Sousa, M. R., Montalto, F. A., and Gurian, P. (2016). Evaluating green infrastructure Stormwater capture performance under extreme precipitation. J. Extreme Events 3:1650006. doi: 10.1142/S2345737616500068
Catlett, C., Beckman, P., Ferrier, N., Papka, M. E., Sankaran, R., Solin, J., et al. (2022). Hands-on computer science: the Array of things experimental urban instrument. Comput. Sci. Eng. 24, 57–63. doi: 10.1109/MCSE.2021.3139405
Chang, J., Qu, Z., Xu, R., Pan, K., Xu, B., Min, Y., et al. (2017). Assessing the ecosystem services provided by urban green spaces along urban center-edge gradients. Sci. Rep. 7:11226. doi: 10.1038/s41598-017-11559-5
Chen, D., Hu, M., Guo, Y., and Dahlgren, R. A. (2016). Changes in river water temperature between 1980 and 2012 in Yongan watershed, eastern China: magnitude, drivers and models. J. Hydrol. 533, 191–199. doi: 10.1016/j.jhydrol.2015.12.005
City of Lancaster. (2019). Green Infrastructure Monitoring Plan. Available at: https://www.cityoflancasterpa.gov/wp-content/uploads/2014/03/Lancaster-GI-Monitoring_FINAL_reduced.pdf
Clean Water Education Partnership. (2023). Green Stormwater Infrastructure (GSI). Available at: https://nc-cleanwater.com/gsi/#what-is-gsi-and-why-is-it-used.
Cook County Board of Commissioners. (2010). Cook County 2008 LiDAR and Topographic Data Services (Contract No. 08–41–342), 1-Foot Contours for Cook County, Illinois. (Version 1.0.) [Geodatabase Vector Digital Data].
Cook, L. M., VanBriesen, J. M., and Samaras, C. (2021). Using rainfall measures to evaluate hydrologic performance of green infrastructure systems under climate change. Sustain. Resilient Infrastruct. 6, 156–180. doi: 10.1080/23789689.2019.1681819
Cristiano, E., ten Veldhuis, M.-C., and van de Giesen, N. (2017). Spatial and temporal variability of rainfall and their effects on hydrological response in urban areas – a review. Hydrol. Earth Syst. Sci. 21, 3859–3878. doi: 10.5194/hess-21-3859-2017
de Jong, S. M., Heijenk, R. A., Nijland, W., and van der Meijde, M. (2020). Monitoring soil moisture dynamics using electrical resistivity tomography under homogeneous Field conditions. Sensors 20:313. doi: 10.3390/s20185313
Elliott, R. M., Adkins, E. R., Culligan, P. J., and Palmer, M. I. (2018). Stormwater infiltration capacity of street tree pits: quantifying the influence of different design and management strategies in new York City. Ecol. Eng. 111, 157–166. doi: 10.1016/j.ecoleng.2017.12.003
Environmental Policy Innovation Center. (2024). Navigating the terms “green infrastructure”, “nature-based solutions” and co. Environmental Policy Innovation Center. Available at: https://www.policyinnovation.org/blog/navigating-the-terms-gi-nbs.
Eskin, J., Price, T., Cooper, J., and Schleizer, W. (2021). A design guide for green Stormwater infrastructure best management practices. Delta Institute. Available at: https://delta-institute.org/wp-content/uploads/2021/10/GI-Designs-Guide-08192021.pdf.
Fassman-Beck, E., Voyde, E., Simcock, R., and Hong, Y. S. (2013). 4 living roofs in 3 locations: does configuration affect runoff mitigation? J. Hydrol. 490, 11–20. doi: 10.1016/j.jhydrol.2013.03.004
Feldman, A., Foti, R., and Montalto, F. (2019). Green infrastructure implementation in urban parks for Stormwater management. J. Sustain. Water Built Environ. 5:05019003. doi: 10.1061/JSWBAY.0000880
Ferreira, V., Barreira, A. P., Loures, L., Antunes, D., and Panagopoulos, T. (2020). Stakeholders’ engagement on nature-based solutions: a systematic literature review. Sustain. For. 12:640. doi: 10.3390/su12020640
Fletcher, T., Bertrand-Krajewski, J.-L., Bonneau, J., Burns, M., Poelsma, P., and Thom, J. (2021). “Chapter 4: measuring the water balance in stormwater control measures” in Metrology in urban drainage and Stormwater management: Plug and pray. ed. T. Fletcher (London: IWA Publishing), 105–134.
Fuentes, S., Tongson, E., and Gonzalez Viejo, C. (2021). Urban green infrastructure monitoring using remote sensing from integrated visible and thermal infrared cameras mounted on a moving vehicle. Sensors 21:295. doi: 10.3390/s21010295
Furberg, D., Ban, Y., and Mörtberg, U. (2020). Monitoring urban green infrastructure changes and impact on habitat connectivity using high-resolution satellite data. Remote Sens. 12:72. doi: 10.3390/rs12183072
Geberemariam, T. K. (2017). Post construction green infrastructure performance monitoring parameters and their functional components. Environments 4:10002. doi: 10.3390/environments4010002
Gellman, B., and Adler-Bell, S. (2017). The disparate impact of surveillance. The Century Foundation. Available at: https://tcf.org/content/report/disparate-impact-surveillance/.
Golden, H. E., and Hoghooghi, N. (2018). Green infrastructure and its catchment-scale effects: an emerging science. WIREs. Water 5:1254. doi: 10.1002/wat2.1254
Gonzalez, L. M. H., Rivera, V. A., Akosa, D., Phillips, C. B., Hatch, S. L., Miller, W. M., et al. (2023). Road salt intrusion dynamics in an ex-urban native wetland complex. PLoS Water 2:e0000148. doi: 10.1371/journal.pwat.0000148
Goodspeed, R., Liu, R., Gounaridis, D., Lizundia, C., and Newell, J. (2022). A regional spatial planning model for multifunctional green infrastructure. Environ. Plan. B Urban Anal. City Sci. 49, 815–833. doi: 10.1177/23998083211033610
Gordon, B. L., Quesnel, K. J., Abs, R., and Ajami, N. K. (2018). A case-study based framework for assessing the multi-sector performance of green infrastructure. J. Environ. Manag. 223, 371–384. doi: 10.1016/j.jenvman.2018.06.029
Gourevitch, J. D., Kousky, C., Liao, Y., Nolte, C., Pollack, A. B., Porter, J. R., et al. (2023). Unpriced climate risk and the potential consequences of overvaluation in US housing markets. Nat. Clim. Chang. 13, 250–257. doi: 10.1038/s41558-023-01594-8
Grey, V., Livesley, S. J., Fletcher, T. D., and Szota, C. (2018). Tree pits to help mitigate runoff in dense urban areas. J. Hydrol. 565, 400–410. doi: 10.1016/j.jhydrol.2018.08.038
Griffin, J. S., Haug, L. A., Rivera, V. A., Gonzalez, L. M. H., Kelly, J. J., Miller, W. M., et al. (2020). Soil hydrology drives ecological niche differentiation in a native prairie microbiome. FEMS Microbiol. Ecol. 96:fiz163. doi: 10.1093/femsec/fiz163
Hernandez Gonzalez, L. M., Rivera, V. A., Phillips, C. B., Haug, L. A., Hatch, S. L., Yeager, L. E., et al. (2019). Characterization of soil profiles and elemental concentrations reveals deposition of heavy metals and phosphorus in a Chicago-area nature preserve, Gensburg Markham prairie. J. Soils Sediments 19, 3817–3831. doi: 10.1007/s11368-019-02315-5
Hey and Associates, Inc (2021). 2020 Stormwater monitoring Field installation report—Indian boundary prairies: Sundrop and paintbrush prairies. Volo, IL: Hey and Associates, Inc.
Hung, F., Harman, C., Hobbs, B., and Sivapalan, M. (2020). Assessment of climate, sizing, and location controls on green infrastructure efficacy: a timescale framework. Water Resour. Res. 56:e2019WR026141. doi: 10.1029/2019WR026141
Institute for Resilient Infrastructure Systems. (n.d.). What is natural infrastructure? Available at: https://iris.uga.edu/about-infrastructure/. (Accessed June 4, 2024).
Jarden, K. M., Jefferson, A. J., and Grieser, J. M. (2016). Assessing the effects of catchment-scale urban green infrastructure retrofits on hydrograph characteristics. Hydrol. Process. 30, 1536–1550. doi: 10.1002/hyp.10736
Johnson, T., Butcher, J., Santell, S., Schwartz, S., Julius, S., and LeDuc, S. (2022). A review of climate change effects on practices for mitigating water quality impacts. J. Water Clim. Change 13, 1684–1705. doi: 10.2166/wcc.2022.363
Kaminski, A., and Bzdusek, P. A. (2017). Regulation and policy: getting green infrastructure right. Available at: https://slideplayer.com/slide/12958124/.
Kato, S., and Ahern, J. (2008). ‘Learning by doing’: adaptive planning as a strategy to address uncertainty in planning. J. Environ. Plan. Manag. 51, 543–559. doi: 10.1080/09640560802117028
Kazemi, H. (2014). Evaluating the effectiveness and hydrological performance of green infrastructure stormwater control measures. Electron. Theses Diss. 2014:1744. doi: 10.18297/etd/1744
Kerkez, B., Gruden, C., Lewis, M., Montestruque, L., Quigley, M., Wong, B., et al. (2016). Smarter Stormwater Systems. Environ. Sci. Technol. 50, 7267–7273. doi: 10.1021/acs.est.5b05870
Khan, U. T., Valeo, C., Chu, A., and van Duin, B. (2012). Bioretention cell efficacy in cold climates: part 1 — hydrologic performance. Can. J. Civ. Eng. 39, 1210–1221. doi: 10.1139/l2012-110
Kim, G., and Miller, P. A. (2019). The impact of green infrastructure on human health and well-being: the example of the Huckleberry Trail and the heritage Community Park and natural area in Blacksburg, Virginia. Sustain. Cities Soc. 48:101562. doi: 10.1016/j.scs.2019.101562
Korgaonkar, Y., Guertin, D. P., Goodrich, D. C., Unkrich, C., Kepner, W. G., and Burns, I. S. (2018). Modeling urban hydrology and green infrastructure using the AGWA urban tool and the KINEROS2 model. Front. Built Environ. 4, 1–15. doi: 10.3389/fbuil.2018.00058
Lamond, J., and Everett, G. (2019). Sustainable blue-green infrastructure: a social practice approach to understanding community preferences and stewardship. Landsc. Urban Plan. 191:103639. doi: 10.1016/j.landurbplan.2019.103639
Lee, N. T., and Chin, C. (2022). Police surveillance and facial recognition: Why data privacy is imperative for communities of color. Brookings. Available at: https://www.brookings.edu/articles/police-surveillance-and-facial-recognition-why-data-privacy-is-an-imperative-for-communities-of-color/.
Lewellyn, C., Lyons, C. E., Traver, R. G., and Wadzuk, B. M. (2016). Evaluation of seasonal and large storm runoff volume capture of an infiltration green infrastructure system. J. Hydrol. Eng. 21:04015047. doi: 10.1061/(ASCE)HE.1943-5584.0001257
Li, L., Bisht, G., Hao, D., and Leung, L. R. (2024). Global 1 km land surface parameters for kilometer-scale earth system modeling. Earth Syst. Sci. Data 16, 2007–2032. doi: 10.5194/essd-16-2007-2024
Li, C., Peng, C., Chiang, P.-C., Cai, Y., Wang, X., and Yang, Z. (2019). Mechanisms and applications of green infrastructure practices for stormwater control: a review. J. Hydrol. 568, 626–637. doi: 10.1016/j.jhydrol.2018.10.074
Lim, T. C., and Welty, C. (2018). Assessing variability and uncertainty in green infrastructure planning using a high-resolution surface-subsurface hydrological model and site-monitored flow data. Frontiers. Built Environ. 4:71. doi: 10.3389/fbuil.2018.00071
Mason, B. E., Schmidt, J. Q., and Kerkez, B. (2021). Sensor networks for real-time green infrastructure monitoring. 15th International Conference on Urban Drainage. Available at: https://asnevents.s3.amazonaws.com/event/1735/abstract/76250/extra/3158/615a358d755b9-ICUD_2021_GISensorNetwork.pdf.
McLaughlin, J., Jones, M., Leo, W., Newman, T., and Stein, J. (2014). Green infrastructure to reduce Stormwater runoff in New York City: Post-construction monitoring over multiple years and lessons learned, pp. 1001–1009.
Meixner, T., Berkowitz, A. R., Downey, A. E., Pillich, J., LeVea, R., Smith, B. K., et al. (2021). Rapid assessment and long-term monitoring of green Stormwater infrastructure with citizen scientists. Sustain. For. 13:520. doi: 10.3390/su132212520
Mignot, E., and Dewals, B. (2022). Hydraulic modelling of inland urban flooding: recent advances. J. Hydrol. 609:127763. doi: 10.1016/j.jhydrol.2022.127763
Miller, S. M., and Montalto, F. A. (2019). Stakeholder perceptions of the ecosystem services provided by green infrastructure in new York City. Ecosyst. Serv. 37:100928. doi: 10.1016/j.ecoser.2019.100928
Mok, S., Mačiulytė, E., Bult, P. H., and Hawxwell, T. (2021). Valuing the invaluable(?)—a framework to facilitate stakeholder engagement in the planning of nature-based solutions. Sustain. For. 13:57. doi: 10.3390/su13052657
Monteiro, R., Ferreira, J. C., and Antunes, P. (2020). Green infrastructure planning principles: An integrated literature review. Land 9:525. doi: 10.3390/land9120525
Moruza, G., Burgis, C., Zhang, W., Henderson, D., and Smith, J. (2021). Runoff reduction by four green Stormwater infrastructure Systems in a Shared Environment. J. Sustain. Water Built Environ. 7:4021004. doi: 10.1061/JSWBAY.0000932
Nanía, L. S., León, A. S., and García, M. H. (2015). Hydrologic-hydraulic model for simulating dual drainage and flooding in urban areas: application to a catchment in the metropolitan area of Chicago. J. Hydrol. Eng. 20:04014071. doi: 10.1061/(ASCE)HE.1943-5584.0001080
National Academies of Sciences, Engineering, and Medicine (2018). Learning through citizen science: Enhancing opportunities by design. Washington, DC: National Academies of Sciences, Engineering, and Medicine.
Nature-Based Solutions IUCN. (n.d.). Available at: https://www.iucn.org/our-work/nature-based-solutions. (Accessed December 7, 2022).
Nemirovsky, E. M., Lee, R. S., and Welker, A. L. (2015). Vertical and lateral extent of the influence of a rain garden on the water table. J. Irrig. Drain. Eng. 141:4014053. doi: 10.1061/(ASCE)IR.1943-4774.0000799
Nowak, D. J., and Greenfield, E. J. (2012). Tree and impervious cover in the United States. Landsc. Urban Plan. 107, 21–30. doi: 10.1016/j.landurbplan.2012.04.005
Ouellet, V., Khamis, K., Croghan, D., Hernandez Gonzalez, L. M., Rivera, V. A., Phillips, C. B., et al. (2021). Green roof vegetation management alters potential for water quality and temperature mitigation. Ecohydrology 14:e2321. doi: 10.1002/eco.2321
Paus, K. H., Muthanna, T. M., and Braskerud, B. C. (2015). The hydrological performance of bioretention cells in regions with cold climates: seasonal variation and implications for design. Hydrol. Res. 47, 291–304. doi: 10.2166/nh.2015.084
Philadelphia Water Department. (2009). Post construction monitoring. Available at: http://archive.phillywatersheds.org/ltcpu/LTCPU_Section12_PostConstructionMonitoring.pdf.
Phillips, T. H., Baker, M. E., Lautar, K., Yesilonis, I., and Pavao-Zuckerman, M. A. (2019). The capacity of urban forest patches to infiltrate stormwater is influenced by soil physical properties and soil moisture. J. Environ. Manag. 246, 11–18. doi: 10.1016/j.jenvman.2019.05.127
Prudencio, L., and Null, S. (2018). Stormwater management and ecosystem services: a review. Environ. Res. Lett. 13:81. doi: 10.1088/1748-9326/aaa81a
Qian, Y., Chakraborty, T. C., Li, J., Li, D., He, C., Sarangi, C., et al. (2022). Urbanization impact on regional climate and extreme weather: current understanding, uncertainties, and future research directions. Adv. Atmos. Sci. 39, 819–860. doi: 10.1007/s00376-021-1371-9
Saaroni, H., Amorim, J. H., Hiemstra, J. A., and Pearlmutter, D. (2018). Urban green infrastructure as a tool for urban heat mitigation: survey of research methodologies and findings across different climatic regions. Urban Clim. 24, 94–110. doi: 10.1016/j.uclim.2018.02.001
Schlea, D., Martin, J. F., Ward, A. D., Brown, L. C., and Suter, S. A. (2014). Performance and water table responses of retrofit rain gardens. J. Hydrol. Eng. 19:05014002. doi: 10.1061/(ASCE)HE.1943-5584.0000797
Sharma, A., Wuebbles, D. J., Kotamarthi, R., Calvin, K., Drewniak, B., Catlett, C. E., et al. (2020). Urban-scale processes in high-spatial-resolution earth system models. Bull. Am. Meteorol. Soc. 101, E1555–E1561. doi: 10.1175/BAMS-D-20-0114.1
Shi, X., and Qin, M. (2018). Research on the optimization of regional green infrastructure network. Sustain. For. 10:649. doi: 10.3390/su10124649
Shuster, W. D., Dadio, S., Drohan, P., Losco, R., and Shaffer, J. (2014). Residential demolition and its impact on vacant lot hydrology: implications for the management of stormwater and sewer system overflows. Landsc. Urban Plan. 125, 48–56. doi: 10.1016/j.landurbplan.2014.02.003
Southwestern Pennsylvania Commission Water Resource Center. (n.d.). Stormwater best management practices. SPC Water Resource Center. Available at: https://spcwater.org/topics/stormwater-management/stormwater-best-management-practices-2/ (Accessed June 4, 2024).
Stewart, R. D., Lee, J. G., Shuster, W. D., and Darner, R. A. (2017). Modelling hydrological response to a fully-monitored urban bioretention cell. Hydrol. Process. 31, 4626–4638. doi: 10.1002/hyp.11386
Sun, J., Cheshmehzangi, A., and Wang, S. (2020). Green infrastructure practice and a sustainability key performance indicators framework for Neighbourhood-level construction of Sponge City Programme. J. Environ. Prot. 11, 82–109. doi: 10.4236/jep.2020.112007
Tabari, H. (2020). Climate change impact on flood and extreme precipitation increases with water availability. Sci. Rep. 10:13768. doi: 10.1038/s41598-020-70816-2
Tang, R., Zhang, S., and Cheng, Z. (2020). Monitoring methods on evaluating the water-related green infrastructure performance. IOP Conf. Ser. Earth Environ. Sci. 432:012004. doi: 10.1088/1755-1315/432/1/012004
Taramelli, A., Lissoni, M., Piedelobo, L., Schiavon, E., Valentini, E., Nguyen Xuan, A., et al. (2019). Monitoring green infrastructure for natural water retention using Copernicus global land products. Remote Sens. 11:583. doi: 10.3390/rs11131583
Thomas, B. F., and Vogel, R. M. (2012). The impact of Stormwater recharge practices on Boston groundwater levels, pp. 2352–2361.
Tu, M., Caplan, J. S., Eisenman, S. W., and Wadzuk, B. M. (2020). When green infrastructure turns Grey: plant water stress as a consequence of overdesign in a tree trench system. Water 12:573. doi: 10.3390/w12020573
U.S. Geological Survey. (2023). Rapid Assessment of Green Infrastructure to Inform Future Implementation in the Great Lakes. Available at: https://www.usgs.gov/centers/upper-midwest-water-science-center/science/rapid-assessment-green-infrastructure-inform?items_per_page=6#overview.
United Nations, Department of Economic and Social Affairs, Population Division (2019). World urbanization prospects: The 2018 revision. New York: United Nations, Department of Economic and Social Affairs, Population Division.
University of Michigan Graham Sustainability Institute. (n.d.). Soil Water Assessment Tool (SWAT) FAQ. Available at: https://graham.umich.edu/water/swat-faq (Accessed June 4, 2024).
University of Oregon, Office of the Vice President for and Research and Innovation. (n.d.). Potential Research Risks or Discomforts to Participants. Available at: https://research.uoregon.edu/manage/research-integrity-compliance/human-subjects-research/potential-research-risks-or-discomforts-participants (Accessed June 4, 2024).
University of Reading. (n.d.). What is Natural Flood Management? NERC Natural Flood Management Research Programme. Available at: https://research.reading.ac.uk/nerc-nfm/what-is-natural-flood-management/ (Accessed June 4, 2024).
US EPA, O. (2015a). Nonpoint Source: Urban Areas [Overviews and Factsheets]. Available at: https://www.epa.gov/nps/nonpoint-source-urban-areas.
US EPA, O. (2015b). What is Green Infrastructure? [Overviews and Factsheets]. Available at: https://www.epa.gov/green-infrastructure/what-green-infrastructure.
Venkataramanan, V., Lopez, D., McCuskey, D. J., Kiefus, D., McDonald, R. I., Miller, W. M., et al. (2020). Knowledge, attitudes, intentions, and behavior related to green infrastructure for flood management: a systematic literature review. Sci. Total Environ. 720:137606. doi: 10.1016/j.scitotenv.2020.137606
Versini, P.-A., Gires, A., Tchinguirinskaia, I., and Schertzer, D. (2016). Toward an operational tool to simulate green roof hydrological impact at the basin scale: a new version of the distributed rainfall–runoff model multi-hydro. Water Sci. Technol. 74, 1845–1854. doi: 10.2166/wst.2016.310
Wadzuk, B., Gile, B., Smith, V., Ebrahimian, A., and Traver, R. (2021). Call for a dynamic approach to GSI maintenance. J. Sustain. Water Built Environ. 7:02521001. doi: 10.1061/JSWBAY.0000945
Weber, T., and Wolf, J. (2000). Maryland’s green infrastructure—using landscape assessment tools to identify a regional conservation strategy. Environ. Monit. Assess. 63, 265–277. doi: 10.1023/A:1006416523955
Weller, C. (2023). Amid strong labor market, state and local governments struggle to hire. Forbes. Available at: https://www.forbes.com/sites/christianweller/2023/02/09/amid-strong-labor-market-state-and-local-governments-struggle-to-hire/.
Winston, R. J., Dorsey, J. D., and Hunt, W. F. (2016). Quantifying volume reduction and peak flow mitigation for three bioretention cells in clay soils in Northeast Ohio. Sci. Total Environ. 553, 83–95. doi: 10.1016/j.scitotenv.2016.02.081
Woznicki, S. A., Hondula, K. L., and Jarnagin, S. T. (2018). Effectiveness of landscape-based green infrastructure for stormwater management in suburban catchments. Hydrol. Process. 32, 2346–2361. doi: 10.1002/hyp.13144
Xie, M., Wang, R., Yang, J., and Cheng, Y. (2021). A monitoring and control system for Stormwater management of urban green infrastructure. Water 13:438. doi: 10.3390/w13111438
Zhang, K., and Chui, T. F. M. (2019). Effect of spatial allocation of green infrastructure on surface-subsurface hydrology in shallow groundwater environment, 147–152.
Zhang, S., Lin, Z., Zhang, S., and Ge, D. (2021). Stormwater retention and detention performance of green roofs with different substrates: observational data and hydrological simulations. J. Environ. Manag. 291:112682. doi: 10.1016/j.jenvman.2021.112682
Keywords: stormwater management, green infrastructure, monitoring, instrumentation, flood reduction, nature-based solutions
Citation: O’Brien CM, Mossman M, Chamberlain L, Jenkins J, Watson J, Wilson R, Williams-Clark D, Singer A, Riggio K, Gallet D, Miller WM and Packman AI (2024) Community-centered instrumentation and monitoring of nature-based solutions for urban stormwater control. Front. Water. 6:1370501. doi: 10.3389/frwa.2024.1370501
Edited by:
Lei Li, The University of Manchester, United KingdomReviewed by:
Andrea Emilio Rizzoli, University of Applied Sciences and Arts of Southern Switzerland, SwitzerlandJeremy Carter, The University of Manchester, United Kingdom
Copyright © 2024 O’Brien, Mossman, Chamberlain, Jenkins, Watson, Wilson, Williams-Clark, Singer, Riggio, Gallet, Miller and Packman. This is an open-access article distributed under the terms of the Creative Commons Attribution License (CC BY). The use, distribution or reproduction in other forums is permitted, provided the original author(s) and the copyright owner(s) are credited and that the original publication in this journal is cited, in accordance with accepted academic practice. No use, distribution or reproduction is permitted which does not comply with these terms.
*Correspondence: Aaron I. Packman, YS1wYWNrbWFuQG5vcnRod2VzdGVybi5lZHU=