Low Energy Laser Treatments Control Annual Ryegrass (Lolium rigidum)
- 1Sydney Institute of Agriculture, School of Life and Environmental Sciences, University of Sydney, Sydney, NSW, Australia
- 2Sydney Astrophotonic Instrumentation Laboratory, School of Physics, University of Sydney, Sydney, NSW, Australia
Increasing concern for the ongoing availability and efficacy of herbicides is driving interest in the development of alternative physical and thermal weed control methods. Fortunately, improvements in weed detection through advancements in computing hardware and deep learning algorithms are creating an opportunity to use novel weed control tools, such as lasers, in large-scale cropping systems. For alternative control options, there are two key weed control timing opportunities, early and late post-crop emergence. Weed density for the early timing is typically higher, with a shorter window for control. Conversely, late post-emergent treatment of surviving and late-emerging weeds would occur in lower densities of larger and more variably sized weeds, given a prior weed control effort, but with a longer available weed control period. Research in laser weeding to date has primarily focused on early growth stage weeds and the ability of this approach to control larger weeds remains unknown. This study used a 25 W, 975 nm fiber-coupled diode laser to evaluate the opportunity for control of annual ryegrass (Lolium rigidum Gaudin) and the influence of four different growth stages (three-leaf, seven-leaf, mid-tillering, and late-tillering). Annual ryegrass plants at each growth stage were treated using a laser-focused to a 5 mm diameter with five different irradiation durations developing energy densities of 1.3, 2.5, 6.4, 19.1, and 76.4 J mm−2. At the three-leaf stage, all plants were controlled at 76.4 J mm−2 and 93.3% controlled at 19.1 J mm−2. Complete control of seven-leaf plants was only achieved at 76.4 J mm−2. Although laser treatments did not control mid-tillering stage plants, 76.4 J mm−2 reduced biomass by 60.2%. No similar reductions in biomass were recorded for the largest plants. This initial research assists in the development of novel weed control options in the context of large-scale conservation cropping systems. Future research should investigate the influence of laser treatments on additional weed species and the impact of increased laser power on larger weeds.
Introduction
The widespread adoption of conservation agriculture in large-scale cropping systems has resulted in improved productivity outcomes by focusing on minimal soil disturbance, increased soil cover, and diverse cropping species. These outcomes are based on enhanced soil health due to improved soil structure, better nutrient cycling and increased organic matter (Kassam et al., 2012). Without the opportunity for using tillage-based weed control in these production systems, weed management is heavily reliant on herbicides. The only alternatives suitable for routine use in cropping systems are agronomic approaches that increase crop competition, including higher plant densities (Lemerle et al., 2001, 2004), narrow row spacing and strategic fertilizer placement (Kristensen et al., 2008; Bajwa et al., 2015). Recently in Australian cropping systems, harvest weed seed control has been adopted as a standard approach for targeting weed seeds collected during crop harvest (Walsh et al., 2013). There are no currently available physical or thermal weed control methods that provide equivalent levels of efficacy and cost-effectiveness as herbicides in large-scale conservation cropping systems (Coleman et al., 2019; Bauer et al., 2020). However, the selection pressure from repeated use of herbicides with similar modes of action has resulted in the widespread evolution of herbicide resistance (Heap, 2020). The problem of resistance for weed control is compounded by a lack of availability of new herbicide modes of action, negative public perception of herbicide use and increasing regulation that is restricting herbicide development (Duke, 2012). Reduced herbicide options are a significant threat to conservation agriculture in large-scale production systems driving the need for research and development of feasible alternatives.
Site-specific application technologies enable the use of non-selective thermal weed control methods in large-scale cropping systems. While research and development has continued for non-chemical control options, these approaches have not typically progressed to commercialization, owing to high or unknown control costs and a lack of in-crop selectivity. Fortunately, site-specific weed control (SSWC) offers an opportunity for selective application of non-selective methods, improving their relevance in large-scale production systems (Coleman et al., 2019). Until recently, the bottleneck in SSWC has been the accurate, precise and reliable detection of weeds. However, advancements in deep learning, now being translated into the agricultural domain, have seen in-crop weed detection become increasingly realistic (López-Granados, 2011; Wang et al., 2019). Greater computational speed, miniaturization, and reduced costs are creating the opportunity for detection and fine-grained identification to be feasible in large-scale cropping systems and at a level of specificity that enables alternative control options. At the highest spatial resolution for weed detection, recent assessments of instance segmentation for common weeds demonstrate the opportunity for exact targeting of plant center and stem locations in both grass and broadleaf weeds (Champ et al., 2020; Lottes et al., 2020). These developments would enable the use of high precision weed control options such as laser and electrical weeding. However, challenges remain in the reliability of detection, generalization to other weed species and the ability to handle diverse environments.
Laser treatments offer one of the highest levels of precision for targeted weed control and can be applied as either pyrolytic spot or stem cutting treatments. A laser is a highly directional beam of electromagnetic energy that results in the heating of the targeted area. Since the 1970s, there have been intermittent research efforts on the use of lasers for the control of seedling and early growth stage monocot and dicot weeds, which have highlighted the opportunity for this approach. Couch and Gangstad (1974) found treatment with a CO2 laser (10,600 nm) reduced biomass of water hyacinth (Eichhornia crassipes [Mart.] Solms). Other glasshouse studies report significant biomass reductions in volunteer rye (Secale cereale L.) and wild oat (Avena fatua L.) by CO2 laser-based stem clipping. However, complete control was not observed with the regrowth of tillers post-clipping (Bayramian et al., 1992).
Further research on the use of laser cutting for weed control found up to 2.3 J per mm of stem thickness was required to cut stems of charlock mustard (Sinapis arvensis L.) with a 50 W CO2 laser and 0.6 mm2 beam area (Heisel et al., 2001). If the cut was performed below the apical meristem, there was a 90% reduction in biomass for the dicot weeds. A significant reduction in biomass was observed when two-leaf stage perennial ryegrass (Lolium perenne L.) plants were cut 2 cm above the soil surface with the laser, compared to the scissors, suggesting a heating effect of the laser treatment on growth. Differences were also found between 10,600 and 355 nm wavelength lasers for cutting efficiency of charlock mustard and winter wheat (Triticum aestivum L.) based on the increased absorption and hence explosive heating of water by the longer wavelength laser (Schou et al., 2002).
Rather than cutting of plant tissue, laser-based cellular ablation, and pyrolysis seek to disrupt the cellular function of the apical meristem through exposure to high temperatures. Evidence of the effectiveness of laser pyrolysis with diode and CO2 lasers has been observed for the control of cultivated tobacco (Nicotiana tabacum L.) (Wöltjen et al., 2008), barnyard grass (Echinochloa crus-galli [L.] P. Beauv.) (Wöltjen et al., 2008; Marx et al., 2012) and redroot pigweed (Amaranthus retroflexus L.) at the seedling, two-leaf and four-leaf growth stages. Marx et al. (2012) found that the lowest lethal energy dose was 54 and 25 J for barnyard grass and redroot pigweed, respectively. The research to date has focused on early growth stage weeds (seedling to four-leaf) with no known studies on laser efficacy on larger/older weeds (seven-leaf to late-tillering). Broadly, two key in-crop weed control timing opportunities exist in large scale cropping systems, early and late post-crop emergence. Weed densities at the early timing are typically larger, with densities in Australian production systems of approximately five plants m−2 (Llewellyn et al., 2016). During this critical crop growth stage, weed control timing within a 2- to 3-week period is vital in minimizing the impact of weeds on yield potential.
In comparison, the control of late post-emergent weeds, which have escaped treatment, is more focused on minimizing seed set for future seasons. Surviving and late-emerging weeds at this stage may be present in lower densities of larger weeds and may be more variable in weed size given the prior weed control effort. However, there is a longer available weed control period. Hence, developing control options that target larger growth stage weeds for complementary use with initial herbicide treatments is important in understanding the use-case for novel tools.
Annual ryegrass (Lolium rigidum Gaudin) is the dominant weed of Australian grain production systems. There are no studies that have investigated the efficacy of laser treatments for the control of annual ryegrass. Addressing these gaps in growth stage and species, this research aimed to evaluate (i) the irradiation energy requirement (as determined by treatment duration) of spot laser treatments to control annual ryegrass and (ii) the influence of growth stage on energy requirement for annual ryegrass control.
Materials and Methods
Pot trials evaluating the effects of spot laser treatments on annual ryegrass growth and survival were conducted in 2019 and 2020, located in an outdoor growth facility at the I.A. Watson International Grains Research Center in Narrabri, NSW, Australia (−30.276790, 149.810460). Plants were established by planting at least five seeds from a commercial annual ryegrass seed lot at a depth of 10 mm in 100 or 200 mm diameter black plastic pots filled with a commercial potting mixture, Ultragrow Platinum potting mix (Centenary Landscaping, Queensland, Australia). The plantings were conducted four times at 2-week intervals, with five replicates per treatment at each planting time, establishing four growth stages for laser treatment. Pots were routinely watered to maintain soil near field capacity and were fertilized as required with a complete liquid fertilizer. Plants were thinned to one plant per pot at the one to two-leaf stage. The resulting annual ryegrass growth stages at the time of laser treatment were three-leaf, seven-leaf, mid-tillering, and late-tillering (Table 1 and Figure 1).
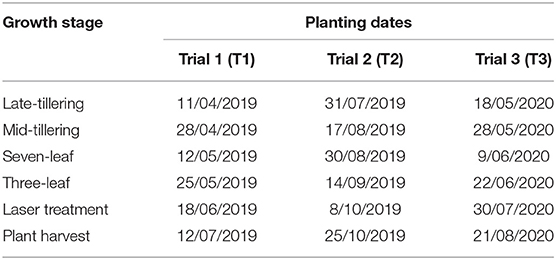
Table 1. Sowing dates and treatment dates for the four growth stages across three separate trials in 2019 and 2020.
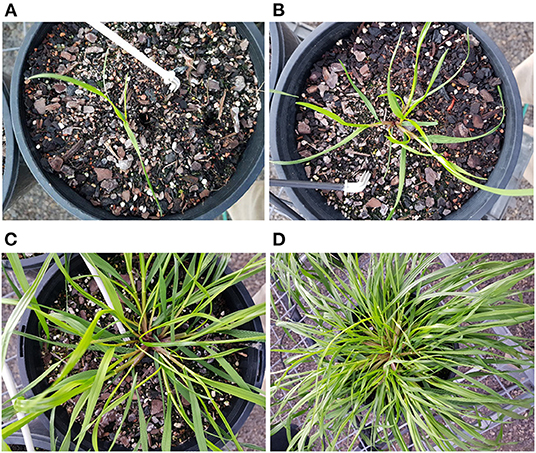
Figure 1. Indicative plant sizes for the four annual ryegrass growth stages treated (A) three-leaf, (B) seven-leaf, (C) mid-tillering, and (D) late-tillering before laser treatment.
Laser Treatment
Pyrolytic laser treatments were applied using a continuous wave (CW) 25 W fiber-coupled laser diode (OptLaser, Piaseczno, Poland). The laser produces a 975 ± 10 nm beam delivered to a fixed focal length lens of 36.3 mm with an optical fiber cable. Power control was provided by adjusting input driver voltage to the laser diode controller between 0 and 5 V through a custom Python 3 (Van Rossum and Drake, 2009) command-line interface software and Arduino driver.
The laser was mounted in a custom-built double-interlock system (Figure 2) enabling precise targeting of weeds and safe operation of the laser, which was contained within an additional interlocked housing. Individual pots were positioned manually. Targeting was achieved using a 3% of total power setting and a Thorlabs NIR Detector Card (ThorLabs Newton New Jersey, USA). Plants were treated with a 5 mm beam width developed at 42 mm from lens surface for 1, 2, 5, 15, and 60 s (Table 2). The selection of laser beam diameter and hence laser beam area (spot size) through laser optics has been shown to impact the total energy required (Wöltjen et al., 2008; Marx et al., 2012; Kaierle et al., 2013), with larger spot sizes improving ease of targeting, whilst smaller diameters reducing potential energy wastage. Earlier studies suggested that the larger spot sizes used provided improved performance, though the results were inconclusive (Mathiassen et al., 2006). A key benefit of larger spot sizes is the reduced requirement for exact positioning. Very narrow beams may miss treating the growing point from small positioning errors. The 5 mm beam diameter used in this study was based on findings from Marx et al. (2012) and Kaierle et al. (2013), where weeds up to the four-leaf stage with laser beam widths up to 6 mm were tested. As plants grow, the growing point also increases in size, such that larger beam sizes may be needed to target the larger growing points. The 5 mm beam width was selected to accommodate both seedling stage annual ryegrass and more mature plants. A fan inside the interlock was used to remove smoke condensing on the lens, thus preventing damage and reduced performance. The laser was positioned directly above the plant, targeting the base of the leaves or center of tillers depending on plant growth stage.
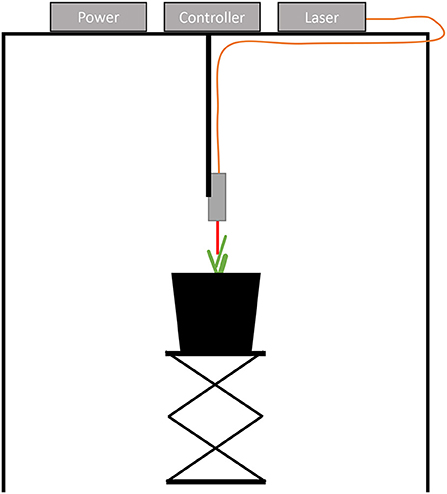
Figure 2. Diagram of laser interlock and manual targeting method for the CW 25 W 975 nm fiber-coupled diode laser.
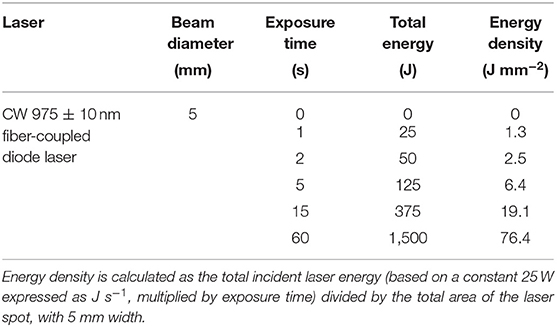
Table 2. Summary of laser exposure and energy parameters for the CW 25 W, 975 nm fiber-coupled diode laser.
Following treatment, plants were returned to the outdoor growth facility. Laser treatment effects were assessed through mortality counts and dry weight cuts at 25, 29, and 22 days after treatment for Trial 1 (T1), Trial 2 (T2), and Trial 3 (T3), respectively. Plants were deemed to be dead if no new growth was present (Table 1 and Figure 3). Surviving plants were harvested by cutting at ground level and placing in an envelope or small paper bag, depending on plant size. Plants were then oven-dried at 70°C for 3 days before weighing. Plant biomass data were converted to a percentage of untreated control for analysis and presentation.
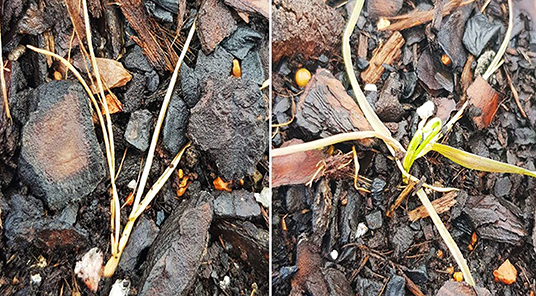
Figure 3. Annual ryegrass three-leaf stage plants at 3 weeks post-laser treatment illustrating plants considered controlled (Left) and uncontrolled (Right) with new growth emerging from the uncontrolled growing point.
Statistical Analysis
Treatment durations were converted to energy density using:
Where ρenergy is the energy density in J mm−2, P is laser power in Watts, t is treatment duration in seconds and rbeam is the radius of the laser beam in millimeters. Energy density allows more straightforward comparison of different beam widths, by standardizing incident energy by spot area. The percentage of untreated control values were analyzed using the Dose-Response Curve (DRC) package (Ritz et al., 2015) implemented in RStudio (RStudio Team, 2015; R Core Team, 2019) statistical software. Dose-response analyses were plotted with ggplot2 (Wickham, 2016).
Dry weight with a standard error of the mean (n = 15) and survival counts as a percentage of total replicates are presented in Table 3, with all three trial results grouped. An analysis of variance was conducted in RStudio with no significant difference at the trial level. The response of plants as a percentage of treated control biomass response to increasing laser energy treatment curves was fitted with the DRC package using a three-parameter log-logistic function:
Where d is the upper limit, b is the slope of the curve, and e is the inflection point or effective dose for 50% control. The choice of model was determined as the optimum fit through comparison with other functions based on the minimization of the Akaike information criterion. Lack of fit testing was performed for the resulting curves, with no significance found (P < 0.05) indicating an appropriate model was chosen. Effective doses (ED) at the 50% (ED50) and 90% (ED90) dry weight reduction levels were determined with the DRC package on the modeled dose-response curves and compared for significance from zero and each other.
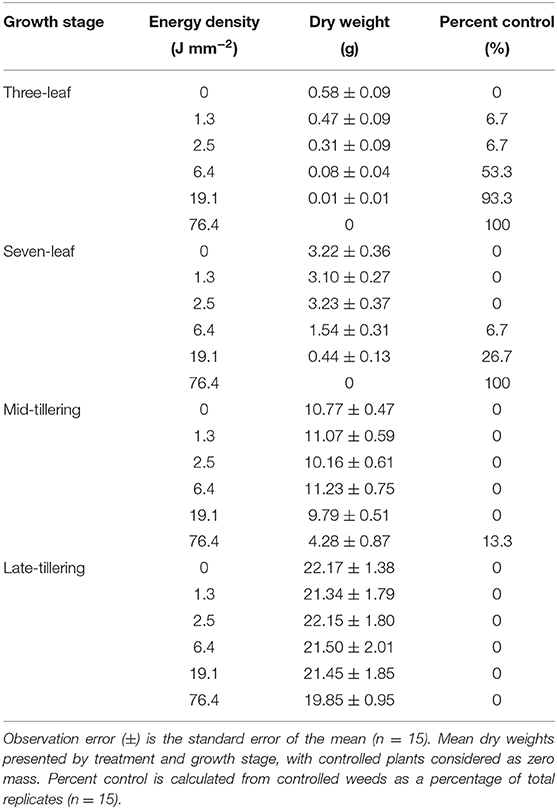
Table 3. Annual ryegrass dry weight in response to increasing laser energy density treatments when applied to four different growth stages based on three trials.
Results
The laser energy levels used in this study were, in general, too low given that the highest treatments tested were only effective on three-leaf and seven-leaf stage annual ryegrass, with no control of older growth stages. Three-leaf annual ryegrass was controlled with 76.4 J mm−2, with only one survivor at 19.1 J mm−2 laser energy treatments. The highest laser energy treatment of 76.4 J mm−2 controlled all seven-leaf plants (Table 3), with no consistent control observed at other treatment energy levels.
Laser treatments consistently reduced annual ryegrass biomass at the earliest growth stages but had little or no effect on tillering plants (Table 3). Biomass reductions of 46.6 and 85.8% were observed for three-leaf stage plants following treatment with the 2.5 and 6.4 J mm−2, respectively. At the seven-leaf stage, there were reductions of 52.1 and 86.2% observed for the 6.4 and 19.1 J mm−2 treatments, respectively. At the mid-tillering stage, annual ryegrass growth was only reduced at the highest energy treatment, which resulted in a 60.2% reduction in biomass. At the late-tillering growth stage, there was a minor decrease in plant biomass of 10.4% observed at the highest laser energy treatment.
Laser Energy Dose-Response
The laser energy density required to reduce the growth of older and larger annual ryegrass plants is substantially greater than the density required to affect the growth of seedlings similarly. Dose-response curves were developed to show the relationship between increasing laser energy doses and annual ryegrass biomass reductions (Figure 4). The growth of annual ryegrass was restricted in a log-logistic relationship with increasing energy treatments. The log-logistic curve failed to fit on the late-tillering growth stage, given the lack of impact on biomass. As highlighted by the ED50 values, there was a 2.4-fold higher energy requirement (P < 0.05) to achieve a 50% reduction in the biomass of seven-leaf annual ryegrass plants (6.13 ± 0.37 J mm−2) compared with three-leaf plants (2.60 ± 0.37 J mm−2) (Figure 4). The energy requirements for ED50 of mid-tillering plants were 10.1 and 23.8-fold greater (P < 0.05) than the seven and three-leaf growth stages. There were no differences (P > 0.05) between the ED90 values of 8.32 ± 2.33 J mm−2 and 13.87 ± 5.27 J mm−2 for the three and seven-leaf growth stage. ED90 values were not found within the range of energy levels tested for either mid-tillering or late-tillering growth stages. The comparatively flat dose-response curves highlight that there was little or no effect of the laser treatments on tillering plants.
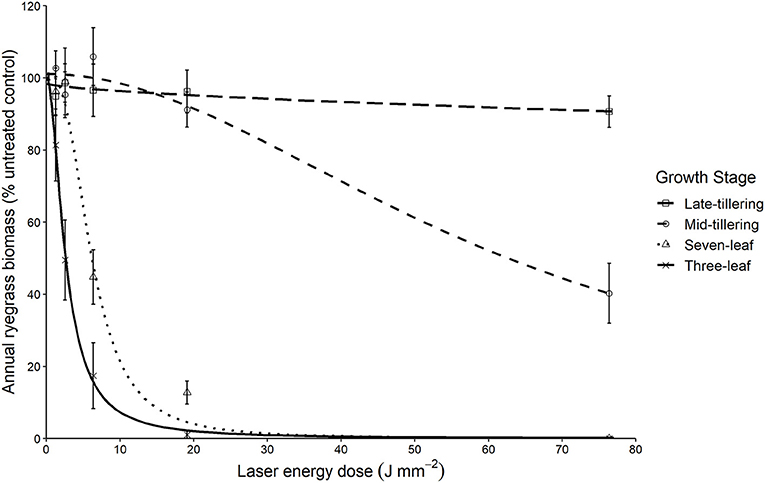
Figure 4. Influence of increasing laser energy density doses on the biomass of annual ryegrass plants when applied at four plant growth stages expressed as the percentage of the untreated control. Growth stages treated included three-leaf, seven-leaf, mid-tillering, and late-tillering. Error bars are provided as standard errors of the mean (n = 15).
Discussion
The use of a laser beam to deliver targeted energy for pyrolytic, thermal plant damage demonstrated the potential for control of three- and seven-leaf annual ryegrass plants, with no control observed for later growth stages. Complete control of three- and seven-leaf plants at 76.4 J mm−2 and 93.3% control of three-leaf at 19.1 J mm−2 illustrate the potential of lasers as an effective SSWC tool for seedling annual ryegrass plants. The lack of control of larger plants even at the highest energy dose indicates that substantially higher laser energy treatments beyond those tested would be required to control, or at least significantly impact the growth of these older annual ryegrass growth stages. Nevertheless, control of three- and seven-leaf weeds is encouraging for the deployment of lasers in large-scale cropping systems, indicating laser capability in weed control, though not necessarily specific energy doses required given the exploratory nature of the energy treatments used.
The lowest dose at which high levels of control of three-leaf stage annual ryegrass was observed was 19.1 J mm−2, considerably higher than results recorded for barnyard-grass. Attempts at controlling four-leaf stage barnyard grass (Marx et al., 2012) were not successful. Control at the three-leaf stage occurred with ~3 J mm−2, though there was substantial variability in the energy required (Wöltjen et al., 2008). Previous studies evaluating lasers for pyrolysis have not attempted to control seven-leaf or larger weeds, with most focusing on dicotyledonous weeds at early growth stages (Mathiassen et al., 2006; Wöltjen et al., 2008; Marx et al., 2012; Kaierle et al., 2013; Xiong et al., 2017). The finding of complete control of seven-leaf annual ryegrass at the highest energy treatment of 76.4 J mm−2 is a significant preliminary finding, however, at the next highest treatment, 19.1 J mm−2, only 23.3% control was achieved. These results suggest that smaller increments in laser energy between the chosen levels are required to determine the point of adequate control more precisely.
Our CW 25 W fiber-coupled diode laser delivered inadequate energy after 60 s for control of tillering annual ryegrass. This result highlighted the inadequate power and hence low rate of energy delivery of the laser in addition to the low maximum treatments used for large weeds. In large-scale crop production environments, even low weed densities of 5 plants m−2 (Llewellyn et al., 2016) dictate that per-weed treatment times must be significantly lower than the 15 and 60 s durations that were found to control three-leaf stage annual ryegrass. These results and this requirement for large-scale systems suggest that (1) more energy is required for control of larger weeds and (2) if lasers are to be relevant in large-scale production systems, laser power must be sufficient to deliver the energy required in a short duration. The present study used energy treatments that were too low for the large weeds tested, with future research seeking to increase total energy delivered. Concerning laser power, it appears that the lethal temperature of thermal control options is inversely related to the exposure time (Sutcliffe, 1977; Ascard et al., 2007), where the rate of delivery does not seem to impact the efficacy of laser weeding. For example, Wöltjen et al. (2008) observed no difference in energy requirement for control of barnyard grass between a 500 W CO2 laser and a 250 W diode laser. It should be noted that previous results have suggested that laser wavelength is an important factor (Kaierle et al., 2013) in the efficiency of control of seedling weeds whereby this comparison used two distinct laser wavelengths. Thus, it remains unclear as to whether a rapid energy delivery rate from a high-power laser would result in adequate heat movement through the larger weeds or simply pyrolyze surface tissue, with char acting as an insulator protecting the plant from further damage. Similar insulating effects of char are used for fire safety practices in buildings (White and Dietenberger, 2010).
The spot size of 5 mm chosen for the present study was based on findings in Marx et al. (2012) and Kaierle et al. (2013). Studies incorporating larger spot sizes and larger ranges of energy densities would be necessary for future research in determining the importance of spot size in controlling large weeds. Further, incorporating adaptive optics techniques that could change the spot size in the field depending on target weed size and environment could help enable smarter targeting of weeds by size.
The present study represents the first investigation of annual ryegrass, and to the best of our knowledge is the first evaluation of lasers for control of grass weeds larger than the four-leaf growth stage. Annual ryegrass was controlled at the highest energy levels at both the three- and seven-leaf growth stages, with larger plants less sensitive to the energy treatments indicating a strong growth stage and plant size effect on efficacy. The variability in efficacy was found to increase with lower doses, indicating consistent levels of weed control requires delivery of larger quantities of energy. A more detailed analysis of temperature changes and heat movement in both the plant and soil at each energy dose may provide some answers on variability and targeting. Further investigation is required to determine the practicality and energy requirements needed for consistent control of tillering and mature annual ryegrass. The use of more powerful lasers would increase the practicality of energy delivery by reducing the required treatment times. The targeted nature of lasers, coupled with advancements in the precision of weed recognition, are offering new opportunities for weed control tools.
Data Availability Statement
The raw data supporting the conclusions of this article will be made available by the authors, without undue reservation.
Author Contributions
GC and CB: conceptualization, methodology, analysis, and writing. CS: conceptualization, methodology, and reviewing. SL-S: conceptualization, reviewing, and editing. MW: conceptualization, methodology, reviewing, and editing. All authors contributed to the article and approved the submitted version.
Funding
This project was provided by the Grains Research and Development Corporation (GRDC) grants Innovative crop weed control for northern region cropping systems (US00084), and Intelligent robotic non-chemical weeding (UOS1806-002AWX).
Conflict of Interest
The authors declare that the research was conducted in the absence of any commercial or financial relationships that could be construed as a potential conflict of interest.
Acknowledgments
The authors would like to thank Alex Shaw at the Sydney Informatics Hub for assistance in data analysis and Linda Heuke and Shona Robilliard at the I.A. Watson International Grains Research Center for help with planting, maintaining, and harvesting the pot trials.
References
Ascard, J., Hatcher, P. E., Melander, B., and Upadhyaya, M. K. (2007). “Thermal weed control,” in Non-Chemical Weed Management: Principles, Concepts and Technology, eds M. K. Upadhyaya and R. E. Blackshaw (Wallingford: CABI), 155–175. doi: 10.1079/9781845932909.0155
Bajwa, A. A., Mahajan, G., and Chauhan, B. S. (2015). Nonconventional weed management strategies for modern agriculture. Weed Sci. 63, 723–747. doi: 10.1614/WS-D-15-00064.1
Bauer, M. V., Marx, C., Bauer, F. V., Flury, D. M., Ripken, T., and Streit, B. (2020). Thermal weed control technologies for conservation agriculture—a review. Weed Res. 60, 241–250. doi: 10.1111/wre.12418
Bayramian, A., Fay, P. K., and Dyer, W. E. (1992). “Weed control using carbon dioxide lasers,” in Proceedings of the Western Society of Weed Science, ed R. G. Lym (Salt Lake City: WSWS), 55–56.
Champ, J., Mora-Fallas, A., Goëau, H., Mata-Montero, E., Bonnet, P., and Joly, A. (2020). Instance segmentation for the fine detection of crop and weed plants by precision agricultural robots. Appl. Plant Sci. 8:e11373. doi: 10.1002/aps3.11373
Coleman, G. R. Y., Stead, A., Rigter, M. P., Xu, Z., Johnson, D., Brooker, G. M., et al. (2019). Using energy requirements to compare the suitability of alternative methods for broadcast and site-specific weed control. Weed Technol. 33, 633–650. doi: 10.1017/wet.2019.32
Couch, R., and Gangstad, E. (1974). Response of waterhyacinth to laser radiation. Weed Sci. 22, 450–453. doi: 10.1017/S0043174500037991
Duke, S. O. (2012). Why have no new herbicide modes of action appeared in recent years? Pest Manag. Sci. 68, 505–512. doi: 10.1002/ps.2333
Heap, I. M. (2020). The International Survey of Herbicide Resistant Weeds. Available online at: http://www.weedscience.com (accessed November 12, 2020).
Heisel, T., Schou, J., Christensen, S., and Andreasen, C. (2001). Cutting weeds with a CO2 laser. Weed Res. 41, 19–29. doi: 10.1046/j.1365-3180.2001.00212.x
Kaierle, S., Marx, C., Rath, T., and Hustedt, M. (2013). Find and irradiate - lasers used for weed control. Laser Tech. J. 10, 44–47. doi: 10.1002/latj.201390038
Kassam, A., Friedrich, T., Derpsch, R., Lahmar, R., Mrabet, R., Basch, G., et al. (2012). Conservation agriculture in the dry mediterranean climate. F. Crop. Res. 132, 7–17. doi: 10.1016/j.fcr.2012.02.023
Kristensen, L., Olsen, J., and Weiner, J. (2008). Crop density, sowing pattern, and nitrogen fertilization effects on weed suppression and yield in spring wheat. Weed Sci. 56, 97–102. doi: 10.1614/WS-07-065.1
Lemerle, D., Cousens, R. D., Gill, G. S., Peltzer, S. J., Moerkerk, M., Murphy, C. E., et al. (2004). Reliability of higher seeding rates of wheat for increased competitiveness with weeds in low rainfall environments. J. Agric. Sci. 142, 395–409. doi: 10.1017/S002185960400454X
Lemerle, D., Verbeek, B., and Orchard, B. (2001). Ranking the ability of wheat varieties to compete with Lolium rigidum. Weed Res. 41, 197–209. doi: 10.1046/j.1365-3180.2001.00232.x
Llewellyn, R., Ronning, D., Ouzman, J., Walker, S., Mayfield, A., and Clarke, M. (2016). Impact of Weeds in Australian Grain Production: The Cost of Weeds to Australian Grain Growers and the Adoption of Weed Management and Tillage Practices. Canberra: GRDC.
López-Granados, F. (2011). Weed detection for site-specific weed management: mapping and real-time approaches. Weed Res. 51, 1–11. doi: 10.1111/j.1365-3180.2010.00829.x
Lottes, P., Behley, J., Milioto, A., Stachniss, C., Chebrolu, N., Milioto, A., et al. (2020). Robust joint stem detection and crop - weed classification using image sequences for plant - specific treatment in precision farming. J. F. Robot. 37, 20–34. doi: 10.1002/rob.21901
Marx, C., Barcikowski, S., Hustedt, M., Hustedt, S., Haferkamp, H., Rath, T., et al. (2012). Design and application of a weed damage model for laser-based weed control. Biosyst. Eng. 113, 148–157. doi: 10.1016/j.biosystemseng.2012.07.002
Mathiassen, S. K., Bak, T., Christensen, S., and Kudsk, P. (2006). The effect of laser treatment as a weed control method. Biosyst. Eng. 95, 497–505. doi: 10.1016/j.biosystemseng.2006.08.010
R Core Team (2019). R: A Language and Environment for Statistical Computing (version 3.6.1). Available online at: https://www.r-project.org/
Ritz, C., Baty, F., Streibig, J. C., and Gerhard, D. (2015). Dose-response analysis using R. PLoS ONE 10:e0146021. doi: 10.1371/journal.pone.0146021
RStudio Team (2015). RStudio: Integrated Development Environment for R (Version 1.1.456). Available online at: http://www.rstudio.com/
Schou, J., Heisel, T., Nordskov, A., Christensen, S., Jensen, P. S., Thestrup, B., et al. (2002). “Quantitative laser cutting of plants,” in International Symposium on High-Power Laser Ablation 2002, ed C. R. Phipps (Taos, NM: SPIE), 9. doi: 10.1117/12.482143
Walsh, M., Newman, P., and Powles, S. (2013). Targeting weed seeds in-crop: a new weed control paradigm for global agriculture. Weed Technol. 27, 431–436. doi: 10.1614/WT-D-12-00181.1
Wang, A., Zhang, W., and Wei, X. (2019). A review on weed detection using ground-based machine vision and image processing techniques. Comput. Electron. Agric. 158, 226–240. doi: 10.1016/j.compag.2019.02.005
White, R. H., and Dietenberger, M. A. (2010). “Chapter 18 - fire safety of wood construction,” in Wood Handbook - Wood as an Engineering Material, ed. J. R. Ross (Madison, WI: Forest Products Laboratory), 18-1–18-22.
Wickham, H. (2016). ggplot2: Elegant Graphics for Data Analysis (Version 3.3.0). New York, NY: Springer-Verlag. Available online at: https://ggplot2.tidyverse.org
Wöltjen, C., Haferkamp, H., Rath, T., and Herzog, D. (2008). Plant growth depression by selective irradiation of the meristem with CO2 and diode lasers. Biosyst. Eng. 101, 316–324. doi: 10.1016/j.biosystemseng.2008.08.006
Keywords: laser weeding, site-specific weed control, annual ryegrass, fiber laser, growth stage
Citation: Coleman G, Betters C, Squires C, Leon-Saval S and Walsh M (2021) Low Energy Laser Treatments Control Annual Ryegrass (Lolium rigidum). Front. Agron. 2:601542. doi: 10.3389/fagro.2020.601542
Received: 01 September 2020; Accepted: 10 December 2020;
Published: 14 January 2021.
Edited by:
Lauren M. Lazaro, Louisiana State University Agricultural Center, United StatesReviewed by:
Simerjeet Kaur, Punjab Agricultural University, IndiaVijay Singh, Virginia Tech, United States
Copyright © 2021 Coleman, Betters, Squires, Leon-Saval and Walsh. This is an open-access article distributed under the terms of the Creative Commons Attribution License (CC BY). The use, distribution or reproduction in other forums is permitted, provided the original author(s) and the copyright owner(s) are credited and that the original publication in this journal is cited, in accordance with accepted academic practice. No use, distribution or reproduction is permitted which does not comply with these terms.
*Correspondence: Guy Coleman, guy.coleman@sydney.edu.au