- 1Department of Chemistry and Biology, Ryerson University, Toronto, ON, Canada
- 2Environment & Climate Change Canada, Delta, BC, Canada
- 3Coastal & Ocean Resources, Victoria, BC, Canada
Intertidal biofilm is a thin layer of microbes and meiofauna enmeshed in an extracellular polymeric matrix within and on top of mudflat sediment. This medium provides a dynamic resource for a variety of consumers in estuarine habitats, and is rich in essential fatty acids that birds require for long-distance migration. We measured seasonal changes in biofilm fatty acid content from spring to summer on the Fraser River Estuary, one of the richest and most important ecosystems for migrant and wintering waterbirds in Canada. Fatty acid content in biofilm showed a strong seasonal pattern with a peak in the spring that is associated with the northward migration of Western Sandpipers (Calidris mauri) to their breeding grounds. This peak is linked to the abundance and physiological state, and hence nutritional condition, of epipelic diatoms, which, in turn, depend on a combination of mudflat topography, salinity, temperature, and nutrients that can fluctuate widely with the freshwater inputs from the Fraser River. Specifically, areas with higher elevation (i.e., ∼1 m) had longer periods of exposure to ambient conditions (i.e., light, warm temperatures, gas exchange into/out of biofilms) that facilitated more biofilm growth and higher fatty acid content. Moreover, springtime changes in water chemistry (i.e., salinity/osmotic stresses and nutrients) and temperature facilitated the production of higher overall total lipid/fatty acid contents in the mudflat biofilms compared to summer. Effective conservation of migrating shorebirds depends on the protection of underlying processes at important stopover sites that promote biofilm communities to escalate their production of lipids, including essential fatty acids, during key times of the year.
Introduction
North American avifauna have suffered a net loss approaching 3 billion birds, or ∼29% relative to 1970 abundance estimates, including steep declines in migratory shorebird species (Rosenberg et al., 2019). The authors attributed this collapse to the “loss of ecosystem integrity, function and services.” For migratory shorebirds, a key aspect of ecosystem integrity is the functional quality of the stopover locations that are so vital to the success of their annual migrations. Often these stopover sites are hotspots of biological diversity and productivity and, more often than not, occur in estuarine ecosystems (Butler et al., 2001). Given ongoing declines in bird numbers, it is of paramount importance, from a conservation perspective, to identify and delineate the most critical aspects underpinning the integrity of estuarine ecosystems for migrating shorebirds. Here, we seek to achieve this goal by linking the temporal and spatial dynamics of essential fatty acids, as available in the microphytobenthos, to the needs of migrating shorebirds.
Much of the primary productivity in estuarine environments comes from microphytobenthos (Underwood and Kromkamp, 1999), including biofilm, a thin layer of microbes and meiofauna embedded in an extracellular polymeric matrix that forms on intertidal mudflats. The individual microbial cells grow and reproduce within sediment pores (e.g., clay, silt, and sand) and on the top of the mudflat, and are exposed to ambient conditions during low-tide periods. Mudflat biofilms in the intertidal zone are grazed by marine invertebrates (Herman et al., 2000), fish (Carpentier et al., 2014), and, of key interest here, migratory shorebirds (Elner et al., 2005; Kuwae et al., 2008, 2012; Mathot et al., 2010; Jardine et al., 2015).
Algal taxa, particularly diatoms, are major sources of essential fatty acids for consumers in aquatic food webs (Kelly and Scheibling, 2012; Quinn et al., 2017). Although many consumers require, to various degrees, specific fatty acids, our focus is on the Western Sandpiper (Calidris mauri). Western Sandpipers extensively graze estuarine intertidal mudflat biofilm (Elner et al., 2005; Kuwae et al., 2008), which serves as a primary source of fatty acids to meet their energetic and physiological demands, particularly during their northward breeding migration each spring (Schnurr et al., 2019). Fats are a preferred migratory fuel because they represent a compact and energy-dense resource compared to carbohydrates and proteins (Guglielmo, 2010). During migration, 90% of the energy required by birds can be derived from lipids, primarily stored as saturated fatty acids (SFA) and monounsaturated fatty acids (MUFA; Guglielmo, 2010), directly or indirectly derived from algae. Further, algae are the main producers (globally) of critically important long-chain polyunsaturated fatty acids (LC-PUFA), including eicosapentaenoic acid (EPA; 20:5n–3) and docosahexaenoic acid (DHA; 22:6n–3) (Hixson et al., 2015). These PUFA are essential to many vertebrates, including birds (Viegas et al., 2017), which benefit from ingesting at least some PUFA (pre-formed) in their diets (Arts et al., 2001).
PUFA are also known to be physiologically important for long distance migration in birds. Consumption of PUFA can increase unsaturation levels of phospholipids in muscle cell membranes (Maillet and Weber, 2007; Weber, 2009), resulting in enhanced membrane fluidity (i.e., decreases in membrane lipid order), which increases permeability, including transmembrane lipid transport (Maillet and Weber, 2006; Weber, 2009). For example, high amounts of EPA and DHA in diets of Semipalmated Sandpipers (Calidris pusilla) enhance oxidative capacity in cellular mitochondria (Maillet and Weber, 2007), positively promoting energy availability required for long distance flights. When muscle cells of Sanderling (Calidris alba) were treated in vitro with n–3 PUFA supplementation, cells increased basal and maximal oxygen consumption (Young, 2019). In addition, PUFA are the precursors of hormones involved in inflammation and cell proliferation, which can facilitate muscle recovery over arduous migration periods (Price, 2010). Therefore, understanding the mechanisms by which estuarine habitats serve to furnish birds with these essential nutrients is essential to effective conservation of migration stopover sites.
Estuarine biofilm communities are dominated by diatoms (Underwood and Paterson, 1993), which can rapidly accumulate fatty acids under specific environmental conditions and growth phases (Schnurr et al., 2019). Under ideal growth conditions diatoms grow and reproduce maximally, causing them to rapidly produce membrane lipids (required for cell division), which consist mostly of phospholipids containing relatively higher proportions of PUFA. When environmental conditions change rapidly (i.e., as a result of an environmental stress), algal cells reduce their rate of cell division and instead store carbon fixed during photosynthesis as triacylglycerol (TAG) – which is comprised of a relatively higher proportion of SFA and MUFA – in lipid droplets located in the cytoplasm (Hu et al., 2008; Yi et al., 2014). For example, marine diatoms in coastal and estuarine ecosystems often experience osmotic stress (Kirst, 1989), and this stress (associated with sudden changes in salinity) induces a lipid/fatty acid accumulation response (Hu and Gao, 2006; Sharma et al., 2012; Mohan and Devi, 2014).
Here, we compared the organic content, and total lipid, chlorophyll-a, and fatty acid contents of intertidal biofilm during spring and summer; two time periods during which shorebirds are migrating northward and southward, respectively, through the Fraser River estuary, British Columbia, Canada. The way in which Western Sandpipers move through the Pacific Flyway differs between the two periods (O’Reilly and Wingfield, 1995; Franks et al., 2014). Northward migration is characterized by huge flocks that rapidly move through to the breeding grounds over narrow time windows (Warnock and Bishop, 1998; Drever et al., 2014). In contrast, southward migration occurs over a protracted period during which birds move through in smaller flocks at a slower pace (Butler et al., 1996). If migrating birds are broadly synchronizing the timing of their northward migration with the peak availability of fatty acids in estuarine biofilm, then seasonal changes in total lipid and fatty acid content should mirror the differences in shorebird use between spring and summer. Given that the realized fatty acid content of estuarine mudflat biofilms is likely a result of complex interactions among a variety of environmental conditions (Schnurr et al., 2019), our aim was to understand how spatial and temporal environmental conditions affect biofilm organic and fatty acid content during these two seasons. Specifically, we investigated how biofilm organic and fatty acid content in biofilms were affected by spatial variables such as mudflat elevation (and how that affects mudflat exposure time), proximity to shore, proximity to the outlet of the Fraser River, and by time-varying factors, including salinity, temperature, nutrient concentrations, and light conditions.
Materials and Methods
Sampling Dates
Sampling dates were selected to represent the spring (northward) and summer (southward) migration periods in 2017 for Western Sandpipers and Dunlin (Calidris alpina), another common shorebird. Sampling during spring migration was conducted during three campaigns: Campaign 1 occurred April 9–14, Campaign 2 occurred April 21–27, and Campaign 3 occurred May 10–18. Peak northward migration of Western Sandpipers in 2017 occurred around 28 April 2017 (MCD, unpublished data). As the southward migration period occurs over a longer timeframe (i.e., months) than the northward migration (Franks et al., 2014), the two final sampling campaigns were conducted a month apart (August 2–5 and September 5–9) during the summer. These sampling periods occurred during a mix of spring and neap tides (Supplementary Material).
Biofilm Sampling Locations
The Fraser River estuary is located south of the City of Vancouver (49.2827° N, 123.1207° W) in southwest British Columbia (Figure 1). It is one of the richest and most important ecosystems for migrant and wintering waterbirds in Canada (Butler and Campbell, 1987). Sampling locations (n = 24) (Figure 1) were determined according to the following criteria: (1) areas that Western Sandpipers are known to utilize for foraging during their stopover periods; and (2) areas across north-south and east-west transects to understand gradients of proximity to shore and proximity to freshwater river outlets within the estuary. A “spine” of sampling locations was set directly adjacent to provincial government monitoring sites so that we could share their salinity data. Temperature probes were deployed along a north-south transect of sampling locations. East-west transects were perpendicular to the main axis of the “spine” locations to determine whether there was an offshore-onshore spatial gradient in biofilm organic and fatty acid content (Figure 1).
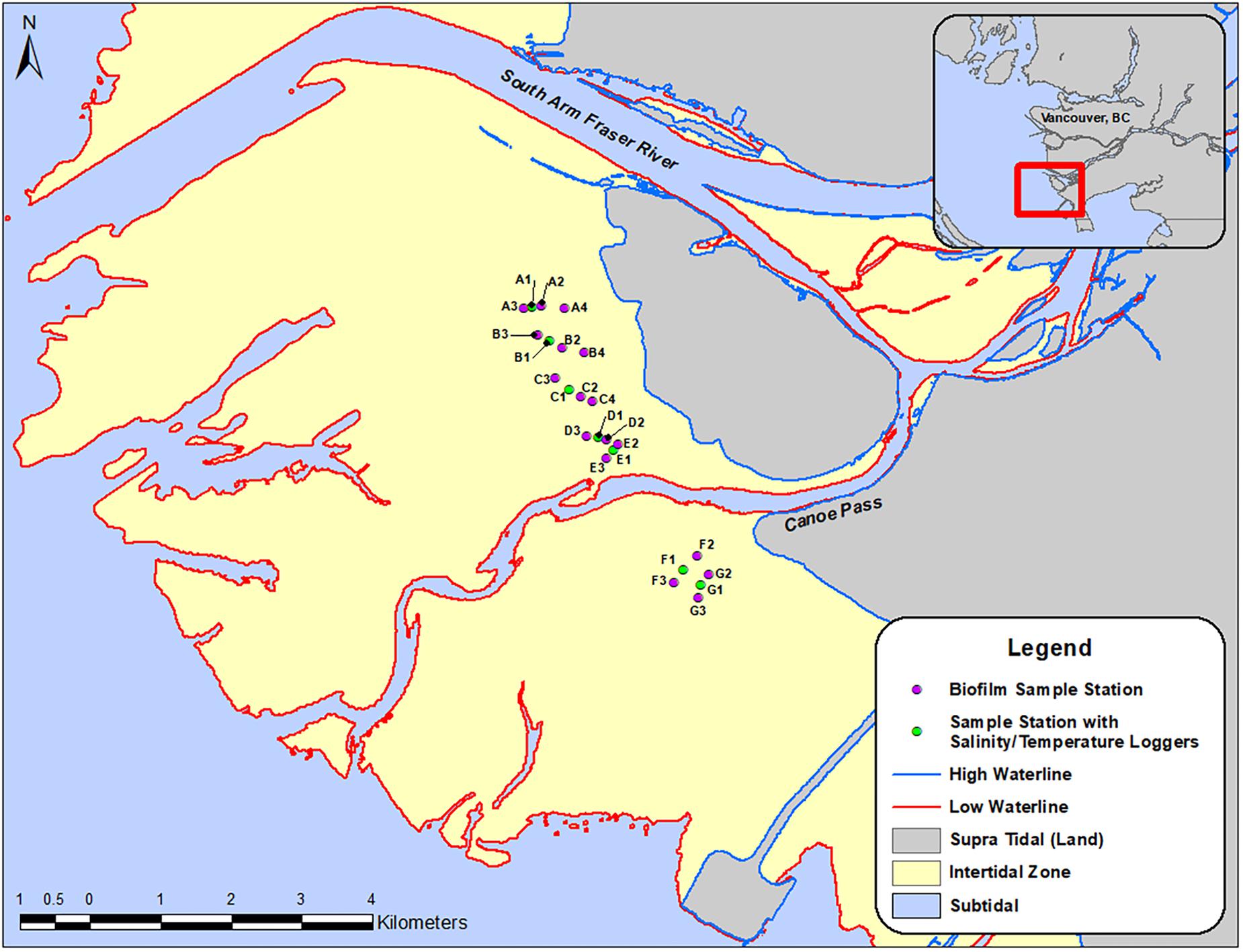
Figure 1. Map of the Roberts Bank study site, with symbols indicating biofilm-sampling locations, April-September 2017. Inset shows the region south of the city of Vancouver, British Columbia, Canada, and the location of the study area within the outflow of the south arm of the Fraser River. Round symbols indicate sampling stations for biofilm measures, and stations in red included monitoring units for salinity and temperature.
Field Sampling Procedures
Biofilm Sampling
Biofilm samples (n = 354) were collected during the five campaigns using the same standard procedure. All samples were collected as the mudflat was exposed during ebbing tides, but some were exposed longer (i.e., range of 10–180 min) than others. Upon arrival at each sampling site, a 2.5 × 2.5 m sampling quadrat was set-up on the ground. This quadrat was divided into a sampling grid, whereby the x- and y-axis had 10 equally spaced 25 cm segments. A random number generator was used to determine sampling locations (n = 3) within the quadrat using the segments as guides. Triplicate biofilm samples were taken, whereby a spatula was used to scrape the top ∼2 mm layer of the biofilm at each location, resulting in a ∼1,000 cm2 sampling area per biofilm sample. These biofilm samples were deposited into 50 ml FalconTM tubes and immediately stored in a cooler containing dry ice.
Water Sampling for Nutrient Analysis
Water samples were collected for dissolved nutrient analyses by attaching four 100 ml aluminum cans to each stake that marked a sampling location. The aluminum cans were attached ∼12, 25, 35, and 45 cm above the mud surface, so that, upon tidal flooding, each container would fill with water representative of that height and time. Once the tide receded the following day, the cans were removed and contents combined to create a composite sample representative of the flood period on each date at each location. Composite water samples (n = 118) were stored in a 4°C refrigerator until they were transported to the Pacific Environmental Science Centre, North Vancouver, British Columbia for analysis of macronutrients (soluble reactive phosphorus, nitrate, nitrite, and ammonia). A sub-volume of each sample (n = 118) was sent to the Canada Centre for Inland Waters (CCIW), Burlington, Ontario, for silica analyses.
Temperature and Salinity Data Collection
Temperature and salinity data were continuously collected along the spine (i.e., stations A1-G1; Figure 1). Alpha Mach iBWetLand 22L (model AM015) temperature sensors and data loggers recorded temperature at 15-min intervals, with a resolution of 0.1°C. Similarly, INW CT2X Conductivity Smart Sensor logged salinity data at 5-min intervals, with a resolution of 0.001 Practical Salinity Units (PSU). Dry zeros were excluded (i.e., when not inundated), and 5th, 50th, and 95th percentiles were calculated for each 24 h and 72-h period around each biofilm sample. Because all these values were strongly inter-correlated (Supplementary Material), we narrowed the list of possible variables by choosing measures related to specific biological hypotheses (Table 1).
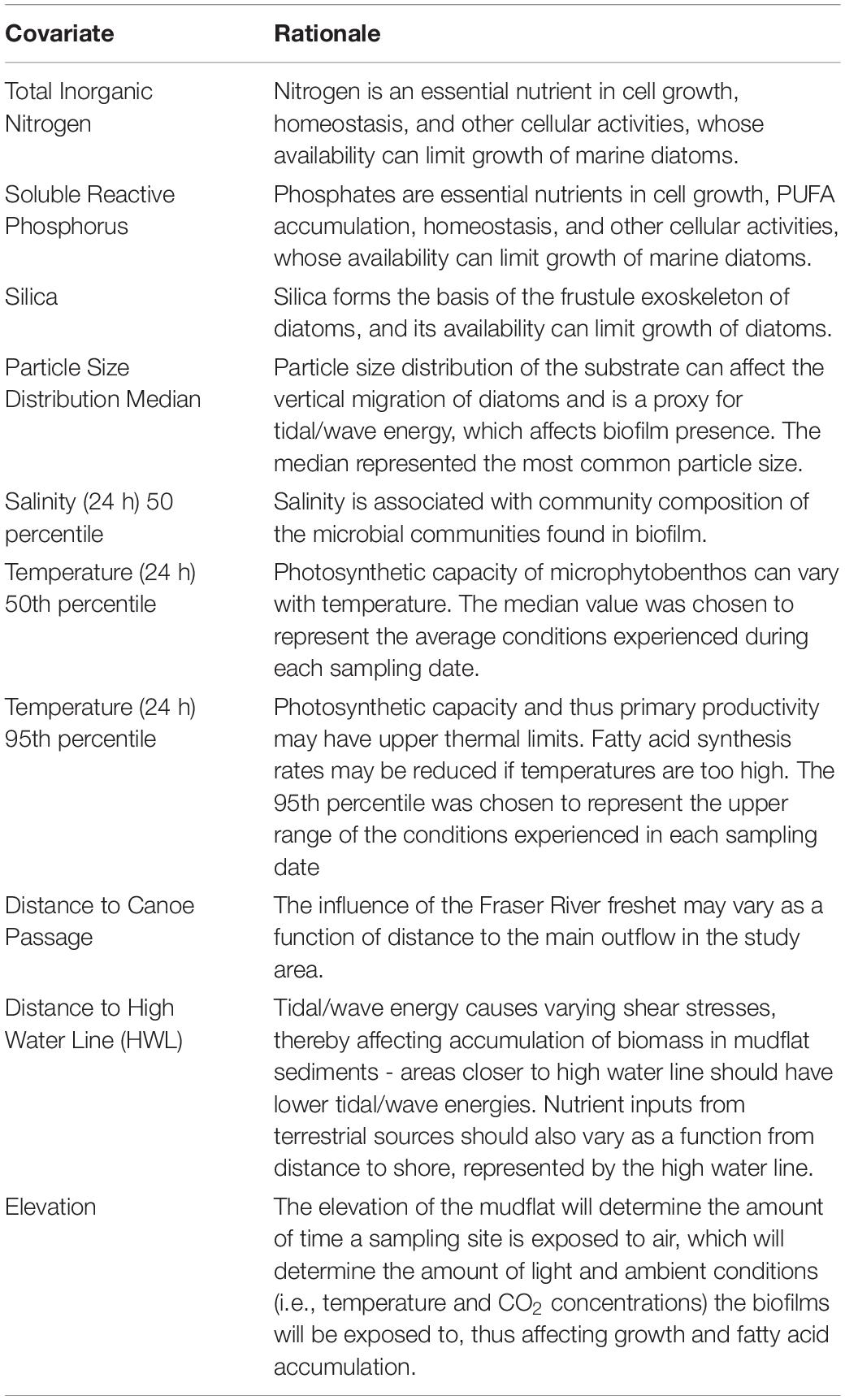
Table 1. Explanatory variables for modeling spatial and temporal variation in measures of biofilm organic and fatty acid content.
Elevation and Distance Measures
Sample locations were referenced to relevant geographic features using the geospatial tools in Esri ArcMap. Distances of sample locations from the high-water line (HWL) were estimated as the shortest distance from the Canadian Hydrographic Service (CHS) digital HWL to sample location. Distances from the midline of the Fraser River outflow were estimated from the shortest distance between the CHS digital low-water line (that delineated Canoe Passage) to sample location (Figure 1). Elevation measures were obtained as available from LiDAR and Orthophotos taken on the estuary during flights conducted on 23 July 2013. Elevation was determined as an average of the points surrounding each sample location, and corresponds to geodetic elevations in meters, using mean sea level as the zero value.
Laboratory Procedures and Analyses
Organic Content
All frozen (−80°C) biofilm samples were lyophilized for 2–3 days until completely dry in a freeze-drier (Labconco FreeZone 2.5). Dry biofilm samples were then homogenized using a porcelain mortar and pestle. Ash free dry weight (AFDW) of each sample was determined by taking ∼3 g subsample from the homogenized sample, and ashing them in an oven at 550°C for 24 h to volatize all organic material, and then re-weighing the samples to determine the difference in mass (i.e., before and after ashing the samples). Organic content was expressed as the percent of the difference from the before-sample.
Chlorophyll-a Content
A subsample of each biofilm sample was used to determine chlorophyll content using a procedure adapted from Arar (1997), and conducted in low lighting conditions to prevent photo-oxidation of extracted chlorophyll compounds. Depending on the organic fraction of each sample, a specific mass was weighed out to achieve ∼20 mg of organic biomass within the subsample. The chlorophyll within the subsample was then extracted with 10 mL of 9:1 acetone: water in a grinding tube exposed to grinding for 2 min at 500 rpm. Solution and solid biomass contents were transferred from the grinding tube to a centrifugation tube, whereby the sample steeped in a dark refrigerator (4°C) for 20–23 h. After steeping, samples were centrifuged for 15 min at 675 g, and the supernatant was analyzed using spectrophotometry (Agilent Cary 60 UV-Vis Spectrophotometer) and 1 cm3 quartz cuvette. A 9:1 acetone: water blank was used to zero the instrument before absorbance measurements were taken. Absorbance values were measured at 750, 664, 647, and 630 nm and applied to the equation [chlorophyll-a (mg/L) = 11.85 × abs664 nm – 1.54 × abs647 nm −0.08 × abs630 nm (Arar, 1997)]. Note, abs750 was subtracted from each of the absorbance measurements at the other three wavelengths for chlorophyll because abs750 is for suspended particles that may affect absorbance readings of chlorophyll pigments. Mass of chlorophyll within the extracted sample was determined by multiplying Chlorophyll-a concentration by volume of acetone: water used (10 mL), and that mass was normalized to both the mass of sample and the AFDW, as described above. These Chlorophyll-a values were not adjusted for phaeophytin levels.
Total Lipid and Fatty Acid Analysis
A subsample of each biofilm sample was used to determine total lipid and fatty acid content of mudflat biofilms. As with the chlorophyll sample sizes, each sample mass used equated to ∼20 mg of organic content when considering the AFDW (organic fraction) of the homogenized sample. The Folch extraction method (Folch et al., 1957) was used to extract the lipid from each of the samples. Tricosanoic acid (23:0; Nu-Chek Prep, Inc), which does not commonly occur naturally in this ecosystem, was used to quantify methylation efficiencies during the extraction and methylation procedures. The aqueous layer was discarded during the extraction because all fatty acids were dissolved in the chloroform layer. After extraction, the lipid-chloroform solution was evaporated under a nitrogen blanket before being re-suspended in 2.0 mL of hexane. Two-100 mL aliquots of the lipid-hexanes solution were taken to evaporate in a pre-weighed aluminum tin (∼0.25 mL). The mass difference after evaporation was used to gravimetrically determine the amount of total lipid in the biofilm samples.
The remaining lipid-hexanes solution was methylated with a 1% solution of H2SO4 in anhydrous methanol (2 mL), which was heat catalyzed for 90 min at 90°C (VWR Digital 2 block heater). After 90 min, a 1 mL aliquot of deionized water was added to samples to stop the reaction, and a 4 mL aliquot of hexanes was added to extract the fatty acids. The solution was then centrifuged at 2,500 rpm for 5 min to separate the hexanes and aqueous layers. The fatty acid-hexanes phase was separated from the aqueous phase with a Pasteur pipette, then dispensed into a clean centrifuge tube. Each sample was then re-extracted two more times with 4 mL hexane aliquots and combined into the sample in the centrifuge tube. Samples were once again evaporated until dry under a nitrogen blanket and re-suspended in 540 μL of hexanes. The final fatty acid-hexanes solution was pipetted into a Gas Chromatograph (GC) vial and stored at −80°C until analysis.
A Shimadzu GC2010 Plus GC, equipped with an AOC-20i autosampler and a Flame Ionization Detector (FID), was used to identify and quantify the fatty acids in biofilm samples. The GC was fitted with a Supelco SP-2560, 100 m × 0.25 mm column used for fatty acid methyl esters (FAME). Samples were injected in splitless mode. Initial column temperature was 60°C, which was then increased at 15°C per minute until 180°C, followed by 2°C per minute to 240°C. The column was held at 240°C for 5 min to elute all fatty acids potentially remaining in the column. Helium was the carrier gas with a flow rate of 1.2 mL/min. The injector and detector temperatures were both at 250°C through the duration of all sample runs. Fatty acids were identified by matching retention times from the FAME standard GLC-463 (Nu-Chek Prep, Inc) to the unknown peaks in the samples. Fatty acids were quantified by creating a series of dilutions of the FAME standard mix GLC-463 (Nu-Chek Prep, Inc), producing calibration curves for each of the fatty acids of interest after running them on the GC, then calculating the concentrations of the unknowns from the peak area of the fatty acid within the unknown. Methylation efficiencies were determined by comparing the theoretical amount of Tricosanoic Acid (23:0) added to each sample (10.2 μg) to the amount actually determined to be within each sample. All fatty acid mass fractions were then adjusted for methylation efficiencies, then normalized to both the mass of sample and the AFDW, as described above.
Particle Size Distribution Analysis
Particle size distribution analysis was conducted on samples collected in May, and assumed to have been constant throughout the study period. Analyses were conducted by the Department of Materials Science and Engineering, University of Toronto, based on several grams of lyophilized and homogenized samples. A Horiba Partica LA-950 Laser Diffraction Particle Size Analyzer was used, and summary statistics (median, mean, D10, D90) were derived for each location. D10 is the particle size diameter of the smallest grains (10th percentile of weight distribution), and D90 is the particle size diameter of the largest grains in the sample (90th percentile of weight distribution).
Water Sample Nutrient Analyses
Analysis for nutrients key to diatom growth were determined in the water column above each sampling site during tidal flood periods at the time of biofilm sampling. Before analyses, all samples were filtered with a 0.45 μm cellulose acetate membrane filter. Analyses for nitrite, nitrate and ammonia was colorimetric using Standard Method 4500-NO2-I (nitrite as nitrogen), Standard Method 4500-NO3-I (nitrate as nitrogen), and Standard Method 4500-NH3 H (ammonia as nitrogen), respectively, flow injection analysis, and Lachat Flow Injection System (APHA et al., 2017). Analysis for soluble reactive phosphorus was colorimetric using the Standard Method 4500-P F (phosphorus), a continuous flow analyzer method, and a Technicon AutoAnalyzer II System – segmented flow. Each analyte of interest within a sample was reacted with specific chemicals and analyzed at a wavelength specific to each chemical complex. The protocols for analysis, including the reactant chemicals and wavelengths used for colorimetric analysis are included in each method, which are cited in the References section. Total inorganic nitrogen (TIN) was calculated from a summation of the nitrate, nitrite, and ammonia data collected. Analyses for silica was conducted by Inductively Coupled Plasma-Optical Emission Spectrometry (ICP-OES) (Thermo Scientific; Model ICAP 6300 Duo) using standard solutions produced in the Environment and Climate Change Canada lab at the Canadian Centre for Inland Waters.
Data Analyses
Data Selection and Biofilm Variables
We used a series of eight variables to represent the organic, total lipid, and fatty acid content of biofilm. Organic content was expressed as the mass of the organic fraction divided by the mass of the sample weight (g/g). Total lipid and fatty acid content varied both as a fraction of the organic matter within the sample, and by the total amount of organic matter enmeshed in the sediment. Therefore, our metric for total lipid and fatty acid content (that would be available to foraging birds) was normalized per gram of sample, such that:
We summed the fatty acid mass fractions into major groups based on their saturation levels, including total values for SFA, MUFA, and PUFA, including n–3, and n–6 fatty acids. Results are presented on a per gram basis, and summary statistics on measures normalized to AFDW are included in the Supplementary Material.
We considered two different combinations of summed fatty acids to test two hypotheses about (1) physiological condition of diatoms, and (2) community composition of biofilm. First, we recognized that algal cells accumulate TAG when they stop or slow down dividing, e.g., during periods of stress (Sharma et al., 2012). Since cells are dividing at a reduced rate, they are not building/accumulating new membranes, which are primarily composed of PUFA-rich phospholipids. Thus, the ratio of (SFA + MUFA)/PUFA would be elevated during these stress periods, as diatoms would be expected to produce proportionately more TAG relative to phospholipids. A ratio with a value >2.0 would indicate a preferential accumulation of total SFA and MUFA in excess of that which would be predicted if all fatty acid groups were present in the same amounts. Second, we assessed proportions of specific fatty acids relative to total fatty acids as chemotaxonomic biomarkers for microbial community composition (Dalsgaard et al., 2003; Kelly and Scheibling, 2012). We used the following markers: the sum of 16:1n–7 + EPA for diatoms (Shin et al., 2008), the sum of 22:6n–3 (DHA) + 18:1n–9 for dinoflagellates (Kelly and Scheibling, 2012), 18:2n–6 for cyanobacteria, and the sum of odd-chain length fatty acids for bacteria (Kunihiro et al., 2014). We acknowledge the inherent uncertainty in using such biomarkers (i.e., some fatty acids may be found in several taxa within microphytobenthos), and treat any results with the understanding that they only provide broad indices of species turnover.
Selection of Covariates
We examined correlations among three datasets derived from three separate sources (biofilm measures, salinity and temperature values from station devices, and nutrient analysis measures) by calculating Pearson correlation matrices among all variables within each data source (Supplementary Material). From these correlations, we identified a series of response and explanatory variables to identify important spatial and seasonal covariates of biofilm organic and fatty acid content related to known or hypothesized relationships between biofilm, diatoms, and environmental conditions (Table 1).
To examine how the fatty acid content of the biofilm changed over time, we fitted a mixed effects model to each biofilm measure with sampling date as a categorical explanatory variable. Sampling station was included as a random effect to account for the repeated measures design, and controlled for consistent spatial differences. Biofilm measures were loge-transformed to normalize residuals. We tested for significance of the date term with a Type III Analysis of Variance (i.e., using Type III sums of squares) using the Satterthwaite’s method [package lmerTest in R (Kuznetsova et al., 2017)], and a critical alpha level of P = 0.00625 to accommodate for multiple tests on correlated dependent variables. For each model, we checked the residuals for normality and heteroscedasticity. To differentiate whether biofilm measures varied among sampling dates, we conducted Tukey’s all-pair comparisons using the glht function in package multcomp in R (Hothorn et al., 2008), and used a compact letter display to reveal groupings of data that were not statistically different.
Correlations With Covariates
We estimated the effect of each covariate individually using a mixed effects modeling approach. A mixed effects model was fitted with each biofilm measure as the response variable, including each covariate as a fixed effect in a model that also included date and sampling station as separate categorical random effects. All covariates were centered and scaled by subtracting the mean and dividing by the standard deviation (i.e., z-score), which allowed inferences about relative effect sizes by directly comparing parameter estimates. Because salinity and temperature data were only available for a subset of the stations their inclusion in a combined model would have resulted in loss of significant quantities of data related to other covariates, and therefore this approach using a series of tests allowed us to make best use of available data.
We tested for significance of each covariate with a t-test using the Satterthwaite’s method [package lmerTest in R (Kuznetsova et al., 2017)]. For each model, we checked the residuals for normality and heteroscedasticity, and calculated pseudo-R2 values (Nakagawa et al., 2017) using package piecewiseSEM (Lefcheck, 2016) in R. Two pseudo-R2 values were derived for each model: the marginal R2 considers only the variance explained by the fixed effects, and the conditional R2 by both the fixed and random effects.
Results
Temporal Trends in Biofilm Measures
The organic content of the sampled sediment ranged between 2 and 3% of the total sample mass, with a tendency toward higher values in August and September (Figure 2). Total lipid varied between a mean of 608 and 719 μg/g biofilm during April and May, respectively, and then declined to 450–237 μg/g biofilm in August and September, respectively. Median Chlorophyll-a values ranged from 32 to 47 μg/g biofilm with no clear seasonal pattern.
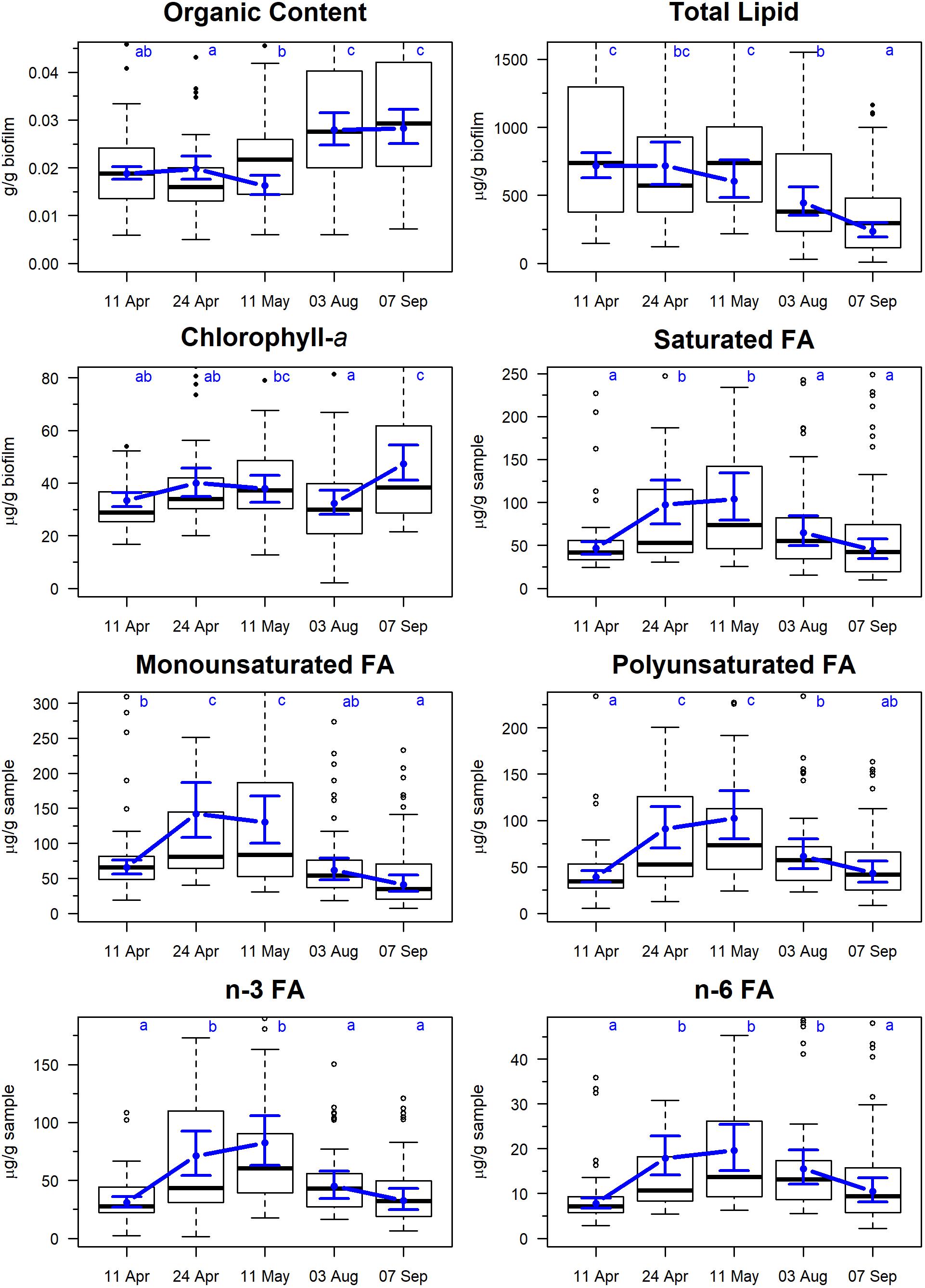
Figure 2. Temporal changes in biofilm measures on Roberts Banks mudflats located on the Fraser River estuary, British Columbia, April-September 2017. Biofilm measures are expressed in units of per 1 g of sample, including inedible sediment. Note that y-axis extends from 0 to 0.90 percentile of each measure to avoid compression of scale from outliers. Box plots represent the distribution of observed values, where midline is the median, with the upper and lower limits of the box being 75th and 25th percentiles. Whiskers extend up to 1.5 × the interquartile range, and outliers are depicted as points. Blue circles indicate predicted means from linear mixed effects models, and bounds are 95% predictions intervals from fixed effects. Letters indicate groupings that are not statistically significantly different according to Tukey’s pairwise comparisons.
The five most abundant fatty acids found in biofilm during spring/summer sampling included 16:0 (palmitic acid), 16:1n–7 (palmitoleic acid) 20:5n–3 (EPA), 22:3n–3 (docosatrienoic acid), and 22:6n–3 (DHA) (Table 2). All summed measures of fatty acid content varied widely by date, with highest values observed on April 24 and May 11 (Figure 2; F4,325 > 15.2, p < 0.0001). For example, SFA had a mean value of 46 μg/g biofilm on 11 April, which doubled to 98 and 104 μg/g biofilm on 24 April and 11 May, respectively. Total SFA then declined to 64 and 44 μg/g biofilm during the August and September sample dates, similar to values observed in early spring (Figure 2). This same pattern of an approximate doubling in measures of fatty acid content from values observed in early spring to late April and May, followed by a decline to summer and early fall period (August/September), was apparent in all the summed measures of fatty acids, including SFA, MUFA, PUFA, n–3, and n–6 fatty acids (Figure 2).
The (SFA + MUFA)/PUFA ratio varied strongly by sampling date (F4,325 = 25.8, p < 0.0001) ranging from 2.3 to 2.9 in spring to 1.9–2.1 in summer. Confidence intervals for summer values of the ratio overlapped 2.0, indicating that summed SFA and MUFA were not being preferentially accumulated during August and September.
The biomarkers for microbial community composition also showed strongly seasonal variation (Figure 3; F4,325 = 4.8, p < 0.001). The marker for diatoms varied from 40 to 44% during the spring samples, and then dropped to ∼36% during summer samples. This change was accompanied by concurrent increases in the markers for dinoflagellates, cyanobacteria and bacteria, indicating a shift in the community composition of biofilm between spring and summer.
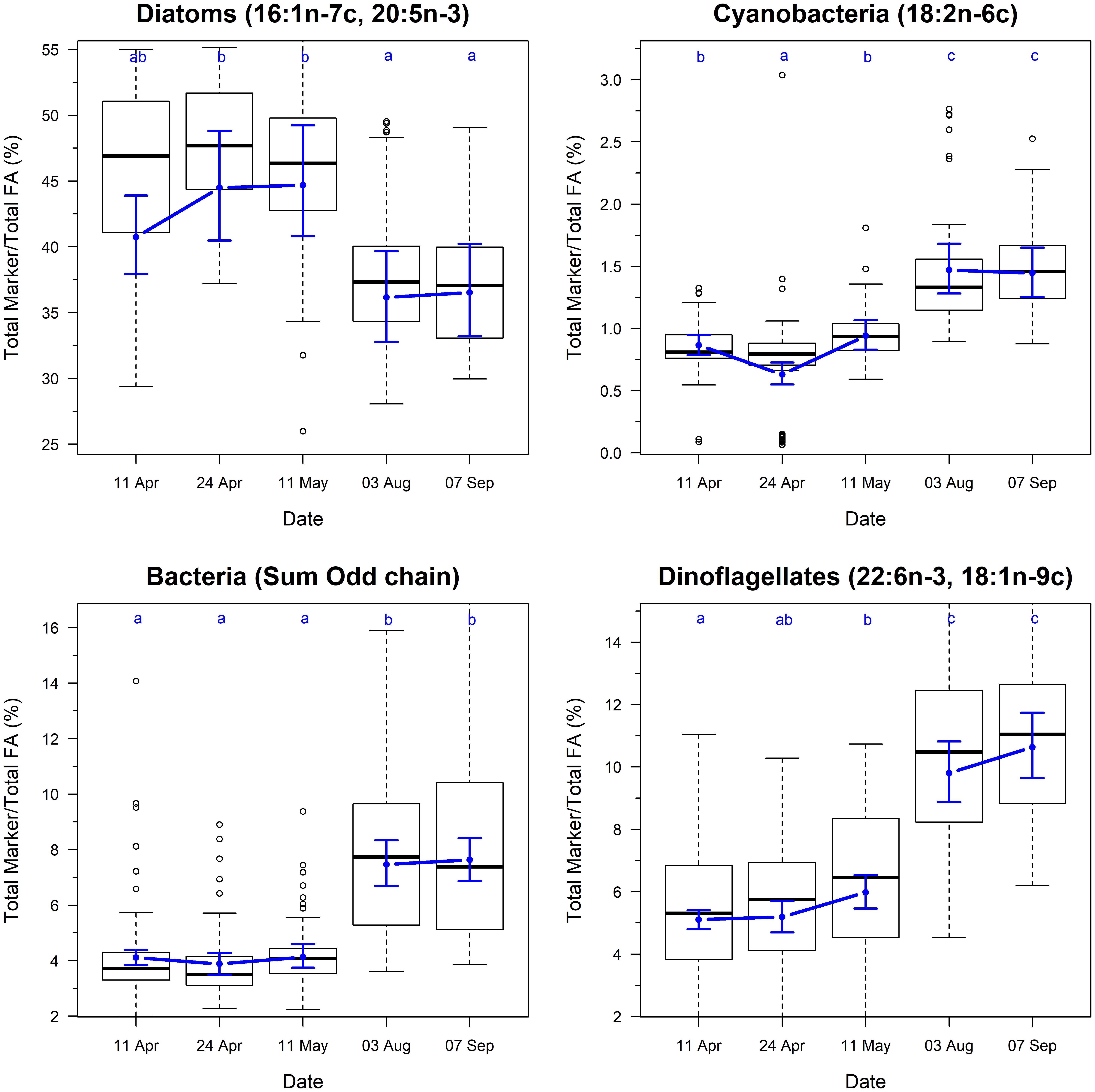
Figure 3. Temporal changes in the ratio of fatty acid groups and chemotaxonomic markers on Roberts Banks mudflats located on the Fraser River estuary, British Columbia, April-September 2017. Note that y-axis for biomarker plots extend from 0.025 to 0.975 percentiles of each measure to avoid compression of scale from outliers. Box plots represent the distribution of observed values, where midline is the median, with the upper and lower limits of the box being 75th and 25th percentiles. Whiskers extend up to 1.5 × the interquartile range, and outliers are depicted as points. Blue circles indicate predicted means from linear mixed effects models, and bounds are 95% prediction intervals from fixed effects. Letters indicate groupings that are not statistically significantly different according to Tukey’s pairwise comparisons.
Correlations With Covariates
Covariates related to nutrients and water conditions varied among sampling dates (Figure 4). TIN was highest (0.25 mg/L) from 11 April to 11 May), after which values remained at ∼0.10 mg/L throughout summer. Similarly, phosphorus declined from 0.021 mg/L in the 11 April to 11 May period, to an average of 0.01 mg/L throughout summer. Both silica and salinity showed clear patterns related to the Fraser River freshet. Silica ranged between 3.2 and 5.1 mg/L and demonstrated little variance over time, except for a strong peak on 11 May, when silica increased to a mean value of 10.8 mg/L. Median salinity from the past 24 h, when averaged across locations, had a mean value of 13.7 PSU in April, which declined over the spring to its lowest mean value of 1.9 PSU during the freshet period in May, and then increased to range between 15−16 PSU during August and September. Median temperature from the past 24 h (i.e., before biofilm sampling) increased from 9.5°C on 11 April to ∼20°C in August and September. The 95th percentile of temperature ranged from a mean of 17°C in April to >22°C by September.
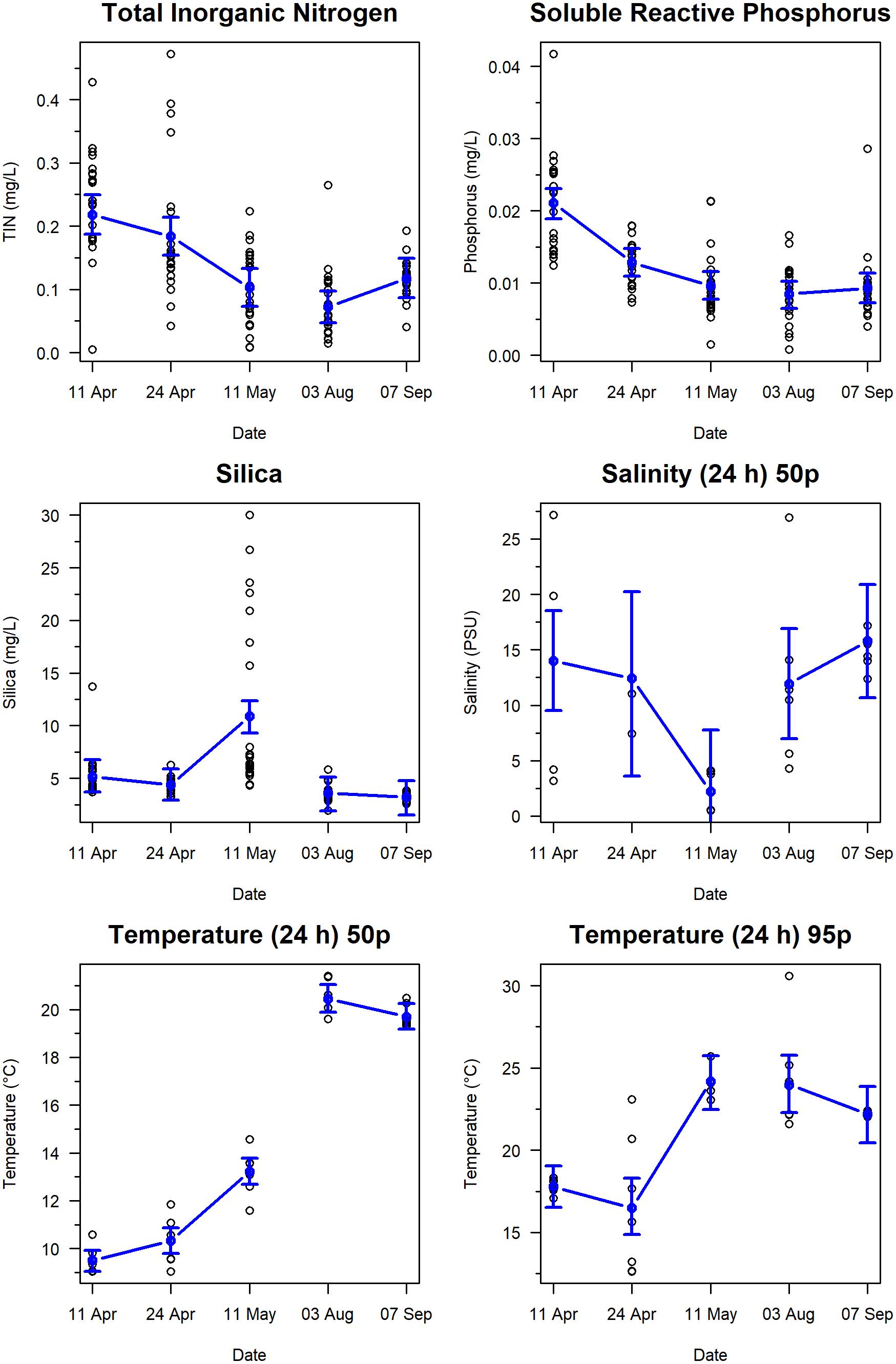
Figure 4. Temporal changes in nutrients and water conditions on Roberts Bank, Fraser River Delta, British Columbia, 2017. Blue circles indicate predicted means, and bounds are 95% prediction intervals from linear mixed effects models. Variables plotted are Total Inorganic Nitrogen, Soluble Reactive Phosphorus, Silica, Salinity (24 h) 50 percentile, Temperature (24 h) 50th percentile, and Temperature (24 h) 95th percentile.
Biofilm measures were significantly correlated with a suite of spatial attributes and water conditions, of which elevation, salinity (24 h) 50th percentile, temperature (24 h) 50th percentile, and temperature (24 h) 95th percentile were the most influential, with details varying by each of the eight biofilm measures (Table 3). Elevation also had a strong and positive effect on all biofilm measures (Table 3), indicating the important role played by local topography in determining biofilm organic and fatty acid content. The other spatial covariate, distance to the HWL, was also negatively correlated with organic content, chlorophyll-a, and SFA, indicating higher values closer to shore (Table 3).
Soluble reactive phosphorus, TIN, and silica showed correlations with biofilm measures. Contrary to expectations, soluble reactive phosphorus and TIN had consistent and negative correlations with measures of fatty acid content (Table 3). Silica had a negative correlation with organic content and a positive correlation with measures of fatty acid content (Table 3).
The two measures of temperature had contrasting correlations with biofilm measures: the 95th percentile of temperature over the past 24 h had a negative association with organic content and chlorophyll-a, whereas median temperature was positively correlated with organic content (Table 3). Of the variables related to water conditions, median salinity over the past 24 h had negative correlations with SFA, n–3, and PUFA, and a positive correlation with total lipid (Table 3).
Discussion
Shorebirds often travel many thousands of kilometers on their annual migrations between wintering and breeding areas. These arduous journeys are only possible if the birds are able to re-fuel with energy and nutrients thereby maintaining themselves in top physiological condition. To achieve this, birds depend on key stopover sites to provide safe staging places to recover and restock the energy and essential nutrients required for the next leg of their migration (Warnock, 2010). Disruptions to habitat quantity or quality at stopover sites can lead to steep declines in shorebird numbers (Weber et al., 1999; Studds et al., 2017). However, it is not just the location of stopover sites that determines their suitability, there is also a confluence of physical and chemical factors that, operating synergistically, make them ideal places for the generally well-timed production (mainly in the biofilm) of accessible energy and essential nutrients required by shorebirds during their annual migrations (Mathot et al., 2018).
We demonstrate that intertidal mudflat biofilm in the Fraser River estuary, especially in spring when these communities are dominated by diatoms, is a rich source of lipid and essential fatty acids. These riches of energy and nutrients occur around the same time that Western Sandpipers and other shorebirds make their annual northward migration through the area in large congregations (Drever et al., 2014; Schnurr et al., 2019). These seasonal changes in fatty acid content may result from three (non-mutually exclusive) processes: (i) changes in the proportion of diatom biomass within the biofilm which results in more fatty acids; (ii) physiological changes within diatoms already present on the mudflats, resulting in an increase in per cell total lipid and individual fatty acid content, and (iii) seasonal species turnover within the biofilm community. Here, we describe the spatial and temporal confluence of triggers that stimulate lipid production in diatoms, and discuss how each of them may function to promote the observed temporal trajectory of essential fatty acids in these intertidal mudflat biofilm communities.
Spatial Variability
Spatially, we found measures of organic content, total lipid, chlorophyll-a, and fatty acids tended to be higher closer to the HWL and at sites with raised elevation (Table 3). Elevated areas have longer times (i.e., several hours per tidal period) when the mudflat is exposed to ambient air conditions. These conditions likely allow algal cells to photosynthesize at higher rates since they are exposed to higher photon flux densities, warmer springtime temperatures (Schnurr et al., 2019), and higher rates of gas exchange (i.e., CO2 into the cells and O2 out of the biofilms). The sites closer to shore would have longer exposure times (to ambient air conditions) and may experience less shear stress associated with tidal and wave energy (Underwood and Paterson, 1993). Generally, low shear stress facilitates formation and growth of thicker algal biofilms (de Brouwer et al., 2005; Besemer et al., 2007). The positive correlation with elevation might also reflect the presence of intertidal hummocks, which appear to be associated with higher densities of epipelic diatoms (Beninger et al., 2018).
Differences in Diatom Biomass
Spring is a time of rapid change in the Fraser River estuary. Temperature, day length and photon flux densities increase (Schnurr et al., 2019), and the tidal cycles switch such that lowest tides occur during daylight hours rather than overnight (Thomson, 1981). These higher photon flux densities (Jensen and Revsbech, 1989; Schnurr and Allen, 2015; Schnurr et al., 2016b) and temperatures (Blanchard et al., 1997; Kudo et al., 2000; Jiang and Gao, 2004; Scholz and Liebezeit, 2013) result in enhanced rates of photosynthesis and growth in microphytobenthos. Since diatoms are the main primary producers in intertidal biofilm at our study site (Beninger et al., 2011; Schnurr et al., 2019), increases in photosynthetic activity likely cause increases in overall diatom biomass in the biofilm. Thus, we found a positive correlation between organic content and temperature (24 h) 50th percentile (Table 3). Temperatures in the 15−20°C range foster ideal benthic diatom growth rates (Admiraal, 1976). The spring temperatures experienced on Roberts Bank in 2017 were often within these optimal temperatures (Figure 4), and outside temperature ranges that can result in growth inhibition and/or cell death (Thompson and Guo, 1992; Kudo et al., 2000; Jiang and Gao, 2004; Woelfel et al., 2014). Although not measured directly, the effect of photon flux density is encapsulated by the strong effect of date on the measures of fatty acid abundance. In addition, the diatom fatty acid biomarkers were higher in the spring compared to summer (Figure 3), consistent with the hypothesis that higher fatty acid content of biofilm results from an overall higher proportion of diatom biomass.
We found that fatty acid content was significantly reduced during summer, and this was likely a result of temperatures that were too high for diatoms to grow and reproduce. Indeed, we regularly observed maximal daytime temperatures on mudflat biofilms in the summertime to be greater than 33°C, which is above optimal temperatures for diatom growth (Thompson and Guo, 1992; Kudo et al., 2000; Jiang and Gao, 2004; Woelfel et al., 2014). Higher temperatures during summer would have also resulted in a stronger “de-watering” effect, wherein changes in the sediment bulk density can result in lower measures of Chl a content of microphytobenthos (Perkins et al., 2003).
Physiological Changes
Differences in fatty acid content of biofilm between spring and summer may also occur from physiological changes within the diatom communities themselves. The existence of a lipid accumulation response during spring was supported by the larger (SFA + MUFA)/PUFA ratios that ranged from 2.3 to 2.9, indicating a preferential production of triacylglycerol relative to summer samples. Laboratory studies have shown lipid accumulation in microalgae occurs in two stages (Rodolfi et al., 2009). First, when all requirements for growth are met, microalgae divide rapidly and synthesize (mostly) phospholipid-dominated membrane lipids, which are predominately composed of MUFA and PUFA (Solovchenko, 2012). Second, when any growth factor becomes limiting, cells enter a lipid-producing phase characterized by a slowdown or cessation of cell division accompanied by an accumulation of triacylglycerol, predominantly composed of SFA and MUFA (Sharma et al., 2012; Solovchenko, 2012). Fatty acid content during the spring sampling dates showed a wide variance, with some locations having very high values relative to the mean (Figure 2). Total fatty acid values had a double mode distribution, and these very high values could account for up to 85% of the total fatty acid content in the spring samples (Supplementary Material). Presently we have no way to differentiate the relative roles of increases in diatom biomass and lipid accumulation response in determining the total amount of fatty acids in intertidal biofilm, and we suggest that these local sites with very high values result from their combined action, i.e., very high local bursts of fatty acids become available when diatoms that are experiencing good growing conditions are suddenly triggered to accumulate lipid.
Sudden shifts in water chemistry such as salinity or pH can cause lipid accumulation responses in microalgae (Sharma et al., 2012). On Roberts Bank, the daily discharge of the Fraser River increases steadily during April and May on account of the spring freshet fueled by snow melt (Supplementary Material), and this influx of freshwater into the tidal estuary results in rapid changes in salinity and water chemistry. We consider rapid changes in salinity as the strongest driver in the lipid accumulation response because the rapid drop in salinity in May coincided with the peak abundance of fatty acids (Figure 4), and the covariate modeling (Table 3) indicated salinity had a stronger role on fatty acid content than temperature. Further, seasonal changes are accompanied by wide daily fluctuations related to tidal cycles (i.e., salinity wedges), whereby dense saltwater flooding the mudflats causes a spike in salinity (Correll, 1978) until the tide peaks and then begins to recede, when diffusion and currents cause the salinity to decrease. This twice-daily event would contribute to a lipid accumulation response in response to the osmotic/salinity stress. In the spring, fatty acid content was highest during the May samples, which occurred during spring tides (Supplementary Material) that, in addition to resulting in longer exposure times, would have accentuated daily variance in salinity.
A lipid accumulation response can be also induced by factors such as changes in nutrients, light levels, and temperature (Sharma et al., 2012; Schnurr et al., 2016a). Diatoms can increase their total lipid (including fatty acids) contents (up to three-fold) when “starved” of nitrogen and/or silica for relatively short periods of time (i.e., 4−120 h) (Opute, 1974; Shifrin and Chisholm, 1981; Roessler, 1988; Sriharan et al., 1991; Hu et al., 2008). Although the negative correlations between fatty acid content with N and P (Table 3) are consistent with lipid accumulation response, these nutrients on Roberts Bank changed gradually over time (Figure 4), and therefore we interpret this correlation as a seasonal depletion, and that N and P are not limiting in a nutrient-rich marine environment. Silica had a negative correlation with organic content, and a positive correlation with measures of fatty acid content (Table 3). Silica (driven by the freshet) is likely fueling the diatom productivity selectively, wherein diatoms require this nutrient to form their exterior frustules, in contrast to other taxa in the microphytobenthos.
Community Composition
The composition of algal communities can have a pronounced effect on their fatty acid profiles (Galloway and Winder, 2015; Schnurr and Allen, 2015). Thus, differences in fatty acid content between spring and summer may have also resulted from changes in community composition of the intertidal biofilm. The Roberts Bank diatom community is dominated by Navicula spp. and Nitzschia spp. (Beninger et al., 2011; Schnurr et al., 2019). Based on fatty acid markers, we suggest that the overall diatom biomass within the biofilm declines from spring to summer (Figure 3), a change accompanied by an increase in bacteria, cyanobacteria, and dinoflagellates. The highest temperatures (>30°C) observed in the summertime would inhibit diatom growth and reproduction (Renaud et al., 1994; Kudo et al., 2000; Jiang and Gao, 2004; Scholz and Liebezeit, 2013; Woelfel et al., 2014), but favor the growth of cyanobacteria, chlorophytes and bacteria (Schnurr and Allen, 2015). These changes tie the fatty acid content of biofilm to the presence of epipelic diatoms, with the caveat that the effect of species turnover on fatty acid content of intertidal biofilm needs to be better explored with more detailed taxonomic data.
Implications for Shorebird Migration
Hitherto, there has been only a limited understanding of why shorebirds choose where, when and how long to stop and forage during long-distance migration. For example, why Western Sandpipers stop at only a few large estuarine mudflats during their northward breeding migration along the Pacific Flyway (Mathot et al., 2018), but are dissipated over multiple smaller sites on their return to non-breeding grounds, has been enigmatic (O’Reilly and Wingfield, 1995). Conventional ornithological hypotheses to explain this dichotomy in migration strategies are based on either access to energy (Farmer and Wiens, 1999) or predation threats (Lank et al., 2003). Our finding that the northward arrival of Western Sandpipers and other shorebirds on Roberts Bank mirrors seasonal increases in total lipid and in the abundance of essential fatty acids found in intertidal biofilm provides a new explanation. The discovery complements previous studies demonstrating that shorebirds alter their foraging behaviors to select areas with high microphytobenthic biomass (Drouet et al., 2015; Jiménez et al., 2015) and the activities of shorebirds can significantly affect benthic diatom growth (Jauffrais et al., 2015). The inference is that migration success for shorebirds hinges on their presence at a stopover site that generally coincides with maximal total lipid and fatty acid production at the site. In the case of Roberts Bank, the main trigger for benthic diatoms switching to their lipid/fatty acid accumulation phase appears to be stress associated with high amplitude oscillations in salinity. We speculate that equivalent mechanisms triggering lipid/fatty acid accumulation responses in diatom-dominated biofilm assemblages are likely also affecting the quality of migratory stopover locations on mudflats elsewhere on the Pacific and along other international flyways. Investigating the existence of and, if so, elucidating the nature of stress triggers at all these sites should be a research priority. Of particular importance is discerning whether these triggers are driven by similar salinity-associated circumstances to Roberts Bank or an alternative mechanism. Meanwhile, maintaining the salinity trigger is an imperative for the effective conservation of migratory shorebirds in the Fraser River estuary and, if a pattern emerges on other stopover sites, this new understanding would galvanize a paradigm shift in shorebird conservation worldwide.
Springtime accumulation of biofilm biomass and fatty acids represents a crucial “pulse” of fuel and essential nutrients into estuarine food webs. Invertebrate populations foraging on primary producers in the microphytobenthos can show rapid increases in abundance (Sahan et al., 2007), and thus accumulation of lipid and fatty acids up the food web (i.e., into fish, birds, etc.). Given the important role of essential fatty acids in the growth and reproduction of a wide variety of organisms within aquatic food webs (Kainz et al., 2004), understanding the physiological response of algae/diatoms to complex and interacting environmental factors in estuarine mudflats is fundamental to designing strategies to conserve and protect migrating birds and estuarine ecosystems in their entirety. We found that total lipid and fatty acid content in biofilm on mudflats varied between spring and summer and that these nutritionally important compounds were significantly affected by the outflow of freshwater from the Fraser River. To the north of our study site lies Sturgeon Bank, another large estuarine mudflat that has experienced extensive alterations to its coastal oceanography and sediment transport (Atkins et al., 2016). The Sturgeon Bank area now sees reduced use by shorebirds during the critical northward migration period relative to Roberts Bank (Jardine et al., 2015). Such differences underscores the need to protect these mudflat habitats in as pristine a state as possible so they may continue to furnish shorebirds with the energy and essential fatty acids required for long-distance migration.
Data Availability Statement
The raw data supporting the conclusions of this article will be made available by the authors, without undue reservation, to any qualified researcher.
Author Contributions
RE, MA, PS, and JH conceived and designed the study. PS collected the data. MD and JH contributed data. MD performed the statistical analyses. PS, MD, RE, JH, and MA wrote the manuscript.
Funding
Funding for this work was provided by the Vancouver Airport Fuel Facilities Corporation to MA and PS, Environment and Climate Change Canada to MD and RE, and Natural Sciences and Engineering Research Council of Canada to MA (NSERC Discovery Grant #04537-2014).
Conflict of Interest
The authors declare that this study received funding from Vancouver Airport Fuel Facilities Corporation. The funder was not involved in the study design, collection, analysis, interpretation of data, the writing of this article or the decision to submit it for publication.
JH was employed by company Coastal & Ocean Resources.
The remaining authors declare that the research was conducted in the absence of any commercial or financial relationships that could be construed as a potential conflict of interest.
Acknowledgments
Lipid and fatty acid analyses (Ryerson University) were assisted by Serena Egidio, Clara Romany, and Cristina Mudalige. Daniel Stewart and Megan Lievesley provided excellent assistance on the mudflats collecting data and samples. Brad Mason of the Community Mapping Network provided the elevation measures. Nutrient analyses were conducted by Environment and Climate Change Canada’s National Laboratory for Environmental Testing, Burlington, Ontario, and the Pacific Environmental Science Centre, North Vancouver, British Columbia. Brent Gurd (Province of British Columbia) and Eric Balke (Ducks Unlimited Canada) contributed salinity data.
Supplementary Material
The Supplementary Material for this article can be found online at: https://www.frontiersin.org/articles/10.3389/fmars.2020.00063/full#supplementary-material
References
Admiraal, W. (1976). Influence of light and temperature on the growth rate of estuarine benthic diatoms in culture. Mar. Biol. 39, 1–9. doi: 10.1007/bf00395586
APHA, AWWA, and WEF (2017). Standard Methods for the Examination of Water and Wastewater, current online edition. Washington, DC: American Public Health Association, 20001–23710.
Arar, E. J. (1997). In Vitro Determination of Chlorophylls a, b, c1 + c2 and Pheopigments in Marine and Freshwater Algae by Visible Spectrometry (Method 446.0). Ohio: National Exposure Research Laboratory Office of Research and Development U.S. Environmental Protection Agency Cincinnati.
Arts, M. T., Ackman, R. G., and Holub, B. J. (2001). “Essential fatty acids” in aquatic ecosystems: a crucial link between diet and human health and evolution. Can. J. Fish. Aquatic Sci. 58, 122–137. doi: 10.1139/f00-224
Atkins, R. J., Tidd, M., and Ruffo, G. (2016). Sturgeon Bank, Fraser River delta, BC, Canada: 150 years of human influences on salt marsh sedimentation. J. Coast. Res. 75(Suppl.1), 790–795.
Beninger, P. G., Cuadrado, D., and van de Koppel, J. (2018). in Sedimentary and Biological Patterns on Mudflats, ed. P. G. Beninger, (Cham: Springer), 185–211.
Beninger, P. G., Elner, R. W., Morancais, M., and Decottignies, P. (2011). Downward trophic shift during breeding migration in the shorebird Calidris mauri (Western Sandpiper). Mar. Ecol. Prog. Ser. 428, 259–269. doi: 10.3354/meps09050
Besemer, K., Singer, G., Limberger, R., Chlup, A. K., Hochedlinger, G., Hödl, I., et al. (2007). Biophysical controls on community succession in stream biofilms. Appl. Environ. Microbiol. 73, 4966–4974. doi: 10.1128/aem.00588-07
Blanchard, G. F., Guarini, J. M., Gros, P., and Richard, P. (1997). Seasonal effect on the relationship between the photosynthetic capacity of intertidal microphytobenthos and temperature. J. Phycol. 33, 723–728. doi: 10.1111/j.0022-3646.1997.00723.x
Butler, R. W., and Campbell, R. W. (1987). The Birds of the Fraser River Delta: Populations, Ecology and International Significance. Occasional Paper. Ottawa: Canadian Wildlife Service, 73.
Butler, R. W., Davidson, N. C., and Morrison, R. G. (2001). Global-scale shorebird distribution in relation to productivity of near-shore ocean waters. Waterbirds 24, 224–232.
Butler, R. W., Delgado, F. S., de la Cueva, H., Pulido, V., and Sandercock, B. K. (1996). Migration routes of the Western Sandpiper. Wilson Bull. 180, 662–672.
Carpentier, A., Como, S., Dupuy, C., Lefrançois, C., and Feunteun, E. (2014). Feeding ecology of Liza spp. in a tidal flat: evidence of the importance of primary production (biofilm) and associated meiofauna. J. Sea Res. 92, 86–91. doi: 10.1016/j.seares.2013.10.007
Dalsgaard, J., St John, M., Kattner, G., Müller-Navarra, D. C., and Hagen, W. (2003). Fatty acid trophic markers in the pelagic marine environment. Adv. Mar. Biol. 46, 225–340. doi: 10.1016/s0065-2881(03)46005-7
de Brouwer, J. D., Wolfstein, K., Ruddy, G. K., Jones, T. E. R., and Stal, L. J. (2005). Biogenic stabilization of intertidal sediments: the importance of extracellular polymeric substances produced by benthic diatoms. Microb. Ecol. 49, 501–512. doi: 10.1007/s00248-004-0020-z
Drever, M. C., Lemon, M. J., Butler, R. W., and Millikin, R. L. (2014). Monitoring populations of Western Sandpipers and Pacific Dunlins during northward migration on the Fraser River Delta, British Columbia, 1991–2013. J. Field Ornithol. 85, 10–22. doi: 10.1111/jofo.12045
Drouet, S., Turpin, V., Godet, L., Cognie, B., Cosson, R. P., and Decottignies, P. (2015). Utilisation of intertidal mudflats by the Dunlin Calidris alpina in relation to microphytobenthic biofilms. J. Ornithol. 156, 75–83. doi: 10.1007/s10336-014-1133-x
Elner, R. W., Beninger, P. G., Jackson, D. L., and Potter, T. M. (2005). Evidence of a new feeding mode in western sandpiper (Calidris mauri) and dunlin (Calidris alpina) based on bill and tongue morphology and ultrastructure. Mar. Biol. 146, 1223–1234. doi: 10.1007/s00227-004-1521-5
Farmer, A. H., and Wiens, J. A. (1999). Models and reality: time–energy trade-offs in pectoral sandpiper (Calidris melanotos) migration. Ecology 80, 2566–2580. doi: 10.1890/0012-9658(1999)080%5B2566:martet%5D2.0.co;2
Folch, J., Lees, M., and Sloane Stanley, G. H. (1957). A simple method for the isolation and purification of total lipids from animal tissues. J. Biol. Chem. 226, 497–509.
Franks, S., Lank, D. B., and Wilson, W. H. (2014). “Western sandpiper (Calidris mauri),” in The birds of North America online, ed. P. Rodewald, (Ithaca: Cornell Laboratory of Ornithology), doi: 10.2173/bna.90
Galloway, A. W. E., and Winder, M. (2015). Partitioning the relative importance of phylogeny and environmental conditions on phytoplankton fatty acids. PLoS One 10:e0130053. doi: 10.1371/journal.pone.0130053
Guglielmo, C. G. (2010). Move that fatty acid: fuel selection and transport in migratory birds and bats. Integr. Comp. Biol. 50, 336–345. doi: 10.1093/icb/icq097
Herman, P. M. J., Middelburg, J. J., Widdows, J., Lucas, C. H., and Heip, C. H. R. (2000). Stable isotopes as trophic tracers: combining field sampling and manipulative labelling of food resources for macrobenthos. Mar. Ecol. Prog. Ser. 204, 79–92. doi: 10.3354/meps204079
Hixson, S. M., Sharma, B., Kainz, M. J., Wacker, A., and Arts, M. T. (2015). Production, distribution, and abundance of long-chain omega-3 polyunsaturated fatty acids: a fundamental dichotomy between freshwater and terrestrial ecosystems. Environ. Rev. 23, 414–424. doi: 10.1139/er-2015-0029
Hothorn, T., Bretz, F., and Westfall, P. (2008). Simultaneous inference in general parametric models. Biometr. J. 50, 346–363. doi: 10.1002/bimj.200810425
Hu, H., and Gao, K. (2006). Response of growth and fatty acid compositions of Nannochloropsis sp. to environmental factors under elevated CO2 concentrations. Biotechnol. Lett. 28, 987–992. doi: 10.1007/s10529-006-9026-6
Hu, Q., Sommerfeld, M., Jarvis, E., Ghirardi, M., Posewitz, M., Seibert, M., et al. (2008). Microalgal triacylglycerols as feedstocks for biofuel production: perspectives and advances. Plant J. 54, 621–639. doi: 10.1111/j.1365-313X.2008.03492.x
Jardine, C. B., Bond, A. L., Davidson, P. J., Butler, R. W., and Kuwae, T. (2015). Biofilm consumption and variable diet composition of Western Sandpipers (Calidris mauri) during migratory stopover. PLoS One 10:e0124164. doi: 10.1371/journal.pone.0124164
Jauffrais, T., Drouet, S., Turpin, V., Méléder, V., Jesus, B., Cognie, B., et al. (2015). Growth and biochemical composition of a microphytobenthic diatom (Entomoneis paludosa) exposed to shorebird (Calidris alpina) droppings. J. Exp. Mar. Biol. Ecol. 469, 83–92. doi: 10.1016/j.jembe.2015.04.014
Jensen, J., and Revsbech, N. P. (1989). Photosynthesis and respiration of a diatom biofilm cultured in a new gradient growth chamber. FEMS Microbiol. Ecol. 62, 29–38. doi: 10.1111/j.1574-6968.1989.tb03655.x
Jiang, H., and Gao, K. (2004). Effects of lowering temperature during culture on the production of polyunsaturated fatty acids in the marine diatom Phaeodactylum tricornutum (Bacillariophyceae). J. Phycol. 40, 651–654. doi: 10.1111/j.1529-8817.2004.03112.x
Jiménez, A., Elner, R. W., Favaro, C., Rickards, K., and Ydenberg, R. C. (2015). Intertidal biofilm distribution underpins differential tide-following behavior of two sandpiper species (Calidris mauri and Calidris alpina) during northward migration. Estuar. Coast. Shelf Sci 155, 8–16. doi: 10.1016/j.ecss.2014.12.038
Kainz, M., Arts, M. T., and Mazumder, A. (2004). Essential fatty acids in the planktonic food web and their ecological role for higher trophic levels. Limnol. Oceanogr. 49, 1784–1793. doi: 10.4319/lo.2004.49.5.1784
Kelly, J. R., and Scheibling, R. E. (2012). Fatty acids as dietary tracers in benthic food webs. Mar. Ecol. Prog. Ser. 446, 1–22. doi: 10.3354/meps09559
Kirst, G. O. (1989). Salinity tolerance of eukaryotic marine algae. Ann. Rev. Plant Physiol. Plant Mol. Biol. 40, 21–53. doi: 10.1093/gbe/evw152
Kudo, I., Miyamoto, M., Noiri, Y., and Maita, Y. (2000). Combined effects of temperature and iron on the growth and physiology of the marine diatom Phaeodactylum tricornutum (Bacillariophyceae). J. Phycol. 36, 1096–1102. doi: 10.1046/j.1529-8817.2000.99042.x
Kunihiro, T., Veuger, B., Vasquez-Cardenas, D., Pozzato, L., Le Guitton, M., Moriya, K., et al. (2014). Phospholipid-derived fatty acids and quinones as markers for bacterial biomass and community structure in marine sediments. PLoS One 9:e96219. doi: 10.1371/journal.pone.0096219
Kuwae, T., Beninger, P. G., Decottignies, P., Mathot, K. J., Lund, D. R., and Elner, R. W. (2008). Biofilm grazing in a higher vertebrate: the western sandpiper Calidris mauri. Ecology 89, 599–606. doi: 10.1890/07-1442.1
Kuwae, T., Miyoshi, E., Hosokawa, S., Ichimi, K., Hosoya, J., Amano, T., et al. (2012). Variable and complex food web structures revealed by exploring missing trophic links between birds and biofilm. Ecol. Lett. 15, 347–356. doi: 10.1111/j.1461-0248.2012.01744.x
Kuznetsova, A., Brockhoff, P. B., and Christensen, R. H. B. (2017). lmerTest package: tests in linear mixed effects models. J. Stat. Softw 82, 1–26.
Lank, D. B., Butler, R. W., Ireland, J., and Ydenberg, R. C. (2003). Effects of predation danger on migration strategies of sandpipers. Oikos 103, 303–319. doi: 10.1034/j.1600-0706.2003.12314.x
Lefcheck, J. S. (2016). piecewiseSEM: piecewise structural equation modelling in R for ecology, evolution, and systematics. Methods Ecol. Evol. 7, 573–579. doi: 10.1111/2041-210x.12512
Maillet, D., and Weber, J. M. (2006). Performance-enhancing role of dietary fatty acids in a long-distance migrant shorebird: the Semipalmated Sandpiper. J. Exp. Biol. 209, 2686–2695. doi: 10.1242/jeb.02299
Maillet, D., and Weber, J. M. (2007). Relationship between n-3 PUFA content and energy metabolism in the flight muscles of a migrating shorebird: evidence for natural doping. J. Exp. Biol. 210, 413–420. doi: 10.1242/jeb.02660
Mathot, K. J., Lund, D. R., and Elner, R. W. (2010). Sediment in stomach contents of Western Sandpipers and Dunlin provide evidence of biofilm feeding. Waterbirds 33, 300–306. doi: 10.1675/063.033.0305
Mathot, K. J., Piersma, T., and Elner, R. W. (2018). “Shorebirds as integrators and indicators of mudflat ecology,” in Mudflat Ecology, ed. P. G. Beninger, (Switzerland: Springer Nature), 309–338. doi: 10.1007/978-3-319-99194-8_12
Mohan, S. V., and Devi, M. P. (2014). Salinity stress induced synthesis to harness biodiesel during dual mode cultivation of mixotrophic microalgae. Bioresour. Technol. 165, 288–294. doi: 10.1016/j.biortech.2014.02.103
Nakagawa, S., Johnson, P. C., and Schielzeth, H. (2017). The coefficient of determination R2 and intra-class correlation coefficient from generalized linear mixed-effects models revisited and expanded. J. R. Soc. Interf. 14:20170213. doi: 10.1098/rsif.2017.0213
Opute, F. (1974). Lipid and fatty acid composition of diatoms. J. Exp. Bot. 25, 823–835. doi: 10.1093/jxb/25.4.823
O’Reilly, K. M., and Wingfield, J. C. (1995). Spring and autumn migration in Arctic shorebirds: same distance, different strategies. Am. Zool. 35, 222–233. doi: 10.1093/icb/35.3.222
Perkins, R. G., Honeywill, C., Consalvey, M., Austin, H. A., Tolhurst, T. J., and Paterson, D. M. (2003). Changes in microphytobenthic chlorophyll a and EPS resulting from sediment compaction due to de-watering: opposing patterns in concentration and content. Continental Shelf Res. 23, 575–586. doi: 10.1016/s0278-4343(03)00006-2
Price, E. R. (2010). Dietary lipid composition and avian migratory flight performance: development of a theoretical framework for avian fat storage. Comp. Biochem. Physiol. Part A 157, 297–309. doi: 10.1016/j.cbpa.2010.05.019
Quinn, J. T., Hamilton, D. J., and Hebert, C. E. (2017). Fatty acid composition and concentration of alternative food of Semipalmated Sandpipers (Calidris pusilla) in the upper Bay of Fundy Canada. Can. J. Zool. 95, 565–573. doi: 10.1139/cjz-2016-0246
Renaud, S. M., Parry, D. L., and Thinh, L. V. (1994). Microalgae for use in tropical aquaculture I: gross chemical and fatty acid composition of twelve species of microalgae from the Northern Territory Australia. Journal of Applied Phycology. 6, 337–345. doi: 10.1007/bf02181948
Rodolfi, L., Zittelli, G. C., Bassi, N., Padovani, G., Biondi, N., Bonini, G., et al. (2009). Microalgae for oil: strain selection, induction of lipid synthesis and outdoor mass cultivation in a low-cost photobioreactor. Biotechnol. Bioeng. 102, 100–112. doi: 10.1002/bit.22033
Roessler, P. G. (1988). Effects of silicon deficiency on lipid composition and metabolism in the diatom Cyclotella cryptica. J. Phycol. 24, 394–400. doi: 10.1111/j.1529-8817.1988.tb00189.x
Rosenberg, K. V., Dokter, A. M., Blancher, P. J., Sauer, J. R., Smith, A. C., Smith, P. A., et al. (2019). Decline of the North American avifauna. Science 366, 120–124. doi: 10.1126/science.aaw1313
Sahan, E., Sabbe, K., Creach, V., Hernandez-Raquet, G., Vyverman, W., Stal, L. J., et al. (2007). Community structure and seasonal dynamics of diatom biofilms and associated grazers in intertidal mudflats. Aquatic Microb. Ecol. 47, 253–266. doi: 10.3354/ame047253
Schnurr, P. J., and Allen, D. G. (2015). Factors affecting algae biofilm growth and lipid production: a review. Renewable Sustain. Energ. Rev. 52, 418–429. doi: 10.1016/j.rser.2015.07.090
Schnurr, P. J., Drever, M. C., Kling, H. J., Elner, R. W., and Arts, M. T. (2019). Seasonal changes in fatty acid composition of estuarine intertidal biofilm: implications for western sandpiper migration. Estuar., Coast. Shelf Sci. 224, 94–107. doi: 10.1016/j.ecss.2019.04.047
Schnurr, P. J., Espie, G. S., and Allen, D. G. (2016a). The effect of photon flux density on algal biofilm growth and internal fatty acid concentrations. Algal Res. 16, 349–356. doi: 10.1016/j.algal.2016.04.001
Schnurr, P. J., Molenda, O., Edwards, E., Espie, G. S., and Allen, D. G. (2016b). Improved biomass productivity in algal biofilms through synergistic interactions between photon flux density and carbon dioxide concentration. Bioresour. Technol. 219, 72–79. doi: 10.1016/j.biortech.2016.06.129
Scholz, B., and Liebezeit, G. (2013). Biochemical characterization and fatty acid profiles of 25 benthic marine diatoms isolated from the Solthörn tidal flat (southern North Sea). J. Appl. Phycol. 25, 453–465. doi: 10.1007/s10811-012-9879-0
Sharma, K. K., Schuhmann, H., and Schenk, P. M. (2012). High lipid induction in microalgae for biodiesel production. Energies 5, 1532–1553. doi: 10.3390/en5051532
Shifrin, N. S., and Chisholm, S. W. (1981). Phytoplankton lipids: interspecific differences and effects of nitrate, silicate and light-dark cycles. J. Phycol. 17, 374–384. doi: 10.1111/j.1529-8817.1981.tb00865.x
Shin, P. K., Yip, K. M., Xu, W. Z., Wong, W. H., and Cheung, S. G. (2008). Fatty acid as markers to demonstrating trophic relationships among diatoms, rotifers and green-lipped mussels. J. Exp. Mar. Biol. Ecol. 357, 75–84. doi: 10.1016/j.jembe.2008.01.002
Solovchenko, A. (2012). Physiological role of neutral lipid accumulation in eukaryotic microalgae under stresses. Russian J. Plant Physiol. 59, 192–202.
Sriharan, S., Bagga, D., and Nawaz, M. (1991). The effects of nutrient and temperature on biomass, growth, lipid production, and fatty acid composition of Cyclotella cryptica Reimann. Lewin and Guillard. Appl. Biochem. Biotechnol. 2, 317–326. doi: 10.1007/bf02922611
Studds, C. E., Kendall, B. E., Murray, N. J., Wilson, H. B., Rogers, D. I., Clemens, R. S., et al. (2017). Rapid population decline in migratory shorebirds relying on Yellow Sea tidal mudflats as stopover sites. Nat. Commun. 8:14895. doi: 10.1038/ncomms14895
Thompson, P. A., and Guo, M. (1992). Effects of variation in temperature. I. on the biochemical composition of eight species of marine phytoplankton. J. Phycol. 28, 481–488. doi: 10.1111/j.0022-3646.1992.00481.x
Thomson, R. E. (1981). “Oceanography of the British Columbia coast,” in Canadian Special Publication of Fisheries and Aquatic Sciences, Vol. 56, ed. Canada. Dept. of Fisheries and Oceans, (Ottawa: Department of Fisheries and Oceans), 291.
Underwood, G. J., and Paterson, D. M. (1993). Seasonal changes in diatom biomass, sediment stability and biogenic stabilization in the Severn Estuary. J. Mar. Biol. Assoc. U. K. 73, 871–887. doi: 10.1017/s0025315400034780
Underwood, G. J. C., and Kromkamp, J. (1999). Primary production by phytoplankton and microphytobenthos in estuaries. Adv. Ecol. Res. 29, 93–153. doi: 10.1016/s0065-2504(08)60192-0
Viegas, I., Araújo, P. M., Rocha, A. D., Villegas, A., Jones, J. G., Ramos, J. A., et al. (2017). Metabolic plasticity for subcutaneous fat accumulation in a long- distance migratory bird traced by 2H2O. J. Exp. Biol. 220, 1072–1078. doi: 10.1242/jeb.150490
Warnock, N. (2010). Stopping vs. staging: the difference between a hop and a jump. J. Avian Biol. 41, 621–626. doi: 10.1111/j.1600-048x.2010.05155.x
Warnock, N., and Bishop, M. A. (1998). Spring stopover ecology of migrant Western Sandpipers. Condor 100, 456–467. doi: 10.2307/1369711
Weber, J. M. (2009). The physiology of long-distance migration: extending the limits of endurance metabolism. J. Exp. Biol. 212, 593–597. doi: 10.1242/jeb.015024
Weber, T. P., Houston, A. I., and Ens, B. J. (1999). Consequences of habitat loss at migratory stopover sites: a theoretical investigation. J. Avian Biol. 30, 416–426.
Woelfel, J., Schoknecht, A., Schaub, I., Enke, N., Schumann, R., and Karsten, U. (2014). Growth and photosynthesis characteristics of three benthic diatoms from the brackish southern Baltic Sea in relation to varying environmental conditions. Phycologia 53, 639–651. doi: 10.2216/14-019.1
Yi, H. Y., Lu, Y., Zheng, J. W., Yang, W. D., and Liu, J. S. (2014). Biochemical and genetic engineering of diatoms for polyunsaturated fatty acid biosynthesis. Mar. Drugs 12, 153–166. doi: 10.3390/md12010153
Keywords: biofilm, diatoms, estuary, fatty acids, mudflat, omega-3, shorebird migration, western sandpiper
Citation: Schnurr PJ, Drever MC, Elner RW, Harper J and Arts MT (2020) Peak Abundance of Fatty Acids From Intertidal Biofilm in Relation to the Breeding Migration of Shorebirds. Front. Mar. Sci. 7:63. doi: 10.3389/fmars.2020.00063
Received: 11 October 2019; Accepted: 28 January 2020;
Published: 18 February 2020.
Edited by:
Vona Meleder, Université de Nantes, FranceReviewed by:
Cédric Hubas, Muséum National d’Histoire Naturelle, FranceDiana Hamilton, Mount Allison University, Canada
Copyright © 2020 Schnurr, Drever, Elner, Harper and Arts. This is an open-access article distributed under the terms of the Creative Commons Attribution License (CC BY). The use, distribution or reproduction in other forums is permitted, provided the original author(s) and the copyright owner(s) are credited and that the original publication in this journal is cited, in accordance with accepted academic practice. No use, distribution or reproduction is permitted which does not comply with these terms.
*Correspondence: Mark C. Drever, bWFyay5kcmV2ZXJAY2FuYWRhLmNh