- 1Centro Regional de Investigación Carillanca, Instituto de Investigaciones Agropecuarias INIA, Vilcún, Chile
- 2Lethbridge Research and Development Centre, Agriculture and Agri-Food Canada, Lethbridge, AB, Canada
- 3Centro Regional de Investigación Remehue, Instituto de Investigaciones Agropecuarias INIA, Osorno, Chile
Limiting global warming to 1.5°C above pre-industrial levels by 2050 requires achieving net zero emissions of greenhouse gases by 2050 and a strong decrease in methane (CH4) emissions. Our aim was to connect the global need for mitigation of the emissions of greenhouse gases and enteric CH4 from ruminant production to basic research on the biological consequences of inhibiting rumen methanogenesis in order to better design strategies for pronounced mitigation of enteric CH4 production without negative impacts on animal productivity or economic returns. Ruminant production worldwide has the challenge of decreasing its emissions of greenhouse gases while increasing the production of meat and milk to meet consumers demand. Production intensification decreases the emissions of greenhouse gases per unit of product, and in some instances has decreased total emissions, but in other instances has resulted in increased total emissions of greenhouse gases. We propose that decreasing total emission of greenhouse gases from ruminants in the next decades while simultaneously increasing meat and milk production will require strong inhibition of rumen methanogenesis. An aggressive approach to pronounced inhibition of enteric CH4 emissions is technically possible through the use of chemical compounds and/or bromoform-containing algae, but aspects such as safety, availability, government approval, consumer acceptance, and impacts on productivity and economic returns must be satisfactorily addressed. Feeding these additives will increase the cost of ruminant diets, which can discourage their adoption. On the other hand, inhibiting rumen methanogenesis potentially saves energy for the host animal and causes profound changes in rumen fermentation and post-absorptive metabolism. Understanding the biological consequences of methanogenesis inhibition could allow designing strategies to optimize the intervention. We conducted meta-regressions using published studies with at least one treatment with >50% inhibition of CH4 production to elucidate the responses of key rumen metabolites and animal variables to methanogenesis inhibition, and understand possible consequences on post-absorptive metabolism. We propose possible avenues, attainable through the understanding of biological consequences of the methanogenesis inhibition intervention, to increase animal productivity or decrease feed costs when inhibiting methanogenesis.
Introduction
All segments of society, including the agricultural industries, are challenged to mitigate greenhouse gas emissions to limit global warming to 1.5°C above pre-industrial levels by 2050 (IPCC, 2018, 2019). Net zero emissions is defined as a stage in which metric-weighted anthropogenic greenhouse gas emissions to the atmosphere are balanced by their removal (IPCC, 2021; Net Zero Climate, 2021). It is estimated that limiting global warming to a maximum of 1.5°C requires reaching net zero emissions globally by 2050 and concomitantly attaining deep reductions in methane (CH4) emissions (Rogelj et al., 2018), with agriculture as a necessary component of emissions mitigation (Leahy et al., 2020). A net zero commitment by 2050 has been taken by more than 130 countries as well as many private sector companies (Black et al., 2021; United Nations Climate Action, 2021). Importantly, various large agribusiness companies and value chain organizations have set ambitious voluntary targets to decrease their emissions of greenhouse gases (Table 1). The 35 largest meat and dairy companies are responsible for 14% of total livestock emissions of greenhouse gases, and as their supply chains account for a major part of their total emissions, their targets of mitigation of emissions of greenhouse gases will impact livestock producers, who will thus need to decrease their emissions of greenhouse gases to sell their products (Leahy et al., 2020).
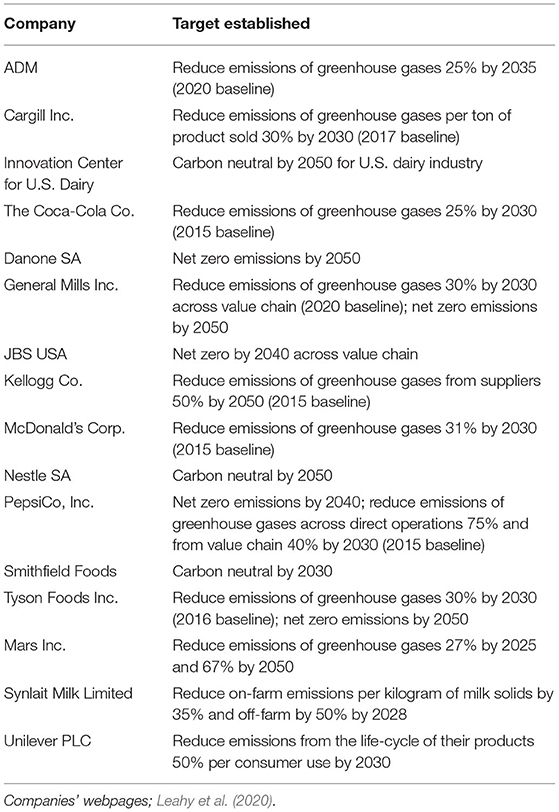
Table 1. Voluntary commitments to reduce the emissions of greenhouse gases stated by various multinational agribusiness companies.
Decreasing the emissions of greenhouse gases from ruminant production is a challenging goal given the projected increased demand for ruminant meat and milk in future decades and the limited mitigation options currently available to producers (Beauchemin et al., 2020). Thus, avenues are being sought to decrease the emissions of greenhouse gases from ruminant production while simultaneously increasing production to meet the rising demand for animal products. To meet the challenge of decreasing the emissions of greenhouse gases while continuing to grow production, the focus has often been on decreasing greenhouse gas emissions intensity (i.e., lowering emissions per unit of product) through production intensification to increase animal productivity (Gerber et al., 2013a; Opio et al., 2013). Decreasing emissions intensity by increasing animal productivity is an attractive strategy in that it can increase farmers' profitability (Gerber et al., 2013a).
In this paper, we put forward the case that improved animal productivity alone is unlikely to achieve a substantial decrease in total emissions of greenhouse gases from ruminant production globally, and in many developed and developing countries and regions. Our first objective in this paper is to show that pronounced decreases in enteric CH4 production per animal are also necessary, and that moderate decreases of enteric CH4 production per animal may be insufficient to mitigate total emissions of greenhouse gases from ruminant production. Methane is the main greenhouse gas emitted by ruminant production, accounting for about half of the 1.0°C increase in global temperature since pre-industrial times. Due to the short half-life of CH4 in the atmosphere, mitigation of CH4 emissions is considered the single most effective strategy to ameliorate global warming in the short term and limit temperature increase to 1.5°C (Beauchemin et al., 2020; European Commission, 2021; Global Methane Initiative, 2021). The evolution of CH4 emissions will affect the targets of carbon dioxide (CO2) decrease necessary to limit global warming to 1.5°C (Reisinger et al., 2021).
Implementing measures that pronouncedly decrease enteric CH4 production is technically possible but will likely increase feed costs and could negatively impact economic returns of ruminant production. Therefore, our second objective for this paper was to explore how strategies aimed at pronounced inhibition of rumen methanogenesis could be made cost effective. Designing effective antimethanogenic strategies attractive for adoption requires understanding the biological consequences of inhibiting rumen methanogenesis on rumen fermentation and animal metabolism. We conducted meta-regressions to relate key rumen metabolites and feed intake to CH4 production using data from studies in which methanogenesis was inhibited by at least 50%. We discuss how changes in rumen and post-absorptive metabolism occurring as a consequence of pronounced inhibition of rumen methanogenesis might be manipulated toward the improvement of ruminant productivity, and ultimately cost effectiveness of CH4 abatement. We finally identify knowledge gaps that need to be addressed by future research to achieve these goals.
Intensification of Ruminant Production and emissions Of Greenhouse Gases
The three major greenhouse gases emitted from animal production are CO2, CH4 and nitrous oxide (N2O). They differ in their heat trapping effects and their lifetimes in the atmosphere. The heat trapping effect of a pulse emission of a mixture of CO2, CH4, and N2O over a certain time period following the pulse emission can be calculated as CO2 equivalents (CO2e), i.e., the sum of the amount of each gas emitted weighted by its global warming potential integrated over the period of time considering its rate of disappearance. In this paper, we will consider global warming potentials over 100 yr, or GWP100 (Opio et al., 2013).
As product outputs (i.e., meat, milk) from individual animals increase due to improvements in genetics, nutrition, health and management, total (or absolute) emissions of enteric CH4 and CO2e per animal increase in parallel due to greater feed intake and digestion and manure output, along with greater upstream emissions of N2O and fossil fuel generated CO2 mainly from feed and farm inputs. However, emissions of CH4 and of CO2e per unit of product, or emissions intensity, decrease due to the dilution of animal maintenance requirements, better herd management and animal health, and greater productivity of feed crops (Capper et al., 2009; Capper, 2011; Gerber et al., 2011). We are interested in understanding how decreasing CO2e emissions intensity of a production system relates to total CO2e emissions, as atmospheric concentration of greenhouse gases and the extent of global warming depends on the latter. Total emissions of CO2e in a certain period of time (e.g., emissions of CO2e from a farm, industry, country or region, or global emissions, in 1 year), result from multiplying the emissions of CO2e per unit of animal product by the total amount of animal product produced during that period. In this way, total emissions of CO2e result from multiplying the emissions of CO2e per unit of product by the production per individual animal and the number of animals (Supplementary Equation 1).
An analysis of milk production from representative farms in 155 countries found that CO2e emissions intensity decreased with increased milk production following an asymptotic function (Gerber et al., 2011). In extensive production systems, in which the potential to decrease CO2e emissions intensity through increasing animal productivity is substantial (Gerber et al., 2011), intensification can accommodate for a simultaneous increase in production of milk or meat without an increase in total CO2e emissions. However, it is less certain that decreasing emissions intensity alone will decrease total CO2e emissions if production continues to expand (Gerber et al., 2013b). Production of beef, lamb and milk in developing countries is predicted to grow by 8.0, 15.1, and 26.2%, respectively, between 2021 and 2030 (OECD/FAO, 2021). If future decreases in CO2e emissions intensities are similar to the rates of increase in production, greenhouse gas emissions from animal production will remain constant, i.e., without any mitigation (Leahy et al., 2020).
While in systems with low productivity, intensification decreases CO2e emissions intensity, intensive systems may find it difficult to further intensify with simultaneous decrease in total emissions of CO2e, to meet carbon neutrality goals (UN Climate Change News, 2021; United Nations Climate Action, 2021) (Table 1). Changes in total CO2e emissions and CO2e emissions intensity for the dairy, beef and lamb industries through different time periods have been estimated for various developed countries, states and provinces (Leslie et al., 2008; Capper et al., 2009; Capper, 2011; Jayasundara and Wagner-Riddle, 2013; Wiedemann et al., 2015; Legesse et al., 2016; Capper and Cady, 2020; Naranjo et al., 2020) and are summarized in Table 2. Based on those studies, we calculated constant fractional yearly rates of increase or decrease in total emissions of CO2e and emissions of CO2e per unit of product, expressed as percentages (Supplementary Equation 2). When not provided in the referenced papers, total CO2e emissions were calculated from total food production and emissions intensity (Supplementary Equation 1).
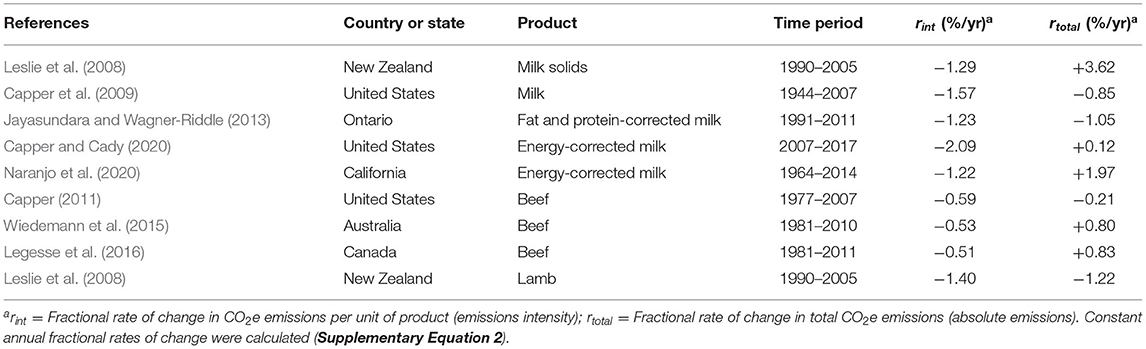
Table 2. Fractional rates of change of total emissions and emissions intensity of carbon dioxide equivalents (CO2e) of the dairy, beef and lamb industries in various regions.
A consistent pattern in Table 2 is a decrease in CO2e emissions intensity as production intensifies (Capper et al., 2009; Capper, 2011; Gerber et al., 2011) and producers use natural resources with greater efficiency (Gerber et al., 2013b). In contrast to CO2e emissions per unit of product, the evolution of total CO2e emissions was more variable, with decreases (Leslie et al., 2008; Capper et al., 2009; Capper, 2011; Jayasundara and Wagner-Riddle, 2013) but also increases in total CO2e emissions (Leslie et al., 2008; Wiedemann et al., 2015; Legesse et al., 2016; Capper and Cady, 2020; Naranjo et al., 2020) in the periods and places studied. A global livestock assessment for the 1961–2010 period reported an increase in total CO2e emissions from developing countries of 117% and a 23% decrease from developed countries (Caro et al., 2014); however, emissions of CO2 associated to the use of fossil fuels and of N2O resulting from the application of fertilizers, which are greater in high input production systems, were not considered in that analysis.
Figure 1 predicts CO2e emissions from the U.S. dairy industry toward 2050 using an asymptotic model (Supplementary Equation 3) to accommodate for an assumption of production growth slowing down in industrialized economies (Briunsma, 2003). The yearly rate of change in total CO2e emissions and CO2e emissions per kilogram of energy-corrected milk (ECM) was calculated from the estimations by Capper and Cady (2020) for the 2007–2017 period, and extrapolated to the 2017–2050 period. We selected the study by Capper and Cady (2020) for predicting the evolution of total CO2e and CO2e emissions per unit of product toward 2050 because it analyzed the latest time period in Table 2. We projected total CO2e emissions and emissions of CO2e per unit of ECM for the U.S. dairy industry toward 2050 under two different scenarios: (i) at a constant CO2e emissions intensity, as estimated by Capper and Cady (2020) for the year 2017, and (ii) at decreasing CO2e emissions intensity with a rate calculated with Supplementary Equation 3 for the 2007-2017 period of the Capper and Cady (2020) study.
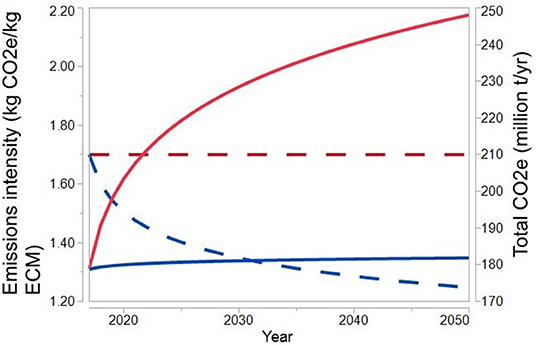
Figure 1. Projected total CO2e emissions and CO2e emissions intensity [CO2e emissions per kilogram of energy-corrected milk (ECM)] for the US dairy industry between 2017 and 2050. Total CO2e emissions and total ECM production and CO2e emissions per kg ECM for the baseline year 2017 are from Capper and Cady (2020). Rates of change of total CO2e emissions and CO2e emissions per kg ECM were calculated for the 2007–2017 period based on Capper and Cady (2020) using an asymptotic model and extrapolated to the 2017–2050 period. Dashed lines correspond to CO2e emissions per kg ECM (CO2e emissions intensity, left axis) and continuous lines correspond to total emissions of CO2e (right axis). Red lines correspond to constant, 2017-levels, of CO2e emissions per kg ECM; blue lines correspond to decreasing CO2e emissions per kg ECM.
The importance of decreasing CO2e emissions intensity is illustrated by a predicted increase in total CO2e emissions of 41% in Figure 1 under a constant emissions intensity scenario, in comparison to a much lower 1.8% increase in total CO2e emissions if CO2e intensity continued to decrease in the 2017–2050 period at a similar pace as it did in the 2007–2017 period. However, according to these projections based on the 2007–2017 period, and if industry growth continues, even with decreasing emissions intensity, if there are no additional mitigation measures, the goal of carbon neutrality for the U.S. dairy industry by 2050 (Table 1) (Innovation Center for U.S. Dairy, 2020) would not be achieved, and there would even be a small increase in total CO2e emissions by 2050.
In developing countries with low productivity systems, there is potential for greater decrease in emissions intensity through increasing animal productivity (Gerber et al., 2011). This approach can help both rural development and food security, but enhancing productivity can also incentivize the use of resources and expansion of land for agriculture, offsetting gains in emissions intensity (Leahy et al., 2020; Reisinger et al., 2021).
Pronounced Decrease of Enteric Methane Emissions
Globally, enteric CH4 emissions are the predominant source of greenhouse gases from ruminant production to the farm gate, comprising 46, 43, 60, and 55% of total CO2e emissions for dairy, beef, buffalo and small ruminant production, respectively (Gerber et al., 2013b). A decrease in the emissions of enteric CH4 of 20% by 2030 from a 2020 baseline was estimated as necessary to keep global temperature increase within 1.5°C (United Nations Environment Programme and Climate and Clean Air Coalition, 2021). By 2050, a 24–47% (interquartile range) decrease in agricultural CH4 emissions relative to 2010 is estimated as necessary to limit global temperature increase to 1.5°C (IPCC, 2018). Otherwise, the pressure to rapidly decrease CO2 emissions will become even more stringent than what it is currently (Wollenberg et al., 2016; Leahy et al., 2020; Reisinger et al., 2021). If ruminant production expands to accommodate the global increases in the demand for meat and milk production (73 and 58%, respectively, by 2050; Beauchemin et al., 2020), CH4 emissions per unit of meat and milk would have to decrease by 56–69% and 52–66%, respectively (calculations not shown) to meet the goal of decreasing enteric CH4 emissions by 24 to 47% during the same time period (relative to 2010), in accordance with mitigation targets set for agricultural CH4. However, with current trends agricultural CH4 emissions are projected to increase by 30% by 2050 relative to 2010 (Reisinger et al., 2021). The large increases in global meat and milk production required to meet human population demands are difficult to accommodate with the enteric CH4 amelioration goals unless measures to strongly decrease CH4 production from individual animals are introduced in livestock production systems.
Various strategies to decrease enteric CH4 emissions from ruminants are being investigated, among them, dietary changes such as additions of concentrates and oils, changes in forage composition and quality, chemical inhibitors of rumen methanogenesis, ionophores, alternative electron acceptors to CO2, plant secondary compounds, algae containing compounds inhibiting methanogenesis, selection of low-CH4 producing animals, vaccination against methanogens, archaeal phages, and others (Martin et al., 2010; Cottle et al., 2011; Hristov et al., 2013a; Knapp et al., 2014; Goopy, 2019; Beauchemin et al., 2020; Terry et al., 2020). Measures that decrease absolute enteric CH4 emissions or CH4 emissions intensity moderately (e.g., <20%) are likely to be offset partially or totally by the predicted increases in animal numbers and/or total animal production. For net zero goals of CO2e emissions from the ruminant production sector to be realized or at least approached, emissions of enteric CH4 as well as fossil fuel CO2 and N2O emissions and manure CH4, would have to be decreased substantially. Strategies that can mitigate enteric CH4 production pronouncedly should at the same time not significantly increase upstream and downstream emissions of fossil fuel CO2, N2O, and manure CH4.
Meta-analyses have identified the dietary inclusion of chemical inhibitors of methanogenesis and the bromoform-containing, red algae Asparagopsis spp., as the most effective strategies to decrease both total enteric CH4 emissions per animal and enteric CH4 emissions expressed per unit of dry matter intake (DMI), or CH4 yield (Veneman et al., 2016; Almeida et al., 2021; Arndt et al., 2021). Although on average the decrease in enteric CH4 yield (CH4 produced per unit of DMI) caused by chemical inhibitors of methanogenesis was 25% (Veneman et al., 2016), 34% (Arndt et al., 2021) or 23% (Almeida et al., 2021), the antimethanogenic effects of chemical inhibitors are dose-dependent (Mitsumori et al., 2012; Martinez-Fernandez et al., 2016; Dijkstra et al., 2018), and considerably greater decreases in CH4 production e.g., >80% are possible (Table 3). Similarly, the decrease in CH4 production and yield by Asparagopsis spp. is related to its dose inclusion (Li et al., 2016; Roque et al., 2019, 2021; Kinley et al., 2020), and considerably greater inhibition of CH4 production than the 49% average (Almeida et al., 2021) has been reported (Table 3). Whilst all studies in Table 3 were conducted with beef and sheep, slightly less but still severe inhibition of methanogenesis (i.e., between 60 and 70%) has been reported in studies conducted with lactating dairy cows (Haisan et al., 2014; Roque et al., 2019).
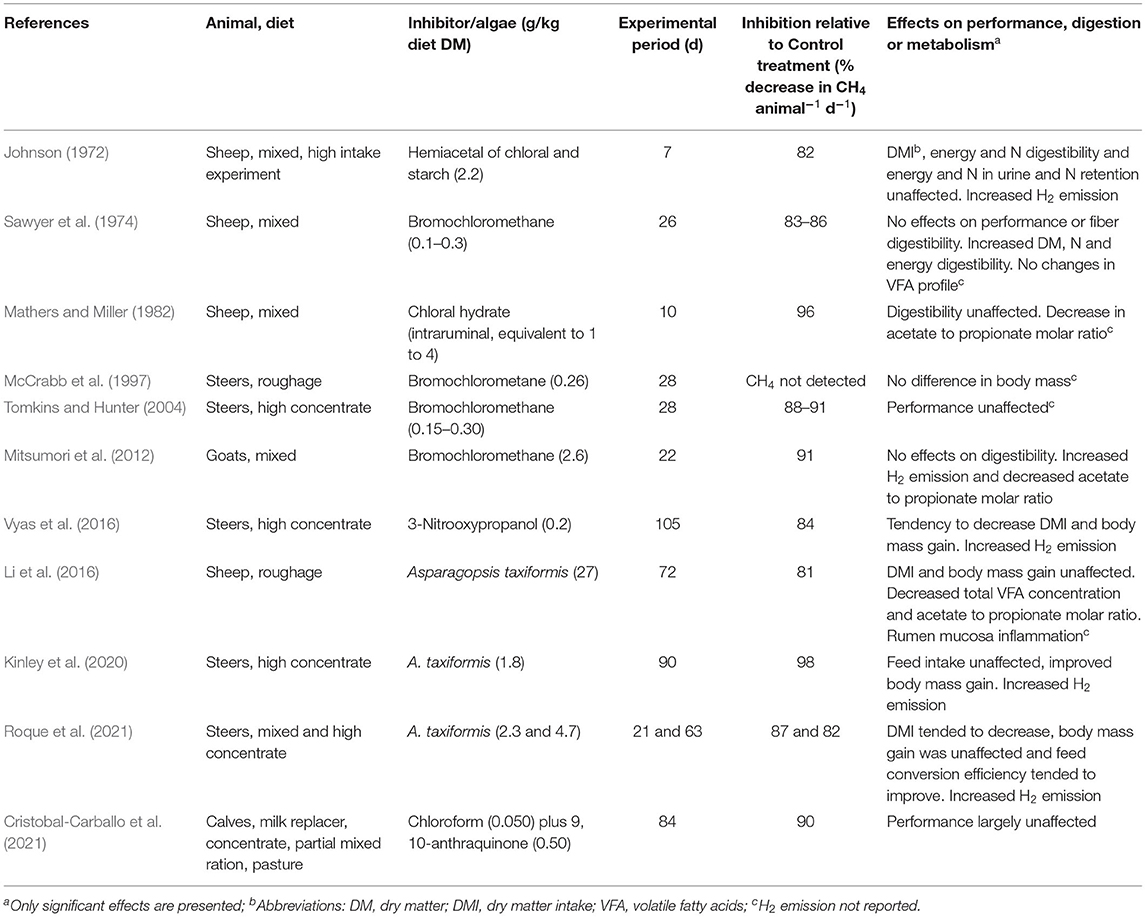
Table 3. In vivo experiments reporting at least one treatment resulting in 80% or more inhibition of rumen methanogenesis.
Currently, chemical inhibitors of methanogenesis and bromoform-containing algae are the most potent proven means to mitigate the emissions of enteric CH4. Equally important, dosing animals with the small amounts required of these additives can largely dilute the upstream emissions of CO2e from fossil fuels required to produce and transport those additives. It must be considered, however, that solutions to decrease CH4 emissions should not negatively affect animal productivity and health, must be cost effective, feasible for adoption in different production systems, safe for the environment and humans, must be approved by government agencies, and must be acceptable to consumers. The inclusion of chemical inhibitors of methanogenesis or bromoform-containing algae in animal feeds will increase feeding costs, and, all else unchanged, could decrease the economic benefits of animal production, which is regarded as critical for wide adoption of CH4 mitigation strategies by producers (Hristov et al., 2013b).
It has long been known that CH4 formation in the rumen and its release to the atmosphere is an energy loss for ruminants (Ritzman and Benedict, 1938). Whilst scientists have attempted to inhibit rumen methanogenesis to improve energy efficiency (Czerkawski and Breckenridge, 1975), this theoretical potential has not been realized through consistent improvements in the efficiency of milk production or animal growth and fattening (Ungerfeld, 2018). Herein, we propose avenues to make the methanogenesis inhibition intervention cost effective through the understanding of its biological consequences. First, we examine through meta-regressions the effects of pronounced inhibition of rumen methanogenesis on rumen and animal variables, discuss possible consequences on post-absorptive metabolism, and identify knowledge gaps that still need to be filled through research (sections Meta-Regressions and Thermodynamic Calculations and Consequences of Inhibiting Enteric Methane Production). In section Cost Effectiveness of Inhibiting Rumen Methanogenesis, we discuss how understanding those biological responses can potentially be used to improve the cost effectiveness of the methanogenesis inhibition intervention.
Meta-Regressions and Thermodynamic Calculations
We analyzed responses in rumen fermentation and animal variables to the inhibition of enteric CH4 production with chemical inhibitors or bromoform-containing algae to understand possible changes in the absorption and utilization of metabolites, which can affect the responses of animal production to the methanogenesis inhibition intervention. Chemical inhibitors and bromoform-containing algae are included in minimal amounts in animal feed, so that the supply of nutrients by those additives is non-existent or negligible, and therefore the effects of methanogenesis inhibition are not confounded with changes in diet composition. A data base of in vivo studies in which enteric CH4 yield was inhibited by 50% or more through the use of chemical compounds or algae was compiled (Ungerfeld, 2021) (Supplementary Table 1). For the meta-regressions, we lowered the 80% methanogenesis inhibition threshold of Table 3 to 50% methanogenesis inhibition to include more studies, because some of the studies in Table 3 did not report all of the responses of interest. The experiments by Johnson (1972), Martinez-Fernandez et al. (2016) and Roque et al. (2021) contained more than one control, as they compared the effects of methanogenesis inhibitors across two levels of DMI (Johnson, 1972) or more than one diet (Martinez-Fernandez et al., 2016; Roque et al., 2021). In those three studies, each set of treatments was separately regressed against its corresponding control. Response variables studied were DMI, energy exhaled in dihydrogen (H2), rumen acetate to propionate molar ratio, rumen propionate molar percentage in total volatile fatty acids (VFA), and rumen molar concentrations of total VFA, acetate, propionate and butyrate.
Animal productivity can be strongly affected by DMI. We first studied the relationship between rumen methanogenesis inhibition and DMI by regressing DMI against the linear and quadratic terms of CH4 production, the random effect of the experiment, and the interaction between the random effect of the experiment and the linear effect of CH4 production.
A typical effect of the inhibition of rumen methanogenesis is an increase in the release of H2 in eructation and respiration (Czerkawski, 1986). Similarly to CH4, the release of H2 also represents a loss of energy, hence it is important to understand what proportion of the energy spared in CH4 not formed when methanogenesis is inhibited is lost to the atmosphere as H2. Energy loss in exhaled H2 was modeled as a function of the random effect of the experiment, the linear effect of energy in exhaled CH4, and the interaction between the random effect of the experiment and the linear effect of energy in exhaled CH4. Because the relationship was non-linear, square root transformations of both the regressor and response variables were conducted. Heats of combustion of H2 and CH4 were obtained from Domalski (1972).
To understand the effects of methanogenesis inhibition on VFA and possible consequences on post-absorptive metabolism, the rumen acetate to propionate molar ratio, propionate molar percentage, and molar concentration of total VFA, acetate, propionate and butyrate were likewise regressed against the linear and quadratic terms of CH4 production, the random effect of the experiment, and the interaction between the random effect of the experiment and the linear effect of CH4 production. Responses were adjusted for DMI, which was included as a co-variable to avoid possible confounding effects of alterations of DMI on response variables when inhibiting rumen methanogenesis (Ungerfeld, 2018). Individual VFA concentrations were calculated by multiplying total VFA concentration by each individual VFA molar percentage.
In all models fit, quadratic terms and interactions with P ≥ 0.10 were removed and the reduced model refitted. A standard procedure in meta-regression is weighting each treatment mean by the reciprocal of its standard error normalized to unity (Sauvant et al., 2008). Standard errors of H2 emission, total VFA concentration, and propionate molar percentage were not available for all of the experiments meta-regressed; for those response variables, models built with both weighted and non-weighted treatment means are reported. In the case of acetate, propionate and butyrate concentration, standard errors were not available for any experiment, as treatment means for those variables were calculated from total VFA concentration and each individual VFA molar percentage; thus, only models built with non-weighted treatment means are reported for acetate, propionate and butyrate concentration.
JMP® (2016) was used in all statistical analyses.
We also estimated if accumulation of H2 might affect fermentation through the inhibition of NADH oxidation to NAD+. Gibbs energy change (ΔG) of NADH oxidation in confurcation with reduced ferredoxin to produce H2 (NADH + + 3 H+ → NAD+ + Fdox + 2 H2) was calculated based on ΔG° = −102 KJ/mol (Van Lingen et al., 2016), R = 8.3145 L kPa mol−1 K−1, T = 312 K, NAD+/NADH = 2.56 (Hino and Russell, 1985), Fdox/ = 0.1 (Buckel and Thauer, 2013) and intracellular pH = 7.0 (Van Lingen et al., 2016).
Consequences of Inhibiting Enteric Methane Production
Inhibiting methanogenesis cannot be considered an isolated intervention. It causes profound changes in the flows of metabolic hydrogen in rumen fermentation, and eventually in the animal's post-absorptive metabolism (Ungerfeld, 2018, 2020). It is important to understand the consequences of inhibiting rumen methanogenesis to design strategies to adapt and optimize the animal nutritional management to take advantage of physiological changes occurring. The ultimate goal is to achieve a pronounced and sustained decrease in enteric CH4 production while maintaining or improving animal productivity and profitability.
Because CH4 release to the atmosphere is a loss of energy for ruminants, it has been proposed that inhibiting enteric CH4 production can improve ruminant productivity (Czerkawski and Breckenridge, 1975). However, a previous meta-analysis found that inhibiting enteric CH4 production did not consistently enhance animal productivity, which was partially attributed to the decrease in CH4 production not being sufficiently pronounced for energy savings to be evident (Ungerfeld, 2018; Beauchemin et al., 2020). A recent large scale trial with growing cattle receiving 3-NOP found a tendency toward improved feed efficiency associated to a 26% decrease in CH4 emissions (Alemu et al., 2021). The latter result suggests that a large number of animals may be required for experiments to have statistical power to detect significant gains in productivity when CH4 decrease and energy savings are moderate. Likewise, some studies with much fewer animals but a pronounced inhibition of enteric CH4 production reported increases in the efficiency of feed conversion to body mass gain (Davies et al., 1982; McCrabb et al., 1997; Vyas et al., 2016; Kinley et al., 2020; Roque et al., 2021). In this regard, both the environmental need to achieve a strong mitigation in the emissions of enteric CH4 as a greenhouse gas (section Pronounced Decrease of Enteric Methane Emissions) and the theoretical expectations that energy savings from CH4 emissions could translate into benefits in animal productivity, point toward seeking pronounced inhibition of rumen methanogenesis as a goal for ruminant production.
In our meta-analysis, inhibition of rumen methanogenesis by 50% or more was overall associated with lower DMI (P = 0.020; Figure 2), in agreement with a previous meta-analysis comprising a broader range of extent of methanogenesis inhibition (Ungerfeld, 2018). A positive relationship between CH4 production and DMI within a large intercontinental database has been reported (Niu et al., 2018). In that study, however, differences in DMI were the result of different diets, hence it was DMI that drove CH4 production as a response. In the present analysis, diet composition was virtually constant within each experiment, therefore changes in DMI were the result of the addition of very small amounts of antimethanogenic additives, and not of changes in diet composition.
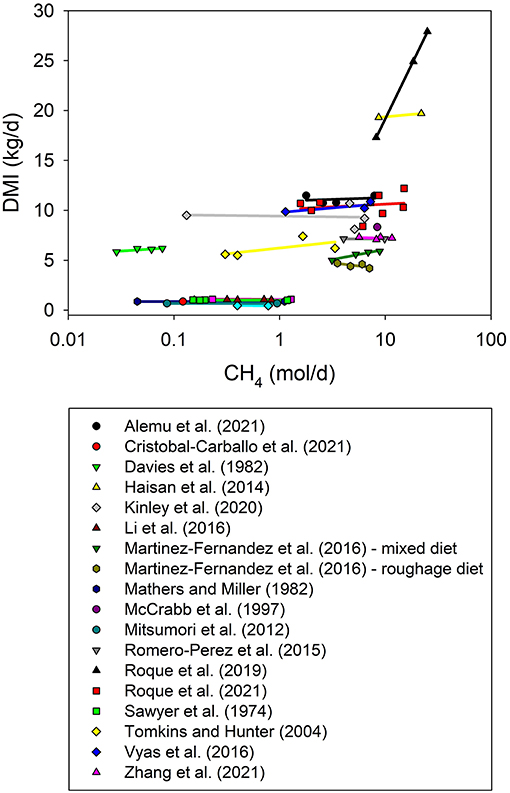
Figure 2. Relationship between dry matter intake (DMI, kg/d) and methane production (CH4, mol/d) by experiment. The x-axis is log10-scaled for better visualization of low CH4 experiments. The adjusted model included the random effect of the experiment (exp) and its interaction with DMI: (1) non-weighted treatment means (N = 64): DMI = 6.80 (± 1.10; P < 0.001) + exp, random (P = 0.003) + 0.14 (± 0.054; P = 0.020) CH4 + exp × CH4, random (P = 0.022); R2 = 0.99; (2) weighted treatment means (N = 46): DMI = 7.93 (± 1.32; P < 0.001) + exp, random (P = 0.012) + 0.14 (± 0.052; P = 0.021) CH4 + exp × CH4, random (P = 0.033); R2 = 0.99.
We found an interaction between CH4 production and the random effect of the experiment on DMI (P = 0.022; Figure 2). In order to understand the interaction between methanogenesis inhibition and the experiment effect on DMI, the random effect of the experiment, both as a main effect and in its interaction with energy losses in CH4, was replaced by: (i) the fixed effect of the type of animal (sheep, goats, heifers, steers or dairy cows), (ii) the fixed effect of the percentage of concentrate in the diet, or (iii) the fixed effect of the type of inhibitor, including halogenated compounds as a group [hemiacetal of chloral and starch, chloral hydrate, chloroform, bromochloromethane, and bromoform contained in Asparagopsis spp. (Stefenoni et al., 2021)] or 3-nitrooxypropanol (3-hydroxypropyl nitrate, or 3-NOP). There were no interactions between CH4 production and the type of animal (P = 0.74) or the percentage of concentrate in the diet (P = 0.23); however, the decrease in DMI with methanogenesis inhibition was more pronounced when CH4 production was inhibited by halogenated compounds than with 3-NOP (P < 0.001; results not shown). Palatability aspects might contribute to explain the interaction, as low palatability has been reported in some studies with Asparagopsis spp. (Muizelaar et al., 2021; Stefenoni et al., 2021), and in contrast, no palatability issues were found with 3-NOP (Lee et al., 2020).
Dihydrogen is a central metabolite in rumen fermentation that is rapidly produced and consumed chiefly by methanogenesis, but also by other pathways such as propionate formation. Because of its fast turnover rate, only traces of H2 are found in the typical rumen fermentation (Hungate, 1967; Ungerfeld, 2020). Scientists conducting the first experiments in which rumen methanogenesis was inhibited in vitro or in vivo noticed the occurrence of an atypical accumulation of H2 (Bauchop, 1967; Rufener and Wolin, 1968; Trei et al., 1971). In the 15 experiments analyzed herein including at least one treatment in which CH4 production was inhibited by 50% or more, the response in energy losses as H2 as function of energy in CH4 was non-linear and variable (Figure 3). Losses of energy in H2 as a proportion of energy saved in CH4 not formed increased with methanogenesis inhibition. For example, energy losses as H2 predicted by the model at 50, 80 and 100% inhibition of methanogenesis represented 7.1% (CI95 = [1.8, 12%]), 11% (CI95 = [4.5, 17%]) and 19% (CI95 = [9.2, 29%]), respectively, of the energy spared from CH4 production with respect to Control treatments.
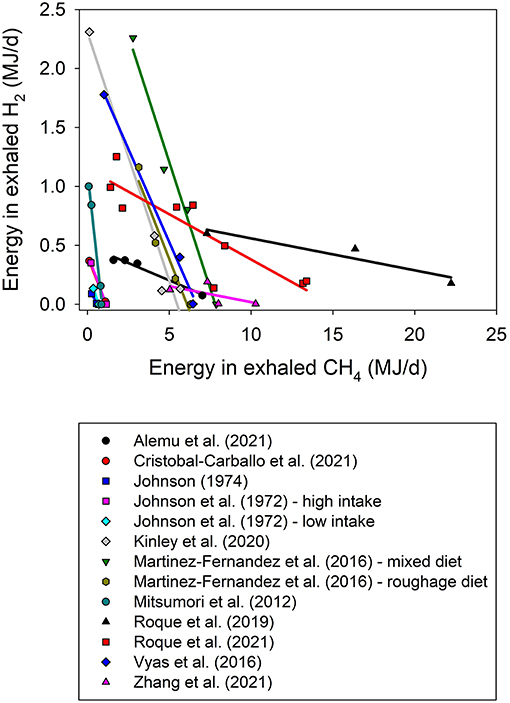
Figure 3. Energy losses as exhaled dihydrogen (H2, MJ/d) as a function of energy losses as exhaled methane (CH4, MJ/d) by experiment. The adjusted model included the random effect of the experiment (exp) and both response and regressor were root-square transformed: (1) non-weighted treatment means (N = 48): √H2 = 1.23 (±0.18; P < 0.001) + exp (P = 0.057) – 0.38 (±0.065; P < 0.001) √CH4; (2) weighted treatment means (N = 33): √H2 = 1.13 (±0.17; P < 0.001) + exp (P = 0.40) – 0.28 (±0.070; P < 0.001) √CH4.
Apart from causing a loss of energy as exhaled H2, elevated H2 concentration in the rumen can inhibit NADH re-oxidation and hence fermentation (Wolin et al., 1997). We examined the theoretical and empirical evidence of the possibility of H2 accumulation inhibiting fermentation and digestion. When rumen methanogenesis is inhibited, concentration of dissolved H2 increases from its typical range of 0.2–30 μM (Ungerfeld, 2020) to up to 550 μM (Zhang et al., 2020). NADH-ferredoxin confurcation appears as a predominant mechanism of NADH re-oxidation to H2 in the rumen as genes encoding for reversible bifurcating hydrogenases are widespread in rumen microorganisms and are highly transcribed (Greening et al., 2019). Given that, we estimated ΔG of NADH oxidation in confurcation with reduced ferredoxin as a function of dissolved H2 concentration. At 550 μM dissolved H2, the reaction would still appear to be weakly exergonic (ΔG = −18.5 kJ; Supplementary Figure 2). In situ fiber degradation at 550 μM H2 was not compromised (Zhang et al., 2020). Similarly, other studies and meta-analyses have not found effects of the inhibition of methanogenesis on apparent digestibility in the overall tract (Jayanegara et al., 2018; Ungerfeld, 2018; Kim et al., 2020) and in situ (Nolan et al., 2010; Martínez-Fernández et al., 2014).
Total VFA concentration can also be a proxy to evaluate the effects of inhibiting rumen methanogenesis on fermentation. Total VFA concentration in the rumen was unaffected by CH4 production adjusted by DMI (P = 0.29; Figure 4). However, the use of total VFA concentration, rather than VFA actual production, as a proxy of fermentation has limitations as VFA concentration is not a sole result of VFA production but it is also affected by VFA absorption, passage, incorporation into microbial biomass, and changes in rumen volume (Dijkstra et al., 1993; Kristensen, 2001; Hall et al., 2015). Even though, digestion and fermentation did not seem to be impaired by the inhibition of methanogenesis and H2 accumulation, it is recommendable to generate results on actual VFA production and true nutrient digestibility to better understand the consequences of elevated H2 concentration resulting from methanogenesis inhibition on fermentation and digestion.
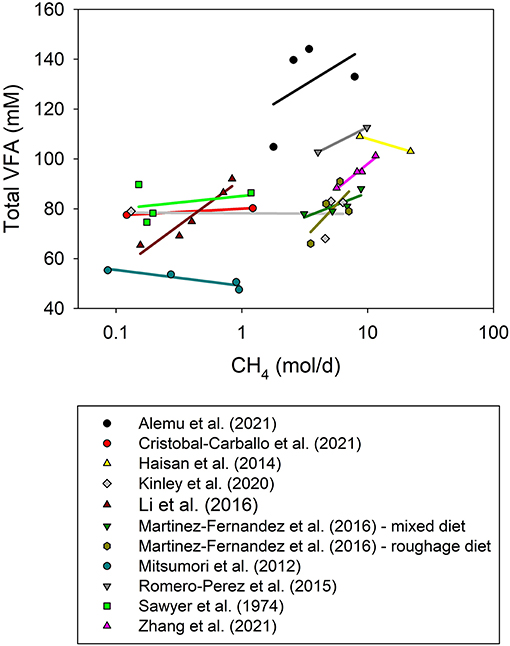
Figure 4. Relationship between total volatile fatty acid concentration (VFA, mM) in the rumen and methane production (CH4, mol/d) by experiment. The x-axis is log10-scaled for better visualization of low CH4 experiments. The adjusted model included the random effect of the experiment (exp) and dry matter intake (DMI, kg/d): (1) non-weighed treatment means (N = 37): total VFA = 75.5 (±7.73; P < 0.001) + exp, random (P = 0.067) + 1.48 (±1.06; P = 0.18) DMI + 0.74 (±0.69; P = 0.29) CH4; R2 = 0.84; (2) weighted treatment means (N = 33): total VFA = 74.0 (±6.69; P < 0.001) + exp, random (P = 0.24) + 2.08 (±1.13; P = 0.086) DMI + 0.62 (±097; P = 0.53) CH4; R2 = 0.71.
Inhibiting methanogenesis shifts rumen fermentation from acetate to propionate (Figure 5 and Supplementary Figure 2), which has been explained based on the Monod function of microbial growth and displacement of thermodynamic equilibrium between acetate and propionate (Janssen, 2010). Even though the acetate to propionate molar ratio decreased with methanogenesis inhibition (P < 0.001; Figure 5), no clear overall relationship between CH4 production adjusted by DMI and rumen propionate concentration was observed in the present analysis (P = 0.12; Figure 6). There was a linear decline in acetate concentration with decreased CH4 production adjusted by DMI (P = 0.015; Supplementary Figure 3), and no association with rumen butyrate concentration (P = 0.95; Supplementary Figure 4).
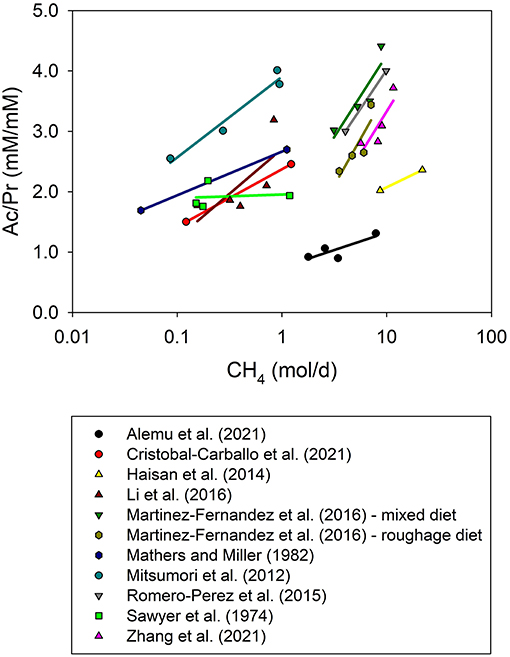
Figure 5. Relationship between the rumen acetate to propionate concentration molar ratio (Ac/Pr, mM/mM) and methane production (CH4, mol/d) by experiment. The x-axis is log10-scaled for better visualization of low CH4 experiments. The adjusted model included the random effect of the experiment (exp) and dry matter intake (DMI, kg/d): (1) non-weighted treatment means (N = 35): Ac/Pr = 2.53 (±0.26; P < 0.001) + exp, random (P = 0.10) – 0.11 (±0.043; P = 0.021) DMI + 0.13 (±0.034; P < 0.001) CH4; R2 = 0.82; (2) weighted treatment means (N = 27): Ac/Pr = 2.98 (±0.39; P < 0.001) + exp, random (P = 0.15) – 0.14 (±0.050; P = 0.019) DMI + 0.12 (±0.036; P = 0.003) CH4; R2 = 0.85.
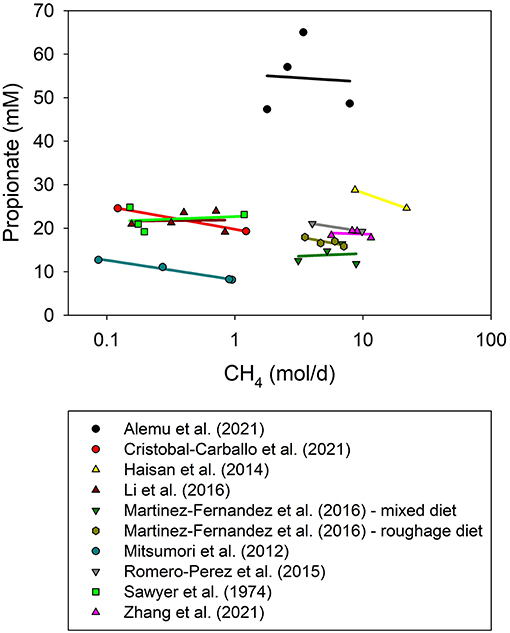
Figure 6. Relationship between rumen propionate concentration (Pr, mM) and methane production (CH4, mol/d) by experiment. The x-axis is log10-scaled for better visualization of low CH4 experiments. The adjusted model included the random effect of the experiment (exp) and dry matter intake (DMI, kg/d): Pr = 18.7 (±4.86; P < 0.001) + exp, random (P = 0.062) +1.06 (±0.64; P = 0.13) DMI – 0.45 (±0.28; P = 0.12) CH4; R2 = 0.94 (N = 33). Treatment means are not weighted by the reciprocal of the standard error (SEM) normalized to unity, as SEM were not reported.
The effects of inhibiting rumen methanogenesis on total and individual VFA concentration in vivo have been reported in numerous studies, but changes in VFA production and absorption caused by the inhibition of methanogenesis have not been characterized. Individual VFA have different post-absorptive effects, and changes in the absorbed VFA profile could have profound effects on ruminant post-absorptive metabolism (DiCostanzo et al., 1999). It would be important to determine the in vivo response of propionate production to methanogenesis inhibition, because propionate is the principal glucose precursor in ruminants (Aschenbach et al., 2010; Larsen and Kristensen, 2013). The response of gluconeogenesis to a potential increase in propionate absorption from the rumen would depend on both the animal's requirements for glucose and the liver uptake of other glucogenic precursors (Loncke et al., 2020). Acetate and propionate have differential effects on the synthesis of milk fat and protein, as shown in intraruminal infusion experiments (Ørskov et al., 1969; Sheperd and Combs, 1998; Maxin et al., 2011; Urrutia and Harvatine, 2017). Also, propionate is a satiety signal in ruminants and an increase in propionate absorption may thus decrease voluntary intake (Allen, 2014). A stimulation of satiety may partially explain the decrease in DMI with methanogenesis inhibition found herein with experiments with >50% inhibition, and previously with a greater range of methanogenesis inhibition (Ungerfeld, 2018).
If the decrease in rumen acetate concentration resulting from methanogenesis inhibition reflected lower acetate production, an ensuing lower flow of acetate absorption could be a potential concern as it would be expected to decrease milk fat content (Maxin et al., 2011; Urrutia and Harvatine, 2017). However, experimental evidence with 3-NOP showed, on the contrary, a tendency to increase milk fat content (Kim et al., 2020). In any case, if some chemical inhibitors of methanogenesis cause decreases in milk fat content, a possibility to overcome this problem, at least partially, could be dosing reductive acetogens to harness accumulated H2 in acetate production (Ungerfeld, 2020).
An increase in plasma glucose concentration in dairy cows with rumen methanogenesis inhibited by 3-NOP might reflect an increase in propionate absorption (Haisan et al., 2017). Conversely, the lack of effect of 3-NOP on plasma glucose concentration observed by Melgar et al. (2020a) may agree with the numerical lack of effect of 3-NOP on propionate concentration (as estimated from total VFA concentration and propionate molar percentage) in that study, if lack of change in propionate concentration in turn indicated lack of effect of 3-NOP on propionate production. Melgar et al. (2020a) suggested that the lower plasma insulin concentration that they observed concomitant with unchanged circulating glucose could be the result of favored glucose partitioning toward milk production in detriment of other tissues competing for glucose uptake.
Less research has examined the consequences of inhibiting rumen methanogenesis on microbial anabolism and its implications for the synthesis of microbial protein, the major source of amino acids for ruminants. Inhibition of methanogenesis in vitro causes a severe and consistent decrease in the recovery of metabolic hydrogen in propionate, butyrate, CH4 and H2, and it was speculated that some of the missing reducing equivalents might be accounted for by incorporation of ammonium into carbon chains to synthesize microbial amino acids (Ungerfeld, 2015). Inhibiting methanogenesis in in vitro rumen cultures has increased microbial nitrogen production in some studies (Guo et al., 2007; Ungerfeld et al., 2007) but not in others (Russell and Martin, 1984; Ungerfeld et al., 2019). In another in vitro study, methanogenesis inhibition stimulated microbial de novo synthesis of amino acids from ammonium with a starch substrate, but not with a less fermentable cellulose substrate (Ungerfeld et al., 2020). Mitigation of enteric CH4 may indirectly affect nitrogen metabolism of the animal, but more research is needed to understand the changes elicited by methanogenesis inhibition.
Effects of inhibiting rumen methanogenesis on microbial long chain fatty acid metabolism have received little attention, and have mostly focused on microbial biohydrogenation of dietary long chain fatty acids rather than on microbial synthesis of long chain fatty acids, a process highly demanding of metabolic hydrogen (Ungerfeld, 2015). Czerkawski et al. (1975) supplemented sheep with linseed oil, and observed a stimulation of the incorporation of 14C-labeled acetate into bacterial lipids, although CH4 production was not reported in that study. Inhibiting rumen methanogenesis in sheep and in vitro with carbon tetrachloride, chloroform or amichloral inhibited biohydrogenation of unsaturated long chain fatty acids, in particular the reductive steps (Kemp and Lander, 1976). In another study with sheep, the methanogenesis inhibitor chloral hydrate had little effect on long chain fatty acid composition of digesta flowing to the small intestine (Mathers and Miller, 1982). 3-Nitrooxypropanol supplemented to heifers did not affect total long chain fatty acids in rumen contents but increased content of saturated fatty acids (Zhang et al., 2021).
Rumen biohydrogenation of long chain fatty acids has important implications for milk fat synthesis (Dewanckele et al., 2020) and fat composition of milk (Nguyen et al., 2019) and meat (Scollan et al., 2017). An increase in the percentage of de novo synthesized short- and medium-chain fatty acids in milk fat of dairy cows and goats supplemented with 3-NOP or bromochloromethane has been reported, as well as less consistent decreases in vaccenic and rumenic acids, and total mono- and polyunsaturated fatty acids, and increases in total saturated fatty acids (Abecia et al., 2012; Hristov et al., 2015; Melgar et al., 2020a,b, 2021). Some of those changes, along with the changes in long chain fatty acids in rumen contents observed by Zhang et al. (2021) might suggest a stimulation of the last steps of rumen biohydrogenation by increased availability of metabolic hydrogen resulting from the inhibition of methanogenesis.
Cost Effectiveness of Inhibiting Rumen Methanogenesis
Inclusion of chemical inhibitors of methanogenesis or antimethanogenic algae in ruminant diets will represent an additional feeding cost. For the adoption of these additives by producers, their addition to ruminant diets will have to be made cost-effective through economic incentives, improvements in animal productivity, favorable physiological changes, or combinations of those possibilities. It is also possible that widespread adoption of antimethanogenic additives will allow scaling up their production thereby decreasing their cost.
Climate friendly labeling of animal products can allow reaching high value niche markets, although the range of consumers willing to pay a premium price may not be broad enough (Leahy et al., 2020). Economic incentives to farmers have been used successfully to decrease greenhouse gas emissions. For example, in France a scheme of Payment for Environmental Services to producers was introduced to stimulate the use of omega-3 rich feed resources such as linseed and grass fodders, resulting in an estimated 11% decrease in CO2e emissions (Le Gloux et al., 2021). A rising global tax to CH4 emissions has also been proposed (United Nations Environment Programme and Climate and Clean Air Coalition, 2021). Possible carbon pricing and taxation schemes to livestock production and regulatory policies have been discussed (FAO, 2019).
Improving cost effectiveness of inhibiting rumen methanogenesis could result from the incorporation of increased H2 emission into additional propionate formation or reductive acetogenesis (Ungerfeld, 2013, 2020). An increase in propionate contribution to gluconeogenesis may save other glucose precursors, such as glucogenic amino acids, for alternative uses. Alternatively, basal diets could perhaps be formulated to be less glucogenic when rumen CH4 production is inhibited, and still meet animal requirements for glucose (Ungerfeld, 2018). This reformulation of diets could decrease the use of concentrates in regions or countries where they are relatively expensive or little available, and contribute to making the methanogenesis inhibition intervention cost effective even if animal productivity is not improved. It is true that diets with less concentrates are more methanogenic (Johnson and Johnson, 1995; Janssen, 2010), but potent inhibitors of methanogenesis can override the diet effect and cause a strong inhibition in enteric CH4 even with high roughage diets (McCrabb et al., 1997; Martinez-Fernandez et al., 2016; Roque et al., 2021). Research is needed on the optimization of the flows of metabolic hydrogen in rumen fermentation and on understanding and matching changes in absorbed VFA to basal diet composition and animal requirements.
If inhibiting methanogenesis stimulates synthesis of microbial amino acids with fermentable diets, it may be possible to replace expensive plant protein supplements with urea (Ungerfeld et al., 2020), which might also help the cost-effectiveness of the intervention.
Other Aspects
Most CH4 mitigation strategies have been developed for intensive production systems in industrialized economies (Harrison et al., 2021), and a critical point to implementation at the farm level is that both chemical inhibitors of methanogenesis and bromoform-containing algae have demonstrated CH4 mitigation effects when incorporated into the ration and supplemented to animals on a daily basis. Globally, 47% of total enteric CH4 is produced by ruminants in grazing systems, with approximately one-half of grazing enteric CH4 emissions coming from non-dairy ruminants [calculated from FAO (2017)]. In particular, beef and sheep are often raised under extensive grazing, especially in developing countries. The possibilities of supplementing those animals with inhibitors mixed with concentrates may be limited as the animals are typically not managed daily, or concentrates may not be available or may be expensive. It would be useful to develop means to deliver inhibitors of methanogenesis into the rumens of extensively grazing animals on a daily or continuous basis. Some possibilities could be slow-release rumen devices lasting for several months, inclusion of additives in blocks of salt and molasses, delivery in drinking water, or genetically modifying forages to produce inhibitors of methanogenesis. The latter possibility, however, may have environmental implications as inhibitors synthesized by plants would be less controllable compared to feeding the inhibitors directly to the animals.
Apart from the use of chemical inhibitors of methanogenesis and bromoform-containing algae, another possible approach to enhance the inhibitory effects of antimethanogenic interventions is to combine various strategies which, when applied alone may exert mild or moderate effects on enteric CH4 production, but may achieve stronger effects when combined (Supplementary Table 2). The strongest effects of combinations of two antimethanogenic strategies in Supplementary Table 2 occurred when nitrate (Van Zijderveld et al., 2010; Guyader et al., 2015, 2016) or 3-NOP (Smith, 2017; Vyas et al., 2018) were included. We are aware of only one study combining more than two antimethanogenic strategies: the combination of lauric and myristic acids, linseed oil and calcium fumarate decreased CH4 production by only 10% in lactating dairy cows (van Zijderveld et al., 2011). Although in none of the studies in Supplementary Table 2 the decrease in enteric CH4 production was as large as in experiments in Table 3 with chemical inhibitors and bromoform-containing algae, this line of research has yet to study various approaches to combining antimethanogenic strategies. Thus, it is still possible that stronger decreases in enteric CH4 could be obtained in the future through combinations of treatments.
Mitigating greenhouse gases emissions from ruminant production requires a multi-factorial approach, including interventions to decrease enteric CH4 production, replacing fossil fuels used in feed production with renewable sources of energy, using manure to generate biogas and recycling nutrients to soils to reduce the use of or replace chemical fertilizers (FAO, 2019). Carbon sequestration in soil is regarded as a tool to potentially offset enteric CH4 emissions from grazing ruminants, especially in developing countries and locations with degraded soils. However, soil carbon content cannot increase indefinitely (Harrison et al., 2021) and therefore carbon sequestration in soil alone cannot offset raising emissions of greenhouse gases in the long term. Amelioration in the emissions of greenhouse gases from livestock can also be approached through the demand side by decreased consumption and waste. However, livestock production has important economic, social, cultural and environmental roles that must also be considered. Nutritionally balanced substitutions of livestock products may not be feasible for all human populations. Also, shifting pastures to croplands will result in oxidation of soil organic matter and increased CO2 emissions (Leahy et al., 2020; Reisinger et al., 2021).
Conclusions
We propose that substantial decreases in the emissions of enteric CH4 from individual animals are required to decrease global enteric CH4 emissions from livestock, in view of the expected global growth of animal production. Chemical inhibitors of methanogenesis and bromoform-containing algae can both cause pronounced inhibition of rumen methanogenesis, but there are some challenges to their widespread adoption. Safety to animals, consumers and the environment has to be ensured, as well as approval by government agencies and acceptance by consumers. Incorporation of additives in ruminant diets has to be made profitable by an increased price paid to producers for animal products and/or enhanced productivity derived from optimizing animal feeding for the methanogenesis inhibition intervention. Research is needed to fully understand the consequences of methanogenesis inhibition on rumen fermentation and post-absorptive metabolism so that nutritional strategies can be designed that optimize the flow of absorbed nutrients altered by the methanogenesis inhibition intervention to match animal requirements and potentially improve animal productivity and efficiency.
Data Availability Statement
Publicly available datasets were analyzed in this study. This data can be found at: Open Science Framework (https://osf.io/xsfe9/?view_only=5bc486ae58314284a5e09b6243e8bcc3) doi: https://osf.io/xsfe9/.
Ethics Statement
Ethical review and approval was not required for the animal study because the present research presents re-analysis (meta-analysis) of previously published research results with ruminants. The present research did not involve any experimentation.
Author Contributions
EU conceived the hypothesis, conducted the research, and wrote the original draft. KB and CM contributed ideas, reviewed, and edited the manuscript. All authors contributed to the article and approved the submitted version.
Funding
This work was funded by Agencia Nacional de Investigación y Desarrollo (ANID), Project Fondecyt 1190574.
Conflict of Interest
The authors declare that the research was conducted in the absence of any commercial or financial relationships that could be construed as a potential conflict of interest.
Publisher's Note
All claims expressed in this article are solely those of the authors and do not necessarily represent those of their affiliated organizations, or those of the publisher, the editors and the reviewers. Any product that may be evaluated in this article, or claim that may be made by its manufacturer, is not guaranteed or endorsed by the publisher.
Supplementary Material
The Supplementary Material for this article can be found online at: https://www.frontiersin.org/articles/10.3389/fanim.2021.795200/full#supplementary-material
References
Abecia, L., Toral, P. G., Martín-García, A. I., Martínez, G., Tomkins, N. W., Molina-Alcaide, E., et al. (2012). Effect of bromochloromethane on methane emission, rumen fermentation pattern, milk yield, and fatty acid profile in lactating dairy goats. J. Dairy Sci. 95, 2027–2036. doi: 10.3168/jds.2011-4831
Alemu, A. W., Pekrul, L. K. D., Shreck, A. L., Booker, C. W., McGinn, S. M., Kindermann, M., et al. (2021). 3-Nitrooxypropanol decreased enteric methane production from growing beef cattle in a commercial feedlot: implications for sustainable beef cattle production. Front. Anim. Sci. 2:641590. doi: 10.3389/fanim.2021.641590
Allen, M. S. (2014). Drives and limits to feed intake in ruminants. Anim. Prod. Sci. 54, 1513–1524. doi: 10.1071/AN14478
Almeida, A. K., Hegarty, R. S., and Cowie, A. (2021). Meta-analysis quantifying the potential of dietary additives and rumen modifiers for methane mitigation in ruminant production systems. Anim. Nutr. 7, 1219–1230. doi: 10.1016/j.aninu.2021.09.005
Arndt, C., Hristov, A., Price, W., McClelland, S., Pelaez, A., Cueva, S., et al. (2021). Strategies to mitigate enteric methane emissions by ruminants -a way to approach the 2.0°C target. agriRxiv. 2021:53. doi: 10.31220/agriRxiv.2021.00040
Aschenbach, J. R., Kristensen, N. B., Donkin, S. S., Hammon, H. M., and Penner, G. B. (2010). Gluconeogenesis in dairy cows: the secret of making sweet milk from sour dough. IUBMB Life 62, 869–877. doi: 10.1002/iub.400
Bauchop, T. (1967). Inhibition of rumen methanogenesis by methane analogues. J. Bacteriol. 94, 171–175. doi: 10.1128/jb.94.1.171-175.1967
Beauchemin, K. A., Ungerfeld, E. M., Eckard, R. J., and Wang, M. (2020). Review: Fifty years of research on rumen methanogenesis: lessons learned and future challenges for mitigation. Animal 14, s2–s16. doi: 10.1017/S1751731119003100
Black, R., Cullen, K., Fay, B., Hale, T., Lang, J., Mahmood, S., et al. (2021). Taking Stock: A Global Assessment of Net Zero Targets. Oxford: University of Oxford.
Briunsma, J. (2003). World Agriculture: Towards 2015/2030: an FAO Perspective. London: Food and Agriculture Organization (FAO).
Buckel, W., and Thauer, R. K. (2013). Energy conservation via electron bifurcating ferredoxin reduction and proton/Na(+) translocating ferredoxin oxidation. Biochim. Biophys. Acta 1827, 94–113. doi: 10.1016/j.bbabio.2012.07.002
Capper, J. L. (2011). The environmental impact of beef production in the United States: 1977 compared with 2007. J. Anim. Sci. 89, 4249–4261. doi: 10.2527/jas.2010-3784
Capper, J. L., and Cady, R. A. (2020). The effects of improved performance in the U.S. dairy cattle industry on environmental impacts between 2007 and 2017. J. Anim. Sci. 98, 1–14. doi: 10.1093/jas/skz291
Capper, J. L., Cady, R. A., and Bauman, D. E. (2009). The environmental impact of dairy production: 1944 compared with 2007. J. Anim. Sci. 87, 2160–2167. doi: 10.2527/jas.2009-1781
Caro, D., Davis, S. J., Bastianoni, S., and Caldeira, K. (2014). Global and regional trends in greenhouse gas emissions from livestock. Clim. Change 126, 203–216. doi: 10.1007/s10584-014-1197-x
Cottle, D. J., Nolan, J. V., and Wiedemann, S. G. (2011). Ruminant enteric methane mitigation: a review. Anim. Prod. Sci. 51, 491–514. doi: 10.1071/AN10163
Cristobal-Carballo, O., McCoard, S. A., Cookson, A. L., Ganesh, S., Lowe, K., Laven, R. A., et al. (2021). Effect of methane inhibitors on ruminal microbiota during early life and its relationship with ruminal metabolism and growth in calves. Front. Microbiol. 12:e710914. doi: 10.3389/fmicb.2021.710914
Czerkawski, J. W. (1986). An Introduction to Rumen Studies. Exeter: Pergamon Press. doi: 10.1016/B978-0-08-025486-9.50007-4
Czerkawski, J. W., and Breckenridge, G. (1975). New inhibitors of methane production by rumen micro-organisms. Development and testing of inhibitors in vitro. Br. J. Nutr. 34, 429–446. doi: 10.1017/S0007114575000499
Czerkawski, J. W., Christie, W. W., Breckenridge, G., and Hunter, M. L. (1975). Changes in the rumen metabolism of sheep given increasing amounts of linseed oil in their diet. Brit. J. Nutr. 34, 25–44. doi: 10.1017/S0007114575000074
Davies, A., Nwaonu, H. N., Stanier, G., and Boyle, F. T. (1982). Properties of a novel series of inhibitors of rumen methanogenesis; in vitro and in vivo experiments including growth trials on 2,4-bis (trichloromethyl)-benzo [1, 3]dioxin-6-carboxylic acid. Brit. J. Nutr. 47, 565–576. doi: 10.1079/BJN19820068
Dewanckele, L., Toral, P. G., Vlaeminck, B., and Fievez, V. (2020). Role of rumen biohydrogenation intermediates and rumen microbes in diet-induced milk fat depression: An update. J. Dairy Sci. 103, 7655–7681. doi: 10.3168/jds.2019-17662
DiCostanzo, A., Williams, J. E., and Keisler, D. H. (1999). Effects of short- or long-term infusions of acetate or propionate on luteinizing hormone, insulin, and metabolite concentrations in beef heifers. J. Anim. Sci. 77, 3050–3056. doi: 10.2527/1999.77113050x
Dijkstra, J., Bannink, A., France, J., Kebreab, E., and van Gastelen, S. (2018). Short communication: Antimethanogenic effects of 3-nitrooxypropanol depend on supplementation dose, dietary fiber content, and cattle type. J. Dairy Sci. 101, 9041–9047. doi: 10.3168/jds.2018-14456
Dijkstra, J., Boer, H., Van Bruchem, J., Bruining, M., and Tamminga, S. (1993). Absorption of volatile fatty acids from the rumen of lactating dairy cows as influenced by volatile fatty acid concentration, pH and rumen liquid volume. Br. J. Nutr. 69, 385–396. doi: 10.1079/BJN19930041
Domalski, E. S. (1972). Selected values of heats of combustion and heats of formation of organic compounds containing the elements C, H, N, O, P, and S. J. Phys. Chem. Ref. Data 1, 221–277. doi: 10.1063/1.3253099
European Commission (2021). Joint EU-US Press Release on the Global Methane Pledge. Brussels: European Commission.
Gerber, P., Vellinga, T., Opio, C., and Steinfeld, H. (2011). Productivity gains and greenhouse gas emissions intensity in dairy systems. Livestock Sci. 139, 100–108. doi: 10.1016/j.livsci.2011.03.012
Gerber, P. J., Hristov, A. N., Henderson, B., Makkar, H., Oh, J., Lee, C., et al. (2013a). Technical options for the mitigation of direct methane and nitrous oxide emissions from livestock: a review. Animal 7, 220–234. doi: 10.1017/S1751731113000876
Gerber, P. J., Steinfeld, H., Henderson, B., Mottet, A., Opio, C., Dijkman, J., et al. (2013b). Tackling Climate Change Through Livestock: A Global Assessment of Emissions and Mitigation Opportunities. Rome: Food and Agriculture Organization of the United Nations (FAO).
Global Methane Initiative (2021). Global Methane Emissions and Mitigation Opportunities. Available online at: https://www.globalmethane.org/ (accessed September 20, 2021).
Goopy, J. (2019). Creating a low enteric methane emission ruminant: what is the evidence of success to the present and prospects for developing economies? Anim. Prod. Sci. 59, 1759–1776. doi: 10.1071/AN18457
Greening, C., Geier, R., Wang, C., Woods, L. C., Morales, S. E., McDonald, M. J., et al. (2019). Diverse hydrogen production and consumption pathways influence methane production in ruminants. ISME J. 13, 2617–2632. doi: 10.1038/s41396-019-0464-2
Guo, Y. Q., Liu, J. X., Zhu, W. Y., and McSweeney, C. S. (2007). Shifts of rumen microbial population detected by real-time PCR when methanogenesis is inhibited. J. Anim. Feed Sci. 16, 107–112. doi: 10.22358/jafs/74464/2007
Guyader, J., Doreau, M., Morgavi, D. P., Gerard, C., Loncke, C., and Martin, C. (2016). Long-term effect of linseed plus nitrate fed to dairy cows on enteric methane emission and nitrate and nitrite residuals in milk. Animal 10, 1173–1181. doi: 10.1017/S1751731115002852
Guyader, J., Eugene, M., Meunier, B., Doreau, M., Morgavi, D. P., Silberberg, M., et al. (2015). Additive methane-mitigating effect between linseed oil and nitrate fed to cattle. J. Anim. Sci. 93, 3564–3577. doi: 10.2527/jas.2014-8196
Haisan, J., Sun, Y., Guan, L. L., Beauchemin, K. A., Iwaasa, A., Duval, S., et al. (2014). The effects of feeding 3-nitrooxypropanol on methane emissions and productivity of Holstein cows in mid lactation. J. Dairy Sci. 97, 3110–3119. doi: 10.3168/jds.2013-7834
Haisan, J., Sun, Y., Guan, L. L., Beauchemin, K. A., Iwaasa, A., Duval, S., et al. (2017). The effects of feeding 3-nitrooxypropanol at two doses on milk production, rumen fermentation, plasma metabolites, nutrient digestibility, and methane emissions in lactating Holstein cows. Anim. Prod. Sci. 57, 282–289. doi: 10.1071/AN15219
Hall, M. B., Nennich, T. D., Doane, P. H., and Brink, G. E. (2015). Total volatile fatty acid concentrations are unreliable estimators of treatment effects on ruminal fermentation in vivo. J. Dairy Sci. 98, 3988–3999. doi: 10.3168/jds.2014-8854
Harrison, M., Cullen, B. R., Mayberry, D. E., Cowie, A. L., Bilotto, F., Badgery, W. B., et al. (2021). Carbon myopia: the urgent need for integrated social, economic and environmental action in the livestock sector. Glob. Chang. Biol. 27, 5726-5761. doi: 10.1111/gcb.15816
Hino, T., and Russell, J. B. (1985). Effect of reducing-equivalent disposal and NADH/NAD on deamination of amino acids by intact rumen microorganisms and their cell extracts. Appl. Environ. Microbiol. 50, 1368–1374. doi: 10.1128/aem.50.6.1368-1374.1985
Hristov, A. N., Oh, J., Firkins, J. L., Dijkstra, J., Kebreab, E., Waghorn, G., et al. (2013a). Mitigation of methane and nitrous oxide emissions from animal operations: I. A review of enteric methane mitigation options1. J. Anim. Sci. 91, 5045–5069. doi: 10.2527/jas.2013-6583
Hristov, A. N., Oh, J., Giallongo, F., Frederick, T. W., Harper, M. T., Weeks, H. L., et al. (2015). An inhibitor persistently decreased enteric methane emission from dairy cows with no negative effect on milk production. Proc. Natl. Acad. Sci. U.S.A. 112, 10663–10668. doi: 10.1073/pnas.1504124112
Hristov, A. N., Oh, J., Lee, C., Meinen, R., Montes, F., Ott, T., et al. (2013b). Mitigation of Greenhouse Gas Emissions in Livestock Production - A Review of Technical Options for Non-CO2 Emissions. Rome: FAO.
Hungate, R. E. (1967). Hydrogen as an intermediate in the rumen fermentation. Arch. Mikrobiol. 59, 158–164. doi: 10.1007/BF00406327
Innovation Center for U.S. Dairy (2020). Dairy Environmental Sustainability. Available online at: https://www.usdairy.com/sustainability/environmental-sustainability (accessed September 21, 2021).
IPCC (2018). “Summary for policymakers,” in Global Warming of 1.5°C. An IPCC Special Report on the Impacts of Global Warming of 1.5°C Above Pre-Industrial Levels and Related Global Greenhouse Gas Emission Pathways, in the Context of Strengthening the Global Response to the Threat of Climate Change, Sustainable Development, and Efforts to Eradicate Poverty, eds. V. Masson-Delmotte, P. Zhai, H.-O. Pörtner, D. Roberts, J. Skea, P.R. Shukla, et al. Geneva.
IPCC (2019). “Global warming of 1.5°C,” in An IPCC Special Report on the Impacts of Global Warming of 1.5°C Above Pre-Industrial Levels and Related Global Greenhouse Gas Emission Pathways, in the Context of Strengthening the Global Response to the Threat of Climate Change, Sustainable Development, and Efforts to Eradicate Poverty. eds V. P. Masson-Delmotte, A. Zhai, S. L. Pirani, C. Connors, T. Péan, S. Berger, et al. Geneva.
IPCC (2021). “Summary for policymakers,” in Climate Change 2021: The Physical Science Basis. Contribution of Working Group I to the Sixth Assessment Report of the Intergovernmental Panel on Climate Change, eds. V. Masson-Delmotte, P. Zhai, A. Pirani, S. L. Connors, C. Péan, S. Berger, et al. (Cambridge, CA: Cambridge University Press).
Janssen, P. H. (2010). Influence of hydrogen on rumen methane formation and fermentation balances through microbial growth kinetics and fermentation thermodynamics. Anim. Feed Sci. Technol. 160, 1–22. doi: 10.1016/j.anifeedsci.2010.07.002
Jayanegara, A., Sarwono, K. A., Kondo, M., Matsui, H., Ridla, M., Laconi, E. B., and Nahrowi (2018). Use of 3-nitrooxypropanol as feed additive for mitigating enteric methane emissions from ruminants: a meta-analysis. Ital. J. Anim. Sci. 17, 1–7. doi: 10.1080/1828051X.2017.1404945
Jayasundara, S., and Wagner-Riddle, C. (2013). Greenhouse gas emissions intensity of Ontario milk production in 2011 compared with 1991. Can. J. Anim. Sci. 94, 155–173. doi: 10.4141/cjas2013-127
Johnson, D. E. (1972). Effects of a hemiacetal of chloral and starch on methane production and energy balance of sheep fed a pelleted diet. J. Anim. Sci. 35, 1064–1068. doi: 10.2527/jas1972.3551064x
Johnson, K. A., and Johnson, D. E. (1995). Methane emissions from cattle. J. Anim. Sci. 73, 2483–2492. doi: 10.2527/1995.7382483x
Kemp, P., and Lander, D. J. (1976). “Inhibition of the biohydrogenation of dietary C18 unsaturated fatty acids by rumen bacteria using some inhibitors of methanogenesis,” in Proceedings of the Nutrition Society 35: 31A. Cambridge: Cambridge University Press.
Kim, H., Lee, H. G., Baek, Y. C., Lee, S., and Seo, J. (2020). The effects of dietary supplementation with 3-nitrooxypropanol on enteric methane emissions, rumen fermentation, and production performance in ruminants: a meta-analysis. J. Anim. Sci. Technol. 62, 31–42. doi: 10.5187/jast.2020.62.1.31
Kinley, R. D., Martinez-Fernandez, G., Matthews, M. K., de Nys, R., Magnusson, M., and Tomkins, N. W. (2020). Mitigating the carbon footprint and improving productivity of ruminant livestock agriculture using a red seaweed. J. Clean. Prod. 259:120836. doi: 10.1016/j.jclepro.2020.120836
Knapp, J. R., Laur, G. L., Vadas, P. A., Weiss, W. P., and Tricarico, J. M. (2014). Invited review: Enteric methane in dairy cattle production: quantifying the opportunities and impact of reducing emissions. J. Dairy Sci. 97, 3231–3261. doi: 10.3168/jds.2013-7234
Kristensen, N. B. (2001). Rumen microbial sequestration of [2-13C]acetate in cattle. J. Anim. Sci. 79, 2491–2498. doi: 10.2527/2001.7992491x
Larsen, M., and Kristensen, N. B. (2013). Precursors for liver gluconeogenesis in periparturient dairy cows. Animal 7, 1640–1650. doi: 10.1017/S1751731113001171
Le Gloux, F., Laporte, M., Duvaleix, S., Dupraz, P., and Letort, E. (2021). “Cost of changing dairy cows' diet to reduce enteric methane emissions in livestock farms,” in Working Papers. Institut National De La Recherche Agronomique (Inra), Agriculture Et Alimentation, Espace Et Environnement.
Leahy, S., Clark, H., and Reisinger, A. (2020). Challenges and prospects for agricultural greenhouse gas mitigation pathways consistent with the Paris agreement. Front. Sustain. Food Syst. 4:69. doi: 10.3389/fsufs.2020.00069
Lee, C., Kim, S.-H., Beauchemin, K., Celi, P., and Duval, S. (2020). Short-term eating preference of beef cattle fed high forage or high grain diets supplemented with 3-nitrooxypropanol. Animals 10:10064. doi: 10.3390/ani10010064
Legesse, G., Beauchemin, K. A., Ominski, K. H., McGeough, E. J., Kroebel, R., MacDonald, D., et al. (2016). Greenhouse gas emissions of Canadian beef production in 1981 as compared with 2011. Anim. Prod. Sci. 56, 153–168. doi: 10.1071/AN15386
Leslie, M., Aspin, M., and Clark, H. (2008). Greenhouse gas emissions from New Zealand agriculture: issues, perspectives and industry response. Austr. J. Exp. Agric. 48, 1–5. doi: 10.1071/EA07306
Li, X., Norman, H. C., Kinley, R. D., Laurence, M., Wilmot, M., Bender, H., et al. (2016). Asparagopsis taxiformis decreases enteric methane production from sheep. Anim. Prod. Sci. 58, 681–688. doi: 10.1071/AN15883
Loncke, C., Nozière, P., Vernet, J., Lapierre, H., Bahloul, L., Al-Jammas, M., et al. (2020). Net hepatic release of glucose from precursor supply in ruminants: a meta-analysis. Animal 14, 1422–1437. doi: 10.1017/S1751731119003410
Martin, C., Morgavi, D. P., and Doreau, M. (2010). Methane mitigation in ruminants: from microbe to the farm scale. Animal 4, 351–365. doi: 10.1017/S1751731109990620
Martínez-Fernández, G., Abecia, L., Arco, A., Cantalapiedra-Hijar, G., Martín-García, A. I., Molina-Alcaide, E., et al. (2014). Effects of ethyl-3-nitrooxy propionate and 3-nitrooxypropanol on ruminal fermentation, microbial abundance, and methane emissions in sheep. J. Dairy Sci. 97, 3790–3799. doi: 10.3168/jds.2013-7398
Martinez-Fernandez, G., Denman, S. E., Yang, C., Cheung, J., Mitsumori, M., and McSweeney, C. S. (2016). Methane inhibition alters the microbial community, hydrogen flow, and fermentation response in the rumen of cattle. Front. Microbiol. 7:1122. doi: 10.3389/fmicb.2016.01122
Mathers, J. C., and Miller, E. L. (1982). Some effects of chloral hydrate on rumen fermentation and digestion in sheep. J. Agric. Sci. 99, 215–224. doi: 10.1017/S0021859600055234
Maxin, G., Glasser, F., Hurtaud, C., Peyraud, J. L., and Rulquin, H. (2011). Combined effects of trans-10,cis-12 conjugated linoleic acid, propionate, and acetate on milk fat yield and composition in dairy cows. J. Dairy Sci. 94, 2051–2059. doi: 10.3168/jds.2010-3844
McCrabb, G. J., Berger, K. T. T. M., May, C., and Hunter, R. A. (1997). Inhibiting methane production in Brahman cattle by dietary supplementation with a novel compound and the effects on growth. Aust. J. Agr. Res. 48, 323–329. doi: 10.1071/A96119
Melgar, A., Harper, M. T., Oh, J., Giallongo, F., Young, M. E., Ott, T. L., et al. (2020a). Effects of 3-nitrooxypropanol on rumen fermentation, lactational performance, and resumption of ovarian cyclicity in dairy cows. J. Dairy Sci. 103, 410–432. doi: 10.3168/jds.2019-17085
Melgar, A., Lage, C. F. A., Nedelkov, K., Räisänen, S. E., Stefenoni, H., and Fetter, M. E.. (2021). Enteric methane emission, milk production, and composition of dairy cows fed 3-nitrooxypropanol. J. Dairy Sci. 104, 357–366. doi: 10.3168/jds.2020-18908
Melgar, A., Welter, K. C., Nedelkov, K., Martins, C. M. M. R., Harper, M. T., Oh, J., et al. (2020b). Dose-response effect of 3-nitrooxypropanol on enteric methane emissions in dairy cows. J. Dairy Sci. 103, 6145–6156. doi: 10.3168/jds.2019-17840
Mitsumori, M., Shinkai, T., Takenaka, A., Enishi, O., Higuchi, K., Kobayashi, Y., et al. (2012). Responses in digestion, rumen fermentation and microbial populations to inhibition of methane formation by a halogenated methane analogue. Brit. J. Nutr. 108, 482–491. doi: 10.1017/S0007114511005794
Muizelaar, W., Groot, M., van Duinkerken, G., Peters, R., and Dijkstra, J. (2021). Safety and transfer study: transfer of bromoform present in asparagopsis taxiformis to milk and urine of lactating dairy cows. Foods 10:584. doi: 10.3390/foods10030584
Naranjo, A., Johnson, A., Rossow, H., and Kebreab, E. (2020). Greenhouse gas, water, and land footprint per unit of production of the California dairy industry over 50 years. J. Dairy Sci. 103, 3760–3773. doi: 10.3168/jds.2019-16576
Net Zero Climate (2021). Net Zero Climate. Available online at: https://netzeroclimate.org/what-is-net-zero/ (accessed September 22, 2021).
Nguyen, Q. V., Malau-Aduli, B. S., Cavalieri, J., Malau-Aduli, A. E. O., and Nichols, P. D. (2019). Enhancing Omega-3 long-chain polyunsaturated fatty acid content of dairy-derived foods for human consumption. Nutrients 11:40743. doi: 10.3390/nu11040743
Niu, M., Kebreab, E., Hristov, A. N., Oh, J., Arndt, C., Bannink, A., et al. (2018). Prediction of enteric methane production, yield, and intensity in dairy cattle using an intercontinental database. Glob. Chang. Biol. 24, 3368–3389. doi: 10.1111/gcb.14094
Nolan, J. V., Hegarty, R. S., Hegarty, J., Godwin, I. R., and Woodgate, R. (2010). Effects of dietary nitrate on fermentation, methane production and digesta kinetics in sheep. Anim. Prod. Sci. 50, 801–806. doi: 10.1071/A.N.09211
Opio, C., Gerber, P., Mottet, A., Falcucci, A., Tempio, G., MacLeod, M., et al. (2013). Greenhouse Gas Emissions From Ruminant Supply Chains - A Global Life Cycle Assessment. Rome: Food and Agriculture Organization of the United Nations (FAO).
Ørskov, E. R., Flatt, W. P., Moe, P. W., and Munson, A. W. (1969). The influence of ruminal infusion of volatile fatty acids on milk yield and composition and on energy utilization by lactating cows. Brit. J. Nutr. 23, 443–453. doi: 10.1079/BJN19690054
Reisinger, A., Clark, H., Cowie, A. L., Emmet-Booth, J., Gonzalez Fischer, C., Herrero, M., et al. (2021). How necessary and feasible are reductions of methane emissions from livestock to support stringent temperature goals? Philos. Trans. Royal Soc. A 379:20200452. doi: 10.1098/rsta.2020.0452
Ritzman, E. G., and Benedict, F. G. (1938). Nutritional Physiology of the Adult Ruminant. Washington, DC: Carnegie Institution of Washington.
Rogelj, J., Shindell, D., Jiang, K., Fifita, S., Forster, P., Ginzburg, V., et al. (2018). “Mitigation pathways compatible with 1.5°C in the context of sustainable development,” in Global Warming of 1.5°C. An IPCC Special Report on the Impacts of Global Warming of 1.5°C Above Pre-Industrial Levels and Related Global Greenhouse Gas Emission Pathways, in the Context of Strengthening the Global Response to the Threat of Climate Change, Sustainable Development, and Efforts to Eradicate Poverty, eds V. Masson-Delmotte, P. Zhai, H.-O. Pörtner, D. Roberts, J. Skea, P. R. Shukla, et al. (Geneva: IPCC).
Roque, B. M., Salwen, J. K., Kinley, R., and Kebreab, E. (2019). Inclusion of Asparagopsis armata in lactating dairy cows' diet reduces enteric methane emission by over 50 percent. J. Clean. Prod. 234, 132–138. doi: 10.1016/j.jclepro.2019.06.193
Roque, B. M., Venegas, M., Kinley, R. D., de Nys, R., Duarte, T. L., Yang, X., et al. (2021). Red seaweed (Asparagopsis taxiformis) supplementation reduces enteric methane by over 80 percent in beef steers. PLoS ONE 16:e0247820. doi: 10.1371/journal.pone.0247820
Rufener, W. H., and Wolin, M. J. (1968). Effect of CCl4 on CH4 and volatile acid production in continuous cultures of rumen organisms and in a sheep rumen. Appl. Microbiol. 16, 1955–1956. doi: 10.1128/am.16.12.1955-1956.1968
Russell, J. B., and Martin, S. A. (1984). Effects of various methane inhibitors on the fementation of amino acids by mixed rumen microorganisms in vitro. J. Anim. Sci. 59, 1329–1338. doi: 10.2527/jas1984.5951329x
Sauvant, D., Schmidely, P., Daudin, J. J., and St-Pierre, N. R. (2008). Meta-analyses of experimental data in animal nutrition. Animal 2, 1203–1214. doi: 10.1017/S1751731108002280
Sawyer, M. S., Hoover, W. H., and Sniffen, C. J. (1974). Effect of a ruminal methane inhibitor on growth and energy metabolism in the ovine. J. Anim. Sci. 38, 908–914. doi: 10.2527/jas1974.384908x
Scollan, N. D., Price, E. M., Morgan, S. A., Huws, S. A., and Shingfield, K. J. (2017). Can we improve the nutritional quality of meat? Proc. Nutr. Soc. 76, 603–618. doi: 10.1017/S0029665117001112
Sheperd, A. C., and Combs, D. K. (1998). Long-term effects of acetate and propionate on voluntary feed intake by midlactation cows. J. Dairy Sci. 81, 2240–2250. doi: 10.3168/jds.S0022-0302(98)75803-5
Smith, M. L. (2017). Assessing the Potential of a Novel Feed Additive and an Unsaturated Fat Alone and in Combination to Lower Methane Emission From Cattle and Reduce Their Contribution to Climate Change. PhD, University of Delaware.
Stefenoni, H. A., Räisänen, S. E., Cueva, S. F., Wasson, D. E., Lage, C. F. A., and Melgar, A.. (2021). Effects of the macroalga Asparagopsis taxiformis and oregano leaves on methane emission, rumen fermentation, and lactational performance of dairy cows. J. Dairy Sci. 104, 4157–4173. doi: 10.3168/jds.2020-19686
Terry, S., Romero, C., Chaves, A., and McAllister, T. (2020). “Nutritional factors affecting greenhouse gas production from ruminants: implications for enteric and manure emissions,” in Improving Rumen Function, eds C. S. Mcsweeney and R. I. Mackie. (Cambridge: Burleigh Dodds Science Publishing). doi: 10.19103/AS.2020.0067.16
Tomkins, N. W., and Hunter, R. A. (2004). “Methane reduction in beef cattle using a novel antimethanogen,” in Proceedings of the Australian Society of Animal Production 25th Biennial Conference: The New Realities, eds R. Stockdale, J. Heard and M. Jenkin (Collingwood, VIC: CSIRO Publishing).
Trei, J. E., Parish, R. C., and Singh, Y. K. (1971). Effect of methane inhibitors on rumen metabolism and feedlot performance of sheep. J. Dairy Sci. 54, 536–540. doi: 10.3168/jds.S0022-0302(71)85882-4
UN Climate Change News (2021). Halving Emissions by 2030 is New Normal - Race To Zero Anniversary. United Nations.
Ungerfeld, E. M. (2013). A theoretical comparison between two ruminal electron sinks. Front. Microbiol. 4:319. doi: 10.3389/fmicb.2013.00319
Ungerfeld, E. M. (2015). Shifts in metabolic hydrogen sinks in the methanogenesis-inhibited ruminal fermentation: a meta-analysis. Front. Microbiol. 6:37. doi: 10.3389/fmicb.2015.00037
Ungerfeld, E. M. (2018). Inhibition of rumen methanogenesis and ruminant productivity: a meta-analysis. Front. Vet. Sci. 5:113. doi: 10.3389/fvets.2018.00113
Ungerfeld, E. M. (2020). Metabolic hydrogen flows in rumen fermentation: principles and possibilities of interventions. Front. Microbiol. 11:589. doi: 10.3389/fmicb.2020.00589
Ungerfeld, E. M. (2021). Pronounced Methanogenesis Inhibition Database. Available online at: https://osf.io/xsfe9/?view_only=5bc486ae58314284a5e09b6243e8bcc3 (accessed December 5, 2021).
Ungerfeld, E. M., Aedo, M. F., Martínez, E. D., and Saldivia, M. (2019). Inhibiting methanogenesis in rumen batch cultures did not increase the recovery of metabolic hydrogen in microbial amino acids. Microorganisms 7:115. doi: 10.3390/microorganisms7050115
Ungerfeld, E. M., Aedo, M. F., Muñoz, C., Urrutia, N. L., Martínez, E. D., and Saldivia, M. (2020). Inhibiting methanogenesis stimulated de novo synthesis of microbial amino acids in mixed rumen batch cultures growing on starch but not on cellulose. Microorganisms 8:799. doi: 10.3390/microorganisms8060799
Ungerfeld, E. M., Rust, S. R., and Burnett, R. (2007). Increases in microbial nitrogen production and efficiency in vitro with three inhibitors of ruminal methanogenesis. Can. J. Microbiol. 53, 496–503. doi: 10.1139/W07-008
United Nations Climate Action (2021). Net Zero #its Possible. Available online at: https://www.un.org/en/climatechange/net-zero-coalition (accessed September 29, 2021).
United Nations Environment Programme and Climate and Clean Air Coalition (2021). Global Methane Assessment: Benefits and Costs of Mitigating Methane Emissions. Nairobi: United Nations.
Urrutia, N. L., and Harvatine, K. J. (2017). Acetate dose-dependently stimulates milk fat synthesis in lactating dairy cows. J. Nutr. 147, 763–769. doi: 10.3945/jn.116.245001
Van Lingen, H. J., Plugge, C. M., Fadel, J. G., Kebreab, E., Bannink, A., and Dijkstra, J. (2016). Thermodynamic driving force of hydrogen on rumen microbial metabolism: a theoretical investigation. PLoS ONE 11:e0161362. doi: 10.1371/journal.pone.0161362
van Zijderveld, S. M., Fonken, B., Dijkstra, J., Gerrits, W. J. J., Perdok, H. B., Fokkink, W., et al. (2011). Effects of a combination of feed additives on methane production, diet digestibility, and animal performance in lactating dairy cows. J. Dairy Sci. 94, 1445–1454. doi: 10.3168/jds.2010-3635
Van Zijderveld, S. M., Gerrits, W. J. J., Apajalahti, J. A., Newbold, J. R., Dijkstra, J., Leng, R. A., et al. (2010). Nitrate and sulfate: effective alternative hydrogen sinks for mitigation of ruminal methane production in sheep. J. Dairy Sci. 93, 5856–5866. doi: 10.3168/jds.2010-3281
Veneman, J. B., Saetnan, E. R., Clare, A. J., and Newbold, C. J. (2016). MitiGate; an online meta-analysis database for quantification of mitigation strategies for enteric methane emissions. Sci. Total Environ. 572, 1166–1174. doi: 10.1016/j.scitotenv.2016.08.029
Vyas, D., Alemu, A. W., McGinn, S. M., Duval, S. M., Kindermann, M., and Beauchemin, K. A. (2018). The combined effects of supplementing monensin and 3-nitrooxypropanol on methane emissions, growth rate, and feed conversion efficiency in beef cattle fed high-forage and high-grain diets. J. Anim. Sci. 96, 2923–2938. doi: 10.1093/jas/sky174
Vyas, D., McGinn, S. M., Duval, S. M., Kindermann, M., and Beauchemin, K. A. (2016). Effects of sustained reduction of enteric methane emissions with dietary supplementation of 3-nitrooxypropanol on growth performance of growing and finishing beef cattle. J. Anim. Sci. 94, 2024–2034. doi: 10.2527/jas.2015-0268
Wiedemann, S. G., Henry, B. K., McGahan, E. J., Grant, T., Murphy, C. M., and Niethe, G. (2015). Resource use and greenhouse gas intensity of Australian beef production: 1981-2010. Agric. Sys. 133, 109–118. doi: 10.1016/j.agsy.2014.11.002
Wolin, M. J., Miller, T. L., and Stewart, C. S. (1997). “Microbe-microbe interactions,” in The Rumen Microbial Ecosystem, eds P. N. Hobson and C. S. Stewart (London: Blackie Academic & Professional), 467–491. doi: 10.1007/978-94-009-1453-7_11
Wollenberg, E., Richards, M., Smith, P., Havlík, P., Obersteiner, M., Tubiello, F. N., et al. (2016). Reducing emissions from agriculture to meet the 2°C target. Global Change Biol. 22, 3859–3864. doi: 10.1111/gcb.13340
Zhang, X. M., Gruninger, R. J., Alemu, A. W., Wang, M., Tan, Z. L., Kindermann, M., et al. (2020). 3-Nitrooxypropanol supplementation had little effect on fiber degradation and microbial colonization of forage particles when evaluated using the in situ ruminal incubation technique. J. Dairy Sci. 103, 8986–8997. doi: 10.3168/jds.2019-18077
Zhang, X. M., Smith, M. L., Gruninger, R. J., Kung, L., Vyas, D., McGinn, S. M., Kindermann, M., et al. (2021). Combined effects of 3-nitrooxypropanol and canola oil supplementation on methane emissions, rumen fermentation and biohydrogenation, and total tract digestibility in beef cattle. J. Anim. Sci. 99:81. doi: 10.1093/jas/skab081
Keywords: global warming, ruminant production, greenhouse gases, enteric methane, methanogenesis inhibition, metabolism, productivity, cost effectiveness
Citation: Ungerfeld EM, Beauchemin KA and Muñoz C (2022) Current Perspectives on Achieving Pronounced Enteric Methane Mitigation From Ruminant Production. Front. Anim. Sci. 2:795200. doi: 10.3389/fanim.2021.795200
Received: 14 October 2021; Accepted: 13 December 2021;
Published: 03 January 2022.
Edited by:
Todd Riley Callaway, University of Georgia, United StatesReviewed by:
Alejandro Belanche, University of Zaragoza, SpainXuezhao Sun, Jilin Agricultural Science and Technology University, China
Copyright © 2022 Ungerfeld, Beauchemin and Muñoz. This is an open-access article distributed under the terms of the Creative Commons Attribution License (CC BY). The use, distribution or reproduction in other forums is permitted, provided the original author(s) and the copyright owner(s) are credited and that the original publication in this journal is cited, in accordance with accepted academic practice. No use, distribution or reproduction is permitted which does not comply with these terms.
*Correspondence: Emilio M. Ungerfeld, ZW1pbGlvLnVuZ2VyZmVsZEBpbmlhLmNs