- 1Functional Materials, Department of Applied Physics, School of Engineering Sciences, KTH Royal Institute of Technology, Stockholm, Sweden
- 2Department of Food Engineering and Technology, Central Institute of Technology Kokrajhar, BTR, Assam, India
- 3Department of Marine Science and Fisheries, Sultan Qaboos University, Muscat, Oman
- 4Center of Excellence in Marine Biotechnology, Sultan Qaboos University, Muscat, Oman
Biofouling is a major concern to the maritime industry. Biofouling increases fuel consumption, accelerates corrosion, clogs membranes and pipes, and reduces the buoyancy of marine installations, such as ships, platforms, and nets. While traditionally marine installations are protected by toxic biocidal coatings, due to recent environmental concerns and legislation, novel nanomaterial-based anti-fouling coatings are being developed. Hybrid nanocomposites of organic-inorganic materials give a possibility to combine the characteristics of both groups of material generating opportunities to prevent biofouling. The development of bio-inspired surface designs, progress in polymer science and advances in nanotechnology is significantly contributing to the development of eco-friendly marine coatings containing photocatalytic nanomaterials. The review mainly discusses photocatalysis, antifouling activity, and formulation of coatings using metal and metal oxide nanomaterials (nanoparticles, nanowires, nanorods). Additionally, applications of nanocomposite coatings for inhibition of micro- and macro-fouling in marine environments are reviewed.
Introduction
Biofouling is a process that any substrate in the marine environment is quickly covered by organisms. Biofouling is referred to as undesirable growth on submerged surfaces of micro- (bacteria and protists) and macro-fouling (invertebrates and algae) organisms (Wahl, 1989). Any submerged substratum is quickly covered with organic molecules and particles that can be colonized by microscopic organisms, forming biofilms (Wahl, 1989). Marine biofilms contain multiple species of bacteria, microalgae and diatoms (Salta et al., 2013). Biofilms can subsequently enhance or reduce settlement of larvae and spores of invertebrates and algae (Dobretsov et al., 2006; Dobretsov and Rittschof, 2020).
The maritime industry and naval forces across the world lose billions of US dollars due to biofouling of marine installations (Yebra et al., 2004; Schultz et al., 2010; Aghajani and Esmaeili, 2021). In order to prevent biofouling, industries apply antifouling coatings on exposed surfaces. The antifouling coatings feature in antimicrobial activity and generally prevent both micro- and macrofouling. The majority of antifouling coatings use toxic inorganic (copper) and organic biocides (isothiazolone) that leach out of the coating and kill biofouling organisms (Yebra et al., 2004). However, toxic biocides affect non-targeted marine organisms as well and accumulate in the marine environment. In 2008, the most potent antifouling agent triorganotin was banned by the International Maritime Organization (IMO) due to its adverse effects on the marine environment (Maréchal and Hellio, 2009). The non-toxic antifouling methods available in the market are often costly and are not as effective as traditional biocidal solutions. Thus, it is necessary to develop environmentally friendly solutions and nanotechnology-based applications can be of great help in order to do create non-toxic or low-toxic antifouling coatings.
Nanotechnology involves manipulation of materials at the nanometer scale in order to improve and obtain new properties of materials (Hornyak, 2008). Nanostructures with highly controlled and tunable properties can be synthesized by self-assembling of atoms. These nanostructures can either be zero dimensional (nanoparticles) (Grzelczak et al., 2008; Hornyak, 2008), one dimensional (nanowires) (Sugunan et al., 2006), two dimensional (thin films) (Aoki et al., 2005) or three dimensional (arrays, hierarchical structures) (Von Freymann et al., 2010). At nano-scale sizes, materials possess unique size-dependent properties that differ from their bulk, which can be exploited for diverse applications, in electronics (Lah and Zubir, 2018), medicine (Uskoković and Bertassoni, 2010), food (Kumar et al., 2019b), fuel (Chung and Manthiram, 2019), solar cells (Fei et al., 2012), sensors (Zhu et al., 2014), and water treatment (Baruah et al., 2012).
Nanocoatings have been used for biofouling prevention. Previous work reviewed the antifouling effects of nano- and micro-scale surface patterns which are inspired by nature (Scardino and De Nys, 2011; Bixler and Bhushan, 2012; Myan et al., 2013; Graham and Cady, 2014; Richards et al., 2020). The best example of such biomimetic antifouling coating is Sharklet AF™ which was inspired by the microtopography of the shark skin (Bechert et al., 2000). The C18 coatings with high levels of nano-roughness were found to inhibit the settlement of Ulva spores and its antifouling effect was shown to exceed that of a commercially available fouling release coating (Majumdar et al., 2008). Superhydrophobic coatings with nano-scaled roughness prevented the settlement of major micro- and macro-fouling species (Scardino et al., 2009). On another hand, engineered nanomaterials have found extensive application in electronics, pharmaceuticals, renewable energies, and antifouling coatings applications, amongst others (Bandala and Berli, 2019). Hybrid nanocomposites of organic–inorganic materials give an opportunity to combine the characteristics of different materials generating opportunities to prevent biofouling (Wassel et al., 2020; Nathanael and Kumar, 2021). Metal and metal oxide nanoparticles, such as silver (Ag), titanium dioxide (TiO2) and zinc oxide (ZnO), possess antifouling properties (Kim et al., 2003; Chapman et al., 2010; Das et al., 2013; Song et al., 2020). Additionally, carbon nanotubes (CNTs) incorporated in coatings prevent macrofouling by inhibition of settlement and adhesion of larvae (Carl et al., 2012; Kim et al., 2014; Yang et al., 2016a; Kim et al., 2016; Aghajani and Esmaeili, 2021).
Nanostructured metal oxide, like ZnO and TiO2, is capable of absorbing visible and ultraviolet light and inhibiting microbial growth due to the photocatalytic process leading to redox reactions (Baruah et al., 2012; Danwittayakul et al., 2018; Spirescu et al., 2021). The generated reactive oxygen species (ROS) like peroxides, superoxides, and hydroxyl radicals that form during photocatalysis, prevent the growth of microorganisms on the surfaces (Sathe et al., 2017; Ganguly et al., 2018). Metal oxide nanostructures can have advantages in antifouling application than other types of nanocoatings due to the fact that ROS are short-lived and have localized surface toxicity (Bora et al., 2017). Antifouling and anti-algal activities of coatings containing ZnO nanorods and TiO2 nanoparticles have been recently studied (Al-Fori et al., 2014; Sathe et al., 2016b; Yemmireddy and Hung, 2017; Zhu et al., 2018).
This review is aimed at providing an overview of recent advances in formulation and antifouling activity of nanocoatings containing active ingredients of polymers, inorganic nanoparticles, or organic-inorganic hybrid materials.
Nanotechnology-Based Antifouling Solutions
Various antifouling strategies, namely fouling-resistant, fouling-release and fouling-degrading, have been explored in preparation of antifouling coatings (Figure 1) to cater for treatment of different types of biofoulings. For instance, fouling-resistant coatings with a highly hydrated surface provide a physical and free-energy barrier to prevents adhesion of foulants such as proteins, algae, or bacteria (Thérien-Aubin et al., 2011). In contrast, the fouling-release coatings allow weak adhesion of foulant, which can be removed afterwards by external force like water flushing (Damodaran and Murthy, 2016). These two coating approaches introduce hydrophilic and hydrogen bond-forming characteristics to the surface, where the tightly bound water molecules form a barrier layer preventing adsorption (Chen et al., 2010). The third type, fouling-degrading coatings, incorporates antimicrobial moieties in the coating to degrades the settled bacteria or microorganisms via oxidation or other bactericidal functionalities (Sakala and Reches, 2018).
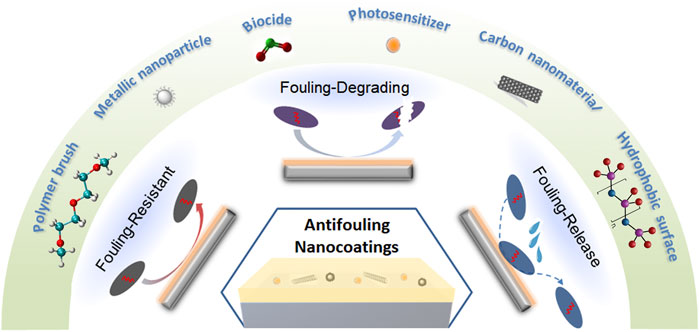
FIGURE 1. Schematic illustration of the principal strategies and active ingredients in coatings for antifouling.
Antifouling Biocides
Antifouling biocides are chemicals that can destroy or render harmless of the microorganisms responsible for biofouling. The previously widely used tri-substituted organostannic compounds, such as tributyltin (TBT) and triphenyltin (TPT), in antifouling paints on ships are no longer permitted for use as biocides within the EU since July 2010 (EU Commission Regulation, 2010), due to the risk of leaching into the aquatic environment and toxicity to aquatic organisms through endocrine disruptive effects. On the other hand, copper compounds are used in antifouling paints for centuries to control hard fouling, such as barnacles, mussels and tube worms, attributed to their effective, available and relatively inexpensive characters compared to other biocides. The most applied copper compounds are cuprous oxide, copper thiocyanate and copper flake, which are used in many different formulations of antifouling paint. Similarly, copper leachate from antifouling paints has been found to impact water quality. Consequently, California’s department of pesticide regulation in the US has established a maximum allowable copper leach rate of 9.5 μg/cm2/day for copper-based antifouling products intended for use on recreational vessels, which became effective on July 1, 2018 (California Notice, 2018). And the state of Washington (US) has regulated to cease the use of copper-based paint for recreational boats by 2021 due to its toxicity to juvenile salmon. European Commission urges the member states to find and invest in alternatives. Apart from copper, organic booster biocides, such as chlorothalonil, dichlofluanid, chlorine dioxide DCOIT, Diuron, Irgarol 1051, TCMS pyridine, zinc pyrithione and Zineb (Guardiola et al., 2012; Venkatnarayanan et al., 2017), are introduced as alternatives to the restricted organotin compounds in antifouling products. However, some of the booster biocides appear to be persistent and have a continuous leaching character from antifouling paints, potentially posing a significant threat to the aquatic environment with risks of accumulation in aquatic products and development of antibiotic resistance in bacteria. Therefore, eco-friendly and non-biocide-release coatings for marine biofouling prevention are urgently needed.
Polymer-Based Antifouling Coatings
Surface PEGylation, i.e., grafting polyethylene glycol (PEG) to surfaces to develop linear PEG brushes, has long been a standard way to resist the adsorption of proteins (Li et al., 2019). The efficient repulsion of PEG brushes to foulants roots from the extensive hydration layer, the rapid conformational changes and steric repulsion (Hui et al., 2017). However, PEG has been found with short-term stability in biochemical environments due to readily subjected to oxidative degradation and enzymatic cleavage, that results in the formation of aldehyde-terminated chains and a subsequent reaction with amino-contained proteins (Chen et al., 2010). Moreover, PEG coatings used to swell in aqueous environments because of their highly hydrated nature, which deteriorates their mechanical strength, as well the chemically different substrates remain difficult for PEG to graft (Xie et al., 2019). Hence, studies on alternatives for PEG, such as polyoxazolines (Divandari et al., 2017), polyglycerol dendrons (Wyszogrodzka and Haag, 2009), polysaccharides (Rendueles et al., 2013), polypeptoids (Lin et al., 2011), polyacrylamide (Liu et al., 2012) and zwitterionic polymers (Knowles et al., 2017), has been conducted and these alternatives show similar or better fouling resistance. Besides linear polymer brushes, cyclic- and loop-structured polymer brushes based on poly(2-alkyl-2-oxazoline)s, such as poly(2-methyl-2-oxazoline) (PMOXA) and poly(2-ethyl-2-oxazoline) (PEOXA), have been studied extensively they can generate denser brushes and thus show better protein-resistant properties (Wang et al., 2019). However, it is still costly and complex to obtain the highly dense cyclic polymers brushes in large quantities for large-scale application.
Poly(dimethylsiloxane) (PDMS) coatings represent another type of antifouling mechanism with fouling-release character, attributed to the chemical inertness and low surface energy of PDMS elastomer (Dafforn et al., 2011). In addition, additive of non-reactive silicone oils provides lubricity to the coating surface and therefore considerably reduces fouling by deceiving the mechanosensing ability of fouling organisms, especially for mussels, deterring secretion of adhesive threads, and decreasing the molecular work of adhesion (Amini et al., 2017). To further increase the hydrophobicity of coating surface, a layer of polystyrene (PS) microspheres could be assembled on top of PDMS coating (see Figure 2) (Mo et al., 2021). As shown in Figure 2 (left part), fouling organisms can adhere to the surface of conventional silicone sample without PS microspheres, where it has more attachment points, and the fouling organisms usually show greater bonding strength. In contrast, on the surface of PS-sphere-coated PDMS (diffused with phenylmethylsilicone oil-PSO, right part in Figure 2), there are fewer attachment points and weakened bonding strength between fouling organism and coatings. Therefore, benthic diatoms are easily removed under the shearing force of seawater, so as to achieve the purpose of antifouling.
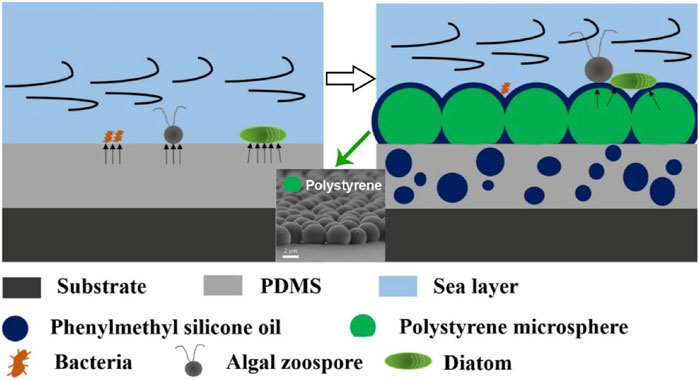
FIGURE 2. Synergistic defense of PSO (phenylmethylsilicone oil)/PDMS (polydimethylsiloxane)-PS (polystyrene) coating against biofoulings (Modified from Ref. Mo et al., 2021).
Hydrogel Coatings for Antifouling
Hydrogel is a polymer network which holds a high content of water (typically 80–90%). As aforementioned, many hydrophilic polymers like PEG and zwitterionic polymers can prevent attachment of various proteins, polysaccharides, and many microorganisms. However, hydrophilic polymers typically have difficulty in making surface coating due to the low adhesion strength to various substrates. Out of the approaches developed to overcome this problem, hydrogel coatings are of especial interest because of their outstanding characters on both antifouling abilities due to superhydrophilicity and fouling-release properties due to the relatively low Young’s modulus to destabilize the attachment of marine organisms (Brady and Singer, 2000). To render their practical application in marine antifouling, improvement of the adhesion strength of hydrogels has been investigated through surface covalent cross-linking (Gao et al., 2020) or by decoupling polymerization from crosslinking and interlinking, hydrogel paints were prepared and applied on various substrates by various operations (brush, cast, dip, spin, or spray) (Yao et al., 2019; Yang et al., 2021). The advantages of hydrogel paint lie in the ease of application, like a common paint, without involving mold, UV light, or oxygen-free environment. Secondly, the hydrogels are commonly made by free-radical polymerization and are readily copolymerized with silanes or hydroxyl-contained molecules. While, the substrate materials, such as glasses, metals, ceramics, or organic elastomers and plastics, have hydroxyl groups on their clean surfaces or can be easily acquired through surface plasma treatment, which results in high adhesion strength. Thirdly, dried hydrogel paint can be ground into powders and redissolved in water for application, and the extended shelf life greatly amplifies the advantage of hydrogel paint.
Inorganic Ingredient-Based Coatings for Prevention of Biofouling
Antifouling marine paints containing nanomaterials have been reported to offer super hydrophobicity, high durability, water repellent, anti-sticking, microbial resistance, elastomeric, and anti-corrosive properties (Yebra et al., 2006; Callow and Callow, 2011), which are novel solutions for the sustainable growth of maritime industries. Nanoparticles can be used efficiently in formulations of antifouling coatings resulting in improved properties (Lakhotia et al., 2018). Silver nanoparticles (AgNPs) (Kumar et al., 2017; Kumari et al., 2017), carbon nanostructures like carbon nanotube (CNT) (Dasgupta et al., 2017) and graphene (Bystrov et al., 2017) are commonly used for antifouling prevention. Additionally, several metal oxides semiconductors like titanium dioxide (TiO2) (Kim et al., 2016), and zinc oxide (ZnO) (Al-Naamani et al., 2017; Kumar et al., 2019a) nanoparticles have been used for nanocomposite formulations showing antifouling activities. The analysis of literature suggests that most of antifouling studies were dealing with metal oxide nanostructures, such as ZnO and TiO2 (Figure 3). Less attention is given to carbon nanostructures such as CNT. Below we reviewed the antifouling activity of nanosilver, carbon nanostructures and nanostructured metal oxides as well as composite nanocoatings.
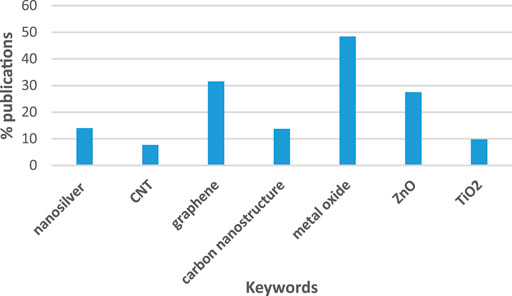
FIGURE 3. Percent of publications dealing with nanostructure-based antifouling solutions. The results include publications from January 1, 2000 to July 19, 2020 performed by SCOPUS search. The search included key words “nanotechnology” and “biofouling” plus one of the key words.
Silver Nanoparticles
Silver-based coatings or silver deposited surfaces are well-known to provide bacteriostatic/bactericidal, fungistatic/fungicidal and algistatic/algicidal properties to surfaces (Devasconcellos et al., 2012). Silver is known to have inhibitory effects against different kinds of microorganisms (Goswami et al., 2015; Kumari et al., 2016; Naskar et al., 2018). Therefore, it finds applications in medical and consumer products, and in environmental applications (Kumar et al., 2014; Rasheed et al., 2017). Nano-sized silver, i.e., silver nanoparticles, have found increasing application as an antimicrobial agent due to high surface to volume ratio and increased surface reactivity (Kumari et al., 2016). Several studies about the antimicrobial activity of silver nanoparticles and their action mechanism have been reported (Kumar et al., 2014; Durán et al., 2016). Silver formulations containing silver ions or silver nanoparticles are generally considered biologically benign and safer than any other heavy metal-based formulations (Rasheed et al., 2017). The bacteriostatic/bactericidal properties of nanosilver can also be useful in antifouling applications against microfouling (Devasconcellos et al., 2012; Li et al., 2013). Only a few studies investigated the effect of nanosilver on eukaryotes or macrofouling organisms. For example, it has been shown that a silver nanoparticle coating prevents the adhesion of marine and freshwater algae (Ren et al., 2014). In another study, nanosilver prevented mussel settlement through modification of the structure of biofilms (Yang et al., 2016b).
The mechanism of the antifouling properties of silver nanoparticles is not yet clear and debatable. One of the most accepted mechanisms is based on the ability of Ag+ ions to anchor to cell walls. It is shown that Ag+ ions penetrate into the bacterial cell and interacts with thiol groups of most of the vital enzymes, leading to deactivation of the enzyme, which ultimately stops bacterial growth leading to the death of the bacterial cell (Kim et al., 2007; Kumar et al., 2014; Pareek et al., 2018). The study with eukaryotes suggested that contact killing of the cells by Ag+ ions is the main antifouling mechanism (Ren et al., 2014). The formation of free radicals by AgNPs is another proposed mechanism. Electron spin resonance (ESR) spectroscopy studies suggested that free radicals generated from AgNPs damage cell membranes when they come in contact with bacteria, resulting in membrane rupture and ultimately cell death (Danilczuk et al., 2006; Kailasa et al., 2019). However, antimicrobial activity of AgNPs depends on several factors including physico-chemical parameters such as size (Ginjupalli et al., 2018; Kumar et al., 2019b), morphology (El-Zahry et al., 2015), crystallinity (Kumar and Münstedt, 2005), surface coatings of nanoparticles (Brobbey et al., 2019), and microbial species in the environment (Pareek et al., 2018).
Graphene and Carbon Nanotube
Graphene is an allotrope of a single layer of carbon atoms arranged in a sp2-bonded hexagonal lattice (Figure 4). They are naturally found as the building blocks of graphite. Graphene materials show high specific surface area, electron conductivity and thermal stability that make it attractive for several environmental applications like photocatalysis, energy production and storage (Sun et al., 2015; Ozer et al., 2017; Xu et al., 2018). Carbon nanotubes (CNTs) represent a hollow, concentric cylindrical structure, typically with a diameter of a few nanometers and a length varying from a few nanometers to several microns (100 μm) up to a few millimeters (4 mm) (Venkataraman et al., 2019). Nanotubes are primarily classified into three categories; single-walled carbon nanotubes (SWCNTs), double-wall carbon nanotubes (DWCNTs), and multi-walled carbon nanotubes (MWCNTs). The environmental application of CNT’s mainly involves the fabrication of nanocomposite materials with biocidal properties and fouling release activities (Upadhyayula and Gadhamshetty, 2010; Dasgupta et al., 2017).
Carbon Nanotube
Graphene
CNTs and graphene exhibit good antimicrobial activity towards both Gram-positive and Gram-negative bacteria as well as bacterial spores (Lukowiak et al., 2016; Al-Jumaili et al., 2017; Sun et al., 2020). CNTs, especially SWCNTs were shown to have significantly greater antibacterial activity than MWNTs, probably due to their smaller sizes, enabling membrane perturbation (Figure 5) (Kang et al., 2008). Additionally, CNTs can impact the recruitment of macro-fouling organisms (Beigbeder et al., 2008). Nano-sized carbon black more effectively prevented settlement of the barnacle Amphibalanus amphitrite larvae compare to single-layer graphene oxide (Mesarič et al., 2013). Just 0.5% weight percent of CNT affected the composition of biofilms by increasing the abundance of Proteobacteria and decreasing the abundance of Bacteroidetes, which in turn decreased the settlement of Mytilus coruscus (Yang et al., 2016a).
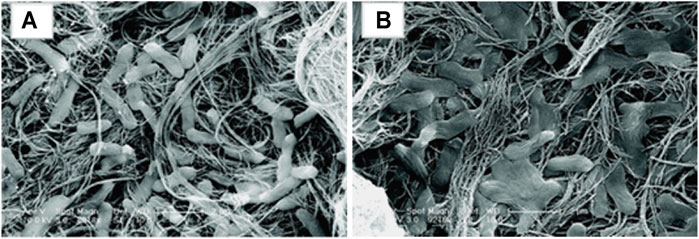
FIGURE 5. SEM micrographs of E. coli exposed to CNTs for 60 min; (A) MWCNTs (B) SWCNTs. Reproduced with permission from (Kang et al., 2008).
One of the first CNT-based antifouling coatings was developed in 2008 (Beigbeder et al., 2008). It was based on the incorporation of synthetic multi-wall CNTs in silicone coatings. Nowadays, CNT-based coatings are actively used as antifouling and anti-corrosion marine coatings in the construction and oil and gas industries (Dustebek et al., 2016). CNTs are generally loaded into a polymeric matrix for application in protective coatings. Epoxy resins have been mostly used in reinforcement with different types of carbon nanotubes (single-wall, double-wall and multi-wall) (Jin F.-L. et al., 2015; Deng et al., 2015; Shen et al., 2016). CNTs act as an efficient adsorbent due to their large surface area, π-bond electrons on the surface, providing more chemically active sites on the nanotubes, and hollow and layered structures. CNTs have high tensile strength and Young’s modulus, and in comparison to steel, CNTs have hundreds and tens of times the higher tensile strength and Young’s modulus (Walters et al., 1999). The reinforcement of the paint matrix with CNTs improves mechanical properties in the coatings/paints. Dustebek et al. (Dustebek et al., 2016) investigated the effect of CNTs on the mechanical strength of a self-polishing antifouling resin-based paint and found that the increase in the amount of MWCNT leads to an improvement in the mechanical strength of self-polishing antifouling paints. Antifouling paints with 0.5% (w/w) and 0.7% (w/w) of MWCNTs showed a significant improvement in impact resistance (Dustebek et al., 2016). This study was further supported by another research that evaluated the effect of reinforcement of MWCNT and graphene oxide (GO) on mechanical properties of poly-dimethyl siloxane (PDMS) marine coatings (Cavas et al., 2017). Incorporation of MWCNTs in composites increased both tensile strength and percent of elongation of PDMS marine coatings. These studies indicate that CNT and GO beside of antifouling properties can improve the mechanical properties of nanocomposite coatings.
Numerous mechanisms of antifouling action of CNTs and GO have been proposed, but the actual mechanism is still not clear. A study suggested that different antifouling mechanisms can be involved (Mesarič et al., 2013). First, CNTs and GO are toxic to microbes and larvae of macrofouling organisms. The toxic effect of CNTs is widely known (Francis and Devasena, 2018). CNTs can increase cell apoptosis, oxidative stress, and inflammation. Second, CNTs and GO can inhibit larval settlement by a non-toxic way simply interfering with the attachment and adhesion of cyprid larvae (Mesarič et al., 2013).
The physical size (in particular the length), diameter, surface area, concentration, treatment time, etc., play a role in the antifouling activity of CNTs. Yang et al. (2010) investigated the effect of length of SWCNTs’ on antimicrobial activity to Salmonella cells using three different lengths of SWCNTs (<1 μm, 1–5 μm, and ca.5 μm) (Yang et al., 2010). Longer SWCNTs were reported to exhibit stronger antimicrobial activity by aggregating bacterial cells more effectively, whereas shorter SWCNTs were reported to aggregate between themselves without involving many bacterial cells (Figure 6). The tube diameter also plays an important task in the inactivation of microorganisms. Smaller diameters CNTs (<10 nm) can damage cell membranes by interaction with the cell-surface, while large diameter CNTs (15–30 nm) mostly interact by their sidewalls with the bacteria (Upadhyayula and Gadhamshetty, 2010).
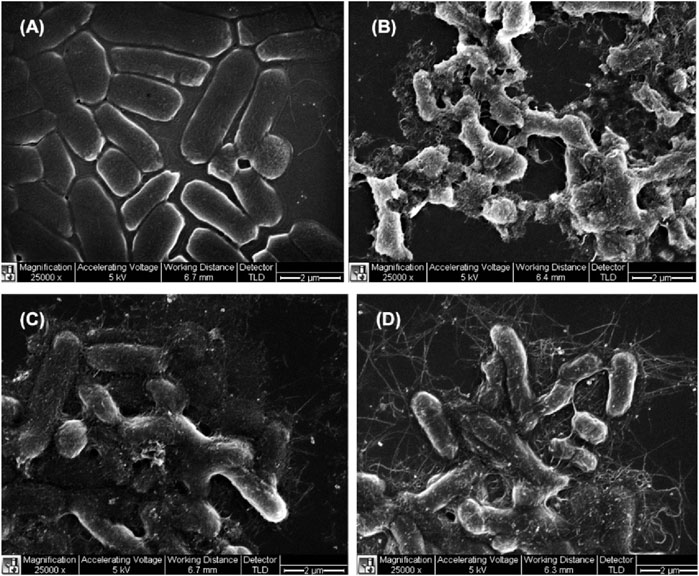
FIGURE 6. SEM images of Salmonella (A) without SWCNTs, and treated with SWCNTs of (B) < 1 μm (C) 1–5 μm, and (D) > 5 μm. Reproduced with permission from (Yang et al., 2010).
Metal Oxides and Metal/Metal Oxide Hybrid Nanostructure
Metal oxide nanoparticles (NPs), such as ZnO, TiO2, SnO2, are highly photoactive and used for antimicrobial, self-cleaning, self-healing, anti-corrosion and anti-biofouling applications. Table 1 summarizes the antifouling effects of various photocatalytic metal oxide nanostructures on aquatic prokaryotes (bacteria) and eukaryotes (microalgae, barnacles, bryozoans, etc.). In general, investigators studied the antifouling effect of metal oxide nanoparticles, nanowires, nanorods, and hybrid nanostructures. ZnO, TiO2 and hybrid metal-metal oxide, as well as hybrid carbon-metal oxides nanostructures were the most commonly used systems for the inhibition of biofouling (Table 1). ZnO nanoparticles have attracted more attention compared to other metal oxides due to their low cost and ready availability (Naskar et al., 2018; Wallenhorst et al., 2018). As per the FDA (Food and Drug Administration, United States) guidelines, ZnO is listed as a safe material for various uses. ZnO NPs are used in the food processing and packaging, as well as in the agriculture sector because of their biocompatibility, low toxicity and antimicrobial properties (Garcia et al., 2018). Photocatalysis of various toxic organic dyes and inorganic pollutants in industrial wastewater has been performed using ZnO and TiO2 semiconductor oxides under light irradiation. TiO2 is widely used in self-cleaning coatings and paints but their application is limited to outdoors, as they require activation by UV light (Xu et al., 2017; Adachi et al., 2018). The most common bacteria used for testing include Gram-positive (B. subtilis, Micrococcus) and Gram-negative (E. coli, P. aeruginosa) pathogens (Table 1). In opposite, the most common eukaryotes used in metal oxide nanostructures studies are environmental species of microalgae and invertebrate larvae.
Silver nanoparticles is a mostly used antimicrobial agent that synthesized with environment-friendly methods and can be non-toxic for mammals. Titanium oxide nanotubes have been used to improve the functional properties of silver nanoparticles because of their superior specific surface area both on inner and outer surfaces of the tubular structure. Yee et al., 2017 proposed an innovative 2-steps hydrothermal synthesis of a silver–titania nanotube (Ag/TNT) composite that showed nanotubular TiO2 structures with Ag nanoparticles uniformly dispersed throughout the nanomaterial (Yee et al., 2017). The inhibitory properties of the composite material against biofilm were studied and the result demonstrated the Ag/TNT reduced the biofilm formation of marine bacterium Halomonas pacifica by 98% compared to bare titania nanotubes. Additionally, Ag/TNT also showed growth inhibition of marine microalgae Dunaliella tertiolecta and Isochrysis sp (Yee et al., 2017). A ternary nanocomposite (PDMS/GO-Al2O3 NRs) superhydrophobic coatings were also developed from PDMS, and graphene oxide anchored with alumina nanorods (GO-Al2O3 NRs) hybrid sheet via solution-casting method (Selim et al., 2018; He et al., 2021). The results showed that the well-dispersed GO-γ-Al2O3 NRs hybrid sheets increased the contact angle (151°), decreased the surface free energy (13.25), and improve micro-nano roughness on the surface, and thus the developed nanocomposites may have promising antifouling applications in the shipping industry (Selim et al., 2018). In a recent study, silicone/graphene-based two novel superhydrophobic nanocomposite were developed, and the results shows PDMS/GO-γ-AlOOH nanorod composite had better antibacterial activity than PDMS/RGO nanocomposite against different bacterial strains might be due high surface area and stabilizing effects of the GO-γ-AlOOH hybrid nanofillers. The GO-γ-AlOOH (3 wt%) nanostructured coating had profound superhydrophobic antifouling properties due to their homogeneity, and high WCA of 151° and a rough surface (Selim et al., 2022).
Aluminum oxides are inexpensive, non-toxic, and one of the most stable inorganic materials. Boehmite is an aluminum oxide hydroxide (γ-AlOOH) particle that contain hydroxyl groups on its surface. Boehmite nanoparticle has the orthorhombic structure, in which the boehmite nanoparticles’ surface are covered with OH groups. Boehmite nanoparticles can improve hydrophilicity and surface properties of the membrane due to presence of extra hydroxyl groups on their surface, and thus reduce fouling of the resulted membranes (Vatanpour et al., 2012; Farjami et al., 2021). Among the alumina compounds, boehmite has highest hydrated surface and hydrophilicity. The effects of two different solvents, dimethylacetamide (DMAc) and N-methyl-2-pyrrolidone (NMP) were evaluated on performance of the pristine and EPVC/nano-boehmite nanocomposite membranes, and the results showed that the membranes prepared in NMP solvent using boehmite nanoparticles had higher anti-fouling and fouling resistance properties (Farjami et al., 2021).
Antifouling properties and toxicity of metal oxide nanomaterials depend on the biological species, with some species being more sensitive than others (Dobretsov et al., 2020). Additionally, physico-chemical properties of nanomaterials such as particle size, shape, surface charges, and surface groups affect their toxicity. The smaller size of nanomaterials can easily enter through cell membranes and other barriers of the living organisms. The amount of uptake of nanomaterials decreases with the increased particle size (Hoshyar et al., 2016). The basic principle of metal oxide based photocatalytic disinfection however involves the generation of highly reactive intermediate species, and these species lead to the photocatalytic destruction of adsorbed entities (organics, micro-organisms, and macro-organisms).
Different surface charges and groups on nanostructures exhibit different surface oxidation reactivity resulting in the generation of ROS at varying levels. The use of TiO2 or ZnO is limited to UV light irradiation due to their wide bandgap and the high recombination rate of photo-generated charges. Thus, efforts have been focused on achieving high photocatalytic efficiency with these materials especially under visible light. In order to render ZnO, TiO2, SnO2 and other semiconductor materials suitable for visible light photocatalysis, several techniques have been developed, such as surface modification of semiconductor materials, creation of oxygen vacancies, doping of metal/non-metal atoms, depositing noble metals, and coupling with other semiconductors or carbon materials (Rehman et al., 2009; Youssef et al., 2018). Doping with metal or nonmetal makes TiO2 and ZnO to absorb visible light. Doping metal or non-metal atoms can generate impurity levels in the bandgap of semiconductors altering the photoelectric properties to cover its spectral absorbance in the visible region (Bora et al., 2017; Raizada et al., 2019).
The antifouling mechanisms of nanostructured metal oxides are based on the release of metal ions, such as Ag+ and Zn2+ ions from respective nanomaterials as well as the formation of ROS (Figure 7). Exposure of metal oxides with UV/visible light irradiation leads to the transfer of electrons from the valence band to the conduction band generating electron-hole pairs. The photo-generated exciton if separated can efficiently reduce and/or oxidize a compound adsorbed on their surface by generating •OH radicals, and/or by the generation of O2− anions (Figure 7). Both the radicals and anions can react with pollutants transform them to lesser harmful byproducts or degrade them (Allahverdiyev et al., 2011; Carré et al., 2014; Khan et al., 2015). Similarly, ROS and metal anions prevent fouling by micro- and macro-organisms (Al-Fori et al., 2014). Production of ROS at low dosage levels resulting in harsh oxidative stress that causes the damage of genetic material such as DNA and RNA, oxidation of lipid, alteration of protein, inhibition of enzymes, etc. (Figure 7), while prokaryotic and eukaryotic cell death occurs at higher concentrations of ROS (Pan et al., 2010; Allahverdiyev et al., 2011; Carré et al., 2014). The generated ROS are short-lived and only affect organisms that come in direct contact with them (Bora et al., 2017).
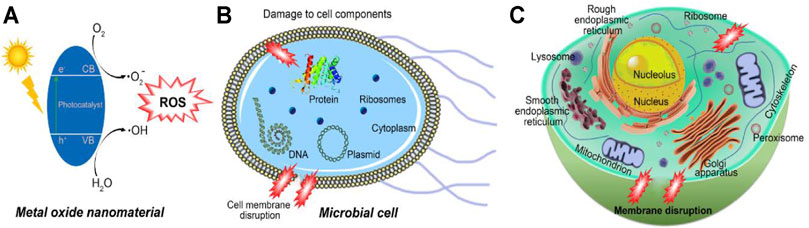
FIGURE 7. Schematic representation of possible antifouling mechanisms of metal oxide nanomaterials (A) generation of ROS; effect of ROS on (B) a prokaryotic cell, and (C) a eukaryotic cell.
Metal oxide nanorods and nanowires based photocatalytic coatings have attracted recent attention owing to its ease of controlled fabrication processes (Wei et al., 2005; Inderan et al., 2015; Jin et al., 2015a; Jin et al., 2015b; Wang et al., 2018). ZnO nanorod-based photocatalytic coatings have been found effective against micro- and macro-fouling on a glass substrate and fishing net substrates in the laboratory (Al-Fori et al., 2014; Spirescu et al., 2021) and field experiments, respectively (Sathe et al., 2017) (Figure 8). ZnO nanorods have increased stability, reduced loss of nanoparticles compared to ZnO nanoparticles and can be fabricated into various types of support materials (Al-Fori et al., 2014). Additionally, ZnO nanorods have lower toxicity compared to ZnO nanoparticles, which was confirmed by experiments with bacteria, microalgae, larvae of invertebrates and adult mussels (Falfushynska et al., 2019; Dobretsov et al., 2020).
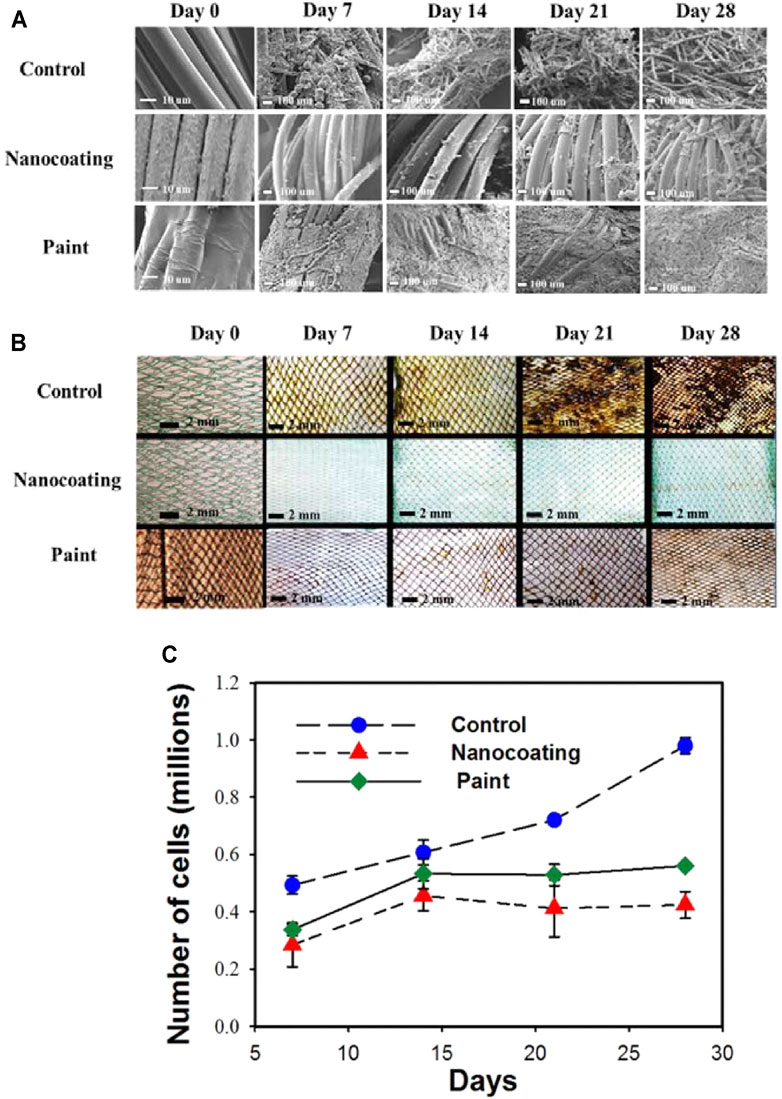
FIGURE 8. Total microbial profusion and surface coverage of the control, ZnO nanocoating and copper-based antifouling paint coated net substrates (A) scanning electron micrographs (B) optical micrographs, and (C) population count. Reproduced with permission from (Sathe et al., 2017).
Inorganic-Polymer Nanocomposite Coatings for Prevention of Marine Biofouling
Apart from purely polymer and organic molecule mixed coatings, organic/inorganic hybrid composites prepared by blending nano-sized inorganic fillers in polymer matrix have been investigated on the fouling-release and fouling-degrading performance. And the nanomaterial-based antifouling coatings have been proved as one of the most promising coatings for biofouling prevention, amongst others (Tobaldi et al., 2017). While metal oxide nanostructures are capable of preventing of micro- and macro-fouling, their effectiveness is low due to their solubility. Additionally, nanoparticles can be lost and cause harmful effects on the environment. In order to enhance stability, reduce environmental impact, and enhance the antifouling effect, metal oxide nanostructures are usually attached to support substrata or incorporated into a stable matrix of coating. For example, ZnO nanorods attached to glass substrata was effective against the marine bacterium Acinetobacter sp., the marine microalga Tetraselmis sp. and the bryozoan Bugula neritina under the artificial sunlight (Al-Fori et al., 2014).
Various nanostructure-based nanocomposite antifouling coatings reported in the literature are summarized in Table 2. ZnO and TiO2 nanoparticles are most commonly used, while Cu2O, CuO and Al2O3 are also incorporated into nanocomposite coatings (Table 2). PDMS has certain advantages as a coating. It has a hydrophobic surface and, thus, used for fouling release antifouling applications (Zhang and Chiao, 2015). Biopolymers, such as chitosan, have been used to develop environmentally friendly nanocomposite antifouling coatings (Carteau et al., 2014; Krishnan et al., 2015; Pounraj et al., 2018). Chitosan and ZnO or TiO2 based nanocomposite coatings have been reported as effective against several marine microorganisms including biofilm-forming bacterium Vibrio fischeri (Baniamerian et al., 2018), Pseudoalteromonas nigrifaciens, and diatoms Navicula sp. (Al-Naamani et al., 2017). While metal oxides have photocatalytic properties that prevent biofouling at the light, the addition of chitosan enhances antifouling properties in dark conditions. Most of the studies with ZnO nanoparticles or nanorods nanocomposite antifouling coatings were conducted in the laboratory conditions (Table 2). Since in the field there are many different species of biofouling organisms, as well as coatings are exposed to different physical and biological factors, it is important to test coatings the field conditions in order to prove their antifouling activity.
Concluding Remarks and Perspectives
Biofouling is a major problem for maritime industries constantly affecting marine installations. Traditional antifouling methods are mainly based on the applications of biocidal agents that detract or kill organisms close to vicinity, which is apparently not environment friendly. This review summarized polymeric and polymer-inorganic composite alternatives to toxic antifouling paints, that show marine antifouling effects via fouling resistant, release, or degrading mechanisms. For polymer and hydrogel antifouling coatings, surface chemistry and coating techniques often determine the antifouling performance. For inorganic-polymer nanocomposite, the intrinsic characteristics of inorganic nanoparticles is responsible for fouling degradation. In photocatalytic nanostructure-based antifouling applications, ROS generated by photocatalytic nanostructures in presence of light can kill /inactivate micro- and macro-organisms. Compared to toxic biocides, the generated ROS are short-lived and only affect organisms that are in close proximity of the coatings. Thus, photocatalytic nanocoatings are less toxic to aquatic organisms compared to traditional antifouling coatings. Metal oxide nanostructure-based coatings are being considered as one of the promising environment-friendly antifouling technologies strategies that is suitable for marine applications. In contrast, only a few studies that reported inhibition of macro-fouling in the field conditions have been conducted. This could be due to the deteriorated efficiency of nanocomposites antifouling coatings in the field or the difficulties in scaling up such coatings.
An ideal antifouling coating is expected to be durable, reliable, easily applicable, cost-effective, eco-friendly, and substrate-independent. It remains very challenging to realize all the desirable requirements simultaneously. No single chemistry/strategy has yet been identified as the universal antifouling method. Instead, one multifunctional coating with synergetic strengths by combining several antifouling strategies is probably the correct way reaching the goal of a successful antifouling coating. With the large amount of high-quality and sophisticated research that is being performed, zwitterionic polymers attract much attention in the development of next-generation antifouling materials, due to the simplicity of synthesis, ease of applicability, abundancy of raw materials and availability of functional groups. At the same time, inorganic nanomaterials possess great advantage in preparation and application for antifouling. Therefore, the inorganic-polymer composite coatings represent another potentially commercial solution, especially considering their effectiveness and low cost. However, it is still necessary to develop new polymers or composite to improve the antifouling performance, especially focusing on long-term durability when used in both static and dynamic environments. Apart from the efficiency enhancement, coating techniques also need to be developed in order to successfully translate these coatings toward large-scale applications to find their way outside the lab. Hence, the frequently used chemical grafting approaches need to be simplified, in order to cope with the physisorption techniques for coating, often by layer-by-layer or spray-painting. Combining simple coating techniques with a self-replenishing antifouling character to enhance antifouling durability and crosslinkers or catch-bonds to enhance mechanical stability, could result in technologically mature antifouling coatings with large-scale applicability in real maritime environmental conditions and on different materials such as vessels, fishing nets, and pipes. Finally, the impacts of environmental toxicity of such coatings should be more thoroughly investigated.
Author Contributions
SK, and JD contributed to conception and design of the study. SD and FY organized the database. SK and FY wrote the first draft of the manuscript. All authors contributed to manuscript revision, read, and approved the submitted version.
Conflict of Interest
The authors declare that the research was conducted in the absence of any commercial or financial relationships that could be construed as a potential conflict of interest.
Publisher’s Note
All claims expressed in this article are solely those of the authors and do not necessarily represent those of their affiliated organizations, or those of the publisher, the editors and the reviewers. Any product that may be evaluated in this article, or claim that may be made by its manufacturer, is not guaranteed or endorsed by the publisher.
Acknowledgments
SK acknowledged Department of Biotechnology (DBT), Government of India for financial support (sanction letter vide no. BT/20/NE/2011) through “Biotechnology Overseas Associateship Award for NER Scientists”.
References
Adachi, T., Latthe, S. S., Gosavi, S. W., Roy, N., Suzuki, N., Ikari, H., et al. (2018). Photocatalytic, Superhydrophilic, Self-Cleaning TiO2 Coating on Cheap, Light-Weight, Flexible Polycarbonate Substrates. Appl. Surf. Sci. 458, 917–923. doi:10.1016/j.apsusc.2018.07.172
Ademola Bode-Aluko, C., Pereao, O., Kyaw, H. H., Al-Naamani, L., Al-Abri, M. Z., Tay Zar Myint, M., et al. (2021). Photocatalytic and Antifouling Properties of Electrospun TiO2 Polyacrylonitrile Composite Nanofibers under Visible Light. Mater. Sci. Eng. B 264, 114913. doi:10.1016/j.mseb.2020.114913
Aghajani, M., and Esmaeili, F. (2021). Anti-biofouling Assembly Strategies for Protein & Cell Repellent Surfaces: a Mini-Review. J. Biomater. Sci. Polym. Edition 32, 1770–1789. doi:10.1080/09205063.2021.1932357
Al-Belushi, M. A., Myint, M. T. Z., Kyaw, H. H., Al-Naamani, L., Al-Mamari, R., Al-Abri, M., et al. (2020). ZnO Nanorod-Chitosan Composite Coatings with Enhanced Antifouling Properties. Int. J. Biol. Macromolecules 162, 1743–1751. doi:10.1016/j.ijbiomac.2020.08.096
Al-Fori, M., Dobretsov, S., Myint, M. T. Z., and Dutta, J. (2014). Antifouling Properties of Zinc Oxide Nanorod Coatings. Biofouling 30, 871–882. doi:10.1080/08927014.2014.942297
Al-Hinai, M. H., Sathe, P., Al-Abri, M. Z., Dobretsov, S., Al-Hinai, A. T., and Dutta, J. (2017). Antimicrobial Activity Enhancement of Poly(ether Sulfone) Membranes by In Situ Growth of ZnO Nanorods. ACS Omega 2, 3157–3167. doi:10.1021/acsomega.7b00314
Al-Jumaili, A., Alancherry, S., Bazaka, K., and Jacob, M. (2017). Review on the Antimicrobial Properties of Carbon Nanostructures. Materials 10, 1066. doi:10.3390/ma10091066
Al-Naamani, L., Dobretsov, S., Dutta, J., and Burgess, J. G. (2017). Chitosan-zinc Oxide Nanocomposite Coatings for the Prevention of marine Biofouling. Chemosphere 168, 408–417. doi:10.1016/j.chemosphere.2016.10.033
Allahverdiyev, A. M., Abamor, E. S., Bagirova, M., and Rafailovich, M. (2011). Antimicrobial Effects of TiO2 and Ag2O Nanoparticles against Drug-Resistant Bacteria and Leishmania Parasites. Future Microbiol. 6, 933–940. doi:10.2217/fmb.11.78
Alpaslan, E., Geilich, B. M., Yazici, H., and Webster, T. J. (2017). pH-Controlled Cerium Oxide Nanoparticle Inhibition of Both Gram-Positive and Gram-Negative Bacteria Growth. Sci. Rep. 7, 45859. doi:10.1038/srep45859
Amini, S., Kolle, S., Petrone, L., Ahanotu, O., Sunny, S., Sutanto, C. N., et al. (2017). Preventing Mussel Adhesion Using Lubricant-Infused Materials. Science 357, 668–673. doi:10.1126/science.aai8977
Aoki, Y., Kunitake, T., and Nakao, A. (2005). Sol−Gel Fabrication of Dielectric HfO2 Nano-Films; Formation of Uniform, Void-free Layers and Their Superior Electrical Properties. Chem. Mater. 17, 450–458. doi:10.1021/cm048971r
Balakrishnan, A., Jena, G., Pongachira George, R., and Philip, J. (2021). Polydimethylsiloxane-graphene Oxide Nanocomposite Coatings with Improved Anti-corrosion and Anti-biofouling Properties. Environ. Sci. Pollut. Res. 28, 7404–7422. doi:10.1007/s11356-020-11068-5
Bandala, E. R., and Berli, M. (2019). Engineered Nanomaterials (ENMs) and Their Role at the Nexus of Food, Energy, and Water. Mater. Sci. Energ. Tech. 2, 29–40. doi:10.1016/j.mset.2018.09.004
Baniamerian, H., Safavi, M., Alvarado-Morales, M., Tsapekos, P., Angelidaki, I., and Shokrollahzadeh, S. (2018). Photocatalytic Inactivation of Vibrio Fischeri Using Fe2O3-TiO2-Based Nanoparticles. Environ. Res. 166, 497–506. doi:10.1016/j.envres.2018.06.011
Baruah, S., Jaisai, M., and Dutta, J. (2012). Development of a Visible Light Active Photocatalytic Portable Water Purification Unit Using ZnO Nanorods. Catal. Sci. Technol. 2, 918. doi:10.1039/c2cy20033c
Bechert, D. W., Bruse, M., and Hage, W. (2000). Experiments with Three-Dimensional Riblets as an Idealized Model of Shark Skin. Exp. Fluids 28, 403–412. doi:10.1007/s003480050400
Beigbeder, A., Degee, P., Conlan, S. L., Mutton, R. J., Clare, A. S., Pettitt, M. E., et al. (2008). Preparation and Characterisation of Silicone-Based Coatings Filled with Carbon Nanotubes and Natural Sepiolite and Their Application as marine Fouling-Release Coatings. Biofouling 24, 291–302. doi:10.1080/08927010802162885
Bixler, G. D., and Bhushan, B. (2012). Biofouling: Lessons from Nature. Phil. Trans. R. Soc. A. 370, 2381–2417. doi:10.1098/rsta.2011.0502
Bojarska, M., Nowak, B., Skowroński, J., Piątkiewicz, W., and Gradoń, L. (2017). Growth of ZnO Nanowires on Polypropylene Membrane Surface-Characterization and Reactivity. Appl. Surf. Sci. 391, 457–467. doi:10.1016/j.apsusc.2016.04.130
Bora, T., Sathe, P., Laxman, K., Dobretsov, S., and Dutta, J. (2017). Defect Engineered Visible Light Active ZnO Nanorods for Photocatalytic Treatment of Water. Catal. Today 284, 11–18. doi:10.1016/j.cattod.2016.09.014
Brady, R. F., and Singer, I. L. (2000). Mechanical Factors Favoring Release from Fouling Release Coatings. Biofouling 15, 73–81. doi:10.1080/08927010009386299
Brobbey, K. J., Haapanen, J., Mäkelä, J. M., Gunell, M., Eerola, E., Rosqvist, E., et al. (2019). Effect of Plasma Coating on Antibacterial Activity of Silver Nanoparticles. Thin Solid Films 672, 75–82. doi:10.1016/j.tsf.2018.12.049
Bystrov, V. S., Bdikin, I. K., Silibin, M. V., Karpinsky, D. V., Kopyl, S. A., Goncalves, G., et al. (2017). Graphene/graphene Oxide and Polyvinylidene Fluoride Polymer Ferroelectric Composites for Multifunctional Applications. Ferroelectrics 509, 124–142. doi:10.1080/00150193.2017.1295745
California Notice (2018). 2018-03, Final Decision Concerning Reevaluation of Copper Based Antifouling Paint Pesticides. CA, US: Department of Pesticide Regulation.
Callow, J. A., and Callow, M. E. (2011). Trends in the Development of Environmentally Friendly Fouling-Resistant marine Coatings. Nat. Commun. 2, 244. doi:10.1038/ncomms1251
Carl, C., Poole, A. J., Vucko, M. J., Williams, M. R., Whalan, S., and De Nys, R. (2012). Enhancing the Efficacy of Fouling-Release Coatings against Fouling by Mytilus Galloprovincialisusing Nanofillers. Biofouling 28, 1077–1091. doi:10.1080/08927014.2012.728588
Carré, G., Hamon, E., Ennahar, S., Estner, M., Lett, M.-C., Horvatovich, P., et al. (2014). TiO 2 Photocatalysis Damages Lipids and Proteins in Escherichia coli. Appl. Environ. Microbiol. 80, 2573–2581. doi:10.1128/aem.03995-13
Carteau, D., Vallée-Réhel, K., Linossier, I., Quiniou, F., Davy, R., Compère, C., et al. (2014). Development of Environmentally Friendly Antifouling Paints Using Biodegradable Polymer and Lower Toxic Substances. Prog. Org. Coat. 77, 485–493. doi:10.1016/j.porgcoat.2013.11.012
Cavas, L., Yildiz, P. G., Mimigianni, P., Sapalidis, A., and Nitodas, S. (2017). Reinforcement Effects of Multiwall Carbon Nanotubes and Graphene Oxide on PDMS marine Coatings. J. Coat. Technol. Res. 15, 105–120. doi:10.1007/s11998-017-9956-z
Chapman, J., Weir, E., and Regan, F. (2010). Period Four Metal Nanoparticles on the Inhibition of Biofouling. Colloids Surf. B: Biointerfaces 78, 208–216. doi:10.1016/j.colsurfb.2010.03.002
Chen, S., Li, L., Zhao, C., and Zheng, J. (2010). Surface Hydration: Principles and Applications toward Low-Fouling/nonfouling Biomaterials. Polymer 51, 5283–5293. doi:10.1016/j.polymer.2010.08.022
Chung, S. H., and Manthiram, A. (2019). Current Status and Future Prospects of Metal–Sulfur Batteries. Adv. Mater. 31, 1901125. doi:10.1002/adma.201901125
Dafforn, K. A., Lewis, J. A., and Johnston, E. L. (2011). Antifouling Strategies: History and Regulation, Ecological Impacts and Mitigation. Mar. Pollut. Bull. 62, 453–465. doi:10.1016/j.marpolbul.2011.01.012
Damodaran, V. B., and Murthy, N. S. (2016). Bio-inspired Strategies for Designing Antifouling Biomaterials. Biomater. Res. 20, 18. doi:10.1186/s40824-016-0064-4
Danilczuk, M., Lund, A., Sadlo, J., Yamada, H., and Michalik, J. (2006). Conduction Electron Spin Resonance of Small Silver Particles. Spectrochimica Acta A: Mol. Biomol. Spectrosc. 63, 189–191. doi:10.1016/j.saa.2005.05.002
Danwittayakul, S., Songngam, S., and Sukkasi, S. (2018). Enhanced Solar Water Disinfection Using ZnO Supported Photocatalysts. Environ. Technol. 41, 349–356. doi:10.1080/09593330.2018.1498921
Das, S. K., Khan, M. M. R., Parandhaman, T., Laffir, F., Guha, A. K., Sekaran, G., et al. (2013). Nano-silica Fabricated with Silver Nanoparticles: Antifouling Adsorbent for Efficient Dye Removal, Effective Water Disinfection and Biofouling Control. Nanoscale 5, 5549. doi:10.1039/c3nr00856h
Dasgupta, A., Rajukumar, L. P., Rotella, C., Lei, Y., and Terrones, M. (2017). Covalent Three-Dimensional Networks of Graphene and Carbon Nanotubes: Synthesis and Environmental Applications. Nano Today 12, 116–135. doi:10.1016/j.nantod.2016.12.011
Deng, C., Jiang, J., Liu, F., Fang, L., Wang, J., Li, D., et al. (2015). Influence of Carbon Nanotubes Coatings onto Carbon Fiber by Oxidative Treatments Combined with Electrophoretic Deposition on Interfacial Properties of Carbon Fiber Composite. Appl. Surf. Sci. 357, 1274–1280. doi:10.1016/j.apsusc.2015.09.178
Deng, Y., Song, G.-L., Zheng, D., and Zhang, Y. (2021). Fabrication and Synergistic Antibacterial and Antifouling Effect of an Organic/inorganic Hybrid Coating Embedded with Nanocomposite Ag@TA-SiO Particles. Colloids Surf. A: Physicochemical Eng. Aspects 613, 126085. doi:10.1016/j.colsurfa.2020.126085
Devasconcellos, P., Bose, S., Beyenal, H., Bandyopadhyay, A., and Zirkle, L. G. (2012). Antimicrobial Particulate Silver Coatings on Stainless Steel Implants for Fracture Management. Mater. Sci. Eng. C 32, 1112–1120. doi:10.1016/j.msec.2012.02.020
Dineshram, R., Subasri, R., Somaraju, K. R. C., Jayaraj, K., Vedaprakash, L., Ratnam, K., et al. (2009). Biofouling Studies on Nanoparticle-Based Metal Oxide Coatings on Glass Coupons Exposed to marine Environment. Colloids Surf. B: Biointerfaces 74, 75–83. doi:10.1016/j.colsurfb.2009.06.028
Divandari, M., Morgese, G., Trachsel, L., Romio, M., Dehghani, E. S., Rosenboom, J.-G., et al. (2017). Topology Effects on the Structural and Physicochemical Properties of Polymer Brushes. Macromolecules 50, 7760–7769. doi:10.1021/acs.macromol.7b01720
Dobretsov, S., Dahms, H.-U., and Qian, P.-Y. (2006). Inhibition of Biofouling by marine Microorganisms and Their Metabolites. Biofouling 22, 43–54. doi:10.1080/08927010500504784
Dobretsov, S., and Rittschof, D. (2020). Love at First Taste: Induction of Larval Settlement by Marine Microbes. Int. J. Mol. Sci. 21, 731. doi:10.3390/ijms21030731
Dobretsov, S., Sathe, P., Bora, T., Barry, M., Myint, M. T. Z., and Abri, M. A. (2020). Toxicity of Different Zinc Oxide Nanomaterials at 3 Trophic Levels: Implications for Development of Low‐Toxicity Antifouling Agents. Environ. Toxicol. Chem. 39, 1343–1354. doi:10.1002/etc.4720
Dong, L.-X., Yang, H.-W., Liu, S.-T., Wang, X.-M., and Xie, Y. F. (2015). Fabrication and Anti-biofouling Properties of Alumina and Zeolite Nanoparticle Embedded Ultrafiltration Membranes. Desalination 365, 70–78. doi:10.1016/j.desal.2015.02.023
Durán, N., Durán, M., De Jesus, M. B., Seabra, A. B., Fávaro, W. J., and Nakazato, G. (2016). Silver Nanoparticles: A New View on Mechanistic Aspects on Antimicrobial Activity. Nanomed.: Nanotechnol. Biol. Med. 12, 789–799. doi:10.1016/j.nano.2015.11.016
Dustebek, J., Kandemir-Cavas, C., Nitodas, S. F., and Cavas, L. (2016). Effects of Carbon Nanotubes on the Mechanical Strength of Self-Polishing Antifouling Paints. Prog. Org. Coat. 98, 18–27. doi:10.1016/j.porgcoat.2016.04.020
El-Zahry, M. R., Mahmoud, A., Refaat, I. H., Mohamed, H. A., Bohlmann, H., and Lendl, B. (2015). Antibacterial Effect of Various Shapes of Silver Nanoparticles Monitored by SERS. Talanta 138, 183–189. doi:10.1016/j.talanta.2015.02.022
EU Commission Regulation (2010). EU COMMISSION REGULATION No 276/2010 of 31 March 2010, Amending Regulation (EC) No 1907/2006 of the European Parliament and of the Council on the Registration, Evaluation, Authorisation and Restriction of Chemicals (REACH) as Regards Annex XVII (Dichloromethane, Lamp Oils and Grill Lighter Fluids and Organostannic Compounds).
Falfushynska, H. I., Wu, F., Ye, F., Kasianchuk, N., Dutta, J., Dobretsov, S., et al. (2019). The Effects of ZnO Nanostructures of Different Morphology on Bioenergetics and Stress Response Biomarkers of the Blue Mussels Mytilus edulis. Sci. Total Environ. 694, 133717. doi:10.1016/j.scitotenv.2019.133717
Farjami, M., Moghadassi, A., and Vatanpour, V. (2021). Influence of Solvent Type on the Ability and Properties of the Boehmite Nanoparticles Embedded Emulsion Polyvinyl Chloride Nanocomposite Ultrafiltration Membranes. Iran Polym. J. 30, 707–721. doi:10.1007/s13726-021-00925-w
Fei, Z., Rodin, A. S., Andreev, G. O., Bao, W., Mcleod, A. S., Wagner, M., et al. (2012). Gate-tuning of Graphene Plasmons Revealed by Infrared Nano-Imaging. Nature 487, 82–85. doi:10.1038/nature11253
Francis, A. P., and Devasena, T. (2018). Toxicity of Carbon Nanotubes: A Review. Toxicol. Ind. Health 34, 200–210. doi:10.1177/0748233717747472
Ganguly, P., Byrne, C., Breen, A., and Pillai, S. C. (2018). Antimicrobial Activity of Photocatalysts: Fundamentals, Mechanisms, Kinetics and Recent Advances. Appl. Catal. B: Environ. 225, 51–75. doi:10.1016/j.apcatb.2017.11.018
Gao, L., Ma, S., Ma, Z., Zheng, Z., Zhou, F., and Liang, Y. (2020). In Situ covalent Bonding in Polymerization to Construct Robust Hydrogel Lubrication Coating on Surface of Silicone Elastomer. Colloids Surf. A: Physicochemical Eng. Aspects 599, 124753. doi:10.1016/j.colsurfa.2020.124753
Garcia, C. V., Shin, G. H., and Kim, J. T. (2018). Metal Oxide-Based Nanocomposites in Food Packaging: Applications, Migration, and Regulations. Trends Food Sci. Technol. 82, 21–31. doi:10.1016/j.tifs.2018.09.021
Ginjupalli, K., Shaw, T., Tellapragada, C., Alla, R., Gupta, L., and Perampalli, N. U. (2018). Does the Size Matter? Evaluation of Effect of Incorporation of Silver Nanoparticles of Varying Particle Size on the Antimicrobial Activity and Properties of Irreversible Hydrocolloid Impression Material. Dental Mater. 34, e158–e165. doi:10.1016/j.dental.2018.03.016
Gomathi Sankar, G., Sathya, S., Sriyutha Murthy, P., Das, A., Pandiyan, R., Venugopalan, V. P., et al. (2015). Polydimethyl Siloxane Nanocomposites: Their Antifouling Efficacy In Vitro and in marine Conditions. Int. Biodeterior. Biodegrad. 104, 307–314. doi:10.1016/j.ibiod.2015.05.022
Goswami, S. R., Sahareen, T., Singh, M., and Kumar, S. (2015). Role of Biogenic Silver Nanoparticles in Disruption of Cell-Cell Adhesion in Staphylococcus aureus and Escherichia coli Biofilm. J. Ind. Eng. Chem. 26, 73–80. doi:10.1016/j.jiec.2014.11.017
Graham, M. V., and Cady, N. C. (2014). Nano and Microscale Topographies for the Prevention of Bacterial Surface Fouling. Coatings 4, 37. doi:10.3390/coatings4010037
Graziani, L., Quagliarini, E., Osimani, A., Aquilanti, L., Clementi, F., and D'orazio, M. (2014). The Influence of clay brick Substratum on the Inhibitory Efficiency of T I O 2 Nanocoating against Biofouling. Building Environ. 82, 128–134. doi:10.1016/j.buildenv.2014.08.013
Grzelczak, M., Pérez-Juste, J., Mulvaney, P., and Liz-Marzán, L. M. (2008). Shape Control in Gold Nanoparticle Synthesis. Chem. Soc. Rev. 37, 1783. doi:10.1039/b711490g
Guardiola, F. A., Cuesta, A., Meseguer, J., and Esteban, M. A. (2012). Risks of Using Antifouling Biocides in Aquaculture. Int. J. Mol. Sci. 13, 1541–1560. doi:10.3390/ijms13021541
He, Z., Lan, X., Hu, Q., Li, H., Li, L., and Mao, J. (2021). Antifouling Strategies Based on Super-phobic Polymer Materials. Prog. Org. Coat. 157, 106285. doi:10.1016/j.porgcoat.2021.106285
Hornyak, G. L. (2008). Introduction to Nanoscience and Nanotechnology. Boca Raton, Florida, USA: CRC Press.
Hoshyar, N., Gray, S., Han, H., and Bao, G. (2016). The Effect of Nanoparticle Size on In Vivo Pharmacokinetics and Cellular Interaction. Nanomedicine 11, 673–692. doi:10.2217/nnm.16.5
Hui, N., Sun, X., Niu, S., and Luo, X. (2017). PEGylated Polyaniline Nanofibers: Antifouling and Conducting Biomaterial for Electrochemical DNA Sensing. ACS Appl. Mater. Inter. 9, 2914–2923. doi:10.1021/acsami.6b11682
Inderan, V., Lim, S. Y., Ong, T. S., Bastien, S., Braidy, N., and Lee, H. L. (2015). Synthesis and Characterisations of SnO2 Nanorods via Low Temperature Hydrothermal Method. Superlattices and Microstructures 88, 396–402. doi:10.1016/j.spmi.2015.09.031
Jin, F.-L., Li, X., and Park, S.-J. (2015a). Synthesis and Application of Epoxy Resins: A Review. J. Ind. Eng. Chem. 29, 1–11. doi:10.1016/j.jiec.2015.03.026
Jin, T., and He, Y. (2011). Antibacterial Activities of Magnesium Oxide (MgO) Nanoparticles against Foodborne Pathogens. J. Nanopart Res. 13, 6877–6885. doi:10.1007/s11051-011-0595-5
Jin, Z., Li, J., Shi, L., Ji, Y., Zhong, Z., and Su, F. (2015b). One-pot Hydrothermal Growth of Raspberry-like CeO2 on CuO Microsphere as Copper-Based Catalyst for Rochow Reaction. Appl. Surf. Sci. 359, 120–129. doi:10.1016/j.apsusc.2015.10.084
Kailasa, S. K., Park, T.-J., Rohit, J. V., and Koduru, J. R. (2019). Antimicrobial Activity of Silver Nanoparticles. William Andrew Publishing 2019, 461–484. doi:10.1016/b978-0-12-816504-1.00009-0
Kang, S., Herzberg, M., Rodrigues, D. F., and Elimelech, M. (2008). Antibacterial Effects of Carbon Nanotubes: Size Does Matter! Langmuir 24, 6409–6413. doi:10.1021/la800951v
Khan, M. M., Adil, S. F., and Al-Mayouf, A. (2015). Metal Oxides as Photocatalysts. J. Saudi Chem. Soc. 19, 462–464. doi:10.1016/j.jscs.2015.04.003
Kim, H. J., Baek, Y., Choi, K., Kim, D.-G., Kang, H., Choi, Y.-S., et al. (2014). The Improvement of Antibiofouling Properties of a Reverse Osmosis Membrane by Oxidized CNTs. RSC Adv. 4, 32802. doi:10.1039/c4ra06489e
Kim, J., Baek, Y., Hong, S. P., Yoon, H., Kim, S., Kim, C., et al. (2016). Evaluation of Thin-Film Nanocomposite RO Membranes Using TiO2 nanotubes and TiO2 nanoparticles: a Comparative Study. Desalination Water Treat. 57, 24674–24681. doi:10.1080/19443994.2016.1152642
Kim, J. S., Kuk, E., Yu, K. N., Kim, J.-H., Park, S. J., Lee, H. J., et al. (2007). Antimicrobial Effects of Silver Nanoparticles. Nanomed.: Nanotechnol. Biol. Med. 3, 95–101. doi:10.1016/j.nano.2006.12.001
Kim, S. H., Kwak, S.-Y., Sohn, B.-H., and Park, T. H. (2003). Design of TiO2 Nanoparticle Self-Assembled Aromatic Polyamide Thin-Film-Composite (TFC) Membrane as an Approach to Solve Biofouling Problem. J. Membr. Sci. 211, 157–165. doi:10.1016/s0376-7388(02)00418-0
Knowles, B. R., Wagner, P., Maclaughlin, S., Higgins, M. J., and Molino, P. J. (2017). Silica Nanoparticles Functionalized with Zwitterionic Sulfobetaine Siloxane for Application as a Versatile Antifouling Coating System. ACS Appl. Mater. Inter. 9, 18584–18594. doi:10.1021/acsami.7b04840
Krishnan, M., Sivanandham, V., Hans-Uwe, D., Murugaiah, S. G., Seeni, P., Gopalan, S., et al. (2015). Antifouling Assessments on Biogenic Nanoparticles: A Field Study from Polluted Offshore Platform. Mar. Pollut. Bull. 101, 816–825. doi:10.1016/j.marpolbul.2015.08.033
Kumar, R., and Münstedt, H. (2005). Polyamide/silver Antimicrobials: Effect of Crystallinity on the Silver Ion Release. Polym. Int. 54, 1180–1186. doi:10.1002/pi.1828
Kumar, S., Bhattacharya, W., Singh, M., Halder, D., and Mitra, A. (2017). Plant Latex Capped Colloidal Silver Nanoparticles: A Potent Anti-biofilm and Fungicidal Formulation. J. Mol. Liquids 230, 705–713. doi:10.1016/j.molliq.2017.01.004
Kumar, S., Boro, J. C., Ray, D., Mukherjee, A., and Dutta, J. (2019a). Bionanocomposite Films of agar Incorporated with ZnO Nanoparticles as an Active Packaging Material for Shelf Life Extension of green Grape. Heliyon 5, e01867. doi:10.1016/j.heliyon.2019.e01867
Kumar, S., Singh, M., Halder, D., and Mitra, A. (2014). Mechanistic Study of Antibacterial Activity of Biologically Synthesized Silver Nanocolloids. Colloids Surf. A: Physicochemical Eng. Aspects 449, 82–86. doi:10.1016/j.colsurfa.2014.02.027
Kumar, S., Ye, F., Dobretsov, S., and Dutta, J. (2019b). Chitosan Nanocomposite Coatings for Food, Paints, and Water Treatment Applications. Appl. Sci. 9, 2409. doi:10.3390/app9122409
Kumar, S., Ye, F., Mazinani, B., Dobretsov, S., and Dutta, J. (2021). Chitosan Nanocomposite Coatings Containing Chemically Resistant ZnO-SnOx Core-Shell Nanoparticles for Photocatalytic Antifouling. Int. J. Mol. Sci. 22, 4513. doi:10.3390/ijms22094513
Kumari, M., Pandey, S., Giri, V. P., Bhattacharya, A., Shukla, R., Mishra, A., et al. (2017). Tailoring Shape and Size of Biogenic Silver Nanoparticles to Enhance Antimicrobial Efficacy against MDR Bacteria. Microb. Pathogenesis 105, 346–355. doi:10.1016/j.micpath.2016.11.012
Kumari, R., Brahma, G., Rajak, S., Singh, M., and Kumar, S. (2016). Antimicrobial Activity of green Silver Nanoparticles Produced Using Aqueous Leaf Extract of Hydrocotyle Rotundifolia. Orient Pharm. Exp. Med. 16, 195–201. doi:10.1007/s13596-016-0236-8
Lah, N. A. C., Zubir, M. N. M., and Samykano, M. A. L. (2018). “Engineered Nanomaterial in Electronics and Electrical Industries,” in Handbook of Nanomaterials for Industrial Applications (Amsterdam, Netherlands: Elsevier).
Lakhotia, S. R., Mukhopadhyay, M., and Kumari, P. (2018). Surface-Modified Nanocomposite Membranes. Separat. Purif. Rev. 47, 288–305. doi:10.1080/15422119.2017.1386681
Li, B., Jain, P., Ma, J., Smith, J. K., Yuan, Z., Hung, H. C., et al. (2019). Trimethylamine N-Oxide–Derived Zwitterionic Polymers: A New Class of Ultralow Fouling Bioinspired Materials. Sci. Adv. 5, eaaw9562. doi:10.1126/sciadv.aaw9562
Li, J.-H., Shao, X.-S., Zhou, Q., Li, M.-Z., and Zhang, Q.-Q. (2013). The Double Effects of Silver Nanoparticles on the PVDF Membrane: Surface Hydrophilicity and Antifouling Performance. Appl. Surf. Sci. 265, 663–670. doi:10.1016/j.apsusc.2012.11.072
Li, Y., Woo, Y., Sekar, M., Narasimalu, S., and Dong, Z. (2020). Effect of Nano-Titanium Dioxide Contained in Titania-Polyurea Coating on Marina Biofouling and Drag Reduction. J Biomed. Nanotechnol. 16, 1530–1541. doi:10.1166/jbn.2020.2980
Lin, S., Zhang, B., Skoumal, M. J., Ramunno, B., Li, X., Wesdemiotis, C., et al. (2011). Antifouling Poly(β-Peptoid)s. Biomacromolecules 12, 2573–2582. doi:10.1021/bm200368p
Liu, Q., Singh, A., Lalani, R., and Liu, L. (2012). Ultralow Fouling Polyacrylamide on Gold Surfaces via Surface-Initiated Atom Transfer Radical Polymerization. Biomacromolecules 13, 1086–1092. doi:10.1021/bm201814p
Lukowiak, A., Kedziora, A., and Strek, W. (2016). Antimicrobial Graphene Family Materials: Progress, Advances, Hopes and Fears. Adv. Colloid Interf. Sci. 236, 101–112. doi:10.1016/j.cis.2016.08.002
Majumdar, P., Lee, E., Patel, N., Ward, K., Stafslien, S. J., Daniels, J., et al. (2008). Combinatorial Materials Research Applied to the Development of New Surface Coatings IX: An Investigation of Novel Antifouling/fouling-Release Coatings Containing Quaternary Ammonium Salt Groups. Biofouling 24, 185–200. doi:10.1080/08927010801894660
Maréchal, J.-P., and Hellio, C. (2009). Challenges for the Development of New Non-toxic Antifouling Solutions. Int. J. Mol. Sci. 10, 4623–4637. doi:10.3390/ijms10114623
M.El Saeed, A., Abd El-Fattah, M., Azzam, A. M., Dardir, M. M., and Bader, M. M. (2016). Synthesis of Cuprous Oxide Epoxy Nanocomposite as an Environmentally Antimicrobial Coating. Int. J. Biol. Macromolecules 89, 190–197. doi:10.1016/j.ijbiomac.2016.04.043
Mesarič, T., Sepčič, K., Piazza, V., Gambardella, C., Garaventa, F., Drobne, D., et al. (2013). Effects of Nano Carbon Black and Single-Layer Graphene Oxide on Settlement, Survival and Swimming Behaviour of Amphibalanus Amphitrite Larvae. Chem. Ecol. 29, 643–652.
Mo, Y., Xue, P., Yang, Q., Liu, H., Zhao, X., Wang, J., et al. (2021). Composite Slow-Release Fouling Release Coating Inspired by Synergistic Anti-fouling Effect of Scaly Fish. Polymers 13, 2602. doi:10.3390/polym13162602
Mooss, V. A., Hamza, F., Zinjarde, S. S., and Athawale, A. A. (2019). Polyurethane Films Modified with Polyaniline-Zinc Oxide Nanocomposites for Biofouling Mitigation. Chem. Eng. J. 359, 1400–1410. doi:10.1016/j.cej.2018.11.038
Mostafaei, A., and Nasirpouri, F. (2013). Preparation and Characterization of a Novel Conducting Nanocomposite Blended with Epoxy Coating for Antifouling and Antibacterial Applications. J. Coat. Technol. Res. 10, 679–694. doi:10.1007/s11998-013-9487-1
Myan, F. W. Y., Walker, J., and Paramor, O. (2013). The Interaction of marine Fouling Organisms with Topography of Varied Scale and Geometry: a Review. Biointerphases 8, 30. doi:10.1186/1559-4106-8-30
Naskar, A., Khan, H., Sarkar, R., Kumar, S., Halder, D., and Jana, S. (2018). Anti-biofilm Activity and Food Packaging Application of Room Temperature Solution Process Based Polyethylene Glycol Capped Ag-ZnO-Graphene Nanocomposite. Mater. Sci. Eng. C 91, 743–753. doi:10.1016/j.msec.2018.06.009
Natarajan, S., Lakshmi, D. S., Thiagarajan, V., Mrudula, P., Chandrasekaran, N., and Mukherjee, A. (2018). Antifouling and Anti-algal Effects of Chitosan Nanocomposite (TiO2/Ag) and Pristine (TiO2 and Ag) Films on marine Microalgae Dunaliella salina. J. Environ. Chem. Eng. 6, 6870–6880. doi:10.1016/j.jece.2018.10.050
Nathanael, A. J., and Kumar, P. S. (2021). “Composite Nanocoatings for Environmental Remediation,” in Handbook of Consumer Nanoproducts. Editors S. Mallakpour, and C. M. Hussain (Singapore: Springer Singapore), 1–17. doi:10.1007/978-981-15-6453-6_64-1:
Ozer, L. Y., Garlisi, C., Oladipo, H., Pagliaro, M., Sharief, S. A., Yusuf, A., et al. (2017). Inorganic Semiconductors-Graphene Composites in Photo(electro)catalysis: Synthetic Strategies, Interaction Mechanisms and Applications. J. Photochem. Photobiol. C: Photochem. Rev. 33, 132–164. doi:10.1016/j.jphotochemrev.2017.06.003
Pan, X., Redding, J. E., Wiley, P. A., Wen, L., Mcconnell, J. S., and Zhang, B. (2010). Mutagenicity Evaluation of Metal Oxide Nanoparticles by the Bacterial Reverse Mutation Assay. Chemosphere 79, 113–116. doi:10.1016/j.chemosphere.2009.12.056
Pareek, V., Gupta, R., and Panwar, J. (2018). Do physico-chemical Properties of Silver Nanoparticles Decide Their Interaction with Biological media and Bactericidal Action? A Review. Mater. Sci. Eng. C 90, 739–749. doi:10.1016/j.msec.2018.04.093
Pounraj, S., Somu, P., and Paul, S. (2018). Chitosan and Graphene Oxide Hybrid Nanocomposite Film Doped with Silver Nanoparticles Efficiently Prevents Biofouling. Appl. Surf. Sci. 452, 487–497. doi:10.1016/j.apsusc.2018.05.009
Raizada, P., Sudhaik, A., and Singh, P. (2019). Photocatalytic Water Decontamination Using Graphene and ZnO Coupled Photocatalysts: A Review. Mater. Sci. Energ. Tech. 2, 509–525. doi:10.1016/j.mset.2019.04.007
Ramanujam Padmavathi, A., Sriyutha Murthy, P., Das, A., and Subba Rao, T. (2021). Enhanced Antifouling Property of Polydimethylsiloxane-CuO Nanocomposite in marine Environment. Mater. Lett. 301, 130342. doi:10.1016/j.matlet.2021.130342
Rasheed, T., Bilal, M., Iqbal, H. M. N., and Li, C. (2017). Green Biosynthesis of Silver Nanoparticles Using Leaves Extract of Artemisia Vulgaris and Their Potential Biomedical Applications. Colloids Surf. B: Biointerfaces 158, 408–415. doi:10.1016/j.colsurfb.2017.07.020
Rasmi, K. R., Vanithakumari, S. C., George, R. P., and Kamachi Mudali, U. (2018). Active Nano Metal Oxide Coating for Bio-Fouling Resistance. Trans. Indian Inst. Met. 71, 1323–1329. doi:10.1007/s12666-017-1264-x
Rehman, S., Ullah, R., Butt, A. M., and Gohar, N. D. (2009). Strategies of Making TiO2 and ZnO Visible Light Active. J. Hazard. Mater. 170, 560–569. doi:10.1016/j.jhazmat.2009.05.064
Ren, J., Han, P., Wei, H., and Jia, L. (2014). Fouling-Resistant Behavior of Silver Nanoparticle-Modified Surfaces against the Bioadhesion of Microalgae. ACS Appl. Mater. Inter. 6, 3829–3838. doi:10.1021/am500292y
Rendueles, O., Kaplan, J. B., and Ghigo, J.-M. (2013). Antibiofilm Polysaccharides. Environ. Microbiol. 15, 334–346. doi:10.1111/j.1462-2920.2012.02810.x
Richards, C., Slaimi, A., O’Connor, N. E., Barrett, A., Kwiatkowska, S., and Regan, F. (2020). Bio-inspired Surface Texture Modification as a Viable Feature of Future Aquatic Antifouling Strategies: A Review. Int. J. Mol. Sci. 21, 5063. doi:10.3390/ijms21145063
Ruffolo, S. A., Macchia, A., La Russa, M. F., Mazza, L., Urzì, C., De Leo, F., et al. (2013). Marine Antifouling for Underwater Archaeological Sites: TiO2 and Ag-Doped TiO2. Int. J. Photoenergy 2013, 251647. doi:10.1155/2013/251647
Sakala, G. P., and Reches, M. (2018). Peptide-based Approaches to Fight Biofouling. Adv. Mater. Inter. 5, 1800073. doi:10.1002/admi.201800073
Salta, M., Wharton, J. A., Blache, Y., Stokes, K. R., and Briand, J.-F. (2013). Marine Biofilms on Artificial Surfaces: Structure and Dynamics. Environ. Microbiol. 15, 2879. doi:10.1111/1462-2920.12186
Sathe, P., Laxman, K., Myint, M. T. Z., Dobretsov, S., Richter, J., and Dutta, J. (2017). Bioinspired Nanocoatings for Biofouling Prevention by Photocatalytic Redox Reactions. Sci. Rep. 7, 3624. doi:10.1038/s41598-017-03636-6
Sathe, P., Myint, M. T. Z., Dobretsov, S., and Dutta, J. (2016a). Removal and Regrowth Inhibition of Microalgae Using Visible Light Photocatalysis with ZnO Nanorods: A green Technology. Separat. Purif. Technol. 162, 61–67. doi:10.1016/j.seppur.2016.02.007
Sathe, P., Richter, J., Myint, M. T. Z., Dobretsov, S., and Dutta, J. (2016b). Self-decontaminating Photocatalytic Zinc Oxide Nanorod Coatings for Prevention of marine Microfouling: a Mesocosm Study. Biofouling 32, 383–395. doi:10.1080/08927014.2016.1146256
Sathya, S., Murthy, P. S., Das, A., Gomathi Sankar, G., Venkatnarayanan, S., Pandian, R., et al. (2016). Marine Antifouling Property of PMMA Nanocomposite Films: Results of Laboratory and Field Assessment. Int. Biodeterior. Biodegrad. 114, 57–66. doi:10.1016/j.ibiod.2016.05.026
Scardino, A. J., and De Nys, R. (2011). Mini Review: Biomimetic Models and Bioinspired Surfaces for Fouling Control. Biofouling 27, 73–86. doi:10.1080/08927014.2010.536837
Scardino, A. J., Zhang, H., Cookson, D. J., Lamb, R. N., and Nys, R. d. (2009). The Role of Nano-Roughness in Antifouling. Biofouling 25, 757–767. doi:10.1080/08927010903165936
Schultz, M. P., Bendick, J. A., Holm, E. R., and Hertel, W. M. (2010). Economic Impact of Biofouling on a Naval Surface Ship. Biofouling 27, 87–98. doi:10.1080/08927014.2010.542809
Selim, M. S., El-Safty, S. A., Fatthallah, N. A., and Shenashen, M. A. (2018). Silicone/graphene Oxide Sheet-Alumina Nanorod Ternary Composite for Superhydrophobic Antifouling Coating. Prog. Org. Coat. 121, 160–172. doi:10.1016/j.porgcoat.2018.04.021
Selim, M. S., Fatthallah, N. A., Higazy, S. A., Hao, Z., and Jing Mo, P. (2022). A Comparative Study between Two Novel Silicone/graphene-Based Nanostructured Surfaces for Maritime Antifouling. J. Colloid Interf. Sci. 606, 367–383. doi:10.1016/j.jcis.2021.08.026
Selim, M. S., Yang, H., Wang, F. Q., Fatthallah, N. A., Huang, Y., and Kuga, S. (2019). Silicone/ZnO Nanorod Composite Coating as a marine Antifouling Surface. Appl. Surf. Sci. 466, 40–50. doi:10.1016/j.apsusc.2018.10.004
Shen, W., Feng, L., Liu, X., Luo, H., Liu, Z., Tong, P., et al. (2016). Multiwall Carbon Nanotubes-Reinforced Epoxy Hybrid Coatings with High Electrical Conductivity and Corrosion Resistance Prepared via Electrostatic Spraying. Prog. Org. Coat. 90, 139–146. doi:10.1016/j.porgcoat.2015.10.006
Song, K., Shim, J., Jung, J.-Y., Lee, C., and Nam, Y. (2020). Endowing Antifouling Properties on Metal Substrata by Creating an Artificial Barrier Layer Based on Scalable Metal Oxide Nanostructures. Biofouling 36, 766–782. doi:10.1080/08927014.2020.1811238
Spirescu, V. A., Șuhan, R., Niculescu, A.-G., Grumezescu, V., Negut, I., Holban, A. M., et al. (2021). Biofilm-Resistant Nanocoatings Based on ZnO Nanoparticles and Linalool. Nanomaterials 11, 2564. doi:10.3390/nano11102564
Sugunan, A., Warad, H. C., Boman, M., and Dutta, J. (2006). Zinc Oxide Nanowires in Chemical bath on Seeded Substrates: Role of Hexamine. J. Sol-gel Sci. Technol. 39, 49–56. doi:10.1007/s10971-006-6969-y
Sun, X.-F., Qin, J., Xia, P.-F., Guo, B.-B., Yang, C.-M., Song, C., et al. (2015). Graphene Oxide-Silver Nanoparticle Membrane for Biofouling Control and Water Purification. Chem. Eng. J. 281, 53–59. doi:10.1016/j.cej.2015.06.059
Sun, Y., Lang, Y., Yan, Z., Wang, L., and Zhang, Z. (2020). High-throughput Sequencing Analysis of marine pioneer Surface-Biofilm Bacteria Communities on Different PDMS-Based Coatings. Colloids Surf. B: Biointerfaces 185, 110538. doi:10.1016/j.colsurfb.2019.110538
Thérien-Aubin, H., Chen, L., and Ober, C. K. (2011). Fouling-resistant Polymer brush Coatings. Polymer 52, 5419–5425. doi:10.1016/j.polymer.2011.09.017
Tobaldi, D. M., Graziani, L., Seabra, M. P., Hennetier, L., Ferreira, P., Quagliarini, E., et al. (2017). Functionalised Exposed Building Materials: Self-Cleaning, Photocatalytic and Biofouling Abilities. Ceramics Int. 43, 10316–10325. doi:10.1016/j.ceramint.2017.05.061
Upadhyayula, V. K. K., and Gadhamshetty, V. (2010). Appreciating the Role of Carbon Nanotube Composites in Preventing Biofouling and Promoting Biofilms on Material Surfaces in Environmental Engineering: A Review. Biotechnol. Adv. 28, 802–816. doi:10.1016/j.biotechadv.2010.06.006
Uskoković, V., and Bertassoni, L. E. (2010). Nanotechnology in Dental Sciences: Moving towards a Finer Way of Doing Dentistry. Materials (Basel) 3, 1674–1691. doi:10.3390/ma3031674
Valenzuela, L., Iglesias, A., Faraldos, M., Bahamonde, A., and Rosal, R. (2019). Antimicrobial Surfaces with Self-Cleaning Properties Functionalized by Photocatalytic ZnO Electrosprayed Coatings. J. Hazard. Mater. 369, 665–673. doi:10.1016/j.jhazmat.2019.02.073
Vatanpour, V., Madaeni, S. S., Rajabi, L., Zinadini, S., and Derakhshan, A. A. (2012). Boehmite Nanoparticles as a New Nanofiller for Preparation of Antifouling Mixed Matrix Membranes. J. Membr. Sci. 401-402, 132–143. doi:10.1016/j.memsci.2012.01.040
Venkataraman, A., Amadi, E. V., Chen, Y., and Papadopoulos, C. (2019). Carbon Nanotube Assembly and Integration for Applications. Nanoscale Res. Lett. 14, 220. doi:10.1186/s11671-019-3046-3
Venkatnarayanan, S., Sriyutha Murthy, P., Kirubagaran, R., and Venugopalan, V. P. (2017). Chlorine Dioxide as an Alternative Antifouling Biocide for Cooling Water Systems: Toxicity to Larval Barnacle Amphibalanus Reticulatus (Utinomi). Mar. Pollut. Bull. 124, 803–810. doi:10.1016/j.marpolbul.2017.01.023
Von Freymann, G., Ledermann, A., Thiel, M., Staude, I., Essig, S., Busch, K., et al. (2010). Three-Dimensional Nanostructures for Photonics. Adv. Funct. Mater. 20, 1038–1052. doi:10.1002/adfm.200901838
Wahl, M. (1989). Marine Epibiosis. I. Fouling and Antifouling: Some Basic Aspects. Mar. Ecol. Prog. Ser. 58, 175–189. doi:10.3354/meps058175
Wallenhorst, L., Gurău, L., Gellerich, A., Militz, H., Ohms, G., and Viöl, W. (2018). UV-blocking Properties of Zn/ZnO Coatings on wood Deposited by Cold Plasma Spraying at Atmospheric Pressure. Appl. Surf. Sci. 434, 1183–1192. doi:10.1016/j.apsusc.2017.10.214
Walters, D. A., Ericson, L. M., Casavant, M. J., Liu, J., Colbert, D. T., Smith, K. A., et al. (1999). Elastic Strain of Freely Suspended Single-wall Carbon Nanotube Ropes. Appl. Phys. Lett. 74, 3803–3805. doi:10.1063/1.124185
Wang, J., Chen, R., Xiang, L., and Komarneni, S. (2018). Synthesis, Properties and Applications of ZnO Nanomaterials with Oxygen Vacancies: A Review. Ceramics Int. 44, 7357–7377. doi:10.1016/j.ceramint.2018.02.013
Wang, P., Dong, Y., Zhang, S., Liu, W., Wu, Z., and Chen, H. (2019). Protein-resistant Properties of poly(N-Vinylpyrrolidone)-Modified Gold Surfaces: The Advantage of Bottle-Brushes over Linear Brushes. Colloids Surf. B: Biointerfaces 177, 448–453. doi:10.1016/j.colsurfb.2019.02.030
Wassel, A. R., El-Naggar, M. E., and Shoueir, K. (2020). Recent Advances in Polymer/metal/metal Oxide Hybrid Nanostructures for Catalytic Applications: a Review. J. Environ. Chem. Eng. 8, 104175. doi:10.1016/j.jece.2020.104175
Wei, H., Wu, Y., Lun, N., and Hu, C. (2005). Hydrothermal Synthesis and Characterization of ZnO Nanorods. Mater. Sci. Eng. A 393, 80–82. doi:10.1016/j.msea.2004.09.067
Wyszogrodzka, M., and Haag, R. (2009). Synthesis and Characterization of Glycerol Dendrons, Self-Assembled Monolayers on Gold: A Detailed Study of Their Protein Resistance. Biomacromolecules 10, 1043–1054. doi:10.1021/bm801093t
Xie, Q., Pan, J., Ma, C., and Zhang, G. (2019). Dynamic Surface Antifouling: Mechanism and Systems. Soft Matter 15, 1087–1107. doi:10.1039/c8sm01853g
Xu, F., Wang, T., Chen, H., Bohling, J., Maurice, A. M., Wu, L., et al. (2017). Preparation of Photocatalytic TiO2 -based Self-Cleaning Coatings for Painted Surface without Interlayer. Prog. Org. Coat. 113, 15–24. doi:10.1016/j.porgcoat.2017.08.005
Xu, H., Ma, L., and Jin, Z. (2018). Nitrogen-doped Graphene: Synthesis, Characterizations and Energy Applications. J. Energ. Chem. 27, 146–160. doi:10.1016/j.jechem.2017.12.006
Yang, C., Mamouni, J., Tang, Y., and Yang, L. (2010). Antimicrobial Activity of Single-Walled Carbon Nanotubes: Length Effect. Langmuir 26, 16013–16019. doi:10.1021/la103110g
Yang, J.-L., Li, Y.-F., Guo, X.-P., Liang, X., Xu, Y.-F., Ding, D.-W., et al. (2016a). The Effect of Carbon Nanotubes and Titanium Dioxide Incorporated in PDMS on Biofilm Community Composition and Subsequent Mussel Plantigrade Settlement. Biofouling 32, 763–777. doi:10.1080/08927014.2016.1197210
Yang, J.-L., Li, Y.-F., Liang, X., Guo, X.-P., Ding, D.-W., Zhang, D., et al. (2016b). Silver Nanoparticles Impact Biofilm Communities and Mussel Settlement. Sci. Rep. 6, 37406. doi:10.1038/srep37406
Yang, J., Xue, B., Zhou, Y., Qin, M., Wang, W., and Cao, Y. (2021). Spray‐Painted Hydrogel Coating for Marine Antifouling. Adv. Mater. Technol. 6, 2000911. doi:10.1002/admt.202000911
Yao, X., Liu, J., Yang, C., Yang, X., Wei, J., Xia, Y., et al. (2019). Hydrogel Paint. Adv. Mater. 31 (31), e1903062. doi:10.1002/adma.201903062
Yebra, D. M., Kiil, S., and Dam-Johansen, K. (2004). Antifouling Technology-Past, Present and Future Steps towards Efficient and Environmentally Friendly Antifouling Coatings. Prog. Org. Coat. 50, 75–104. doi:10.1016/j.porgcoat.2003.06.001
Yebra, D. M., Kiil, S., Weinell, C. E., and Dam-Johansen, K. (2006). Effects of marine Microbial Biofilms on the Biocide Release Rate from Antifouling Paints-A Model-Based Analysis. Prog. Org. Coat. 57, 56–66. doi:10.1016/j.porgcoat.2006.06.003
Yee, M. S.-L., Khiew, P. S., Lim, S. S., Chiu, W. S., Tan, Y. F., Kok, Y.-Y., et al. (2017). Enhanced marine Antifouling Performance of Silver-Titania Nanotube Composites from Hydrothermal Processing. Colloids Surf. A Physicochem. Eng. Asp. 520, 701–711. doi:10.1016/j.colsurfa.2017.02.034
Yemmireddy, V. K., and Hung, Y.-C. (2017). Photocatalytic TiO2 Coating of Plastic Cutting Board to Prevent Microbial Cross-Contamination. Food Control 77, 88–95. doi:10.1016/j.foodcont.2017.01.025
Yong, H. E., Krishnamoorthy, K., Hyun, K. T., and Kim, S. J. (2015). Preparation of ZnO Nanopaint for marine Antifouling Applications. J. Ind. Eng. Chem. 29, 39–42. doi:10.1016/j.jiec.2015.04.020
Youssef, Z., Colombeau, L., Yesmurzayeva, N., Baros, F., Vanderesse, R., Hamieh, T., et al. (2018). Dye-sensitized Nanoparticles for Heterogeneous Photocatalysis: Cases Studies with TiO2, ZnO, Fullerene and Graphene for Water Purification. Dyes Pigm. 159, 49–71. doi:10.1016/j.dyepig.2018.06.002
Zhang, H., and Chiao, M. (2015). Anti-fouling Coatings of Poly(dimethylsiloxane) Devices for Biological and Biomedical Applications. J. Med. Biol. Eng. 35, 143–155. doi:10.1007/s40846-015-0029-4
Zhang, J., Pan, M., Luo, C., Chen, X., Kong, J., and Zhou, T. (2016). A Novel Composite Paint (TiO2/fluorinated Acrylic Nanocomposite) for Antifouling Application in marine Environments. J. Environ. Chem. Eng. 4, 2545–2555. doi:10.1016/j.jece.2016.05.002
Zhu, C., Yang, G., Li, H., Du, D., and Lin, Y. (2014). Electrochemical Sensors and Biosensors Based on Nanomaterials and Nanostructures. Anal. Chem. 87, 230–249. doi:10.1021/ac5039863
Keywords: nanocoating, antifouling, biocide, polymer, hydrogel, nanomaterial
Citation: Kumar S, Ye F, Dobretsov S and Dutta J (2021) Nanocoating Is a New Way for Biofouling Prevention. Front. Nanotechnol. 3:771098. doi: 10.3389/fnano.2021.771098
Received: 05 September 2021; Accepted: 05 November 2021;
Published: 22 November 2021.
Edited by:
Amitava Mukherjee, VIT University, IndiaReviewed by:
Sriyutha Murthy, Bhabha Atomic Research Centre, IndiaKamel A. Abd-Elsalam, Agricultural Research Center, Egypt
Copyright © 2021 Kumar, Ye, Dobretsov and Dutta. This is an open-access article distributed under the terms of the Creative Commons Attribution License (CC BY). The use, distribution or reproduction in other forums is permitted, provided the original author(s) and the copyright owner(s) are credited and that the original publication in this journal is cited, in accordance with accepted academic practice. No use, distribution or reproduction is permitted which does not comply with these terms.
*Correspondence: Joydeep Dutta, Sm95ZGVlcEBrdGguc2U=; Sergey Dobretsov, c2VyZ2V5QHNxdS5lZHUub20=