- Department of Animal Science, Luiz de Queiroz College of Agriculture, University of São Paulo, Piracicaba, Brazil
Feeding a liquid diet to the newborn calf has considerable implications for developing the intestinal microbiota, as its composition can shift the population to a highly adapted microbiota. The present work evaluated 15 Holstein calves individually housed and fed one of the three liquid diets: I – whole milk (n = 5), II – milk replacer (22.9% CP; 16.2% fat; diluted to 14% solids; n = 5) and III – acidified whole milk to pH 4.5 with formic acid (n = 5). All animals received 6 L of liquid diet, divided into two meals, being weaned at week 8 of life. Calves also had free access to water and starter concentrate. After weaning, all calves were grouped on pasture, fed with starter concentrate, and hay ad libitum. The fecal samples were collected at birth (0) and at weeks 1, 2, 4, 8, and 10 of life. The bacterial community was assessed the through sequencing of the V3-V4 region of the 16S rRNA gene on the Illumina MiSeq platform and analyzed using the DADA2 pipeline. Diversity indices were not affected by the liquid diets, but by age (P < 0.001) with weeks 1 and 2 presenting lower diversity, evenness, and richness values. The bacterial community structure was affected by diet, age, and the interaction of these factors (P < 0.01). Twenty-eight bacterial phyla were identified in the fecal samples, and the most predominant phyla were Firmicutes (42.35%), Bacteroidota (39.37%), and Proteobacteria (9.36%). The most prevalent genera were Bacteroides (10.71%), Lactobacillus (8.11%), Alloprevotella (6.20%). Over the weeks, different genera were predominant, with some showing significant differences among treatments. The different liquid diets altered the fecal bacterial community during the pre-weaning period. However, differences in the initial colonization due to different liquid diets are alleviated after weaning, when animals share a common environment and solid diet composition.
Introduction
The intestinal microbiota is essential for the stable development of the gastrointestinal tract (GIT) and a healthy immune system (Czarnecki-Maulden, 2008). Nutrition and management, among others, can affect the GIT microbiota of newborn calves (Malmuthuge et al., 2015). Nutrition is the most influential factor in pre-weaned calves' gut bacterial community (Klein-Jöbstl et al., 2014; Guzman et al., 2015). Indeed, the composition of the diet offered to calves affects the gut microbiota structure because it provides different substrates for the growth of bacterial communities (Maslowski and Mackay, 2011; Li et al., 2012; Kasparovska et al., 2016). However, most studies that evaluated the effects of feeding management on the calves' gut microbiota have focused on the impact of a solid diet (Callaway et al., 2010; Shanks et al., 2011; Petri et al., 2013a; Dias et al., 2018), and little information is available on the effect of liquid diet composition on gut microbial composition (Górka et al., 2011; Edrington et al., 2012; Deng et al., 2017).
The main liquid diet offered to pre-weaned dairy calves in Brazilian and Canadian dairy herds is whole milk (Vasseur et al., 2010; dos Santos and Bittar, 2015), while milk replacer is mainly used in USA (United States Department of Agriculture, 2014). Other liquid diets include waste milk, pasteurized waste milk, transitional milk, or acidified milk (milk replacer, waste milk, or whole milk; Vasseur et al., 2010; United States Department of Agriculture, 2014; dos Santos and Bittar, 2015). The liquid diet is commonly offered for 60–90 days after birth, but in some cases, calves are weaned after 90 days.
Whole milk feeding has considerable implications for gut microbiota development and selection for a highly adapted intestinal microbiota dominated by Bifidobacterium (Kelly et al., 2016). These bacteria have probiotics effects on gut development and the prevention of dysbiosis (Hidalgo-Cantabrana et al., 2018). Probiotic is defined as a living microorganism that promotes a benefit to host's health (Hill et al., 2014). Various milk constituents, such as oligosaccharides and glycoconjugates, selectively enrich this type of microorganism (Pacheco et al., 2015). In humans, newborns fed on breast milk compared to infant formula have a gut microbiota with a profound relationship to neonatal enterocyte genes that influence host protection and development (Schwartz et al., 2012). Also, formula-fed babies have more significant colonization by Clostridium spp. and particularly C. difficile (Penders et al., 2005, 2006; Vael and Desager, 2009), microorganisms associated with various diseases, including diarrhea (Dial, 2004; Poutanen, 2004; Lees et al., 2020).
In many dairy herds, especially those that have ad libitum feeding, the liquid diet is acidified with organic acids up to pH 4.2–4.5 to maintain its microbiological quality throughout the day (Todd et al., 2017). This type of liquid diet is an alternative for feeding dairy calves and has been studied as a simple and low-cost method (Yanar et al., 2006). Acidification can modulate the gut microbiota, retarding growth, or eliminate pathogenic microorganisms (Richard et al., 1988; Jaster et al., 1990), sensitive to lower pH values. Besides, acidification can favor the growth of beneficial microorganisms, such as Lactobacillus, Bifidobacterium, and Faecalibacterium (Deng et al., 2017), that will compete for nutrients with pathogenic microorganisms.
The use of commercial formulas (milk replacer) can be an option to replace whole milk, either for economic reasons, for consistency in the liquid diet composition, or for the opportunity to increase the solids content and consequently the performance of calves. Many studies have shown that milk replacers may be suitable to replace whole milk, ensuring animal performance, as long as they have an adequate composition as to the sources and levels of nutrients (Cooper and Watson, 2013; Bittar et al., 2018; Badman et al., 2019; Bai et al., 2020). The various ingredients used in the milk replacer composition, such as dairy products or vegetable origin products (Bittar et al., 2018), can affect the gastrointestinal microbiome (Badman et al., 2019).
Some studies have used fecal sampling to study the gut microbial community in dairy calves (Uyeno et al., 2010; Oikonomou et al., 2013; Badman et al., 2019), especially for ease of sampling, and because it is a non-invasive method, allowing repeated sampling (Claesson et al., 2017). However, due to the microbial community's difference between the mucosa and digesta, and differences along the intestinal tract, the fecal microbial community should not be used to represent the entire gut microbiota (Tang et al., 2020). It is more accepted as a proxy for the distal gut microbiota (Claesson et al., 2017).
This study aimed to compare the fecal bacterial community of calves fed different liquid diets (whole milk, acidified whole milk, and milk replacer) in the pre- and post-weaning phase. We have investigated the fecal bacterial community of dairy calves using 16s rRNA amplicon sequencing as a non-invasive proxy for the intestinal bacterial community in fifteen young dairy calves. We hypothesized that feeding different liquid diets can cause fecal bacterial community changes during the pre-weaning period, and that these changes are persistent after weaning.
Materials and Methods
Animals, Facilities, and Feeding System
This study was conducted at the calf facilities of the Department of Animal Science, Luiz de Queiroz College of Agriculture, University of São Paulo, located in Piracicaba – São Paulo, Brazil. All animal procedures followed the guidelines recommended by the Animal Care and Use Committee (Protocol no. 2018.5.586.11.7).
This study was part of a performance study with 36 newborn Holstein calves (male calves, birth weight of 32.84 ± 1.54 kg; and females, 28.22 ± 0.81 kg), blocked according to sex, age, and birth weight and evaluated in a randomized block design in the pre-weaning period for performance and health. Previous results were published in the abstract by Coelho et al. (2020a). For the present study, 15 calves were assigned to 5 blocks so that each block had 3 calves with similar sex, age, and BW at the beginning of the experiment. Then, three male blocks (birth weight 28.52 ± 0.91 kg), and two female blocks (birth weight 35.89 ± 2.24 kg), were used to assess the impact of different liquid diets on the fecal bacterial community.
All animals were fed 10% of birth-weight of high-quality colostrum (>50 g IgG/L) within the first 6 h of life (Godden, 2008). All calves had serum protein above 5.5 g/dL at 48 h of life, as recommended by Elsohaby et al. (2019). From the second day of life, each calf within each block was fed one of the three evaluated liquid diets: 1 - Whole milk (WM); 2 - Acidified whole milk (AWM); and 3 - Milk replacer (MR; Sprayfo Azul, Sloten from Brazil Ltda, SP, Brazil) diluted to 14% solids. The composition of the MR and whole milk are described in Table 1.
The total volume of whole milk was collected daily in the milking parlor and divided into two portions, one part was immediately refrigerated, and the other was acidified. The pH was measured with a pH-meter (Tecnal, SP, Brazil) during the acidification process, which was stopped when pH reached 4.5. The acidification was done with the milk at 5°C to avoid the formation of protein clots, with the addition of formic acid (formic acid 85%, Dinâmica Química Contemporânea Ltda, SP, Brazil). The milk was acidified at least 12 h before feeding and kept at room temperature. Because the pH dropped during storage, it was corrected back to 4.5 by adding whole milk before being supplied to the calves. All liquid diets were heated to 38–40°C before feeding. Liquid diets were supplied from 2 d of life till weaning, 3 L of liquid diet was individually offered twice daily (7 and 17 h). Calves were trained from 2 d of life to drink milk from an open bucket. At 57 d of age, the weaning process was initiated in the morning by reducing the total daily liquid diet supply by 1 L every day until complete weaning at 62 d.
Calves Housing
Immediately after birth, the calves were housed in individual suspended cages (113 × 140 cm) with sawdust beds in a ventilated barn, where they remained until 15 d of life. At 2 d, the calves had free access to water and starter concentrate in open buckets located in the cage's front. From 16 d until the end of weaning at 62 d, the calves were individually housed outdoors in a wood shelter with free access to water and starter concentrate. The commercial starter concentrate (Bezerra Ag Milk Agroceres Multimix Animal Nutrition Ltda., Rio Claro, SP, Brazil) was offered daily ad libitum, always after supplying the liquid diet. The composition of starter concentrate is described in Table 1.
After weaning, calves were grouped on pasture, with free access to water, the same starter concentrate, and hay ad libitum. The animals were followed up to 70 days of age when the study ended.
Animal Health and Measurement
The individual consumption of the liquid diet and the starter concentrate, was measured daily. Calves were weighed at birth and weekly until week 8 on a mechanical scale (ICS-300, Coimma Ltda., Dracena, SP, Brazil), always before morning feeding. Average daily gain (ADG) and feed efficiency (kg of BW gain/kg of total DMI) were calculated for the pre-weaning period (0–56 d).
The fecal score was monitored daily, as described by Larson et al. (1977), based on the fluidity of feces: (1) normal and firm; (2) soft; (3) aqueous; (4) fluid. Diarrhea was considered when the calves had a fecal score ≥ of 3 for more than 1 day. Calves with a score ≥ of 3 received oral rehydration solution1 in a volume of 8% of body weight, 2 h after morning feeding, with a bottle until the fecal score returned to normal. Calves' rectal temperature was measured daily using a digital thermometer, and fever was considered when the calf had more than 39.4°C. Health problems were monitored and treated according to veterinary recommendations.
Evaluation of Bacterial Community
Fecal Samples Collections
Fecal samples were collected at day 0 (±1 h after birth, before colostrum feeding) and at days 7 (S1), 14 (S2), 28 (S4), 56 (S8, weaning), and 70 (S10, post-weaning). The samples were collected manually with gloves, directly from the animals' rectum, and the gloves were discarded at each collection to avoid cross-contamination among samples. About 2 g of feces were collected, placed in sterile tubes, and immediately frozen at −20°C.
DNA Extraction, Library Preparation, and Sequencing
DNA extraction from fecal samples was performed with the QIAamp® Fast DNA Stool Minikit extraction (Qiagen, Hilden, Germany), following the modifications suggested by Yu and Morrison (2004). The quality of the DNA samples was evaluated by electrophoresis on 0.8% agarose gel and concentrations were quantified with a spectrophotometer (NanoDrop® ND-2000; Thermo Fisher Scientific, Wilmington, DE, USA).
The libraries were prepared following Illumina's recommendations. The primers used for locus-specific amplification of bacteria flank the V4 region. Overhang sequence of adapters is included in locus-specific primers. Illumina adapter sequences, which were hybridized with the immobilized sequences on the sequencing sheet, were:
Forward overhang: 5′-TCGTCGGCAGCGTCAGATGTGTATAAGAGACAG-[locus-specific sequence]
Reverse overhang: 5′-GTCTCGTGGGCTCGGAGATGTGTATAAGAGACAG-[locus-specific sequence]
The first PCR was performed for locus-specific amplification. Then, AMPure XP beads were used to purify the PCR reaction, and the size of the fragments generated in the PCR reaction was evaluated by agarose gel electrophoresis. The second PCR was performed to connect the barcodes of the Nextera XT kit, and new steps for purifying the PCR and validating the libraries were performed. Subsequently, the libraries were quantified so that all samples/libraries were joined in an equimolar manner in a single pool.
A heterogeneous control, the phage phi-X, was combined with the amplicon pool to introduce complexity to the sequencing. Finally, the libraries and phi-X have been denatured to allow sequencing. Sequencing was performed in Illumina Miseq system (Illumina, San Diego, CA, USA) and produced readings were 2 × 250 bp. All raw DNA sequence reads were deposited in NCBI's Sequence Read Archive under BioProject PRJNA639165, submission SUB7576848.
Bioinformatic Analyses
The data were analyzed as a previously published pipeline (Callahan et al., 2016b), using a set of packages implemented in the R language2 (R Core Team) and available through the BioConductor project (Gentleman et al., 2004; Huber et al., 2015).
First, multiplexed readings were assigned to biological samples. The DADA2 program (Callahan et al., 2016a), an open-source package implemented in the R language, was used to model and correct amplicon errors without building OTUs. Callahan et al. (2016a) show that DADA2 identified more real variants in several simulated communities and produced fewer spurious sequences than other methods. The DADA2 package has a complete pipeline implemented to transform the sequencer fastq files into sequences of inferred, dismembered samples and without chimeras.
The filtering of fastq files was performed to cut the PCR primers' sequences and filter the 3′ends of the readings due to the quality decay (Q < 30), but maintaining the overlap for later joining of the readings and reassembly of the fragment of the V4 region. The DADA2 algorithm uses a parametric error model, and each set of amplicon data has a different set of error rates.
After the initial processing of the sequencing data by DADA2, taxonomies were assigned to each ASV (Amplicon Sequencing Variants) using an implementation of the DADA2 program of the naive Bayesian classifier method for this purpose (Wang et al., 2007). The assignTaxonomy function takes as input a set of sequences (ASVs) to be classified, and a set of training of reference sequences with known and assigned taxonomies. The SILVA database was used as a reference (Glöckner et al., 2017).
The taxonomic classifications generated by DADA2, and their quantifications, were imported into the phyloseq program (McMurdie and Holmes, 2013), also implemented in R. The α and β diversity analyses were performed with the phyloseq package, as described in Callahan et al. (2016b). For the β-diversity analysis, a multivariate permutational analysis of variance (PERMANOVA) was performed, using weighted UniFrac distances, testing the treatment effect, week, and interaction. ASVs that have not been classified until the family level were filtered, and ASVs marked as being the same species have been clustered. After applying these filters, the tables of gross abundance and relative abundance counts were obtained.
Then, the taxonomic counts in the phyloseq object were imported into the edgeR package (Robinson et al., 2010) to normalize the sizes of each sample's libraries (Robinson and Oshlack, 2010), subsequently the counts were transformed to the base 2 logarithms of the counts per million (log CPM) of each sample (voom transformation; Law et al., 2014). These transformations allow the linear models implemented in the limma package (Ritchie et al., 2015) to analyze differential abundance. Finally, after adjusting the linear model with limma, the differential taxonomic abundance was tested for each contrast (pair of treatments) with moderate t-tests (Smyth, 2004).
Statistical Analysis
The experimental design was a randomized block design, with the animals allocated in the blocks according to birth weight, age, and sex. Before model construction, the normality of residues for all variables was verified by the Shapiro -Wilk test using the PROC UNIVARIATE procedure of SAS 9.4 (SAS Inst. Inc., Cary, NC). Average daily gain (ADG), starter concentrate intake, consumption of the liquid diet, feed efficiency, and rectal temperature were analyzed as time repeated measures, using the MIXED procedure of SAS (version 9.4, SAS Institute Inc., Cary, NC), according to model: Yijk = μ + Ti + Bj + Eij + Wk + TWik + Eijk, where, Yijk = variable response; μ = general mean; Ti = fixed effect of treatment (liquid diets); Bj = random block effect; Eij = residual error A; Wk = fixed age effect (weeks); TWik = fixed effect of interaction treatment and age; Eijk = residual error B. The covariance structures “compound symmetry, heterogeneous compound symmetry, autoregressive, heterogeneous autoregressive, unstructured, banded, variance components, toeplitz, antidependence and heterogeneous toeplitz” were tested and defined according to the lowest value obtained for “Akaike's Information Criterion corrected” (AICC).
The variable cumulative days per calf affected by diarrhea and days in fever were analyzed by “Restricted Maximum Likelihood” ANOVA for a randomized complete block design (RCBD) using PROC MIXED. As the days with diarrhea was not normally distributed, its analysis was performed based on log-transformed data. If significance was detected by ANOVA F test, the Student's T-test was assessed for the comparison among the means. The fixed variables were evaluated using the following statistical model: Yji = μ + Ti + bj + eij, where μ = general mean; Ti = fixed effect of treatment (liquid diets); bj = random block effect; and eij = residual error.
Kaplan-Meier survival curves were plotted for cumulative diarrhea events for WM, MR, and AWM groups using SAS 9.4 (SAS Inst. Inc., Cary, NC). The endpoint of interest was survival time, which was defined as the time to first diarrhea event (fecal score ≥ 3; 1 to 4-point scale) or the end of the study in days. For this, a data set was organized containing the variables treatment (WM, MR, and AWM), time (representing the disease-free survival time), and status (censoring indicator, with the value 1 indicating an event time and the value 0 indicating a censored time). The STRATA command of SAS 9.4 (SAS Inst. Inc., Cary, NC) was used to test the null hypothesis between different survival curves according to the log-rank (Mantel-Cox) test. Mantel Haenszel hazard ratio and its confidence interval were performed using the Cox proportional hazard model using the slope of the survival curve. It was conducted to compare the rate of a diarrhea event occurring between the treatments over time. Additionally, Cox proportional hazard model was fitted to compare the rate of diarrhea incidence up to 15 days after enrollment date. For all the analyses, differences detected at P ≤ 0.05 were considered significant.
Results
Feed Intake and Health of Calves
Body weight at birth did not differ among treatments. However, at weaning AWM and WM-fed animals tended to have higher BW when compared to those fed MR (Table 2; P = 0.073). The starter concentrate intake increased with age (Figure 1B; P < 0.001) but was not affected by treatment or by the treatment interaction with age (Table 2). The rectal temperature was higher in the first 4 weeks of life (Figure 1D; P < 0.001), but the values were not indicative of fever, and it was also not affected by the treatment or interaction of both factors (Table 2). The number of days with fever was also not affected by the treatments (Table 2). The consumption of the liquid diet was affected by age and interaction of age and treatments, and tended to be affected by treatment (Table 2; P < 0.001; P = 0.043; P = 0.097, respectively). At week 1, intake was higher for WM and MR than AWM (Figure 1A; P = 0.033), and this effect tended to be observed at week 3 as well (Figure 1A; P = 0.053). The ADG was affected by treatments, age and interaction of both factors (Table 2; P = 0.025; P < 0.001; P = 0.011). In the pre-weaning period, the ADG was variable (Figure 1C), at week 2 and 3 WM animals tended to present greater gain than MR and AWM, respectively (P = 0.069; P = 0.052). At week 4, WM had a greater gain than AWM and MR (P < 0.001), and at weeks 5 and 6, MR-calves had the smallest gain (P = 0.014; P = 0.038, respectively). Feed efficiency was affected by treatments, age and interaction of both factors (Table 2; P < 0.001; P = 0.019; P = 0.050). The efficiency was also variable during the pre-weaning period (Figure 1E). At weeks 2, 3, and 4, WM-fed calves had the greatest feed efficiency (P = 0.031; P = 0.047; P < 0.001, respectively) and MR-fed calves tended to be less efficient than AWM calves at weeks 5 and 6 (P = 0.072; P = 0.054, respectively).
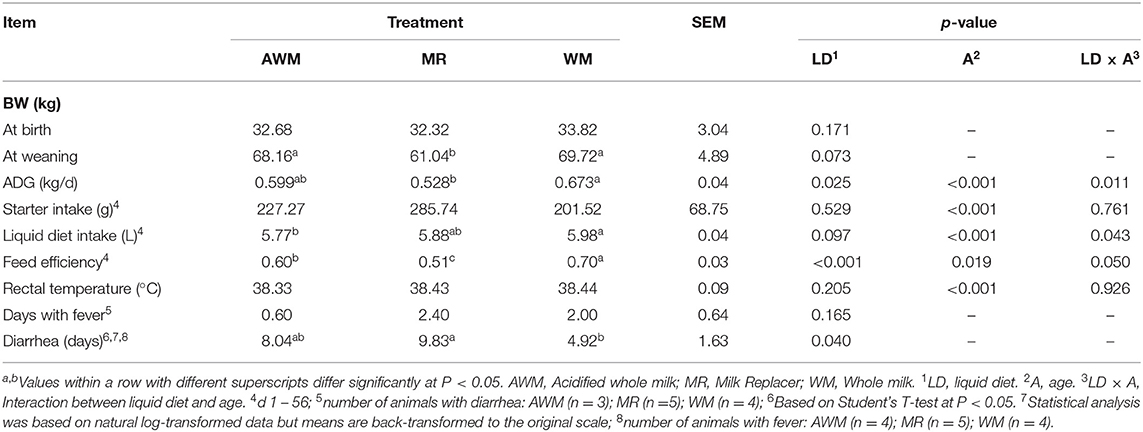
Table 2. Performance, intake and health from birth to weaning of calves fed with different liquid diets.
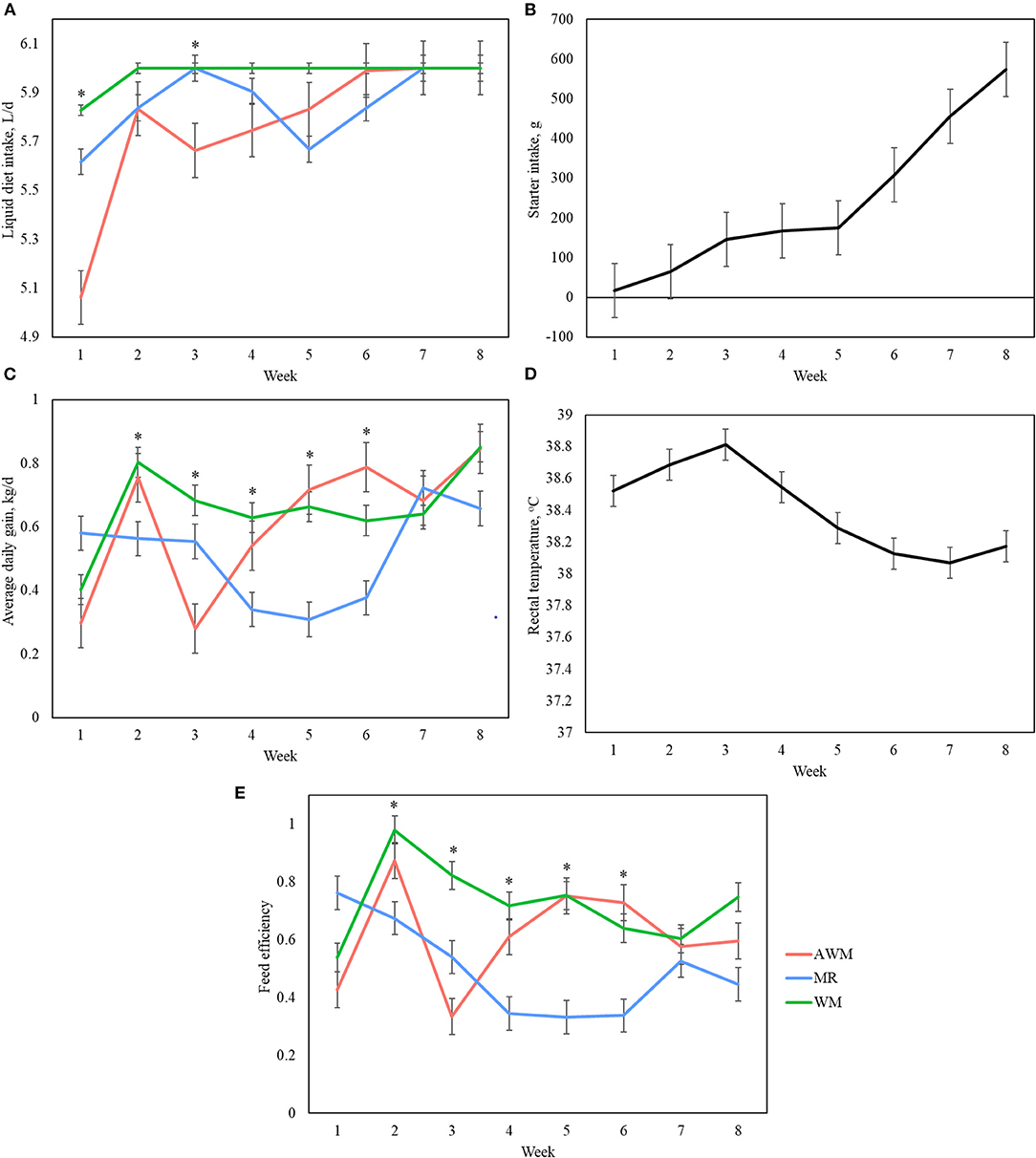
Figure 1. Liquid diet (A) starter intake (B) average daily gain (C) rectal temperature (D) and feed efficiency (E) of dairy calves fed different liquid diets. AWM, Acidified whole milk; MR, Milk replacer; WM, Whole Milk. *Denotes difference among treatments in the respective week.
Number of cumulative days affected by diarrhea was higher for calves fed MR compared to WM, whereas AWM-calves were similar to the two other groups (P = 0.040; Table 2). However, the Log-Rank model's diarrhea incidence during the experimental period did not differ among treatments (Table 3). The median days for 50% of the animals in each group to be diagnosed with diarrhea showed no difference among treatments (Figure 2). However, more MR calves tended to be diagnosed with diarrhea in the first 15 days (P = 0.09; Table 4), as compared to the WM calves.

Table 3. Log-rank (Mantel-Cox) and Mantel Haenszel hazard ratio comparison between the cumulative incidence of diarrhea from birth to weaning in calves fed with different liquid diets.
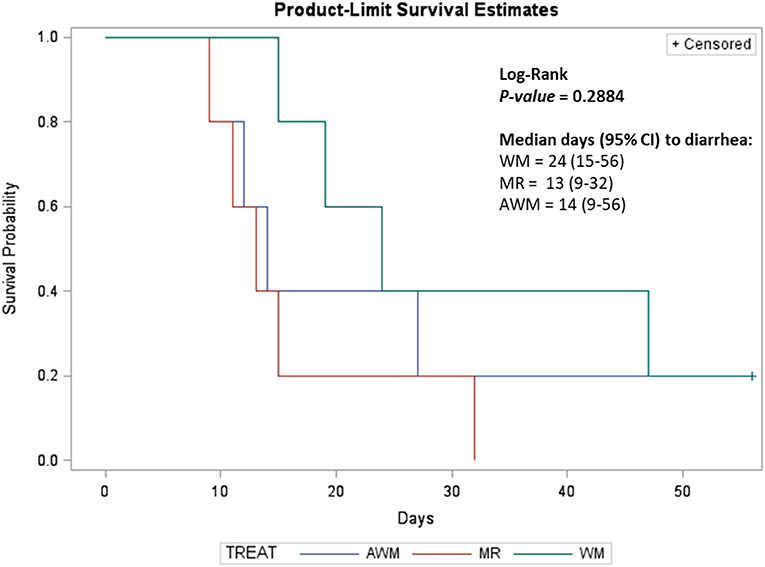
Figure 2. Median days for 50% of animals to be diagnosed with diarrhea in each group of dairy calves fed different liquid diets. AWM, Acidified whole milk; MR, Milk replacer; WM, Whole Milk.
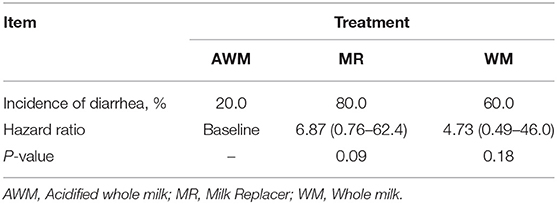
Table 4. Incidence of diarrhea up to 15 days relative to birth date in calves fed with different liquid diets.
Bacterial Community
Sequencing information (Number of raw reads, number of quality-filtered reads, Number ASVs identified) is shown in Supplementary Tables 1, 2.
The microbial profile analysis was performed using data from the amplicon sequence of the 16S rRNA gene. Table 5 shows α-diversity indices. The indices were not affected by different liquid diets. However, all indices were affected by age (P < 0.001), but there was no interaction between the liquid diet and age (Table 5).
Samples collected at birth (0) had greater diversity (Shannon) and richness (Chao1). Weeks 1 and 2 had less diversity (Shannon and Simpson) and evenness (Pielou), and week 1 had less richness (Chao1; Figure 3).
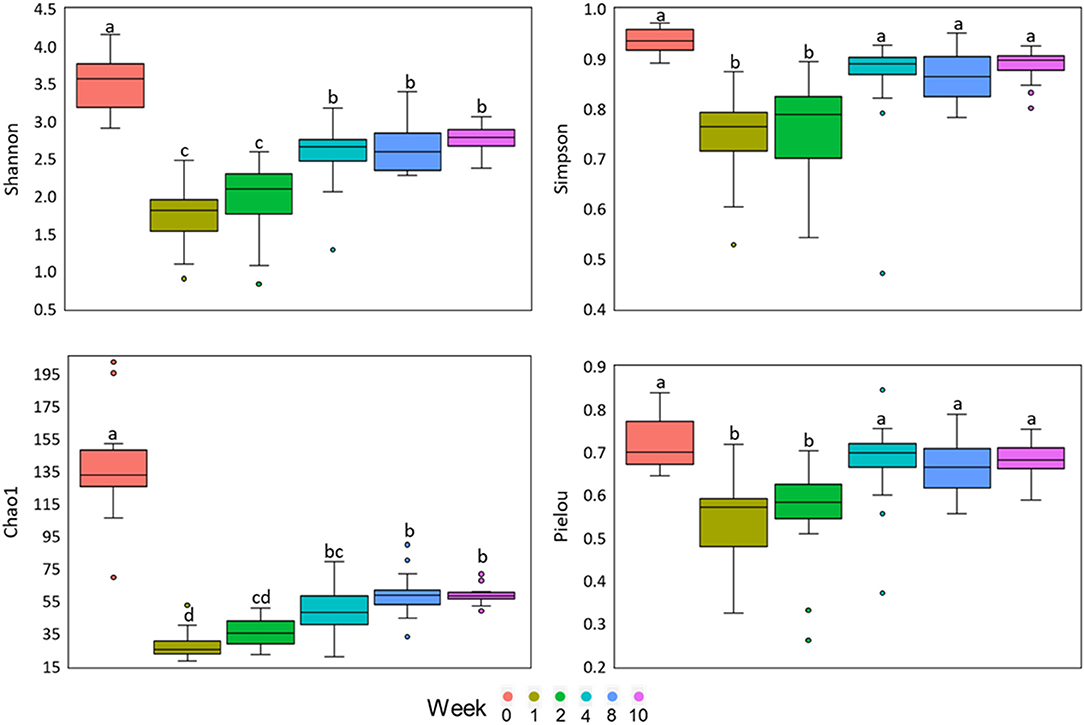
Figure 3. α-diversity indices in fecal samples from dairy calves fed different liquid diets. Data are visualized as box-plots showing the median and the interquartile (midspread) range (boxes containing 50% of all values), the whiskers (representing the 25 and 75 percentiles) and the extreme data points. Letters above boxes indicate significant differences at P > 0.05.
β-diversity was affected by the liquid diet (P = 0.001), the age of the animals (P = 0.001), and also by the interaction of these factors (P = 0.03). While there were dissimilarities in the pre-weaning phase, weaned calves' fecal bacterial community presented similarities in structure (Figure 4).
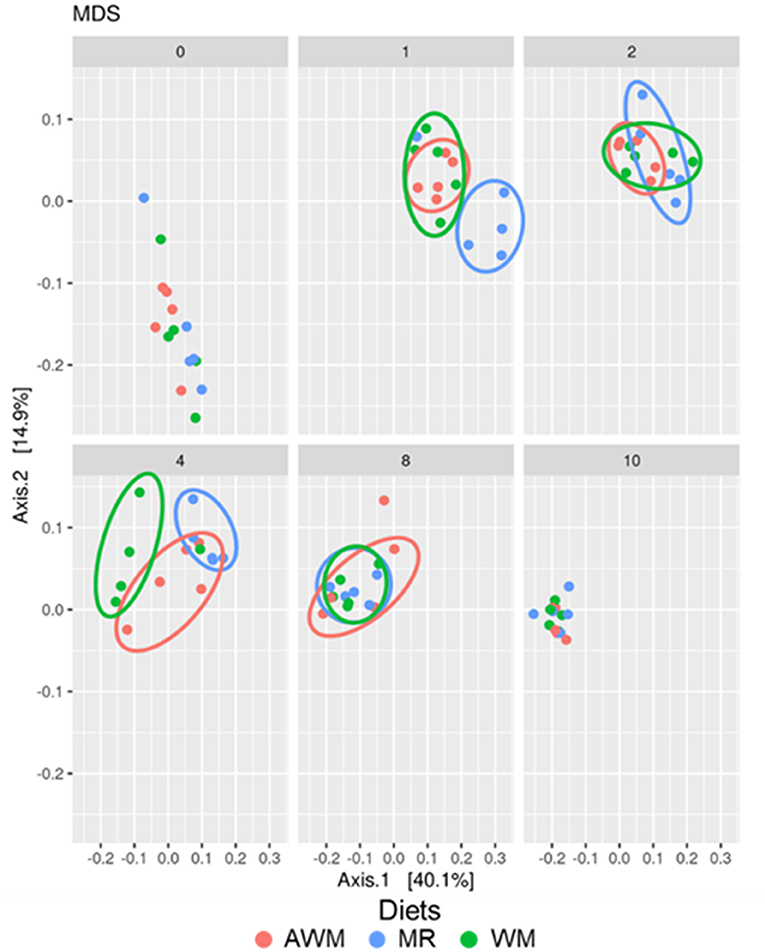
Figure 4. Effect of the interaction of liquid diets with the different ages of the dairy calves on the structure of the microbial community in fecal samples in dairy calves fed different liquid diets. Multidimensional scaling (MDS) showing the weighted UniFrac distance metric. AWM, Acidified whole milk; MR, Milk Replacer; WM, Whole milk.
Twenty-eight bacterial phyla were identified in the fecal samples. In general, the predominant phyla were Firmicutes (42.35%), Bacteroidota (39.37%), Proteobacteria (9.36%), Fusobacteriota (4.08%), and Actinobacteriota (3.02%), corresponding to 98.18% of total (Supplementary Table 3). Abundance of these phyla over the weeks is shown in Figure 5A. Proteobacteria decreased at week 1. Bacteroidota was the most abundant at birth. Firmicutes had the greatest abundance until week 4, when it was surpassed at week 8 by the phylum Bacteroidota.
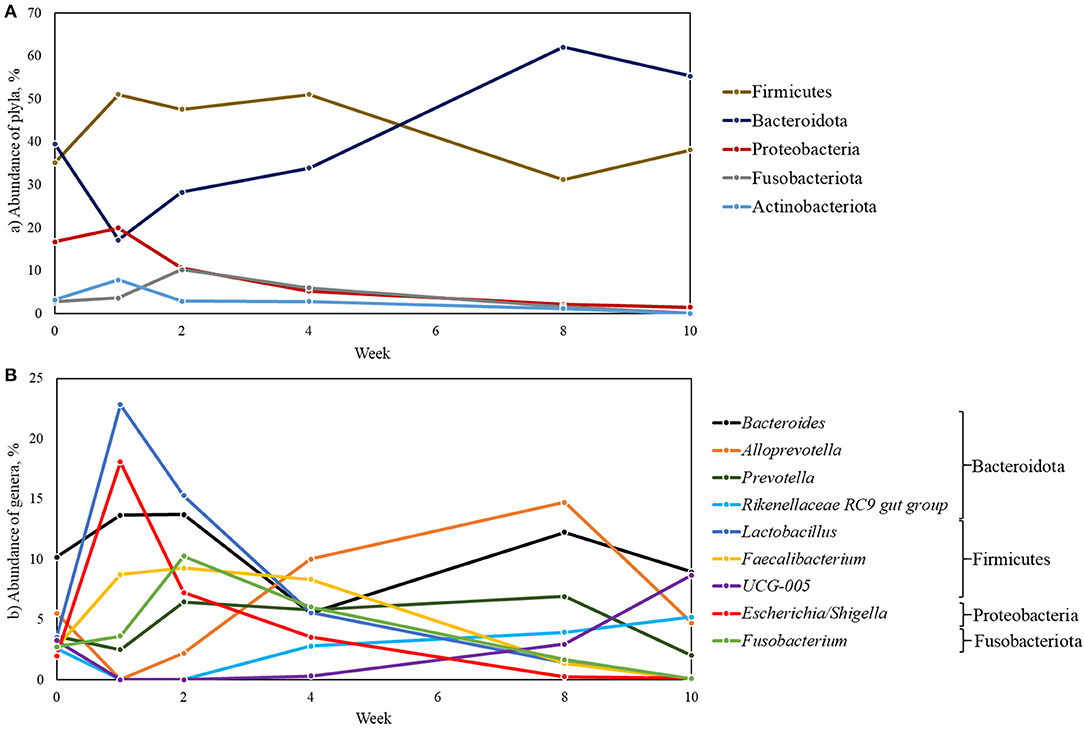
Figure 5. Relative abundance of bacterial phyla (A) and genera (B) in fecal samples during pre- and post-weaning in dairy calves fed different liquid diets.
Of these 28 phyla, 559 bacterial genera were identified. Among these genera, the most prevalent were Bacteroides (10.71%), Lactobacillus (8.11%), Alloprevotella (6.20%), Escherichia/Shigella (5.21%), and Faecalibacterium (5.07%) (Figure 6).
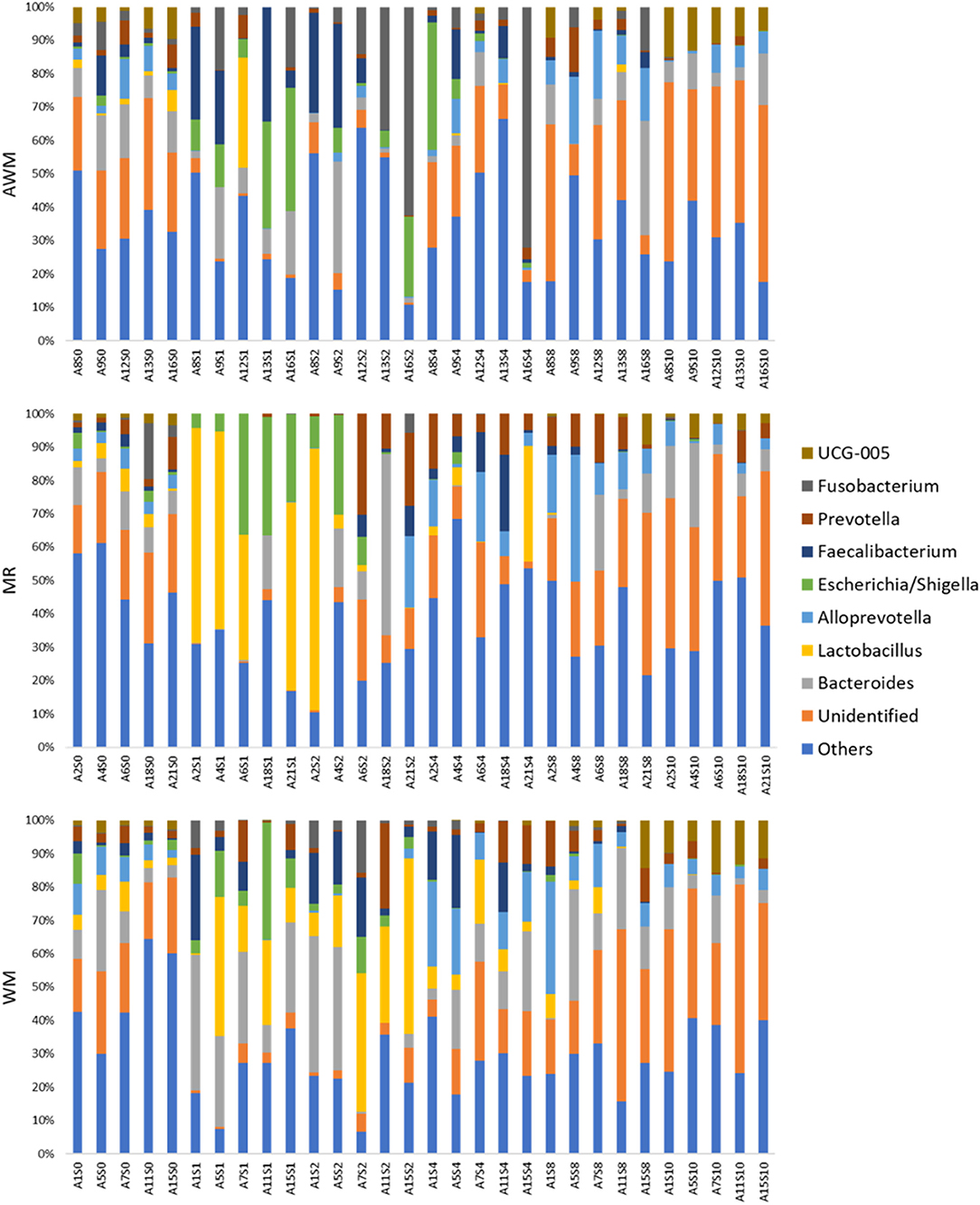
Figure 6. Bacterial relative abundance for the genus level in fecal samples in dairy calves fed different liquid diets. Each bar represents the identification of the analyzed sample: Axx-Sy, where Axx is the animal, Sy, the week in which the collection was performed. AWM, Acidified milk; MR, Milk Replacer; WM, Whole milk.
However, the nine most abundant genera exhibited a distinct abundance profile over time (Figure 5B). At birth, the bacterial community was dominated by the genus Bacteroides (10.17%). At week 1, however, Lactobacillus (22.82%) and Escherichia/Shigella (18.06%) overlapped Bacteroides (13.65%) and were the most abundant genera, reaching their highest values. After week 1, the abundance of Escherichia/Shigella and Lactobacillus decreased considerably until week 10 (0.15 and 0.01%, respectively). Even though its abundance decreased, compared to the previous week, Lactobacillus remained the most abundant genus in week 2, followed by Bacteroides and Fusobacterium (15.29, 13.71, and 10.29%, respectively). At week 4, there was a predominance of the genera Alloprevotella, Faecalibacterium, and Fusobacterium (10.00, 8.33, and 6.02%, respectively). At week 8, Alloprevotella reached its highest abundance value (14.71%), being predominant at this time, and was followed in abundance by Bacteroides and Prevotella (12.24 and 6.81%, respectively). At week 10, there was a decline in abundance values for these three genera. However, Bacteroides remained the most abundant genus (8.94%), followed by UCG-005 and Rikenellaceae RC9 gut group (8.68 and 5.21%, respectively), which reached their highest abundance values at this point. Data for all genera over the weeks are in Supplementary Table 4.
All taxonomic abundance differential data are reported in Supplementary Table 5. Figure 7 shows 15 bacterial genera in a relative abundance of ≥1% on a heat-map. At week 0 and week 10, no genus differed among treatments. Weeks 1 and 4 showed the most significant number of differences among treatments. Alloprevotella was the most abundant at week 8 (Figure 6), and there was no difference among treatments. The differences were evident when Alloprevotella had low abundance (weeks 1 and 2). Bacteroides had higher, while Bifidobacterium had lower abundance in WM than MR at weeks 1 and 4. At week 2, Bifidobacterium was lower with AWM. Butyricicoccus was higher at week 1 for AWM and WM. Collinsella was more abundant at weeks 2, 4, and 8 for WM compared to AWM. Faecalibacterium had lower abundance at weeks 1 and 2 in MR-fed calves. Fusobacterium was less abundant at weeks 1, 2, 4, and 8 in AWM-fed calves compared to MR. Lactobacillus was abundant in WM over the weeks. Parabacteroides, Phascolarctobacterium, Prevotella, Rikenellaceae RC9 gut group, and UCG-005 were less abundant throughout the studied weeks, but these genera were more abundant for WM compared to MR-fed calves.
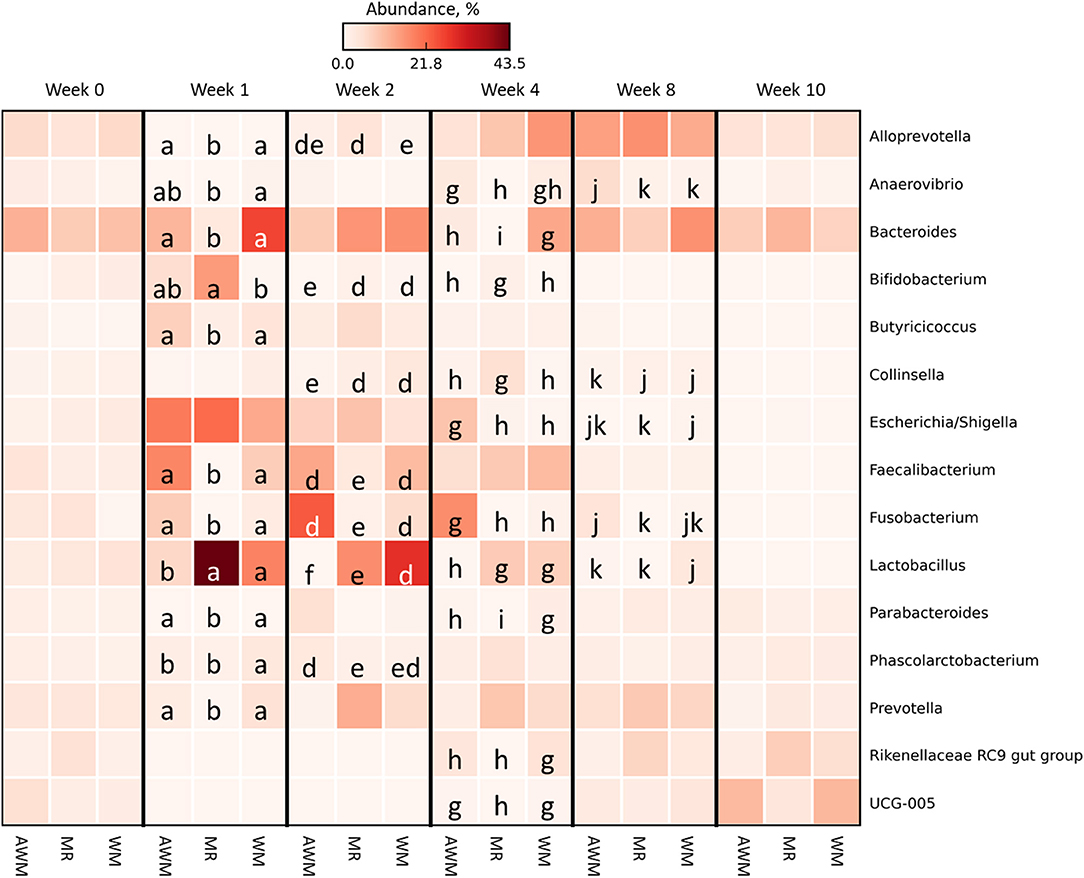
Figure 7. Differential abundance in most abundant bacterial genera in fecal samples of dairy calves fed different liquid diets. Data are visualized as heat-map. Comparisons are among treatments within week. Means followed by the same letter are not significantly different by the t-test (P > 0.05). Genera rows without letters were not significantly different. AWM, Acidified milk; MR, Milk Replacer; WM, Whole milk.
Discussion
Composition of the Fecal Bacterial Community
In the pre-weaning period, the liquid diet is the primary source of energy and protein. When reaching the small intestine, it serves as a substrate for the growth of microorganisms (Górka et al., 2011). Characterizing the gut microbiota in the pre-weaning phase is of great importance to understand host-microbiome interactions (Badman et al., 2019). In our study, the fecal bacterial community was affected by different liquid diets. It is interesting to note that each diet promoted a greater abundance of specific microorganisms throughout the pre-weaning period, which have directly affected animals' performance and health.
Whole milk, especially unpasteurized milk, in human nutrition has been described in several studies as capable of improving intestinal health (Fagnani et al., 2019; Butler et al., 2020), as it promotes the growth of probiotic microorganisms, as Lactobactillus, Faecalibacterium, and Bifidobacterium (Hill et al., 2014). Recent studies have shown that the intake of unpasteurized whole milk increased the abundance of Lactobacillus in the human gut microbiota (Butler et al., 2020). In our study, the consumption of WM was constant in the pre-weaning period, and higher at week 1 than AWM-fed calves. This consumption may have stimulated the genus Lactobacillus in these animals compared to those fed with AWM. Probably, this initial stimulus remained until week 4, when consumption did not differ anymore among treatments, as the genus Lactobacillus remained more abundant with MR compared to AWM. Lactobacillus spp. have been associated with minor infections and diarrheal disorders, in addition to stimulating the mucosal immune system (Abe et al., 1995; Macfarlane et al., 2007). It is interesting to note that the genus Lactobacillus was also present in this period, being the most prevalent in the first 2 weeks of age, probably helping to minimize and control diarrhea. The higher initial prevalence of another beneficial bacteria in feces, Faecalibacterium spp., was associated with a lower incidence of diarrhea in the first 4 weeks of life, and increased average daily gain in calves (Oikonomou et al., 2013). Similarly, in our study, calves fed WM showed a greater abundance of Faecalibacterium at week 1 and a lower incidence of diarrhea, and consequently higher ADG and feed efficiency. Probably, WM feeding provides the necessary substrate for the growth of Faecalibacterium, like acetate (Duncan et al., 2002). Unfortunately, we did not analyze short-chain fatty acids in fecal samples to discuss this point.
Milk acidification can promote the same benefits as whole milk, in addition to making the environment unfavorable for the pathogenic bacteria growth that are sensitive to the lower pH (Deng et al., 2017; Coelho et al., 2020b). Another benefit includes the modulation of the digesta' pH (Coelho et al., 2020b), which possibly can benefit the growth of other beneficial microorganisms. Similar to our findings, Deng et al. (2017) observed a greater abundance of beneficial bacteria in the gut of calves fed with waste milk acidified with formic acid. Although this author did not analyze the fecal score, they did investigate the expression of intestinal mucosa genes, and suggested an improvement in general health conditions. Besides in our work, milk acidification increased the age for the first case of diarrhea when compared to WM (15 vs. 9 days). Yanar et al. (2006) also found similar results when acidifying milk replacer with formic acid. Although use of formic acid on acidification process was acceptable, Zou et al. (2017) observed higher inflammation scores in the jejunum and ileum. In general, studies with acidified milk have shown beneficial results for the calves' health as shown in our results on the bacterial community.
Other bacterial genera are described as commensal and beneficial to the gut environment, such as Alloprevotella, Parabacteroides, and Phascolarctobacterium (production of succinate and acetate, lower inflammatory activity; Sakamoto and Benno, 2006; Watanabe et al., 2012; Li et al., 2018) or Anaerovibrio and Butyricicoccus (improvement of the intestinal barrier; Eeckhaut et al., 2013; Chen et al., 2019). These bacteria were more abundant at weeks 1 and 2 in samples collected from animals fed WM and AWM. The abundance of these genera may indicate the modulating effect of these liquid diets on the gut bacterial community.
The composition of MR can alter the gut microbiota (Badman et al., 2019). Some studies report that MR may contain oligosaccharides that act as feed components with high bioactive potential, which can help establishing a beneficial gut microbiota (Aldredge et al., 2013; Badman et al., 2019). Besides, the presence of vegetable oils in MR can affect the bacterial community. The MR in the present study contained coconut oil and palm oil.3 Recent studies suggest that coconut oil may have a beneficial role in modulating the human bacterial community, increasing Lactobacillus and Bifidobacterium's population (Djurasevic et al., 2018; Rolinec et al., 2020), as observed in our study. However, palm oil may not have the same beneficial effects as coconut oil (Mancini et al., 2015). Palmitic acid, present in palm oil, affects the integrity of the intestinal epithelium, causing an unbalanced immune response and stimulating the production of inflammatory cytokines, favoring a pro-inflammatory bowel condition (Ghezzal et al., 2020). This condition may be related to the greater abundance of Collinsella at weeks 2, 4, and 8. This genus is associated with pro-inflammatory dysbiosis (Candela et al., 2016; Astbury et al., 2020), increasing intestinal permeability, reducing expression of tight junctions in enterocytes, and stimulating gut leakage (Chen et al., 2016). The loss in intestinal integrity has probably favored diarrhea in MR-fed calves leading to lower ADG, efficiency and BW at weaning compared to WM-fed calves.
Other observations regarding the bacterial community were common to the different liquid diets. We have observed in our data that the abundance of the genera Lactobacillus, Bifidobacterium, and Faecalibacteirum decreased with age. Despite their effect on gut development, they were overcome by competition overtime with fiber-degrading bacteria (Dill-McFarland et al., 2017), such as the genera Fibrobacter and Ruminococcus (Supplementary Table 4). The presence of carbohydrate fermenting bacteria, such as Bacteroides during pre-weaning, suggests an increased ability to use complex carbohydrates from the starter including cellulose, hemicellulose, resistant starch, and xylans, which reach the large intestine (Dias et al., 2017; Zhang et al., 2017). Although the AWM and WM-fed calves had greater abundance of Bacteroides than the calves MR-fed, this did not impact the starter concentrate intake.
Before week 10 of life, there was an increase in Prevotella genus' abundance probably due to the increasing starter concentrate intake. This genus has a wide range of metabolic capacities (Petri et al., 2013b; Rubino et al., 2017), as it can use soluble carbohydrates, pectins, proteins, and hemicellulose (Huws et al., 2016). In studies targeting the ruminal bacterial community, Prevotella was shown to be the predominant genus. However, although increasing with time, abundance of Prevotella in our study remained low, confirming the work of Lourenco et al. (2020) in beef calves, which was probably due to low consumption of a solid diet and competition with other genera. Indeed, starting at week 10, the abundance of Prevotella started to drop, probably due to the increase in abundance of Fibrobacter and Ruminococcus and the change from individual to group-housing. In a study with chimpanzees, Amaral et al. (2017) also observed a decrease in the Prevotella genus after the animals had been housed collectively.
α and β-Diversity of the Fecal Bacterial Community
The GIT of newborn calves was traditionally considered sterile at birth and quickly colonized by a diverse microbial population (Mayer et al., 2012). Recent studies have indicated the presence of microorganisms in the meconium of newborn calves (Alipour et al., 2018; Elolimy et al., 2019), and the fetal GIT (Guzman et al., 2020). In our study, we also found a significant bacterial community in the meconium, which may indicate microbial colonization during the fetal period. However, most microorganisms present in meconium were not found at 7 d of life. The greater α-diversity at birth (week 0) suggests that most of the microorganisms at this point are transient, and perhaps they may have a role to play in the initial colonization after birth. According to Fischer et al. (2018), these initial microorganisms can interact uniquely with the host, leading to a high individual bacterial community variation.
The decrease in the α-diversity between weeks 1 and 2 may be a consequence of the development of microorganisms adapted to the extra-uterine environment and, mainly, to colostrum and the liquid diet. The increase in α-diversity has been related to calves' age in the pre-weaning period, as observed by Badman et al. (2019) and Oikonomou et al. (2013). Lower α-diversity in this period can also be associated to higher incidence of neonatal diarrhea, as observed in other studies (Oikonomou et al., 2013; Xie et al., 2013; Nakamura et al., 2017; Zeineldin et al., 2018). Diarrhea is a gut disorder associated with dysbiosis in the bacterial community, and its higher incidence was indicated by the highest average of the fecal score between weeks 1 and 2 (Coelho et al., 2020b). When comparing the bacterial community and the diarrhea data, we can associate this disorder to the genera Escherichia/Shigella and Fusobacterium, which were more abundant in weeks 1 and 2. The genus Escherichia/Shigella includes the enterotoxigenic Escherichia coli species, which are responsible for most cases of neonatal diarrhea in calves (Rigobelo et al., 2006; Izzo et al., 2011). Fusobacterium species appear abundant in dysbiosis conditions (Huh and Roh, 2020), and is associated with inflammatory bowel diseases (Ohkusa, 2003). Excessive growth of E. coli can be limited by lactic acid-producing bacteria, such as Lactobacillus spp. (Ripamonti et al., 2013).
This subsequent increase in α-diversity will likely assist the development of GIT and the following transition from a liquid to an exclusively solid diet. In general, there is a shift in the microbiota to that of adult animals, as the animal increases the starter concentrate intake, which develops the rumen and prepares for weaning (Lallès, 2012). Besides, analysis of β-diversity indicated similarities among treatments, mainly at week 10 when calves were already weaned, group-housed, and under the same management. Cohabitation allows hosts to share microorganisms (Song et al., 2013; Wang et al., 2016; Diao et al., 2019), decreasing dissimilarity among animals.
The different liquid diets altered the fecal bacterial community during the pre-weaning period. The supply of whole milk was associated with a higher abundance of beneficial bacteria and consequently higher performance. The supply of acidified whole milk can be an alternative for the pre-weaning period, considering the gut microbiota, even if the calves are less efficient than those fed with whole milk. The supply of milk replacer must be carefully evaluated. However, differences in the initial colonization due to different liquid diets are alleviated after weaning, when animals share a common environment and solid diet composition.
Data Availability Statement
The datasets presented in this study can be found in online repositories. The names of the repository/repositories and accession number(s) can be found below: https://www.ncbi.nlm.nih.gov/, BioProject PRJNA639165, submission SUB7576848.
Ethics Statement
The animal study was reviewed and approved by the Animal Research Ethics Committee of “Luiz de Queiroz” College of Agriculture, University of São Paulo (Protocol No. 2018.5.586.11.7).
Author Contributions
CB and GV conceived and designed the experiment, wrote, reviewed, and edited the manuscript. CB and LC provided experimental and laboratorial resources. MC and AT managed the calves. GV, MC, and AT conducted sample collection. GV performed the lab analyses. HM conducted data analyses and interpretation of results. All authors read and approved the final manuscript.
Funding
The authors wish to express their appreciation for the financial support provided by National Council for Scientific and Technological Development (CNPq) as a scholarship for GV, and to the São Paulo Research Foundation for project financial support (2017/13601-3).
Conflict of Interest
The authors declare that the research was conducted in the absence of any commercial or financial relationships that could be construed as a potential conflict of interest.
Acknowledgments
The authors would like to acknowledge the continued support received from Luiz de Queiroz College of Agriculture. We would like to thank Marcela Paduan and Letícia R. S. Batista (Prof. Coutinho's laboratory) for their support in laboratory and sequencing analysis.
Supplementary Material
The Supplementary Material for this article can be found online at: https://www.frontiersin.org/articles/10.3389/fanim.2021.649468/full#supplementary-material
Footnotes
1. ^Composition: 1 L of warm water, 1 g of potassium chloride, 80 g of dextrose, 4 g of sodium bicarbonate, and 5 g of sodium chloride.
2. ^https://www.R-project.org/
3. ^Trouw Nutrition: Personal Communication.
References
Abe, F., Ishibashi, N., and Shimamura, S. (1995). Effect of administration of bifidobacteria and lactic acid bacteria to newborn calves and piglets. J. Dairy Sci. 78, 2838–2846. doi: 10.3168/jds.S0022-0302(95)76914-4
Aldredge, D. L., Geronimo, M. R., Hua, S., Nwosu, C. C., Lebrilla, C. B., and Barile, D. (2013). Annotation and structural elucidation of bovine milk oligosaccharides and determination of novel fucosylated structures. Glycobiology 23, 664–676. doi: 10.1093/glycob/cwt007
Alipour, M. J., Jalanka, J., Pessa-Morikawa, T., Kokkonen, T., Satokari, R., Hynönen, U., et al. (2018). The composition of the perinatal intestinal microbiota in cattle. Sci. Rep. 8:10437. doi: 10.1038/s41598-018-28733-y
Amaral, W. Z., Lubach, G. R., Proctor, A., Lyte, M., Phillips, G. J., and Coe, C. L. (2017). Social influences on prevotella and the gut microbiome of young monkeys. Psychosom. Med. 79, 888–897. doi: 10.1097/PSY.0000000000000454
Astbury, S., Atallah, E., Vijay, A., Aithal, G. P., Grove, J. I., and Valdes, A. M. (2020). Lower gut microbiome diversity and higher abundance of proinflammatory genus Collinsella are associated with biopsy-proven nonalcoholic steatohepatitis. Gut Microbes 11, 569–580. doi: 10.1080/19490976.2019.1681861
Badman, J., Daly, K., Kelly, J., Moran, A. W., Cameron, J., Watson, I., et al. (2019). The effect of milk replacer composition on the intestinal microbiota of pre-ruminant dairy calves. Front. Vet. Sci. 6, 1–9. doi: 10.3389/fvets.2019.00371
Bai, Y., Liu, T., Hultquist, K., Wu, J., and Casper, D. P. (2020). Feeding an amino acid formulated milk replacer for Holstein calves. J. Anim. Sci. 98, 1–10. doi: 10.1093/jas/skaa099
Bittar, C. M. M., Silva, J. T., and da, Chester-Jones, H. (2018). Macronutrient and amino acids composition of milk replacers for dairy calves. Rev. Bras. Saúde e Produção Anim. 19, 47–57. doi: 10.1590/s1519-99402018000100005
Butler, M. I., Bastiaanssen, T. F. S., Long-Smith, C., Berding, K., Morkl, S., Cusack, A.-M., et al. (2020). Recipe for a healthy gut: intake of unpasteurised milk is associated with increased lactobacillus abundance in the human gut microbiome. Nutrients 12:1468. doi: 10.3390/nu12051468
Callahan, B. J., McMurdie, P. J., Rosen, M. J., Han, A. W., Johnson, A. J. A., and Holmes, S. P. (2016a). DADA2: High-resolution sample inference from Illumina amplicon data. Nat. Methods 13, 581–583. doi: 10.1038/nmeth.3869
Callahan, B. J., Sankaran, K., Fukuyama, J. A., McMurdie, P. J., and Holmes, S. P. (2016b). Bioconductor workflow for microbiome data analysis: from raw reads to community analyses. F1000Research 5:1492. doi: 10.12688/f1000research.8986.2
Callaway, T. R., Dowd, S. E., Edrington, T. S., Anderson, R. C., Krueger, N., Bauer, N., et al. (2010). Evaluation of bacterial diversity in the rumen and feces of cattle fed different levels of dried distillers grains plus solubles using bacterial tag-encoded FLX amplicon pyrosequencing1. J. Anim. Sci. 88, 3977–3983. doi: 10.2527/jas.2010-2900
Candela, M., Biagi, E., Soverini, M., Consolandi, C., Quercia, S., Severgnini, M., et al. (2016). Modulation of gut microbiota dysbioses in type 2 diabetic patients by macrobiotic Ma-Pi 2 diet. Br. J. Nutr. 116, 80–93. doi: 10.1017/S0007114516001045
Chen, J., Wright, K., Davis, J. M., Jeraldo, P., Marietta, E. V., Murray, J., et al. (2016). An expansion of rare lineage intestinal microbes characterizes rheumatoid arthritis. Genome Med. 8:43. doi: 10.1186/s13073-016-0299-7
Chen, J., Yu, B., Chen, D., Zheng, P., Luo, Y., Huang, Z., et al. (2019). Changes of porcine gut microbiota in response to dietary chlorogenic acid supplementation. Appl. Microbiol. Biotechnol. 103, 8157–8168. doi: 10.1007/s00253-019-10025-8
Claesson, M. J., Clooney, A. G., and O'Toole, P. W. (2017). A clinician's guide to microbiome analysis. Nat. Rev. Gastroenterol. Hepatol. 14, 585–595. doi: 10.1038/nrgastro.2017.97
Coelho, M. G., Silva, F. L. M., Silva, M. D., Silva, A. P., da, Cezar, A. M., Slanzon, G. S., et al. (2020a). Acidified milk for feeding dairy calves in tropical raising systems. J. Anim. Feed Sci. 29, 215–223. doi: 10.22358/jafs/127690/2020
Coelho, M., Tomaluski, C., Dondé, S., Toledo, A. F., de Bernardes, J. P., Jeronymo, N., et al. (2020b). PSIX-21 Performance and health of dairy calves fed with acidified milk in tropical climates. J. Anim. Sci. 98, 419–420. doi: 10.1093/jas/skaa278.731
Cooper, R., and Watson, I. (2013). A guide to feeding and assessment of calf milk replacer. Livestock 18, 216–222. doi: 10.12968/live.2013.18.6.216
Czarnecki-Maulden, G. L. (2008). Effect of dietary modulation of intestinal microbiota on reproduction and early growth. Theriogenology 70, 286–290. doi: 10.1016/j.theriogenology.2008.05.041
Deng, Y. F., Wang, Y. J., Zou, Y., Azarfar, A., Wei, X. L., Ji, S. K., et al. (2017). Influence of dairy by-product waste milk on the microbiomes of different gastrointestinal tract components in pre-weaned dairy calves. Sci. Rep. 7:42689. doi: 10.1038/srep42689
Dial, S. (2004). Risk of Clostridium difficile diarrhea among hospital inpatients prescribed proton pump inhibitors: cohort and case-control studies. Can. Med. Assoc. J. 171, 33–38. doi: 10.1503/cmaj.1040876
Diao, Q., Zhang, R., and Fu, T. (2019). Review of strategies to promote rumen development in calves. Animals 9:490. doi: 10.3390/ani9080490
Dias, J., Marcondes, M. I., de Souza, S. M., da Mata e Silva, B. C., Noronha, M. F., Resende, R. T., et al. (2018). Bacterial community dynamics across the gastrointestinal tracts of dairy calves during preweaning development. Appl. Environ. Microbiol. 84, e02675–e02617. doi: 10.1128/AEM.02675-17
Dias, J., Marcondes, M. I., Noronha, M. F., Resende, R. T., Machado, F. S., Mantovani, H. C., et al. (2017). Effect of pre-weaning diet on the ruminal archaeal, bacterial, and fungal communities of dairy calves. Front. Microbiol. 8:1553. doi: 10.3389/fmicb.2017.01553
Dill-McFarland, K. A., Breaker, J. D., and Suen, G. (2017). Microbial succession in the gastrointestinal tract of dairy cows from 2 weeks to first lactation. Sci. Rep. 7:40864. doi: 10.1038/srep40864
Djurasevic, S., Bojic, S., Nikolic, B., Dimkic, I., Todorovic, Z., Djordjevic, J., et al. (2018). Beneficial effect of virgin coconut oil on alloxan-induced diabetes and microbiota composition in rats. Plant Foods Hum. Nutr. 73, 295–301. doi: 10.1007/s11130-018-0689-7
dos Santos, G., and Bittar, C. M. M. (2015). A survey of dairy calf management practices in some producing regions in Brazil. Rev. Bras. Zootec. 44, 361–370. doi: 10.1590/S1806-92902015001000004
Duncan, S. H., Hold, G. L., Harmsen, H. J. M., Stewart, C. S., and Flint, H. J. (2002). Growth requirements and fermentation products of Fusobacterium prausnitzii, and a proposal to reclassify it as Faecalibacterium prausnitzii gen. nov., comb. nov. Int. J. Syst. Evol. Microbiol. 52, 2141–2146. doi: 10.1099/00207713-52-6-2141
Edrington, T., Farrow, R., Carter, B., Islas, A., Hagevoort, G., Callaway, T., et al. (2012). Age and diet effects on fecal populations and antibiotic resistance of a multi-drug resistant Escherichia coli in dairy calves. Agric. food Anal. Bacteriol. 2:162. Available at: www.afabjournal.com.
Eeckhaut, V., Machiels, K., Perrier, C., Romero, C., Maes, S., Flahou, B., et al. (2013). Butyricicoccus pullicaecorum in inflammatory bowel disease. Gut 62, 1745–1752. doi: 10.1136/gutjnl-2012-303611
Elolimy, A., Alharthi, A., Zeineldin, M., Parys, C., Helmbrecht, A., and Loor, J. J. (2019). Supply of methionine during late-pregnancy alters fecal microbiota and metabolome in neonatal dairy calves without changes in daily feed intake. Front. Microbiol. 10, 1–20. doi: 10.3389/fmicb.2019.02159
Elsohaby, I., McClure, J. T., Waite, L. A., Cameron, M., Heider, L. C., and Keefe, G. P. (2019). Using serum and plasma samples to assess failure of transfer of passive immunity in dairy calves. J. Dairy Sci. 102, 567–577. doi: 10.3168/jds.2018-15070
Fagnani, R., Ito Eleodoro, J., and Osti Zanon, E. (2019). Milk-borne infections awareness and the health status of consumers: an on-line survey. Int. Dairy J. 96, 85–92. doi: 10.1016/j.idairyj.2019.04.013
Fischer, A. J., Song, Y., He, Z., Haines, D. M., Guan, L. L., and Steele, M. A. (2018). Effect of delaying colostrum feeding on passive transfer and intestinal bacterial colonization in neonatal male Holstein calves. J. Dairy Sci. 101, 3099–3109. doi: 10.3168/jds.2017-13397
Gentleman, R. C., Carey, V. J., Bates, D. M., Bolstad, B., Dettling, M., Dudoit, S., et al. (2004). Bioconductor: open software development for computational biology and bioinformatics. Genome Biol. 5:R80. doi: 10.1186/gb-2004-5-10-r80
Ghezzal, S., Postal, B. G., Quevrain, E., Brot, L., Seksik, P., Leturque, A., et al. (2020). Palmitic acid damages gut epithelium integrity and initiates inflammatory cytokine production. Biochim. Biophys. Acta Mol. Cell Biol. Lipids 1865:158530. doi: 10.1016/j.bbalip.2019.158530
Glöckner, F. O., Yilmaz, P., Quast, C., Gerken, J., Beccati, A., Ciuprina, A., et al. (2017). 25 years of serving the community with ribosomal RNA gene reference databases and tools. J. Biotechnol. 261, 169–176. doi: 10.1016/j.jbiotec.2017.06.1198
Godden, S. (2008). Colostrum management for dairy calves. Vet. Clin. North Am. Food Anim. Pract. 24, 19–39. doi: 10.1016/j.cvfa.2007.10.005
Górka, P., Kowalski, Z. M. M., Pietrzak, P., Kotunia, A., Jagusiak, W., and Zabielski, R. (2011). Is rumen development in newborn calves affected by different liquid feeds and small intestine development? J. Dairy Sci. 94, 3002–3013. doi: 10.3168/jds.2010-3499
Guzman, C. E., Bereza-Malcolm, L. T., De Groef, B., and Franks, A. E. (2015). Presence of selected methanogens, fibrolytic bacteria, and proteobacteria in the gastrointestinal tract of neonatal dairy calves from birth to 72 hours. PLoS ONE 10:e0133048. doi: 10.1371/journal.pone.0133048
Guzman, C. E., Wood, J. L., Egidi, E., White-Monsant, A. C., Semenec, L., Grommen, S. V. H., et al. (2020). A pioneer calf foetus microbiome. Sci. Rep. 10:17712. doi: 10.1038/s41598-020-74677-7
Hidalgo-Cantabrana, C., Delgado, S., Ruiz, L., Ruas-Madiedo, P., Sánchez, B., and Margolles, A. (2018). “Bifidobacteria and their health-promoting effects,” in Bugs as Drugs, eds R. A. Britton and P. D. Cani (Washington, DC: ASM Press), 73–98. doi: 10.1128/9781555819705.ch3
Hill, C., Guarner, F., Reid, G., Gibson, G. R., Merenstein, D. J., Pot, B., et al. (2014). The International Scientific Association for Probiotics and Prebiotics consensus statement on the scope and appropriate use of the term probiotic. Nat. Rev. Gastroenterol. Hepatol. 11, 506–514. doi: 10.1038/nrgastro.2014.66
Huber, W., Carey, V. J., Gentleman, R., Anders, S., Carlson, M., Carvalho, B. S., et al. (2015). Orchestrating high-throughput genomic analysis with bioconductor. Nat. Methods 12, 115–121. doi: 10.1038/nmeth.3252
Huh, J.-W., and Roh, T.-Y. (2020). Opportunistic detection of Fusobacterium nucleatum as a marker for the early gut microbial dysbiosis. BMC Microbiol. 20:208. doi: 10.1186/s12866-020-01887-4
Huws, S. A., Edwards, J. E., Creevey, C. J., Rees Stevens, P., Lin, W., Girdwood, S. E., et al. (2016). Temporal dynamics of the metabolically active rumen bacteria colonizing fresh perennial ryegrass. FEMS Microbiol. Ecol. 92:fiv137. doi: 10.1093/femsec/fiv137
Izzo, M., Kirkland, P., Mohler, V., Perkins, N. R., Gunn, A., and House, J. (2011). Prevalence of major enteric pathogens in Australian dairy calves with diarrhoea. Aust. Vet. J. 89, 167–173. doi: 10.1111/j.1751-0813.2011.00692.x
Jaster, E. H., McCoy, G. C., Tomkins, T., and Davis, C. L. (1990). Feeding acidified or sweet milk replacer to dairy calves. J. Dairy Sci. 73, 3563–3566. doi: 10.3168/jds.S0022-0302(90)79056-X
Kasparovska, J., Pecinkova, M., Dadakova, K., Krizova, L., Hadrova, S., Lexa, M., et al. (2016). Effects of isoflavone-enriched feed on the rumen microbiota in dairy cows. PLoS ONE 11:e0154642. doi: 10.1371/journal.pone.0154642
Kelly, W. J., Cookson, A. L., Altermann, E., Lambie, S. C., Perry, R., Teh, K. H., et al. (2016). Genomic analysis of three Bifidobacterium species isolated from the calf gastrointestinal tract. Sci. Rep. 6:30768. doi: 10.1038/srep30768
Klein-Jöbstl, D., Schornsteiner, E., Mann, E., Wagner, M., Drillich, M., and Schmitz-Esser, S. (2014). Pyrosequencing reveals diverse fecal microbiota in Simmental calves during early development. Front. Microbiol. 5:622. doi: 10.3389/fmicb.2014.00622
Lallès, J. P. (2012). Long term effects of pre- and early postnatal nutrition and environment on the gut1. J. Anim. Sci. 90, 421–429. doi: 10.2527/jas.53904
Larson, L. L., Owen, F. G., Albright, J. L., Appleman, R. D., Lamb, R. C., and Muller, L. D. (1977). Guidelines toward more uniformity in measuring and reporting calf experimental data. J. Dairy Sci. 60, 989–991. doi: 10.3168/jds.S0022-0302(77)83975-1
Law, C. W., Chen, Y., Shi, W., and Smyth, G. K. (2014). voom: precision weights unlock linear model analysis tools for RNA-seq read counts. Genome Biol. 15:R29. doi: 10.1186/gb-2014-15-2-r29
Lees, E. A., Carrol, E. D., Ellaby, N. A. F., Roberts, P., Corless, C. E., Lenzi, L., et al. (2020). Characterization of circulating clostridium difficile strains, host response and intestinal microbiome in hospitalized children with diarrhea. Pediatr. Infect. Dis. J. 39, 221–228. doi: 10.1097/INF.0000000000002559
Li, R. W., Connor, E. E., Li, C., Baldwin, V. I. R. L, and Sparks, M. E. (2012). Characterization of the rumen microbiota of pre-ruminant calves using metagenomic tools. Environ. Microbiol. 14, 129–139. doi: 10.1111/j.1462-2920.2011.02543.x
Li, Y., Guo, Y., Wen, Z., Jiang, X., Ma, X., and Han, X. (2018). Weaning stress perturbs gut microbiome and its metabolic profile in piglets. Sci. Rep. 8:18068. doi: 10.1038/s41598-018-33649-8
Lourenco, J. M., Kieran, T. J., Seidel, D. S., Glenn, T. C., Silveira, M. F., da Callaway, T. R., et al. (2020). Comparison of the ruminal and fecal microbiotas in beef calves supplemented or not with concentrate. PLoS ONE 15:e0231533. doi: 10.1371/journal.pone.0231533
Macfarlane, G. T., Steed, H., and Macfarlane, S. (2007). Bacterial metabolism and health-related effects of galacto-oligosaccharides and other prebiotics. J. Appl. Microbiol. 104:070907095856003. doi: 10.1111/j.1365-2672.2007.03520.x
Malmuthuge, N., Griebel, P. J., and Guan, L. L. (2015). The gut microbiome and its potential role in the development and function of newborn calf gastrointestinal tract. Front. Vet. Sci. 2:2016. doi: 10.3389/fvets.2015.00036
Mancini, A., Imperlini, E., Nigro, E., Montagnese, C., Daniele, A., Orrù, S., et al. (2015). Biological and nutritional properties of palm oil and palmitic acid: effects on health. Molecules 20, 17339–17361. doi: 10.3390/molecules200917339
Maslowski, K. M., and Mackay, C. R. (2011). Diet, gut microbiota and immune responses. Nat. Immunol. 12, 5–9. doi: 10.1038/ni0111-5
Mayer, M., Abenthum, A., Matthes, J. M. M., Kleeberger, D., Ege, M. J. J., Hölzel, C., et al. (2012). Development and genetic influence of the rectal bacterial flora of newborn calves. Vet. Microbiol. 161, 179–185. doi: 10.1016/j.vetmic.2012.07.023
McMurdie, P. J., and Holmes, S. (2013). phyloseq: an R package for reproducible interactive analysis and graphics of microbiome census data. PLoS ONE 8:e61217. doi: 10.1371/journal.pone.0061217
Nakamura, S.-I., Kim, Y. H., Takashima, K., Kimura, A., Nagai, K., Ichijo, T., et al. (2017). Composition of the microbiota in forestomach fluids and feces of Japanese Black calves with white scours. J. Anim. Sci. 95:3949. doi: 10.2527/jas2017.1431
Ohkusa, T. (2003). Induction of experimental ulcerative colitis by Fusobacterium varium isolated from colonic mucosa of patients with ulcerative colitis. Gut 52, 79–83. doi: 10.1136/gut.52.1.79
Oikonomou, G., Teixeira, A. G. V., Foditsch, C., Bicalho, M. L., Machado, V. S., and Bicalho, R. C. (2013). Fecal microbial diversity in pre-weaned dairy calves as described by pyrosequencing of metagenomic 16S rDNA. Associations of Faecalibacterium Species with Health and Growth. PLoS ONE 8:e63157. doi: 10.1371/journal.pone.0063157
Pacheco, A. R., Barile, D., Underwood, M. A., and Mills, D. A. (2015). The impact of the milk glycobiome on the neonate gut microbiota. Annu. Rev. Anim. Biosci. 3, 419–445. doi: 10.1146/annurev-animal-022114-111112
Penders, J., Thijs, C., Vink, C., Stelma, F. F., Snijders, B., Kummeling, I., et al. (2006). Factors influencing the composition of the intestinal microbiota in early infancy. Pediatrics 118, 511–521. doi: 10.1542/peds.2005-2824
Penders, J., Vink, C., Driessen, C., London, N., Thijs, C., and Stobberingh, E. E. (2005). Quantification of Bifidobacterium spp., Escherichia coli and Clostridium difficile in faecal samples of breast-fed and formula-fed infants by real-time PCR. FEMS Microbiol. Lett. 243, 141–147. doi: 10.1016/j.femsle.2004.11.052
Petri, R. M., Schwaiger, T., Penner, G. B., Beauchemin, K. A., Forster, R. J., McKinnon, J. J., et al. (2013a). Changes in the rumen epimural bacterial diversity of beef cattle as affected by diet and induced ruminal acidosis. Appl. Environ. Microbiol. 79, 3744–3755. doi: 10.1128/AEM.03983-12
Petri, R. M., Schwaiger, T., Penner, G. B., Beauchemin, K. A., Forster, R. J., McKinnon, J. J., et al. (2013b). Characterization of the core rumen microbiome in cattle during transition from forage to concentrate as well as during and after an acidotic challenge. PLoS ONE 8:e83424. doi: 10.1371/journal.pone.0083424
Poutanen, S. M. (2004). Clostridium difficile-associated diarrhea in adults. Can. Med. Assoc. J. 171, 51–58. doi: 10.1503/cmaj.1031189
Richard, A. L., Muller, L. D., and Heinrichs, A. J. (1988). Ad libitum or twice daily feeding of acidified milk replacer to calves housed individually in warm and cold environments. J. Dairy Sci. 71, 2193–2202. doi: 10.3168/jds.S0022-0302(88)79793-3
Rigobelo, E. C., Gamez, H. J., Marin, J. M., Macedo, C., Ambrosin, J. A., and Ávila, F. A. (2006). Virulence factors of Escherichia coli isolated from diarrheic calves. Arq. Bras. Med. Veterinária e Zootec. 58, 305–310. doi: 10.1590/S0102-09352006000300003
Ripamonti, B., Tirloni, E., Stella, S., Bersani, C., Agazzi, A., Maroccolo, S., et al. (2013). Effects of a species-specific probiotic formulation on multiresistant Escherichia coli isolates from the gut of veal calves. Czech J. Anim. Sci. 58, 201–207. doi: 10.17221/6748-CJAS
Ritchie, M. E., Phipson, B., Wu, D., Hu, Y., Law, C. W., Shi, W., et al. (2015). limma powers differential expression analyses for RNA-sequencing and microarray studies. Nucleic Acids Res. 43:e47. doi: 10.1093/nar/gkv007
Robinson, M. D., McCarthy, D. J., and Smyth, G. K. (2010). edgeR: a bioconductor package for differential expression analysis of digital gene expression data. Bioinformatics 26, 139–140. doi: 10.1093/bioinformatics/btp616
Robinson, M. D., and Oshlack, A. (2010). A scaling normalization method for differential expression analysis of RNA-seq data. Genome Biol. 11:R25. doi: 10.1186/gb-2010-11-3-r25
Rolinec, M., Medo, J., Gábor, M., Miluchová, M., Bíro, D., Šimko, M., et al. (2020). The effect of coconut oil addition to feed of pigs on rectal microbial diversity and bacterial abundance. Animals 10:1764. doi: 10.3390/ani10101764
Rubino, F., Carberry, C. M., Waters, S., Kenny, D., McCabe, M. S., and Creevey, C. J. (2017). Divergent functional isoforms drive niche specialisation for nutrient acquisition and use in rumen microbiome. ISME J. 11, 932–944. doi: 10.1038/ismej.2016.172
Sakamoto, M., and Benno, Y. (2006). Reclassification of Bacteroides distasonis, Bacteroides goldsteinii and Bacteroides merdae as Parabacteroides distasonis gen. nov., comb. nov., Parabacteroides goldsteinii comb. nov. and Parabacteroides merdae comb. nov. Int. J. Syst. Evol. Microbiol. 56, 1599–1605. doi: 10.1099/ijs.0.64192-0
Schwartz, S., Friedberg, I., Ivanov, I. V., Davidson, L. A., Goldsby, J. S., Dahl, D. B., et al. (2012). A metagenomic study of diet-dependent interaction between gut microbiota and host in infants reveals differences in immune response. Genome Biol. 13:R32. doi: 10.1186/gb-2012-13-4-r32
Shanks, O. C., Kelty, C. A., Archibeque, S., Jenkins, M., Newton, R. J., McLellan, S. L., et al. (2011). Community structures of fecal bacteria in cattle from different animal feeding operations. Appl. Environ. Microbiol. 77, 2992–3001. doi: 10.1128/AEM.02988-10
Smyth, G. K. (2004). Linear models and empirical bayes methods for assessing differential expression in microarray experiments. Stat. Appl. Genet. Mol. Biol. 3, 1–25. doi: 10.2202/1544-6115.1027
Song, S. J., Lauber, C., Costello, E. K., Lozupone, C. A., Humphrey, G., Berg-Lyons, D., et al. (2013). Cohabiting family members share microbiota with one another and with their dogs. Elife 2:e00458. doi: 10.7554/eLife.00458.018
Tang, Q., Jin, G., Wang, G., Liu, T., Liu, X., Wang, B., et al. (2020). Current sampling methods for gut microbiota: a call for more precise devices. Front. Cell. Infect. Microbiol. 10:151. doi: 10.3389/fcimb.2020.00151
Todd, C. G., Leslie, K. E., Millman, S. T., Bielmann, V., Anderson, N. G., Sargeant, J. M., et al. (2017). Clinical trial on the effects of a free-access acidified milk replacer feeding program on the health and growth of dairy replacement heifers and veal calves. J. Dairy Sci. 100, 713–725. doi: 10.3168/jds.2016-11401
United States Department of Agriculture (2014). Dairy 2014: Dairy Cattle Management Practices in the United States. Fort Collins, CO. Available online at: http://www.aphis.usda.gov/nahms (accessed February 12, 2021).
Uyeno, Y., Sekiguchi, Y., Tajima, K., Takenaka, A., Kurihara, M., and Kamagata, Y. (2010). An rRNA-based analysis for evaluating the effect of heat stress on the rumen microbial composition of Holstein heifers. Anaerobe 16, 27–33. doi: 10.1016/j.anaerobe.2009.04.006
Vael, C., and Desager, K. (2009). The importance of the development of the intestinal microbiota in infancy. Curr. Opin. Pediatr. 21, 794–800. doi: 10.1097/MOP.0b013e328332351b
Vasseur, E., Borderas, F., Cue, R. I., Lefebvre, D., Pellerin, D., Rushen, J., et al. (2010). A survey of dairy calf management practices in Canada that affect animal welfare. J. Dairy Sci. 93, 1307–1316. doi: 10.3168/jds.2009-2429
Wang, L., Xu, Q., Kong, F., Yang, Y., Wu, D., Mishra, S., et al. (2016). Exploring the goat rumen microbiome from seven days to two years. PLoS ONE 11:e0154354. doi: 10.1371/journal.pone.0154354
Wang, Q., Garrity, G. M., Tiedje, J. M., and Cole, J. R. (2007). Naïve Bayesian classifier for rapid assignment of rRNA sequences into the new bacterial taxonomy. Appl. Environ. Microbiol. 73, 5261–5267. doi: 10.1128/AEM.00062-07
Watanabe, Y., Nagai, F., and Morotomi, M. (2012). Characterization of Phascolarctobacterium succinatutens sp. nov., an Asaccharolytic, succinate-utilizing bacterium isolated from human feces. Appl. Environ. Microbiol. 78, 511–518. doi: 10.1128/AEM.06035-11
Xie, G., Duff, G. C., Hall, L. W., Allen, J. D., Burrows, C. D., Bernal-Rigoli, J. C., et al. (2013). Alteration of digestive tract microbiome in neonatal Holstein bull calves by bacitracin methylene disalicylate treatment and scours1. J. Anim. Sci. 91, 4984–4990. doi: 10.2527/jas.2013-6304
Yanar, M., Güler, O., Bayram, B., and Metin, J. (2006). Effects of feeding acidified milk replacer on the growth, health and behavioural characteristics of holstein friesian calves. Turkish J. Vet. Anim. Sci. 30, 235–241.
Yu, Z., and Morrison, M. (2004). Improved extraction of PCR-quality community DNA from digesta and fecal samples. Biotechniques 36, 808–812. doi: 10.2144/04365ST04
Zeineldin, M., Aldridge, B., and Lowe, J. (2018). Dysbiosis of the fecal microbiota in feedlot cattle with hemorrhagic diarrhea. Microb. Pathog. 115, 123–130. doi: 10.1016/j.micpath.2017.12.059
Zhang, N., Song, C., Wang, M., Liu, Y., Hui, M., and Cui, Z. (2017). Diversity and characterization of bacteria associated with the deep-sea hydrothermal vent crab Austinograea sp. comparing with those of two shallow-water crabs by 16S ribosomal DNA analysis. PLoS ONE 12:e0187842. doi: 10.1371/journal.pone.0187842
Zou, Y., Wang, Y., Deng, Y., Cao, Z., Li, S., and Wang, J. (2017). Effects of feeding untreated, pasteurized and acidified waste milk and bunk tank milk on the performance, serum metabolic profiles, immunity, and intestinal development in Holstein calves. J. Anim. Sci. Biotechnol. 8:53. doi: 10.1186/s40104-017-0182-4
Keywords: animal nutrition, Bifidobacterium, dairy calf, gut health, gut microbiota
Citation: Virgínio Júnior GF, Coelho MG, de Toledo AF, Montenegro H, Coutinho LL and Bittar CMM (2021) The Liquid Diet Composition Affects the Fecal Bacterial Community in Pre-weaning Dairy Calves. Front. Anim. Sci. 2:649468. doi: 10.3389/fanim.2021.649468
Received: 04 January 2021; Accepted: 31 March 2021;
Published: 29 April 2021.
Edited by:
Otávio R. Machado Neto, São Paulo State University, BrazilCopyright © 2021 Virgínio Júnior, Coelho, de Toledo, Montenegro, Coutinho and Bittar. This is an open-access article distributed under the terms of the Creative Commons Attribution License (CC BY). The use, distribution or reproduction in other forums is permitted, provided the original author(s) and the copyright owner(s) are credited and that the original publication in this journal is cited, in accordance with accepted academic practice. No use, distribution or reproduction is permitted which does not comply with these terms.
*Correspondence: Gercino Ferreira Virgínio Júnior, Z2VyY2lub2ZlcnJlaXJhQHVzcC5icg==; Carla Maris Machado Bittar, Y2FybGFiaXR0YXJAdXNwLmJy