Effects of Rumen-Protected Creatine Pyruvate on Meat Quality, Hepatic Gluconeogenesis, and Muscle Energy Metabolism of Long-Distance Transported Beef Cattle
- 1Jiangxi Province Key Laboratory of Animal Nutrition, College of Animal Science and Technology, Jiangxi Agricultural University, Nanchang, China
- 2Jiangxi Province Key Laboratory of Animal Nutrition/Animal Nutrition and Feed Safety Innovation Team, College of Animal Science and Technology, Jiangxi Agricultural University, Nanchang, China
- 3College of Animal Science and Technology, Jiangxi Agricultural University, Nanchang, China
Pre-slaughter long-distance transport resulted in a rapid depletion of muscle glycogen and led to a higher rate of dark, firm and dry (DFD) meat. Therefore, enhancing muscle glycogen reserves is critical for beef cattle prior to transportation. Creatine pyruvate (CrPyr) can provide simultaneous pyruvate and creatine and both are proven to promote the glycogen reserves. This study aimed to investigate the effects of transport treatment and dietary supplementation of rumen-protected (RP)-CrPyr on the meat quality, muscle energy metabolism, and hepatic gluconeogenesis of beef cattle. Twenty 18 month-old male Simmental crossbred cattle (659 ± 16 kg) were allotted 4 treatments based on a 2 × 2 factorial arrangement with two RP-CrPyr levels (140 g/d or 0 g/d) and two transport treatments (12 h or 5 min): ST_CrPyr0, ST_CrPyr140, LT_CrPyr0 and LT_CrPyr140. Three cattle per group were slaughtered after 30 days of feeding. The interaction of transport and RP-CrPyr had a significant effect on the muscle pH45 min, redness, glycogen content, GP, and AMP level (P < 0.05). Compared with short-distance transport, long-distance transport increased the muscle pH45 min value, redness, yellowness, drip loss, creatine level (P < 0.05), decreased muscle glycogen content, glycolytic potential (GP), and liver glucose amount (P < 0.05). Supplementation of RP-CrPyr decreased the activities of creatine kinase and lactate dehydrogenase in serum, muscle pH24 h value, redness, yellowness, lactate content, AMP level, and AMP/ATP (P < 0.05), increased the muscle glycogen content, GP, hexokinase activity, ATP and ADP levels, and ATP/ADP, liver pyruvate and glucose contents, activity of pyruvate carboxylase in the liver of cattle than those in the nonsupplemented treatments (P < 0.05). These results indicated that dietary RP-CrPyr supplementation might be favorable to improve meat quality and regulatory capacity of energy metabolism of beef cattle suffering long-distance transport followed with recovery treatment by increasing muscle glycogen storage, energy supply, and hepatic gluconeogenesis.
Introduction
The separation of breeding and slaughtering is the dominant model of the beef cattle industry in China. The beef cattle are inevitably subjected to stress during the centralized transportation process. Transport stress has been reported to increase the physical energy consumption of beef cattle, resulting in a decrease in muscle glycogen content at slaughter. The insufficient muscle glycogen cannot produce enough lactic acid and H+ and leads to a high ultimate pH (pHu) in postmortem muscle, which results in dark, firm, and dry (DFD) meat. Higher rates of DFD meat cause substantial economic losses to the beef cattle industry (Ferguson and Warner, 2008; Ponnampalam et al., 2017). In Australia, the incidence of “Dark Cutting” (DC) beef is close to 10%, resulting in a potential loss about AU $36 million to the cattle industry, which equates to $0.45/kg hot carcass weight. The DC meat in the US was estimated to cost about $165 million to the beef industry in the year 2000, an amount equal to an estimated $5.43 per fed beef animal harvested (Ponnampalam et al., 2017). In China, the average incidence of DFD in beef cattle was as high as 10.07%, of which a major factor that affects the rate of DFD meat is pre-slaughter transport time and quality (Zhao, 2013). Ponnampalam et al. (2017) summarized that short-distance transport (< 400 km) has little effect on the postmortem muscle pHu value of beef cattle, while long-distance transport can increase the pHu value by 0.1 to 0.2 pH units. Therefore, alleviating pre-slaughter long-distance transport stress is an important guarantee to produce high-quality beef.
Pre-slaughter nutritional management is an efficient technological means to regulate energy metabolism, relieve transportation stress, reduce depletion of muscle glycogen, and improve beef quality in transported beef cattle (Ponnampalam et al., 2017). Creatine pyruvate (CrPyr, C7H13N3O5, 219.20) is a new multifunctional nutrient that contains pyruvate and creatine at a ratio of 40:60 (Jäger et al., 2011). CrPyr can provide pyruvate and creatine, which are both the products of normal cellular metabolism and plays critical roles in the process of energy metabolism. Pyruvate is a pivotal component in intermediary metabolism (glucose metabolism, lipid metabolism, and amino acid metabolism), can regulate energy metabolism through gluconeogenesis/glycolysis pathway and tricarboxylic acid cycle (Chen et al., 2011). Guo et al. (2009) reported that supplement of calcium pyruvate for 3 weeks could improve liver and muscle glycogen content at the start of exhaustive exercise. Shetty et al. (2012) showed that exogenous pyruvate could enhance internal glycogen stores and therefore increase the energy buffering capacity of hippocampal slices under low glucose condition. Additional studies have reported that pyruvate could enhance hypoxia and even anaerobic tolerance of cells by restoring pyruvate dehydrogenase complex activity and increasing glycolytic enzymes activity (Sharma et al., 2009; Hu et al., 2016). Creatine, as an important material for the synthesis of energy storage substance phosphocreatine, is the backup guarantee of skeletal muscle ATP production (Allen, 2012). Creatine supplementation could promote the absorption of creatine by human and animal skeletal muscle, increase the content of creatine and phosphocreatine in muscle, enhance the energy buffering effects of phosphogen system, and improve the energy metabolism of skeletal muscle (Schoch et al., 2006). Moreover, creatine supplementation could facilitate glycogen accumulation in muscle of mammalian and reduce the glycogen depletion under normal physiological conditions or during exercise (Ceddia and Sweeney, 2004; Ju et al., 2005).
Interestingly, the effect of CrPyr feeding on muscle energy metabolism of broilers was significantly higher than that of its separate active ingredients, pyruvate and creatine (Chen et al., 2012). A previous study discovered that CrPyr supplementation was helpful for athletes to further promote aerobic capacity and improve endurance performance (Jäger et al., 2008). Chen (Chen et al., 2011) discovered that CrPyr supplementation has a positive influence on enhancing the activity of creatine kinase and phosphocreatine concentration in broilers muscle and increased muscle glycogen reserve by reducing glycogenolysis via decreasing the activity of phosphorylase-b. Recently, another study reported that in ovo feeding of CrPyr could promote broilers liver and muscle energy reserves on the day of hatch and enhance glycolysis in chest through increasing the activity of glycolytic enzyme (Zhao, 2017). However, the information about the impacts of CrPyr on energy metabolism and whether CrPyr can relieve the glycogen consumption in the transported beef cattle is largely unknown. We hypothesized that CrPyr might affect meat quality by influencing the muscle energy metabolism and hepatic gluconeogenesis that beef cattle suffer during transportation. Therefore, the present study was designed to test this hypothesis.
Materials and Methods
Animal Care
This experiment was approved by the Committee for the Care and Use of Experimental Animals at Jiangxi Agricultural University (JXAULL-2021-10).
Animal Treatments and Experimental Diets
Experimental design of experimental cattle has been described in a joint experiment (Mao et al., 2022). Briefly, a total of twenty 18-mouths-old male Chinese Simmental crossbred cattle, raised under identical feeding and environmental conditions, weighing 659 ± 16 kg, with similar genetic backgrounds were used in this study. They were allotted four treatments based on a 2 × 2 factorial arrangement with two supplemental levels of RP-CrPyr (140 g/d/head or 0 g/d/head) in basal diets and two treatments of transport (12 h or 5 min that were ST_CrPyr0 (0 g/d/head RP-CrPyr + 5 min transport), ST_CrPyr140 (140 g/d/head RP-CrPyr + 5 min transport), LT_CrPyr0 (0 g/d/head RP-CrPyr + 12 h transport), LT_CrPyr140 (140 g/d/head RP-CrPyr + 12 h transport). For long-distance transport, we decided 12 h because one previous study indicated that 12 h of transportation pre-slaughter resulted in decreases in slaughter performance and beef quality of Simmental crossbred cattle (Lu et al., 2015) and for short-distance transport we decided 5min because transport to the abattoir near the farm (1 km) took approximately 5 min. Three cattle from each treatment were randomly slaughtered and samples were collected after feeding for 30 days. The cattle in the short-distance transport group were fed at 04:00 pm on September 16, 2020, loaded at 04:00 am on September 17, 2020, and after 5 min of transportation the cattle reached the abattoir near the farm (1 km). After unloading, cattle were given 2 hours rest and then subjected to slaughter by electric shock (at 06:00 am on September 17, 2020). The cattle in the long-distance transport group were fed at 06:00 am on September 16, 2020. The transport journey was from Xuchang (01:00 pm on September 16, 2020) to Nanyang (01:00 am on September 17, 2020), Henan Province, which took 12 h (mean velocity of 60-70 km/h). During the 12 h of transport, the temperature and humidity were 22–32°C and 30–72%, respectively. The cattle were allowed 2 h to rest and then subjected to slaughter by electric shock (03:00 am on September 17, 2020).
CrPyr (purity of 99.9%) was bought from Shanghai Jinli Technology Co., Ltd. (Shanghai, China). The coating processing was completed by Hangzhou King Techina Feed Co., Ltd (Hangzhou, China), the content of CrPyr in the final RP-CrPyr was 40%, 12 h of rumen bypass ratio was 82.8%, and 12 h of intestinal fluid dissolution was 78.6%. RP-CrPyr was added in concentrate and fed twice a day (06:00 am and 04:00pm). Concentrate was given after the rice straw was fed. All animals were placed individual pens in a closed cowshed with available access to clean water during the entire experimental period. Table 1 shows the ingredients and nutrient levels of the experimental diet.
Sample Collection
Blood sampling was performed from cattle by jugular vein puncture before slaughter and then placed in evacuated anticoagulated tubes and stored at room temperature. Serum was obtained by centrifugation (3000 g, 10 min, 4°C) and then stored at –20°C to measure indices. Shortly afterwards, cattle were slaughtered after being stunned by electric shock. Immediately, the liver sample and the M. longissimus thoracis (LT) muscle (taken from the last rib of the left-half carcass) was stored at –80°C to analyze the indices including hepatic gluconeogenesis and muscle energy metabolism. About 5 cm thickness steaks of LT, without extra-muscular fat and connective tissues from the last rib of the left-half carcass, were collected within 30 min after slaughter. After muscle pH 45 min determination, these chops were vacuum-packed at 4°C to measure muscle pH24 h, color, drip loss, and texture analyzer determination.
Serum Parameters Determination
The activities of creatine kinase (CK) and lactate dehydrogenase (LDH) and the pyruvate concentration in serum were determined by commercial CK, LDH and pyruvate kits (Nanjing Jiancheng Bioengineering Institute, Nangjing, China) according to kit instructions. The serum glucose concentration was determined by a commercial glucose kit (Shanghai RongSheng Biotech Co. Ltd., Shanghai, China). The serum creatine concentration was measured by 1’-Hydroxy-2’-acetonaphthone colorimetry. Firstly, a standard curve was performed using different concentrations of creatine. Briefly, 1 mL of the reaction system contained 250 μL of 5% ZnSO4, 250 μL of 5% Ba(OH)2 and 500 μL of different dilutions of creatine standard was mixed and centrifuged (3000 r/min for 10 min) to get supernatant. Then, 0.5 mL of supernatant was pooled with 2.5 mL of chromogenic reagent (ten-fold volume of 7 g/L a-naphthol solution mixed with one-fold volume of 0.1% biacetyl reagent, 1 L of 7 g/L a-naphthol solution contained 128 g of anhydrous Na2CO3, 60 g of NaOH, 7 g of a-naphthol). After 5 min of rest, the mixture was incubated at 30°C for 30 min. Following incubation, 2.5 mL of distilled water was added and absorbance was measured at 520 nm. Finally, the solubility curve was drawn. For serum creatine content determination, the appropriate amount of serum sample was taken and distilled water was added to it, sonicated for 20 min, shocked for 15 min, and centrifuged (4000 r/min for 10 min) to get supernatant. Subsequently, 250 µL of the supernatant was collected and 250 µL of distilled water, 250 μL of 5% ZnSO4, and 250 μL of 5% Ba(OH)2 were added and followed by the protocol described above. Lastly, the absorbance values were recorded and quantified using standard curves established.
Meat Quality Measurements
Muscle pH45 min and pH24 h were determined using an insertiontype portable pH meter (HI99163N, Hanna, Padova, Italy). Triplicates were done for each chop at different areas and averaged to calculate the results.
Color of the meat was determined using a spectrocolorimeter (WSC-S, Shanghai, China) at 24 h postmortem. The meat color was presented in (CIE) L* (lightness), a* (redness), and b* (yellowness). The illuminant and observer angle were D65 and 10°, respectively. The L*, a*, and b* values were collected from three different areas of each steak with freshly cut surface (exposure to air for 20 min).
To measure drip loss, approximately 30 g of rectangle muscle were cut along the fibre direction at 24 h postmortem. The samples were suspended in a polyethylene plastic bag to avoid any contact with the bag. Samples were re-weighed to calculate the drip loss after 24 h at 4°C.
Texture profile analysis (TPA) values of cooked samples were determined using a CT3 texture analyzer (Brookfield, Middleboro, MA, USA). Briefly, at 48 h postmortem, LT muscle samples were cut in approximately 2.5 cm thick pieces. The pieces were individually vacuumed packaged then placed in a hot-water bath (80°C) to reach a center temperature of 75°C. The packaged samples were cooled down to room temperature by running water, then removed from bags, and wiped dry with absorbent paper. The blot-dried samples were cut in 1.0 cm thick pieces to ensure a flat surface. Finally, the meat pieces were placed beneath the probe and analyzed for five TPA parameters: hardness, cohesiveness, springiness, gumminess, and chewiness. Each sample was measured thrice, and the relevant parameters were designed as follows: the probe model was TA3/100, the pre-measurement speed was 2 mm/s, the test speed was 0.5 mm/s, the post-measurement speed was 0.5 mm/s, the sample height was 10 mm, and the trigger force was 5 g.
Muscle Glycolytic Potential and Glycolytic Enzyme Activities
The measurement of glycogen was conducted as described by a previous study (Zhang et al., 2009). The glycogen was hydrolysed to glucose by reaction with amyloglucosidase and the glucose-6-phosphate was catalysed to glucose by reaction with glucose-6-phosphatase. Finally, the glucose determination operations were carried out according to a commercial glucose kit (Shanghai RongSheng Biotech Co. Ltd.). The lactate content was performed using a commercial diagnostic kit (Nanjing Jiancheng Bioengineering Institute) in accordance with kit instructions. The glycolytic potential (GP) was calculated according to the formula from Monin and Sellier (1985): GP = 2 × glycogen + lactate.
For muscle glycolytic enzyme activities determination, 0.5 g frozen LT muscle sample was homogenized in 4.5 mL ice-cold physiological saline for 1 min in an ice bath and centrifuged (2,700 g, 10 min, 4°C) to get supernatants. The supernatants of LT muscle were subjected to the determination of the activities of phosphofructokinase (PFK), hexokinase (HK), pyruvate kinase (PK), and LDH. The concentration of total protein in LT muscle was determined by TP kit (Nanjing Jiancheng Bioengineering Institute) according to kit instructions. The activities of PFK, HK, PK, and LDH were determined by commercial PFK, HK, PK, and LDH kits (Nanjing Jiancheng Bioengineering Institute). The enzyme activities in LT muscle were normalized by total protein concentration in LT muscle according to kit instructions.
Phosphocreatine, Creatine, and Adenosine Phosphates Determination
The phosphocreatine (PCr), creatine, and adenosine phosphates (ATP, ADP, and AMP) contents of LT muscle were assayed using a high-performance liquid chromatography (HPLC) according to previous study (Li et al., 2017). The reference standard samples used in the analysis were creatine anhydrous (98%), creatine phosphate disodium salt (97%), adenosine 5’-stiphosphate disodium salt, adenosine 5’-diphoshate, adenosine 5’-monophosphate. All of the standard samples were purchased from Shanghai Yuan Mu Biotechnology Co., Ltd (Shanghai, China). The extracted muscle sample solution was prepared as described previously (Monin and Sellier, 1985) and separated on a Shimadzu LC-2030 Plus HPLC (Shimadzu, Kyoto, Japan) equipped with a YMC-Pack ODS-AQ column (250 mm × 4.6 mm I.D., 5 μm, YMC Co., Ltd., Kyoto, Japan) at 25°C for PCr and creatine determination and at 30°C for ATP, ADP, and AMP determination. The mobile phase of creatine and PCr determination was HPLC methyl cyanides and phosphate buffer (29.4 mM potassium dihydrogen orthophosphate,1.15 mM tetra-butylammonium hydrogen sulphate, 2%/98%), and adenosine phosphates determination was HPLC grade methanol and phosphate buffer (2.5 mM tetra-butylammonium hydrogen sulphate, 0.06 M dipotassium hydrogen orthophosphate, 0.04 M potassium dihydrogen orthophosphate, 13.5%/86.5%). The UV detection for creatine and PCr was at 210 nm and adenosine phosphates was at 254 nm. The flow-rate was set to 1.0 mL/min and an auto-sequence injection was set for sample measurement. Peaks were identified and quantified using standard curves.
Liver Pyruvate, Glucose, and Glycogen Concentration, and Enzyme Activity Determination
The contents of pyruvate and glycogen were determined by commercial pyruvate and glycogen kits (Nanjing Jiancheng Bioengineering Institute) according to kit instructions. The glucose concentration was determined by a commercial glucose kit (Shanghai RongSheng Biotech Co. Ltd.). For liver enzyme activities determination, 0.5 g frozen liver sample was homogenized in 4.5 mL ice-cold physiological saline for 1 min in ice bath and centrifuged (2,700 g, 10 min, 4°C) to get supernatants. The supernatants of liver were subjected to the determination of the activities of phosphoenolpyruvate carboxykinase (PEPCK), glucose-6-phosphatase (G-6-pase), and pyruvate carboxylase (PC). The concentration of total protein in liver was determined by TP kit (Nanjing Jiancheng Bioengineering Institute) according to kit instructions. The activities of PEPCK, G-6-pase, and PC were determined by commercial PEPCK kit (Nanjing Jiancheng Bioengineering Institute), commercial G-6-pase and PC kits (Shanghai enzyme linked Biotechnology Co., Ltd). Except for PEPCK, other enzyme activities in the liver were normalized by total protein concentration in the liver according to kit instructions.
Statistical Analysis
Data processing was performed using SPSS (version 17.0, IBM, Armonk, NY, USA). All treatment groups were analyzed by analysis of univariate using the general linear model (GLM) as a 2 × 2 factorial arrangement with transport, RP-CyPry and their interactions as the main effects. The results are shown as the mean and standard error mean (SEM). Differences among means were determined using Tukey’s multiple range test was done when the interaction was significant. The level of statistical significance was set at P < 0.05.
Results
Serum Parameters
As presented in Table 2, RP-CrPyr and transport had no interaction on serum parameters. The beef cattle in the RP-CrPyr-supplemented group had a lower activities of serum CK (0.170 vs. 0.947 U/mL, P < 0.01) and LDH (1.05 vs. 1.25 U/mL, P < 0.01) than unsupplemented cattle. However, transport treatment has no significant effects on serum CK and LDH activities. The contents of serum pyruvate, glucose, and creatine appeared to be unaffected by transport or RP-CrPyr (P > 0.05).
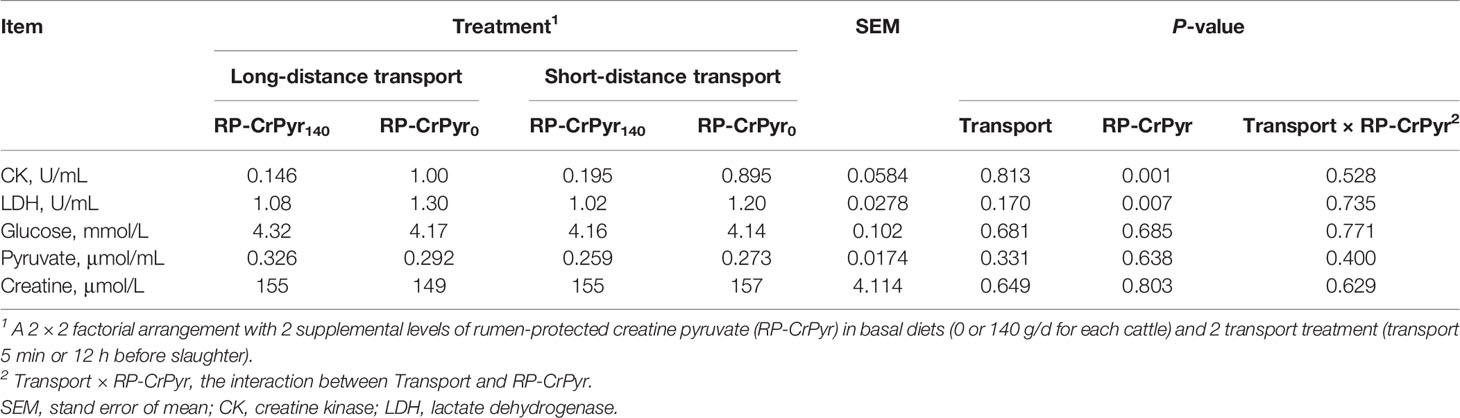
Table 2 Effects of transport and rumen-protected creatine pyruvate on the serum indicators of beef cattle.
Meat Quality
The results of meat quality can be seen in Table 3. Except for pH45 min value and redness, RP-CrPyr and transport had no significant interaction influence on other meat quality characteristics. Compared with cattle in the nontransport group, the pH45 min value (6.36 vs. 6.20, P < 0.01), drip loss (1.81% vs. 1.08%, P < 0.05), redness (34.6 vs. 27.9, P < 0.01), and yellowness (22.1 vs. 15.8, P < 0.05) of LT muscle were significantly increased in group transport. Dietary RP-CrPyr supplementation decreased the LT muscle pH24 h value (5.47 vs. 5.59, P < 0.05), redness (28.2 vs. 34.3, P < 0.01), and yellowness (16.1 vs. 21.7, P < 0.05) of beef cattle than those in the unsupplemented groups. RP-CrPyr or transport had no effect on the meat color and texture properties of LT muscle (P > 0.05).
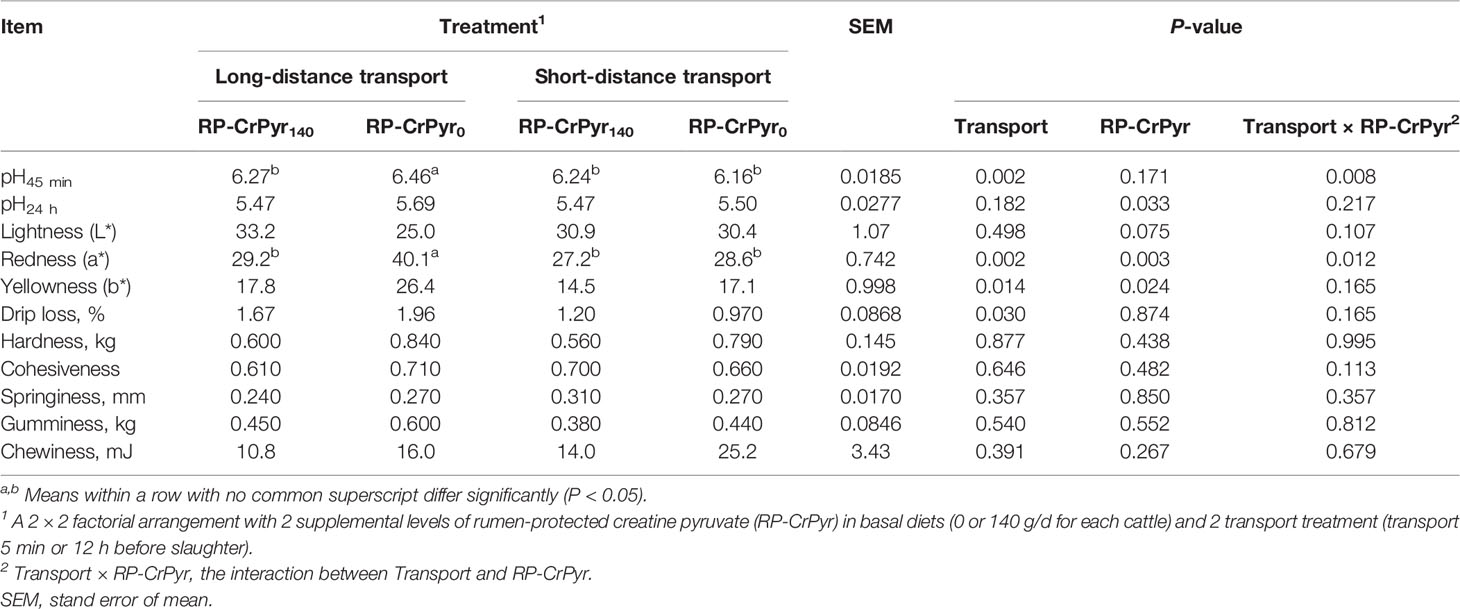
Table 3 Effects of transport and rumen-protected creatine pyruvate on the M. longissimus thoracis meat quality of beef cattle.
Muscle Glycolytic Potential and Glycolytic Enzyme Activity
As shown in Table 4, there is a significant transport × RP-CrPyr interaction influence on muscle glycogen and GP. However, there is no notable RP-CrPyr and transport interaction on glycogen, glucose, lactate, and glycolytic enzymes. Compared with the beef cattle in nontransport groups, the muscle glycogen content (37.3 vs. 48.3 μmol/g, P < 0.05) and GP (118 vs. 139 μmol/g, P < 0.01) of beef cattle decreased significantly in the transport groups. In addition, no notable differences were identified in the contents of muscle glucose and lactate between the nontransport and transport treatment (P > 0.05). Dietary supplementation of RP-CrPyr increased the muscle glycogen content (53.5 vs. 32.1 μmol/g) and GP (146 vs. 110 μmol/g) (P < 0.01), and decreased the muscle lactate content (39.2 vs. 46.0 μmol/g, P < 0.01) of beef cattle compared to those in the RP-CrPyr unsupplemented groups. Glycolytic enzymes, except for muscle HK activity (7.64 vs. 2.49 U/mgprot, P < 0.01), were significantly increased by RP-CrPyr supplementation compared to those in the unsupplemented groups, and the activities of PFK, PK, and LDH were not affected by transport or RP-CrPyr supplementation.
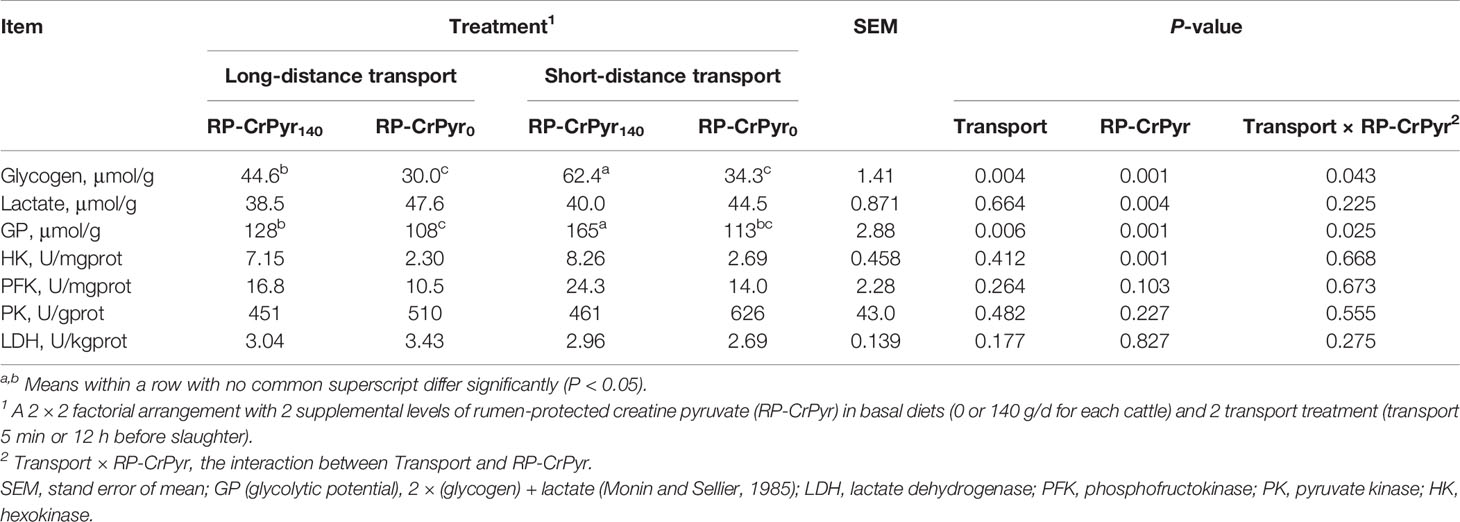
Table 4 Effects of transport and rumen-protected creatine pyruvate on the contents of glycogen and lactate, glycolytic potential, and glycolytic enzymes activity in M. longissimus thoracis of beef cattle.
Phosphocreatine, Creatine, and Adenosine Phosphates in Muscle
The results of muscle PCr, creatine, and adenosine phosphates are showed in Table 5. Except for muscle AMP content, RP-CrPyr and transport had no significant interaction on the other characteristics. Compared with the beef cattle in nontransport groups, the beef cattle in transport groups had higher muscle creatine content (22.4 vs. 21.2 µmol/g, P < 0.05). Dietary RP-CrPyr supplementation significantly decreased the level of muscle AMP (67.4 vs. 76.8 µg/g, P < 0.01), muscle AMP/ATP (0.13 vs. 0.68, P < 0.05), and significantly increased the levels muscle ATP (579 vs. 217 µg/g), muscle ADP (330 vs. 238 µg/g), and muscle ATP/AMP (1.59 vs 0.790) (P < 0.05).
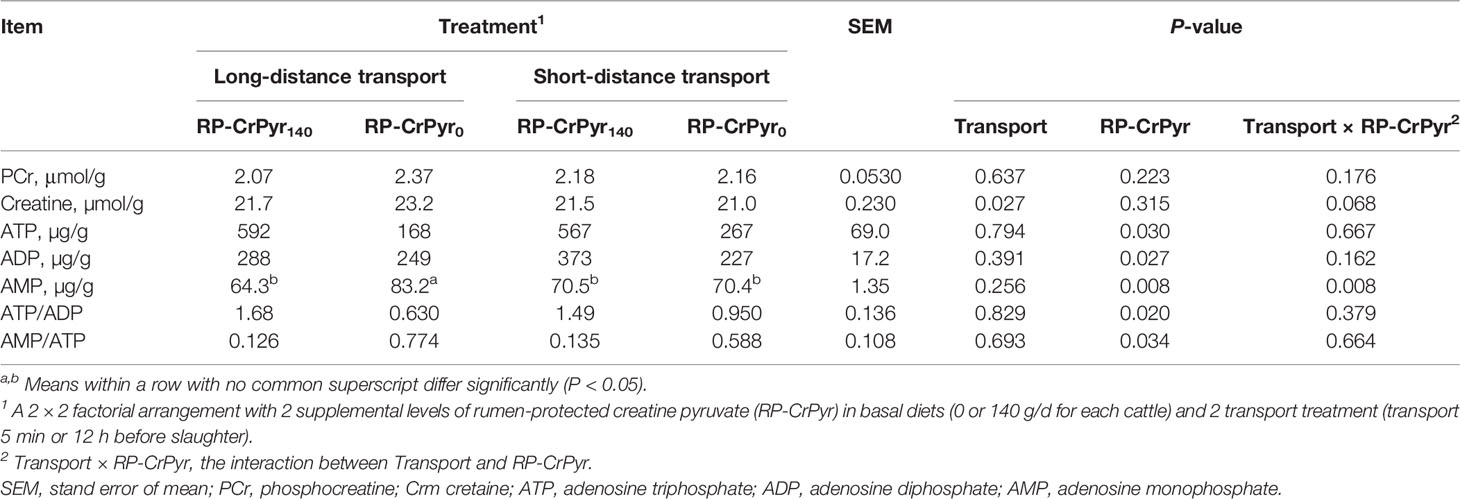
Table 5 Effects of transport and rumen-protected creatine pyruvate on the contents of phosphocreatine, creatine, and adenosine phosphates in M. longissimus thoracis of beef cattle.
Hepatic Gluconeogenesis
As shown in Table 6, RP-CrPyr and transport had no significant interaction influence on any of these parameters. Compared with the beef cattle in nontransport groups, the beef cattle with transport treatment had lower liver glucose content (0.171 vs. 0.209 mmol/g, P < 0.05). Dietary supplementation of RP-CrPyr significantly increased the concentrations of liver pyruvate (41.8 vs. 29.9 μmol/gprot, P < 0.01) and glucose (0.213 vs. 0.167 mmol/g, P < 0.05), and the level of PC (19.2 vs. 13.7 ng/mgprot, P < 0.05) compared with the beef cattle in RP-CrPyr nosupplementation groups. Transport or RP-CrPyr had no effect on the liver glycogen content and the levels of G-6-Pase and PEPCK (P > 0.05).
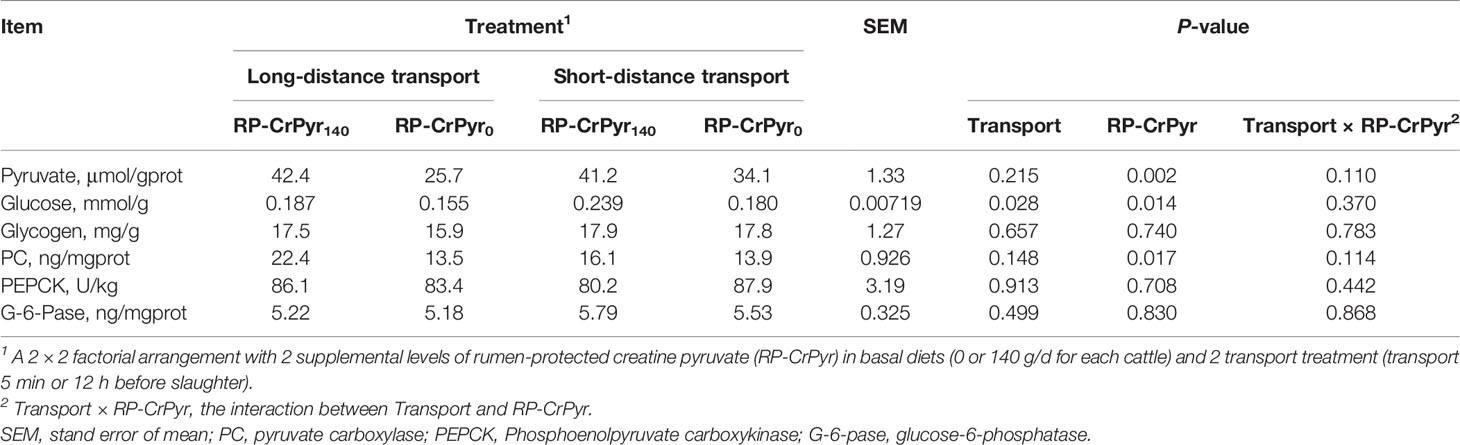
Table 6 Effects of transport and rumen-protected creatine pyruvate on the hepatic gluconeogenesis of beef cattle.
Discussion
The results of our previous study indicated that transport significantly decreased the catalase activity and total antioxidant capacity in serum, which shows that transport leads to oxidative damage to beef cattle (Mao et al., 2022). It is widely known that oxidative damage might be harmful to the cellular and alter membrane permeability and thus, the stress enzymes such as CK and LDH are released into the serum through leakage arising from altered membrane permeability (Mader, 2003; Sattler and Fürll, 2004). Consistently, previous studies indicated that serum alanine aminotransferase, aspartate aminotransferase, CK, and LDH activities were increased in transported wild ungulates due to tissue damage, poor muscular tissue perfusion, reduced heat dissipation, hypoxia, and fatigue (López-Olvera et al., 2006). In this study, serum CK and LDH activities were lower in the RP-CrPyr-supplemented group which indicates that oxidative damage was minimized. Correspondingly, our previous study presented that RP-CrPyr reduced oxidative stress, to some extent, by tending to decrease the serum MDA concentration (Mao et al., 2022). Creatine and pyruvate are both energetic and antioxidant substances (Rieger et al., 2017), RP-CrPyr can be absorbed in the form of pyruvate and creatine in the small intestine after ingestion, thereby enhancing the antioxidant properties of beef cattle. Blood glucose level is used as a metabolic indicator of energy status in cattle. Transportation without feed and water for 2 days caused a decrease in serum glucose of steer calves immediately after transportation (Takemoto et al., 2018). While, another study reported that the blood glucose of bulls increased significantly after 9 h of transport (Earley et al., 2013). Our result was different with both of these study that there was no difference in serum glucose between the long-distance transport and short-distance transport. This may be due to the differences in the transportation time, which can be summarized as: 2 days of transport results in a decrease, 9 h of transport results in an increase, and 12 h of transport with 2 h of recovery did not cause change in blood glucose. Moreover, the present study showed that RP-CrPyr supplementation had no effect on serum pyruvate and creatine contents. For this, one possible explanation might be that the pyruvate and creatine entered the tissues from blood when sampling.
It is well known that pre-slaughter stress results in depletion of glycogen stores in the muscle, further limits post-mortem glycolysis and the formation of lactic acid, and leads to a high pHu (Ponnampalam et al., 2017; Shange et al., 2019). Among which, transport stress is one of the major factors. In the present study, the transport × RP-CrPyr interaction significantly affected the pH45 min value of muscle, which seems to be associated with the observed interaction for glycogen and GP in muscle (Table 4). This suggests that RP-CrPyr could restore the long-distance transport-induced rice in pH45 min,by increasing muscle glycogen content. Moreover, long-distance transport followed with recovery treatment significantly increased the pH45 min value and decreased glycogen and GP, while had no effect on pH24 h value. This situation may be assumed because of the reduced muscle glycogen retention related to transport stress but it was not low enough to result in high pH24 h. Indeed, it was suggested the relationship between initial muscle glycogen content and ultimate pH is only linear at very low levels of glycogen (Marıía et al., 2003). Similarly, Fernandez et al. (1996) reported that there was no significant change in the pH at 48 h postmortem while 11 h of transport significantly reduced muscle GP and increased pH at 4 h postmortem. Moreover, a previous study reported beef meat that has a pH24 h greater than 5.5 is considered a result of pre-slaughter glycogen depletion and comes with a lack of glycogen in muscle that accumulated adequate lactate concentration (Kannan et al., 2002). Consistently in this study, the muscle from long-distance transported beef cattle without RP-CrPyr supplementation had pH24 h greater than 5.5 (pH24 h = 5.69). Meat color is the most important component in physical appearance and is used by consumers to evaluate the quality and freshness of meat. When the color of meat changes from bright red (oxymyoglobin, the oxygenated form of the muscle pigment myoglobin) to brown (metmyoglobin), it is well known that a* values are most closely related to the oxymyoglobin content of the meat (Renerre et al., 1996) while L* will decrease with the increase of a* and the flesh color will gradually become dark. Transport stress is accompanied by oxidative stress, which leads to the decline of muscle antioxidant capacity and increase in oxymyoglobin content of meat. Consistently, the long-distance transported beef cattle without RT-CrPyr (antioxidants) supplementation had lower L* value and higher a* value, while long-distance transported beef cattle fed with RT-CrPyr increased L* value and decreased a* value. In addition, our findings showed that long-distance transport followed with recovery treatment significantly increased muscle drip loss. This result was in accordance with at least one study (Honkavaara et al., 2003) but in contrast to others (Fernandez et al., 1996; Xin et al., 2018).
In the current study, RP-CrPyr supplementation reduced the pH24 h value and this was attributed to RP-CrPyr increasing the muscle glycogen content and glycolytic potential at the early postmortem period, providing sufficient glycogen and lactate for the reduction of muscle pH at 24 h postmortem. Previous research indicated that exogenous pyruvate, creatine, and CrPyr could promote glycogen stores in muscle of mammalian (Ceddia and Sweeney, 2004; Ju et al., 2005; Guo et al., 2009; Chen et al., 2012; Shetty et al., 2012; ), therefore, the muscle glycogen level in cattle fed RP-CrPyr was higher in this study. During early postmortem metabolism, the phosphagen system and glycolysis contribute to the maintenance of ATP levels (Scheffler and Gerrard, 2007). Creatine supplementation has been shown to promote the increase in intramuscular PCr, which could improve the energy supply substrates of the phosphagen system, relatively reduce glycogenolysis, and thereby decrease lactate production (Li et al., 2018). Consistent with this, the present study shows that RP-CrPyr increased muscle ATP reserve and decreased the muscle lactate content at early postmortem. Glycolytic enzymes are the key factors regulating glycolytic flow and lactate accumulation in muscle (Scheffler and Gerrard, 2007). Oxidative stress causes the inactivation of key glycolytic enzymes in mammalian cells (Reichmann et al., 2018). Our previous study showed that RP-CrPyr could reduce oxidative stress induced by transport stress to some extent (Mao et al., 2022). Therefore, in this study the enhanced activity of glycolytic enzyme HK by RP-CrPyr might be attributed to the antioxidant properties of CrPyr. Similarly, Zhao et al. (2018) reported that in ovo feeding of CrPyr increased glycolytic enzyme activity and promoted glycolysis in chest of broilers. Bortoluzzi et al. (2019) found that creatine, plus pyruvate supplementation, prevented the oxidative stress induced decrease in HK and PK cell activities in the brain of rats subjected to chemically-induced phenylketonuria.
For ruminants, the gluconeogenesis in liver accounts for 80% of endogenous glucose (Aschenbach et al., 2010). Propionate, glucogenic amino acids, lactate, glycerol, and pyruvate are all substrates for hepatic gluconeogenesis in ruminants. Previous research has found that supplementation of gluconeogenic precursors increased glycogen content in muscle and liver via stimulating the rate of gluconeogenesis (Volpi-Lagreca and Duckett, 2017). Our results show that RP-CrPyr supplementation increase the liver pyruvate content and provide an additional source of substrate for gluconeogenesis, which in turn increases hepatic glucose production and glucose levels. In the process of gluconeogenesis, PC is the primary key enzyme for the conversion of pyruvate to oxaloacetic acid, which is then converted to phosphoenolpyruvate through PEPCK, undergoes a series of non-enzymatic reactions to generate glucose-6-phosphate, and finally catalyzes by G-6-Pase enzyme to produce glucose. The PC, PEPCK, and G-6-Pase are the rate-limiting enzymes responsible for gluconeogenesis (Aschenbach et al., 2010), and their activity is regulated transcriptionally and post-transcriptionally, such the supply of gluconeogenic precursors to the liver as well as by insulin and glucagon (She et al., 1999; Bobe et al., 2009). In this study, we found that RP-CrPyr supplementation increased the activity of PC. Similarly, Zhao et al. (2018) reported that in ovo feeding of CrPyr up-regulated the mRNA expression levels of PC and PEPCK in broilers. Hence, the increased hepatic gluconeogenic enhanced the glucose generation, which might be useful to the muscle glycogen deposition.
In conclusion, dietary supplementation, with RP-CrPyr, improved meat quality and energy metabolism regulatory capacity in beef cattle suffering from long-distance transport followed with recovery treatment. This may show that RP-CrPyr attributes to increased muscle glycogen storage and energy supply and hepatic gluconeogenesis (Figure 1).
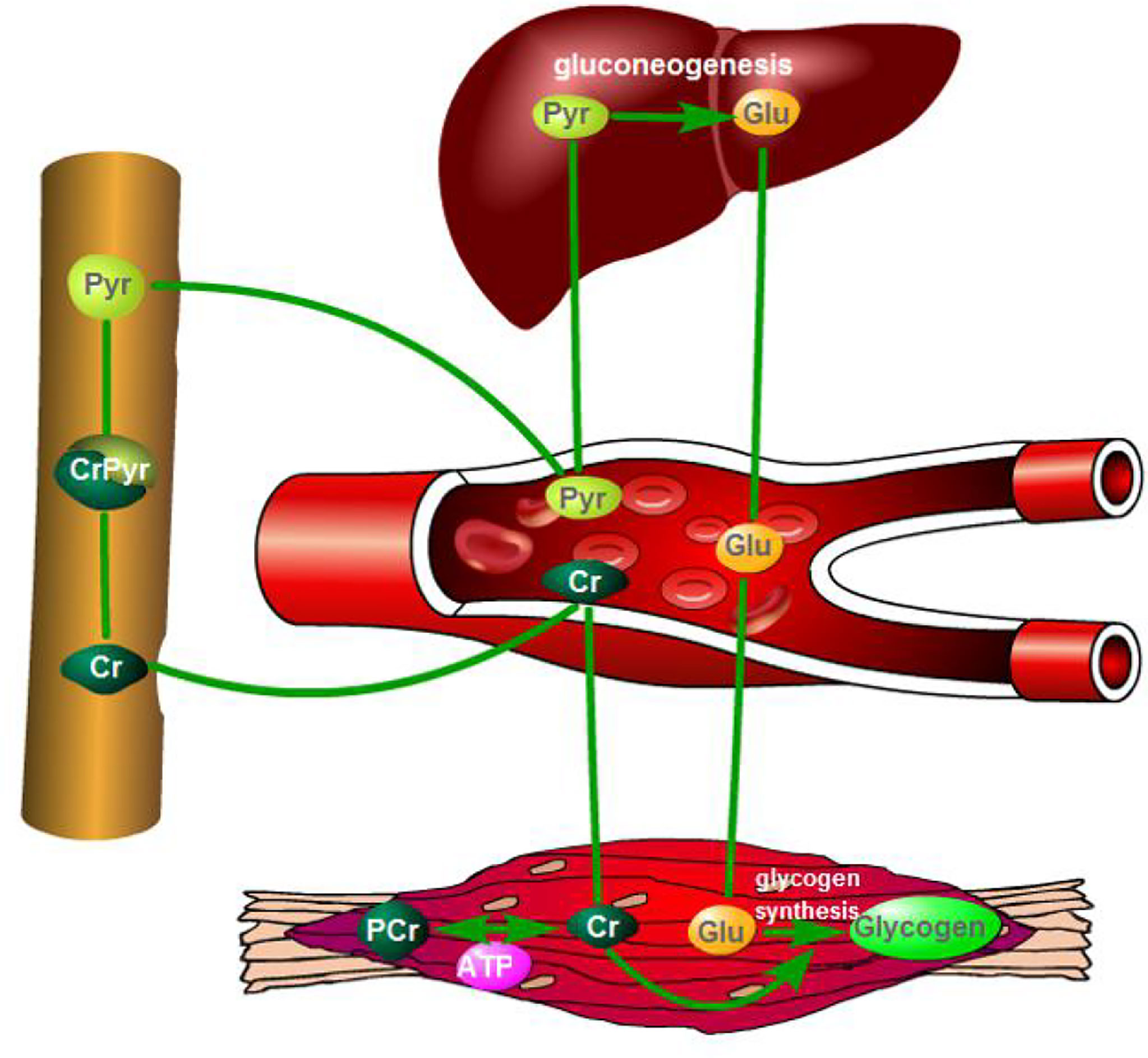
Figure 1 The possible mechanisms diagram of creatine pyruvate promoting beef muscle glycogen deposit. CrPyr, creatine pyruvate; Cr, creatine; Py, pyruvate; Glu, glucose; ATP, adenosine triphosphate.
Data Availability Statement
The datasets presented in this article are not readily available because the study data are owned by Jiangxi Province Key Laboratory of Animal Nutrition. Access to these data would require additional approval beyond that of the authors. Requests to access these datasets should be directed to Dr. Yanjiao Li, yanjiaoli221@163.com.
Ethics Statement
This experiment was approved by the Committee for the Care and Use of Experimental Animals at Jiangxi Agricultural University (JXAULL-2021-10).
Author Contributions
GL and YL designed the overall study. GL, KM, YZ, XZ, QQ, MQ, and KO performed experiments. GL and YL wrote the manuscript. All authors contributed to the article and approved the submitted version.
Funding
This work was supported by the National Natural Science Foundation of China (No. 32160814), the Jiangxi Provincial Youth Scientist Foundation (20202BAB215010), and the China Agriculture Research System of MOF and MARA (CARS-37).
Conflict of Interest
The authors declare that the research was conducted in the absence of any commercial or financial relationships that could be construed as a potential conflict of interest.
Publisher’s Note
All claims expressed in this article are solely those of the authors and do not necessarily represent those of their affiliated organizations, or those of the publisher, the editors and the reviewers. Any product that may be evaluated in this article, or claim that may be made by its manufacturer, is not guaranteed or endorsed by the publisher.
Acknowledgments
The authors appreciate all the helps from our colleagues and collaborators.
References
Allen P. J. (2012). Creatine Metabolism and Psychiatric Disorders: Does Creatine Supplementation Have Therapeutic Value? Neurosci. Biobehav. Rev. 36 (5), 1442–1462. doi: 10.1016/j.neubiorev.2012.03.005
Aschenbach J. R., Kristensen N. B., Donkin S. S., Hammon H. M., Penner G. B. (2010). Gluconeogenesis in Dairy Cows: The Secret of Making Sweet Milk From Sour Dough. IUBMB. Life. 62 (12), 869–877. doi: 10.1002/iub.400
Bobe G., Velez J. C., Beitz D. C., Donkin S. S. (2009). Glucagon Increases Hepatic mRNA Concentrations of Ureagenic and Gluco-Neogenic Enzymes in Early-Lactation Dairy Cows. J. Dairy. Sci. 92, 5092–5099. doi: 10.3168/jds.2009-2152
Bortoluzzi V. T., Brust L., Preissler T., de Franceschi I. D., Wannmacher C. M.D. (2019). Creatine Plus Pyruvate Supplementation Pre-Vents Oxidative Stress and Phosphotransfer Network Disturbances in the Brain of Rats Subjected to Chemically-Induced Phe-Nylketonuria. Metab. Brain. Dis. 34 (6), 1649–1660. doi: 10.1007/s11011-019-00472-7
Ceddia R. B., Sweeney G. (2004). Creatine Supplementation Increases Glucose Oxidation and AMPK Phosphorylation and Reduces Lactate Production in L6 Rat Skeletal Muscle Cells. J. Physiol. 555 (2), 409–421. doi: 10.1113/jphysiol.2003.056291
Chen J., Huang J., Deng J., Ma H., Zou S. (2012). Use of Comparative Proteomics to Identify the Effects of Creatine Pyruvate on Lipid and Protein Metabolism in Broiler Chickens. Vet. J. 193 (2), 514–521. doi: 10.1016/j.tvjl.2012.01.034
Chen J., Wang M., Kong Y., Ma H., Zou S. (2011). Comparison of the Novel Compounds Creatine and Pyruvateon Lipid and Protein Metabolism in Broiler Chickens. Animal 5 (7), 1082–1089. doi: 10.1017/S1751731111000085
Earley B., Drennan M., O'Riordan E. G. (2013). The Effect of Road Transport in Comparison to a Novel Environment on the Physio-Logical, Metabolic and Behavioural Responses of Bulls. Res. Vet. Sci. 95, 811–818. doi: 10.1016/j.rvsc.2013.04.027
Ferguson D. M., Warner R. D. (2008). Have We Underestimated the Impact of Pre-Slaughter Stress on Meat Quality in Ruminants? Meat. Sci. 80 (1), 12–19. doi: 10.1016/j.meatsci.2008.05.004
Fernandez X., Monin G., Culioli J., Legrand I., Quilichini Y. (1996). Effect of Duration of Feed Withdrawal and Transportation Time on Muscle Characteristics and Quality in Friesian-Holstein Calves. J. Anim. Sci. 74 (7), 1576–1583. doi: 10.2527/1996.7471576x
Guo Y., Gong D., Li X, Cui L. L., Wei M., Hu J. Y., et al. (2009). Effects of Calcium Pyruvate Supplemen-Tation on Exercise Ability in Mice. J. Dalian. Med. Univ. 31 (2), 145–147,161.
Honkavaara M., Rintasalo E., Ylönen J., Pudas T. (2003). Meat Quality and Transport Stress of Cattle. Dtsch. Tierarztl. Wochenschr. 110 (3), 125–128.
Hu S., Lin Z. L., Zhao Z. K., Liu R., Ma L., Luo H. M., et al. (2016). Pyruvate Is Superior to Citrate in Oral Rehy-Dration Solution in the Protection of Intestine via Hypoxia Inducible Factor-1 Activation in Rats With Burn Injury. JPEN. J. Parenter. Enteral. Nutr. 40 (7), 924–933. doi: 10.1177/0148607115577817
Jäger R., Metzger J., Lautmann K., Shushakov V., Purpura M., Geiss K. R., et al. (2008). The Effects of Creatine Pyruvate and Creatine Citrate on Performance During High Intensity Exercise. J. Int. Soc Sports. Nutr. 5 (1), 1–9. doi: 10.1007/s00726-011-0874-6
Jäger R., Purpura M., Shao A., Inoue T., Kreider R. B. (2011). Aalysis of the Efficacy, Safety, and Regulatory Status of Novel Forms of Creatine. Amino. Acids 40 (5), 1369–1383. doi: 10.1007/s00726-011-0874-6
Ju J. S., Smith J. L., Oppelt P. J., Fisher J. S. (2005). Creatine Feeding Increases GLUT4 Expression in Rat Skeletal Muscle. Am. J. Physiol. Endocrinol. Metab. 288 (2), E347–E352. doi: 10.1152/ajpendo.00238.2004
Kannan G., Chawan C. B., Kouakou B., Gelaye S. (2002). Inflfluence of Packaging Method and Storage Time on Shear Value and Mechanical Strength of Intramuscular Connective Tissue of Chevon. J. Anim. Sci. 80, 2383–2389. doi: 10.1051/gse:2002028
Li Y. J., Gao T., Li J. L., Zhang L., Gao F., Zhou G. H. (2017). Effects of Dietary Starch Types on Early Postmortem Muscle Energy Metabolism in Finishing Pigs. Meat. Sci. 133, 204–209. doi: 10.1016/j.meatsci.2017.07.008
Li J., Zhang L., Fu Y., Li Y., Jiang Y., Zhou G., Gao F. (2018). Creatine Monohydrate and Guanidinoacetic Acid Supplementation Affects the Growth Performance, Meat Quality, and Creatine Metabolism of Finishing Pigs. J. Agric. Food Chem. 66 (38), 9952–9959. doi: 10.1021/acs.jafc.8b02534
López-Olvera J. R., Marco I., Montané J., Lavín S. (2006). Transport Stress in Southern Chamois (Rupicapra Pyrenaica) and its Modulation by Acepromazine. Vet. J. 172 (2), 347–355. doi: 10.1016/j.tvjl.2005.06.007
Lu C., Cao Y., Li J., Gao Y., Li Q., Yang J., et al. (2015). Effects of Preslaughter Transportation Stress on Slaughter Performance and Beef Quality of Meet Cattle. Chin. J. Vet. Sci. 35 (12), 2045–2048. doi: 10.16303/j.cnki.1005-4545.2015.12.30
Mader T. L. (2003). Environmental Stress in Confined Beef Cattle. J. Anim. Sci. 81 (E. Suppl. 2), E110–E119. doi: 10.2527/2003.8114_suppl_2E110x
Mao K., Lu G., Li Y., Zang Y., Zhao X., Qiu Q., et al. (2022). Effects of Rumen-Protected Creatine Pyruvate on Blood Bio-Chemical Parameters and Rumen Fluid Characteristics in Transported Beef Cattle. BMC. Vet. Res. 18 (1), 1–15. doi: 10.1186/s12917-021-03134-y
Marıía G. A., Villarroel M., Sañudo C., Olleta J. L., Gebresenbet G. (2003). Effect of Transport Time and Ageing on Aspects of Beef Quality. Meat. Sci. 65 (4), 1335–1340. doi: 10.1016/S0309-1740(03)00054-8
Monin G., Sellier P. (1985). Pork of Low Technological Quality With a Normal Rate of Muscle pH Fall in the Immediate Post-Mortem Period: The Case of the Hampshire Breed. Meat. Sci. 13, 49–63. doi: 10.1016/S0309-1740(85)80004-8
Ponnampalam E. N., Hopkins D. L., Bruce H., Li D., Baldi G., Bekhit A. E.D., et al. (2017). Causes and Contributing Factors to “Dark Cutting” Meat: Current Trends and Future Directions: A Review Compr. Rev. Food Sci. Food Saf. 16 (3), 400–430. doi: 10.1111/1541-4337.12258
Reichmann D., Voth W., Jakob U. (2018). Maintaining a Healthy Proteome During Oxidative Stress. Mol. Cell. 69 (2), 203–213. doi: 10.1016/j.molcel.2017.12.021
Renerre M., Dumont F., Gatellier P.. (1996). Antioxidant Enzyme Activities in Beef in Relation to Oxidation of Lipid and Myoglobin. Meat. Sci. 43, 111–121. doi: 10.1016/0309-1740(96)84583-9
Rieger E., de Franceschi I. D., Preissler T., Wannmacher C. M.D. (2017). Neuroprotective Effect of Creatine and Pyruvate on Enzyme Ac-Tivities of Phosphoryl Transfer Network and Oxidative Stress Alterations Caused by Leucine Administration in Wistar Rats. Neurotox. Res. 32, 575–584. doi: 10.1007/s12640-017-9762-5
Sattler T., Fürll M. (2004). Creatine Kinase and Aspartate Aminotransferase in Cows as Indicators for Endometritis. J. Vet. Med. A. Physiol. Pathol. Clin. Med. 51 (3), 132–137. doi: 10.1111/j.1439-0442.2004.00612.x
Scheffler T. L., Gerrard D. E. (2007). Mechanisms Controlling Pork Quality Development: The Biochemistry Controlling Postmortem Energy Metabolism. Meat. Sci. 77 (1), 7–16. doi: 10.1016/j.meatsci.2007.04.024
Schoch R. D., Willoughby D., Greenwood M. (2006). The Regulation and Expression of the Creatine Transporter: A Brief Review of Creatine Supplementation in Humans and Animals. J. Int. Soc Sports. Nutr. 3 (1), 60–66. doi: 10.1186/1550-2783-3-1-60
Shange N., Gouws P., Hoffman L. C. (2019). Changes in Ph, Colour and the Microbiology of Black Wildebeest (Connochaetes Gnou) Longissimus Thoracis Et Lumborum (LTL) Muscle With Normal and High (DFD) Muscle pH. Meat. Sci. 147, 13–19. doi: 10.1016/j.meatsci.2018.08.021
Sharma P., Benford B., Li Z. Z., Ling G. S. (2009). Role of Pyruvate Dehydrogenase Complex in Traumatic Brain Injury and Meas-Urement of Pyruvate Dehydrogenase Enzyme by Dipstick Test. J. Emerg. Trauma. Shock. 2 (2), 67–72. doi: 10.4103/0974-2700.50739
She P., Lindberg G. L., Hippen A. R., Beitz D. C., Young J. W. (1999). Regulation of Messenger Ribonucleic Acid Expression for Gluconeogenic Enzymes During Glucagon Infusions Into Lactating Cows. J. Dairy. Sci. 82, 1153–1163. doi: 10.3168/jds.S0022-0302(99)75338-5
Shetty P. K., Sadgrove M. P., Galeffi F., Turner D. A. (2012). Pyruvate Incubation Enhances Glycogen Stores and Sustains Neuronal Function During Subsequent Glucose Deprivation. Neurobiol. Dis. 45 (1), 177–187. doi: 10.1016/j.nbd.2011.08.002
Takemoto S., Funaba M., Matsui T. (2018). Effect of Niacin Supplementation in Long-Distance Transported Steer Calves. Anim. Sci. J. 89, 1442–1450. doi: 10.1111/asj.13084
Volpi-Lagreca G., Duckett S. (2017). Supplementation of Glycerol or Fructose via Drinking Water to Grazing Lambs on Tissue Gly-Cogen Level and Lipogenesis. J. Anim. Sci. 95, 2558–2575. doi: 10.2527/jas.2017.1449
Xin L. I., Xia A. Q., Chen L. J., Du M. T., Li C., Ning K., et al. (2018). Effects of Lairage After Transport on Post Mortem Muscle Glycolysis, Protein Phosphorylation and Lamb Meat Quality. J. Integr. Agric. 17 (10), 2336–2344. doi: 10.1016/S2095-3119(18)61922-7
Zhang L., Yue H. Y., Zhang H. J., Xu L., Wu S. G., Yan H. J., et al. (2009). Transport Stress in Broilers: I. Blood Metabolism, Glycolytic Potential, and Meat Quality. Poultry. Sci. 88, 2033–2041. doi: 10.3382/ps.2009-00128
Zhao Q. (2013). Survey on DFD Beef Incidences in China and Specification in Pre-Slaughter Management of Cattle. [Master’s Thesis] (Taian, China: Shandong Agricultural University).
Zhao M. M. (2017). Regulatory Mechanism of in Ovo Feeding of Creatine Pyruvate on Energy Metabolism and Muscle Development of Broiler Chickens. [Master’s Thesis] (Nanjing, China: Nanjing Agricultural University).
Zhao M., Gong D., Gao T., Zhang L., Li J., Lv P. A., et al. (2018). In Ovo Feeding of Creatine Pyruvate Increases the Glycolysis Pathway, Glucose Transporter Gene Expression, and AMPK Phosphorylation in Breast Muscle of Neonatal Broilers. J. Agric. Food Chem. 66 (29), 7684–7691. doi: 10.1021/acs.jafc.8b02557
Keywords: beef cattle, creatine pyruvate, meat quality, muscle glycogen, transport stress
Citation: Lu G, Li Y, Mao K, Zang Y, Zhao X, Qiu Q, Qu M and Ouyang K (2022) Effects of Rumen-Protected Creatine Pyruvate on Meat Quality, Hepatic Gluconeogenesis, and Muscle Energy Metabolism of Long-Distance Transported Beef Cattle. Front. Anim. Sci. 3:904503. doi: 10.3389/fanim.2022.904503
Received: 25 March 2022; Accepted: 05 May 2022;
Published: 14 June 2022.
Edited by:
Otávio R. Machado Neto, São Paulo State University, BrazilReviewed by:
Mateus P. Gionbelli, Universidade Federal de Lavras, BrazilJohn Michael Gonzalez, University of Georgia, United States
Copyright © 2022 Lu, Li, Mao, Zang, Zhao, Qiu, Qu and Ouyang. This is an open-access article distributed under the terms of the Creative Commons Attribution License (CC BY). The use, distribution or reproduction in other forums is permitted, provided the original author(s) and the copyright owner(s) are credited and that the original publication in this journal is cited, in accordance with accepted academic practice. No use, distribution or reproduction is permitted which does not comply with these terms.
*Correspondence: Yanjiao Li, yanjiaoli221@163.com