- 1Department of Biosciences, Oslo, Norway
- 2Fram Centre, Norwegian Polar Institute, Tromsø, Norway
- 3Norwegian Institute for Air Research (NILU), Tromsø, Norway
- 4UMR 7372 CNRS, Centre d’Etudes Biologiques de Chizé (CEBC), La Rochelle Université, Chizé, France
- 5UMR 7266 – CNRS, Littoral Environnement et Sociétés (LIENSs), Université de La Rochelle, La Rochelle, France
- 6UMR 5805 EPOC-LPTC, University of Bordeaux, Talence, France
- 7CNRS, UMR 5805 Environnements et Paléoenvironnements Océaniques et Continentaux (EPOC), Talence, France
- 8Institut Universitaire de France (IUF), Paris, France
- 9Department of Paraclinical Sciences, Norwegian University of Life Sciences, Ås, Norway
- 10Fram Centre, Norwegian Institute for Nature Research (NINA), Tromsø, Norway
Per and polyfluoroalkyl substances (PFASs) are found in Antarctic wildlife, with high levels in the avian top predator south polar skua (Catharacta maccormicki). As increasing PFAS concentrations were found in the south polar skua during the breeding season in Antarctica, we hypothesised that available prey during the breeding period contributes significantly to the PFAS contamination in skuas. To test this, we compared PFAS in south polar skuas and their main prey from two breeding sites on opposite sides of the Antarctic continent: Antarctic petrel (Thalassoica antarctica) stomach content, eggs, chicks, and adults from Svarthamaren in Dronning Maud Land and Adélie penguin chicks (Pygoscelis adeliae) from Dumont d’Urville in Adélie Land. Of the 22 PFAS analysed, seven were present in the majority of samples, except petrel stomach content [only perfluoroundecanoate (PFUnA) present] and Adélie penguins (only four compounds present), with increasing concentrations from the prey to the skuas. The biomagnification factors (BMFs) were higher at Dumont d’Urville than Svarthamaren. When adjusted to reflect one trophic level difference, the BMFs at Svarthamaren remained the same, whereas the ones at Dumont d’Urville doubled. At both the colonies, the skua PFAS pattern was dominated by perfluorooctanesulfonic acid (PFOS), followed by PFUnA, but differed with the presence of branched PFOS and perfluorotetradecanoate (PFTeA) and lack of perfluorononanoate (PFNA) and perfluorodecanoate (PFDA) at Dumont d’Urville. At Svarthamaren, the pattern in the prey was comparable to the skuas, but with a higher relative contribution of PFTeA in prey. At Dumont d’Urville, the pattern in the prey differed from the skuas, with the domination of PFUnA and the general lack of PFOS in prey. Even though the PFAS levels are low in Antarctic year-round resident prey, the three lines of evidence (pattern, BMF difference, and BMF adjusted to one trophic level) suggest that the Antarctic petrel are the significant source of PFAS in the Svarthamaren skuas, whereas the skuas in Dumont d’Urville have other important sources to PFAS than Adélie penguin, either in the continent or external on the inter-breeding foraging grounds far from Antarctica.
Introduction
Per- and polyfluoroalkyl substances (PFASs) have a wide industrial application and use, from surfactants in aqueous film-forming foams (Moody and Field, 2000), ski wax (Kotthoff et al., 2015), to water-repellent coatings and non-stick surfaces in carpets, clothes, papers and non-stick cookware (Paul et al., 2009). PFASs are anthropogenic-made hydrocarbons where many or all of the substituents are fluorine atoms, forming the moiety CnF2n+1– (Buck et al., 2011). In the environment, PFASs are ubiquitous organic pollutants that reach remote locations including Polar Regions. Several of the PFAS compounds accumulate in biota with an increasing level up to the food webs, indicating that they are biomagnified (Tomy et al., 2004; Haukås et al., 2007; Franklin, 2016).
In Antarctic wildlife, PFASs have been quantified in migratory species that are suggested to be exposed outside the Antarctic Circumpolar Current, bringing the PFAS with them to the continent as biovectors (Bengtson Nash et al., 2010; Munoz et al., 2017; Roscales et al., 2019). One of these migrating species is the south polar skua (Catharacta maccormicki), which overwinters in tropical or temperate waters outside the Southern Ocean and returns to Antarctica to breed in the austral summer (Weimerskirch et al., 2015). PFASs have been quantified in south polar skuas during breeding across Antarctica (Tao et al., 2006; Munoz et al., 2017; Midthaug et al., 2021), with some of the highest PFAS concentrations recorded in wildlife in sub-tropical and sub-Antarctic waters (Roscales et al., 2019). In the inland colony of Svarthamaren at Dronning Maud Land, the south polar skua PFAS levels increased during the breeding season (Midthaug et al., 2021), which suggests that local prey is a significant source of PFAS.
Diet is the major source of PFAS in avian wildlife and biomagnification factors (BMFs) reflect the relative change in contaminant concentration from prey to predator (Mackay et al., 2013; Borgå and Ruus, 2019). BMF is assumed to reflect a steady state condition where the predator reflects the contaminant status in its dominant prey. Thus, if the contamination load in the predator reflects its local prey, BMFs should be comparable across areas and vary due to physicochemical properties and recalcitrance.
Our aim was to investigate if local prey during the breeding season in Antarctica could account for the PFAS occurrence (both the concentrations and pattern) in south polar skuas. We quantified PFAS in main prey items and south polar skuas at opposite sides of the Antarctic continent where the skuas breed during the austral summer, preying on eggs and chicks of other seabirds. At the inland colony of Svarthamaren, Dronning Maud Land, the south polar skua feeds almost exclusively on Antarctic petrel (Thalassoica antarctica) eggs and chicks (Brooke et al., 1999; Busdieker et al., 2020), whereas they feed mainly on Adélie penguins but also on other prey such as fish in the coastal colony such as in Dumont d’Urville (Young, 1963; Furness, 2010; Olsen, 2010; Carravieri et al., 2017; Pacoureau et al., 2019). We analysed Antarctic petrel (Thalassoica antarctica) eggs, blood of chicks, adults, and their stomach samples from Svarthamaren, Dronning Maud Land and blood of Adélie penguin chicks (Pygoscelis adeliae) from Dumont d’Urville, Adélie Land. We also analysed and compared stable isotopes of carbon (δ13C) and nitrogen (δ15N) as dietary markers to assess, respectively, the feeding habitat and relative trophic positions (TPs) of the prey and skuas. We assumed the analysed prey to be the main prey and that the estimated BMFs reflected one trophic level difference between predator and prey. We expected the skua PFAS to reflect their local prey and, thus, the BMFs to be comparable between two colonies, one inland, and one coastal as well as comparable to avian PFAS BMFs from other areas. This study is based on a combination of new data and published PFAS data from skuas at Svarthamaren (Midthaug et al., 2021) and Dumont d’Urville (Munoz et al., 2017) as well as stable isotope data in Adélie penguin chicks (Carravieri et al., 2020). All the data from Svarthamaren are from the 2013/2014 and 2015/2016 breeding seasons and Dumont d’Urville in the 2011/2012 breeding season.
Materials and Methods
Area and the Main Prey
Svarthamaren is located at 71°53′S, 5°10′E in Dronning Maud Land, 200 km inland from the sea, while Dumont d’Urville is located at 66°39′47′′S, 140°00′10′′E in Adélie Land, close to the ocean (Figure 1). Although the Antarctic petrels are also migratory, they spend most of the inter-breeding period within the Marginal Ice Zone and generally winter south of the Antarctic Circumpolar Current (Descamps et al., 2016; Delord et al., 2020). The Antarctic petrel’s diet is comprised mostly of crustaceans but also includes fish and cephalopods (Lorentsen et al., 1998; Descamps et al., 2016).
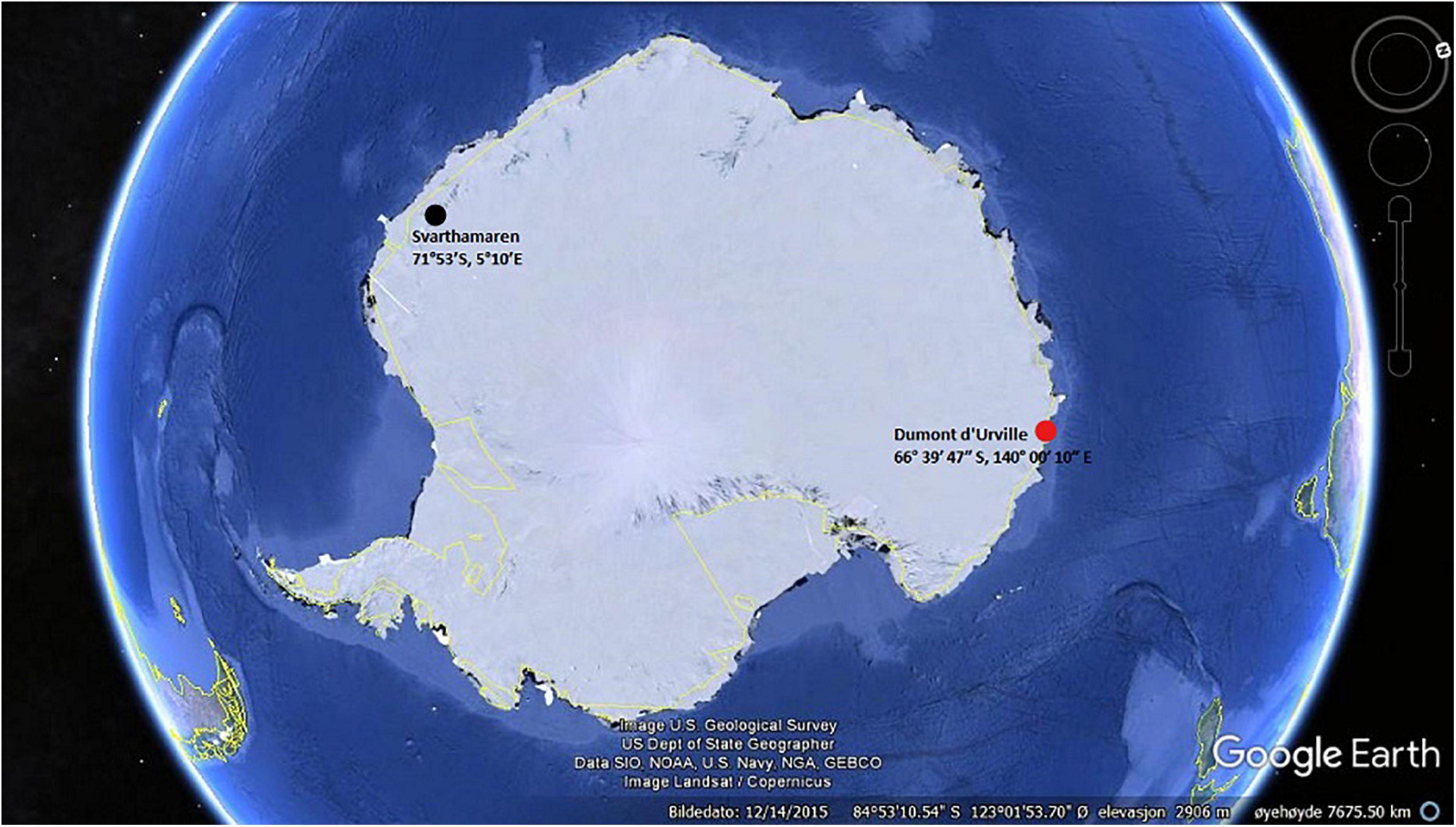
Figure 1. Antarctica with the study sites of Svarthamaren in Dronning Maud Land and Dumont d’Urville in Adélie Land marked. Source Google maps.
In Adélie Land, the Adélie penguins’ diet is crustaceans and fish similar to that of the Antarctic petrels (Ridoux and Offredo, 1989; Wienecke et al., 2000; Cherel, 2008). Adélie penguins winter within the pack ice south in Antarctic waters (Thiébot et al., 2019).
Sample Collection
At Svarthamaren, whole blood samples were collected from 30 breeding adult south polar skuas, 19 Antarctic petrel chicks in addition to 20 Antarctic petrel eggs during the 2013/2014 breeding season (Supplementary Table 1). During the 2015/2016 breeding season, blood samples were collected from 24 breeding adult Antarctic petrels and 20 breeding adult south polar skuas. Two of these skuas were recaptured individuals from 2013 to 2014. In addition, the stomach contents of six adult petrels in 2015/2016 were collected by lavage according to Wilson (1984) and Descamps et al. (2016). Antarctic petrel chicks and most of the adults were captured on the nest by hand. Few adult petrels did not let us approach too close and were captured with a 1-m noose pole. Adult skuas were captured either with a net attached to a 2-m pole, with a baited trap triggered at a distance or with air propelled net gun. In both the seasons, birds were randomly selected from the Svarthamaren colony and approximately 0.5 ml of blood was drawn from the brachial vein of petrels and 5 ml from skuas. The samples were kept in a cooler and taken to the camp. The blood samples of petrel chicks from 2013 to 2014 and all the samples from 2015 to 2016 were centrifuged 6–8 h after collection to separate plasma from blood cells. Afterward, plasma was removed with a micropipette. Skua blood samples from 2013 to 2014 were frozen without centrifugation. The Antarctic petrel eggs were sampled from abandoned nests at the petrel colony or depredated eggs found close to the skua nests. Approximately, 2 ml of egg yolk and egg whites were collected from each egg, together with some egg membrane. The egg yolk was allocated to PFAS analyses, whereas stable isotopes were analysed in the egg whites.
The samples from Svarthamaren were stored in freezers at −20°C. Later, the petrel chick blood samples were dried as preparation for stable isotope analyses and were, thereafter, stored in Eppendorf tubes at room temperature at the Norwegian Polar Institute facilities in Tromsø, Norway.
At Dumont d’Urville, blood samples were collected from the wing of 10 Adélie penguin chicks that were in the moulting phase (Carravieri et al., 2020) as well as from five adult breeding south polar skuas (Munoz et al., 2017) during the 2011/2012 breeding season. These samples were centrifuged and separated into red blood cells and plasma, frozen at −20°C, and transported for further analysis. An overview of the samples analysed is found in Supporting Information (Supplementary Table 1).
Per- and Polyfluoroalkyl Substance Analysis
The south polar skuas from Svarthamaren collected in 2013/2014 (whole blood) and from Dumont d’Urville in 2011/2012 (plasma) were analysed for PFAS using liquid chromatography/mass spectrometry (LC/MS) as described in Munoz et al. (2017) and Midthaug et al. (2021), respectively. These PFAS analyses followed the quality assurance (QA) procedures specified in Grønnestad et al. (2017) for the Norwegian University of Life Sciences (NMBU) and Munoz et al. (2017) for the National Centre for Scientific Research (CNRS). The targetted 22 PFAS are given as footnotes to Table 1 and in a separate Supplementary Table 2 in Supporting Information and detailed results from these studies are reported in detail in Munoz et al. (2017) and Midthaug et al. (2021).
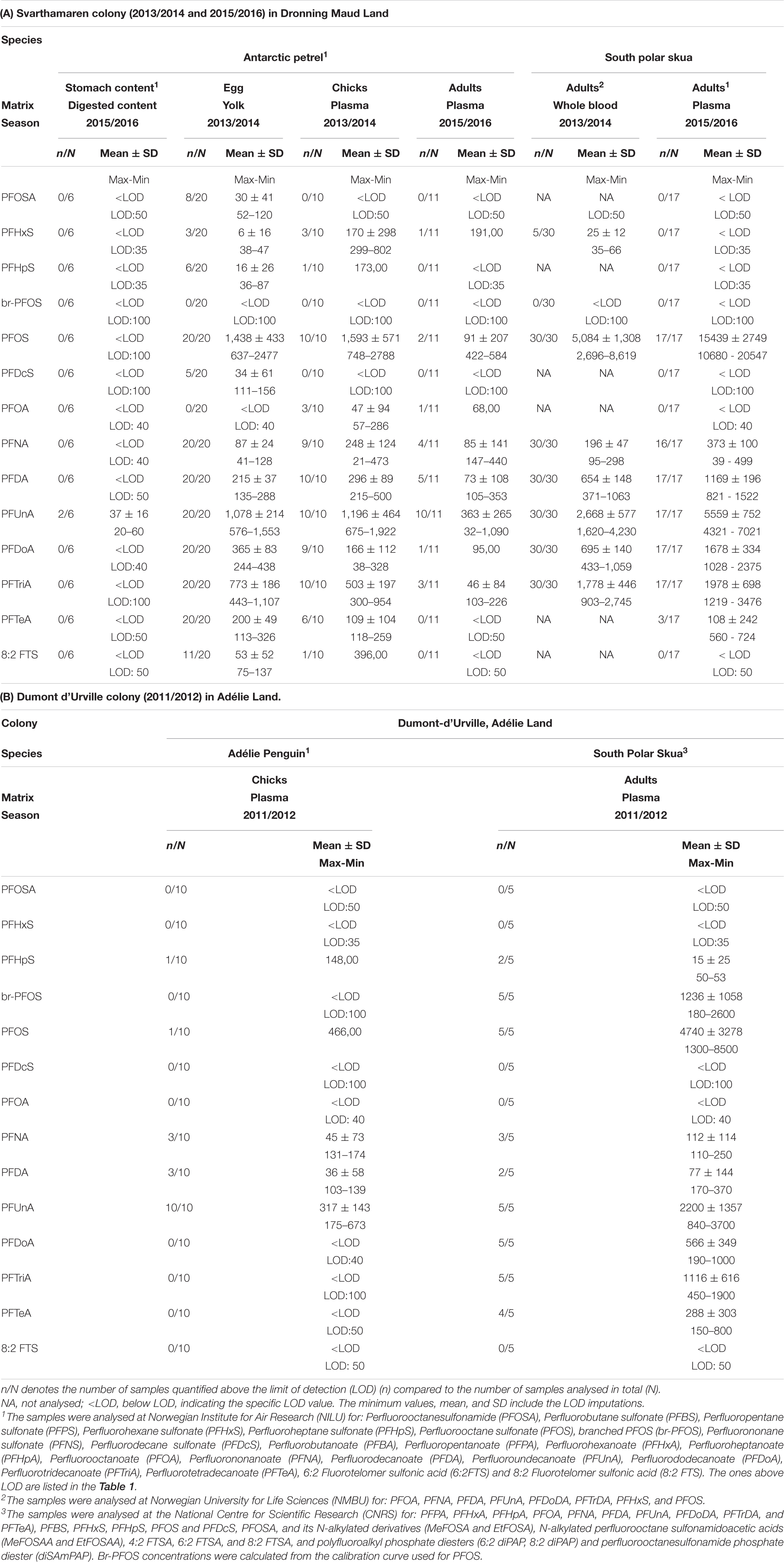
Table 1. Per- and polyfluoroalkyl substance (PFAS) concentrations (pg/g wet weight) in south polar skuas and food web items.
The new samples included in this study (all the samples from petrels and Adélie penguins and Svarthamaren south polar skua blood collected in 2015/2016) were analysed for PFAS at the Norwegian Institute of Air Research (NILU) in Tromsø, Norway, using methods previously described in Jouanneau et al. (2020) for plasma and Warner et al. (2019) for eggs. Details can be found in Supporting Information (PFAS analysis). At the NILU, approximately, 1 g of egg yolk, 1 g of stomach content, or 200 μl of plasma was extracted with methanol according to Hanssen et al. (2013). The extracted PFASs were separated applying ultra-high pressure LC with triple-quadrupole mass spectrometry (UHPLC-MS/MS) according to Hanssen et al. (2013), in the TSQ Vantage LC/MS equipment (Thermo Fisher Scientific, San Jose, CA, United States). The chromatograms were quantified with LCQuan software (version 2.6, Thermo Fisher Scientific Incorporation, Waltham, MA, United States), using the isotopic dilution approach with 13C mass labelled compounds applying an eight-point calibration curve with a concentration range from 0.02 to 50 pg μl–1. For the isotopic dilution method, carbon- and/or deuterium-labelled compounds were used (Supplementary Table 3).
Quality Control and Quality Assurance
The samples were all processed for PFAS analysis at laboratories following comparable QA and QC protocols, including analysis with appropriate blanks and standards of standard reference materials (SRM). The methods are described in detail by Grønnestad et al. (2017) for the NMBU, Munoz et al. (2017) for the CNRS, and Hanssen et al. (2013) for the NILU. In addition, the Norwegian laboratories at the NILU and the NMBU are a part of the Arctic Monitoring and Assessment Program Ring Test for Persistent Organic Pollutants in Human Serum, which is an inter-laboratory comparison program (Hanssen et al., 2013).
Quality control includes a reference sample and a blank sample for every 10 samples analysed. For the petrel and stomach content samples, a blank and a reference were included for each analysis batch to verify the quality of the prepared samples, test the reproducibility and precision of the method. The blank was methanol in the case of plasma and acetonitrile in the case of egg yolk and stomach content. The reference sample was SRM human serum Institute national de santè publique du Quebec (INSPQ) within the Arctic Monitoring and Assessment Program Ring Test for the Plasma Samples and fish muscle for the egg and stomach content samples. For the Svarthamaren 2015/2016 skua analysis, three blanks and one reference sample were included. Both the blank and the internal reference were spiked with internal and recovery standards and were analysed with the same procedure as the samples. In the total 10 blank samples included in this study, perfluorohexanoate (PFHxA) and perfluorooctanoate (PFOA) were the only PFAS that were regularly detected, leading to an elevated limit of detection (LOD) for these compounds (Supplementary Table 3). All the samples were blank corrected for these compounds. The determined PFAS concentrations varied between 78 and 125% of the target value for the reference sample (Supplementary Table 3). The recoveries of all the internal standard compounds varied between 62 and 110%, except perfluorohexane sulphonate (PFHxS) which was close to 40%. We used the labelled counterpart for almost every individual PFAS determined in this study (Supplementary Table 3), allowing for a 40% limit of acceptable recovery as a QA/QC requirement. None of the here reported PFAS fall below that limit.
The limit of detection was defined as three times the signal-to-noise ratio for the specific matrix or in the case of detection in the blanks as the sum of the average of the blank level and three times SD. All the concentrations are presented on a wet weight basis (ww). Compound-dependent LOD ranged from 0.035 to 0.10 ng/g.
Among the south polar skuas from Svarthamaren, two individuals were sampled both in 2013/2014 and again in 2015/2016. Despite being analysed at the NMBU (whole blood, 2013/2014) and the NILU (plasma, 2015/2016), respectively, these recaptures had a similar PFAS pattern. Based on the similar PFAS pattern in skuas from different matrices, years, and laboratories as well as on the QA and QC of the different laboratories, we assume that variance in the results is caused by other factors than analytical. As matrix analysed can affect the concentrations, e.g., whole blood vs. plasma, we convert all the blood data to plasma as explained in detail in Supporting Information and Supplementary Table 4, to assess the effect on the trophic magnification.
Stable Isotope Analysis
To determine the relative TP of the studied individuals and calculate biomagnification of PFAS in the two analysed food webs, we quantified values of δ13C and δ15N. Available stable isotope data from the previous studies of the same material were included: skua red blood cells from Svarthamaren in 2013/2014 as part of Midthaug et al. (2021). All the samples from Dumont d’Urville in 2011/2012 were analysed for stable isotopes at Littoral Environnement et Sociétés (LIENSs) (Adélie penguins, Carravieri et al., 2020; south polar skuas, Carravieri et al., unpublished1).
The new samples analysed for stable isotopes in this study include Antarctic petrel egg whites, chicks and adults red blood cells, stomach samples, and skua red blood cells from Svarthamaren in 2015/2016. These were analysed at the Institute for Energy Technology (IFE) in Kjeller, Norway, except petrel chick that was analysed at LIENSs at the University La Rochelle, France.
The sample material was dried and packed into tin cups and combusted at 1,700°C to carbon dioxide (CO2) and nitrogen gas (Nox) in the ThermoQuest NCS 2500 Elemental Analyser (EA) (Thermo Instrument Systems Incorporation, Waltham, MA, United States). Afterward, each gas was separated and detected by a Micromass Optima isotope ratio mass spectrometry (IRMS) (Micromass, Manchester, United Kingdom).
The proportion of each stable isotope was expressed in delta (δ) notation, expressed in parts per thousand (‰) indicating deviation from a known standard (PeeDee Belemnite—Vienna for 13C and atmospheric N2 for 15N) according to Eq. 1.
Lipids were not extracted from any of the samples prior to analysis.
Data Treatment
All the data were analysed using R Statistical Software (version 3.4.2, R Core Team, 2018) using the non-parametric Wilcoxon–Mann–Whitney (WMW) U tests for comparison across life stages, species and colonies.
To compare PFAS concentrations across matrices (i.e., egg yolk, plasma, and whole blood), we conservatively used the highest PFAS LOD, which was from the NILU, as the LOD for all the samples. The LOD was established as three times the signal-to-noise ratio found in the samples. PFASs with at least 70% of the data above LOD were included in the statistical analysis. For the stomach content, this was adjusted to 40% to allow their inclusion in this study. For PFAS included in the statistics, we replaced any non-detects with a value between zero and the LOD, based on the distribution of the detected values, generated by maximum likelihood-based imputation (Baccarelli et al., 2005). In total, nine values (1% of the data) were replaced by imputation: two values for perfluorononanoate (PFNA), five values for perfluoroundecanoate (PFUnA), and one value for perfluorododecanoate (PFDoA) in the new data and one value for perfluorotetradecanoate (PFTeA) in south polar skuas from Dumont d’Urville.
Biomagnification and Trophic Magnification Factors
To estimate biomagnification at Svarthamaren and Dumont d’Urville of PFAS compounds that were quantified in both the predator and prey, we calculated the BMF and the BMF adjusted for one TP (BMF-TP). The TP adjustment of the BMFs is based on the relative TPs estimated for the prey and predator based on the δ15N values. The calculation of TP for birds at Dumont d’Urville used an average Adélie penguin chick δ15N value as a baseline as this showed only minor variation (10.2 ± 0.2‰, 10.56–11.29‰) (Cherel, 2008). At Svarthamaren, the lowest δ15N value measured in the petrel stomachs was selected as a baseline, as these spanned a wide range (7.2 ± 2.1‰, 5.10–10.30‰).
We estimated TMFs by regressing the PFAS concentrations onto the TP for Svarthamaren, as it was the only colony where we had three trophic levels (Borgå et al., 2012; Kidd et al., 2019). The corresponding equations can be found in Borgå and Ruus (2019) and in Supporting Information.
Results and Discussion
Of the PFAS analysed, seven substances were present in most samples across colonies, species and/or matrices: perfluorooctanesulfonic acid (PFOS), PFNA, perfluorodecanoate (PFDA), PFUnA, PFDoA, perfluorotridecanoate (PFTriA), and PFTeA (Table 1 and Figure 2). The exception was petrel stomach content where only PFUnA was present and Adélie penguins where only PFNA, PFDA, and PFUnA were present in more than one individual as well as perfluoroheptane sulphonate (PFHpS) and PFOS being present in one of ten individuals. Overall, PFAS concentrations were lower in the prey than the predators and were in general higher at Svarthamaren than Dumont d’Urville (Table 1). PFOS concentrations in the skuas (Svarthamaren: 10–20 ng/g wet weight plasma, Dumont d’Urville: 1.3–8.5 ng/g wet weight plasma, Table 1) were comparable to or in the lower range of concentrations reported in other seabirds (summarised in Escoruela et al., 2018).
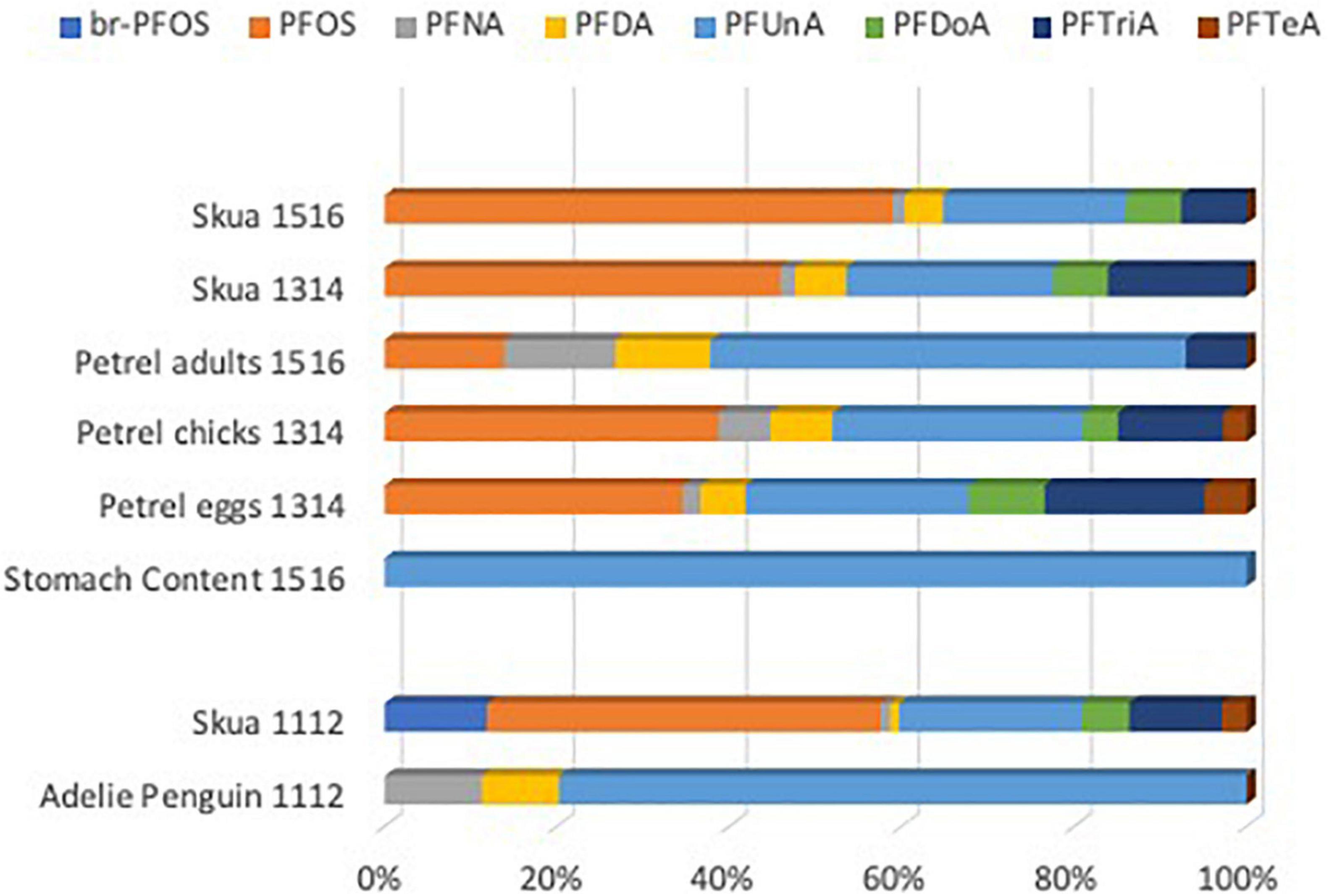
Figure 2. Relative pattern of the per- and polyfluoroalkyl substance (PFAS) compounds (% of summed PFAS) found in more than the four groups of samples at Svarthamaren, Dronning Maud’s Land in 2013/2014 and 2015/2016 and Dumont d’Urville, Adélie Land in 2011/2012 in Antarctica. The patterns show PFAS compounds with two or more detections, representing the mean of values for the respective compound.
Dietary Descriptors
At Svarthamaren, the petrel stomach content stable isotope values split the samples into one group with low δ15N and δ13C values and another group with higher δ15N values (accounting for approximately one trophic level) and slightly higher δ13C (Figure 3 and Table 2), suggesting a petrel diet of crustaceans or fish and squid, respectively. The petrel stomach content with the highest δ15N value was similar to the petrel blood and one stomach content was traced to a specific adult petrel that had an even higher δ15N value than the adult blood (Figure 3). This reflects the classical challenge of the stomach content approach, as it only reflects the immediate short-term diet (i.e., last meal) and not the main prey in the long term. In addition, the chitin exoskeleton of zooplankton, which has a low δ15N value, resists the digestion process longer than other tissues, resulting in lower stomach content δ15N value the longer the digestion (Cherel, 2008). In this study, the stomach content was too digested to allow detailed evaluation of prey composition or duration of the digestion process; however, as discussed above, the highest δ15N values in the stomach probably reflect a diet of fish and cephalopods in addition to krill. Direct observations in the field from 2015 to 2016 showed a surprisingly low krill content compared to both 1992 (Lorentsen et al., 1998) and 2013 (Descamps et al., 2016; Descamps, personal observation).
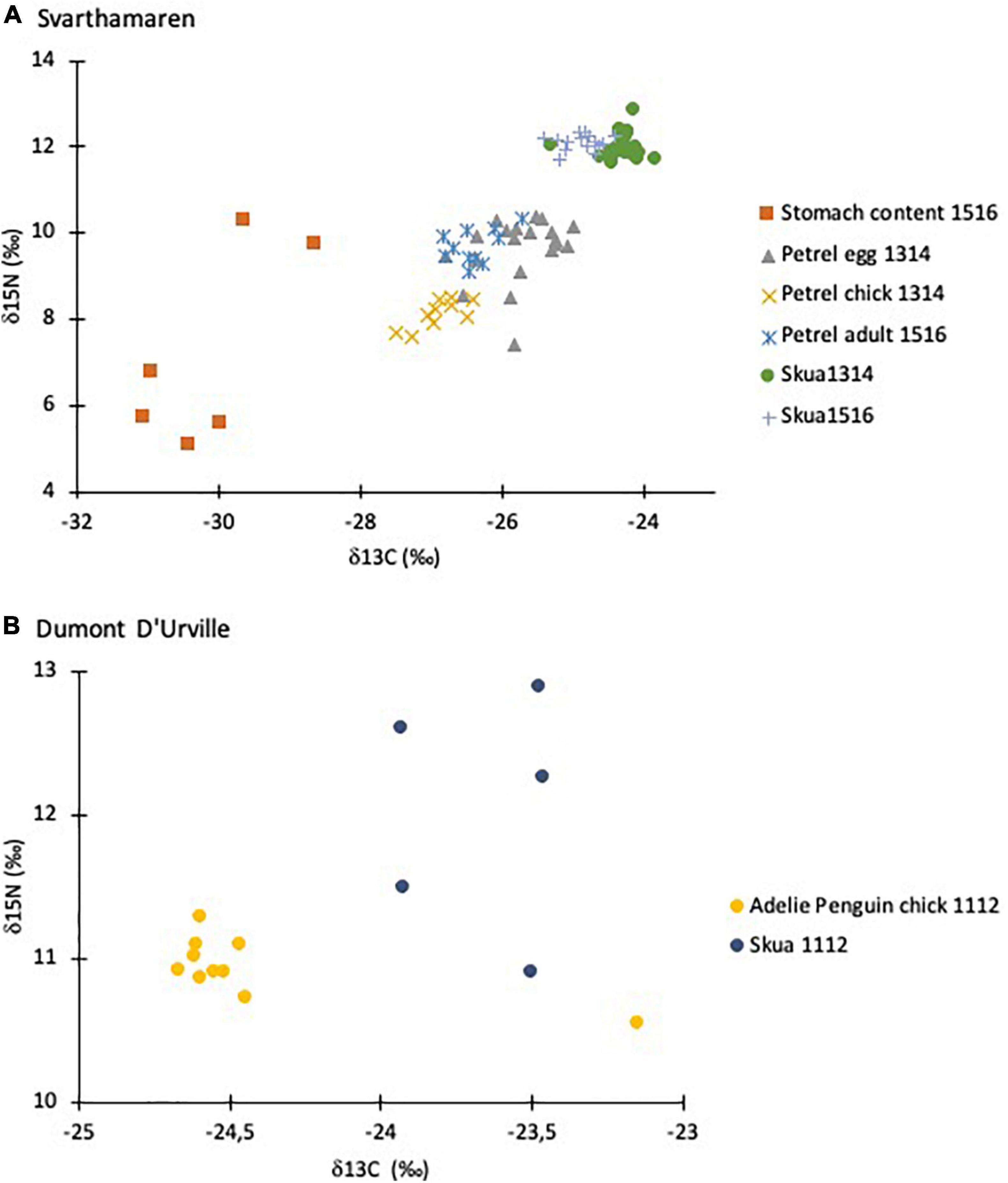
Figure 3. Stable isotope values of carbon (δ13C) and nitrogen (δ15N) of the Svarthamaren food web, Dronning Maud Land (A) and Dumont d’Urville, Adélie Land (B) in Antarctica. The two stomach contents with higher values of δ13C and δ15N were the ones with perfluoroundecanoate (PFUnA) concentrations quantified above the limit of detection. The arrows indicate the stomach sample (red) with detectable PFAS levels and the adult it was collected from (blue).
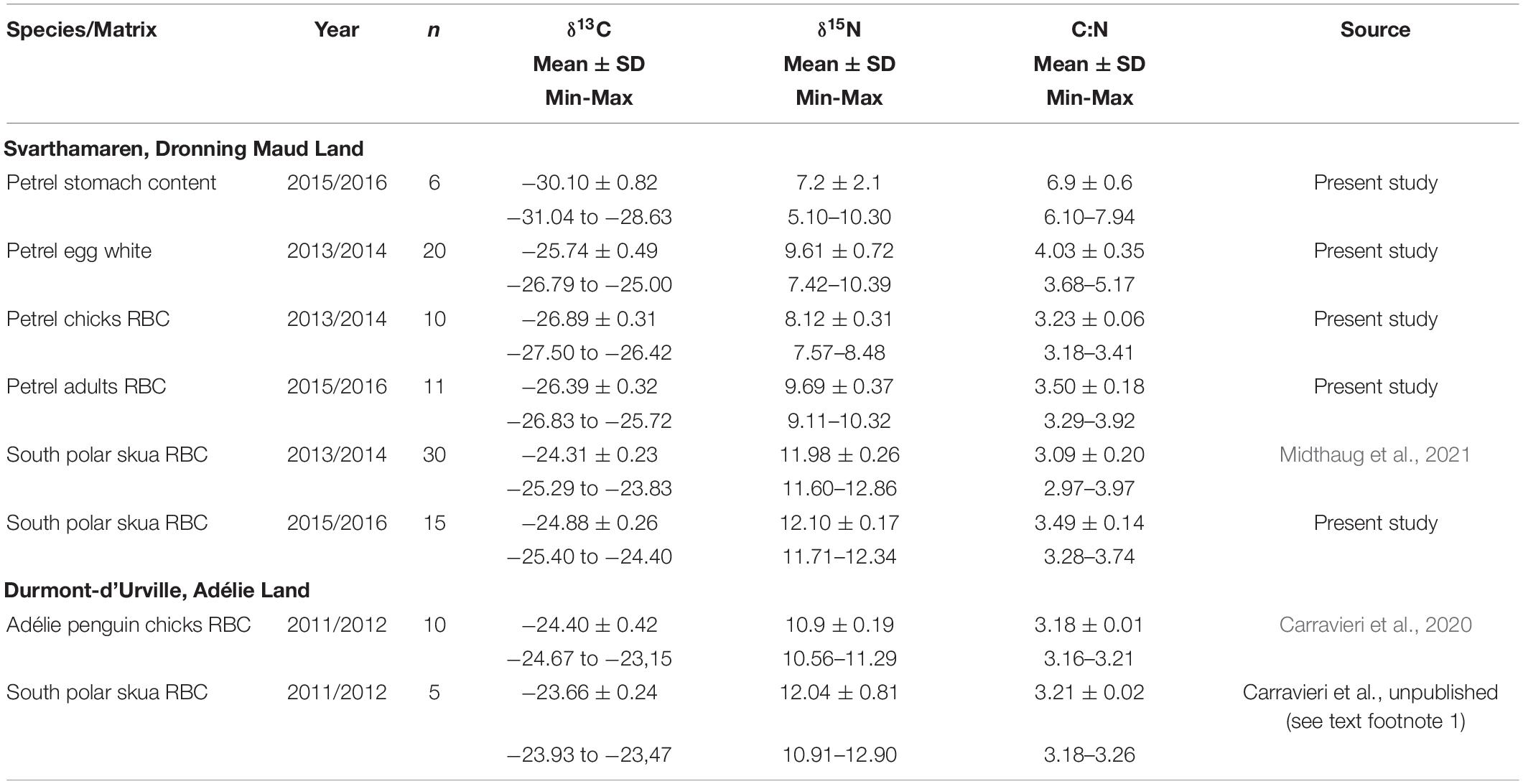
Table 2. Bulk isotopic values measured in Antarctic petrel stomach content, egg white, chick, and adult red blood cells (RBCs) and Adélie penguin chick RBC and south polar skua RBC in Antarctica during specified breeding seasons.
Red blood cell δ15N values in Antarctic petrels and south polar skuas from Svarthamaren reflected their middle and top TPs, respectively. All the blood C:N ratios were below 3.97 (Table 2), which indicate no or limited influence of lipid content on the δ13C in the blood matrices (Cherel et al., 2005). However, the petrel egg white C:N ranged from 3.7 to 5.2 and lipids, therefore, lowered the δ13C compared to tissues with all the C:N below 4. This makes a comparison of stable isotope values difficult when including different matrices; however, some details can be found in Supporting Information under section stable isotopes.
At Dumont d’Urville, the variance in both the δ15N and δ13C values was lower in Adélie penguins than in south polar skuas (Figure 2 and Table 2). The δ15N values of some south polar skua individuals overlap with those of penguins, which reflect that Adélie penguin chicks are not the sole prey of south polar skuas, in line with the observation of a varied south polar skuas diet in the coastal colonies, including krill and fish (Young, 1963; Furness, 2010; Olsen, 2010; Carravieri et al., 2017). This varied diet including krill and fish may explain the lower PFAS concentrations in Dumont d’Urville compared to Svarthamaren.
Per- and Polyfluoroalkyl Substance at Svarthamaren
At Svarthamaren, only PFUnA was quantified above LOD in the Antarctic petrel stomach content, in only two of the six samples, with values close to the LOD (51 and 60 pg/g ww vs. LOD 50 pg/g ww). The PFUnA was above LOD in the stomach samples with elevated δ15N values, supporting biomagnification, compared to the samples with low δ15N and PFUnA below LOD. This precludes a strict crustacean-based diet (Cherel, 2008) and based on the few stomach samples available, we interpret the main diet of the adult petrels to be crustaceans, fish and cephalopods, which is also supported by previous studies (Lorentsen et al., 1998; Descamps et al., 2016; Delord et al., 2020; Carravieri et al., 2021). PFOS was below LOD in the stomach contents, even in the ones with higher δ15N.
In the Antarctic petrel eggs, chicks and adults, seven of the PFAS were quantified above LOD in sufficient samples across matrices to allow statistical analysis (PFOS, PFNA, PFDA, PFUnA, PFDoA, PFTriA, and PFTeA; Table 1). Adults had lower concentrations than chicks for all the PFAS (p < 0.05), except PFNA (p = 0.63) and PFDoA (p = 0.9). The petrel adults had a slightly different PFAS pattern than chicks, with more PFUnA and less PFOS in adults compared to chicks (Figure 2). This pattern difference coincides with higher δ15N values in adults than chicks, reflecting a more fish-rich diet in adults both the outside breeding and at beginning of breeding (Delord et al., 2020; Carravieri et al., 2021), compared to chicks that are fed mainly with Antarctic krill Euphausia superba (Lorentsen et al., 1998; Descamps et al., 2016). As PFUnA was the only analyte quantified in the stomach content that also represented a higher relative TP, fish and cephalopods in the Antarctic waters are likely rich in PFUnA. Thus, the PFAS pattern of Antarctic petrel adults likely reflects PFAS accumulation from fish, while the chick’s PFAS pattern likely reflects PFAS accumulation from krill. The high content of PFUnA has also been found in fish-eating seabirds from other regions such as Spanish breeding colonies (Escoruela et al., 2018; Colomer-Vidal et al., 2022). The higher PFAS concentration in chicks than adults suggests that krill in Antarctica has relatively high PFAS concentrations and/or that maternal transfer to the egg is efficient. Maternal transfer efficiency is inconclusive from this study, as we do not know the sex of the adult petrels and as PFAS concentrations in egg yolks were similar, higher or lower than in adult and chick red blood cells, depending on the compound; refer to further details in Supporting Information. The variable and generally low maternal transfer have been reported for PFAS in other seabirds (Hitchcock et al., 2019; Knudtzon et al., 2021); however, eggs are considered as the favourable matrix for monitoring PFAS (Pereira et al., 2021).
Svarthamaren skuas had quantifiable concentrations of six PFAS in both years (PFOS, PFNA, PFDA, PFUnA, PFDoA, and PFTriA). In addition, three of the 17 skuas from 2015 to 2016 had low but quantifiable levels of PFTeA and PFHxS was found in five of 30 skuas from 2013 to 2014. The PFAS concentrations were higher in skuas in 2015/2016 than 2013/2014 (p < 0.05), with the exception of PFTriA (p > 0.05). However, it is not possible to make temporal inferences, as the 2013/2014 samples were whole blood, whereas the 2015/2016 samples were plasma. When converting the PFAS concentrations from whole blood to plasma to allow comparison (Supplementary Table 1; Kärrman et al., 2006; Hanssen et al., 2013; Lucia et al., 2017; Poothong et al., 2017), the PFAS concentrations still increased from 2013/2014 to 2015/2016 at Svarthamaren and were slightly higher than in skuas at Dumont d’Urville.
Per- and Polyfluoroalkyl Substance at Dumont d’Urville
Only PFUnA was quantified above LOD in all the ten plasma samples of Adélie penguins from Dumont d’Urville. We also found detectable levels of PFNA, PFDA, PFHpS, and PFOS in some individuals, but in lower concentrations than in the Antarctic petrels at Svarthamaren (Table 1). The south polar skuas from Dumont d’Urville had concentrations of PFHpS, branched PFOS (br-PFOS), PFOS, PFNA, PFDA, PFUnA, PFDoA, PFTriA, and PFTeA above LOD. Of these, PFNA, PFDA, and PFHpS had a low frequency of detection and, therefore, were not included in the statistical analysis.
Per- and Polyfluoroalkyl Substance Comparison Across Colonies
The absolute PFAS concentrations in south polar skuas were higher at Svarthamaren (both the years) than Dumont d’Urville (p < 0.001), except PFTeA that was highest at Dumont d’Urville (p < 0.01). The quantified PFAS was the same across colonies, with the exception of quantifiable levels of branched PFOS and PFTeA at Dumont d’Urville. As perfluoropentane sulphonate (PFPS), perfluorononane sulphonate (PFNS), perfluorodecane sulphonate (PFDcS), and perfluorobutanoate (PFBA) were not analysed in south polar skuas from Dumont d’Urville, we cannot compare these across colonies.
The skua PFAS pattern was dominated by PFOS and PFUnA, but differed between colonies with the presence of branched PFOS and PFTeA in most skuas from Dumont d’Urville and of PFNA and PFDA in skuas from Svarthamaren (Figure 1). As the biotransformation ability depends on species, pattern differences most likely reflect extrinsic factors such as exposure, either within Antarctica or during wintering. The higher relative contribution of PFOS in the skuas compared to other PFAS (Figure 2) can be explained by the slower elimination and higher retention of PFOS compared to other PFAS caused by slower urinary excretion of PFOS (Harada et al., 2005; Kowalczyk et al., 2012) and recirculation in the entero-hepatic system (Johnson et al., 1984; Lau et al., 2004). This would lead to greater biomagnification of PFOS compared to other PFAS.
At Svarthamaren, the skua PFAS pattern was dominated by PFOS followed by PFUnA, which was closer to that of Antarctic petrel eggs and chicks (more PFOS), which constitute the skuas’ prey, than to the adult petrel (more PFUnA) (Figure 2). The Antarctic petrel eggs and chicks also had a higher relative contribution of PFTeA. Higher PFOS levels and a greater proportion of perfluorinated carboxylic acids (PFCA) is expected at northern latitude compared to southern latitudes such as Antarctica, possibly due to the importance of the Circumpolar Current in protecting Antarctic food webs from water-phase-transported PFAS. However, we observed the reverse, with Svarthamaren having higher levels than Adélie Land, despite Svarthamaren skuas using more tropical areas during winter as compared to Adelie Land birds who reach sub-tropical/sub-antarctic areas (Weimerskirch et al., 2015).
At Dumont d’Urville, the PFAS pattern in the prey differed from the skuas, with the domination of PFUnA and a general lack of PFOS in Adélie penguin, being quantified above LOD in only one individual (Table 1). This is contrary to a recent comparison of muscle PFAS in Adélie penguins and south polar skuas from the Australian Antarctic sector which reported comparable patterns between the two species (Wild et al., 2022). In this study, penguins had the lowest PFAS concentrations of all the studied birds and also the fewest PFAS above LOD. The PFASs found in penguins were also found in the petrels and skuas from Svarthamaren, but PFNA and PFDA were not found in Dumont d’Urville skuas (Figure 2). The low PFAS levels in penguins reflect their local exposure within the Antarctic region, as they remain within the pack-ice year-round (Thiébot et al., 2019). The lower PFAS levels in penguins than petrels that also feed only within Antarctic waters (Delord et al., 2020) may be due to differences in regional PFAS exposure at the base of the food web, feeding on different trophic levels or differences in PFAS maternal transfer efficiencies across the species. Our data are, however, not applicable to address this hypothesis.
For skuas from Dumont d’Urville, the lack of PFNA and PFDA that were found in all the other avian samples, along with the presence of br-PFOS and PFTeA that were not found in any other samples including Adélie penguins, support that the dominating source of PFAS, including br-PFOS, is outside the Antarctic region in the wintering area, i.e., in the tropical and temperate regions along the Pacific Ocean to Japan (Weimerskirch et al., 2015). This would expose the skuas to continued emissions from Chinese factories, including both the linear and branched PFOS (Martin et al., 2010). PFAS concentrations in blood may very well reflect exposure on wintering grounds, as temporal integration of PFAS in avian blood is long, with estimated blood half-lives for PFOS of 230 days in chicken in a study of low chronic exposure (Tarazona et al., 2015). It was, however, surprising that the PFAS concentrations were lower in Dumont d’Urville skuas than at Svarthamaren, as we would expect feeding closer to areas of high emissions to result in elevated accumulated concentrations. The low concentrations may be an indication of feeding at lower trophic levels either during breeding or wintering or that the baseline PFAS exposure in the respective food web is lower in the Pacific Ocean or the Antarctic waters near Adélie Land. Svarthamaren skuas, on the other hand, reflect the PFAS found in the petrels, which are, therefore, a likely source.
Per- and Polyfluoroalkyl Substance Biomagnification and Trophic Magnification
Biomagnification factors were calculated for PFAS compounds quantified in both the predator and prey (Table 3). This included PFOS, PFNA, PFDA, PFUnA, PFDoA, and PFTriA at Svarthamaren and PFOS and PFUnA at Dumont d’Urville.
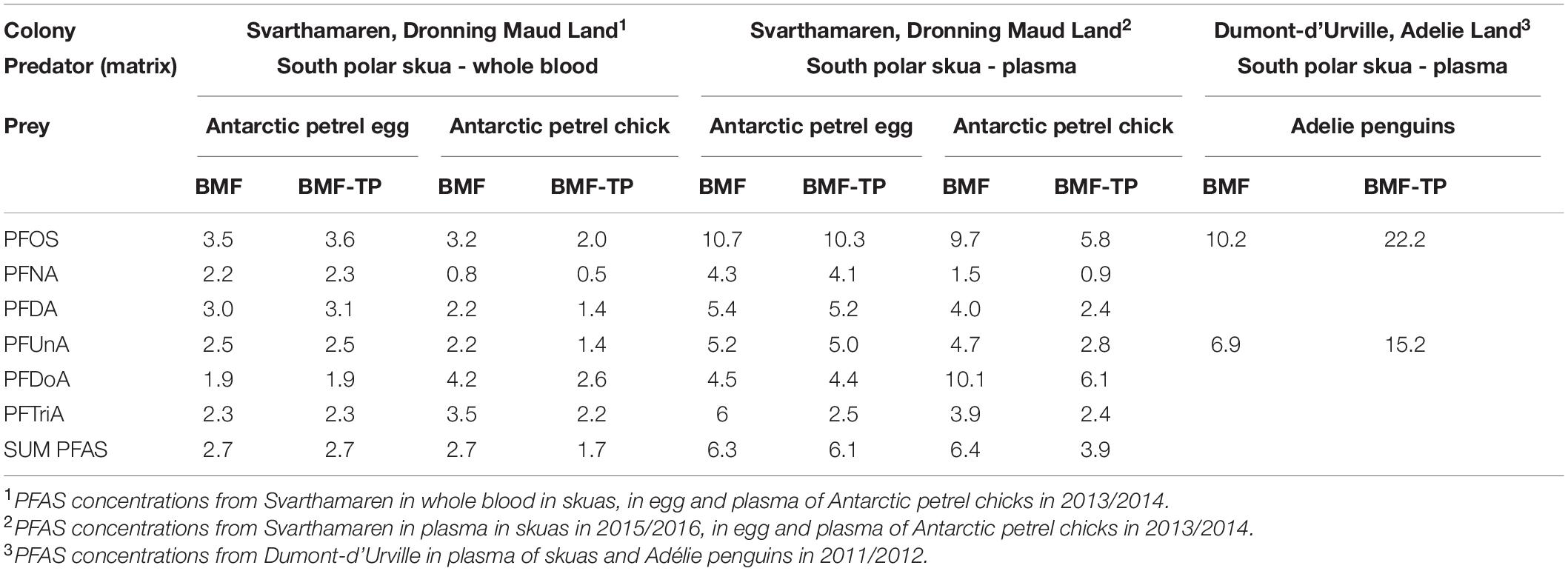
Table 3. Biomagnification factor (BMF) and BMF adjusted to one trophic position (BMF-TP) for PFAS biomagnifying to south polar skuas as a predator at the colony in Svarthamaren (season 2013/2014) and the colony of Dumont d’Urville (season 2011/2012).
As the skua PFAS from Svarthamaren was analysed in different tissues in 2013/2014 and 2015/2016, with different PFAS concentrations, year-specific BMFs were calculated, using the same prey (Table 3). The PFAS concentrations in the skuas from Svarthamaren (both the sampling years) were higher than those in Antarctic petrels (all the life stages), resulting in BMFs for all the PFAS at Svarthamaren above 1, except for BMFPFNA which was 0.8 in 2013/2014, but 1.5 in 2015/2016. Due to higher PFAS levels in skuas in 2015/2016 (p < 0.001), the BMFs were accordingly higher. Adjustment by TP (BMF-TP) did not change the BMF values significantly (Table 3), which confirms the correct prey for the Svarthamaren skuas. The comparable BMF and BMF-TP values for the colony of Svarthamaren support biomagnification of PFAS in south polar skuas locally from the Antarctic petrels during breeding.
The BMFPFOS and BMFPFUnA for Dumont d’Urville are also above 1 and were higher than at Svarthamaren (Table 3). We expected that if we had analysed the representative prey of skuas at the two colonies, the BMFs for PFAS should be comparable across populations. In addition, after adjusting the Dumont d’Urville BMF to represent one trophic level, the BMF-TP was almost double the BMF, suggesting that penguins cannot explain alone the PFAS exposure of Dumont d’Urville south polar skuas. This is counter to Wild et al. (2022) that attributed one order of magnitude increase in PFAS concentrations from Adélie penguins to skuas in the Australian sector to skuas’ higher TP rather than sources within vs. outside of the Antarctica. This conclusion was also based on their similar PFAS pattern (Wild et al., 2022). In this study, the Dumont d’Urville BMFPFOS and BMF-TPPFOS were based on only one PFOS measurement above the detection limit in Adélie penguins.
At both the colonies, the BMFPFOS was within the reported range for Arctic predatory birds (Tomy et al., 2004; Haukås et al., 2007), which, however, is broad, with BMFPFOS from 5 to 39. The BMFPFUnA comparison was hampered by the limited information on biomagnification of PFUnA in birds, but comparisons with other animals indicate that our results from both the colonies are within the reported BMFPFUnA range of 3 to 7 for similar TPs (Powley et al., 2008; Tomy et al., 2009).
Perfluoroundecanoate from Svarthamaren was the only compound for which the TMF could be calculated, as it was the sole PFAS with levels above LOD through the food web. Using the measured and converted concentrations from whole-blood skua plasma (Supplementary Table 3), it resulted in TMFPFUnA from 2.7 ± 1.2 to 4.9 ± 1.2 depending on conversion and data (Figure 3). The TMFPFUnA averaged 3.1 ± 1.2 (p < 0.001), including only measured concentrations from both the sampling years, whole blood in 2013/2014 and plasma in 2015/2016 (Figure 4). If all the stomach content samples are included in the regression, even the ones with PFUnA levels below LOD, the TMFPFUnA for measured data increased to 3.7 ± 1.2. The TMFPFUnA range in this study (2.7–4.9) was comparable to previously reported TMF for a food chain that included bird predators (TMF = 3.1; Xu et al., 2014).
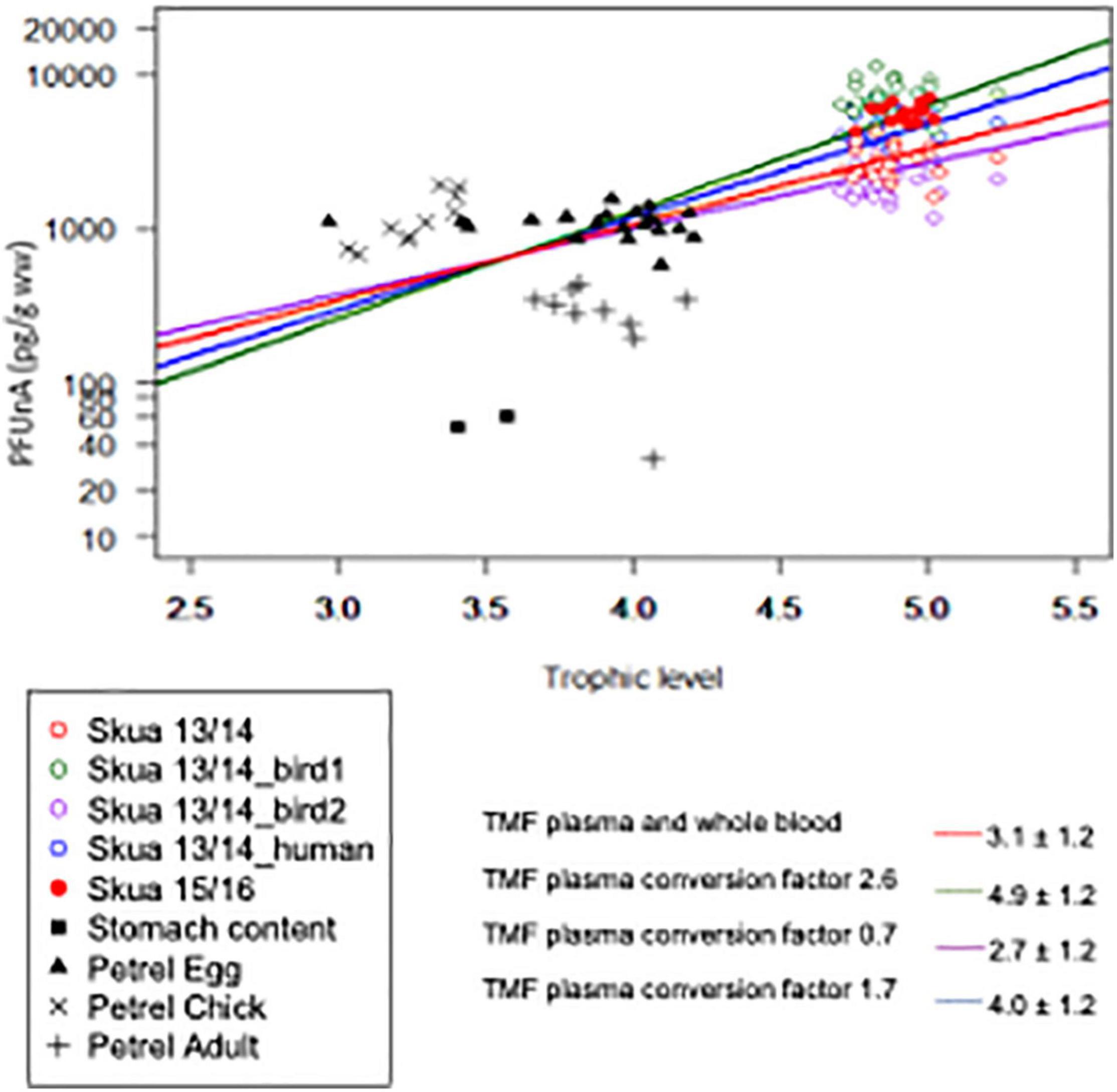
Figure 4. Trophic magnification factors (TMFs) for PFUnA in the Svarthamaren food web leading to south polar skuas. The different regressions reflect the inclusion of the skua data with original data with skua whole blood from 2013 to 2014 (Skua_13/14—red, TMF = 3.1), converted skua plasma concentrations using the conversion factor 2.6 based on one ivory gull whole blood to plasma comparison in 2013 (Skua_13/14_bird 1—green TMF = 4.9), conversion factor 0.7 based on different ivory gulls from 2011 to 2014 (Skua_13/14_bird 2—purple, TMF = 2.7; Lucia et al., 2017) or conversion factor 1.7 based on human blood to plasma conversion (Skua_13/14_human—blue, TMF = 4.0; Poothong et al., 2017). All the regressions included measured skua plasmas from 2015 to 2016 (Skua_15/16, filled red symbol).
In summary, the PFAS concentrations in south polar skuas varied between years and were higher at Svarthamaren in Dronning Maud Land than at Dumont d’Urville in Adélie Land. From three lines of evidence (PFAS pattern, the difference in BMF between Dumont d’Urville and Svarthamaren and the difference between BMF and trophic level adjusted BMF in Dumont d’Urville and not in Svarthamaren), we suggest that the PFAS concentrations in south polar skua at Svarthamaren can be explained by the prey at the colony (i.e., Antarctic petrel chicks and eggs), whereas the PFAS sources at Dumont d’Urville must be additional to Adélie penguin, either within or outside the Antarctic region.
Data Availability Statement
The raw data supporting the conclusions of this article will be made available by the authors, without undue reservation.
Ethics Statement
The animal study was reviewed and approved by Norwegian Food Safety Authority.
Author Contributions
KB and JOB contributed to the conception and design of the study. SD, OC, AC, YC, and JOB organised or conducted the fieldwork. DH, AP, LAAG, PL, and HB were responsible for, or conducted, the PFAS analyses. PB for the stable isotope analyses from Dumont d’Urville. LAAG organised the data, performed the statistical analysis, and wrote the first draft of the manuscript. KB wrote sections of the manuscript. KB, JOB, and GWG supervised LAAG. All authors contributed to manuscript revision, read, and approved the submitted version.
Funding
This study at Svarthamaren was funded by the Norwegian Research Council project no. 243763 (Polar Ecotox), 247698 (IceBird) and the Norwegian Climate and Environment Ministry (Arctic 2030—Toxkart no. QZA-15/0137). The Institut Polaire Français Paul Emile Victor (IPEV) (Program N° 109, C Barbraud) and ANR PolarTOP (O Chastel) funded the work in Adélie Land. We thank the Contrat de Projet Etat-Région (CPER) and the European Regional Development Fund (FEDER) for funding the IRMS of LIENSs. We also thank the Institut Universitaire de France (IUF) for support to PB as a senior member.
Conflict of Interest
The authors declare that the research was conducted in the absence of any commercial or financial relationships that could be construed as a potential conflict of interest.
Publisher’s Note
All claims expressed in this article are solely those of the authors and do not necessarily represent those of their affiliated organizations, or those of the publisher, the editors and the reviewers. Any product that may be evaluated in this article, or claim that may be made by its manufacturer, is not guaranteed or endorsed by the publisher.
Acknowledgments
We thank Arnaud Tarroux, Eeva Soininen, Géraldine Mabille, and Alice Trevail for assistance in the field; Hilde Midthaug and Norith Eckbo for contributions with the preparation of skua samples from Svarthamaren for PFAS analyses at the NMBU and the NILU, respectively, and Daniel Hitchcock for assistance with R. We are grateful to G. Guillou from the Plateforme Analyses Isotopiques of LIENSs laboratory for running isotope analyses.
Supplementary Material
The Supplementary Material for this article can be found online at: https://www.frontiersin.org/articles/10.3389/fmars.2022.819525/full#supplementary-material
Footnotes
- ^ Carravieri, A., Bustamante, P., Labadie, P., Weimerskirch, H., Delord, K., Budzinski, H., et al. (unpublished). Effect of Wintering Habitat on Exposure to Metals and Persistent Organic Pollutants in Southern Ocean Skuas. Bordeaux: Université de Bordeaux [Environnements et Paléoenvironnements Océaniques et Continentaux (EPOC)].
References
Baccarelli, A., Pfeiffer, R., Consonni, D., Pesatori, A. C., Bonzini, M., Patterson, D. G., et al. (2005). Handling of dioxin measurement data in the presence of non-detectable values: overview of available methods and their application in the Seveso chloracne study. Chemosphere 60, 898–906. doi: 10.1016/j.chemosphere.2005.01.055
Bengtson Nash, S., Rintoul, S. R., Kawaguchi, S., Staniland, I., van den Hoff, J., Tierney, M., et al. (2010). Perfluorinated compounds in the Antarctic region: ocean circulation provides prolonged protection from distant sources. Environ. Pollut. 158, 2985–2991. doi: 10.1016/j.envpol.2010.05.024
Borgå, K., Kidd, K. A., Muir, D. C., Berglund, O., Conder, J. M., Gobas, F. A., et al. (2012). Trophic magnification factors: considerations of ecology, ecosystems, and study design. Integr. Environ. Assess. Manag. 8, 64–84. doi: 10.1002/ieam.244
Borgå, K., and Ruus, A. (2019). “Quantifying Bioaccumulation in the Aquatic Environment,” in Springer Nature Experiments Series: methods in Pharmacology and Toxicology, ed. Y. J. Kang (Berlin: Springer Nature), doi: 10.1007/7653_2019_36
Brooke, M. D., Keith, D., and Røv, N. (1999). Exploitation of inland-breeding Antarctic petrels by south polar skuas. Oecologia 121, 25–31. doi: 10.1007/s004420050903
Buck, R. C., Franklin, J., Berger, U., Conder, J. M., Cousins, I. T., de Voogt, P., et al. (2011). Perfluoroalkyl and polyfluoroalkyl substances in the environment: terminology, classification, and origins. Integr. Environ. Assess. Manag. 7, 513–541. doi: 10.1002/ieam.258
Busdieker, K. M., Patrick, S. C., Trevail, A. M., and Descamps, S. (2020). Prey density affects predator foraging strategy in an Antarctic ecosystem. Ecol. Evol. 10, 350–359. doi: 10.1002/ece3.5899
Carravieri, A., Bustamante, P., Labadie, P., Budzinski, H., Chastel, O., and Cherel, Y. (2020). Trace elements and persistent organic pollutants in chicks of 13 seabird species from Antarctica to the subtropics. Environ. Int. 134:105225. doi: 10.1016/j.envint.2019.105225
Carravieri, A., Cherel, Y., Brault-Favrou, M., Churlaud, C., Peluhet, L., Labadie, P., et al. (2017). From Antarctica to the subtropics: contrasted geographical concentrations of selenium, mercury, and persistent organic pollutants in skua chicks (Catharacta spp.). Environ. Pollut. 228, 464–473. doi: 10.1016/j.envpol.2017.05.053
Carravieri, A., Warner, N. A., Herzke, D., Brault-Favrou, M., Tarroux, A., Fort, J., et al. (2021). Trophic and fitness correlates of mercury and organochlorine compound residues in egg-laying Antarctic petrels. Environ. Res. 193:110518. doi: 10.1016/j.envres.2020.110518
Cherel, Y. (2008). Isotopic niches of emperor and Adélie penguins in Adélie Land, Antarctica. Mar. Biol. 154, 813–821. doi: 10.1007/s00227-008-0974-3
Cherel, Y., Hobson, K. A., Bailleul, F., and Groscolas, R. (2005). Nutrition, physiology, and stable isotopes: new Information from fasting and molting penguins. Ecology 86, 2881–2888.
Colomer-Vidal, P., Bertolero, A., Alcaraz, C., Garreta-Lara, E., Santos, F. J., and Lacorte, S. (2022). Distribution and ten-year temporal trends (2009-2018) of perfluoroalkyl substances in gull eggs from Spanish breeding colonies. Environ. Pollut. 293:118555. doi: 10.1016/j.envpol.2021.118555
Delord, K., Kato, A., Tarroux, A., Orgeret, F., Cotté, C., Ropert-Coudert, Y., et al. (2020). Antarctic petrels ‘on the ice rocks’: wintering strategy of an Antarctic seabird. R. Soc. Open Sci. 7:191429. doi: 10.1098/rsos.191429
Descamps, S., Tarroux, A., Cherel, Y., Delord, K., Godø, O. R., Kato, A., et al. (2016). At-sea distribution and prey selection of Antarctic petrels and commercial krill fisheries. PLoS One 11:e0156968. doi: 10.1371/journal.pone.0156968
Escoruela, J., Garreta, E., Ramos, R., González-Solís, J., and Lacorte, S. (2018). Occurrence of Per- and Polyfluoroalkyl substances in Calonectris shearwaters breeding along the Mediterranean and Atlantic colonies. Mar. Pollut. Bull. 131, 335–340. doi: 10.1016/j.marpolbul.2018.04.032
Franklin, J. (2016). How reliable are field-derived biomagnification factors and trophic magnification factors as indicators of bioaccumulation potential? Conclusions from a case study on per- and polyfluoroalkyl substances. Integr. Environ. Assess. Manag. 12, 6–20. doi: 10.1002/ieam.1642
Grønnestad, R., Villanger, G. D., Polder, A., Kovacs, K. M., Lydersen, C., Jenssen, B. M., et al. (2017). Maternal transfer of perfluoroalkyl substances in hooded seals. Environ. Toxicol. Chem. 36, 763–770. doi: 10.1002/etc.3623
Hanssen, L., Dudarev, A. A., Huber, S., Odland, J. Ø, Nieboer, E., and Sandanger, T. M. (2013). Partition of perfluoroalkyl substances (PFASs) in whole blood and plasma, assessed in maternal and umbilical cord samples from inhabitants of arctic Russia and Uzbekistan. Sci. Total Environ. 447, 430–437. doi: 10.1016/j.scitotenv.2013.01.029
Harada, K., Inoue, K., Morikawa, A., Yoshinaga, T., Saito, N., and Koizumi, A. (2005). Renal clearance of perfluorooctane sulfonate and perfluorooctanoate in humans and their species-specific excretion. Environ. Res. 99, 253–261. doi: 10.1016/j.envres.2004.12.003
Haukås, M., Berger, U., Hop, H., Gulliksen, B., and Gabrielsen, G. W. (2007). Bioaccumulation of per- and polyfluorinated alkyl substances (PFAS) in selected species from the Barents Sea food web. Environ. Pollut. 148, 360–371. doi: 10.1016/j.envpol.2006.09.021
Hitchcock, D. J., Andersen, T., Varpe, Ø, and Borgå, K. (2019). Effects of Maternal Reproductive Investment on Sex-Specific Pollutant Accumulation in Seabirds: a Meta-Analysis. Environ. Sci. Technol. 53, 7821–7829. doi: 10.1021/acs.est.9b01296
Johnson, D., Gibson, S. J., and Ober, R. E. (1984). Cholestyramine-enhanced fecal elimination of carbon-14 in rats after administration of ammonium [14C]-perfluorooctanoate or potassium [14C]-perfluorooctane sulfonate. Toxicol. Sci. 4, 972–976. doi: 10.1093/toxsci/4.6.972
Jouanneau, W., Bårdsen, B. J., Herzke, D., Johnsen, T. V., Eulaers, I., and Bustnes, J. O. (2020). Spatiotemporal analysis of perfluoroalkyl substances in white-tailed eagle (Haliaeetus albicilla) nestlings from Northern Norway-a ten-year study. Environ. Sci. Technol. 54, 5011–5020. doi: 10.1021/acs.est.9b06818
Kärrman, A., van Bavel, B., Järnberg, U., Hardell, L., and Lindström, G. (2006). Perfluorinated chemicals in relation to other persistent organic pollutants in human blood. Chemosphere 64, 1582–1591. doi: 10.1016/j.chemosphere.2005.11.040
Kidd, K. A., Burkhard, L. P., Babut, M., Borgå, K., Muir, D. C., Perceval, O., et al. (2019). Practical advice for selecting or determining trophic magnification factors for application under the European Union Water Framework Directive. Integr. Environ. Assess. Manag. 15, 266–277. doi: 10.1002/ieam.4102
Knudtzon, N. C., Thorstensen, H., Ruus, A., Helberg, M., Bæk, K., Enge, E. K., et al. (2021). Maternal transfer and occurrence of siloxanes, chlorinated paraffins, metals, PFAS and legacy POPs in herring gulls (Larus argentatus) of different urban influence. Environ. Int. 152:106478. doi: 10.1016/j.envint.2021.106478
Kotthoff, M., Müller, J., Jürling, H., Schlummer, M., and Fiedler, D. (2015). Perfluoroalkyl and polyfluoroalkyl substances in consumer products. Environ. Sci. Pollut. Res. 22, 14546–14559.
Kowalczyk, J., Ehlers, S., Fürst, P., Schafft, H., and Lahrssen-Wiederholt, M. (2012). Transfer of perfluorooctanoic acid (PFOA) and perfluorooctane sulfonate (PFOS) from contaminated feed into milk and meat of sheep: pilot study. Arch. Environ. Contam. Toxicol. 63, 288–298. doi: 10.1007/s00244-012-9759-2
Lau, C., Butenhoff, J. L., and Rogers, J. M. (2004). The developmental toxicity of perfluoroalkyl acids and their derivatives. Toxicol. Appl. Pharmacol. 198, 231–241. doi: 10.1016/j.taap.2003.11.031
Lorentsen, S.-H., Klages, N., and Røv, N. (1998). Diet and prey consumption of Antarctic petrels Thalassoica antarctica at Svarthamaren, Dronning Maud Land, and at sea outside the colony. Polar Biol. 19, 414–420. doi: 10.1007/s003000050267
Lucia, M., Strøm, H., Bustamante, P., Herzke, D., and Gabrielsen, G. W. (2017). Contamination of ivory gulls (Pagophila eburnea) at four colonies in Svalbard in relation to their trophic behaviour. Polar Biol. 40, 917–929. doi: 10.1007/s00300-016-2018-7
Mackay, D., Arnot, J. A., Gobas, F. A., and Powell, D. E. (2013). Mathematical relationships between metrics of chemical bioaccumulation in fish. Environ. Toxicol. Chem. 32, 1459–1466. doi: 10.1002/etc.2205
Martin, J. W., Asher, B. J., Beesoon, S., Benskin, J. P., and Ross, M. S. (2010). PFOS or PreFOS? Are perfluorooctane sulfonate precursors (PreFOS) important determinants of human and environmental perfluorooctane sulfonate (PFOS) exposure? J. Environ. Monit. 12, 1979–2004. doi: 10.1039/C0EM00295J
Midthaug, H. K., Hitchcock, D. J., Bustnes, J. O., Polder, A., Descamps, S., Tarroux, A., et al. (2021). Within and between breeding-season changes in contaminant occurrence and body condition in the Antarctic breeding south polar skua. Environ. Pollut. 284:117434. doi: 10.1016/j.envpol.2021.117434
Moody, C. A., and Field, J. A. (2000). Perfluorinated surfactants and the environmental implications of their use in fire-fighting foams. Environ. Sci. Technol. 34, 3864–3870.
Munoz, G., Labadie, P., Geneste, E., Pardon, P., Tartu, S., Chastel, O., et al. (2017). Biomonitoring of fluoroalkylated substances in Antarctica seabird plasma: development and validation of a fast and rugged method using on-line concentration liquid chromatography tandem mass spectrometry. J. Chromatogr. A 1513, 107–117. doi: 10.1016/j.chroma.2017.07.024
Pacoureau, N., Delord, K., Jenouvrier, S., and Barbraud, C. (2019). Demographic and population responses of an apex predator to climate and its prey: a long-term study of South Polar skuas. Ecol. Monogr. 89:e01388.
Paul, A. G., Jones, K. C., and Sweetman, A. J. (2009). A first global production, emission, and environmental inventory for perfluorooctane sulfonate. Environ. Sci. Technol. 43, 386–392. doi: 10.1021/es802216n
Pereira, M. P., Lacorte, S., Walker, L. A., and Shore, R. F. (2021). Contrasting long term temporal trends in perfluoroalkyl substances (PFAS) in eggs of the northern gannet (Morus bassanus) from two UK colonies. Sci. Total Environ. 754:141900. doi: 10.1016/j.scitotenv.2020.141900
Poothong, S., Thomsen, C., Padilla-Sanchez, J. A., Papadopoulou, E., and Haug, L. S. (2017). Distribution of novel and well-known poly- and perfluoroalkyl substances (PFASs) in human serum, plasma, and whole blood. Environ. Sci. Technol. 51, 13388–13396. doi: 10.1021/acs.est.7b03299
Powley, C. R., George, S. W., Russell, M. H., Hoke, R. A., and Buck, R. C. (2008). Polyfluorinated chemicals in a spatially and temporally integrated food web in the Western Arctic. Chemosphere 70, 664–672. doi: 10.1016/j.chemosphere.2007.06.067
R Core Team (2018). R: A Language and Environment for Statistical Computing. Vienna: R Foundation for Statistical Computing. Available online at: https://www.R-project.org
Ridoux, V., and Offredo, C. (1989). The diets of five summer breeding seabirds in Adélie Land. Antarctica. Polar Biol. 9, 137–145. doi: 10.1007/bf00297168
Roscales, J. L., Vicente, A., Ryan, P. G., González-Solís, J., and Jiménez, B. (2019). Spatial and Interspecies Heterogeneity in Concentrations of Perfluoroalkyl Substances (PFASs) in Seabirds of the Southern Ocean. Environ. Sci. Technol. 53, 9855–9865. doi: 10.1021/acs.est.9b02677
Tao, L., Kannan, K., Kajiwara, N., Costa, M. A., Fillman, G., Takahashi, S., et al. (2006). Perfluorooctanesulfonate and related fluorochemicals in albatrosses, elephant seals, penguins and polar skuas from the Southern Ocean. Environ. Sci. Technol. 40, 7642–7648. doi: 10.1021/es061513u
Tarazona, J. V., Rodríguez, C., Alonso, E., Sáez, M., González, F., San Andrés, M. D., et al. (2015). Toxicokinetics of perfluorooctane sulfonate in birds under environmentally realistic exposure conditions and development of a kinetic predictive model. Toxicol. Lett. 232, 363–368. doi: 10.1016/j.toxlet.2014.11.022
Thiébot, J. B., Ropert-Coudert, Y., Raclot, T., Poupart, T., Kato, A., and Takahashi, A. (2019). Adélie penguins’ extensive seasonal migration supports dynamic Marine Protected Area planning in Antarctica. Mar. Policy 109:103692. doi: 10.1016/j.marpol.2019.103692
Tomy, G. T., Budakowski, W., Halldorson, T., Helm, P. A., Stern, G. A., Friesen, K., et al. (2004). Fluorinated organic compounds in an Eastern Arctic marine food web. Environ. Sci. Technol. 38, 6475–6481. doi: 10.1021/es049620g
Tomy, G. T., Pleskach, K., Ferguson, S. H., Hare, J., Stern, G., MacInnis, G., et al. (2009). Trophodynamics of some PFCs and BFRs in a Western Canadian Arctic marine food web [WWW Document]. Environ. Sci. Technol. 43, 4076–4081. doi: 10.1021/es900162n
Warner, N. A., Sagerup, K., Kristoffersen, S., Herzke, D., Gabrielsen, G. W., and Jenssen, B. M. (2019). Snow buntings (Plectrophenax nivealis) as bio-indicators for exposure differences to legacy and emerging persistent organic pollutants from the Arctic terrestrial environment on Svalbard. Sci. Total Environ. 667, 638–647. doi: 10.1016/j.scitotenv.2019.02.351
Weimerskirch, H., Tarroux, A., Chastel, O., Delord, K., Cherel, Y., and Descamps, S. (2015). Population-specific wintering distributions of adult south polar skuas over three oceans. Mar. Ecol. Prog. Ser. 538, 229–237. doi: 10.3354/meps11465
Wienecke, B. C., Lawless, R., Rodary, D., Bost, C.-A., Thomson, R., Pauly, T., et al. (2000). Adélie penguin foraging behaviour and krill abundance along the Wilkes and Adélie land coasts, Antarctica. Deep Sea Res. Part II Top. Stud. Oceanogr. 47, 2573–2587. doi: 10.1016/S0967-0645(00)00036-9
Wild, S., Eulaers, I., Covaci, A., Bossi, R., Hawker, D., Cropp, R., et al. (2022). South polar skua (Catharacta maccormicki) as biovectors for long-range transport of persistent organic pollutants to Antarctica. Environ. Pollut. 292:118358. doi: 10.1016/j.envpol.2021.118358
Wilson, R. P. (1984). An improved stomach pump for penguins and other seabirds. J. Field Ornithol. 55, 109–112.
Xu, J., Guo, C.-S., Zhang, Y., and Meng, W. (2014). Bioaccumulation and trophic transfer of perfluorinated compounds in a eutrophic freshwater food web. Environ. Pollut. 184, 254–261. doi: 10.1016/j.envpol.2013.09.011
Keywords: biomagnification, PFAS, seabirds, Thalassoica antarctica, Pygoscelis adeliae
Citation: Alfaro Garcia LA, Descamps S, Herzke D, Chastel O, Carravieri A, Cherel Y, Labadie P, Budzinski H, Munoz G, Bustamante P, Polder A, Gabrielsen GW, Bustnes JO and Borgå K (2022) Bioaccumulation of Per and Polyfluoroalkyl Substances in Antarctic Breeding South Polar Skuas (Catharacta maccormicki) and Their Prey. Front. Mar. Sci. 9:819525. doi: 10.3389/fmars.2022.819525
Received: 21 November 2021; Accepted: 14 February 2022;
Published: 31 March 2022.
Edited by:
Stefania Ancora, University of Siena, ItalyReviewed by:
Juliana Leonel, Federal University of Bahia, BrazilLieven Bervoets, University of Antwerp, Belgium
Silvia Lacorte, Institute of Environmental Assessment and Water Research (CSIC), Spain
Copyright © 2022 Alfaro Garcia, Descamps, Herzke, Chastel, Carravieri, Cherel, Labadie, Budzinski, Munoz, Bustamante, Polder, Gabrielsen, Bustnes and Borgå. This is an open-access article distributed under the terms of the Creative Commons Attribution License (CC BY). The use, distribution or reproduction in other forums is permitted, provided the original author(s) and the copyright owner(s) are credited and that the original publication in this journal is cited, in accordance with accepted academic practice. No use, distribution or reproduction is permitted which does not comply with these terms.
*Correspondence: Katrine Borgå, a2F0cmluZS5ib3JnYUBpYnYudWlvLm5v