- Takuvik Joint International Laboratory (CNRS & ULaval), Département de Biologie, Université Laval, Pavillon Alexandre-Vachon, Québec, QC, Canada
Thalassiosira gravida is a major Arctic diatom responsible for the under-ice spring bloom. We investigated T. gravida physiological plasticity growing it at two temperatures (0 and 5°C) and under different light intensities typically found in its natural environment. T. gravida showed remarkable thermal- and photo-acclimatory plasticity including: low light saturation parameter for growth (KE) and photosynthesis (EK), low μmax but relatively high Chl a/C, low C/N, and decreasing light-saturated carbon fixation rate (PmC) with increasing growth irradiance. T. gravida also showed remarkable photoprotective features, namely a strong sustained non-photochemical quenching (NPQs, hour kinetics relaxation) supported by a high amount of xanthophyll cycle pigments. T. gravida growth remained possible under a wide range of irradiances but photosynthetic plasticity was higher at moderately low light (up to ~50 μmol photons m−2 s−1), nevertheless corresponding to the mean in situ conditions under which it predominates, i.e., underneath the spring thin-ice punctuated with melting ponds. The potential role of NPQs in the photophysiological plasticity of T. gravida is discussed.
Introduction
Thalassiosira gravida is a centric diatom that is among the five most abundant phytoplankton in the Arctic Ocean (Poulin et al., 2011). It is a major and intense spring bloomer that generally appears early during the productive season (Booth et al., 2002), when the ice-pack is still present, even blooming underneath the ice thanks to the increase in light transmittance due to the formation of melt ponds (Arrigo et al., 2012). T. gravida can also be found abundant later in melt and even open waters when the average irradiance is higher (Booth et al., 2002; Poulin et al., 2011). In spring, the light availability in the water column is mainly controlled by the photoperiod and by the ice and snow optical properties (Perovich and Polashenski, 2012). Hence the habitat of Arctic diatoms is characterized by low temperatures and very low light intensities to which they are adapted (Petrou et al., 2016; Lacour et al., 2017). Nevertheless, during the transition to summer, diatoms can experience relatively large fluctuations in light dose generated by the fast increase in photoperiod coupled to sometimes sudden, prolonged and unpredictable increase in irradiance mainly due to flash snow melting and break-up of the ice (Kauko et al., 2017). Melt ponds and leads formation is a more local but essential feature that also drastically increases light transmittance and allows blooms underneath the ice (Arrigo et al., 2012; Assmy et al., 2017; Horvat et al., 2017). With the ongoing climate change, the Arctic Ocean light climate is facing deep modifications, especially in seasonally sea-ice covered areas (Nicolaus et al., 2012). Dramatic light changes are mainly driven by the decrease of the “albedo effect” due to thinner snow and sea-ice cover (Nicolaus et al., 2012; Perovich and Polashenski, 2012), and the earlier onset of melt pond formation and the expansion of their surface (Markus et al., 2009; Arrigo et al., 2012; Horvat et al., 2017). The general shrinking of sea-ice (Stroeve et al., 2012; Notz and Stroeve, 2016) is progressively leading sea-ice dominated habitats to disappear at the expense of open water column (Lee et al., 2017). As a consequence, and because of more open, warmer, and less salty (due to snow and ice melting) surface waters, stronger ocean stratification has been occurring (Wassmann and Reigstad, 2011; Blais et al., 2017), trapping diatoms near the surface. In such conditions, one of the anticipated stresses diatoms will face is higher average coupled irradiances and temperatures (Wassmann and Reigstad, 2011; Nicolaus et al., 2012), with more frequent exposures to excess light (Leu et al., 2016). Although the relative increases in light availability and temperature are predicted to boost the pan-Arctic ocean primary production (Wassmann and Reigstad, 2011), with increase in frequency and extent of blooms underneath the ice (Horvat et al., 2017), the harmful effects of excess light and higher temperature is not well-documented, especially on Arctic phytoplankton (as opposed to Antarctica strains; see Petrou et al., 2016). Recently, a negative effect on both Arctic diatoms photosynthetic productivity and lipid content and quality has been reported (Leu et al., 2016). In this study, we aimed at understanding how the major Arctic strain T. gravida thrives under the relatively large variations in irradiance and temperature in its natural habitat due to both seasonal and on-going climate change.
The combined effects of temperature and irradiance on polar phytoplankton physiology are poorly understood (Lyon and Mock, 2014). In a recent review, Lacour et al. (2017) showed that a decrease in temperature leads to a decrease in the light saturation parameter for cell growth (KE), thus deeply affecting photoacclimation at the cell level. Few reports (Davison, 1991; Mock and Hoch, 2005) also highlighted the strong interactions between acclimation to low temperature and to irradiance, by evidencing the role of intra-cellular energy balance in the control of cell response to the environment (i.e., “photochilling” stress), i.e., temperature affects phytoplankton growth by altering the balance between light energy absorption and utilization (Fanesi et al., 2016). Photoacclimation refers to phenotypic adjustments, which often take place in the plastids, in response to relatively sustained variations of environmental irradiance (Falkowski and Laroche, 1991; Macintyre et al., 2002). Macintyre et al. (2002) reviewed photoacclimation of pigment content and the photosynthesis-irradiance response curve changes of a wide range of temperate phytoplankton species. It is only recently that light response has been studied in several polar species, including diatoms (Kropuenske et al., 2009, 2010; Arrigo et al., 2010; Mills et al., 2010; Petrou et al., 2010, 2011; Petrou and Ralph, 2011; van de Poll et al., 2011). Several characteristics (i.e., ability to exploit variable light, photoprotection, etc.), similar to “photostasis” in overwintering evergreen land plants (Öquist and Huner, 2003; Míguez et al., 2015), were identified to explain their distribution and succession among different polar ecosystems and habitats that show drastic seasonal environmental changes. These studies specifically highlighted how non-photochemical quenching (NPQ) is a crucial physiological mechanism for the survival of polar diatoms at low temperature coupled with other stresses such as high light (including UV radiations) and salinity changes (Petrou et al., 2016).
NPQ has been recently recognized as a key player in global marine primary production (Lin et al., 2016). In diatoms, NPQ is composed of two major components (Lavaud and Goss, 2014; Goss and Lepetit, 2015): (1) qE, a high-energy state quenching component which is closely correlated to the activity of the xanthophyll cycle (XC), which involves a light-driven enzymatic conversion of the xanthophyll diadinoxanthin (Dd) to diatoxanthin (Dt), and 2) qI, an ill-defined quenching component which would be mainly related to photosystem II (PSII) photoinactivation and damage (i.e., photoinhibition). The distinction between these two components is often based on differences in relaxation kinetics in the lower light/dark subsequent to light exposure. qE relaxation is generally much faster (timescale of minutes) than qI relaxation (timescale of hours) (Goss and Lepetit, 2015). The nature of qI in diatoms has been re-examined because examples of sustained xanthophyll-related NPQ (NPQs, Wu et al., 2012) have been reported in diatoms exposed to prolonged excess irradiance (Lavaud and Lepetit, 2013) and/or sub-optimal temperatures (Wu et al., 2012), with similarity to its long-lasting observation in overwintering evergreen plants (Demmig-Adams et al., 2014; Verhoeven, 2014; Míguez et al., 2017). NPQs is sometimes referred to as “dark NPQ” (Perkins et al., 2011) since it remains present in darkness together with Dt, especially under harsh in situ conditions (Serôdio et al., 2012; Lavaud and Goss, 2014). In the Arctic Ocean, high concentrations of Dt are commonly found in diatom communities (Kashino et al., 2002; Ha et al., 2016) even after on-board incubation during 2 h under very low irradiance (Lacour et al., unpublished), suggesting the possible occurrence of a strong NPQs (Míguez et al., 2017).
In the present study, we aimed at understanding the physiological ability of T. gravida to acclimate to changing coupled temperature and light conditions. In order to do so, the growth rate, pigment composition, and several photosynthetic parameters were measured in cultures grown at four light intensities (10, 50, 80, and 400 μmol photons m−2 s−1) and at two temperatures (0 and 5°C). These conditions were chosen as representative of spring to summer transition changes as can be monitored in the natural habitat of T. gravida (Alou-Font et al., 2016; Fragoso et al., 2017; Hoppe et al., 2018), and the maxima (400 μmol photons m−2 s−1 and 5°C) are predicted foreseen average maxima in the upper layer of some parts of the Arctic Ocean (Thomas et al., 2012). We especially targeted specific processes (xanthophyll synthesis, PSII repair, NPQ) likely crucial for survival of polar microalgae in their extreme environment (Kropuenske et al., 2009, 2010; Arrigo et al., 2010; Mills et al., 2010; Petrou et al., 2010, 2011; Petrou and Ralph, 2011; van de Poll et al., 2011). NPQs was identified as a key photoacclimatory mechanism in T. gravida and probably in other polar diatoms.
Methods
Acclimation of Algal Cultures
Unialgal cultures of Thalassiosira gravida (CCMP986) were grown in semi-continuous cultures in pre-filtered f/2 medium (Guillard, 1975) enriched with silicate. Culture conditions were maintained semi-constant by diluting cultures with fresh medium once a day (Macintyre and Cullen, 2005), and gently aerated through 0.3 μm-pore-filters. Cell density was maintained at relatively low cell density (<106 cells mL−1) in order to keep them optically thin. The illumination was provided continuously by white fluorescent tubes at 10, 50, 80, and 400 μmol photons m−2 s−1 as measured with a QSL-100 quantum sensor (Biospherical Instruments, San Diego, CA, USA) placed in the culture vessel. Cultures were grown in a growth chamber that allowed maintenance of temperature at 0 or 5°C. Culture sampling was undertaken when cultures reached steady state (sensu Macintyre and Cullen, 2005) i.e., after cells were completely acclimated to the growth conditions after a minimum of 10 cell generations. We used daily measurements of the culture growth rate, cell diameter and chlorophyll a (Chl a) content (Table 1) to monitor the acclimation of the culture to the growth conditions (Lacour et al., 2012). Cultures in triplicate were sampled for measuring cell number, pigment composition, particulate organic carbon (C) and nitrogen (N), the rate of carbon fixation, and variable Chl a fluorescence. The irradiance at which cultures were acclimated is from hereafter called “growth irradiance,” while the irradiance used in assays is called “incubation irradiance.” Acclimated cultures were additionally incubated to perform three different experiments: a dark relaxation experiment, an inhibitor experiment, and a high-light shift experiment (see Table 2).
The relationship between the growth rate (μ, d−1) and growth irradiance (E, μmol photons m−2 s−1) was modeled by fitting to the data the following the equation suggested by Macintyre et al. (2002):
where μm is the maximum growth rate (d−1) and KE is the light saturation parameter for growth (μmol photons m−2 s−1). The relationship was fitted to the data (4 data points per growth temperature) by non-linear least-squares. At 0°C, the estimation of KE may be partially erroneous as, even at the lowest irradiance (10 μmol photons m−2 s−1); growth was not clearly light limited.
Cell Number, C and N Content
T. gravida cells were counted and sized (cell diameter) before and after culture dilution, using a Beckman Multisizer 4 Coulter Counter (Miami, US). The concentrations of particulate C and N were determined daily on triplicate samples. For each sample, an aliquot of 10 mL of algal culture was filtered onto glass-fiber filters (0.7 μm, 25 mm) pre-combusted at 500°C for 12 h. The filters were kept dry before elemental analysis with a CHN analyzer (2400 Series II CHNS/O; Perkin Elmer, Norwalk, CT, USA).
Pigment Analysis
For pigment analysis, an aliquot of algal culture (5 mL) was filtered onto GF/F glass-fiber filters (Whatman®), immediately flash-frozen in liquid nitrogen and stored at −80°C until analysis by High Performance Liquid Chromatography (HPLC). Pigments were extracted from the frozen filters by sonication in 2.5 mL of 95% methanol, cleared by centrifugation, and filtered with PTFE syringe filters (pore size 0.2 mm) into HPLC vials. The extracts were then put under argon and kept at 4°C in the dark in the HPLC autosampler to prevent pigment degradation. Shortly following extraction, 100 μL of pigment extracts were injected into a Thermo Scientific Accela 600 HPLC system equipped with a Thermo Scientific Hypersil Gold C8 column. The solvent protocol followed that of Zapata et al. (2000). Chlorophylls were detected by fluorescence (excitation 440 nm, emission 650 nm) and carotenoids by photodiode array (PDA) spectroscopy (350–750 nm) set to a slit width of 2 nm. Absorbance chromatograms were obtained at 450 nm for carotenoids. Standards for identification (based on PDA spectra and retention times) and quantification (using calibration coefficients) of pigments were obtained from Sigma Inc. (St Louis, MO, USA) (Chl a, β,β-carotene) and DHI Water & Environment (Hørsholm, Denmark) Chl c, diadinoxanthin, diatoxanthin, fucoxanthin) to calibrate the HPLC system.
The xanthophyll de-epoxidation state (DES in %) was calculated as Dt/(Dd + Dt)*100, where Dd is diadinoxanthin, the epoxidized form and Dt is diatoxanthin, the de-epoxidized form (Lavaud et al., 2007).
14C Experiments
The relationship between the rate of carbon fixation and irradiance was determined according to Lewis and Smith (1983). A 50-mL culture sample was collected in the three replicate cultures, and inoculated with inorganic 14C (NaH14CO3, 2 μCi mL−1 final concentration). To determine the total activity of added bicarbonate, three 20-μL aliquots of inoculated cultures were added to 50 μL of an organic base (ethanolamine) and 6 mL of the scintillation cocktail (EcoLumeTM, Costa Mesa, US) into glass scintillation vials. One milliliter aliquots of the inoculated culture sample were dispensed into twenty-eight 7 mL glass scintillation vials already cooled in their separate thermo-regulated alveoli (0 or 5°C). The vials were exposed to 28 different light levels provided by separate LEDs (LUXEON Rebel, Philips lumileds, USA) from the bottom of each alveolus. The PAR (Photosynthetic Active Radiation, μmol photons m−2 s−1) in each alveolus was measured before incubation with an irradiance quantum meter (Walz US-SQS + LI-COR LI-250A, USA) equipped with a 4π spherical collector. After 20 min of incubation, culture aliquots were “killed” with 50 μL of buffered formalin then acidified (250 μL of HCl 50%) under the fume hood for 3 h in order to remove the excess inorganic carbon (Knap et al., 1996). Finally, 6 mL of scintillation cocktail were added to each vial prior to counting in a liquid scintillation counter (Tri-Carb, PerkinElmer, Boston, USA). The Chl a-specific carbon fixation rate was finally computed according to Parsons et al. (1984). We fitted the data with the model of Platt et al. (1980) to obtain the light-saturated rate of photosynthesis and the initial slope of the PE curve normalized to Chl a ( and α*, respectively), to particulate organic carbon ( and αC) and to cell concentration ( and αCELL), and the light saturation parameter (EK) of the carbon fixation rate vs. incubation irradiance curve at each growth condition.
Active CHL a Fluorescence Measurements
Variable Chl a fluorescence measurements were performed using a Fluorescence Induction and Relaxation (FIRe) fluorometer (Satlantic, Halifax, NS, Canada) and using a Phyto-PAM (Pulse Amplitude Modulated) Fluorometer (Walz GmbH, Effeltrich, Germany). The FIRe was used to determine Fv/Fm and ΔF/Fm', and the Phyto-PAM was used to perform rapid light curves (RLCs) in order to estimate dynamic NPQ (NPQd). Both fluorometers apply a saturating pulse to the incubated sample and generates a fluorescence (detected at 680 nm) induction curve that can be used to estimate the minimum fluorescence (F0 if dark-acclimated), the steady-state fluorescence at light (FS) and the maximum fluorescence (Fm if dark-acclimated and Fm' if light-acclimated). F0, Fm, FS, Fm' were measured on culture subsamples that were dark-acclimated for 20 min.
We estimated the apparent maximum quantum yield of PSII (Fv/Fm) as follows (see Van Kooten and Snel, 1990):
Here, we call it “apparent” maximum Fv/Fm because it underestimates the actual maximum Fv/Fm, particularly at the highest irradiances due to a sustained NPQ (see just below and the Results section).
As mentionned by Demmig-Adams et al. (2014), the measurement of NPQ as the quenching of maximal fluorescence in the light (Fm′) relative to a control level of Fm after dark acclimation is straightforward when no dark/sustained NPQ is present, i.e., when the quenching of Fm is fully relaxed at the end of the dark period. In T. gravida, because of sustained NPQ, calculation of NPQ based on Fm after 20 min darkness underestimates NPQ. Therefore, we separated NPQ into 2 components, sustained and dynamic NPQ, respectively named NPQs and NPQd (Wu et al., 2012).
NPQs, the sustained part of NPQ, which was induced particularly at the highest growth irradiance, was estimated as follows:
where is Fm of cells incubated in the dark for 24 h (see “Dark relaxation experiment” below). Because in cells acclimated to 400 μmol photons m−2 s−1 NPQs was probably not fully relaxed after 24 h (see the Results section) NPQs was underestimated.
NPQd was calculated as follows:
It is important to note that when NPQs is high the calculation of NPQd without taking into account a relaxed Fm () can lead to a large underestimation of NPQd (see Figure S1). This is the case also when Fm24h is not fully relaxed, i.e., for 400 μmol photons m−2 s−1 cells.
1-qP (qP is the photochemical quenching of Chl a fluorescence) estimates the fraction of reduced quinone-QA and thus the reduction level of PSII reaction center; it illustrates the degree of excitation pressure on PSII: the higher 1-qP, the higher the excitation pressure (Büchel and Wilhelm, 1993; Materna et al., 2009):
With F0' computed as follow (Oxborough and Baker, 1997):
Targeted Experiments (Table 2)
High Light Shift Experiment
To document the contribution of PSII repair in the light-response of T. gravida at low temperatures, triplicates of cultures previously acclimated to 50 μmol photons m−2 s−1 at 0 and 5°C were incubated during 120 min at 400 μmol photons m−2 s−1 in the presence and absence of lincomycin (final concentration: 500 μg.mL−1), an inhibitor of plastid protein synthesis, and particularly of the D1 protein (PsbA) of the PSII reaction center (Wu et al., 2012; Lavaud et al., 2016). The apparent maximum quantum yield of PSII (Fv/Fm) was estimated from variable Chl a fluorescence after 15 min dark incubation following 0, 30, 60, and 120 min light exposure, to monitor PSII functionality with (-lincomycin) and without (+lincomycin) PSII repair.
Gradual Light Increase Experiment
To document the role of NPQ in the cell response to short term variation of irradiance, rapid light curves (RLCs) were measured on cultures using a Phyto-PAM with pre-installed software routine, where the actinic illumination was incremented in eight steps (90 s per step) (White and Critchley, 1999; Ralph and Gademann, 2005). Dynamic NPQ (NPQd) vs. incubation irradiance was calculated from Chl a fluorescence parameters. Dithiothreitol (DTT) is a well-known inhibitor of the de-epoxidase enzyme responsible for the de-epoxidation of Dd into Dt. It thus impairs both the xanthophyll cycle and NPQ development (Lavaud et al., 2002). Triplicates of cultures previously acclimated to 50 and 400 μmol photons m−2 s−1 at 0 and 5°C were treated with and without DTT and incubated 20 min in the dark before variable Chl a fluorescence measurements. DTT dissolved in pure water was added from a freshly prepared 50 mM stock solution to a final concentration of 500 μM, a concentration high enough to inhibit all Dd de-epoxidation (Lavaud et al., 2002). Note that it is not expected that such a short light treatment induced a strong NPQs (see Wu et al., 2012) but maybe for the highest intensity (2000 μmol photons m−2 s−1) although most probably the extent of NPQs remained negligible under such conditions.
Dark Acclimation Experiment
To document the relaxation kinetics of NPQ, we incubated in complete darkness during 24 h triplicates of cultures previously acclimated to 50 and 400 μmol photons m−2 s−1 (0°C). We monitored pigment composition (by HPLC) and Chl a fluorescence properties after 20 min, 3 h, 6 h and 24 h of dark incubation.
Statistical Tests
To test for differences between temperatures with regard to physiological characteristics we used a one-way ANCOVA model with irradiance as a covariate. The use of the covariate in the model allows a statistical control for the effects of irradiance to evaluate the temperature effect on the physiological parameters. Following a significant treatment effect, Tukey's multiple comparison method was used to compare temperatures. The normality assumption was verified using Shapiro–Wilk statistics. Data analyses were performed using the Sigma Plot 12.5. We also tested differences between means using t-test.
Results
Acclimation of Thalassiosira gravida to Growth Irradiance and Temperature
Growth Rate, C and N, Chl a Content
The growth rate of T. gravida was highly influenced by growth temperature (one-way ANCOVA, F = 44.464; P <10−5) with a maximum growth rate (μm) almost twice as high at 5°C (0.5 d−1) than at 0°C (0.27 d−1) (Figure 1A). In the present study, at 0°C, growth was almost saturated for the lowest growth irradiance, suggesting a light saturation parameter for growth (KE) below 10 μmol photons m−2 s−1. The C:N ratio of T. gravida (~6 mol mol−1) was below the Redfield ratio (6.62 mol mol−1; Figure 1B, gray dotted line, Redfield, 1934) and the mean one of temperate diatoms (7.3 ± 1.2 mol mol−1; Figure 1B, gray line, Sarthou et al., 2005). It was not significantly affected by growth irradiance and was significantly affected by growth temperature with higher values at 5°C (t-test, P = 0.008). The Chl a to carbon ratio (Chl a/C) was rather similar at both temperatures (one-way ANCOVA; F = 0.02; P = 0.90). It decreased with increasing growth irradiance (Figure 1C, see also Chl a/Cell in Figure S2). For the lowest growth irradiance (10 μmol photons m−2 s−1), Chl a/C was twice as high at 0°C. Chl a/C reached a similar value (~20 mg Chl a g−1 C) for irradiances of 50 and 80 μmol photons m−2 s−1 at 0°C and 5°C, respectively. Figure 1C also shows Chl a/C of temperate microalgae grown at 20°C as modeled by Geider (1987) and Cloern et al. (1995). Surprisingly, Chl a/C in T. gravida was to some extent in the range of temperate microalgae.
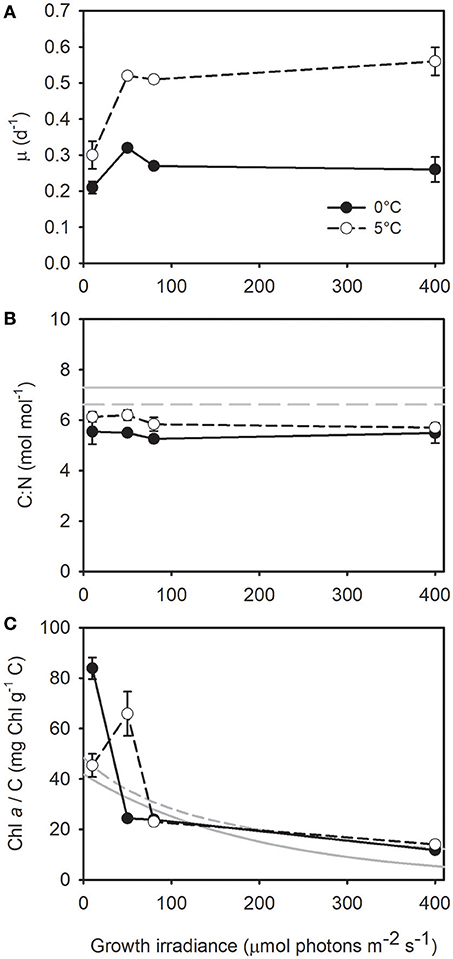
Figure 1. (A) Growth rate, (B) carbon to nitrogen ratio, and (C) Chl a to carbon ratio as a function of growth irradiance in Thalassiosira gravida cells acclimated to 0°C (closed circles) and 5°C (open circles). Each data point is the mean of 3 independent cultures, error bars represent standard deviations. In (B) the continuous gray line represent the mean C:N ratio in diatoms computed by Sarthou et al. (2005), and the dotted line represent the Redfield ratio (Redfield, 1934). In (C) the gray lines represent the relationship between Chl a/C and growth irradiance as modeled at 20°C by Cloern et al. (1995) (Chl a/C = 0.003 + 41.861 exp(-0.00509 E), continuous line) and Geider (1987) (Chl a/C = 1/(0.0206 + 0.00015 E), dashed line).
Pigment Content
Beyond the Chl a cell content, T. gravida also strongly modulated its “accessory” pigments Chl c and fucoxanthin contents as a function of growth irradiance and temperature. Noticeably, (1) the high fucoxanthin content (compared to temperate strains) at the lowest growth irradiance, i.e., there was as much fucoxanthin as Chl a, (2) the higher fucoxanthin content at 5°C vs. 0°C from 80 μmol photons m−2 s−1 on (i.e., irradiance for which the lowest Chl a/C was reached independent of growth temperature). The decrease in the content of Chl c and fucoxanthin with increasing growth irradiance was more drastic than the decline in Chl a, which resulted in a ~2-times decrease in the Chl c/Chl a and fucoxanthin/Chl a ratios at both temperatures (Figures 2A,B). At both temperatures, the (Dd+Dt)/Chl a, β-carotene/Chl a and Dt/Chl a increased linearly with increasing growth irradiance with a higher content in cells acclimated to 0°C (one-way ANCOVA, respectively F = 14; P < 10−3; F = 8.71; P < 10−2 and F = 9.68; P < 10−2), especially at the highest growth irradiance (Figures 2C,D,F). Strikingly, cells acclimated to 0°C and 400 μmol photons m−2 s−1 got even more Dd+Dt than Chl a molecules (~120 mol Dd+Dt 100 mol Chl a−1, Figure 2C), the same was true for fucoxanthin in cells acclimated at 10 μmol photons m−2 s−1 independent of the growth temperature (~105 mol fucoxanthin 100 mol Chl a−1, Figure 2B). The de-epoxidation ratio (DES = Dt/(Dd +Dt)) increased with growth irradiance but was only slightly affected by growth temperature (one-way ANCOVA, F = 4.85; P = 0.04) (Figure 2E).
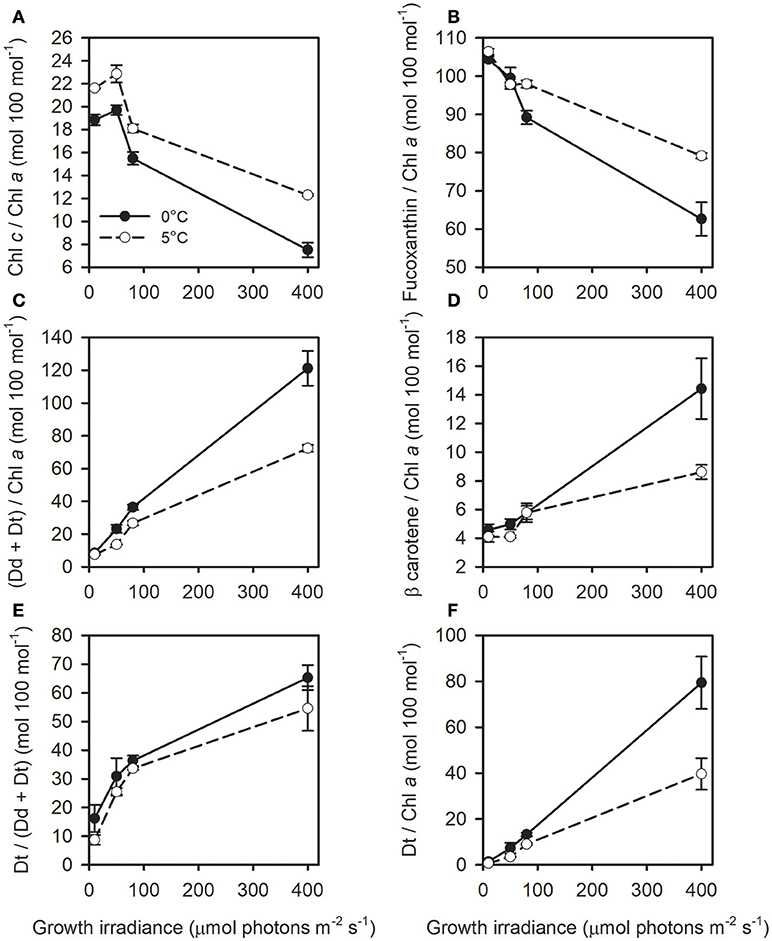
Figure 2. (A) Chl c/Chl a, (B) Fucoxanthin/Chl a, (C) (Dd+Dt)/Chl a, (D) β-carotene/Chl a, (E) de-expoxidation ratio (DES = Dt/(Dd+Dt)x100), and (F) Dt/Chl a as a function of growth irradiance in T. gravida cells acclimated to 0°C (closed circles) and 5°C (open circles). All pigments ratios (but Dt/(Dd+Dt)) are expressed in moles relative to 100 mol Chl a. See Table 1 for parameters definition. Each data point is the mean of three independent cultures, error bars represent standard deviations.
Photosynthetic Parameters
We observed no significant differences in values between 0 and 5°C (one-way ANCOVA, F = 4.04; P = 0.06) and a similar ~3 fold drop down from the lowest growth irradiance to 80 μmol photons m−2 s−1 (Figure 3A). A similar decrease was also observed for although with a lower magnitude, especially at 5°C (Figure S3). The Chl a-specific initial slope of the photosynthesis-irradiance curve (α*) is a measure of the photosynthetic efficiency at low incubation irradiance. We observed a large decrease in α* (and also αC and αCELL) from the lowest to the highest growth irradiance (Figure 3B and Figure S3). EK, the light saturation parameter for photosynthesis, increased linearly with growth irradiance with no difference between 0 and 5°C (one-way ANCOVA, F = 0.10; P = 0.74), except at 400 μmol photons m−2 s−1 (higher EK at 0°C, Figure 3C). In comparison to temperate diatoms grown at higher temperatures (gray line in Figure 3C; Lacour et al. (2017) and for a given growth irradiance, saturation of photosynthesis occurs at much lower intensity in T. gravida (i.e., lower EK). Carbon fixation rate (P expressed in d−1) at the growth irradiance was very similar to the cell growth rate (μ in d−1) (Figure S4). The apparent maximum quantum yield of PSII photochemistry (Fv/Fm) decreased with increasing growth irradiance, particularly at 0°C (~50% decrease, Figure 3D, one-way ANCOVA, F = 18.24; P < 10−3).
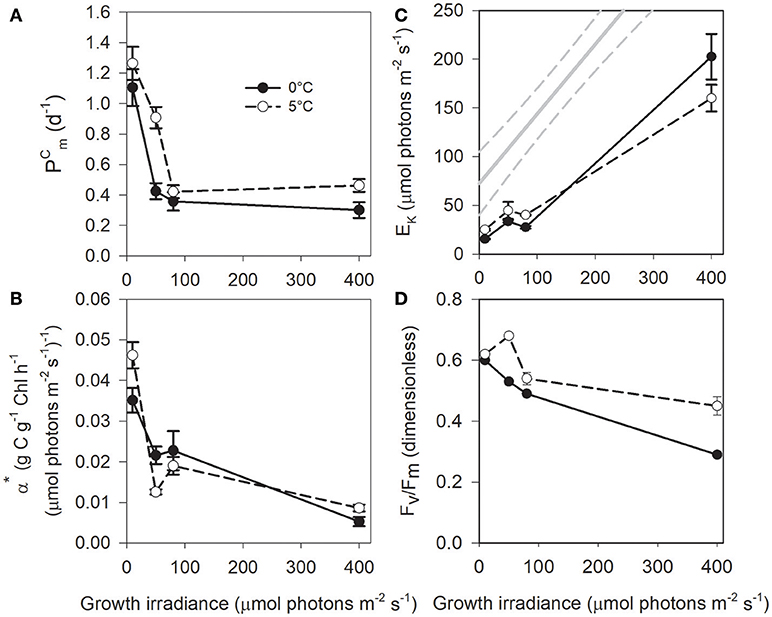
Figure 3. (A) , (B) α*, (C) EK, and (D) Fv/Fm as a function of growth irradiance in T. gravida cells acclimated to 0°C (closed circles) and 5°C (open circles). See Table 1 for parameters definition. Each data point is the mean of 3 independent cultures, error bars represent standard deviations. In (C), the continuous gray line represents the relationship between EK and growth irradiance in temperate diatoms as computed by Lacour et al. (2017), 95% confidence bands are plotted as dotted lines.
Short Term Light-Response of T. gravida Acclimated to Low Temperatures (see Table 2)
PSII Repair
To test the contribution of PSII repair in the light-response of T. gravida at low temperatures, cells acclimated at 0 and 5°C were shifted for 2 h from moderate (50 μmol photons m−2 s−1) to high irradiance (400 μmol photons m−2 s−1) with and without lincomycin, an inhibitor of the synthesis of PSII reaction center D1 protein (see Campbell and Tyystjarvi, 2012). In all treatments (Figure 4), PSII functionality (as measured by Fv/Fm) dramatically decreased, especially during the first 30 min of light exposure (~-50%). While, this decrease is usually related to the degradation of the D1 protein, we cannot exclude that part of it could be due to the induction of a sustained NPQ (NPQs, see below).While lincomycin had no effect at 0°C, at 5°C, Fv/Fm in cells treated with lincomycin showed stronger PSII damage (+15–20%) after 2 h of high light exposure, reaching the level of cells acclimated at 0°C (i.e., Fv/Fm ~0.18, t-test; P < 10−3).
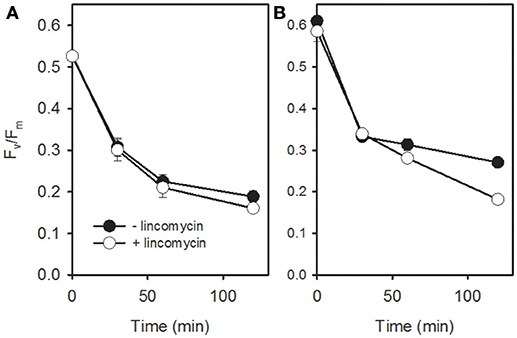
Figure 4. Apparent maximum quantum yield (Fv/Fm) in cells of T. gravida acclimated to 0°C (A) and 5°C (B) during a 120 min shift from 50 μmol photons m−2 s−1 to 400 μmol photons m−2 s−1 in the presence (open circles) and absence (closed circles) of lincomycin. Each data point is the mean of 3 independent cultures, error bars represent standard deviations.
Photochemistry and Induction of the Dynamic NPQ (NPQd)
The fraction of reduced QA (1-qP) was lower at 5°C than at 0°C in cells acclimated to 50 μmol photons m−2 s−1, a difference that was partly abolished by the addition of DTT (Figure S5). When cells were acclimated to 400 μmol photons m−2 s−1, no difference in 1-qP was found for the different treatments (Figure S5). Concomitantly, 0°C-grown cells acclimated to 50 μmol photons m−2 s−1 showed a much higher NPQd than cells acclimated to 400 μmol photons m−2 s−1 (Figure 5). Nearly all NPQd was related to the presence of Dt synthesized from Dd de-epoxidation as shown by the quasi total inhibition of NPQd by DTT (Figure 5).
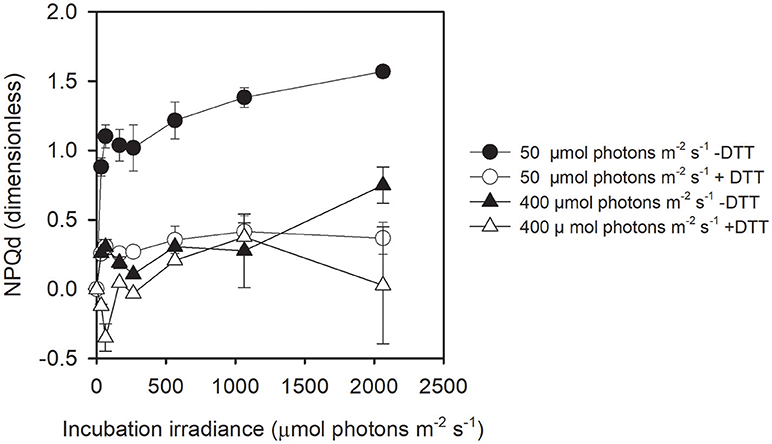
Figure 5. Dynamic NPQ (NPQd) as a function of incubation irradiance in cells acclimated to 50 μmol photons m−2 s−1 and 400 μmol photons m−2 s−1 at 0°C with and without DTT. Note that NPQd is not available at 5°C. Each data point is the mean of three independent cultures; error bars represent standard deviations.
Relaxation Kinetics of the Sustained (NPQs): Impact on Fv/Fm and Role of Dt
Because we suspected the presence of a sustained NPQ (NPQs), i.e., even after 20 min of dark acclimation, the Chl a fluorescence would still be quenched (see Perkins et al., 2011), we proceeded to a 24 h dark incubation of cells acclimated to 0°C at 400 and 50 μmol photons m−2 s−1 (see Table 2). As expected, NPQs was high in cells acclimated to 400 μmol photons m−2 s−1 and it slowly relaxed during the 24 h dark incubation (Figure 6A). In parallel, Fv/Fm restored 50% of its value (Figure 6B), taking hours of dark relaxation instead of the tens of minutes usually reported in temperate diatom species. NPQs was much lower in cells acclimated to 50 μmol photons m−2 s−1 inducing a small, but existing, underestimation of Fv/Fm (Figures 6A,B). In parallel to NPQs relaxation, there was a concomitant decrease in Dt due to the slow epoxidation back to Dd (Figures 6C,D). When NPQs is plotted against Dt content (Figure 6E), it became obvious that its extent mainly, if not completely, depended on Dt content at both growth irradiances, with the main difference being that for cells acclimated at 400 μmol photons m−2 s−1 there was a high amount of Dt (~28 mol 100 mol Chl a−1) remaining after 24 h darkness (Figure 6D). A strong linear relationship was also true between Fv/Fm and Dt (R2 = 0.96; Figure 6F) and between Fv/Fm and NPQs in cells acclimated to 50 μmol photons m−2 s−1 (R2 = 0.88; Figure S6); in cells acclimated to 400 μmol photons m−2 s−1 with a stronger NPQs, the relationship deviated from linearity for values of NPQs above ca. 2.5 (Figure S6).While in cells acclimated to 50 μmol photons m−2 s−1, the progressive relaxation of NPQs in the dark did not strongly infer on NPQd extent (Figure 6G), it was different for cells acclimated to 400 μmol photons m−2 s−1 (Figure 6H) where NPQd extent showed a large increase over the first 6 h of dark relaxation, followed by a decrease after 24 h.
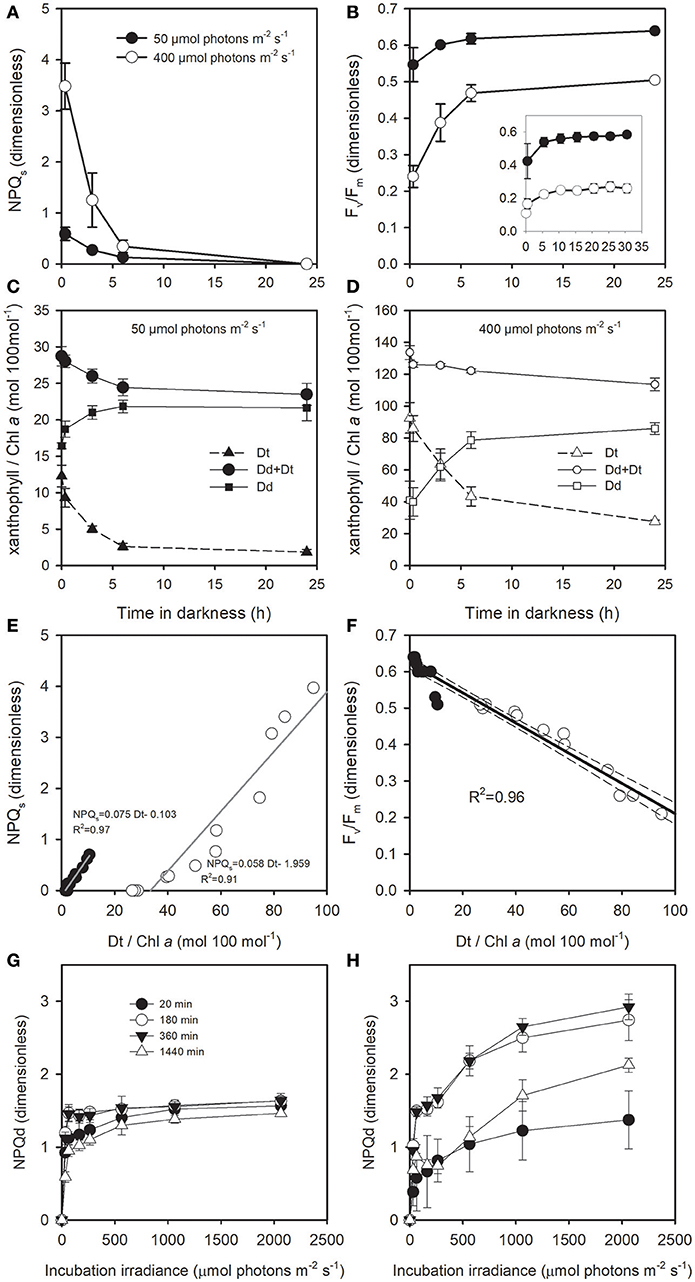
Figure 6. Darkness time dependent change (A) in the sustained NPQ (NPQs), (B) in Fv/Fm, and in Dd and Dt content of T. gravida cells acclimated to 0°C and to growth irradiances (C) 50 μmol photons m−2 s−1 and (D) 400 μmol photons m−2 s−1. (E,F): relationship between Dt/Chl a and NPQs, and Fv/Fm, respectively; data are from (A–D). In (A,B,E,F), closed and open symbols represent cells acclimated to 50 and 400 μmol photons m−2 s−1, respectively. NPQd as a function of incubation irradiance in cell previously acclimated to (G) 50 μmol photons m−2 s−1 and (H) 400 μmol photons m−2 s−1 and after 20, 180, 360, and 1140 min dark acclimation. Each data point is the mean of 3 independent cultures, error bars represent standard deviations.
Discussion
In order to better understand the physiological fitness of T. gravida at the basis of its ecological success, we grew it at different light intensities and temperatures typically found during spring in the surface of Arctic Ocean. As expected, T. gravida showed a μ (see Table 2 for the definition of all symbols) that saturated at light intensities much lower (i.e., KE below 10 μmol photons m−2 s−1) than those reported for temperate diatoms (Gilstad and Sakshaug, 1990; Lacour et al., 2017). This feature was already reported before for polar and sea-ice diatoms (Thomas et al., 2012), although here at 0°C, μm was low even compared to the mean growth rate of polar species (0.27 d−1 vs. 0.44 d−1; Lacour et al., 2017). Since μm was twice as high at 5°C, it was obviously temperature limited at 0°C. T. gravida proved to be able to grow at least at up to 400 μmol photons m−2 s−1 even at 0°C. Two conclusions can be drawn from these observations: (1) the slighest increase in temperature, especially over 0°C, can boost T. gravida growth, (2) even when growth is temperature limited, T. gravida can cope with irradiance that is largely over-saturating for both its photosynthetic capacity and growth (above 50 × KE). We further explored how T. gravida manages such performance.
T. gravida Acclimatory Light Energy Utilization
Low μm can be explained by the limitation of C fixation (Calvin cycle) that is thought to be the growth-limiting step at low temperature (Young et al., 2015), likely due to rate limitation of many enzymes, and especially Rubisco. This situation is usually counterbalanced by (1) a dramatic increase in enzymes (and thus protein) concentration (Lyon and Mock, 2014), especially Rubisco (Young et al., 2015), (2) the use of most organic carbon for growth at the expense of the accumulation of carbon reserves, both generating a low C:N ratio as observed here. The fact that μ was very similar to at high irradiance, and that it occurred for a lower irradiance at 0°C than at 5°C, strengthens the conclusion that μm was limited by C fixation over a certain irradiance (between 50 and 80 μmol photons m−2 s−1), and that this threshold was reached earlier at 0°C (because growth saturated for a lower irradiance). It also implies that the capacity to exploit a sudden increase in irradiance above the mean growth irradiance (Kana and Glibert, 1987; Cullen and Macintyre, 1998) was drasticly reduced at over-saturating growth irradiance. The decrease of with growth irradiance is very unusual in eukaryotic microalgae (Macintyre et al., 2002). At low temperature (i.e., 0°C), many processes allowing microalgae to optimize photosynthesis at moderate/high irradiance may be limited by the total amount of proteins available. Indeed, the need for proteins associated with, for instance, photoprotection (e.g., see below, NPQ, PSII repair cycle, alternative electron cycles) (Wagner et al., 2006; Halsey and Jones, 2015), may lead to a reduction of resources that are available to maintain or increase Rubisco content to balance the low catalitic rate at 0°C. When temperature was higher (5°C), this pressure was partially leveraged, and the irradiance threshold was shifted toward higher values, i.e., + 30 μmol photons m−2 s−1. Although such low irradiance difference for a +5°C increase might not seem large, for such very low-light adapted strain like Arctic diatoms, this is physiologically relevant.
The rate of light absorption (and thus the amount of light harvesting pigments) is a major determinant of light- and temperature-limited photosynthesis (Macintyre et al., 2002). It is well-known that microalgae show lower Chl a content as temperature decreases (Yoder, 1979; Verity, 1982; Geider, 1987) which helps decreasing the excitation pressure on the photosynthetic apparatus, especially in conditions where growth capacity is limited (like cold for instance) (Halsey and Jones, 2015). Interestingly, Chl a/C in T. gravida was in the range of temperate microalgae, which nevertheless have much higher growth rates (Lacour et al., 2017). It suggests that at low temperature, in T. gravida, a smaller part of the absorbed light energy was directed to growth. The same holds true when comparing T. gravida cells grown at 0 vs. 5°C (i.e., rather similar Chl a/C but very different μ). These findings illustrate a refined balance in ressource allocation strategies (together with photoprotection, see below and Talmy et al., 2013) as a function of irradiance and temperature (Halsey and Jones, 2015), which is probably central in enabling T. gravida to maintain growth under such differential environmental conditions.
T. gravida PSII Repair During Excess Light Exposure
At 0°C, the repair cycle of photo-inactivated/-damaged PSII reaction centers during excess light exposure by synthesis and replacement of D1 (PsbA) protein was not significant. Similar data were obtained in Fragilariopsis cylindrus at 2°C (Kropuenske et al., 2009). Petrou et al. (2010) showed that in sea-ice diatoms PSII repair rates are slower compared to temperate diatoms. This is to be expected because lower temperatures slow down enzyme kinetics and metabolic processes (Morgan-Kiss et al., 2006). This is supported by the fact that at 5°C, PSII repair was significant, showing that PSII repair indeed takes place at “higher” temperatures (also reported in Petrou et al., 2010). This finding is of paramount importance as in the temperate counterpart of T. gravida, T. pseudonana (Wu et al., 2012), NPQs induction and extent is directly related to clearance and replacement of damaged PsbA, i.e., NPQs accumulates when PSII photoinactivation outruns PsbA repair. Hence, the lack of PSII repair at 0°C most probably explains the high NPQs level under 400 μmol photons m−2 s−1 acclimation. This is consistent with previous reports (Kropuenske et al., 2009; Petrou et al., 2010, 2011; Petrou and Ralph, 2011; van de Poll et al., 2011) suggesting that polar diatoms seem to preferentially rely on the XC and NPQ to reduce the excitation pressure on PSII, which consequently reduces the need for a high rate of repair at ca. 0°C to keep pace with photoinactivation and damage, as shown in temperate strains (Wu et al., 2011, 2012; Lavaud et al., 2016). This was best illustrated by the higher reduction level of QA (1-qP) in cells exhibiting lower NPQd (+vs. – DTT) as well as lower NPQs (50 vs. 400 μmol photons m−2 s−1) (see below).
Light and Dark T. gravida Xanthophyll Content
Dd and Dt contents vs. irradiance reflected an increased need for photoprotection with a ~2x higher content at 0°C and a Dd+Dt content even higher than Chl a for the highest growth irradiance. Similar high values were reported in F. cylindrus (Kropuenske et al., 2009) and Chaetoceros brevis (van de Poll et al., 2011), as well as in sea-ice diatom communities (Robinson et al., 1997) especially at low snow sites during post-bloom period (Alou-Font et al., 2013), and for phytoplankton in Arctic waters (Ha et al., 2016). This feature is strongly different from temperate strains where carotenoid synthesis vs. increasing irradiance is much more moderate independent of the species (Willemoës and Monas, 1991; Goericke and Welschmeyer, 1992; Mouget et al., 1999; Anning et al., 2000; Schumann et al., 2007). The 0 vs. 5°C difference in Dd+Dt can be explained by the higher excitation pressure on PSII at 0°C leading to the sequential build-up of a more reduced plastoquinone (PQ) and quinone pools as shown here (see Figure S5) and before in F. cylindrus (Mock and Hoch, 2005). PQ redox state indeed modulates the Dd+Dt synthesis, i.e., the more reduced is PQ, the higher the Dd+Dt content (Lepetit et al., 2013).
With a DES reaching up to 60%, the activity of the de-epoxidase enzyme responsible for the conversion of Dd to Dt was not restricted by low temperature as reported in Arctic waters (Kashino et al., 2002; Ha et al., 2016), and in overwintering land plants, such as evergreen conifers, and alpine plants that face season-long subfreezing temperatures (Demmig-Adams et al., 1999; Streb and Cornic, 2012). This is opposite to the very slow (hour kinetics) dark epoxidation of Dt back to Dd which was even incomplete after 24 h darkness. This is in accordance with previous reports on temperate diatom strains (Goss et al., 2006; Lavaud and Lepetit, 2013) as well as sea-ice diatom communities (Robinson et al., 1997). In diatoms the epoxidase enzyme (DEP) responsible for the XC back conversion is strongly controlled by both the thylakoid proton gradient (ΔpH) and by the NADPH, H+ co-factor availability (Lavaud and Goss, 2014). Inhibition of DEP by high ΔpH in darkness can be caused by extensive energetic exchanges between plastids and mitochondria (Bailleul et al., 2015). NADPH, H+ shortage can be due to its use by the transitionally on-going Calvin Cycle during a sudden shift from high light to darkness (Lavaud and Lepetit, 2013; Lepetit et al., 2013) and by the above mentionned NADPH/ATP exchanges between platids and mitochondria (Bailleul et al., 2015). The pattern of Dt epoxidation was similar in cells acclimated to 50 and 400 μmol photons m−2 s−1 and mostly the 7–8x higher concentration of Dt at the beginning of the dark period apparently accounted for the incomplete Dt epoxidation in cells acclimated to 400 μmol photons m−2 s−1. In this case, another, and not exclusive possibility to the above explanation, is the presence of a pool of Dt in the lipid matrix of the thylakoids that does not participate to NPQ (Schumann et al., 2007; Lavaud and Lepetit, 2013) but instead was proposed to prevent lipid peroxidation by the direct scavenging of reactive O2 species (Lepetit et al., 2010).
Two NPQ Components in T. gravida
The above described differences in temperature and irradiance driven Dd+Dt content and kinetics are of importance as they directly define the extent and kinetics of the photoprotective NPQ. Constitutive Dt was involved in a strong NPQs, especially in T. gravida cells acclimated at 0°C-400 μmol photons m−2 s−1. In these cells, NPQs reached up to 4 corresponding to heat dissipation of ~50–60% (Hendrickson et al., 2004). When cells were suddendly exposed to an increasing light gradient, NPQd developed due to additional Dt synthesized from Dd de-epoxidation (as proven by DTT effect). NPQd was higher in cells acclimated to 50 μmol photons m−2 s−1 (i.e., with the lowest NPQs), and its potential increased concurrently to NPQs relaxation during the first 6 h of prolonged dark acclimation in cells acclimated to 400 μmol photons m−2 s−1. This is in agreement with the observations reported in T. pseudonana (Wu et al., 2012), although here the range of temperatures for which it occurs is ca. 10°C lower. The role of Dt in the molecular mechanism of both NPQd and NPQs has been recently proposed (Chukhutsina et al., 2014; Lavaud and Goss, 2014; Goss and Lepetit, 2015). As regards to NPQs in diatoms, it is believed that it partly originates from the retention of Dt (due to a slow expoxidation back to Dd) which would keep the LHC system in a dissipative state. More specifically, the part of the LHC antenna that energetically uncouples from PSII during the light-driven NPQ induction, and which serves as excess energy sink, would not be able to reattach as long as Dt is present in the LHC of PSII. Although LHCx proteins were not quantified in this study, some isoforms are suspected to play a central role in NPQs (Zhu and Green, 2010; Wu et al., 2012; Lepetit et al., 2013).
The strong relationships between Fv/Fm, Dt and NPQs were noticeably very similar to the relationships reported in overwintering evergreen land plants (See Figure S6B and Verhoeven et al., 1996). It highlights the crucial role of Dt and NPQs in the sustained depression of Fv/Fm at 0°C, exactly as in evergreens for zeaxanthin and antheraxanthin pigments and NPQs (Verhoeven et al., 1996; Verhoeven, 2014). In evergreens, a recent mechanistic model has been proposed based on the distinction between fastly (qE), slowly (qZ), and very slowly (qI) reversible phases of NPQ and NPQs (Verhoeven, 2014). Although the reversal of NPQs in T. gravida shows striking similarity (Figure 6B vs. Figure 1 in Verhoeven, 2014) with two apparent rapid and slow components, (i) environmental conditions are very different, i.e., warming and/or low light in plants vs. 0°C in darkness in T. gravida, and, (ii) as for qE regulation, the same mechanistic scheme cannot directly apply in diatoms mainly due to the mandatory role of Dt in the overall NPQ compared to zeaxanthin in plants (Lavaud and Goss, 2014; Goss and Lepetit, 2015).
The Possible Role of NPQs in the Ecophysiology of Arctic Diatoms
The role of NPQd in the ecophysiology of diatoms has been described elsewhere (Lavaud and Goss, 2014). What is the specific role for NPQs? This is so far scarcely documented. In land plants where it has been extensively studied, NPQs occurs during times when there is no net carbon fixation, as for example during periods with subfreezing temperatures (Demmig-Adams et al., 2014; Verhoeven, 2014). The phenomenon is particularly important in conifers that maintain green needles in a highly dissipative state rather than decreasing their overall harvesting capacity (by dropping their needles or decreasing their Chl a content). The benefit for maintaining their excessive harvesting capacity was proposed to be the ability to resume growth relatively promptly when conditions become favorable uppon warming (Demmig-Adams et al., 2014). Similarly, T. gravida was able to maintain a high capacity to harvest light energy (i.e., high Chl a/C) even when growing at over-saturating irradiance and 0°C. NPQs is, by definition, locked-in, and cannot be rapidly relaxed in case of sudden light intensity decrease. The major consequence of a large persistent and unflexible level of energy dissipation is a decrease in both the maximum and the apparent quantum yields of PSII photochemistry, i.e., a lower proportion of the excessive absorbed energy is directed to photochemistry. This is illustrated in Figure 3D, by the decrease in Fv/Fm with growth irradiance and by the lower Fv/Fm measured at 0°C that were probably partly due to NPQs. Another major consequence was a decrease in the Chl a-specific initial slope of the P vs. E curve (α*) with increasing irradiance (Figure 3B), which is not a common feature of microalgae photoacclimation (Macintyre et al., 2002). Cells can thus maintain excessive light harvesting capacity under very unfavorable environmental conditions without suffering the consequences of an excessive excitation pressure on PSII (i.e., μ remains high). NPQs also lead to a decrease in NPQd as observed here for T. gravida cells acclimated to 400 μmol photons m−2 s−1 (Figure 5). Consequently, cells with a high NPQs are likely less efficient in exploiting short term decreases in irradiance, and in general irradiance fluctuations (Lavaud et al., 2007), as reported in overwintering evergreen land plants (Verhoeven, 2014). These features fit well with the strategy of a “layer former” (in contrast to a “mixer” strategy) as was reported for F. cylindrus (Kropuenske et al., 2009, 2010; Arrigo et al., 2010), i.e., adapted to both the stable low irradiance sea-ice and/or underneath the ice habitat, and to the high irradiance environment of shallow mixed layers associated with melting-ice waters or stratified upper mixed layer.
Another hypothetical role for NPQs could be the additional production of heat at sub-microscale in the plastids. Thermo-optic changes in the photosynthetic machinery generated by local heat dissipation were shown in land plants (Garab, 2014). Such heating process at sub-0°C could also aim at increasing the reaction rate of enzymes whose activity is highly sensitive to temperature, such as, for instance, Rubisco. Recently, new tools have evidenced endogenous thermogenesis and subcellular temperature gradients, although these methods have been criticized (Baffou et al., 2014). Interestingly, at larger scale, when sea-ice diatom biomass is high at the bottom of the ice horizon in early spring, it was suggested that the heat dissipated by photosynthesis could possibly generate local ice melting (Zeebe et al., 1996; Lavoie et al., 2005).
Concluding Remarks
This report illustrates how the Arctic diatom T. gravida is able to withstand large variations in irradiance and temperature due to the seasonal and on-going climate change modifications of its natural habitat. The strategy of T. gravida is supported by a strong photoacclimatory ability. This is related to a fine-tuned balance between NPQd and NPQs and surely other photoacclimatory processes, supporting a likely in-depth rearrangement of the photosynthetic machinery, similar to the scheme proposed in overwintering evergreen land plants (Demmig-Adams et al., 2008). More precisely, at over-saturating irradiance, Dt-related NPQ allows sufficient protection of the cells over increasing light gradients (NPQd) or stable excess light conditions (NPQs). Nevertheless, the relatively slow relaxation kinetics of NPQs impairs the cell ability to cope with sudden and deep light fluctuations (for instance induced by a strong mixing of the water column). T. gravida photosynthesis thus appears more efficient at moderately low (up to ~50 μmol photons m−2 s−1) and rather stable irradiance. Nevertheless, it also performs well at over-saturating irradiances (at least up to 400 μmol photons m−2 s−1) and it maintains its growth rate. This light range corresponds to the in situ conditions under which it predominates in the Arctic, i.e., underneath the spring thin-ice punctuated with melting pounds through which light transmittance is much higher (Arrigo et al., 2012) as well as in more open waters (Booth et al., 2002). Strikingly, this is similar to the “layer former” strategy proposed for F. cylindricus in Antarctica (Kropuenske et al., 2009, 2010). The balance between NPQd and NPQs (and Chl a/C) illustrates well how T. gravida, and likely other polar diatoms (F. cylindrus, Chaetoceros sp. Kropuenske et al., 2009; van de Poll et al., 2011), as well as cold-adapted Chlorophytes (La Rocca et al., 2015), are able, as a function of, at least, temperature and light (intensity and duration of exposure) to finely modulate their acclimatory strategy which can ultimately define their persistence/proliferation in a given habitat during the polar seasonal productive species succession (Kropuenske et al., 2009; Petrou and Ralph, 2011). Interestingly, it has been recently suggested that the evolution of psychrophily in polar microalgae must be tightly related with their adaptive ability to acclimate to coupled low temperatures and light climate (Lyon and Mock, 2014; Cvetkovska et al., 2017) such as reported for overwintering evergreen land plants (i.e., “photostasis,” Öquist and Huner, 2003).
The role of NPQ as a crucial functional trait defining the niche distribution of ecotypes (Bailleul et al., 2010), species (Lavaud and Lepetit, 2013), and growth forms (Barnett et al., 2015) is of growing interest. In polar diatoms, Ralph and co-workers (Petrou and Ralph, 2011; Petrou et al., 2011; Sackett et al., 2013) demonstrated how NPQd, and in general fast regulatory responses, likely support differential photoadaptative strategies that define, in part, ecological niche adaptation, and species distribution along the strong seasonal polar habitat changes (i.e., from winter-ice covered waters to summer-free water column via spring-meltwaters). Such differential phenotypic plasticity to light, temperature, and other environmental cues, among diatom species (and other groups, i.e., Phaeocystis sp. Kropuenske et al., 2009, 2010; Mills et al., 2010; van de Poll et al., 2011) is probably essential in defining the onset and fate of spring phytoplankton bloom in Arctic waters. Likely the balance of NPQs vs. NPQd is an important feature polar diatoms rely on in order to maintain growth rate under different light climates, a strategy that needs to be further examined in different life forms of polar strains (sea-ice vs. planktonic, bloomer vs. persistent, etc.).
As regards to the impact of higher temperature, we observed a lower excitation pressure on PSII and a stronger PSII repair leading to a higher maximal quantum yield of PSII (higher Fv/Fm), as compared to 0°C. Ultimately, the growth rate was higher (at least up to 5°C) probably thanks to a higher general metabolism (i.e., higher carbon fixation per cell). Hence, in the future warmer Arctic Ocean, T. gravida response to its brigther light environment may not be impaired and its predominance, at least among diatoms, might even increase together with the frequency and extent of sub-ice blooms (Arrigo et al., 2012; Horvat et al., 2017).
Data Availability Statement
All relevant data is contained within the manuscript: All datasets generated for this study are included in the manuscript and the Supplementary Files.
Author Contributions
TL and MB planned and designed the research. TL, JaL, JF, and FB performed experiments. All the authors analyzed and interpreted data and contributed to the writing of the manuscript.
Conflict of Interest Statement
The authors declare that the research was conducted in the absence of any commercial or financial relationships that could be construed as a potential conflict of interest.
Acknowledgments
We thank the joint contribution to the research programs of UMI Takuvik CNRS-Université Laval, ArcticNet (Network Centre of Excellence of Canada), the Canada Excellence Research Chair in Remote Sensing of Canada's New Arctic Frontier, and the Canada Research Chair program.
Supplementary Material
The Supplementary Material for this article can be found online at: https://www.frontiersin.org/articles/10.3389/fmars.2018.00354/full#supplementary-material
References
Alou-Font, E., Mundy, C., Roy, S., Gosselin, M., and Agusti, S. (2013). Snow cover affects ice algal pigment composition in the coastal Arctic Ocean during spring. Mar. Ecol. Prog. Ser. 474, 89–104. doi: 10.3354/meps10107
Alou-Font, E., Roy, S., Agustí, S., and Gosselin, M. (2016). Cell viability, pigments and photosynthetic performance of Arctic phytoplankton in contrasting ice-covered and open-water conditions during the spring-summer transition. Mar. Ecol. Prog. Ser. 543, 89–106. doi: 10.3354/meps11562
Anning, T., Macintyre, H. L., Pratt, S. M., Sammes, P. J., Gibb, S., and Geider, R. J. (2000). Photoacclimation in the marine diatom Skeletonema costatum. Limnol. Oceanogr. 45, 1807–1817. doi: 10.4319/lo.2000.45.8.1807
Arrigo, K. R., Mills, M. M., Kropuenske, L. R., Van Dijken, G. L., Alderkamp, A. C., and Robinson, D. H. (2010). Photophysiology in two major southern ocean phytoplankton taxa: photosynthesis and growth of Phaeocystis antarctica and Fragilariopsis cylindrus under different irradiance levels. Integr. Comp. Biol. 50, 950–966. doi: 10.1093/icb/icq021
Arrigo, K. R., Perovich, D. K., Pickart, R. S., Brown, Z. W., Van Dijken, G. L., Lowry, K. E., et al. (2012). Massive phytoplankton blooms under Arctic sea ice. Science 336:1408. doi: 10.1126/science.1215065
Assmy, P., Fernández-Méndez, M., Duarte, P., Meyer, A., Randelhoff, A., Mundy, C. J., et al. (2017). Leads in Arctic pack ice enable early phytoplankton blooms below snow-covered sea ice. Sci. Rep. 7:40850. doi: 10.1038/srep40850
Baffou, G., Rigneault, H., Marguet, D., and Jullien, L. (2014). A critique of methods for temperature imaging in single cells. Nat. Methods 11, 899–901. doi: 10.1038/nmeth.3073
Bailleul, B., Berne, N., Murik, O., Petroutsos, D., Prihoda, J., Tanaka, A., et al. (2015). Energetic coupling between plastids and mitochondria drives CO2 assimilation in diatoms. Nature 524, 366–369. doi: 10.1038/nature14599
Bailleul, B., Rogato, A., De Martino, A., Coesel, S., Cardol, P., Bowler, C., et al. (2010). An atypical member of the light-harvesting complex stress-related protein family modulates diatom responses to light. Proc. Natl. Acad. Sci.U.S.A. 107, 18214–18219. doi: 10.1073/pnas.1007703107
Barnett, A., Meleder, V., Blommaert, L., Lepetit, B., Gaudin, P., Vyverman, W., et al. (2015). Growth form defines physiological photoprotective capacity in intertidal benthic diatoms. ISME J. 9, 32–45. doi: 10.1038/ismej.2014.105
Blais, M., Ardyna, M., Gosselin, M., Dumont, D., Bélanger, S., Tremblay, J.-É., et al. (2017). Contrasting interannual changes in phytoplankton productivity and community structure in the coastal Canadian Arctic Ocean. Limnol. Oceanogr. 62, 2480–2497. doi: 10.1002/lno.10581
Booth, B. C., Larouche, P., Bélanger, S., Klein, B., Amiel, D., and Mei, Z. P. (2002). Dynamics of Chaetoceros socialis blooms in the North Water. Deep Sea Res. Part II Topical Stud. Oceanogr. 49, 5003–5025. doi: 10.1016/S0967-0645(02)00175-3
Büchel, C., and Wilhelm, C. (1993). In vivo analysis of slow chlorophyll fluorescence induction kinetics in algae: progress, problems and perspectives. Photochem. Photobiol. 58, 137–148. doi: 10.1111/j.1751-1097.1993.tb04915.x
Campbell, D. A., and Tyystjarvi, E. (2012). Parameterization of photosystem II photoinactivation and repair. Biochim. Biophys. Acta 1817, 258–265. doi: 10.1016/j.bbabio.2011.04.010
Chukhutsina, V. U., Büchel, C., and Van Amerongen, H. (2014). Disentangling two non-photochemical quenching processes in Cyclotella meneghiniana by spectrally-resolved picosecond fluorescence at 77K. Biochim. Biophys. Acta 1837, 899–907. doi: 10.1016/j.bbabio.2014.02.021
Cloern, J. E., Grenz, C., and Vidergarlucas, L. (1995). An empirical model of the phytoplankton chlorophyll:carbon ratio - The conversion factor between productivity and growth rate. Limnol. Oceanogr. 40, 1313–1321. doi: 10.4319/lo.1995.40.7.1313
Cullen, J. J., and Macintyre, J. G. (1998). “Behavior, physiology and the niche of depth-regulating phytoplankton,” in Physiological Ecology of Harmful Algal Blooms, eds Heidelberg, D. M. Anderson, A. D. Cembella, and G. M. Hallegraeff (Berlin: Springer-Verlag), 559–580.
Cvetkovska, M., Hüner, N. P. A., and Smith, D. R. (2017). Chilling out: the evolution and diversification of psychrophilic algae with a focus on Chlamydomonadales. Polar Biol. 40, 1169–1184. doi: 10.1007/s00300-016-2045-4
Davison, I. R. (1991). Environmental effects on algal photosynthesis: temperature. J. Phycol. 27, 2–8. doi: 10.1111/j.0022-3646.1991.00002.x
Demmig-Adams, B., Adams, W. W., Ebbert, V., and Logan, B. A. (1999). “Ecophysiology of the xanthophyll cycle,” in The Photochemistry of Carotenoids, eds. H. A. Frank, A. J. Young, G. Britton, and R. J. Cogdell (Dordrecht: Springer Netherlands), 245–269.
Demmig-Adams, B., Ebbert, V., Zarter, C. R., and Adams, W. W. (2008). “Characteristics and species-dependent employment of flexible versus sustained thermal dissipation and photoinhibition,” in Photoprotection, Photoinhibition, Gene Regulation, and Environment, eds. B. Demmig-Adams, W. W. Adams, and A. K. Mattoo (Dordrecht: Springer Netherlands), 39–48.
Demmig-Adams, B., Koh, S. C., Cohu, C., Muller, O., Stewart, J., and Adams, W. III. (2014). “Non-photochemical fluorescence quenching in contrasting plant species and environments,” in Non-Photochemical Quenching and Energy Dissipation in Plants, Algae and Cyanobacteria, eds B. Demmig-Adams, G. Garab, W. Adams III, and Govindjee (Dordrecht: Springer Netherlands), 531–552.
Falkowski, P. G., and Laroche, J. (1991). Acclimation to spectral irradiance in algae. J. Phycol. 27, 8–14. doi: 10.1111/j.0022-3646.1991.00008.x
Fanesi, A., Wagner, H., Becker, A., and Wilhelm, C. (2016). Temperature affects the partitioning of absorbed light energy in freshwater phytoplankton. Freshw. Biol. 61, 1365–1378. doi: 10.1111/fwb.12777
Fragoso, G. M., Poulton, A. J., Yashayaev, I. M., Head, E. J. H., and Purdie, D. A. (2017). Spring phytoplankton communities of the Labrador Sea (2005–2014): pigment signatures, photophysiology and elemental ratios. Biogeosciences 14, 1235–1259. doi: 10.5194/bg-14-1235-2017
Garab, G. (2014). Hierarchical organization and structural flexibility of thylakoid membranes. Biochim. Biophys. Acta 1837, 481–494. doi: 10.1016/j.bbabio.2013.12.003
Geider, R. J. (1987). Light and temperature dependence of the carbon to chlorophyll a ratio in microalgae and cyanobacteria: implications for physiology and growth of phytoplankton. New Phytol. 106, 1–34. doi: 10.1111/j.1469-8137.1987.tb04788.x
Gilstad, M., and Sakshaug, E. (1990). Growth rates of 10 diatoms species from the Barents sea at different irradiances and day lengths. Mar. Ecol. Prog. Ser. 64, 169–173. doi: 10.3354/meps064169
Goericke, R., and Welschmeyer, N. A. (1992). Pigment turnover in the marine diatom Thalassiosira weissflogii. ii. the 14CO2-labeling kinetics of carotenoids. J. Phycol. 28, 507–517. doi: 10.1111/j.0022-3646.1992.00507.x
Goss, R., Ann Pinto, E., Wilhelm, C., and Richter, M. (2006). The importance of a highly active and ΔpH-regulated diatoxanthin epoxidase for the regulation of the PS II antenna function in diadinoxanthin cycle containing algae. J. Plant Physiol. 163, 1008–1021. doi: 10.1016/j.jplph.2005.09.008
Goss, R., and Lepetit, B. (2015). Biodiversity of NPQ. J. Plant Physiol. 172, 13–32. doi: 10.1016/j.jplph.2014.03.004
Guillard, R. R. L. (1975). “Culture of phytoplankton for feeding marine invertebrates,” in Culture of Invertabrate Animals, eds W. L. Smith, M. H. Chanley (Boston, MA: Springer).
Ha, S.-Y., Lee, D. B., Kang, S.-H., and Shin, K.-H. (2016). Strategy of photo-protection in phytoplankton assemblages in the Kongsfjorden, Svalbard, Arctic. Chin. J. Oceanol. Limnol. 34, 1–12. doi: 10.1007/s00343-015-4295-3
Halsey, K. H., and Jones, B. M. (2015). Phytoplankton strategies for photosynthetic energy allocation. Ann. Rev. Mar. Sci. 7, 265–297. doi: 10.1146/annurev-marine-010814-015813
Hendrickson, L., Furbank, R., and Chow, W. (2004). A simple alternative approach to assessing the fate of absorbed light energy using chlorophyll fluorescence. Photosyn. Res. 82, 73–81. doi: 10.1023/B:PRES.0000040446.87305.f4
Hoppe, C. J. M., Schuback, N., Semeniuk, D., Giesbrecht, K., Mol, J., Thomas, H., et al. (2018). Resistance of Arctic phytoplankton to ocean acidification and enhanced irradiance. Polar Biol. 41, 399–413. doi: 10.1007/s00300-017-2186-0
Horvat, C., Jones, D. R., Iams, S., Schroeder, D., Flocco, D., and Feltham, D. (2017). The frequency and extent of sub-ice phytoplankton blooms in the Arctic Ocean. Sci. Adv. 3:e1601191. doi: 10.1126/sciadv.1601191
Kana, T. M., and Glibert, P. M. (1987). Effect of irradiances up to 2000 μE m−2 s−1 on marine Synechococcus WH7803—II. Photosynthetic responses and mechanisms. Deep Sea Res. Part A Oceanogr. Res. Papers 34, 497–516. doi: 10.1016/0198-0149(87)90002-1
Kashino, Y., Kudoh, S., Hayashi, Y., Suzuki, Y., Odate, T., Hirawake, T., et al. (2002). Strategies of phytoplankton to perform effective photosynthesis in the North Water. Deep Sea Res. Part II Topical Stud. Oceanogr. 49, 5049–5061. doi: 10.1016/S0967-0645(02)00177-7
Kauko, H. M., Taskjelle, T., Assmy, P., Pavlov, A. K., Mundy, C. J., Duarte, P., et al. (2017). Windows in Arctic sea ice: light transmission and ice algae in a refrozen lead. J. Geophys. Res. Biogeosci. 122, 1486–1505. doi: 10.1002/2016JG003626
Knap, A., Michaels, A., Close, A., Ducklow, H., and Dickson, A. (1996). Protocols for the Joint Global OCEAN flux Study (JGOFS) core measurements. JGOFS, Reprint of the IOC Manuals and Guides No. 29, UNESCO 1994 119.
Kropuenske, L. R., Mills, M. M., Van Dijken, G. L., Alderkamp, A.-C., Mine Berg, G., Robinson, D. H., et al. (2010). Strategies and rates of photoacclimation in two major southern ocean phytoplankton taxa: Phaeocystis antarctica (Haptophyta) and Fragilariopsis cylindrus (Bacillariophyceae). J. Phycol. 46, 1138–1151. doi: 10.1111/j.1529-8817.2010.00922.x
Kropuenske, L. R., Mills, M. M., Van Dijken, G. L., Bailey, S., Robinson, D. H., Welschmeyer, N. A., et al. (2009). Photophysiology in two major Southern Ocean phytoplankton taxa: Photoprotection in Phaeocystis antarctica and Fragilariopsis cylindrus. Limnol. Oceanogr. 54, 1176–1196. doi: 10.4319/lo.2009.54.4.1176
La Rocca, N., Sciuto, K., Meneghesso, A., Moro, I., Rascio, N., and Morosinotto, T. (2015). Photosynthesis in extreme environments: responses to different light regimes in the Antarctic alga Koliella antarctica. Physiol. Plant. 153, 654–667. doi: 10.1111/ppl.12273
Lacour, T., Larivière, J., and Babin, M. (2017). Growth, Chl a content, photosynthesis, and elemental composition in polar and temperate microalgae. Limnol. Oceanogr. 62, 43–58. doi: 10.1002/lno.10369
Lacour, T., Sciandra, A., Talec, A., Mayzaud, P., and Bernard, O. (2012). Neutral lipid and carbohydrate productivities as a response to nitrogen status in Isochrysis sp (T-iso; Haptophyceae): starvation vs. limitation. J. Phycol. 48, 647–656. doi: 10.1111/j.1529-8817.2012.01154.x
Lavaud, J., and Goss, R. (2014). “The peculiar features of non-photochemical fluorescence quenching in diatoms and brown algae,” in Non-Photochemical Quenching and Energy Dissipation in Plants, Algae and Cyanobacteria, eds. B. Demmig-Adams, G. Garab, W. Adams, and Govindjee (Dordrecht: Springer Netherlands), 421–443.
Lavaud, J., and Lepetit, B. (2013). An explanation for the inter-species variability of the photoprotective non-photochemical chlorophyll fluorescence quenching in diatoms. Biochim. Biophys. Acta 1827, 294–302. doi: 10.1016/j.bbabio.2012.11.012
Lavaud, J., Rousseau, B., and Etienne, A. L. (2002). In diatoms, a transthylakoid proton gradient alone is not sufficient to induce a non-photochemical fluorescence quenching. FEBS Lett. 523, 163–166. doi: 10.1016/S0014-5793(02)02979-4
Lavaud, J., Six, C., and Campbell, D. (2016). Photosystem II repair in marine diatoms with contrasting photophysiologies. Photosynth. Res. 127, 189–199. doi: 10.1007/s11120-015-0172-3
Lavaud, J., Strzepek, R. F., and Kroth, P. G. (2007). Photoprotection capacity differs among diatoms: possible consequences on the spatial distribution of diatoms related to fluctuations in the underwater light climate. Limnol. Oceanogr. 52, 1188–1194. doi: 10.4319/lo.2007.52.3.1188
Lavoie, D., Denman, K., and Michel, C. (2005). Modeling ice algal growth and decline in a seasonally ice-covered region of the Arctic (Resolute Passage, Canadian Archipelago). J. Geophys. Res. 110:C11009. doi: 10.1029/2005JC002922
Lee, J. R., Raymond, B., Bracegirdle, T. J., Chadès, I., Fuller, R. A., Shaw, J. D., et al. (2017). Climate change drives expansion of Antarctic ice-free habitat. Nature 547, 49. doi: 10.1038/nature22996
Lepetit, B., Sturm, S., Rogato, A., Gruber, A., Sachse, M., Falciatore, A., et al. (2013). High light acclimation in the secondary plastids containing diatom Phaeodactylum tricornutum is triggered by the redox state of the plastoquinone pool. Plant Physiol. 161, 853–865. doi: 10.1104/pp.112.207811
Lepetit, B., Volke, D., Gilbert, M., Wilhelm, C., and Goss, R. (2010). Evidence for the existence of one antenna-associated, lipid-dissolved and two protein-bound pools of diadinoxanthin cycle pigments in diatoms. Plant Physiol. 154, 1905–1920. doi: 10.1104/pp.110.166454
Leu, E., Graeve, M., and Wulff, A. (2016). A (too) bright future? Arctic diatoms under radiation stress. Polar Biol. 39, 1711–1724. doi: 10.1007/s00300-016-2003-1
Lewis, M. R., and Smith, J. C. (1983). A small volume, short-incubation-time method for measure-ment of photosynthesis as a function of incident irradiance. Mar. Ecol. Prog. Ser. 13, 99–102. doi: 10.3354/meps013099
Lin, H., Kuzminov, F. I., Park, J., Lee, S., Falkowski, P. G., and Gorbunov, M. Y. (2016). The fate of photons absorbed by phytoplankton in the global ocean. Science 351, 264–267. doi: 10.1126/science.aab2213
Lyon, B. R., and Mock, T. (2014). Polar microalgae: new approaches towards understanding adaptations to an extreme and changing environment. Biology 3, 56–80. doi: 10.3390/biology3010056
Macintyre, H. L., and Cullen, J. J. (2005). “Using cultures to investigate the physiological ecology of microalgae,” in Algal Culturing Techniques, ed R. A. Anderson (Burlington: Academic Press), 287–326.
Macintyre, H. L., Kana, T. M., Anning, T., and Geider, R. J. (2002). Photoacclimation of photosynthesis irradiance response curves and photosynthetic pigments in microalgae and cyanobacteria. J. Phycol. 38, 17–38. doi: 10.1046/j.1529-8817.2002.00094.x
Markus, T., Stroeve, J. C., and Miller, J. (2009). Recent changes in Arctic sea ice melt onset, freezeup, and melt season length. J. Geophys. Res. 114:024. doi: 10.1029/2009JC005436
Materna, A. C., Sturm, S., Kroth, P. G., and Lavaud, J. (2009). First induced plastid genome mutations in an alga with secondary plastids: psbA mutations in the diatom Phaeodactylum tricornutum (Bacillariophyceae) reveal consequences on the regulation of photosynthesis. J. Phycol. 45, 838–846. doi: 10.1111/j.1529-8817.2009.00711.x
Míguez, F., Fernández-Marín, B., Becerril, J. M., and García-Plazaola, J.-I. (2017). Diversity of winter photoinhibitory responses: a case study in co-occurring lichens, mosses, herbs and woody plants from subalpine environments. Physiol. Plant. 160, 282–296. doi: 10.1111/ppl.12551
Míguez, F., Fernández-Marín, B., Becerril, J. M., and García-Plazaola, J. I. (2015). Activation of photoprotective winter photoinhibition in plants from different environments: a literature compilation and meta-analysis. Physiol. Plant. 155, 414–423. doi: 10.1111/ppl.12329
Mills, M. M., Kropuenske, L. R., Van Dijken, G. L., Alderkamp, A. C., Berg, G. M., Robinson, D. H., et al. (2010). Photophysiology in two southern ocean phytoplankton taxa: photosynthesis of Phaeocystis antarctica (Prymnesiophyceae) and Fragilariopsis cylindrus (Bacillariophyceae) under simulated mixed-layer irradiance. J. Phycol. 46, 1114–1127. doi: 10.1111/j.1529-8817.2010.00923.x
Mock, T., and Hoch, N. (2005). Long-term temperature acclimation of photosynthesis in steady-state cultures of the polar diatom Fragilariopsis cylindrus. Photosyn. Res. 85, 307–317. doi: 10.1007/s11120-005-5668-9
Morgan-Kiss, R. M., Priscu, J. C., Pocock, T., Gudynaite-Savitch, L., and Huner, N. P. A. (2006). Adaptation and acclimation of photosynthetic microorganisms to permanently cold environments. Microbiol. Mol. Biol. Rev. 70, 222–252. doi: 10.1128/MMBR.70.1.222-252.2006
Mouget, J. L., Tremblin, G., Morant-Manceau, A., Morançais, M., and Robert, J. M. (1999). Long-term photoacclimation of Haslea ostrearia (Bacillariophyta): effect of irradiance on growth rates, pigment content and photosynthesis. Eur. J. Phycol. 34, 109–115. doi: 10.1080/09670269910001736162
Nicolaus, M., Katlein, C., Maslanik, J., and Hendricks, S. (2012). Changes in Arctic sea ice result in increasing light transmittance and absorption. Geophys. Res. Lett. 39. doi: 10.1029/2012GL053738
Notz, D., and Stroeve, J. (2016). Observed Arctic sea-ice loss directly follows anthropogenic CO2 emission. Science 354, 747–750. doi: 10.1126/science.aag2345
Öquist, G., and Huner, N. P. A. (2003). Photosynthesis of overwintering evergreen plants. Annu. Rev. Plant Biol. 54, 329–355. doi: 10.1146/annurev.arplant.54.072402.115741
Oxborough, K., and Baker, N. R. (1997). Resolving chlorophyll a fluorescence images of photosynthetic efficiency into photochemical and non-photochemical components – calculation of qP and Fv-/Fm-; without measuring Fo. Photosyn. Res. 54, 135–142. doi: 10.1023/A:1005936823310
Parsons, T. R., Maita, Y., and Lalli, C. M. (1984). “5.1 - Photosynthesis as measured by the uptake of radioactive carbon,” in A Manual of Chemical & Biological Methods for Seawater Analysis, ed. T. R. Parsons, M. Maita, and C. M. Lalli (Amsterdam: Pergamon), 115–120.
Perkins, R. G., Kromkamp, J. C., Serôdio, J., Lavaud, J., Jesus, B., Mouget, J. L., et al. (2011). “The application of variable chlorophyll fluorescence to microphytobenthic biofilms,” in Chlorophyll a Fluorescence in Aquatic Sciences: Methods and Applications, eds J. D. Suggett, O. Prášil, and A. M. Borowitzka (Dordrecht: Springer Netherlands), 237–275.
Perovich, D. K., and Polashenski, C. (2012). Albedo evolution of seasonal Arctic sea ice. Geophys. Res. Lett. 39:L08501. doi: 10.1029/2012GL051432
Petrou, K., Doblin, M., and Ralph, P. (2011). Heterogeneity in the photoprotective capacity of three Antarctic diatoms during short-term changes in salinity and temperature. Mar. Biol. 158, 1029–1041. doi: 10.1007/s00227-011-1628-4
Petrou, K., Hill, R., Brown, C. M., Campbell, D. A., Doblin, M. A., and Ralph, P. J. (2010). Rapid photoprotection in sea-ice diatoms from the East Antarctic pack ice. Limnol. Oceanogr. 55:8. doi: 10.4319/lo.2010.55.3.1400
Petrou, K., Kranz, S. A., Trimborn, S., Hassler, C. S., Ameijeiras, S. B., Sackett, O., et al. (2016). Southern Ocean phytoplankton physiology in a changing climate. J. Plant Physiol. 203, 135–150. doi: 10.1016/j.jplph.2016.05.004
Petrou, K., and Ralph, P. (2011). Photosynthesis and net primary productivity in three Antarctic diatoms: possible significance for their distribution in the Antarctic marine ecosystem. Mar. Ecol. Prog. Ser. 437, 27–40. doi: 10.3354/meps09291
Platt, T., Gallegos, C. L., and Harrison, W. G. (1980). Photoinhibition of photosynthesis in natural assemblages of marine phytoplankton. J. Mar. Res. 38, 687–701.
Poulin, M., Daugbjerg, N., Gradinger, R., Ilyash, L., Ratkova, T., and Von Quillfeldt, C. (2011). The pan-Arctic biodiversity of marine pelagic and sea-ice unicellular eukaryotes: a first-attempt assessment. Mar. Biodiv. 41, 13–28. doi: 10.1007/s12526-010-0058-8
Ralph, P. J., and Gademann, R. (2005). Rapid light curves: a powerful tool to assess photosynthetic activity. Aquat. Bot. 82, 222–237. doi: 10.1016/j.aquabot.2005.02.006
Redfield, A. C. (1934). On the Proportions of Organic Derivatives in Sea Water and Their Relation to the Composition of Plankton. Liverpool: University Press of Liverpool.
Robinson, D. H., Kolber, Z., and Sullivan, C. W. (1997). Photophysiology and photoacclimation in surface sea ice algae from McMurdo Sound, Antarctica. Mar. Ecol. Prog. Ser. 147, 243–256. doi: 10.3354/meps147243
Sackett, O., Petrou, K., Reedy, B., De Grazia, A., Hill, R., Doblin, M., et al. (2013). Phenotypic plasticity of Southern Ocean diatoms: key to success in the sea ice habitat? PLoS ONE 8:e81185. doi: 10.1371/journal.pone.0081185
Sarthou, G., Timmermans, K. R., Blain, S., and Tréguer, P. (2005). Growth physiology and fate of diatoms in the ocean: a review. J. Sea Res. 53, 25–42. doi: 10.1016/j.seares.2004.01.007
Schumann, A., Goss, R., Jakob, T., and Wilhelm, C. (2007). Investigation of the quenching efficiency of diatoxanthin in cells of Phaeodactylum tricornutum (Bacillariophyceae) with different pool sizes of xanthophyll cycle pigments. Phycologia 46, 113–117. doi: 10.2216/06-30.1
Serôdio, J., Ezequiel, J., Barnett, A., Mouget, J. L., Méléder, V., Laviale, M., et al. (2012). Efficiency of photoprotection in microphytobenthos: role of vertical migration and the xanthophyll cycle against photoinhibition. Aquat. Microbial. Ecol. 67, 161–175. doi: 10.3354/ame01591
Streb, P., and Cornic, G. (2012). “Photosynthesis and antioxidative protection in alpine herbs,” in Plants in Alpine Regions: Cell Physiology of Adaption and Survival Strategies, ed C. Lütz (Vienna: Springer Vienna), 75–97.
Stroeve, J. C., Serreze, M. C., Holland, M. M., Kay, J. E., Malanik, J., and Barrett, A. P. (2012). The Arctic's rapidly shrinking sea ice cover: a research synthesis. Clim. Change 110, 1005–1027. doi: 10.1007/s10584-011-0101-1
Talmy, D., Blackford, J., Hardman-Mountford, N. J., Dumbrell, A. J., and Geider, R. J. (2013). An optimality model of photoadaptation in contrasting aquatic light regimes. Limnol. Oceanogr. 58, 1802–1818. doi: 10.4319/lo.2013.58.5.1802
Thomas, M. K., Kremer, C. T., Klausmeier, C. A., and Litchman, E. (2012). A global pattern of thermal adaptation in marine phytoplankton. Science 338, 1085–1088. doi: 10.1126/science.1224836
van de Poll, W. H. V., Lagunas, M., De Vries, T., Visser, R. J. W., and Buma, A. G. J. (2011). Non-photochemical quenching of chlorophyll fluorescence and xanthophyll cycle responses after excess PAR and UVR in Chaetoceros brevis, phaeocystis Antarctica and coastal Antarctic phytoplankton. Mar. Ecol. Prog. Ser. 426, 119–131. doi: 10.3354/meps09000
Van Kooten, O., and Snel, J. F. (1990). The use of chlorophyll fluorescence nomenclature in plant stress physiology. Photosyn. Res. 25, 147–150. doi: 10.1007/BF00033156
Verhoeven, A. (2014). Sustained energy dissipation in winter evergreens. New Phytolo. 201, 57–65. doi: 10.1111/nph.12466
Verhoeven, A. S., Adams, W. W., and Demmig-Adams, B. (1996). Close relationship between the state of the xanthophyll cycle pigments and photosystem II efficiency during recovery from winter stress. Physiol. Plant. 96, 567–576. doi: 10.1111/j.1399-3054.1996.tb00228.x
Verity, P. G. (1982). Effects of temperature, irradiance, and daylength on the marine diatom Leptocylindrus danicus Cleve. IV. Growth. J. Exp. Mar. Biol. Ecol. 60, 209–222. doi: 10.1016/0022-0981(82)90160-5
Wagner, H., Jakob, T., and Wilhelm, C. (2006). Balancing the energy flow from captured light to biomass under fluctuating light conditions. New Phytol. 169, 95–108. doi: 10.1111/j.1469-8137.2005.01550.x
Wassmann, P., and Reigstad, M. (2011). Future arctic ocean seasonal ice zones and implications for pelagic-benthic coupling. Oceanography 24, 220–231. doi: 10.5670/oceanog.2011.74
White, A. J., and Critchley, C. (1999). Rapid light curves: a new fluorescence method to assess the state of the photosynthetic apparatus. Photosyn. Res. 59, 63–72. doi: 10.1023/A:1006188004189
Willemoës, M., and Monas, E. (1991). Relationship between growth irradiance and the xanthophyll cycle pool in the diatom Nitzschia palea. Physiol. Plant. 83, 449–456. doi: 10.1111/j.1399-3054.1991.tb00119.x
Wu, H., Cockshutt, A. M., McCarthy, A., and Campbell, D. A. (2011). Distinctive photosystem II photoinactivation and protein dynamics in marine diatoms. Plant Physiol. 156, 2184–2195. doi: 10.1104/pp.111.178772
Wu, H., Roy, S., Alami, M., Green, B. R., and Campbell, D. A. (2012). Photosystem II photoinactivation, repair, and protection in marine centric diatoms. Plant Physiol. 160, 464–476. doi: 10.1104/pp.112.203067
Yoder, J. A. (1979). Effect of temperature on light-limited growth and chemical composition of Skeletonema costatum (Bacillariophyceae). J. Phycol. 15, 362–370. doi: 10.1111/j.1529-8817.1979.tb00706.x
Young, J. N., Goldman, J. A. L., Kranz, S. A., Tortell, P. D., and Morel, F. M. M. (2015). Slow carboxylation of Rubisco constrains the rate of carbon fixation during Antarctic phytoplankton blooms. New Phytol. 205, 172–181. doi: 10.1111/nph.13021
Zapata, M., Rodriguez, F., and Garrido, J. L. (2000). Separation of chlorophylls and carotenoids from marine phytoplankton: a new HPLC method using a reversed phase C8 column and pyridine-containing mobile phases. Mar. Ecol. Prog. Ser. 195, 29–45. doi: 10.3354/meps195029
Zeebe, R. E., Eicken, H., Robinson, D. H., Wolf-Gladrow, D., and Dieckmann, G. S. (1996). Modeling the heating and melting of sea ice through light absorption by microalgae. J. Geophys. Res. 101, 1163–1181. doi: 10.1029/95JC02687
Keywords: Arctic diatom, photosynthesis, sustained NPQ, irradiance, temperature
Citation: Lacour T, Larivière J, Ferland J, Bruyant F, Lavaud J and Babin M (2018) The Role of Sustained Photoprotective Non-photochemical Quenching in Low Temperature and High Light Acclimation in the Bloom-Forming Arctic Diatom Thalassiosira gravida. Front. Mar. Sci. 5:354. doi: 10.3389/fmars.2018.00354
Received: 19 April 2018; Accepted: 14 September 2018;
Published: 22 October 2018.
Edited by:
Hongyue Dang, Xiamen University, ChinaReviewed by:
Mark Moore, University of Southampton, United KingdomBenjamin Bailleul, Centre National de la Recherche Scientifique (CNRS), France
Copyright © 2018 Lacour, Larivière, Ferland, Bruyant, Lavaud and Babin. This is an open-access article distributed under the terms of the Creative Commons Attribution License (CC BY). The use, distribution or reproduction in other forums is permitted, provided the original author(s) and the copyright owner(s) are credited and that the original publication in this journal is cited, in accordance with accepted academic practice. No use, distribution or reproduction is permitted which does not comply with these terms.
*Correspondence: Thomas Lacour, dGhvbWFzLmxhY291ckBpZnJlbWVyLmZy
†Present Address: Lacour Thomas, PBA IFREMER, Centre Atlantique, Nantes, France