- 1Lighthouse Field Station, School of Biological Sciences, University of Aberdeen, Cromarty, United Kingdom
- 2Centre for Environment, Fisheries and Aquaculture Science (Cefas), Lowestoft, United Kingdom
Offshore windfarm developments are expanding, requiring assessment and mitigation of impacts on protected species. Typically, assessments of impacts on marine mammals have focused on pile-driving, as intense impulsive noise elicits adverse behavioral responses. However, other construction activities such as jacket and turbine installation also change acoustic habitats through increased vessel activity. To date, the contribution of construction-related vessel activity in shaping marine mammal behavioral responses at windfarm construction sites has been overlooked and no guidelines or mitigation measures have been implemented. We compared broad-scale spatio-temporal variation in harbor porpoise occurrence and foraging activity between baseline periods and different construction phases at two Scottish offshore windfarms. Following a Before-After Control-Impact design, arrays of echolocation click detectors (CPODs) were deployed in 25 km by 25 km impact and reference blocks throughout the 2017–2019 construction. Echolocation clicks and buzzes were used to investigate porpoise occurrence and foraging activity, respectively. In parallel, we characterized broadband noise levels using calibrated noise recorders (SoundTraps and SM2Ms) and vessel activities using AIS data integrated with engineering records. Following an impact gradient design, we then quantified the magnitude of porpoise responses in relation to changes in the acoustic environment and vessel activity. Compared to baseline, an 8–17% decline in porpoise occurrence was observed in the impact block during pile-driving and other construction activities. The probability of detecting porpoises and buzzing activity was positively related to the distance from vessel and construction activities, and negatively related to levels of vessel intensity and background noise. Porpoise displacement was observed at up to 12 km from pile-driving activities and up to 4 km from construction vessels. This evidence of broad-scale behavioral responses of harbor porpoises to these different construction activities highlights the importance of assessing and managing all vessel activities at offshore windfarm sites to minimize potential impacts of anthropogenic noise.
Introduction
Offshore windfarm developments are currently expanding in response to global efforts to meet decarbonization targets. Many countries aim to generate significant proportions of electricity from offshore wind sources by 2030 (BEIS, 2019), but these developments must be in line with international conservation agreements such as the EU Habitats Directive 92/43/EEC and Environmental Impact Assessment 2014/52/EU Directives (Le Lièvre, 2019). This requires assessment and mitigation of construction, operation and decommissioning activities to reduce potential impacts on marine wildlife. In particular, there have been concerns over the effect of high levels of underwater noise from different anthropogenic activities on cetaceans, with potential to cause either injury or behavioral disturbance (Richardson et al., 1995; Dolman and Simmonds, 2010; Bailey et al., 2014).
Harbor porpoises (Phocoena phocoena), protected under the EU Habitats and Species Directive, are the most common cetacean species in offshore energy development sites within the North Sea (Thomsen et al., 2011; Hammond et al., 2013; Waggitt et al., 2020). Due to their high metabolic requirements, harbor porpoises are vulnerable to starvation (Wisniewska et al., 2016, 2018; Kastelein et al., 2019; Booth, 2020) and, as a consequence, could be especially vulnerable to anthropogenic disturbance. In exposed areas, such as offshore windfarm sites, individuals have to make trade-offs between using energy to leave the area or remaining in exposed areas and tolerating higher levels and/or rates of disturbance (Frid and Dill, 2002). These decisions are likely to be individual-based, context-dependent and site-specific, impacting individual activity budgets and fitness through reduced foraging performance (Booth, 2020). As such, animals may be responding to natural environmental variation, and a variety of different anthropogenic stressors such as fisheries, shipping noise and construction activity. In turn, either individually or in combination, this may have significant long-term biological consequences at a population level (Pirotta et al., 2014a).
The construction and operation of offshore windfarms involves a variety of vessels and activities that could each generate many types of anthropogenic noise that potentially disturb harbor porpoises or other marine mammals. Previously, however, impact assessments have focused on the loudest of these sources; impulsive noise from the pile-driving hammers used to install turbine foundations at most offshore windfarms (Madsen et al., 2006). Where these piling methods are used, mitigation typically involves either minimizing the likelihood that animals are within the injury zone when piling is initiated (Thompson et al., 2020), or using noise abatement techniques such as bubble curtains (Dähne et al., 2017). Extensive research conducted around North Sea windfarm sites has demonstrated that harbor porpoises may be displaced at distances of up to 26 km from piling (e.g., Tougaard et al., 2009; Brandt et al., 2011; Scheidat et al., 2011; Dähne et al., 2013; Haelters et al., 2015; Brandt et al., 2018). However, porpoises are also known to be displaced by vessel noise at distances of up to 7 km (Hermannsen et al., 2014; Dyndo et al., 2015; Wisniewska et al., 2018), with the level of response dependent upon vessel type and behavior (e.g., heading, speed) (Oakley et al., 2017; Hermannsen et al., 2019). Furthermore, even where animals are not displaced, porpoise foraging efficiency may be temporarily affected by exposure both to impulsive noise (Pirotta et al., 2014a; Sarnocińska et al., 2020) and vessel noise (Wisniewska et al., 2018).
Whilst previous studies recognized that construction vessel activity influenced porpoise displacement around pile-driving activities (Brandt et al., 2018; Graham et al., 2019), there remains uncertainty over the cumulative effects of different windfarm construction activities on displacement, foraging efficiency and population fitness. From a management perspective, this constrains efforts to assess and mitigate potential disturbance from windfarm construction activities other than pile-driving. For example, the installation of jackets, turbines and cables may also disturb animals by altering acoustic habitats through intense vessel activity (Merchant et al., 2012; Merchant et al., 2014). Consequently, there may be opportunities to better manage vessels throughout construction and operation to minimize cumulative impacts of shipping movements that could affect harbor porpoise occurrence and behavior. Furthermore, efforts to reduce impulsive noise levels during intermittent periods of pile-driving may result in longer-term noise from additional vessels. Better data on how harbor porpoises respond to different construction and operational phases of windfarm construction is therefore required to understand how different conservation interventions could affect broad-scale habitat displacement and foraging success, particularly within harbor porpoise Special Areas of Conservation (SAC) (JNCC and Natural England, 2019).
In this study, we aimed to compare broad-scale spatio-temporal variation in harbor porpoise occurrence and foraging activity between baseline periods and different phases of construction at two offshore windfarms in the Moray Firth, NE Scotland. The Beatrice offshore windfarm (commissioned in 2019) is composed of 84 (7 MW) turbines and two substations mounted on quadrapod jackets, while the Moray East offshore windfarm (to be commissioned in 2021) will have 100 (9.5 MW) turbines and three substations mounted on tripod jackets. Previous studies in this area used two complementary approaches to assess harbor porpoise responses to impulsive noise from seismic surveys (Thompson et al., 2013; Pirotta et al., 2014a) and pile-driving (Graham et al., 2019). First, Before-After Control-Impact (BACI) designs (Underwood, 1992; Smith, 2002) were used to determine whether variations in porpoise occurrence (Thompson et al., 2013) and activity (Pirotta et al., 2014a) were related to these anthropogenic disturbances. Second, impact gradient sampling designs (Ellis and Schneider, 1997) were also applied in each of these studies to estimate the spatial scale of effects (Graham et al., 2019). Here, we build on these studies, using a BACI design to determine how porpoise occurrence and activity were impacted during different construction phases, and a gradient design to explore how responses varied in relation to the distance from piling vessels as they undertook different activities. Finally, we characterized finer-scale variation in vessel activity and noise levels during different phases of construction and explored how these influenced spatio-temporal variation in porpoise occurrence and activity within the construction sites.
Materials and Methods
Study Area and Context
The study was carried out in 2017, 2018, and 2019 during the construction of the Beatrice Offshore Windfarm and the first phase of construction at Moray East Offshore Windfarm (Figure 1). Beatrice was constructed between March 2017 and May 2019. From April 2017 to December 2017, an anchored piling vessel used impulsive pile-driving to install four 2.2 m diameter steel piles at 86 locations (Graham et al., 2019). Jackets were then installed onto each set of foundation piles between August 2017 and August 2018 using a jack-up vessel. This vessel was also used to install towers, nacelles and blades on each jacket, and the windfarm was fully operational in May 2019 (see Figure 1). Other activities such as boulder removal, inter-array and export cable installation and protection took place during the windfarm construction phase but were not investigated specifically in this study. Construction at Moray East started in May 2019 and the windfarm is anticipated to be fully operational in 2021. Between May and December 2019, a jack-up vessel used impulsive pile-driving to install three 2.5 m diameter steel piles at the first 90 Moray East locations. There was no overlap between the piling campaign and the jacket foundation installation phase at Moray East (see Supplementary Table 1).
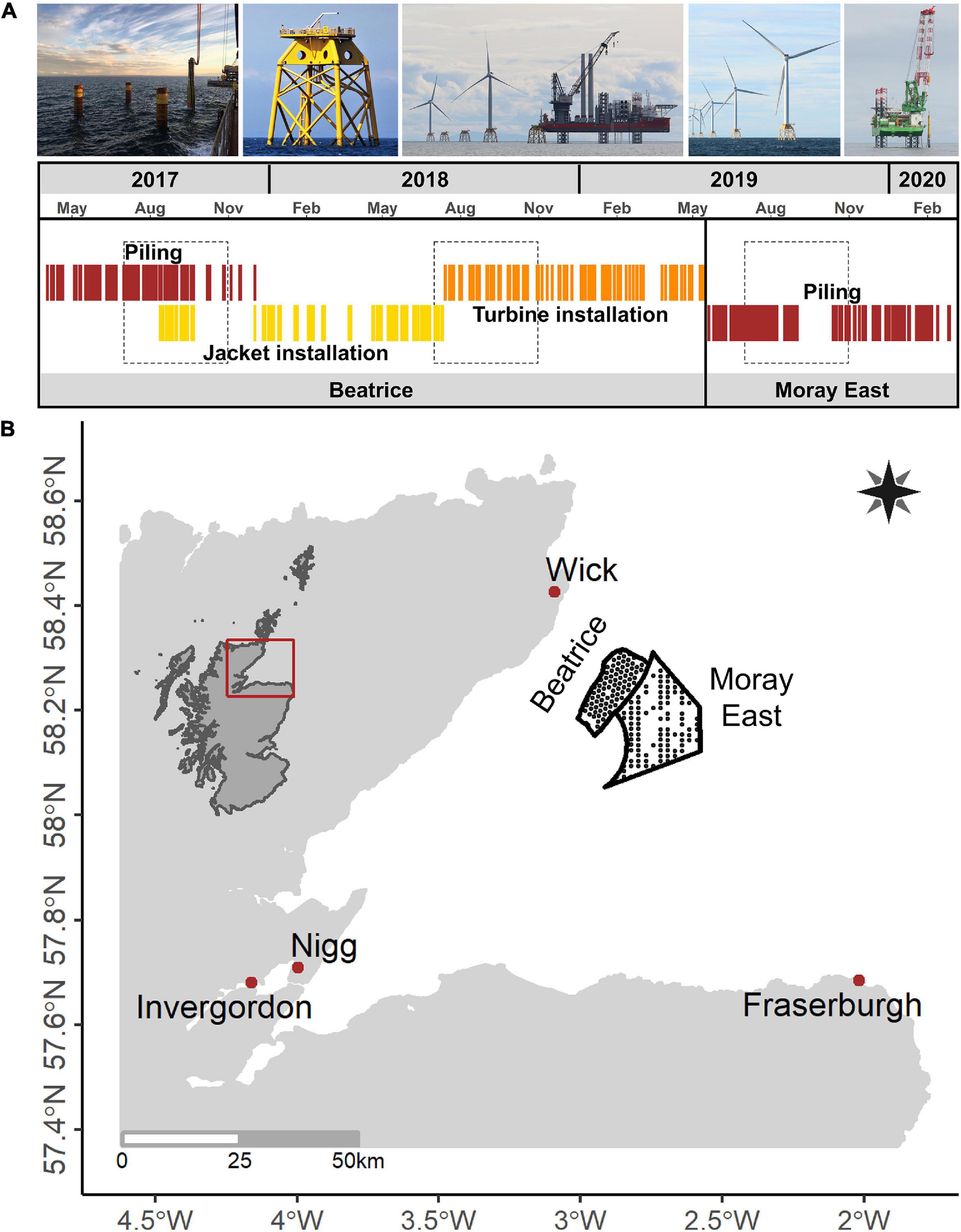
Figure 1. (A) Timeline of key construction activities at two offshore windfarms, between 2017 and 2019, i.e., pile-driving at Beatrice and Moray East (in red), jacket foundation installation at Beatrice (in yellow), wind turbine installation at Beatrice (in orange); the time periods used in the Before-After Control-Impact models to compare baseline periods with key construction activities are represented with dashed rectangles. (B) Map showing the location of the Moray Firth in Scotland, the Beatrice and Moray East windfarm boundaries (black line) and turbine locations (black dots), and the harbors used as construction bases (red dots); Source (first picture): Beatrice Offshore Wind Limited.
Passive Acoustic Monitoring
Sampling Design and Data Collection
Following the sampling design used by Thompson et al. (2013), we investigated variation in harbor porpoise occurrence and buzzing activity using arrays of echolocation click detectors (V.0 and V.1 CPODs1). These devices were deployed (a) in a 25 km by 25 km impact and reference block throughout construction in 2017, 2018, and 2019 and (b) along a gradient of exposure from construction activities within the two windfarm sites (Figure 2). These data were also compared to baseline data that had been collected in 2010 and 2011 to support Environmental Impact Assessments (EIA) (Beatrice Offshore Windfarm Limited, 2012; Moray Offshore Renewables Limited, 2012). In parallel, calibrated noise recorders (Ocean Instruments SoundTrap and Wildlife Acoustics SM2M) were deployed at three locations to characterize variation in underwater noise levels (Figure 3 and Supplementary Figures 1A,B).
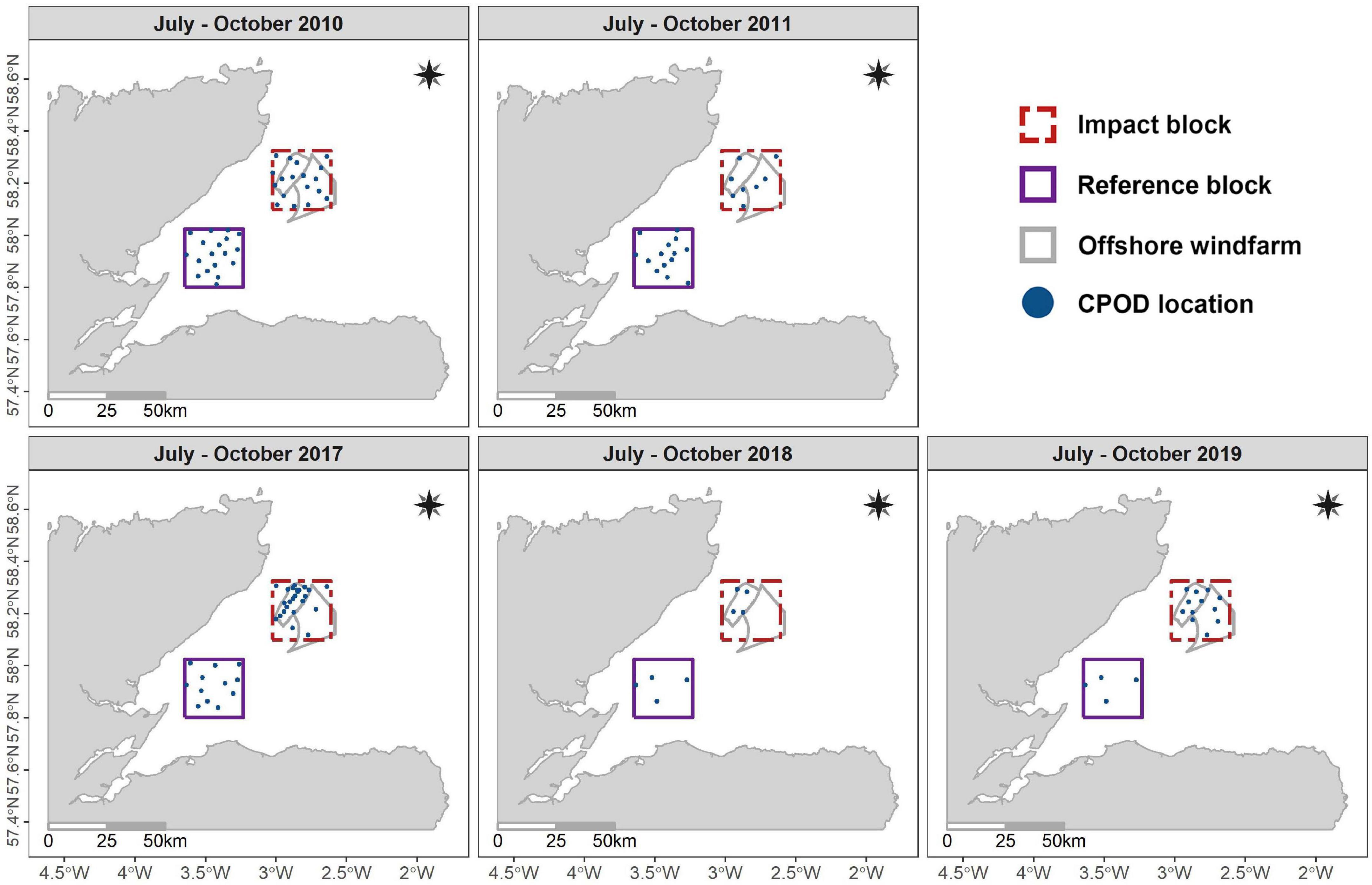
Figure 2. Spatio-temporal distribution of echolocation click detectors (CPODs) within the reference and impact blocks and the offshore windfarms between 2010 and 2019.
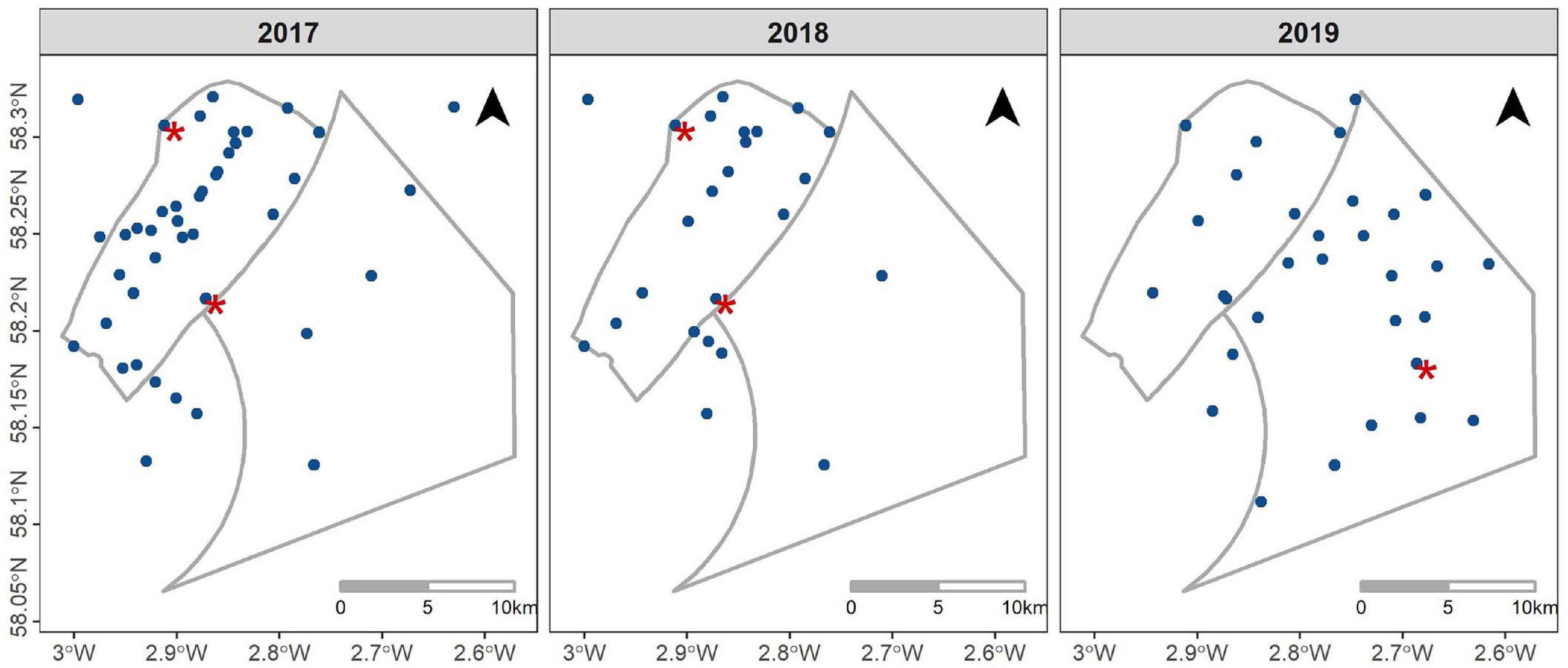
Figure 3. Spatio-temporal distribution of the Passive Acoustic Monitoring array, CPODs (blue circles) and noise recorders (red stars), within and around the two offshore windfarms between 2017 and 2019.
Measuring Variation in Harbor Porpoise Occurrence
Echolocation click characteristics (e.g., time of occurrence, duration, center frequency, bandwidth) logged by the CPODs were processed and extracted with the manufacturer’s software CPOD.exe (v2.044). The standard built-in “KERNO” classifier allocates click trains into one of four signal classes (Narrow Band High Frequency “NBHF,” “Other cetaceans,” “Boat Sonars” and “Unclassified”) and one of four quality categories (high “Hi,” moderate “Mod,” low “Lo” and doubtful “?”). No information on the design of the classifier is currently available, but based on the manufacturer’s CPOD manual (Tregenza, 2014), the classification algorithm searches for specific click parameters and inter-click intervals within trains (Clausen et al., 2019). High and moderate quality NBHF echolocation click trains of porpoise origin were extracted as Detection Positive Minutes per hour and then converted into presence-absence of porpoise detections per hour to assess hourly porpoise occurrence.
To extend endurance, CPODs are typically set up to log a maximum of 4,096 clicks per minute. This means that high levels of background noise can quickly saturate the CPODs and prevent any further data logging until the start of the next minute (Wilson et al., 2013). Additionally, the probability of detecting acoustic signals can be affected by the acoustic environment (Clausen et al., 2019). To estimate the distance at which CPODs were unlikely to saturate because of piling or construction vessel noise around each turbine site, we first extracted the number of unfiltered clicks (Nall) logged per minute by each device during the 10 months of piling activity in 2017. We then summarized these data in relation to distance from the piling vessel (Supplementary Figure 2) and took a conservative approach to prevent false-negative detections; discarding all data from CPODs within 2 km of the piling vessel and all hours with less than 60 min logged.
Estimating Variation in Harbor Porpoise Foraging Activity
We used variation in inter-click intervals (ICIs) to identify buzzes and provide a proxy for foraging activity (Pirotta et al., 2014b). The ICIs of logged NBHF click trains were calculated, normalized by natural log-transformation and categorized into three groups representing specific biological processes. The first group represents the high repetition rate click trains called buzzes that may be used for both foraging activity and social communication (Sorensen et al., 2018; Sarnocińska et al., 2020). Currently, it is not possible to distinguish between these two behaviors but, as in earlier studies (Pirotta et al., 2014a,b; Sarnocińska et al., 2020), we assumed that buzzes can be used as a proxy for foraging. The second group includes regular click trains and the third group represents the time between different click trains (Pirotta et al., 2014b). To identify the multimodal distribution of ICIs and allocate each ICI to one of the processes, Gaussian mixture models were fitted to the time series of ICIs, using the package mixtools (Benaglia et al., 2009) in R (v 3.6.0) (R Core Team, 2019). The number of component distributions k was initially set equal to three. However, at some locations, the low number of ICIs prevented the model from identifying the distribution centered on the buzz ICIs and so the number of components (k) was increased to four. If the model still did not discriminate the buzz ICI distribution using four components, data were pooled, so that datasets with higher proportions of buzz ICIs helped identify the buzz ICIs in datasets with overall lower numbers of detections. Additionally, when models did not converge after 1000 iterations, we increased the number of iterations to 2000 (and on one occasion reduced the convergence precision (epsilon) to 0.0001). Mixture models with 3 or 4 components were compared, choosing the model with the maximum loglikelihood. Results from the best model were then used to categorize each ICI into one of the three processes (e.g., Supplementary Figure 3) and the number of buzzes, regular and inter-train interval clicks were summarized per hour. The number of buzzes was converted into binary presence-absence of buzzes per hour, reducing the potential bias due to differences in sensitivity and detection range between acoustic devices and locations, respectively.
Before-After Control-Impact Analyses of Variation in Porpoise Occurrence and Foraging Activity in Relation to Different Phases of Windfarm Construction
For the BACI models, variation in both porpoise occurrence and foraging activity within each 25 km by 25 km block (Figure 2) were compared between the baseline and each monitoring phase. These analyses focused on data collected between July and October when comparable data were available in all years (see Table 1). Baseline data used in both windfarm EIAs were collected in 2010 and 2011 (Moray Offshore Renewables Limited, 2012). As seismic surveys were conducted in the current study’s reference block between 1 and 11 September 2011, we excluded these data from the analyses. For the BACI modeling, the Beatrice piling phase was from July to October 2017, during which 221 piling events occurred at 52 turbine locations and 24 jackets were installed. The turbine installation phase was from July to October 2018, during which 32 turbines and the last 6 jackets were installed. The Moray East piling phase, from July to October 2019, included 165 piling events at 47 turbine locations. No further construction work occurred at Beatrice during this time period, but operations and maintenance vessels visited the site regularly once it became fully operational in May 2019 (Figure 2).
Spatial Scale of Porpoise Responses to Different Piling Vessel Activities
An impact gradient approach was used to assess finer scale variation in porpoise occurrence and buzzing activity in relation to distance from the piling vessels at both Beatrice and Moray East as they undertook different construction activities in 2017 and 2019. The position of each piling vessel was extracted from an Automatic Identification System (AIS) vessel-tracking dataset for the Moray Firth region. The mean and minimum distance between each CPOD and the piling vessel were then calculated for each monitoring hour, using the sf package (Pebesma, 2018). Information on the activity of the vessels was extracted from the developers’ daily construction reports, and the factor “piling” or “no piling” was allocated to each hour monitored. Distance from the piling vessel was used as a proxy for the distance from construction activities (i.e., the noise/disturbance source), as the piling vessel was supported by two pilot vessels for anchoring, at Beatrice, and a tug bringing the piles on site. Hourly porpoise occurrence and buzzing activity were each modeled as a function of distance from the piling vessel in interaction with the vessel’s activity (“piling”/“no piling”) (Table 2).

Table 2. Sampling effort used for the gradient models, within the impact block, to assess harbor porpoise responses to pile-driving activities at Beatrice between March and December 2017 and Moray East between May and December 2019; mean harbor porpoise occurrence and mean foraging activity when porpoises were detected during and outside piling hours.
Characterizing Vessel Activity
To characterize variation in the extent to which harbor porpoises were exposed to both piling vessels and other construction vessels, we integrated data from the developers’ engineering records with AIS vessel-tracking data (Wright et al., 2019). AIS data for the entire Moray Firth were sourced at 5 min (2017) or 1 min (2018 and 2019) resolution from Astra Paging Ltd.2 and Anatec Ltd.3.
A 4 km by 4 km grid was created across the Moray Firth and the area of each grid cell calculated after any grid cells overlapping coastlines were cropped. AIS data were projected into WGS84 UTM 30N and then processed to produce hourly summaries a) within each of these grid cells and b) within a 5 km buffer around each of the passive acoustic monitoring sites. AIS data were interpolated every 5 min to calculate the time that each individual vessel stayed in a grid cell or buffer area. These data were then used to estimate measures of both vessel density and vessel intensity for each hour within each grid cell or buffer area. These two metrics provide complementary information highlighting variation in vessel behavior and distribution across the Moray Firth. At windfarm sites, construction-related vessels are often stationary for several hours, while other vessels (not involved in the construction) are likely to be transiting and consequently contribute less to the overall vessel intensity. Here, we defined vessel density as the number of individual vessels present in that hour per kilometer squared, and vessel intensity as the sum of residence times for all vessels present in that hour per kilometer squared. The minimum and mean distance from each CPOD or noise recorder to all vessels within each buffer area were also calculated and summarized for each hour and location.
Information on the vessels involved in the windfarm construction was extracted from the developers’ weekly construction reports and used to filter AIS data to provide separate measures of vessel density and intensity (a) for construction vessels and (b) for other third-party marine traffic. To estimate the vessel density and intensity within each of the windfarm construction sites, these AIS data were filtered by location, and vessels were categorized following Table 1 in Metcalfe et al. (2018).
Variation in Background Noise Levels at the Construction Site
Underwater broadband noise recorders were deployed for periods of 2–6 months at three sites within the impact block to characterize noise levels in different phases of construction (Table 3, Figure 4, and Supplementary Figure 1). Recorders collected data at sampling rates of either 48 or 96 kHz, with duty cycle rates varying depending upon device and sampling rates (Supplementary Table 2). Data were processed in MATLAB following Merchant et al. (2015). Broadband noise levels were quantified between 25 Hz and 24 kHz to provide hourly root-mean-square (RMS) averaged sound pressure levels (SPL) in decibels (dB) relative to a reference pressure of 1 μPa (Kinsler et al., 1999; Merchant et al., 2015).
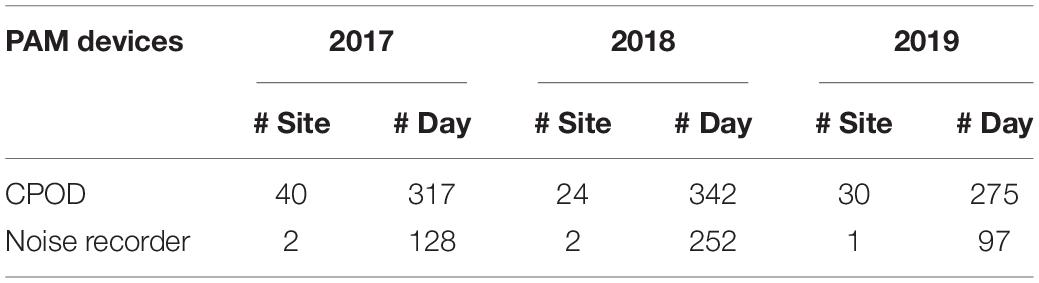
Table 3. Sampling effort to investigate harbor porpoise responses to vessel activity and underwater broadband noise levels.
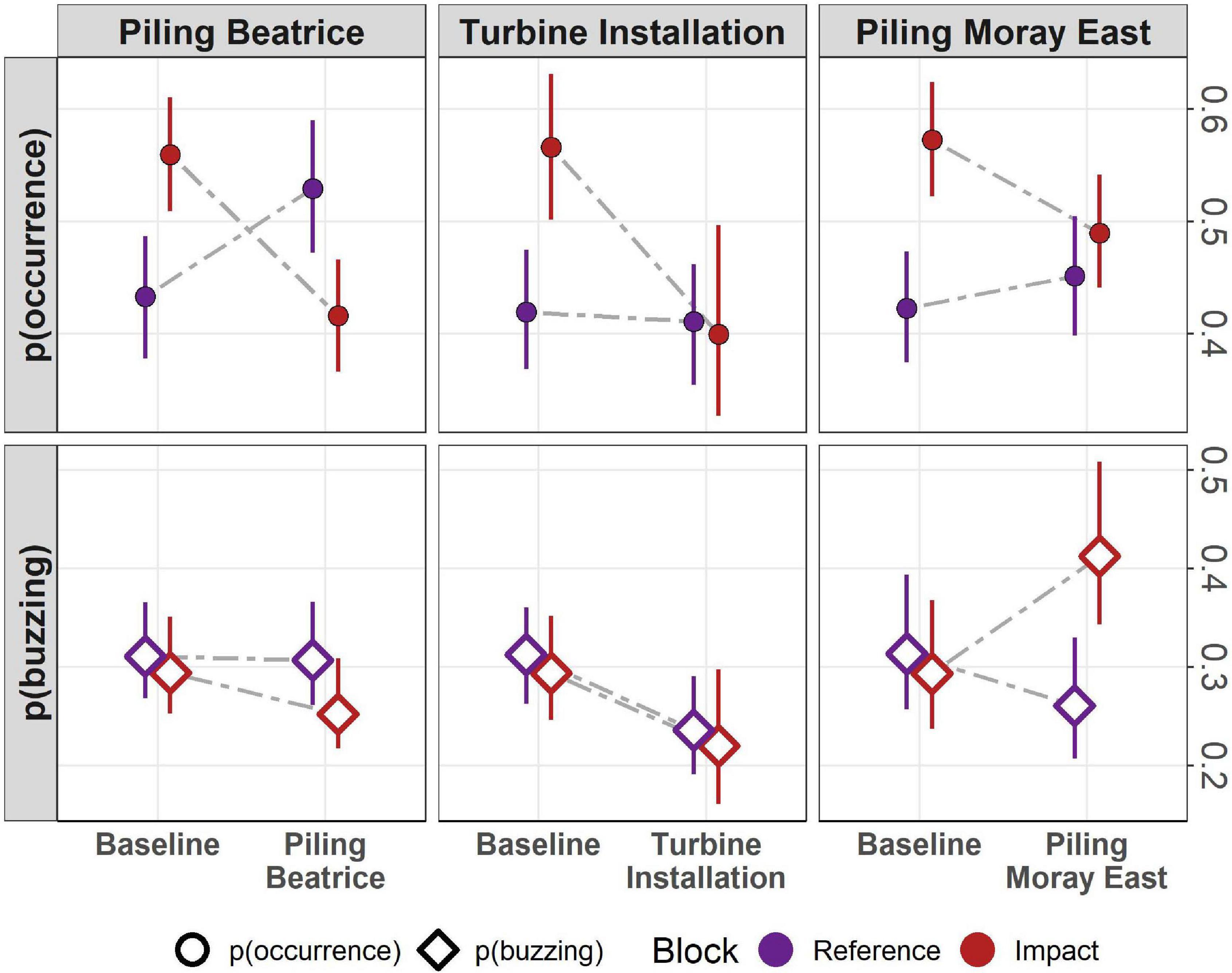
Figure 4. The probability of harbor porpoise occurrence (circle) and buzzing activity (diamond) per hour between the reference (in purple) and impact (in red) blocks and between the baseline monitoring period and key construction activities (i.e., pile-driving and wind turbine installation at Beatrice offshore windfarm and pile-driving at Moray East offshore windfarm).
Modeling
BACI Models
To compare between the baseline and each construction phase, the hourly occurrence of porpoise detections and buzz detections were modeled as binomial response variables using six Generalized Linear Mixed-effects Models (GLMM). For each model, the link function was chosen based on the lowest Akaike Information Criterion (AIC), using either the cloglog or probit link function. The interaction between block and construction phase was used as the explanatory variable in all models.
Based on previous studies and preliminary data analyses, it was known that porpoises display diel and seasonal patterns in occurrence and foraging activity in this study area (Williamson et al., 2017; Graham et al., 2019). To focus on changes associated with the windfarm construction, month and diel phase were used as random factors in the BACI model. Diel phase (i.e., sunrise, day, sunset, or night) was allocated based on local sunrise and sunset times. Additionally, the CPOD location was also used as random effect to control for any site-specific environmental differences. To assess the significance of fixed effects and their interactions, a sequential analysis of deviance table (Type II Wald chi-square tests) was computed using the R package car (Fox and Weisberg, 2019). For each model, the response variable was predicted and the uncertainty (95% confidence intervals, CI) calculated using a bootstrapping approach (100 simulations) with the bootMer function of the lme4 package (Bates et al., 2015). To validate models, we checked for temporal autocorrelation in model residuals using the partial auto-correlation function. The DHARMa package was used to verify the uniformity, dispersion, spatial and temporal autocorrelation of residuals (Hartig, 2020).
Impact Gradient Models
To investigate the spatial scale of the effects of pile-driving, vessel activity and underwater noise on harbor porpoise occurrence and foraging activity, hourly occurrence of porpoise detections and buzz ICIs were modeled as a function of: (1) the interaction between the distance from construction activities and piling occurrence (piling effect models); (2) the interaction between the vessel intensity per hour and the mean distance from vessels (vessel effect models); (3) the averaged broadband sound pressure levels and piling occurrence (noise effect models).
For the piling effect models, we only considered sites within the impact block to investigate meso-scale response to construction activities. Additionally, we filtered the dataset to explore the magnitude of porpoise responses between 2 and 30 km from the piling vessel as it has been shown in other studies that a response to piling activities was apparent up to 26 km from piling (Tougaard et al., 2009; Dähne et al., 2013; Haelters et al., 2015; Brandt et al., 2016, 2018). Similarly, to investigate porpoise occurrence and activity in relation to broadband noise levels and the presence or absence of piling activity, we used data from the CPODs that were deployed at the same location as the noise recorders (Figure 3). Hours in which piling activities occurred within 2 km of these sites were not used in the noise effect models.
For the vessel effect models, we excluded hours in which piling activities occurred to focus only on the effect of vessels. To prevent masking effects of vessel noise on porpoise echolocation clicks, we followed Pirotta et al. (2014a) and excluded hours in which vessels were within 1 km of CPOD locations.
For the six models, binary generalized linear models with either a probit or cloglog link function were fitted using generalized estimating equations (GEE-GLMs), to account for temporal autocorrelation. The correlation structure was selected based on the lowest Quasi Information Criterion (QICr), resulting in using an “independence” correlation structure for all models. To determine whether the two-way interaction term should be retained, we used the dredge function of the MuMIn package, which ranks all model possibilities according to QIC (Barton, 2020). Year, Julian day and site ID were used to define a blocking variable in the GEE, allowing model residuals from each site within each day to be autocorrelated. Wald’s tests were used to assess significance and bootstrapped coefficients from the GEE-GLM were used to estimate uncertainty (95% CI) and plot relationships between response and explanatory variables.
Results
Variability in Porpoise Occurrence and Foraging Activity Between Different Phases of Windfarm Construction
In the BACI comparison, variation in harbor porpoise occurrence and foraging activity was best explained by the interaction between block and construction phase (Figure 4). The baseline probability of occurrence prior to any construction was around 0.42 in the reference block and 0.55 in the impact block, while the baseline probability of detecting buzz ICIs, when porpoises were present, was around 0.3 for both blocks. In comparison with the baseline, harbor porpoise occurrence significantly decreased by 14.3% in the impact block during the Beatrice piling phase (Wald test: χ2 = 725.267, p < 0.001) and by 8% during the Moray East piling phase (Wald test: χ2 = 126.024, p < 0.001). A decrease in porpoise occurrence (−16.7%) was also observed between the baseline and the Beatrice turbine installation phase (Wald test: χ2 = 6.269, p = 0.012). Despite these significant decreases, harbor porpoises were regularly detected within these construction sites throughout the monitoring period (Supplementary Figure 4).
When porpoises were present, the probability of detecting buzz ICIs also significantly decreased by 4.2% between the baseline and piling phase at Beatrice (Wald test: χ2 = 14.216, p < 0.001), although no significant change in buzzing activity was observed during turbine installation (Wald test: χ2 = 0.009, p = 0.923). In contrast, during the Moray East piling phase, the probability of detecting buzzing ICIs increased by 11% in the impact block but decreased by 5% in the reference block (Wald test: χ2 = 176.517, p < 0.001) (Figure 4).
Spatial Scale of Porpoise Responses to Different Piling Vessel Activities
During the construction period, finer-scale variation in harbor porpoise occurrence and foraging activity were best explained in the piling effect model by the interaction between the distance from the piling vessel and the presence or absence of piling (Beatrice: porpoise occurrence—Wald test: χ2 = 482, p < 0.001; buzzing activity—Wald test: χ2 = 18.3, p < 0.001. Moray East: porpoise occurrence—Wald test: χ2 = 421, p < 0.001; buzzing activity—Wald test: χ2 = 6, p < 0.014). During piling activity, the probability of porpoise occurrence increased significantly with distance from the source vessel in a similar fashion at both sites. When there was no piling activity, occurrence still decreased slightly closer to the vessel (−9.3% at Beatrice; −20.9% at Moray East) (Figure 5). During piling activities, when harbor porpoises were acoustically detected, buzzing activity at Beatrice decreased by 54% close to the piling vessel, but this effect was not as strong at Moray East (−40%). Again, when there was no piling activity, the probability of buzzing ICIs at both sites was slightly lower closer to the piling vessel (−19.6% at Beatrice; −22.7% at Moray East) (Figure 5).
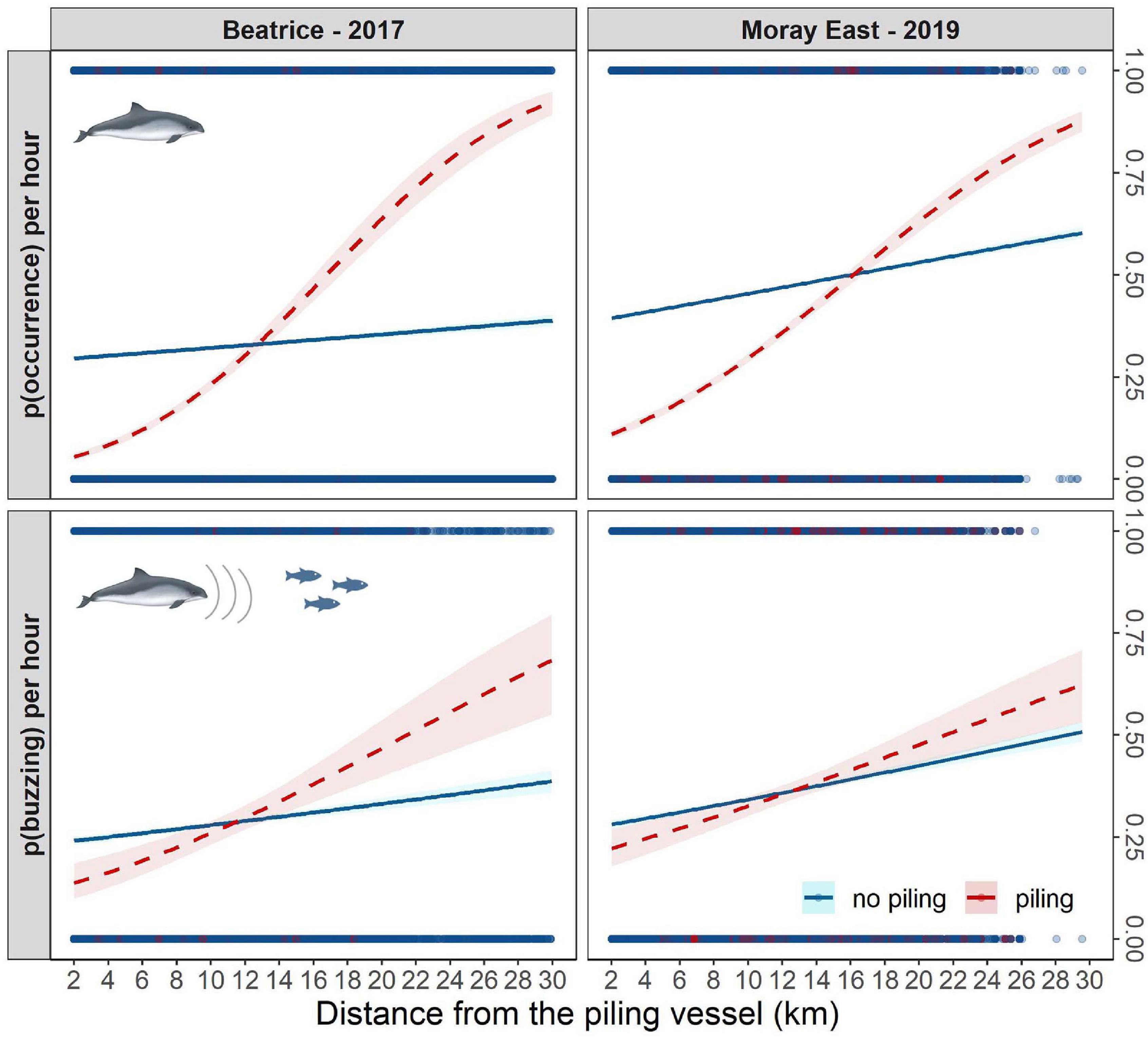
Figure 5. The probability of harbor porpoise occurrence and buzzing activity per hour during (dashed red line) and outwith (blue line) pile-driving hours, in relation to distance from the pile-driving vessel at Beatrice (left) and Moray East (right); confidence intervals (shaded areas) estimated for uncertainty in fixed effects only; points represent the raw data distribution along the distance gradient during piling (red) and no piling (blue) activities; see the raw data frequency distribution in Supplementary Figure 6.
Response of Porpoises to Vessel Activity at Windfarm Construction Sites
Figures 6A,B summarize the broad-scale spatial variation in the intensity and density of construction-related vessels across the Moray Firth between 2017 and 2019 (for further information on the overall vessel density and intensity across the Moray Firth between 2017 and 2019, see Supplementary Figures 5A,B). Over this period, median construction-related vessel density was 1.4 vessels.km–2 (range 0.06–64.8 vessels.km–2) across the Moray Firth. Vessel density was highest in 2019 when both windfarms were under construction. Similarly, the median construction-related vessel intensity, across the Moray Firth, was 2.2 h.km–2 (range 0–29,006.8 h.km–2). Most vessels occurred over the windfarm sites, but construction-related vessels also worked along export cable routes and between local ports and harbors, including Wick, Invergordon and Fraserburgh (Figure 1B).
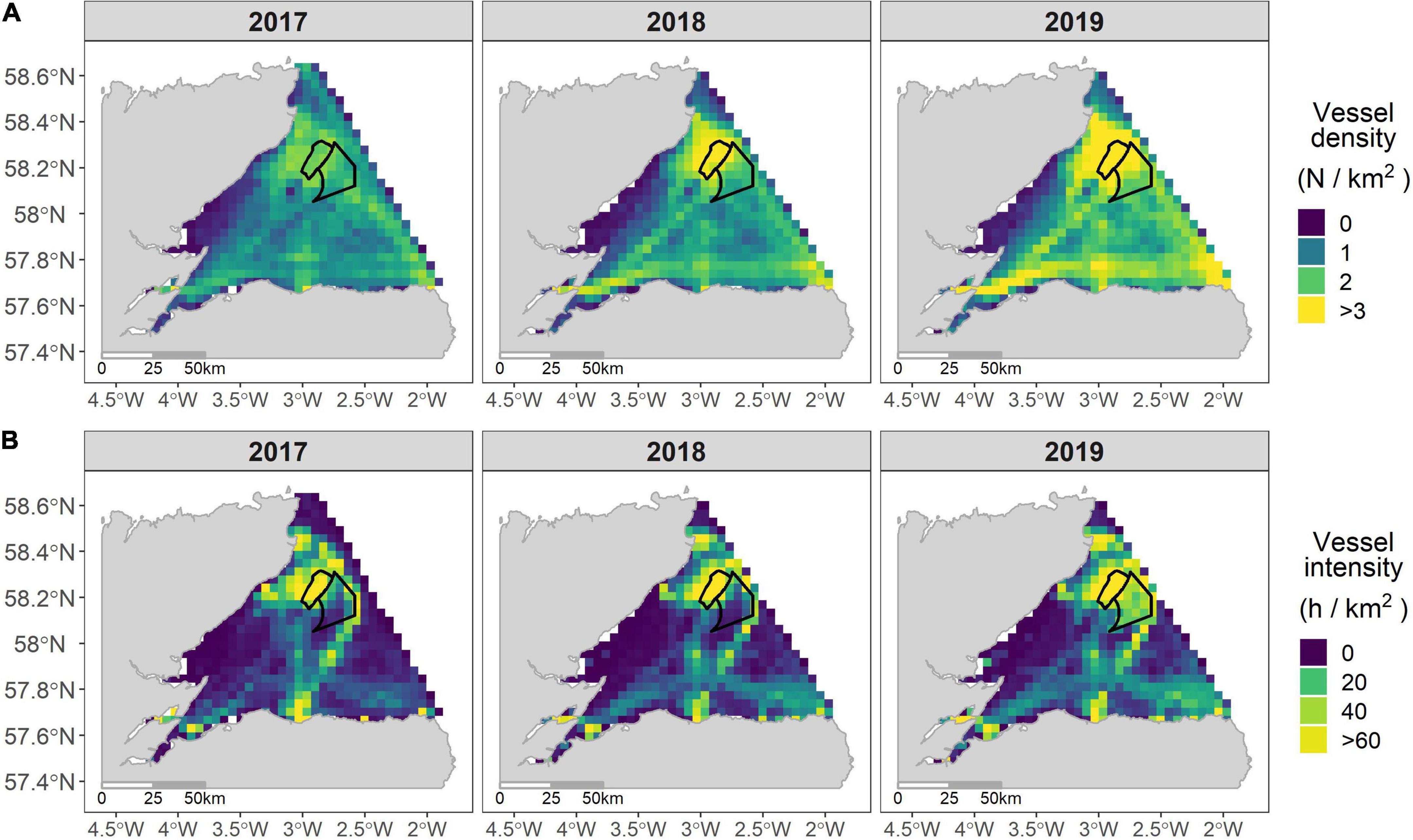
Figure 6. Construction-related vessel density (number of vessels/km2) (A) and intensity (h/km2) (B) in the Moray Firth (4 × 4 km grid) between 2017 and 2019; Black lines are the boundaries of the two offshore windfarms in development; the upper limit of both the vessel density and intensity color scales is greater than 95th percentile.
In addition to the key offshore service vessels used for pile-driving and jacket or turbine installation, construction-related vessel traffic included fishing vessels working as guard vessels, passenger vessels for crew-transfers and some port service craft or unassigned vessels. Details of vessels used in each development are included in Supplementary Table 3.
Safety zones of 500–1,500 m were maintained around structures under construction, and of 50 m around installed structures waiting to be commissioned. Nevertheless, both the Beatrice and Moray East sites remained accessible to fishermen and other third-party vessel traffic throughout their construction. Within the two windfarm sites, the density of fishing, bulk carrier, cargo and unassigned vessels that were not involved in the construction ranged from 0.15 to 0.21 vessels.km–2 between 2017 and 2019. Fishing vessel density decreased at Beatrice in 2018 and 2019 and at Moray East in 2019. However, parallel increases in the intensity of fishing vessels suggested that the fishing vessels that were present spent more time in the area (Table 4).
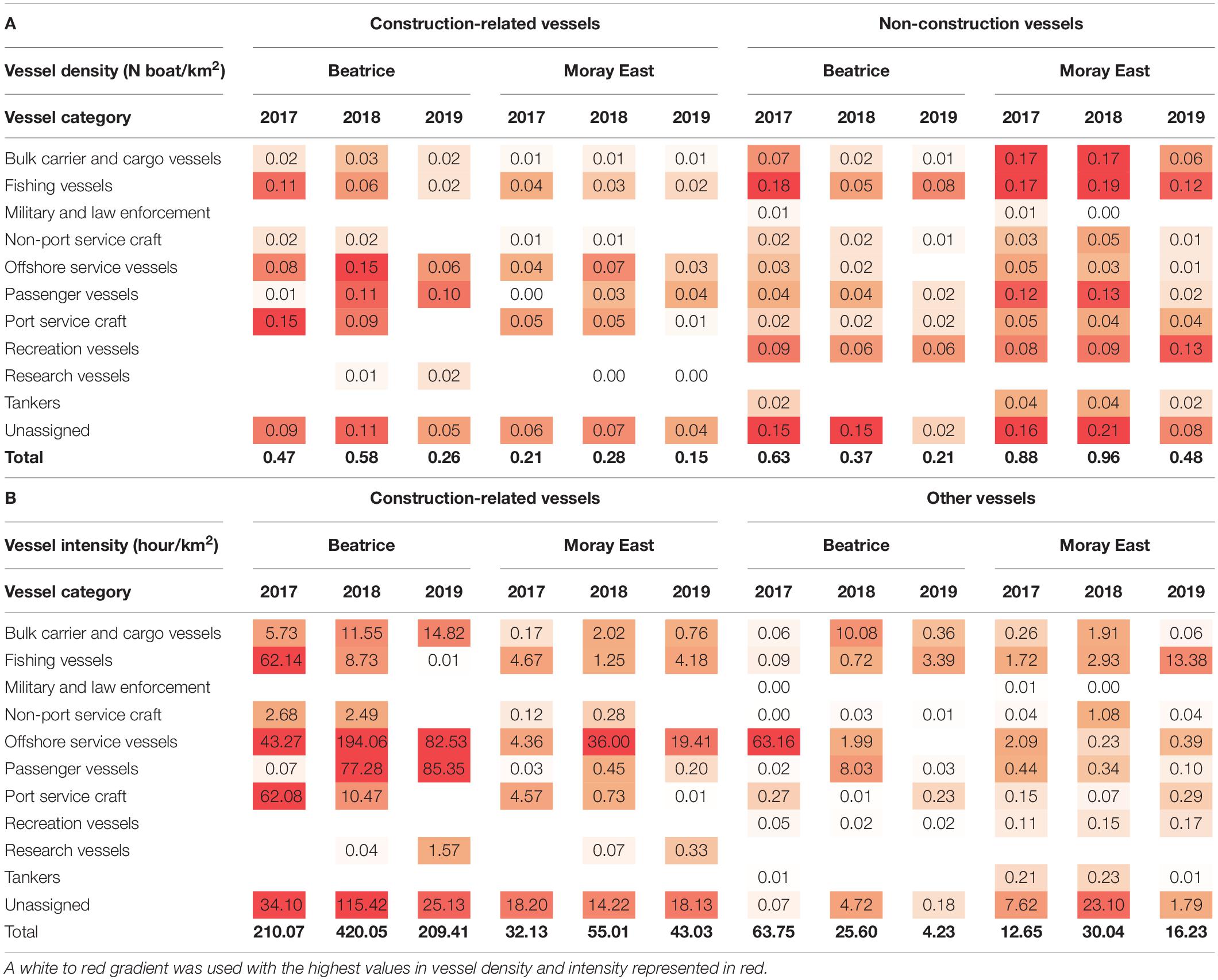
Table 4. Density (A) and intensity (B) of vessels involved or not involved in the construction at Beatrice and Moray East offshore windfarms between 2017 and 2019, grouped by vessel category.
During the 245- and 284-day pile-driving campaigns, the piling vessel was within the windfarm footprint for around 4,090 h (69.5% of the time) at Beatrice and 6,525 h (95.7% of the time) at Moray East. However, it should be noted that the piling vessel at Moray East was jacked-up for most of this time. The total number of hours in which piling occurred was around 437 h (7.4% of the time) at Beatrice and 773 h (11.3% of the time) at Moray East.
Estimates of vessel intensity around each of the passive acoustic monitoring sites were similar in 2017 and 2019 (Figure 7A), with the third quantile around 1.21–1.28 min.km–2 and a peak between 0.6 and 0.9 min.km–2. Although the shape of the distribution of vessel intensities in 2018 was similar, the third quantile was around 1.78 min.km–2, highlighting that vessel activity at Beatrice was higher during the installation of jackets and turbines. There was also spatial variability in vessel density and intensity between years and sites (Figure 7B). In 2017, the higher levels of vessel intensity occurred across Beatrice but in 2018 was more localized around the south-east boundary of the windfarm. In 2019, vessel intensity was spread across the two windfarm sites, but levels of vessel intensity remained highest at Beatrice (Figure 7B).
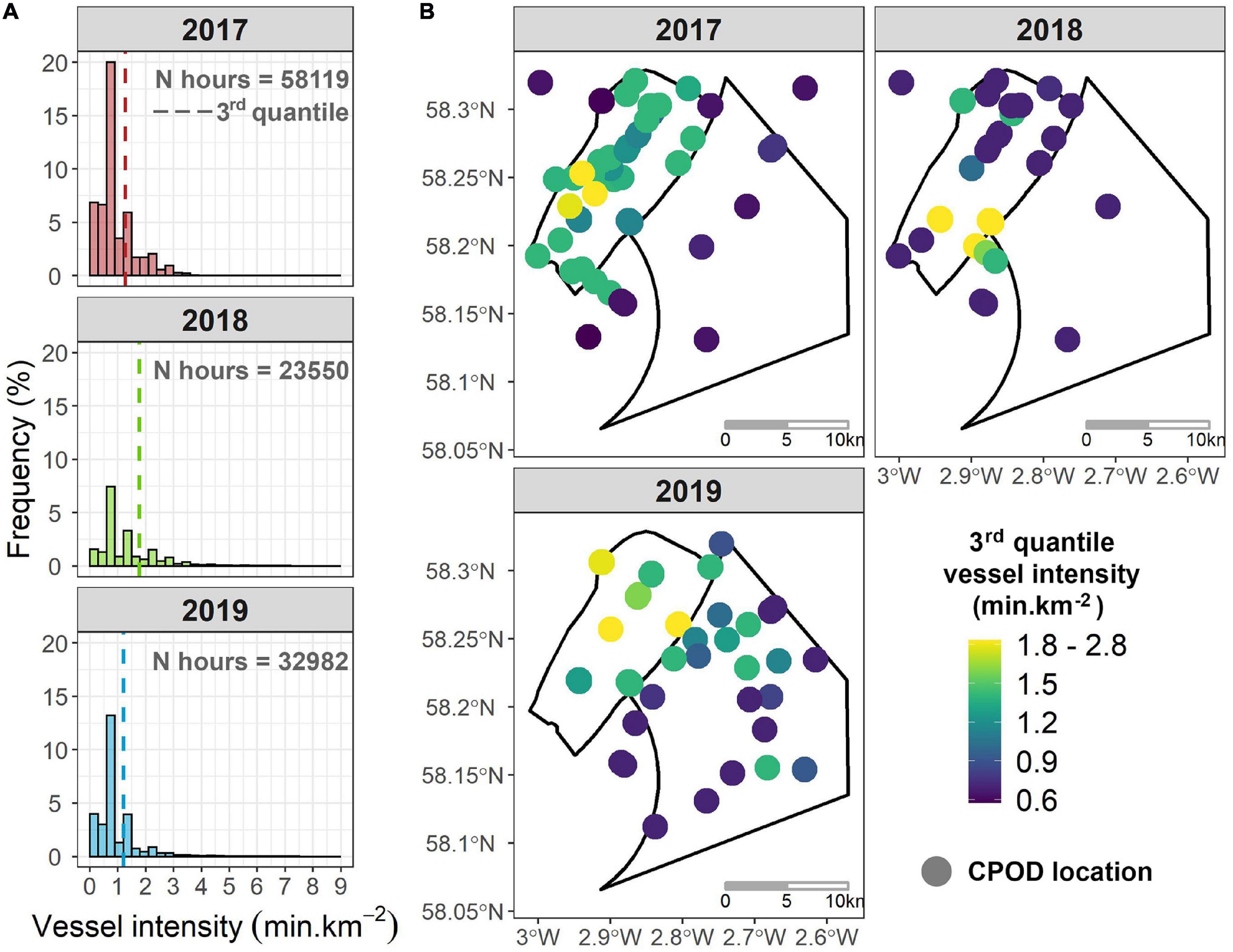
Figure 7. (A) Histograms of the vessel intensity (min.km– 2) per hour in 2017 (pink), 2018 (green), 2019 (blue); dashed lines represent the third quantile of vessel intensity per hour; the sample size is showed by the number of hours monitored per year (N hours); (B) spatio-temporal distribution of the vessel intensity per hour within and around the two offshore windfarms between 2017 and 2019; the color gradient indicates the annual third quantile of vessel intensity within a 5 km buffer of each CPOD site.
Based on the best fit vessel effect model, finer-scale variation in harbor porpoise occurrence within the windfarm sites was explained by the interaction between the vessel intensity and the mean vessel distance from each CPOD site within a 5 km buffer area (Wald test: χ2 = 73.3, p < 0.001) (Figure 8A). At a mean vessel distance of 2 km, porpoise occurrence decreased by up to 35.2% as vessel intensity increased, decreasing from 0.37 (95% CI: 0.36–0.39) when vessel intensity was zero (0 min.km–2) to 0.02 (95% CI: 0.01–0.05) for a vessel intensity of 9.8 min.km–2. Porpoise responses decreased as the mean vessel distance increased (−24% at 3 km) until no apparent response was observed at 4 km (+ 7.2%).
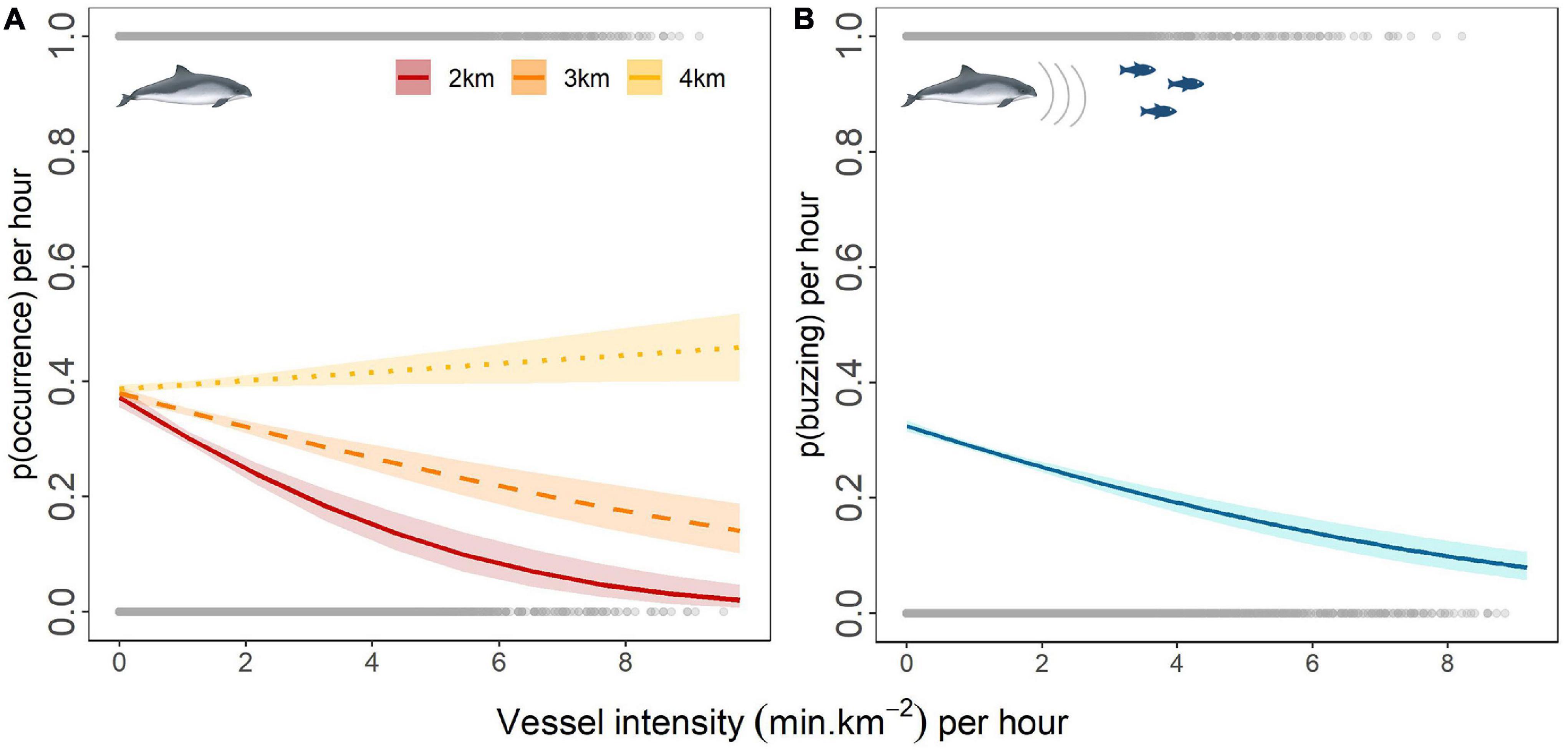
Figure 8. The probability of harbor porpoise occurrence per hour in relation to vessel intensity per hour at a mean vessel distance of 2 km (red line), 3 km (dashed orange line), 4 km (dotted yellow line) (A); the probability of buzzing activity per hour in relation to vessel intensity (blue line) (B); confidence intervals (shaded areas) estimated for the uncertainty in fixed effects only; gray points represent the raw data distribution along the vessel intensity gradient; see the raw data frequency distribution in Supplementary Figures 7, 8.
Vessel intensity also had a significant effect on the probability of buzzing (Wald test: χ2 = 110, p < 0.001). Throughout the 3 years, the probability of detecting buzzes in each hour that porpoises were present decreased by up to 24.5%, from 0.32 (95% CI: 0.32–0.33) when vessels were absent to 0.08 (95% CI: 0.06–0.11) for hours with a vessel intensity of 9.17 min.km–2 (Figure 8B). Mean distance to the vessel had no significant effect on the probability of detecting buzzes.
Variation in Occurrence and Foraging Activity of Porpoises in Relation to Noise
Both averaged broadband sound pressure levels in each hour (Wald test: χ2 = 28.4, p < 0.001) and piling occurrence (Wald test: χ2 = 57.7, p < 0.001) had significant effects on the probability of detecting harbor porpoises. Outside piling hours, porpoise detections decreased by 17% as SPL increased, decreasing from 0.44 (95% CI: 0.39–0.48) at 102 dB re 1 μPa to 0.26 (95%CI: 0.2–0.34) at 159 dB re 1 μPa. During piling activities, porpoise occurrence was initially lower (0.16 95% CI: 0.11–0.23 at 102 dB re 1 μPa) and decreased by 9% as SPL increased by 59 dB (0.07 95% CI: 0.04–0.12 at 159 dB re 1 μPa) (Figure 9A).
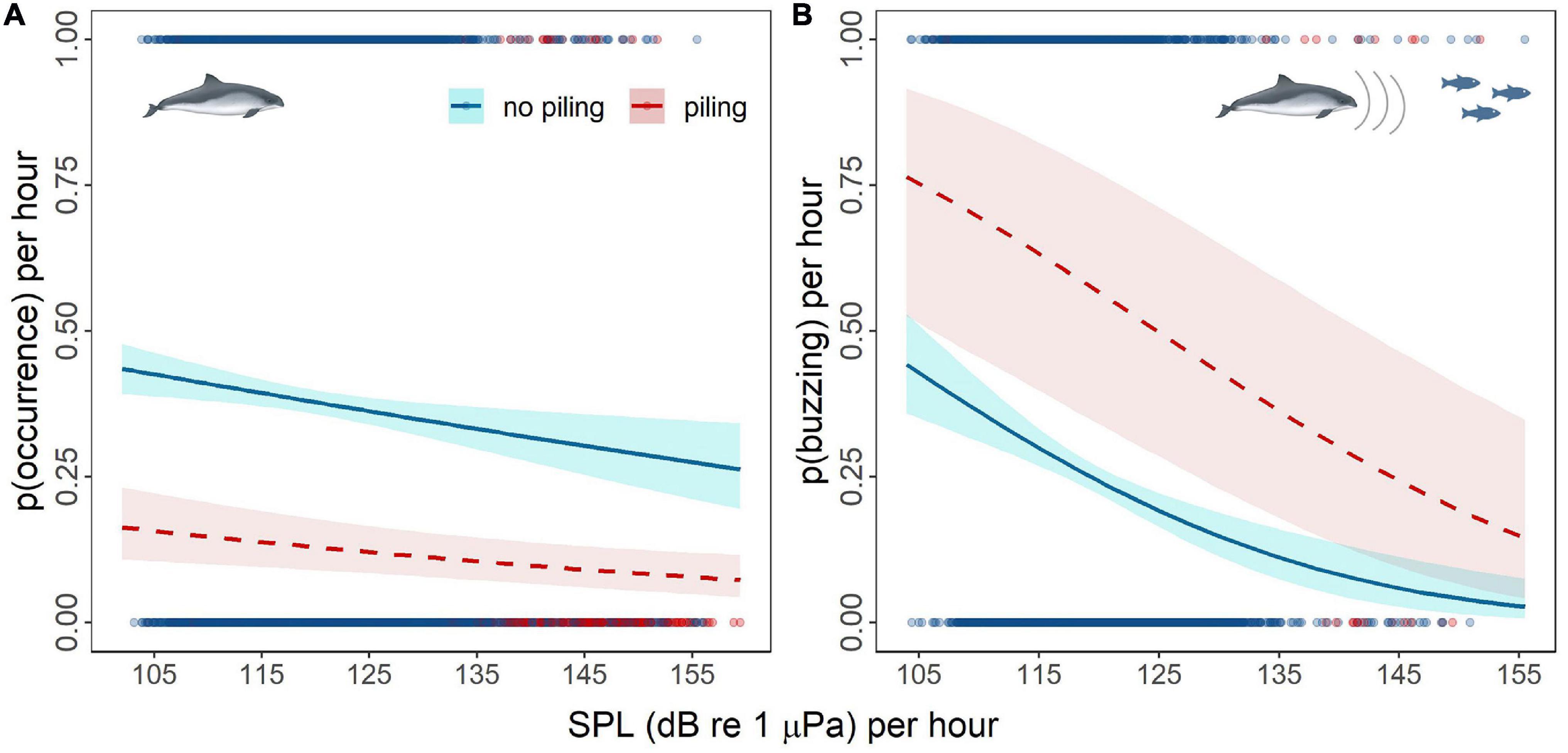
Figure 9. The probability of harbor porpoise occurrence (A) and buzzing activity (B) per hour in relation to the broadband sound pressure level (SPL) per hour during (dashed red line) and outside (blue line) pile-driving hours; confidence intervals (shaded areas) estimated for the uncertainty in fixed effects only; points represent the raw data distribution along the SPL gradient during piling (red) and no piling (blue) activities; see the raw data frequency distribution in Supplementary Figure 9.
Similarly, variation in the probability of detecting buzzes was also explained by both SPL (Wald test: χ2 = 19.53, p < 0.001) and piling occurrence (Wald test: χ2 = 8.73, p < 0.01). However, while the probability of detecting buzzes decreased with increasing noise levels in either the presence or absence of piling, porpoises detected during piling exhibited higher levels of buzzing activity (Figure 9B). Outside piling hours, the probability of detecting buzzes decreased by up to 41.5% as the SPL increased, ranging from 0.44 (95% CI: 0.36–0.53) at 104 dB re 1 μPa to 0.03 (95% CI: 0.01–0.08) at 155 dB re 1 μPa. During piling hours, buzzing occurrence decreased by up to 61.8% as the SPL increased, ranging from 0.76 (95% CI: 0.53–0.92) at 104 dB re 1 μPa to 0.15 (95% CI: 0.04–0.35) at 155 dB re 1 μPa (Figure 9B).
Discussion
Uncertainties exist over the extent to which marine mammal occurrence and foraging activity varies through different phases of offshore windfarm construction. This, in turn, currently constrains efforts to balance the development of renewable energy to meet carbon reduction targets with the need to minimize disturbance to protected wildlife populations. Our BACI analyses provide evidence of broad-scale behavioral responses of harbor porpoises both to pile-driving and other construction-related activities (Figure 4). In addition, impact gradient analyses show that the magnitude of response varied depending on the activity type and distance from the disturbance source (Figure 5), and the cumulative pressure associated with vessels (Figure 8) and anthropogenic noise (Figure 9). Together, these analyses allowed us to quantify response levels during different construction contexts, while also highlighting that harbor porpoises continued to regularly use these sites throughout the 3-year construction period (Supplementary Figure 4). These findings now provide new data to parameterize energetics and population simulation models [e.g., DEPONS Nabe-Nielsen et al. (2018) and iPCoD Booth et al. (2017)] that can explore potential population-level consequences of these cumulative disturbances.
Changes in Porpoise Occurrence During the Two Piling Campaigns
As expected from previous studies of harbor porpoise responses to impulsive noise both at this (Thompson et al., 2013; Graham et al., 2019) and other (Tougaard et al., 2009; Brandt et al., 2011; Dähne et al., 2013; Haelters et al., 2015; Nabe-Nielsen et al., 2018). North Sea sites, our BACI analyses demonstrated a significant decrease in porpoise detections when pile-driving occurred at both the Beatrice and Moray East windfarm sites (Figure 4). In the BACI analyses, the observed changes in porpoise occurrence and buzzing activity between blocks and monitoring periods may be confounded by the varying sampling effort although similar relationships were found using the impact gradient analyses. In PAM based studies such as this, it is recognized that short-term decreases in acoustic detections could result from animals ceasing to vocalize rather than being displaced. However, given the high energetic requirements and foraging rates of harbor porpoises (Booth, 2020), the broader-scale changes observed here are most likely to result from avoidance behavior leading to lower densities over the impact block. In the German North Sea, the decrease in relative porpoise acoustic detection rates within 10 km of the pile-driving noise source, was associated with an increase in detection rates at 25 and 50 km distance, and matched the lower porpoise density, observed through visual aerial surveys, within 20 km of the noise source (Dähne et al., 2013). Similar findings were observed in response to seismic surveys over a range of 5–10 km in Thompson et al. (2013) and in response to a seal scarer in Brandt et al. (2013). In these studies, the aerial surveys supported the assumption that porpoises exposed to anthropogenic noise sources such as pile-driving, airgun and acoustic deterrent devices left the ensonified area rather than ceasing vocalizing. Consequently, even though our study relied solely on PAM data, decreases in acoustic detections in response to impulsive noise disturbance is likely to result from displacement. Approaches used for assessing the spatial scale of responses to piling have varied across studies. Nevertheless, our observed responses at distances of 10–15 km at both sites (Figure 5) are of a similar order of magnitude to results from the subset of Beatrice sites analyzed in Graham et al. (2019) and those from other North Sea windfarms (Tougaard et al., 2009; Brandt et al., 2011; Dähne et al., 2013; Haelters et al., 2015; Nabe-Nielsen et al., 2018). One limitation of using CPODs in these situations is that elevated background noise close to piling locations may affect detection probability (Clausen et al., 2019). When analyzing the magnitude of near-field responses to pile-driving (Figure 5), we accounted for this by excluding data from all CPOD locations that were within 2 km of the piling vessel.
Although patterns in porpoise detections were similar during the piling campaigns at the two Moray Firth sites, the magnitude of change in porpoise occurrence during the Moray East piling phase was lower than at Beatrice (Figure 4). Graham et al. (2019) showed that responses to pile driving noise at Beatrice diminished through 2017. The scale of response at Moray East in 2019 may therefore be smaller due to the increased tolerance of individuals remaining in the area (Bejder et al., 2009). However, little is known of broader-scale movement patterns of North Sea porpoises (see Sveegaard et al., 2011) and it is not currently possible to follow individual porpoises over multiple years. Thus, it remains unclear whether or not displaced individuals returned to impacted areas or whether porpoises exposed in 2017 were still present in subsequent years (Graham et al., 2019). Alternatively, variation in the magnitude of response in the two piling phases could result from local changes in habitat quality or other differences in the nature of the disturbance during pile-driving. For example, our impact block was large, and included both windfarms. Thus, during the 2019 Moray East piling phase, the northern part of the impact block also contained 86 operational structures within the Beatrice windfarm. Harbor porpoise occurrence in this part of the impact block could therefore have increased, as seen in the Egmond aan Zee windfarm in Dutch waters (Scheidat et al., 2011). Scheidat et al. (2011) suggest that such changes could result from increases in prey due to artificial reef effects within established windfarms, or because most shipping is excluded from Dutch windfarm sites; potentially allowing porpoises to shelter from vessel disturbance. However, our analysis of AIS data suggests that a sheltering effect is unlikely in the Moray Firth as there continued to be high levels of windfarm and third-party traffic over the Beatrice site in 2019 (Figure 6). Finally, the two piling campaigns used different installation infrastructure that may explain observed differences between the responses in 2017 and 2019. The piling vessel at Beatrice used eight anchors, requiring the presence of additional pilot and anchor-handling vessels, while an independent jack-up piling vessel was used at Moray East. Our study design did not enable us to discriminate between the fine and meso-scale spatio-temporal impact of this diverse range of construction-related activities, but these findings highlight the need for further work to explore how different pile installation techniques may affect the scale of response.
Changes in Porpoise Activity During the Two Piling Campaigns
Building on previous work that has focused on displacement during pile-driving, we also used information from the echolocation click characteristics to explore broad-scale changes in the activity of those porpoises that continued to use the windfarm sites. During an earlier seismic survey in this area, harbor porpoise occurrence decreased close to the noise source (Thompson et al., 2013), and animals remaining in exposed areas also exhibited a decrease in buzzing activity (Pirotta et al., 2014a). In the present BACI study, porpoises that remained in the impact block during pile-driving at Beatrice in 2017 also reduced their buzzing activity by 4.2% compared to baseline but, in contrast, buzzing activity during Moray East piling in 2019 was higher than baseline (Figure 4). As discussed in relation to differences in the magnitude of displacement during the two piling campaigns, differences in buzzing activity of porpoises remaining in the impact area may also result from local changes in habitat quality. The introduction of hard substrates (e.g., jacket foundations and scour protection) are likely to have enhanced the fine-scale habitat and changed fish assemblages, potentially increasing the prey availability for opportunistic and generalist feeders such as porpoises (Santos and Pierce, 2003) and explaining higher buzzing activity during this period. Better understanding of any reef effects following construction is now urgently required so that potential ecosystem benefits can be integrated into an evaluation of the lifetime cumulative impacts of windfarm construction and operation on these populations (King et al., 2015; Booth et al., 2017; Nabe-Nielsen et al., 2018).
Within the impact area, gradient analyses of data collected through both piling phases also suggest that the probability of detecting buzzes decreased by 54% with decreasing distance from the piling vessel (Figure 5), and by 61.8% as hourly RMS SPL increased from 104 to 155 dB re 1 μPa (Figure 9B). Thus, individuals remaining nearest exposed areas did spend less time buzzing, while porpoises displaced from exposed areas increased their buzzing activity, potentially compensating for lost foraging opportunities or increased energy expenditure. During extended periods of disturbance, porpoises must make trade-offs between fleeing, either permanently or temporarily, or remaining in areas that have a higher risk of disturbance or predation. Baseline distribution patterns suggest that the vicinity of both impact and reference areas represent high-quality feeding habitat (Brookes et al., 2013), and fleeing the area may incur high energetic costs and the risk of spending time in lower-quality habitat. Individual responsiveness to anthropogenic disturbances is therefore likely to be context-dependent and related to animal fitness (van Beest et al., 2018). Theoretically, individuals in poorer condition are less likely to leave high-quality habitats after a disturbance, as the energetic cost and risk of missing foraging opportunities may be too high (Gill et al., 2001; Beale and Monaghan, 2004; van Beest et al., 2018). In this seascape of fear, marine mammals can alter activity budgets according to perceived levels of predation risk (Wirsing et al., 2008), and are expected to perceive anthropogenic disturbance, such as pile-driving and vessel activity, as a form of predation (Frid and Dill, 2002). Consequently, porpoises in the vicinity of construction activities may reduce their buzzing activity as they adjust activity budgets to spend more time avoiding noise sources and less time engaged in foraging and/or social activities (Pirotta et al., 2014a; Wisniewska et al., 2018). Decreases in buzzing activity could also be explained by reduced prey availability or foraging performance as a result of the displacement or changed behavior of prey species in response to anthropogenic noise (Hassel et al., 2004; Mueller-Blenkle et al., 2010; Herbert-Read et al., 2017). Further field studies on the behavioral responses of different prey to pile-driving activities are required to understand the extent to which any spatio-temporal variation in the local prey availability and abundance may have indirect consequences on individual porpoise fitness (Hassel et al., 2004; Mueller-Blenkle et al., 2010).
Changes in Porpoise Occurrence and Activity in Relation to Vessels and Other Construction Activity
During the turbine installation phase, the broadscale BACI analysis showed that porpoise occurrence was also significantly lower at the impact block than during the baseline, even though no piling activities occurred (Figure 4). During this period (July–October 2018), various construction activities such as jacket foundation, turbine and cable installation occurred simultaneously at different locations within the windfarm, leading to high levels of vessel traffic (Figures 7A,B). Additionally, gradient analyses showed that the probability of detecting porpoises within the site decreased by up to 35.2% as vessel intensity increased, and as distance to the nearest vessel decreased (Figure 8A). Previous experimental studies demonstrated that captive harbor porpoises displayed strong behavioral responses when exposed to low levels of medium to high frequency vessel noise (Dyndo et al., 2015). Many vessels emit high frequency noises that overlap with frequency bands biologically relevant for porpoises, which may lead to acoustic masking and/or elicit adverse behavioral responses (Hermannsen et al., 2014). In our study, increased vessel activity led to a significant decrease in porpoise acoustic detections and activity (Figures 8A,B) at distances of up to 4 km (Figure 8A). However, studies using sound and movement recording tags that can detect finer-scale responses highlight that porpoise foraging may be disrupted at greater distances of up to 7 km (Wisniewska et al., 2018).
Using only data collected in the absence of pile-driving, we found that the probability of detecting porpoises decreased by up to 17% as the broadband sound pressure levels increased by 57 dB (Figure 9A). Increased levels of broadband noise emitted during other construction-related vessel activities may reduce the porpoise detection probability of CPODs (Clausen et al., 2019). However, to reduce the risk of vessel noise masking ultrasonic click detections and so the probability of false-negative detections, we discarded any hours with less than 60 min logged and during which vessels were within 1 km of CPODs (as Pirotta et al., 2014a), and additionally chose a coarse binary metric (i.e., presence/absence per hour) (Williamson et al., 2016). Thus, in this case, the decline in porpoise detections, in the absence of piling activities, is unlikely to be caused by a reduction in the effective detection area of the devices. In contrast, the decline in porpoise detections suggests that porpoises have exhibited a behavioral response to high levels of background noise associated with vessel and construction activities. In Wisniewska et al.’s (2018) study, tagged harbor porpoises responded to fast ferry passages by making deeper dives, increasing swimming effort, and ceasing echolocation and foraging for several minutes. Although these individuals lived in highly trafficked coastal waters, they did not seem to have habituated to vessel noise (Wisniewska et al., 2018). Similarly, throughout our 3-year monitoring, buzzing activity decreased by up to 24.5% as the vessel intensity increased in the study area (Figure 8B) and by up to 41.5% as the hourly RMS sound pressure levels increased from 104 to 155 dB re 1 μPa (Figure 9B). However, for the same levels of broadband noise, buzzing activity appeared to be higher during rather than in the absence of piling activities (Figure 9B). This increase in porpoise buzzing activity during piling may be indicative of behavioral changes in echolocation activity in response to noise (the Lombard effect). Harbor porpoises may increase the signal level of their clicks or the signal repetition rate (Branstetter et al., 2018) to compensate for the increased noise levels during social interactions (Sorensen et al., 2018) or foraging activity. Alternatively, adverse effects of piling noise on prey (e.g., Herbert-Read et al., 2017) may benefit predators by locally increasing prey availability and/or enhancing their foraging performance. Either way, these results highlight how chronic exposure to regular vessel activity and associated levels of anthropogenic noise could influence the foraging and/or social activity of those individuals which continue to use offshore construction sites during the pile-driving phase.
Vessel-tracking data provide a robust measure of the spatial distribution of windfarm construction vessels which are legally required to carry AIS. However, there are several reasons why these data may not fully capture variation in the soundscapes affecting species such as harbor porpoises and their prey. In coastal areas, many recreational vessels without AIS dominate the anthropogenic soundscape (Hermannsen et al., 2019). This is less likely to be an issue offshore but reports from guard vessels indicate that local fishing boats without active AIS commonly used areas over and around the construction sites throughout our study period. Furthermore, although information on construction vessel locations was available, detailed information on variation in the activity of those vessels, which could affect their acoustic signature, was not. This could be particularly important for construction vessels that jacked up to install jacket foundations and turbines, and vessels which periodically used dynamic positioning. Greater understanding of how acoustic signatures vary between vessels, and in relation to speed or activity, could in future help identify ways in which vessel management plans could reduce broader scale disturbance during windfarm construction and operation.
In the absence of more detailed information on the acoustic signatures or activities of vessels detected using AIS, our recordings of broadband noise at three sample locations provide a valuable measure of broad-scale variation in noise exposure to animals through construction phases, and opportunities for comparison with other study systems (Hermannsen et al., 2014). Even here, though, these measures may be biased by proximity to particular vessels. To characterize this variation, we used an unweighted RMS SPL based on a sampling rate of 48 kHz. These frequencies are appropriate for characterizing long-term variation in shipping and pile-driving noise (Merchant et al., 2012, 2014; Thompson et al., 2020), and analysis of a higher sample rate recording at the site (Supplementary Figure 10) indicated that almost all of the acoustic energy from both pile driving and shipping noise was contained below 24 kHz (the highest acoustic frequency that can be measured at a sampling rate of 48 kHz). However, these 48 kHz recordings do not capture those higher frequencies that may be particularly important to porpoises (Tougaard et al., 2015). More focused investigation of porpoise behavioral responses to vessel noise would require an increase in sampling rate and focus on biologically significant spectral bands by using audiogram weighted SPL (Dyndo et al., 2015; Tougaard et al., 2015).
Management Implications
The planned expansion of offshore windfarms to meet decarbonization targets must proceed within frameworks for safeguarding protected wildlife populations and minimizing cumulative environmental impacts (Le Lièvre, 2019; Thompson et al., 2020). Efforts to understand and mitigate impacts on marine mammals have focused on the effects of impulsive noise produced during pile-driving. Whilst pile-driving does produce the highest amplitude noise, active piling occurred for <10% of the time in the 9–10-month piling phases at Beatrice and Moray East. Whilst responses to these short but intense periods of impulsive noise sources are of greater magnitude, we showed that harbor porpoise occurrence and buzzing activity also decreased in response to more chronic exposure to vessel traffic throughout construction. Further disturbance may also be expected from routine operation and maintenance vessels, although this may be offset by benefits resulting from the creation of new reef habitat. Further understanding of the relative importance of these different disturbance sources is now required if we are to assess the broader scale cumulative impact of construction, operation and decommissioning over the life cycle of an offshore windfarm. Our data should now be integrated into existing tools (e.g., Nabe-Nielsen et al., 2018) to explore the scale of these different disturbance impacts at both individual and population levels. This could then provide a framework that could be used by policy makers to explore how cumulative impacts can be minimized by combining existing mitigation measures to reduce piling impacts with other regulatory measures to manage vessel traffic and other maritime activities occurring in or around construction sites.
Data Availability Statement
The article’s data and R code are available from the Dryad Digital Repository: https://doi.org/10.5061/dryad.v6wwpzgw4.
Ethics Statement
Ethical review and approval was not required for the animal study because this was a non-invasive, acoustic observational study of harbor porpoise responses to construction activities during the development of two commercial offshore windfarms. The authors had no control or influence over the duration or scheduling of construction activities including pile-driving. No animals were captured or tagged during this study and no research or animal ethical assessments were required. Porpoise responses were determined using remote passive acoustic devices on seabed moorings licensed for scientific use by Marine Scotland, and consented by the Crown Estate. Moorings were deployed and recovered using vessels with appropriate certification, accreditation and endorsements.
Author Contributions
AB, PT, and IG conceived and designed the study based on data collection that has been led by PT and IG. NM analyzed all the noise data. AB processed the data, carried out the data analysis, and prepared all the tables and figures, under the supervision of IG and PT. AB drafted the manuscript. All authors contributed critically to the drafts and gave final approval for publication.
Funding
This study was partly funded by Beatrice Offshore Wind Ltd. and Moray Offshore Wind Farm (East) Ltd. using equipment previously purchased by UK Department of Energy and Climate Change, Scottish Government, Oil and Gas UK, COWRIE and Moray Offshore Renewables Ltd. PT and IG were core funded by University of Aberdeen. AB was core funded by the collaboration between University of Aberdeen and Marine Scotland Science through the MarCRF Ph.D. studentship. NM was core funded by Centre for Environment, Fisheries and Aquaculture Science.
Conflict of Interest
The authors have no known conflict of interest. This study was partly funded by two commercial developers, Beatrice Offshore Wind Ltd. and Moray Offshore Wind Farm (East) Ltd. However, these funding bodies had no input into data collection, analysis and interpretation nor into the paper redaction. The aims, scope and sampling design of the study were agreed by the statutory advisors represented on the Moray Firth Renewables Advisory Group (MFRAG) and the regulator Marine Scotland Licensing and Operations Team.
Acknowledgments
We thank Bill Ruck at Moray First Marine and University of Aberdeen’s Lighthouse Field Station colleagues for support and assistance with data collection. We also thank the Beatrice Offshore Wind Ltd. and Moray Offshore Wind Farm (East) Ltd. teams for facilitating fieldwork and data collection during windfarm construction and providing details of the construction programs. We finally thank the members of MFRAG for their advice and two reviewers for their constructive comments.
Supplementary Material
The Supplementary Material for this article can be found online at: https://www.frontiersin.org/articles/10.3389/fmars.2021.664724/full#supplementary-material
Footnotes
References
Bailey, H., Brookes, K. L., and Thompson, P. M. (2014). Assessing environmental impacts of offshore wind farms: lessons learned and recommendations for the future. Aquat. Biosyst. 10:8. doi: 10.1186/2046-9063-10-8
Barton, K. (2020). MuMIn: Multi-Model Inference. Available online at: https://CRAN.R-project.org/package=MuMIn (accessed August 24, 2020).
Bates, D., Machler, M., Bolker, B. M., and Walker, S. C. (2015). Fitting linear mixed-effects models using lme4. J. Stat. Softw. 67, 1–48.
Beale, C. M., and Monaghan, P. (2004). Behavioural responses to human disturbance: a matter of choice? Anim. Behav. 68, 1065–1069. doi: 10.1016/j.anbehav.2004.07.002
Beatrice Offshore Windfarm Limited (2012). Beatrice Offshore Wind Farm Environmental Statement - Non Technical Summary. Available online at: https://marine.gov.scot/sites/default/files/00392805.pdf (accessed August 28, 2020).
BEIS (2019). Department of Business, Energy and Industrial Strategy. Available online at: https://www.gov.uk/government/publications/offshore-wind-sector-deal (accessed May 28, 2020).
Bejder, L., Samuels, A., Whitehead, H., Finn, H., and Allen, S. (2009). Impact assessment research: use and misuse of habituation, sensitisation and tolerance in describing wildlife responses to anthropogenic stimuli. Mar. Ecol. Prog. Ser. 395, 177–185. doi: 10.3354/meps07979
Benaglia, T., Chauveau, D., Hunter, D. R., and Young, D. S. (2009). mixtools: an R package for analyzing finite mixture models. J. Stat. Softw. 32, 1–29. doi: 10.1007/978-0-387-35768-3_1
Booth, C. G. (2020). Food for thought: harbor porpoise foraging behavior and diet inform vulnerability to disturbance. Mar. Mamm. Sci. 36, 195–208. doi: 10.1111/mms.12632
Booth, C. G., Harwood, J., Plunkett, R., Mendes, S., and Walker, R. (2017). Using the Interim PCoD Framework to Assess the Potential Impacts of Offshore wind Developments in Eastern English Waters on Harbour Porpoises in the North Sea. Natural England Joint Report no 024. York: Natural England.
Brandt, M. J., Diederichs, A., Betke, K., and Nehls, G. (2011). Responses of harbour porpoises to pile driving at the Horns Rev II offshore wind farm in the Danish North Sea. Mar. Ecol. Prog. Ser. 421, 205–216. doi: 10.3354/meps08888
Brandt, M. J., Dragon, A. C., Diederichs, A., Bellmann, M. A., Wahl, V., Piper, W., et al. (2018). Disturbance of harbour porpoises during construction of the first seven offshore wind farms in Germany. Mar. Ecol. Prog. Ser. 596, 213–232. doi: 10.3354/meps12560
Brandt, M. J., Dragon, A. C., Diederichs, A., Schubert, A., Kosarev, V., Nehls, G., et al. (2016). Effects of Offshore Pile Driving on Harbour Porpoise Abundance in the German Bight”. Report Prepared for Offshore Forum Windenergie. Hamburg: BioConsultSH.
Brandt, M. J., Höschle, C., Diederichs, A., Betke, K., Matuschek, R., Witte, S., et al. (2013). Far-reaching effects of a seal scarer on harbour porpoises, Phocoena phocoena. Aquat. Conserv. Mar. Freshw. Ecosyst. 23, 222–232. doi: 10.1002/aqc.2311
Branstetter, B. K., Bowman, V. F., Houser, D. S., Tormey, M., Banks, P., Finneran, J. J., et al. (2018). Effects of vibratory pile driver noise on echolocation and vigilance in bottlenose dolphins (Tursiops truncatus). J. Acoust. Soc. Am. 143, 429–439. doi: 10.1121/1.5021555
Brookes, K. L., Bailey, H., and Thompson, P. M. (2013). Predictions from harbor porpoise habitat association models are confirmed by long-term passive acoustic monitoring. J. Acoust. Soc. Am. 134, 2523–2533. doi: 10.1121/1.4816577
Clausen, K. T., Tougaard, J., Carstensen, J., Delefosse, M., and Teilmann, J. (2019). Noise affects porpoise click detections - the magnitude of the effect depends on logger type and detection filter settings. Bioacoustics 28, 443–458. doi: 10.1080/09524622.2018.1477071
Dähne, M., Gilles, A., Lucke, K., Peschko, V., Adler, S., Krügel, K., et al. (2013). Effects of pile-driving on harbour porpoises (Phocoena phocoena) at the first offshore wind farm in Germany. Environ. Res. Lett. 8:025002. doi: 10.1088/1748-9326/8/2/025002
Dähne, M., Tougaard, J., Carstensen, J., Rose, A., and Nabe-Nielsen, J. (2017). Bubble curtains attenuate noise from offshore wind farm construction and reduce temporary habitat loss for harbour porpoises. Mar. Ecol. Prog. Ser. 580, 221–237. doi: 10.3354/meps12257
Dolman, S., and Simmonds, M. (2010). Towards best environmental practice for cetacean conservation in developing Scotland’s marine renewable energy. Mar. Policy 34, 1021–1027. doi: 10.1016/j.marpol.2010.02.009
Dyndo, M., Wisniewska, D. M., Rojano-Donate, L., and Madsen, P. T. (2015). Harbour porpoises react to low levels of high frequency vessel noise. Sci. Rep. 5:11083.
Ellis, J. I., and Schneider, D. C. (1997). Evaluation of a gradient sampling design for environmental imapct assessment. Environ. Monit. Assess. 48, 157–172.
Frid, A., and Dill, L. (2002). Human-caused disturbance stimuli as a form of predation risk. Conserv. Ecol. 6:11.
Gill, J. A., Norris, K., and Sutherland, W. J. (2001). Why behavioural responses may not reflect the population consequences of human disturbance. Biol. Conserv. 97, 265–268. doi: 10.1016/s0006-3207(00)00002-1
Graham, I. M., Merchant, N. D., Farcas, A., Barton, T. R., Cheney, B., Bono, S., et al. (2019). Harbour porpoise responses to pile-driving diminish over time. R. Soc. Open Sci. 6:190335. doi: 10.1098/rsos.190335
Haelters, J., Duliere, V., Vigin, L., and Degraer, S. (2015). Towards a numerical model to simulate the observed displacement of harbour porpoises Phocoena phocoena due to pile driving in Belgian waters. Hydrobiologia 756, 105–116. doi: 10.1007/s10750-014-2138-4
Hammond, P. S., Macleod, K., Berggren, P., Borchers, D. L., Burt, L., Canadas, A., et al. (2013). Cetacean abundance and distribution in European Atlantic shelf waters to inform conservation and management. Biol. Conserv. 164, 107–122. doi: 10.1016/j.biocon.2013.04.010
Hartig, F. (2020). DHARMa: Residual Diagnostics for Hierarchical (Multi-Level/Mixed) Regression Models. Available online at: https://CRAN.R-project.org/package=DHARMa (accessed May 29, 2020).
Hassel, A., Knutsen, T., Dalen, J., Skaar, K., Lokkeborg, S., Misund, O. A., et al. (2004). Influence of seismic shooting on the lesser sandeel (Ammodytes marinus). ICES J. Mar. Sci. 61, 1165–1173. doi: 10.1016/j.icesjms.2004.07.008
Herbert-Read, J. E., Kremer, L., Bruintjes, R., Radford, A. N., and Ioannou, C. C. (2017). Anthropogenic noise pollution from pile-driving disrupts the structure and dynamics of fish shoals. Proc. R. Soc. B Biol. Sci. 284, 20171627. doi: 10.1098/rspb.2017.1627
Hermannsen, L., Beedholm, K., Tougaard, J., and Madsen, P. T. (2014). High frequency components of ship noise in shallow water with a discussion of implications for harbor porpoises (Phocoena phocoena). J. Acoust. Soc. Am. 136, 1640–1653. doi: 10.1121/1.4893908
Hermannsen, L., Mikkelsen, L., Tougaard, J., Beedholm, K., Johnson, M., and Madsen, P. T. (2019). Recreational vessels without Automatic Identification System (AIS) dominate anthropogenic noise contributions to a shallow water soundscape. Sci. Rep. 9:15477.
JNCC, and Natural England, (2019). Norfolk Vanguard Offshore Wind Farm Harbour Porpoise (Phocoena phocoena) Special Area of Conservation: Southern North Sea. Conservation Objectives and Advice on Operations. Peterborough: JNCC.
Kastelein, R. A., Helder-Hoek, L., Jennings, N., van Kester, R., and Huisman, R. (2019). Reduction in body mass and blubber thickness of harbor porpoises (Phocoena phocoena) due to near-fasting for 24 Hours in four seasons. Aquat. Mamm. 45, 37–47. doi: 10.1578/am.45.1.2019.37
King, S. L., Schick, R. S., Donovan, C., Booth, C. G., Burgman, M., Thomas, L., et al. (2015). An interim framework for assessing the population consequences of disturbance. Methods Ecol. Evol. 6, 1150–1158. doi: 10.1111/2041-210x.12411
Kinsler, L. E., Frey, A. R., Coppens, A. B., and Sanders, J. V. (1999). Fundamentals of Acoustics. Hoboken, NJ: John Wiley & Sons.
Le Lièvre, C. (2019). Sustainably reconciling offshore renewable energy with Natura 2000 sites: an interim adaptive management framework. Energy Policy 129, 491–501. doi: 10.1016/j.enpol.2019.02.007
Madsen, P. T., Wahlberg, M., Tougaard, J., Lucke, K., and Tyack, P. (2006). Wind turbine underwater noise and marine mammals: implications of current knowledge and data needs. Mar. Ecol. Prog. Ser. 309, 279–295. doi: 10.3354/meps309279
Merchant, N. D., Fristrup, K. M., Johnson, M. P., Tyack, P. L., Witt, M. J., Blondel, P., et al. (2015). Measuring acoustic habitats. Methods Ecol. Evol. 6, 257–265.
Merchant, N. D., Pirotta, E., Barton, T. R., and Thompson, P. M. (2014). Monitoring ship noise to assess the impact of coastal developments on marine mammals. Mar. Pollut. Bull. 78, 85–95. doi: 10.1016/j.marpolbul.2013.10.058
Merchant, N. D., Witt, M. J., Blondel, P., Godley, B. J., and Smith, G. H. (2012). Assessing sound exposure from shipping in coastal waters using a single hydrophone and Automatic Identification System (AIS) data. Mar. Pollut. Bull. 64, 1320–1329. doi: 10.1016/j.marpolbul.2012.05.004
Metcalfe, K., Breheret, N., Chauvet, E., Collins, T., Curran, B. K., Parnell, R. J., et al. (2018). Using satellite AIS to improve our understanding of shipping and fill gaps in ocean observation data to support marine spatial planning. J. Appl. Ecol. 55, 1834–1845. doi: 10.1111/1365-2664.13139
Moray Offshore Renewables Limited (2012). Environmental Statement Technical Appendix 4.4 A Marine Mammals Baseline. Available online at: https://www.morayeast.com/download_file/216/219 (accessed August 28, 2020).
Mueller-Blenkle, C., McGregor, P. K., Gill, A. B., Andersson, M. H., Metcalfe, J., Bendall, V., et al. (2010). Effects of Pile-Driving Noise on the Behaviour of Marine Fish. COWRIE Ref: Fish 06-08, Technical Report. Cranfield: Cranfield University.
Nabe-Nielsen, J., van Beest, F. M., Grimm, V., Sibly, R. M., Teilmann, J., and Thompson, P. M. (2018). Predicting the impacts of anthropogenic disturbances on marine populations. Conserv. Lett. 11:e12563. doi: 10.1111/conl.12563
Oakley, J. A., Williams, A. T., and Thomas, T. (2017). Reactions of harbour porpoise (Phocoena phocoena) to vessel traffic in the coastal waters of South West Wales, UK. Ocean Coast. Manag. 138, 158–169. doi: 10.1016/j.ocecoaman.2017.01.003
Pebesma, E. (2018). Simple features for R: standardized support for spatial vector data. R J. 10, 439–446. doi: 10.32614/rj-2018-009
Pirotta, E., Brookes, K. L., Graham, I. M., and Thompson, P. M. (2014a). Variation in harbour porpoise activity in response to seismic survey noise. Biol. Lett. 10:20131090. doi: 10.1098/rsbl.2013.1090
Pirotta, E., Thompson, P. M., Miller, P. I., Brookes, K. L., Cheney, B., Barton, T. R., et al. (2014b). Scale-dependent foraging ecology of a marine top predator modelled using passive acoustic data. Funct. Ecol. 28, 206–217. doi: 10.1111/1365-2435.12146
R Core Team, (2019). R: A Language and Environment for Statistical Computing. Vienna: R Foundation for Statistical Computing.
Richardson, W., Greene, C., Malme, C., and Thompson, D. (1995). Marine Mammals and Noise. San Diego, CA: Academic Press.
Santos, M., and Pierce, G. (2003). The diet of harbour porpoise (Phocoena phocoena) in the northeast Atlantic. Oceanogr. Mar. Biol. 41, 355–390.
Sarnocińska, J., Teilmann, J., Balle, J. D., van Beest, F. M., Delefosse, M., and Tougaard, J. (2020). Harbor porpoise (Phocoena phocoena) reaction to a 3D seismic airgun survey in the North Sea. Front. Mar. Sci. 6:824. doi: 10.3389/fmars.2019.00824
Scheidat, M., Tougaard, J., Brasseur, S., Carstensen, J., Petel, T. V., Teilmann, J., et al. (2011). Harbour porpoises (Phocoena phocoena) and wind farms: a case study in the Dutch North Sea. Environ. Res. Lett. 6:025102. doi: 10.1088/1748-9326/6/2/025102
Smith, E. P. (2002). “BACI Design,” in Encyclopedia of Environmetrics, eds A. H. El-Shaarawi and W. W. Piegorsch (Chichester: John Wiley & Sons, Ltd).
Sorensen, P. M., Wisniewska, D. M., Jensen, F. H., Johnson, M., Teilmann, J., and Madsen, P. T. (2018). Click communication in wild harbour porpoises (Phocoena phocoena). Sci. Rep. 8:9702.
Sveegaard, S., Teilmann, J., Tougaard, J., Dietz, R., Mouritsen, K. N., Desportes, G., et al. (2011). High-density areas for harbor porpoises (Phocoena phocoena) identified by satellite tracking. Mar. Mamm. Sci. 27, 230–246. doi: 10.1111/j.1748-7692.2010.00379.x
Thompson, P. M., Brookes, K. L., Graham, I. M., Barton, T. R., Needham, K., Bradbury, G., et al. (2013). Short-term disturbance by a commercial two-dimensional seismic survey does not lead to long-term displacement of harbour porpoises. Proc. Biol. Sci. 280:20132001. doi: 10.1098/rspb.2013.2001
Thompson, P. M., Graham, I. M., Cheney, B., Barton, T. R., Farcas, A., and Merchant, N. D. (2020). Balancing risks of injury and disturbance to marine mammals when pile driving at offshore windfarms. Ecol. Solut. Evid. 1:e12034.
Thomsen, F., McCully, S. R., Weiss, L. R., Wood, D. T., Warr, K. J., Barry, J., et al. (2011). Cetacean stock assessments in relation to exploration and production industry activity and other human pressures: review and data needs. Aquat. Mamm. 37, 1–93. doi: 10.1578/am.37.1.2011.1
Tougaard, J., Carstensen, J., Teilmann, J., Skov, H., and Rasmussen, P. (2009). Pile driving zone of responsiveness extends beyond 20 km for harbor porpoises (Phocoena phocoena (L.)). J. Acoust. Soc. Am. 126, 11–14. doi: 10.1121/1.3132523
Tougaard, J., Wright, A. J., and Madsen, P. T. (2015). Cetacean noise criteria revisited in the light of proposed exposure limits for harbour porpoises. Mar. Pollut. Bull. 90, 196–208. doi: 10.1016/j.marpolbul.2014.10.051
Tregenza, N. (2014). CPOD.exe: A Guide for Users. Available online at: https://www.chelonia.co.uk/downloads/CPOD.pdf (accessed May 4, 2021).
Underwood, A. J. (1992). Beyond BACI: the detection of environmental impacts on populations in the real, but variable, world. J. Exp. Mar. Biol. Ecol. 161, 145–178. doi: 10.1016/0022-0981(92)90094-q
van Beest, F. M., Teilmann, J., Hermannsen, L., Galatius, A., Mikkelsen, L., Sveegaard, S., et al. (2018). Fine-scale movement responses of free-ranging harbour porpoises to capture, tagging and short-term noise pulses from a single airgun. R. Soc. Open Sci. 5:170110. doi: 10.1098/rsos.170110
Waggitt, J. J., Evans, P. G. H., Andrade, J., Banks, A. N., Boisseau, O., Bolton, M., et al. (2020). Distribution maps of cetacean and seabird populations in the North−East Atlantic. J. Appl. Ecol. 57, 253–269.
Williamson, L. D., Brookes, K. L., Scott, B. E., Graham, I. M., Bradbury, G., Hammond, P. S., et al. (2016). Echolocation detections and digital video surveys provide reliable estimates of the relative density of harbour porpoises. Methods Ecol. Evol. 7, 762–769. doi: 10.1111/2041-210x.12538
Williamson, L. D., Brookes, K. L., Scott, B. E., Graham, I. M., and Thompson, P. M. (2017). Diurnal variation in harbour porpoise detection — potential implications for management. Mar. Ecol. Prog. Ser. 570, 223–232. doi: 10.3354/meps12118
Wilson, B., Benjamins, S., and Elliott, J. (2013). Using drifting passive echolocation loggers to study harbour porpoises in tidal-stream habitats. Endanger. Species Res. 22, 125–143. doi: 10.3354/esr00538
Wirsing, A. J., Heithaus, M. R., Frid, A., and Dill, L. M. (2008). Seascapes of fear: evaluating sublethal predator effects experienced and generated by marine mammals. Mar. Mamm. Sci. 24, 1–15. doi: 10.1111/j.1748-7692.2007.00167.x
Wisniewska, D. M., Johnson, M., Teilmann, J., Rojano-Donate, L., Shearer, J., Sveegaard, S., et al. (2016). Ultra-high foraging rates of harbor porpoises make them vulnerable to anthropogenic disturbance. Curr. Biol. 26, 1441–1446. doi: 10.1016/j.cub.2016.03.069
Wisniewska, D. M., Johnson, M., Teilmann, J., Siebert, U., Galatius, A., Dietz, R., et al. (2018). High rates of vessel noise disrupt foraging in wild harbour porpoises (Phocoena phocoena). Proc. Biol. Sci. 285:20172314. doi: 10.1098/rspb.2017.2314
Keywords: anthropogenic disturbance, cumulative impacts, offshore windfarm, passive acoustic monitoring, underwater noise, behavioral response, foraging, marine mammal conservation
Citation: Benhemma-Le Gall A, Graham IM, Merchant ND and Thompson PM (2021) Broad-Scale Responses of Harbor Porpoises to Pile-Driving and Vessel Activities During Offshore Windfarm Construction. Front. Mar. Sci. 8:664724. doi: 10.3389/fmars.2021.664724
Received: 05 February 2021; Accepted: 25 May 2021;
Published: 02 July 2021.
Edited by:
Kerri D. Seger, Applied Ocean Sciences, United StatesReviewed by:
Roel Müller, Defense, Safety and Security, Netherlands Organization for Applied Scientific Research (TNO), NetherlandsTristan Lippert, Aqustix, Germany
Copyright © 2021 Benhemma-Le Gall, Graham, Merchant and Thompson. This is an open-access article distributed under the terms of the Creative Commons Attribution License (CC BY). The use, distribution or reproduction in other forums is permitted, provided the original author(s) and the copyright owner(s) are credited and that the original publication in this journal is cited, in accordance with accepted academic practice. No use, distribution or reproduction is permitted which does not comply with these terms.
*Correspondence: Aude Benhemma-Le Gall, YS5iZW5oZW1tYS1sZWdhbGwuMTdAYWJkbi5hYy51aw==