- 1Centre for Marine Bio-Innovation, School of Biological, Earth and Environmental Sciences, University of New South Wales, Sydney, NSW, Australia
- 2Sydney Institute of Marine Science, Sydney, NSW, Australia
- 3Singapore Centre for Environmental Life Sciences Engineering, Nanyang Technological University, Singapore, Singapore
- 4School of Life and Environmental Sciences, The University of Sydney, Sydney, NSW, Australia
- 5National Biodiversity Centre, National Parks Board, Singapore Botanic Gardens, Singapore, Singapore
- 6Experimental Marine Ecology Laboratory, Department of Biological Science, National University of Singapore, Singapore, Singapore
- 7Advanced Environmental Biotechnology Centre, Nanyang Technological University, Singapore, Singapore
- 8School of Natural and Environmental Sciences, Newcastle University, Newcastle upon Tyne, United Kingdom
Scleractinian corals are vulnerable to a range of environmental disturbances, but generally suffer the highest rates of mortality during early life-history stages, i.e., from larval settlement until a few months post-settlement. Variations in survival rates of corals during this period play a key role in structuring adult coral populations. Many coral reefs have experienced reductions in herbivory rates due to overfishing and consequent increases in macroalgae, however, the effect of increased coral-algal interactions may vary between coral life-history stages and among locations. Therefore understanding the relative importance of different drivers of mortality across early life-history stages, under a range of environmental conditions, is essential to effectively manage and restore coral reefs. To date, however, relatively few studies have (a) examined coral-algal interactions across several early life-history stages (i.e., from planulae to juvenile colonies) and (b) done so in highly disturbed reefs close to large urban centers. We investigated the effect of algal-coral-herbivore interactions on early life history stages in the coral Pocillopora acuta on coral reefs off mainland Singapore, a heavily disturbed “urbanized reef environment”. Larval settlement rates were estimated in the presence of six macroalgal species ex situ. The effect of direct interaction with two macroalgal species on newly settled spat was examined in situ and the effect of reduced herbivory was tested with exclusion cages on naturally settled 9-month-old juveniles in situ. We found significant reductions in P. acuta settlement in the presence of four macroalgal species. Newly settled spat of P. acuta had significantly lower survivorship when in contact with Sargassum sp. on the reef crest but not with Bryopsis sp. on the reef flat. Herbivore exclusion reduced survivorship of juvenile corals, which was associated with increased sediment accumulation, but not with algal biomass. Our results suggest coral recruitment on heavily disturbed reefs can be impacted by species-specific macroalgal effects via reduced settlement on ephemeral substrata and reduced survivorship when in direct contact with Sargassum sp. Furthermore, recruitment may be negatively impacted by reductions in herbivory, possibly via increased abundance of epilithic algal matrix leading to sediment trapping.
Introduction
The world’s coral reefs are under sustained pressure from multiple human impacts such as climate change, overfishing and coastal land-use changes (Hughes, 1994). In response to these anthropogenic stressors, coral cover, and structural complexity have declined dramatically on many reefs in recent decades (De’ath et al., 2012; Hughes et al., 2018). Relatively isolated reefs can recover rapidly following natural disturbances if ecological processes such as coral recruitment and herbivory have not been compromised by previous human disturbances (e.g., Gilmour et al., 2013). Much less is known about the recovery potential of corals subjected to chronic disturbances found on inshore, highly urbanized coral reefs (Cleary et al., 2014; Guest et al., 2016a) such as turbidity, sedimentation, and pollution (Fabricius, 2005).
Recruitment is a key ecological process in the recovery and persistence of coral reef communities. The early life history of reef-building corals involves a series of critical stages, all of which need to be successful for recruitment to occur (Vermeij and Sandin, 2008; Ritson-Williams et al., 2009; Doropoulos et al., 2016). These stages include a pelagic larval phase; a benthic “searching” phase, for larvae to identify suitable substratum for settlement and metamorphosis; and a period of somatic growth and sexual maturation (Babcock and Mundy, 1996; Heyward and Negri, 1999; Raimondi and Morse, 2000). Survivorship of early life history stages for corals has been likened to “running the gauntlet” (Arnold et al., 2010) because coral juveniles are vulnerable to high mortality rates (i.e., type III survivorship, sensu Deevey, 1947) during this period. Newly settled and juvenile corals are also more vulnerable to removal, overgrowth, sediment burial, shading and abrasion compared to adults due to their smaller size and lack of height (Zilberberg and Edmunds, 2001; Raymundo and Maypa, 2004; Birrell et al., 2005; Box and Mumby, 2007; Tebben et al., 2014). Thus, environmental perturbations may have a greater effect on the survival of juvenile corals compared to their adult counterparts and hence may strongly influence the structuring of adult coral populations.
Interactions with macroalgae can be particularly damaging to corals during the early life history stages. Reductions in successful settlement can occur due to macroalgae occupying potential settlement space (Olsen et al., 2015), direct inhibition of settlement via allelochemicals (Paul et al., 2011; Dixson et al., 2014), reductions in settlement surface due to increased sediment trapping by algae (Birrell et al., 2005), reductions in light availability (Strader et al., 2015), and direct physical abrasion of newly settled and juvenile corals (Box and Mumby, 2007; Venera-Ponton et al., 2011).
The extent of macroalgal herbivory on a reef can also govern coral recruitment success (Hughes et al., 2007). When herbivory is markedly reduced by overfishing, reef resilience is reduced and there is a greater chance of a reef undergoing a shift towards macroalgal dominance following acute disturbances (Hughes, 1994; Edwards et al., 2014). Paradoxically, fish herbivory may also contribute to early coral mortality via accidental removal of newly settled corals and coral juveniles (Brock, 1979; Baria et al., 2010; Venera-Ponton et al., 2011). Therefore, understanding the effects of macroalgae on recruitment success requires consideration of other ecological processes such as fish herbivory.
Tropical coastal zones are experiencing unprecedented rates of change, driven by human population growth and marked coastal development (Dsikowitzky et al., 2016). As a result, an increasing number of coral reefs will experience the effects of “urbanization” in the Anthropocene. Urban coral reefs are considered a distinctive group of marginal reefs characterized by extremely low water quality, high abundance of macroalgae, low herbivory and depth restricted coral communities composed of stress tolerant taxa (reviewed by Heery et al., 2018). Despite the extreme abiotic conditions, coral communities on some urbanized reefs can actually be quite resilient to acute disturbances (e.g., Guest et al., 2012, 2016a), suggesting that these reefs may have significant ecological and conservation value. Unfortunately, there are still relatively few studies examining coral-macroalgal-herbivore interactions from highly disturbed inshore-reefs, with studies limited to Kenya (Mwachireya et al., 2017) and India (Manikandan and Ravindran, 2017). Our lack of knowledge of key ecological processes such as recruitment and herbivory on these important reefs hinders our ability to effectively manage them.
In this study, we examined the effect of macroalgae and herbivory on Pocillopora acuta’s likelihood of successfully recruiting in Singapore reefs, a highly disturbed coral reef system. To achieve this, we (1) compared coral larval settlement rates in the presence of several macroalgal species, (2) examined the effect of direct interaction between macroalgae and newly settled spat, and (3) investigated the effect of reduced herbivory on juvenile coral growth and survival.
Materials and Methods
Study Site and Species
Singapore’s coral reefs have experienced multiple human disturbances over the last 200 years (Corlett, 1992), and are currently subject to high rates of sedimentation, eutrophication and other stressors (Todd et al., 2004; Van Maren et al., 2014). Despite being severely disturbed, diverse coral communities (>250 coral species) still persist on the fringing reefs surrounding Singapore’s southern islands (Huang et al., 2009). Hard corals are largely restricted to ∼8 m depth due to very high light attenuation and communities are mostly composed of stress tolerant and generalist taxa (Browne et al., 2015; Guest et al., 2016a). The mean cover of coral in Singapore at 3–4 m and at 6–7 m depth is ∼36 and ∼21%, respectively (Guest et al., 2016a). Meanwhile, macroalgae, particularly Sargassum spp., is highly abundant on shallow reef flats (0–2 m depth) (Low, 2015) but lower in cover ∼10% and ∼3% at 3–4 m and 6–7 m depths, respectively (Guest et al., 2016a). Singapore reefs also generally exhibit relatively low herbivory rates; herbivore biomass on reefs is dominated by browsers and small non-obligate herbivores with a very low abundance of scrapers and excavators (Guest et al., 2016b; Bauman et al., 2017). The sites used in the present study were two fringing reefs adjacent to Kusu Island (1°13′26″N, 103°51′39″E) and Sister’s Island (1°12′55.4″N 103°50′09.3″E). The brooding species P. acuta was chosen as the study species due to its relatively high abundance at the study sites (Huang et al., 2009; Poquita-Du et al., 2017).
Algal-Coral Interactions
The Effects of Macroalgae on Settlement of Coral Larvae
Ten P. acuta colonies (15–20 cm diameter) were collected from the fringing reef of Kusu Island 2–3 days before the new moon in May and June 2014. Colonies were kept in flow-through, artificially lit indoor, seawater tanks. Larvae released in tanks were collected through outflow tubes fitted with 800 μm mesh nets each morning. Larvae were pooled together, cleaned with 0.2 μm filtered seawater (FSW), and kept in aerated tanks prior to experiments. Six locally abundant macroalgal species (Lee et al., 2009; Goh and Lim, 2015) were collected from Kusu and maintained in aquaria 2–3 days prior to experiments. The algal species collected were Bryopsis pennata var. secunda, Hypnea pannosa, Halymenia durvillei, Lobophora variegata, Sargassum siliquosum and Padina sp., (the latter could not be reliably identified to species; refer to Supplementary Data 1B for a detailed description on algal species identification).
To test for effects of algae on coral settlement, larval settlement rates in the presence and absence of each algal species was estimated. We used 15 replicate sterile petri dishes per species (90 mm × 11 mm). Each replicate contained 30 ml of 0.2 μm filtered seawater (FSW), a 2 cm × 2 cm × 0.5 cm conditioned ceramic tile to induce planula settlement (Heyward and Negri, 1999), 15 coral planulae and 0.5 ± 0.1 g of wet algal mass following Vermeij et al. (2009). The control treatment replicates contained conditioned tiles but no macroalgae. Ceramic tiles were biologically conditioned for several weeks in a flow-through seawater tank at Tropical Marine Science Institute at St. John’s Island prior to the study. Tiles contained at least 50% cover of crustose coralline algae (CCA) of a multi species assemblage. CCA derived from these tanks have been used in several previous coral larval experiments and are therefore known to be settlement inducers (e.g., Tebben et al., 2014, 2015). In all treatment replicates, the tiles and macroalgae pieces were positioned haphazardly in the petri dishes but no more than 1 cm apart in situ (Vermeij et al., 2011). Replicates were covered with transparent covers to prevent evaporation and drying out of CCA, planulae, and macroalgae, but not fully sealed to allow gaseous exchange and prevent hypoxia for both macroalgae and larvae. Algal mimics were not included in the experiment as the aim of the study was to test for changes in settlement rates in the presence of macroalgae, and not to determine the mechanisms driving these changes (e.g., physical vs. allelochemical or microbial).
Replicates were placed into 29°C-water baths, with temperatures comparable to mean ambient outdoor sea surface temperature (28–31°C) and left for 24 h. The proportions of successfully settled spat were quantified under a dissecting microscope. Successful settlement is defined here as settlement and metamorphosis of a spat on any substrate (Babcock et al., 2003). Settlement proportions were measured in two categories; (1) on all surfaces (either on the tile, petri dish surface or algae) and (2) on surfaces excluding algal surfaces. We excluded settlement counts on algal surfaces in (2) in order to discriminate between absolute settlement rates on all surfaces and settlement on unsuitable and ephemeral substrata such as algal surfaces sensu Nugues and Szmant (2006).
We performed a one-way ANOVA to test for differences in the proportion of larval settlement among algal treatments. Separate ANOVAs were performed for settlement proportions in (1) and (2), followed by respective Tukey post hoc tests with a Bonferroni correction for multiple testing. Datasets were not transformed as they met homoscedasticity and normality assumptions prior to ANOVA. Analyses were performed with the R statistical software (R Core Team, 2014; version, 3.1.1).
Effects of Macroalgae on Early Post-settlement Mortality of Corals in situ
Sixty unglazed terracotta tiles (10 cm × 10 cm × 1 cm), each fabricated with a 1 cm-diameter hole in the center, were preconditioned in tanks fitted with flow-through of 0.2 μm filtered FSW for 3 months to develop biofilms that facilitate coral larval settlement (Harrison and Wallace, 1990). Conditioned tiles, only containing biofilm were placed at the bottom of settlement trays in 0.2 μm filtered FSW. Collected larvae were added to the trays and allowed to settle on the upper surface of the tiles for 24 h. The numbers of settled and living spat on each tile were counted under a dissecting microscope and only tiles containing between 8 and 65 non-fused settled spat were used for the study. A total of 38 tiles were labeled and placed in flow-through tanks 3 days before they were deployed on the reef.
Individuals of Sargassum sp. and Bryopsis sp. were used for this experiment as these algae are locally abundant and have been shown to reduce post-settlement survivorship in previous studies (Lee et al., 2012; Goh and Lim, 2015). The study was conducted on the fringing reef crest and flat of Sister’s Island where Sargassum sp. and Bryopsis sp. were relatively abundant on the reef crest and flat, respectively (≥10 individuals per site). Sargassum sp. was uncommon on the reef flat whereas Bryopsis sp. were largely absent on the reef crest, preventing us from carrying out a transplant or an algal exclusion experiment involving both species in both habitats. Ten Sargassum sp. individuals, nine Bryopsis sp. individuals and their equivalent number of control plots (i.e., plots of absent of algal individuals) were selected and tagged. Tiles containing settled spats were assigned to either algal or control patches and carefully attached to the reef substratum with stainless steel nails and bolts. For the algal treatment, the attached tiles were placed within 5 cm of individual alga to maximize physical contact. In each reef habitat, all control and treatment tiles were placed within a meter from each other, placed at the same depth, and on similar substrate composition to account for similarity in environmental conditions (e.g., light irradiance and wave exposure) and to minimize the possibility of micro-site differences. Treatments in each habitat (i.e., four levels in total) had a comparable number of recruits per tile at the start of the study (ANOVA, F3,34 = 0.792, p = 0.508).
Tiles were left for 3 weeks and photographed at the start, and at the end of the study. Tiles were subsequently removed, placed into individual, sealable plastic bags. All live spat were counted within 2 h of retrieval. The number of surviving spat was counted for each tile by the end of the study. Wet sediment on each tile was brushed off into re-sealable plastic bags, decanted using 45 μm pre-weighed filter sheets, dried (at 100°C) and weighed to obtain dry sediment mass. The fouling community cover on each tile was quantified from photographs using Coral Point Count with Excel extensions [CPCe] (2006) software (version 3.2) using a random-25 point grid. The benthic categories quantified on each tile included: epilithic algal matrix (EAM), CCA, macroalgae, bare space (absence of fouling organisms) and other living organisms (e.g., bryozoans, keelworms, sponges, etc.).
Generalized linear models were used to measure effect of macroalgae i.e., “treatment type” [control vs. macroalgae presence (Sargassum sp. and Bryopsis sp.)] on the survivorship of the newly settled spats. The “habitat type” (crest vs. flat), and its interaction with “treatment type” were included in the model to account for habitat effects. Post hoc Tukey analyses were carried out for the significant categorical covariates. A separate, generalized linear model was also used to compare survivorship of spat across continuous covariates (i.e., dry sediment biomass, dry algal biomass and benthic cover percentages). This was due to the presence of collinearity between several categorical and continuous variables (e.g., turf cover was significantly higher in the Sargassum sp. compared to Bryopsis sp. treatment; two sample T-test; t = 7.707, df = 36, p < 0.001). The response variable in the models, survivorship of spat, assumed a binomial distribution with a logit link function (i.e., “live” vs. “dead” for each spat). Initial spat count density was found to be positively associated to density-dependent survivorship (sensu Vermeij et al., 2009; Doropoulos et al., 2017) (see Supplementary Data 2A,C) and hence were included into both models as a continuous predictor variable. Sediment dry mass data were log (x + 1) transformed while all benthic cover percentages were square-root transformed prior to the analysis to reduce skewness. All continuous covariates were normalized across their respective means and standard deviations prior to analyses. Prior to all analyses, we performed data exploration as suggested by Zuur et al. (2009) and model validation using plots of residuals vs. fitted values as per Zuur and Ieno (2016). Generalized linear models (functions “generalized linear model” followed by “Anova” from “car” package; Fox and Sanford, 2011) and Tukey analyses (“glht” function from “multcomp” package; Hothorn et al., 2008) were performed with R (version, 3.1.1) (R Core Team, 2014).
The Effect of in situ Herbivore Exclusion on Survival of Juvenile Corals
For the exclusion experiment, 120 fabricated terracotta tiles, with identical specifications to tiles used in the experiment above (Section B), were deployed at ∼1 m intervals along the reef crest at Kusu Island at 3–4 m depth in November 2012. After 7 months, 41 tiles, each containing 1–3 naturally settled live P. acuta juveniles on the upper surface were located in situ with the aid of a Nightsea FL-1 fluorescent dive light, and sides of each tile were tagged. The geometric mean diameter (GMD) of each coral was measured with Vernier calipers and locations of each coral on tiles were mapped. Tiles were randomly divided into three treatments: full cage (n = 13 tiles), partial cage (n = 13 tiles) and no cage (n = 15 tiles) with comparable mean GMDs across all treatments (controls = 6.0 ± 2.7 mm, partial cages = 6.4 ± 3.7 mm, full cages = 6.6 ± 3.1 mm; ANOVA, F2,54 = 0.204, p = 0.816).
Full cages were constructed of stainless steel wire mesh (1.2 mm thickness and 10 mm mesh size) with each side measuring 30 cm. Partial cages had the same dimensions but consisted of two open sides and a closed top. Partial cages acted as procedural controls to physical artifacts of the full cage treatments (e.g., potential shading and reduced water flow). All cages were attached to the substrate surrounding individual tiles, monitored at intervals of 1, 3, 6, and 8 weeks and cleaned after monitoring to avoid excessive fouling. Damaged cages were immediately replaced with new cages. Newly settled corals were recorded but not monitored. Fouling EAM and macroalgae on each tile were not disturbed throughout the experimental period. Differences in incident light intensity (lux) across treatments were measured during monitoring surveys, with Hobo Pendant loggers and were not statistically significant between treatments (10 randomly sampled plots per treatment (2–3 per treatment per session), 1 h per treatment, approximately 1200 to 1300 h; ANOVA, F2,38 = 0.826, p = 0.445).
Fish bite rates on partially caged tiles and controls were measured using underwater video cameras (GoPro Hero 3) mounted on aluminum angle iron bars ∼20 cm away from each tile. One partial and one control tile were randomly chosen for monitoring each time for approximately 2 h between 1200 and 1400 which resulted in 21.7 and 20.6 h of footage, respectively. Each fish bite on tiles were counted and bite rates were calculated as bites h−1. Where possible, fish were identified to species.
At the end of the experiment, sediments on each tile were brushed off into re-sealable plastic bags, decanted using 45 μm filter sheets, dried (at 100°C) and weighed. To estimate dry algal biomass, all algae (EAM and macroalgae) on each tile were scraped off and dried at 65°C. Photographs of tiles were taken at the start (week 0), middle (week 3) and end (week 8) of the experiment and were used to quantify change in benthic community cover with CPCe (version 3.2) using the same calculation method for benthic groups from Section B.
Generalized linear models were used to examine the effects of caging treatments on the survivorship of juvenile corals. Initial juvenile count per tile did not contribute to density-dependent mortality (Vermeij et al., 2009; Doropoulos et al., 2017) (see Supplementary Data 3A) therefore was excluded in the models. Post hoc Tukey test was used to compare the 8-week survivorships amongst all caging treatments. Survivorship of the corals was also modeled as a function of all continuous covariates using a generalized linear model. Dry sediment and algal mass data were log (x + 1) transformed prior to the model analyses. Similar to Section B’s model analyses, data exploration and model validation sensu Zuur et al. (2009) and Zuur and Ieno (2016) were also carried out. Bite rates were found to be heteroskedastic and non-normal, even after the transformation. Therefore, the non-parametric Mann–Whitney U-test was used to compare differences in bite rates between control and partially caged tiles throughout the experiment duration. Changes in each benthic category cover were also compared across treatments with ANOVA tests with additional Tukey tests when necessary. ANOVAs, generalized linear models, and Tukey tests were carried out with the same R software and packages as listed in Section B. Additional information on all analyses for the caging experiment can be found in Supplementary Data 3.
Results
The Effects of Macroalgae on Settlement of Coral Larvae
Settlement of P. acuta larvae differed significantly among algal treatments on all surfaces (ANOVA, F6,105 = 3.656, p < 0.001) and on surfaces excluding settlement on macroalgae (ANOVA, F6,105 = 5.646, p < 0.001). Excluding direct settlement on macroalgae, settlement was significantly higher in the control treatment (77.7 ± SE 4.1%) compared to larvae exposed to B. pennata (47.7 ± SE 6.5%), L. variegata (45.4 ± SE 3.6%), Padina sp. (45.0 ± SE 5.1%) and S. siliquosum (48.4 ± SE 7.0%) (post hoc Tukey HSD tests, p < 0.05, see Figure 1 and Supplementary Data 1A). With the inclusion of settlement on macroalgae, settlement on Sargassum sp. increased by 25.4 to 73.7 ± SE 5.1% and was comparable to controls. The total settlement for B. pennata, L. variegata, and Padina sp. was still significantly lower than on controls even with the addition of larvae settling directly on macroalgae.
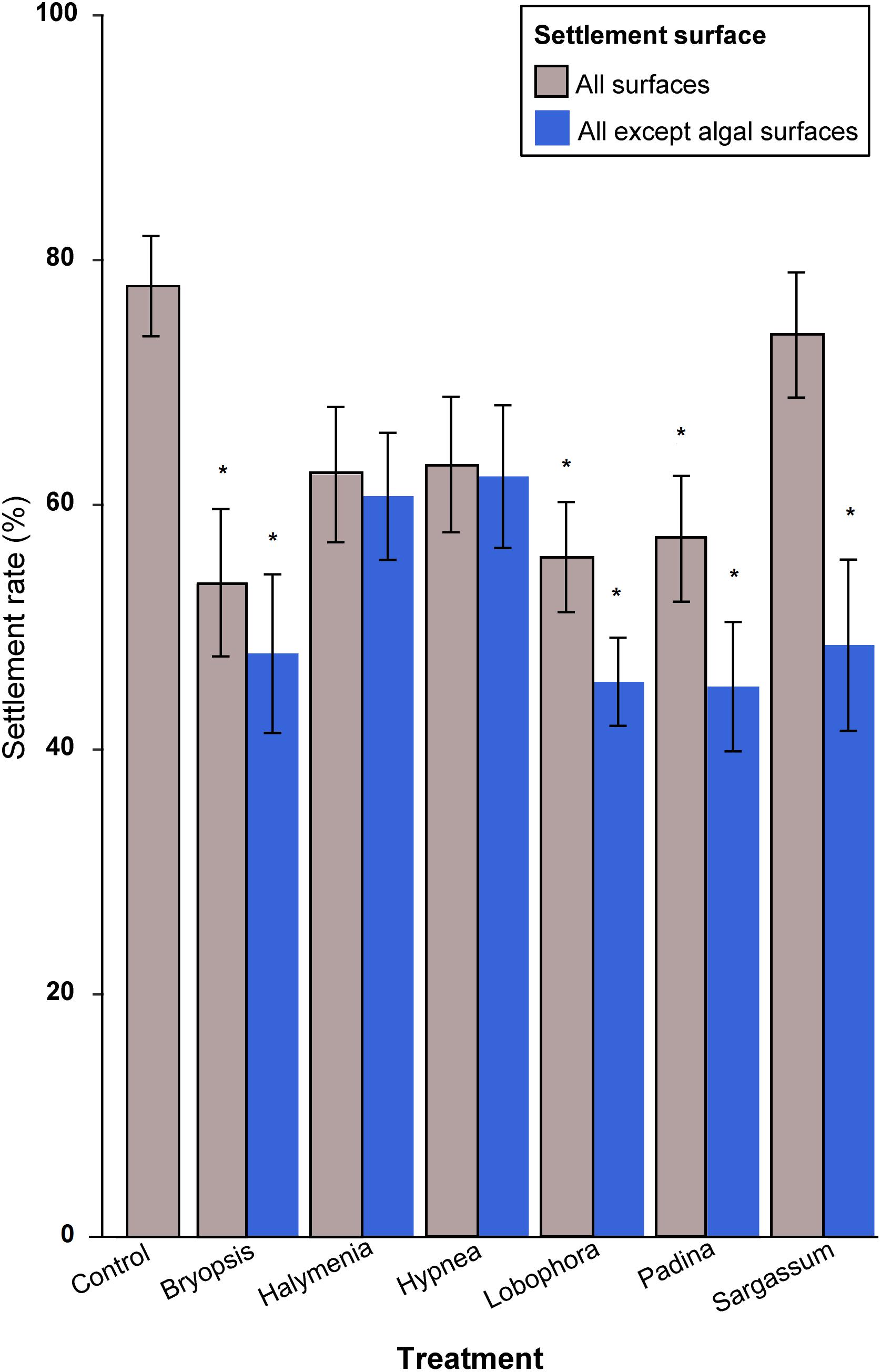
FIGURE 1. Mean percentage larval settlement across all treatment groups for all algae substrate treatments. All macroalgae species labels in this figure are simplified to their respective genus names. The symbol ∗ denotes significant differences in settlement percentages in comparison to the control treatment (post hoc Tukey HSD; p-value < 0.05) in all surfaces (gray bars) and settlement excluding leaf blade surfaces (blue bars), respectively. Error bars represent standard errors (±SE).
Effects of Macroalgae on Early Post-settlement Mortality of Corals in situ
There was a significant interaction between habitat types and treatments (LRT χ2 = 10.318, df = 1, p < 0.001, Supplementary Data 2A-ii). Post hoc Tukey tests (Figure 2 and Supplementary Data 2B) revealed differences in mortality rates between spat interacting with Sargassum sp. (20.0 ± SE 6.7%) and spat in control treatments on the reef crest (50.0 ± SE 8.6%). Survivorship of spat was not significantly different between Bryopsis sp. (14.3 ± SE 5.6%) and control treatments on the reef flat (16.6 ± SE 3.9%). There were significant differences in survival rates between habitats for control treatments (i.e., reef crest vs. flat; 50.0 ± SE 8.6% vs. 16.6 ± SE 3.9%; p < 0.001) but not for algal treatments (20.0 ± SE 6.7% at reef crest vs. 14.3 ± SE 5.6% at reef flat; p = 0.988). Among continuous variables, only CCA (slope = 0.304, p < 0.001) and other living organisms covers were positively correlated with survivorship of coral spat (slope = 0.224, p = 0.002). There was no significant relationship between EAM cover and survivorship of spat (slope = −0.165, p = 0.215) (see Supplementary Data 2C for generalized linear model analysis with continuous variables).
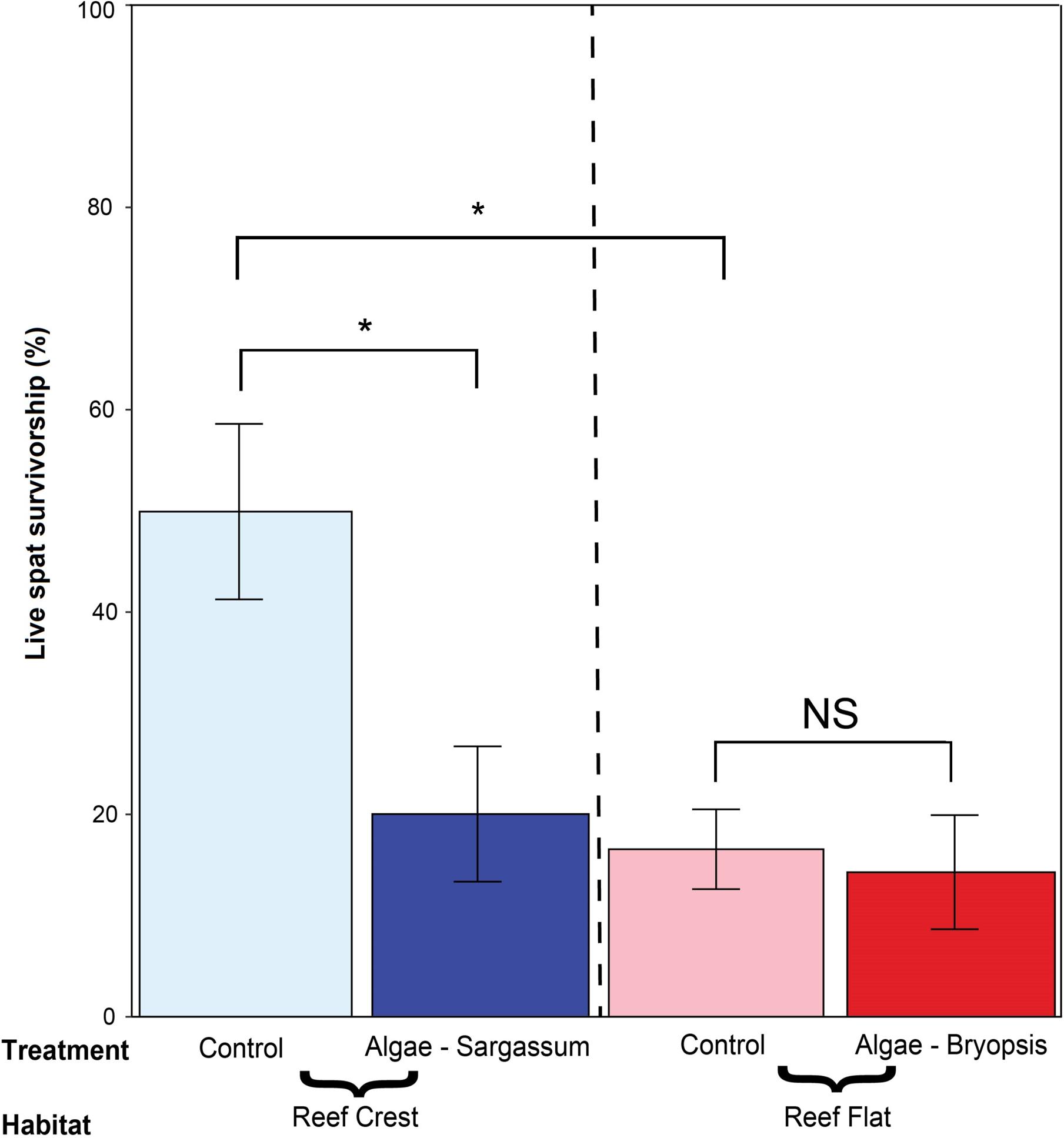
FIGURE 2. Mean percentage of survivorship of spat across habitat types (Reef Crest vs. Rest Flat) and algal treatments (Bryposis sp. or Sargassum sp. vs. control). Error bars represent standard errors (±SE). The symbol ‘∗’ and NS denote significant and non-significant differences, respectively, across pair-wise, post hoc Tukey comparison of variable levels at Bonferroni corrected (α = 0.05/4).
The Effect of in situ Herbivore Exclusion on Survival of Juvenile Corals
Caging had a significant effect on juvenile coral survivorship (LRT χ2 = 6.690, df = 1, p = 0.031) with significantly lower survivorship in the full cages (F; 30.8%) compared to the uncaged controls (C; 80.0%) (Post hoc Tukey HSD; F ≠ C, Z = −2.396, p = 0.044, Supplementary Data 3B and Figure 3). There were no differences in survivorship between partially caged plots (P; 65.4%) and uncaged controls (P = C, p = 0.709), or between caged and partial caged plots (F = P, p = 0.185) (Figure 3). Juvenile corals were not affected by density dependence through the initial spat number (Z = −1.018, p = 0.309, Supplementary Data 3A). Fish bite rates, however, were significantly higher on control tiles in comparison to partial caged tiles (4.6 ± SE 5.1 vs. 0.5 ± SE 0.7 bites h−1; Mann–Whitney-U-test, W = 117, p = 0.031, Figure 4B). The fish grazers observed consisted of three species: Halichoeres sp., an invertivorous wrasse, was responsible for 90% of total bites (91 bites); Pomancentrus littoralis, a herbivorous damselfish, which took 9% of total bites (9 bites); and Chaetodontoplus mesoleucus, an omnivorous angelfish, which took 1% (1 bite) of the total bites.
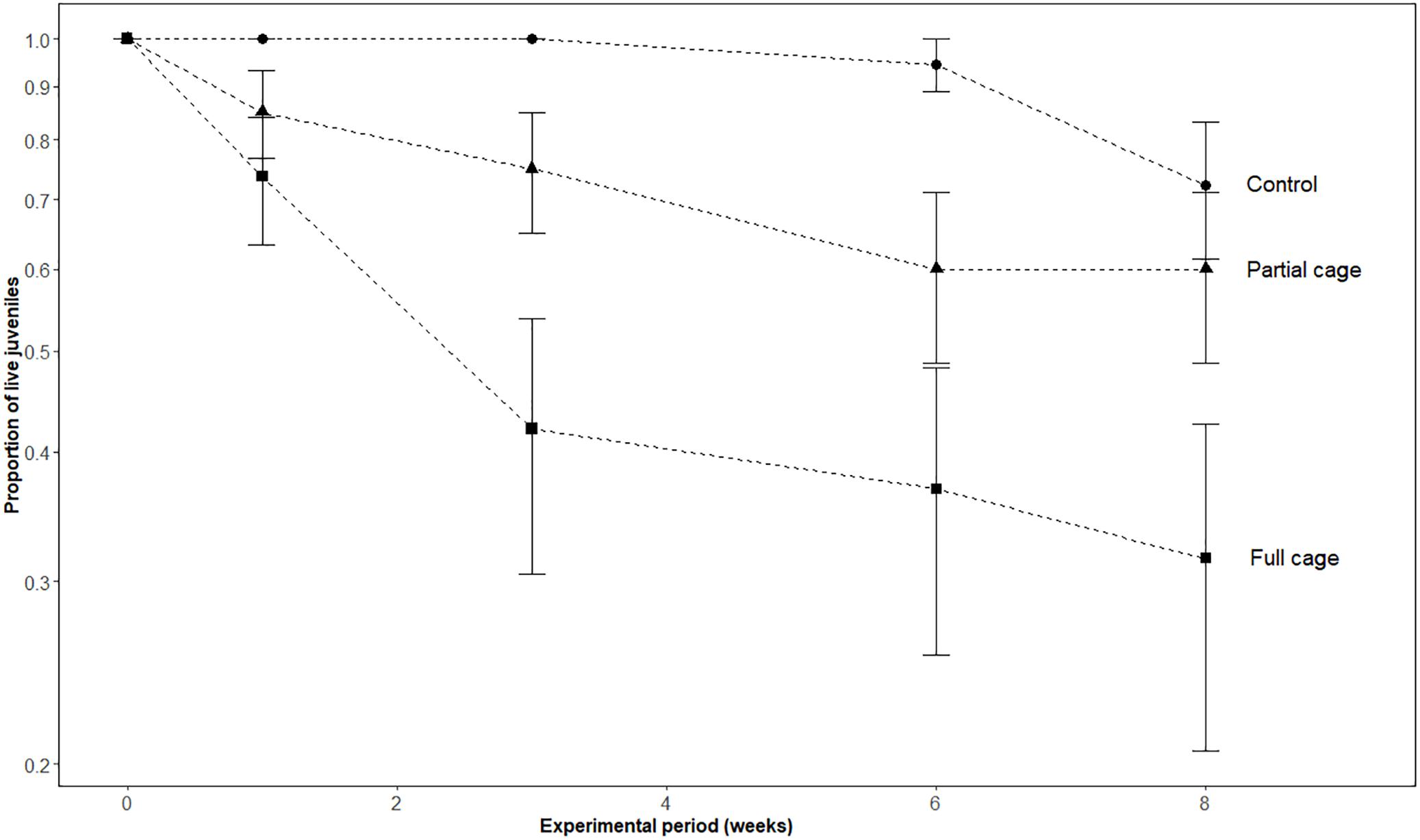
FIGURE 3. Proportion of live coral juveniles across caging treatments during the experimental period. Points and error bars correspond to mean proportion and standard errors (± SE), respectively, at each monitoring period.
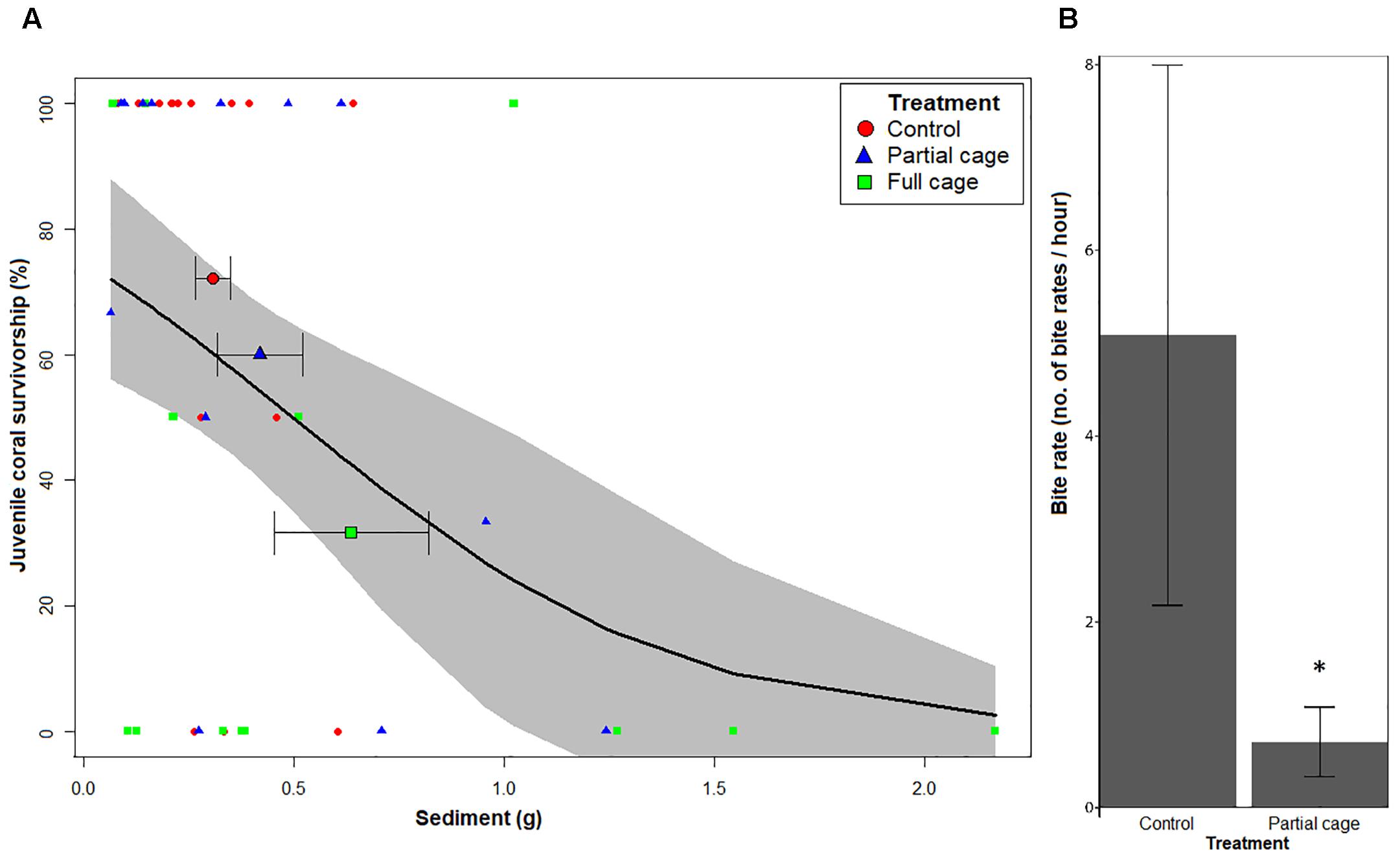
FIGURE 4. (A) Survivorship of juvenile corals against dry sediment mass in the fitted generalized linear model. Bold line and shaded region represent the fitted generalized linear model and its 95% confidence interval (CI). Red circles, blue triangles and green squares represent the raw sediment mass and coral survivorship on each tile according to control, partial cage and full cage treatments, respectively. Enlarged bold points for each treatment represent the mean survivorship treatment and sediment mass. Horizontal error bars represent standard errors (± SE) for corresponding mean sediment mass. The symbol ‘∗’ denotes variable showing a significant association to coral survivorship at α = 0.05 in the model. (B) Mean bite rates across caging treatment levels. The symbol ‘∗’ denotes significant differences in bite rates in partial cages in comparison to control tiles (Mann–Whitney-U-test; p-value < 0.05). Error bars are ± SE.
Among the continuous covariates, there was an association between juvenile coral mortality and dry sediment mass (slope = −1.183, p = 0.030, Figure 4A and Supplementary Data 3C), but no association with algal biomass (slope = −0.079, p = 0.876), EAM (slope = −0.348, p = 0.471), macroalgal cover (slope = −0.611, p = 0.264) or change in cover of other living organisms (slope = −0.218, p = 0.606). There were no significant differences across the caging treatments for either dry sediment mass (ANOVA, F2,38 = 0.564, p = 0.573) or dry algal biomass (ANOVA, F2,38 = 1.479, p = 0.241). There were also no significant differences in change of benthic cover across all treatments and benthic categories (refer to analyses and results in Supplementary Data 3C).
Discussion
With increasing human impact through the Anthropocene era, more coral reefs will be subjected to the types of disturbances found in Singapore and in other urbanized reefs (Heery et al., 2018). It is therefore essential to better understand the factors affecting coral recruitment success in these systems across multiple life history phases to mitigate reef degradation and manage restoration. Our study demonstrates that a combination of direct settlement inhibition and increased availability of unsuitable settlement substratum may contribute to significant reductions in successful coral settlement in the presence of macroalgae. Survival rates of newly settled coral spat were significantly lower when in physical contact with Sargassum sp. on the reef crest, but not with Bryopsis sp. on the reef flat, although overall mortality rates were higher on the reef flat. Exclusion of grazers led to higher mortality rates of juvenile corals in situ despite relatively low numbers of fish bite recorded, suggesting that fish grazing may still play an important role in coral recruitment success even in areas with relatively low biomass of herbivores (Guest et al., 2016b; Bauman et al., 2017). Our study, therefore, implies the need to monitor changes in the abundance of macroalgae in Singapore’s reefs and other urbanized reefs, and potentially place mitigation measures to control the proliferation of certain taxa (e.g., Sargassum).
Lower larval settlement in the presence of macroalgae corroborate previous studies showing that interaction with macroalgae can inhibit settlement (Kuffner et al., 2006; Vermeij et al., 2009; Paul et al., 2011; Dixson et al., 2014). Bryopsis, Lobophora, Padina, and Sargassum spp. have all been shown to impact settlement and metamorphosis of larvae of several coral species including Isopora palifera, Stylophora pistillata (Baird and Morse, 2004), Porites astreoides (Kuffner et al., 2006), Platygyra daedalea (Diaz-Pulido et al., 2010), Pocillopora damicornis (Maypa and Raymundo, 2004), and P. acuta (Lee et al., 2012) to varying degrees either in the field or ex situ. Nevertheless, in these studies, direct settlement on algal fronds was either not quantified or was negligible. Therefore, macroalgae such as Sargassum may have two types of effects on coral recruitment: firstly, they reduce the probability of settlement; and secondly, they can serve as a substrate for settlement. However, given the fact that algae is not a stable substratum, the settled coral is unlikely to survive to adulthood (Nugues and Szmant, 2006; Olsen et al., 2016). Therefore, even if overall settlement rates are not reduced, recruitment may be negatively impacted. Clearly, further studies are needed to confirm the extent that corals settle directly on Sargassum sp. by quantifying in situ, natural rates of settlement and post-settlement survival of corals on algal fronds.
Larvae from brooding corals are often competent to settle soon after release (Nozawa and Harrison, 2005) and may be less discriminating in terms of settlement preferences compared to larvae from broadcast spawners (Olsen et al., 2016; Ritson-Williams et al., 2016). Therefore, our study can offer important insights into potential mechanisms determining recruitment success for brooding corals on highly disturbed reefs. Further studies with larvae from broadcast spawning corals are required to test the generality of these responses. In Singapore, the reduced settlement rates due to interaction with Sargassum sp. has important implications for coral recruitment success as Sargassum spp. are the most abundant macroalgal taxa on these reefs (Low, 2015). Indeed, reefs with the lowest abundance of Sargassum were found to have the highest natural settlement rates and adult abundance of P. acuta in Singapore (Bauman et al., 2015, 2017) suggesting a negative role for Sargassum sp. in recruitment success for this coral species (Maypa and Raymundo, 2004).
A number of manipulative field experiments have demonstrated that physical contact with macroalgae can negatively impact juvenile and adult coral health (McCook et al., 2001; Ritson-Williams et al., 2009; Rasher and Hay, 2010a,b). For example, survivorship of newly settled Acropora millepora spat was reduced by almost 80% in 9 months in caged plots dominated by Sargassum sp. (Webster et al., 2015). Mechanisms by which macroalgae can damage corals are less well understood, but include physical damage due to abrasion, allelopathy (McCook, 2001) and indirectly through changes in microbial activity or composition (Smith et al., 2006; Nelson et al., 2013) upon algal-coral contact (Nugues et al., 2004). In our study, Sargassum sp. effect on newly settled spat on the reef crest may have been due to water-flow assisted abrasion as this taxa has leathery and cortified fronds capable of physically damaging newly settled corals (Maypa and Raymundo, 2004; Birrell et al., 2008). While we cannot rule out the possibility of chemical effects on newly settled spats, a number of studies have failed to find allelopathic effects by Sargassum sp. on corals (Rasher and Hay, 2010b; Rasher et al., 2011; Bonaldo and Hay, 2014), suggesting an important role of physical contact by Sargassum sp. to the survivorship newly settled corals. Control tiles were placed in areas devoid of algae but similar in environmental conditions as treatment tiles. We cannot, however, rule out the possibility that macroalgae may grow in specific micro-sites that are unfavorable to coral spat survival and this contributed to the differences in survival. Our results also demonstrate the importance of habitat in determining survivorship of newly settled spat. For example, there was a clear reduction (30%) in survivorship of spat between controls and treatments at the reef crest; however, the same was not true for control and treatment tiles on the reef flat. Although differences may also be due to different algal species, the clear difference in survivorship of spat between control tiles at the reef flat (14.3 ± SE 5.6%) and the reef crest (50.0 ± SE 8.6%) strongly suggest that local habitat processes in each habitat are equally important in determining survival rates of newly settled spat.
Removal of benthic algae by herbivores encourages coral post-settlement survival (Jompa and McCook, 2002) and there is a demonstrable positive relationship between herbivore biomass and recovery rates on many coral reefs (Gilmour et al., 2013; Graham et al., 2015). Paradoxically, grazing by some fishes may actively remove new coral spat (Raimondi and Morse, 2000; Rotjan and Lewis, 2008; Penin et al., 2010). Several studies have shown that excluding herbivores actually improves the survival of newly settled spat (Gleason, 1996; Baria et al., 2010; White and O’Donnell, 2010). Our study found that fish exclusion increased juvenile coral mortality by almost 50%, suggesting an imporatnt role for fish grazing in juvenile coral survival in the study system. Algal cover and biomass in caged plots increased in comparison to non-caged plots, however, the differences were not significant. Furthermore, algal abundance did not emerge as a significant explanatory variable, which suggests that macroalgae alone was not the main driver of increased juvenile coral mortality. It is possible that a non-significant increase in dry algal mass in caged plots might have an effect on the amount of trapped sediments, which in turn may reduce survivorship of juvenile corals (Birrell et al., 2005).
Contrasting results, in terms of juvenile coral survival, among herbivore exclusion studies most likely result from differences in coral age classes, life histories and the types and abundance of grazers in each study location. In studies that have shown positive effects of caging on spat survival (e.g., Baria et al., 2010), either spat were newly settled, making them more vulnerable to removal, or on coral reefs where large scraping or excavating parrotfish were abundant (e.g., Trapon et al., 2013). In our study, corals already had an average diameter of 6.3 mm at the start of the study, and therefore are likely to have exceeded a size vulnerable to grazers (Doropoulos et al., 2012). In addition, Singapore’s reefs have low abundances of sea urchins, large scaping parrotfish and relatively low grazing rates (Guest et al., 2016b). In the present study 90% of bites on tiles were carried out by Halichoeres sp.. Coupled with the absence of graze marks (pers. obs), our results suggest these fishes were opportunistically picking epiphytes off the tiles. Nevertheless, the results of our caging experiment suggest that even low rates of “grazing” by non-obligate herbivores has a positive effect on juvenile coral survival, likely through cascading effects from predation of invertebrates (Dulvy et al., 2004) and occasional removal of sediment and EAM surrounding corals (Birrell et al., 2005; Goatley and Bellwood, 2010). Our results highlight the importance of maintaining diverse fish populations on heavily impacted reefs. The precise role of herbivores, specifically, small non-obligate species such as damselfishes, in controlling algal turfs in turbid environments needs to be better understood and potentially these species may need to be considered for protection alongside larger browsing species (Bauman et al., 2017).
Author Contributions
JG, PS, and RL to the experimental design. CL, AB, EL, JL, and RL did the field and laboratory work. EM and RL analyzed the data. RL and JG did the drafting. All authors revised the manuscript.
Funding
Rehabilitation of Singapore’s reefs using sexually reared corals.
Conflict of Interest Statement
The authors declare that the research was conducted in the absence of any commercial or financial relationships that could be construed as a potential conflict of interest.
Acknowledgments
All research was carried out with a research grant from National Parks Board, Singapore and under Permit no. NP/RP11-073. We are thankful to Aaron and Juward Ho from South East (S.E.A) Aquarium of Resorts World Sentosa for the provision of aquaria and assistance with the aquaria setup. We are grateful for logistics and administration assistance from Melvin Lee (Dolphin Explorer) and Ricky Lim from Advanced Environmental Biotechnology Center. We would also like to thank Dr. Adriana Vergés (University of New South Wales) and Dr. Alexandra Campbell (University of the Sunshine Coast) for valuable advice about experimental design.
Supplementary Material
The Supplementary Material for this article can be found online at: https://www.frontiersin.org/articles/10.3389/fmars.2018.00385/full#supplementary-material
References
Arnold, S. N., Steneck, R. S., and Mumby, P. J. (2010). Running the gauntlet: inhibitory effects of algal turfs on the processes of coral recruitment. Mar. Ecol. Prog. Ser. 414, 91–105. doi: 10.3354/meps08724
Babcock, R., and Mundy, C. (1996). Coral recruitment: consequences of settlement choice for early growth and survivorship in two scleractinians. J. Exp. Mar. Biol. Ecol. 206, 179–201. doi: 10.1016/S0022-0981(96)02622-6
Babcock, R. C., Baird, A. H., Piromvaragorn, S., Thomson, D. P., and Willis, B. L. (2003). Identification of scleractinian coral recruits from Indo-Pacific reefs. Zool. Stud. 42, 211–226.
Baird, A. H., and Morse, A. N. C. (2004). Induction of metamorphosis in larvae of the brooding corals Acropora palifera and Stylophora pistillata. Mar. Freshw. Res. 55, 469–472. doi: 10.1071/MF03121
Baria, M. V. B., Guest, J. R., Edwards, A. J., Aliño, P. M., Heyward, A. J., and Gomez, E. D. (2010). Caging enhances post-settlement survival of juveniles of the scleractinian coral Acropora tenuis. J. Exp. Mar. Biol. Ecol. 394, 149–153. doi: 10.1016/j.jembe.2010.08.003
Bauman, A. G., Guest, J. R., Dunshea, G., Low, J., Todd, P. A., and Steinberg, P. D. (2015). Coral settlement on a highly disturbed equatorial reef system. PLoS One 10:e0127874. doi: 10.1371/journal.pone.0127874
Bauman, A. G., Hoey, A. S., Dunshea, G., Feary, D. A., Low, J., and Todd, P. A. (2017). Macroalgal browsing on a heavily degraded, urbanized equatorial reef system. Sci. Rep. 7:8352. doi: 10.1038/s41598-017-08873-3
Birrell, C. L., McCook, L. J., and Willis, B. L. (2005). Effects of algal turfs and sediment on coral settlement. Mar. Pollut. Bull. 51, 408–414. doi: 10.1016/j.marpolbul.2004.10.022
Birrell, C. L., Mccook, L. J., Willis, B. L., and Diaz-Pulido, G. A. (2008). Effects of benthic algae on the replenishment of corals and the implications for the resilience of coral reefs. Oceanogr. Mar. Biol. Annu. Rev. 46, 25–63. doi: 10.1201/9781420065756.ch2
Bonaldo, R. M., and Hay, M. E. (2014). Seaweed-coral interactions: variance in seaweed allelopathy, coral susceptibility, and potential effects on coral resilience. PLoS One 9:e85786. doi: 10.1371/journal.pone.0085786
Box, S. J., and Mumby, P. J. (2007). Effect of macroalgal competition on growth and survival of juvenile Caribbean corals. Mar. Ecol. Prog. Ser. 342, 139–149. doi: 10.3354/meps342139
Brock, R. E. (1979). An experimental study on the effects of grazing by parrotfishes and role of refuges in benthic community structure. Mar. Biol. 51, 381–388. doi: 10.1007/BF00389216
Browne, N. K., Tay, J. K. L., Low, J., Larson, O., and Todd, P. A. (2015). Fluctuations in coral health of four common inshore reef corals in response to seasonal and anthropogenic changes in water quality. Mar. Environ. Res. 105, 39–52. doi: 10.1016/j.marenvres.2015.02.002
Cleary, D. F. R., Polónia, A. R. M., Renema, W., Hoeksema, B. W., Wolstenholme, J., Tuti, Y., et al. (2014). Coral reefs next to a major conurbation: a study of temporal change (1985-2011) in Coral cover and composition in the reefs of Jakarta. Indonesia. Mar. Ecol. Prog. Ser. 501, 89–98. doi: 10.3354/meps10678
Coral Point Count with Excel extensions [CPCe]. (2006). A Visual Basic program for the determination of coral and substrate coverage using random point count methodology. Comput. Geosci. 32, 1259–1269. doi: 10.1016/j.cageo.2005.11.009
Corlett, R. T. (1992). The ecological transformation of Singapore, 1819-1990. J. Biogeogr. 19, 411–420. doi: 10.2307/2845569
De’ath, G., Fabricius, K. E., Sweatman, H., and Puotinen, M. (2012). The 27-year decline of coral cover on the great barrier reef and its causes. Proc. Natl. Acad. Sci. U.S.A. 109, 17995–17999. doi: 10.1073/pnas.1208909109
Deevey, E. S. (1947). Life tables for natural populations of animals. Q. Rev. Biol. 22, 283–314. doi: 10.1086/395888
Diaz-Pulido, G., Harii, S., McCook, L. J., and Hoegh-Guldberg, O. (2010). The impact of benthic algae on the settlement of a reef-building coral. Coral Reefs 29, 203–208. doi: 10.1007/s00338-009-0573-x
Dixson, D. L., Abrego, D., and Hay, M. E. (2014). Chemically mediated behavior of recruiting corals and fishes: a tipping point that may limit reef recovery. Science 345, 892–897. doi: 10.1126/science.1255057
Doropoulos, C., Evensen, N. R., Gómez-Lemos, L. A., and Babcock, R. C. (2017). Density-dependent coral recruitment displays divergent responses during distinct early life-history stages. R. Soc. Open Sci. 4:170082. doi: 10.1098/rsos.170082
Doropoulos, C., Roff, G., Bozec, Y. M., Zupan, M., Werminghausen, J., and Mumby, P. J. (2016). Characterizing the ecological trade-offs throughout the early ontogeny of coral recruitment. Ecol. Monogr. 86, 20–44. doi: 10.1890/15-0668.1
Doropoulos, C., Ward, S., Marshell, A., Diaz-Pulido, G., and Mumby, P. J. (2012). Interactions among chronic and acute impacts on coral recruits: the importance of size-escape thresholds. Ecology 93, 2131–2138. doi: 10.1890/12-0495.1
Dsikowitzky, L., Ferse, S., Schwarzbauer, J., Vogt, T. S., and Irianto, H. E. (2016). Impacts of megacities on tropical coastal ecosystems - the case of Jakarta. Indonesia. Mar. Pollut. Bull. 110, 621–623. doi: 10.1016/j.marpolbul.2015.11.060
Dulvy, N. K., Freckleton, R. P., and Polunin, N. V. C. (2004). Coral reef cascades and the indirect effects of predator removal by exploitation. Ecol. Lett. 7, 410–416. doi: 10.1111/j.1461-0248.2004.00593.x
Edwards, C. B., Friedlander, A. M., Green, A. G., Hardt, M. J., Sala, E., Sweatman, H. P., et al. (2014). Global assessment of the status of coral reef herbivorous fishes: evidence for fishing effects. Proc. R. Soc. B Biol. Sci. 281:20131835. doi: 10.1098/rspb.2013.1835
Fabricius, K. E. (2005). Effects of terrestrial runoff on the ecology of corals and coral reefs: review and synthesis. Mar. Pollut. Bull. 50, 125–146. doi: 10.1016/j.marpolbul.2004.11.028
Fox, J., and Sanford, W. (2011). An {R} Companion to Applied Regression, 2nd Edn, Thousand Oaks, CA: Sage.
Gilmour, J. P., Smith, L. D., Heyward, A. J., Baird, A. H., and Pratchett, M. S. (2013). Recovery of an isolated coral reef system following severe disturbance. Science 340, 69–71. doi: 10.1126/science.1232310
Gleason, M. G. (1996). Coral recruitment in Moorea, French Polynesia:the importance of patch type and temporal variation. J. Exp. Mar. Biol. Ecol. 207, 79–101. doi: 10.1016/s0022-0981(96)02647-0
Goatley, C. H. R., and Bellwood, D. R. (2010). Biologically mediated sediment fluxes on coral reefs: sediment removal and off-reef transportation by the surgeonfish Ctenochaetus striatus. Mar. Ecol. Prog. Ser. 415, 237–245. doi: 10.3354/meps08761
Goh, B. P. L., and Lim, D. Y. F. (2015). Distribution and abundance of sea urchins in Singapore reefs and their potential ecological impacts on macroalgae and coral communities. Ocean Sci. J. 50, 211–219. doi: 10.1007/s12601-015-0018-0
Graham, N. A. J., Jennings, S., MacNeil, M. A., Mouillot, D., and Wilson, S. K. (2015). Predicting climate-driven regime shifts versus rebound potential in coral reefs. Nature 518, 94–97. doi: 10.1038/nature14140
Guest, J. R., Baird, A. H., Maynard, J. A., Muttaqin, E., Edwards, A. J., Campbell, S. J., et al. (2012). Contrasting patterns of coral bleaching susceptibility in 2010 suggest an adaptive response to thermal stress. PLoS One 7:e33353. doi: 10.1371/journal.pone.0033353
Guest, J. R., Tun, K., Low, J., Vergés, A., Marzinelli, E. M., Campbell, A. H., et al. (2016a). 27 years of benthic and coral community dynamics on turbid, highly urbanised reefs off Singapore. Sci. Rep. 6:36260. doi: 10.1038/srep36260
Guest, J. R., Vergés, A., Bauman, A. G., Campbell, A. H., Chou, L. M., Feary, D. A., et al. (2016b). Examining the relationship between fish herbivore biomass, coral and macroalgal cover on Singapore’s heavily disturbed reefs. PeerJ [Preprint]. doi: 10.7287/peerj.preprints.1907v2
Harrison, P. L., and Wallace, C. C. (1990). “Reproduction, dispersal and recruitment of scleractinian corals,” in Ecosystems of the World: Coral Reefs, ed. Z. Dubinsky (Amsterdam: Elsevier), 132–207.
Heery, E. C., Hoeksema, B. W., Browne, N. K., Reimer, J. D., Ang, P. O., Huang, D., et al. (2018). Urban coral reefs: degradation and resilience of hard coral assemblages in coastal cities of East and Southeast Asia. Mar. Pollut. Bull. 135, 654–681. doi: 10.1016/j.marpolbul.2018.07.041
Heyward, A. J., and Negri, A. P. (1999). Natural inducers for coral larval metamorphosis. Coral Reefs 18, 273–279. doi: 10.1007/s003380050193
Hothorn, T., Bretz, F., and Westfall, P. (2008). Simultaneous inference in general parametric models. Biom. J. 50, 346–363. doi: 10.1002/bimj.200810425
Huang, D., Tun, K. P. P., Chou, L. M., and Todd, P. A. (2009). An inventory of zooxanthellate scleractinian corals in Singapore, including 33 new records. Raffles Bull. Zool. 22, 69–80.
Hughes, T. P. (1994). Catastrophes, phase shifts, and large-scale degradation of a Caribbean coral reef. Science 265, 1547–1551. doi: 10.1126/science.265.5178.1547
Hughes, T. P., Anderson, K. D., Connolly, S. R., Heron, S. F., Kerry, J. T., Lough, J. M., et al. (2018). Spatial and temporal patterns of mass bleaching of corals in the Anthropocene. Science 359, 80–83. doi: 10.1126/science.aan8048
Hughes, T. P., Rodrigues, M. J., Bellwood, D. R., Ceccarelli, D., Hoegh-Guldberg, O., McCook, L., et al. (2007). Phase shifts, herbivory, and the resilience of coral reefs to climate change. Curr. Biol. 17, 360–365. doi: 10.1016/j.cub.2006.12.049
Jompa, J., and McCook, L. J. (2002). Effects of competition and herbivory on interactions between a hard coral and a brown alga. J. Exp. Mar. Biol. Ecol. 271, 25–39. doi: 10.1016/S0022-0981(02)00040-0
Kuffner, I. B., Walters, L. J., Becerro, M. A., Paul, V. J., Ritson-Williams, R., and Beach, K. S. (2006). Inhibition of coral recruitment by macroalgae and cyanobacteria. Mar. Ecol. Prog. Ser. 323, 107–117. doi: 10.3354/meps323107
Lee, C., Walford, J., and Lee, B. (2012). “The effect of benthic macroalgae on coral settlement,” in Contributions to Marine Science: a Commemorative Volume Celebrating 10 Years of Research on St John’s Island, ed. K. S. Tan (Singapore: National University of Singapore), 89–93.
Lee, C. S., Walford, J., and Goh, B. P. L. (2009). Adding coral rubble to substrata enhances settlement of Pocillopora damicornis larvae. Coral Reefs 28, 529–533. doi: 10.1007/s00338-009-0467-y
Low, J. (2015). Sargassum on Singapore’s Reefs. Available at: https://scholarbank.nus.edu.sg/bitstream/10635/118571/1/Sargassum%20on%20Singapore%20reefs_thesis_edited_20150107.pdf [Accessed October 14, 2017].
Manikandan, B., and Ravindran, J. (2017). Differential response of coral communities to Caulerpa spp. bloom in the reefs of Indian Ocean. Environ. Sci. Pollut. Res. 24, 3912–3922. doi: 10.1007/s11356-016-8136-5
Maypa, A. P., and Raymundo, L. J. (2004). Algae-coral interactions: mediation of coral settlement, easrly survival, and growth by macroalgae. Silliman J. 45, 76–95.
McCook, L. (2001). Competition between corals and algal turfs along a gradient of terrestrial influence in the nearshore central great barrier reef. Coral Reefs 19, 419–425. doi: 10.1007/s003380000119
McCook, L. J., Jompa, J., and Diaz-Pulido, G. (2001). Competition between corals and algae on coral reefs: a review of evidence and mechanisms. Coral Reefs 19, 400–417. doi: 10.1007/s003380000129
Mwachireya, S. A., Nzioka, A. M., and Mutiso, D. N. (2017). Coral recruit-algae interactions in coral reef lagoons are mediated by riverine influences. Int. J. Ecol. 2017:1351854. doi: 10.1155/2017/1351854
Nelson, C. E., Goldberg, S. J., Wegley Kelly, L., Haas, A. F., Smith, J. E., Rohwer, F., et al. (2013). Coral and macroalgal exudates vary in neutral sugar composition and differentially enrich reef bacterioplankton lineages. ISME J. 7, 962–979. doi: 10.1038/ismej.2012.161
Nozawa, Y., and Harrison, P. L. (2005). Temporal settlement patterns of larvae of the broadcast spawning reef coral Favites chinensis and the broadcast spawning and brooding reef coral Goniastrea aspera from Okinawa. Japan. Coral Reefs 24, 274–282. doi: 10.1007/s00338-005-0476-4
Nugues, M. M., Smith, G. W., Van Hooidonk, R. J., Seabra, M. I., and Bak, R. P. M. (2004). Algal contact as a trigger for coral disease. Ecol. Lett. 7, 919–923. doi: 10.1111/j.1461-0248.2004.00651.x
Nugues, M. M., and Szmant, A. M. (2006). Coral settlement onto Halimeda opuntia: a fatal attraction to an ephemeral substrate? Coral Reefs 25, 585–591. doi: 10.1007/s00338-006-0147-0
Olsen, K., Paul, V. J., and Ross, C. (2015). Direct effects of elevated temperature, reduced pH, and the presence of macroalgae (Dictyota spp.) on larvae of the Caribbean coral Porites astreoides. Bull. Mar. Sci. 91, 255–270. doi: 10.5343/bms.2014.1050
Olsen, K., Sneed, J. M., and Paul, V. J. (2016). Differential larval settlement responses of Porites astreoides and Acropora palmata in the presence of the green alga Halimeda opuntia. Coral Reefs 35, 521–525. doi: 10.1007/s00338-015-1394-8
Paul, V. J., Kuffner, I. B., Walters, L. J., Ritson-Williams, R., Beach, K. S., and Becerro, M. A. (2011). Chemically mediated interactions between macroalgae Dictyota spp. and multiple life-history stages of the coral Porites astreoides. Mar. Ecol. Prog. Ser. 426, 161–170. doi: 10.3354/meps09032
Penin, L., Michonneau, F., Baird, A. H., Connolly, S. R., Pratchett, M. S., Kayal, M., et al. (2010). Early post-settlement mortality and the structure of coral assemblages. Mar. Ecol. Prog. Ser. 408, 55–64. doi: 10.3354/meps08554
Poquita-Du, R., Ng, C. S. L., Loo, J., Bin Afiq-Rosli, L., Tay, Y. C., Todd, P., et al. (2017). New evidence shows that Pocillopora ‘damicornis-like’ corals in Singapore are actually Pocillopora acuta (Scleractinia: Pocilloporidae). Biodivers. Data J. 5:e11407. doi: 10.3897/BDJ.5.e11407
R Core Team (2014). R Foundation for Statistical Computing. Available at: http://www.r-project.org/ [Accessed April 15, 2017].
Raimondi, P. T., and Morse, A. N. C. (2000). The consequences of complexlarval behavior in a coral. Ecology 81, 3193–3211.
Rasher, D. B., and Hay, M. E. (2010a). Chemically rich seaweeds poison corals when not controlled by herbivores. Proc. Natl. Acad. Sci. U.S.A. 107, 9683–9688. doi: 10.1073/pnas.0912095107
Rasher, D. B., and Hay, M. E. (2010b). Seaweed allelopathy degrades the resilience and function of coral reefs. Commun. Integr. Biol. 3, 564–566. doi: 10.4161/cib.3.6.12978
Rasher, D. B., Stout, E. P., Engel, S., Kubanek, J., and Hay, M. E. (2011). Macroalgal terpenes function as allelopathic agents against reef corals. Proc. Natl. Acad. Sci. U.S.A. 108, 17726–17731. doi: 10.1073/pnas.1108628108
Raymundo, L. J., and Maypa, A. P. (2004). Getting bigger faster: mediation of size-specific mortality via fusion in juvenile coral transplants. Ecol. Appl. 14, 281–295. doi: 10.1890/02-5373
Ritson-Williams, R., Arnold, S. N., Fogarty, N. D., Steneck, R. S., Vermeij, M. J. A., and Paul, V. J. (2009). New perspectives on ecological mechanisms affecting coral recruitment on reefs. Smithson. Contrib. Mar. Sci. 38, 437–457. doi: 10.5479/si.01960768.38.437
Ritson-Williams, R., Arnold, S. N., and Paul, V. J. (2016). Patterns of larval settlement preferences and post-settlement survival for seven Caribbean corals. Mar. Ecol. Prog. Ser. 548, 127–138. doi: 10.3354/meps11688
Rotjan, R. D., and Lewis, S. M. (2008). Impact of coral predators on tropical reefs. Mar. Ecol. Prog. Ser. 367, 73–91. doi: 10.3354/meps07531
Smith, J. E., Shaw, M., Edwards, R. A., Obura, D., Pantos, O., Sala, E., et al. (2006). Indirect effects of algae on coral: algae-mediated, microbe-induced coral mortality. Ecol. Lett. 9, 835–845. doi: 10.1111/j.1461-0248.2006.00937.x
Strader, M. E., Davies, S. W., and Matz, M. V. (2015). Differential responses of coral larvae to the colour of ambient light guide them to suitable settlement microhabitat. R. Soc. Open Sci. 2:150358. doi: 10.1098/rsos.150358
Tebben, J., Guest, J. R., Sin, T. M., Steinberg, P. D., and Harder, T. (2014). Corals like it waxed: paraffin-based antifouling technology enhances coral spat survival. PLoS One 9:e87545. doi: 10.1371/journal.pone.0087545
Tebben, J., Motti, C. A., Siboni, N., Tapiolas, D. M., Negri, A. P., Schupp, P. J., et al. (2015). Chemical mediation of coral larval settlement by crustose coralline algae. Sci. Rep. 5:10803. doi: 10.1038/srep10803
Todd, P. A., Ladle, R. J., Lewin-Koh, N. J. I., and Chou, L. M. (2004). Genotype x environment interactions in transplanted clones of the massive corals Favia speciosa and Diploastrea heliopora. Mar. Ecol. Prog. Ser. 271, 167–182. doi: 10.3354/meps271167
Trapon, M. L., Pratchett, M. S., Hoey, A. S., and Baird, A. H. (2013). Influence of fish grazing and sedimentation on the early post-settlement survival of the tabular coral Acropora cytherea. Coral Reefs 32, 1051–1059. doi: 10.1007/s00338-013-1059-4
Van Maren, D. S., Liew, S. C., and Hasan, G. M. J. (2014). The role of terrestrial sediment on turbidity near Singapore’s coral reefs. Cont. Shelf Res. 76, 75–88. doi: 10.1016/j.csr.2013.12.001
Venera-Ponton, D. E., Diaz-Pulido, G., McCook, L. J., and Rangel-Campo, A. (2011). Macroalgae reduce growth of juvenile corals but protect them from parrotfish damage. Mar. Ecol. Prog. Ser. 421, 109–115. doi: 10.3354/meps08869
Vermeij, M. J. A., Dailer, M. L., and Smith, C. M. (2011). Crustose coralline algae can suppress macroalgal growth and recruitment on hawaiian coral reefs. Mar. Ecol. Prog. Ser. 422, 1–7. doi: 10.3354/meps08964
Vermeij, M. J. A., and Sandin, S. A. (2008). Density-dependent settlement and mortality structure the earliest life phases of a coral population. Ecology 89, 1994–2004. doi: 10.1890/07-1296.1
Vermeij, M. J. A., Smith, J. E., Smith, C. M., Vega Thurber, R., and Sandin, S. A. (2009). Survival and settlement success of coral planulae: independent and synergistic effects of macroalgae and microbes. Oecologia 159, 325–336. doi: 10.1007/s00442-008-1223-7
Webster, F. J., Babcock, R. C., Van Keulen, M., and Loneragan, N. R. (2015). Macroalgae inhibits larval settlement and increases recruit mortality at Ningaloo Reef. Western Australia. PLoS One 10:e0124162. doi: 10.1371/journal.pone.0124162
White, J. S., and O’Donnell, J. (2010). Indirect effects of a key ecosystem engineer alter survival and growth of foundation coral species. Ecology 91, 3538–3548. doi: 10.1890/09-2322
Zilberberg, C., and Edmunds, P. J. (2001). Competition among small colonies of Agaricia: the importance of size asymmetry in determining competitive outcome. Mar. Ecol. Prog. Ser. 221, 125–133. doi: 10.3354/meps221125
Zuur, A., Ieno, E. N., Walker, N., Saveliev, A. A., and Smith, G. M. (2009). Mixed Effects Models and Extensions in Ecology with R. New York, NY: Springer.
Keywords: coral recruitment, settlement, post-settlement mortality, sedimentation, urban reefs, Pocillopora acuta
Citation: Leong RC, Marzinelli EM, Low J, Bauman AG, Lim EWX, Lim CY, Steinberg PD and Guest JR (2018) Effect of Coral-Algal Interactions on Early Life History Processes in Pocillopora acuta in a Highly Disturbed Coral Reef System. Front. Mar. Sci. 5:385. doi: 10.3389/fmars.2018.00385
Received: 31 May 2018; Accepted: 01 October 2018;
Published: 26 October 2018.
Edited by:
Michael Sweet, University of Derby, United KingdomReviewed by:
Deron Burkepile, University of California, Santa Barbara, United StatesCraig W. Osenberg, University of Georgia, United States
Copyright © 2018 Leong, Marzinelli, Low, Bauman, Lim, Lim, Steinberg and Guest. This is an open-access article distributed under the terms of the Creative Commons Attribution License (CC BY). The use, distribution or reproduction in other forums is permitted, provided the original author(s) and the copyright owner(s) are credited and that the original publication in this journal is cited, in accordance with accepted academic practice. No use, distribution or reproduction is permitted which does not comply with these terms.
*Correspondence: Rick C. Leong, bGVvbmdjaGlucmlja0BnbWFpbC5jb20= James R. Guest, anJndWVzdEBnbWFpbC5jb20=