- 1Animal Science Department, Western Paraná State University, Marechal Cândido Rondon, PR, Brazil
- 2Forage-Animal Production Research Unit, USDA-ARS, Lexington, KY, United States
- 3Department of Animal Science, University of Tennessee, Knoxville, TN, United States
- 4Department of Plant and Soil Sciences, University of Kentucky, Lexington, KY, United States
- 5Department of Animal and Food Science, University of Kentucky, Lexington, KY, United States
Dopamine has multiple physiological functions including feed intake control in which it can act as an anorectic or orexigenic agent. This study had the objective to evaluate intra-abomasal administration of L-DOPA (levodopa; L-3,4-dihydroxyphenylalanine) from -Mucuna pruriens on circulating catecholamines, indicators of energy metabolism and feed intake in cattle. Eight Holstein steers (340 ± 20 kg) fitted with ruminal cannula were used in a replicated 4 x 4 Latin Square design experiment. Intra-abomasal infusion of L-DOPA at 0, 0.5, 1 and 2 mg/kg BW was carried out for seven days and blood samples were collected at 0, 30, 60, 120, 240 and 480 min from L-DOPA infusion on day 7. The area under the curve (AUC) of plasma L-DOPA and free dopamine increased quadratically with the administration of L-DOPA. However, the AUC of plasma total dopamine had a positive linear response with the increase of L-DOPA. Conversely, the serum 5-hydroxytriptophan (5-HTP), plasma serotonin, serum serotonin, serum tyrosine, plasma glucose and plasma free fatty acids were not affected by the intra-abomasal infusion of L-DOPA. The circulating concentration of the epinephrine, norepinephrine, serotonin, glucose and free fatty acids did not change with L-DOPA infusion. It can be concluded that intra-abomasal L-DOPA administration produced a strong increase in circulating dopamine with no change in energy metabolites and feed intake in cattle.
1. Introduction
The dopaminergic system plays many important roles, such as motor control, motivation, reward, cognitive function, and reproductive behaviors (Klein et al., 2019). Dopamine itself is a neurotransmitter associated with reward salience and is linked to food addiction in humans (Mills et al., 2020). Studies suggest that dopamine may function as a central caloric sensor that mediates adjustments in intake according to the caloric density of a meal (Araujo et al., 2012) and palatable foods have been shown to stimulate brain dopamine release (Hajnal et al., 2004). Dopamine also has a role in energy balance (Mietlicki-Baase et al., 2015) and glucose metabolism (Ren et al., 2010). Dopamine D2 receptors affect insulin synthesis (Melkersson and Jansson, 2007; García-Tornadú et al., 2010) as well as glucose tolerance (García-Tornadú et al., 2010). The modulation of dopamine metabolism and consequently its roles (e.g. behavior and feed intake) has potential to impact livestock production.
The effects of the dopaminergic system on feed intake control are very complex and not completely understood. The expression of both the insulin and leptin receptors in dopaminergic neurons support the notion that post-absorptive signals may directly affect the activity of the dopaminergic system in both central and peripheral systems (Aslanoglou et al., 2021, Billes et al., 2012, Omrani et al., 2021). However, the dopamine response on feed intake can also act independently of insulin or leptin (Liu et al., 2011) with effects associated with ghrelin (Andrews et al., 2009) and neuropeptide Y (Kuo, 2002). Dopamine in the central neural system controls feed intake largely by motivation (Morales and Berridge, 2020, Ott and Nieder, 2019) while in the peripheral system feed intake is controlled mainly through effects on energy metabolism, sympathetic stress regulation, digestion and gastrointestinal motility (Mills et al., 2020).
Dopamine does not cross the blood-brain barrier (BBB); therefore, dopaminergic signaling in the brain is functionally distinct from peripheral pathways and can have many roles such as the regulation of respiration, gastrointestinal motility and blood pressure (Rubí and Maechler, 2010). However, L-DOPA can cross BBB and be used for dopamine synthesis (Figure 1) in both central and peripheral systems (Dayan, 2003) and it is not as closely regulated as tyrosine, the primary precursor (González-Sepúlveda et al., 2022). Although the dopamine pools are independent between those systems, they also have interconnected responses that can occur by vagus-mediated dopamine neuron activity (Fernandes et al., 2020, Müller et al., 2022). Because dopamine can control both initiation and termination of a meal (Joshi et al., 2022), the determination of the optimum dose of its precursor is critical to the viability of the use of L-DOPA in farm animals.
Although L-DOPA has been used to treat Parkinson’s disease for decades (Lenka et al., 2022), its potential benefits for use in livestock is unknown. L-DOPA extraction from plants is relatively simple using acidified water (Dhanani et al., 2015). Most studies have used Mucuna pruriens, a high L-DOPA content plant, as a protein ingredient (Vadivel and Pugalenthi, 2010; Madzimure et al., 2014; Peniche-Gonzalez et al., 2018) and it is usually treated to reduce L-DOPA or restricted to a low inclusion of seeds and leaves when fed to livestock to avoid toxicity by excessive L-DOPA.
Many studies with lab animals have produced conflicting results regarding dopamine effects on feed intake in which a dopamine receptor is responsible for mediating the effect (Davis et al., 2009; Land et al., 2014). Increased dopamine D2/D3 receptor binding in the anteroventral striatum has been reported in patients with anorexia nervosa (Frank et al., 2005). Conversely, stimulation of the D1 dopamine neurons increases feeding in rats (Land et al., 2014). Szczypka et al. (2000) observed that rats incapable of synthesizing dopamine in dopaminergic neurons gradually become aphagic and died of starvation. The differing results are caused by multiple factors such as the concentration of dopamine, site of action (peripherical or central system), receptors, type of stimuli (reward), behavior, association with hormones (insulin, leptin, ghrelin), energy balance and others. Dopamine contributes to addiction through reinforcing positive stimuli (Volkow et al., 2017). Joint activity between both dopaminergic and serotonergic systems have been suggested (Fischer and Ullsperger, 2017; Jennings, 2013) However, in studies using rats the relation between concentration and synthesis of those neurotransmitters has shown divergent results (Algeri and Cerletti, 1974; Biskup et al., 2012). The complexity of dopaminergic metabolism can modulate other systems. Dopamine is a precursor of epinephrine (Meiser et al., 2013) which is related to stress events and produces an anorexigenic response (Wallace and Fordahl, 2022). There is a lack of information about the modulation of dopamine metabolism without triggering an undesirable response in livestock. Overall, information about dopamine metabolism in farm animals is scarce, there are few studies evaluating L-DOPA and its potential benefits in cattle, and no information regarding intestinal dosing effects on feed intake. This study had the objective of evaluating intra-abomasal infusion of pure L-DOPA on circulating catecholamines, indicators of energy metabolism and feed intake in cattle.
2. Materials and methods
The experiment was performed under approval by the Animal Care and Use Committee at the University of Kentucky.
2.1. Animals and design
Eight Holstein steers (340 ± 20 kg) fitted with ruminal cannulas (Bar Diamond, Inc., Parma, ID, USA) were used in a replicated 4 x 4 Latin Square design experiment. Temporary i.v. catheters (14 Ga x 13 cm, Mila International, Inc., Florence, KY, USA) were inserted into the jugular vein one day before sampling in each period.
All steers were housed indoors in individual pens (3 x 3 m) each with its own feed bunk and water supply. A thermoneutral status was maintained at 22°C with a light:dark cycle of 14 h light and 10 h dark.
The experiment included one 12-day period for acclimation to diet and facilities and four experimental periods of 7 days. In each 7-day period, the first 2 days were used for a washout of the previous treatment. The L-DOPA was administered from day 3 to 7 and samples were collected on day 7. Preliminary tests (unpublished) in steers indicated low residual effects of L-DOPA on circulating dopamine. Body weight (BW) was measured before feeding at the beginning and the end of each 7-day period.
2.2. Treatments and diet
The treatments were intra-abomasal infusion of L-DOPA at 0, 0.5, 1 and 2 mg/kg BW. The doses were chosen based on published information from i.v. infusions in cattle (Kasuya et al., 2017). The steers had ad libitum access to alfalfa cubes offered once a day at 0730 h allowing for 5% orts. Sixty grams of mineral supplement were given daily (Table 1).
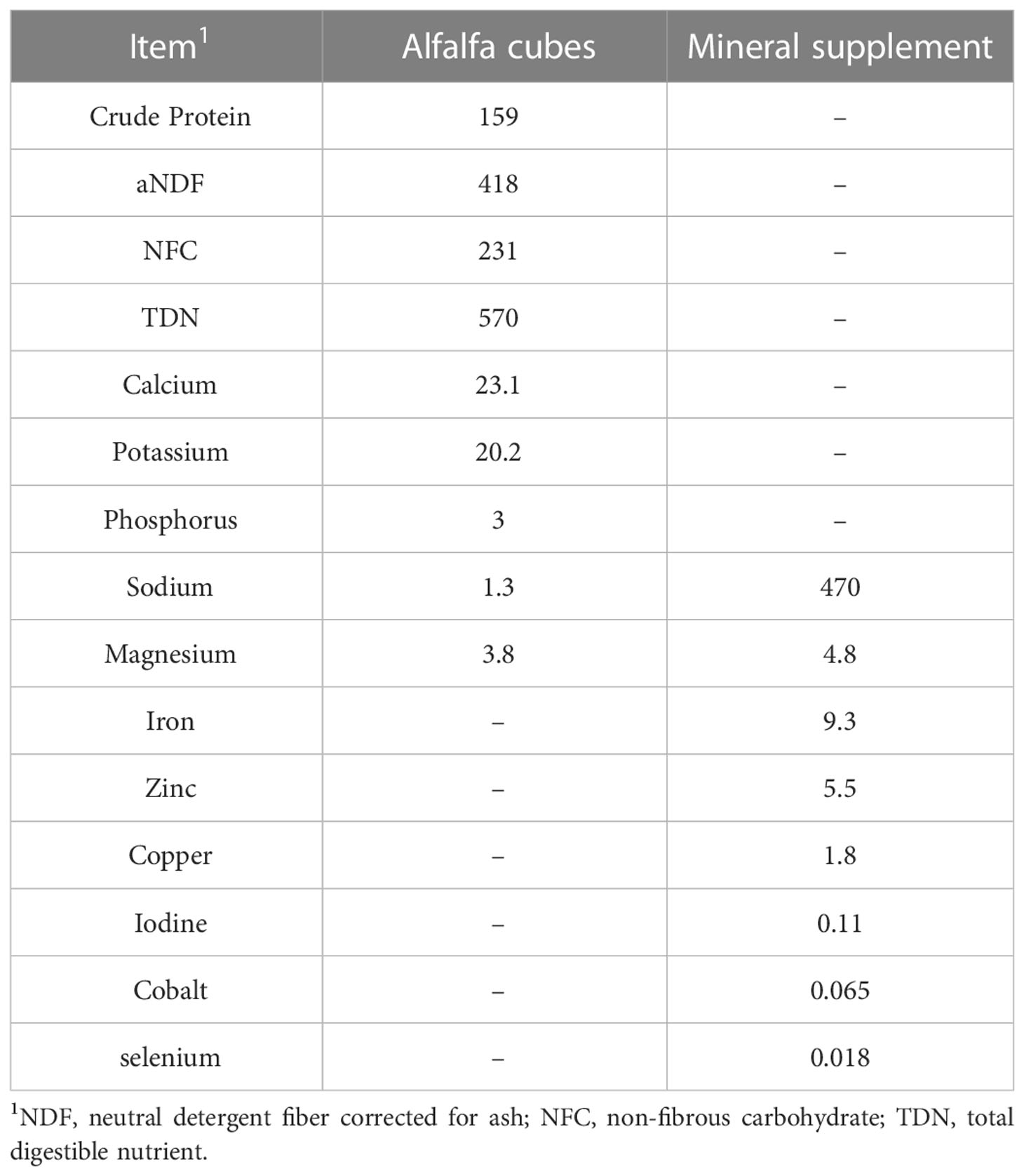
Table 1 Chemical composition (g/kg) of the diet fed to steers dosed abomasally with levodopa (L-DOPA).
Intra-abomasal infusions were accomplished by placing an infusion line made of flexible 6.3 mm o.d. tubing (Tygon®) and a plastisol washer (60 mm o.d.) through the reticulo-omasal orifice into the abomasum. The omasal pillar was used as a landmark and the washer was manipulated until it was distal to the pillar. The infusion line was inserted at the beginning of the experiment.
Pure L-DOPA from Mucuna pruriens (Nutrivita, Lake Forest, CA, United States) was infused in a single dose using a syringe in the course of 1-2 min immediately before feeding (0730 h). The L-DOPA dilutions were dissolved in 40 mL of ultrapure water immediately before the infusion. Only water (40 mL) was infused in the treatment without L-DOPA. After the infusion, 30 mL of water was infused to purge the infusion line of any remaining L-DOPA. The dosage was calculated based on individual body weight measured and adjusted each period.
2.3. Feed intake
The offered feed was adjusted daily according to the orts from the previous day, allowing for 5% orts. Feed intake was recorded daily. Feed and orts were sampled every week and analyzed for dry matter (DM, index 920.39) according to AOAC (1990). Additionally, dry matter intake (DMI) was expressed relative to body weight (g/kg BW) with BW estimated by linear regression using BW taken throughout the experiment.
The DMI from day 2 was used as basal intake and data from day 3 to 7 (5 days) were used as treatment response. The feed intake distribution throughout the day was monitored every minute by an electronic scale with datalogger attached to each animal’s feed bunk (Campbell Scientific, Logan, Utah, United States). Feeding behavior (frequency of meals (meals/d), average meal size and duration) was calculated for each animal on each day (from day 3 to 7) as described previously (Egert-McLean et al., 2019).
2.4. Blood sampling
Blood was collected on day 7 from the jugular vein of each steer at 0, 30, 60, 120, 240 and 480 min from L-DOPA infusion. Before blood sample collection, 10 mL of blood and lock solution were aspirated from the catheter and discarded. Then a 20-mL sample of blood from the jugular catheter was collected into syringes, 12 mL was transferred to a 15-mL tube with 20 µL of HCl (6 M), 400 µL EDTA disodium salt (0.1M) and 80 µL Na2S205 (0.5M), 4 mL of blood was transferred to a tube with clot activator for serum and 4 mL were transferred to a tube with sodium fluoride. After blood collection, a lock solution of heparin (20 U/mL of saline) was infused (5 mL) into the catheter to flush blood and maintain patency. Blood samples in the tube with clot activator were kept at room temperature in the dark for 45-60 min for clotting to occur before centrifugation. Blood samples for plasma were immediately centrifuged after collection and samples for serum were centrifuged after clotting at 3600 x g for 15 min at 10 °C. Plasma and serum aliquots were transferred to 1.5 mL tubes and kept at -80°C until analysis. Plasma for dopamine analysis was deproteinized using 4M HClO4 at the ratio of plasma:HClO4 of 1:0.2.
2.5. Urine sampling
Total urine excretion was collected under vacuum on day 7 using an abdominal funnel connected to a bucket. A solution of HCl (120 mL, 6 M) was added to the buckets to prevent microbial growth and oxidation of dopamine metabolites in the urine. Urine was weighed and sampled at 4, 8 and 24 h after L-DOPA infusion. After each sampling, the bucket was emptied.
2.6. Biochemical analysis
Plasma samples were analyzed for content of L-DOPA, free and total dopamine, and free serotonin while serum was analyzed for concentration of 5-hydroxytryptophan (5-HTP), total serotonin, tyrosine, tryptophan using high performance liquid chromatography (HPLC) with fluorescence detection.
Dopamine was analyzed as described previously (Boomsma et al., 1992) with modifications. For free dopamine extraction, 2 mL of plasma or 0.1 mL of urine were deproteinized with 0.4 or 0.02 mL of 4M HClO4 and centrifuged at 10,000 x g for 10 min. The supernatant was transferred to a 2-mL tube and combined with 2 mg of acid-activated alumina, 1 mL of 2 M Tris-HCl buffer (pH 8.6) and 5 ng of dihydroxybenzylamine as internal standard. To complete the extraction of the catecholamines, the alumina-treated samples were vigorously agitated in a vortex (1200 rpm) for 15 min followed by a 1-min centrifugation (5,000 x g) and the supernatant was discarded. Afterwards, the alumina was washed 2 times with 1 mL of ultrapure water, shaken for 15 s, centrifuged for 1 min and supernatant was discarded. Catecholamines were subsequently eluted from the alumina with 50 μL of 0.05 M HClO4 and sonicated for 10 min. For total dopamine, after deproteinization the samples were heated at 100 °C for 60 min then cooled in an ice bath. Afterwards, the samples were centrifuged, and the extraction of dopamine was carried out as described above.
The 5-HTP, serotonin, tyrosine and tryptophan were analyzed as described previously (Valente et al., 2021) with modifications. Serum and plasma samples were deproteinized by adding 0.2 mL of 4 M HClO4 to 1 mL of sample, followed by vortexing, and centrifuging at 20,000 × g for 10 min at 4°C. The supernatant was transferred to another tube diluted four-fold with ultrapure water and the pH was adjust to 3-4 with KOH and centrifuged at 20,000 × g for 10 min at 4°C. Ultimately, 20 μL of this supernatant was injected into the HPLC for analysis.
Separation of compounds were carried out using a HPLC (model 2695; Waters, Milford, Massachusetts, EUA) with a fluorescence detector (model 2475; Waters, Milford, Massachusetts, EUA) and an ultraviolet detector (Model 2487; Waters, Milford, Massachusetts, EUA). A C18-PFP column (4.6×150 mm, 3 μm, ACE) was used at 40 °C. The mobile phase consisted of 0.05 M KH2PO4 with methanol (85:15, v/v; pH 4.3), at a flow rate of 1.0 mL/min. The fluorescence detector was used with excitation wavelength set at 278 nm and the emission wavelength at 338 nm for 5-HTP, serotonin, tyrosine and tryptophan. For dopamine and L-DOPA the mobile phase consisted of 0.05 M KH2PO4 with methanol (92:8, v/v; pH 2.8), at a flow rate of 1.0 mL/min, the excitation wavelength was set at 280 nm and the emission wavelength at 320 nm. Urinary dopamine was analyzed as described above after centrifuging at 10,000 x g for 15 minutes and diluting 50-fold with water.
Analysis of norepinephrine and epinephrine, with dopamine-d4 as an internal standard, was performed with a Waters Acquity H-Plus ultra-performance liquid chromatography (UPLC) system equipped with a Waters Xevo TQ-S Cronos triple quadrupole mass spectrometer (Waters, Milford, MA USA). Hydrophilic interaction chromatography (HILIC) was utilized for separation using a Waters Acquity UPLC BEH amide column (2.1mm x 150mm x 1.7 um) operated at 45°C with a 10 uL injection volume (Waters, Ireland). The mobile phase consisted of a mixture of 0.1% formic acid in water (A) and 0.1% formic acid in acetonitrile (B) with a flow rate of 0.6 mL/min. Initial conditions of 15% A and 85% B were held from 0 to 0.6 min, followed by a linear gradient to 35% A and 65% B over 8 min, with a return to initial conditions and held until 12 min. The mass spectrometer was operated in positive electrospray ionization mode (ESI+) with detection and quantitation of the ions performed by multiple reaction monitoring (MRM) of the ion transitions: 152 m/z to 107 m/z for norepinephrine, 184 m/z to 166 m/z for epinephrine and 158 m/z to 141 m/z for dopamine-d4 internal standard.
Glucose and free fatty acid (FFA) contents were analyzed in plasma samples using an automatic analyzer (Konelab 20XTI; Thermo Electron Corporation) and a commercial glucose hexokinase assay kit and an enzymatic colorimetric assay kit (Infinity TM, Thermo Scientific), respectively.
2.7. Statistical analysis
All data were analyzed as a replicated 4 x 4 Latin square using the MIXED procedure of SAS (OnDemand for Academics, 2022; SAS Inst. Inc. Cary, NC). The area under the curve (AUC) was calculated using GraphPad Prism 9.4 (GraphPad Software, San Diego, CA, United States) with the linear trapezoidal method.
The circulation metabolites were analyzed as following model:
Where, Yijk is the dependent variable, µ is the overall mean, Si is the effect of steer, Pj is the effect of period, DOPAk is the effect of the L-DOPA level, LS is the effect of Latin Square and eijkl is the residual error.
Intake, feeding behavior and metabolites in the urine were analyzed as repeated measurements over time. The model included the fixed effects of treatment, the hour or day of sampling/measuring and their interaction, and the random effect of animal and period. Various covariance structures of errors were fitted; the best structure for each variable was selected based on the lowest Bayesian information criterion (BIC). The following model was used:
Where, Yijklmn is the dependent variable, µ is the overall mean, Si is the effect of steer, Pj is the effect of period, DOPAk is the effect of the L-DOPA level, Tl is the effect of sampling/measuring time, DOPA x Tm is the interaction between treatment and time, LS is the effect of Latin Square and eijklmn is the residual error.
Shapiro and Wilk’s (1965) test for normality was performed using procedure Univariate of SAS. The L-DOPA, dopamine and serotonin data were transformed according (Box and Cox, 1964) because of the lack of normality as following:
The lambda for each variable was calculated using SAS Transreg with model boxcox ranging from -2 to 2. After statistical analysis, the data were back-transformed to concentration for presentation. The standard error (SE) of the back-transformed data was calculated from the confidence limits of the transformed data as follows:
Where upper CL is back-transformed upper confidence limit and lower CL is back-transformed lower confidence limit.
The DM intake before infusion (basal intake) was used as a covariate for evaluation of DM intake after infusion. For all datasets, an orthogonal partition of the sum of squares of treatments into linear and quadratic responses was obtained after the analysis of variance using the ORPOL function in PROC IML to obtain the appropriate coefficients for the CONTRAST statement due to the unequal spacing between treatments. Statistical significance was considered at P ≤ 0.05 and tendency was considered at 0.05 < P ≤ 0.10.
3. Results
The area under curve (AUC) of plasma L-DOPA, free and total dopamine increased (P< 0.01) quadratically with the administration of L-DOPA (Table 2). Conversely, the serum 5-HTP, plasma serotonin, serum serotonin, serum tyrosine, plasma glucose and plasma free fatty acids were not affected (P > 0.05) by the intra-abomasal infusion of L-DOPA. Serum tryptophan tended to increase quadratically (P = 0.088) with the 0.5 mg/kg BW dose before returning to baseline.
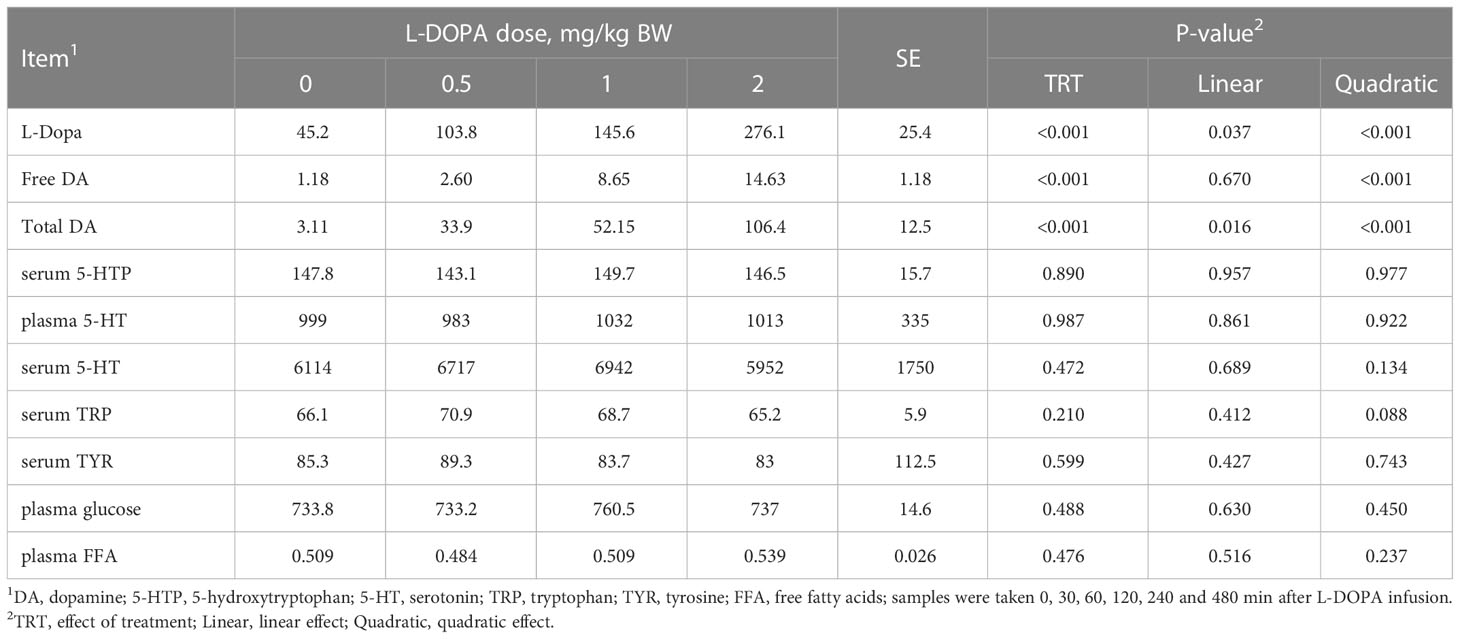
Table 2 Area under the curve of circulating metabolites in steers receiving intra-abomasal infusion of levodopa (L-DOPA).
There was an interaction (P< 0.001) between treatment and time for plasma L-DOPA though no interaction (P > 0.05) was found for free and total dopamine (Figure 2). Plasma L-DOPA had a peak at 1 h after dosing with the concentration decreasing close to baseline after 2 h with no difference (P > 0.05) between doses (Figure 2A). Plasma free dopamine had a peak at 2 h after intra-abomasal L-DOPA administration at the 1 and 2 mg/kg BW doses, and a less evident peak at 1 h after the infusion at the 0.5 mg/kg BW dose (Figure 2C). However, plasma free dopamine was steady after 4 h. Plasma total dopamine had a peak at 1 h after the L-DOPA infusion (Figure 2E). Plasma free dopamine had a slight decrease post-peak followed by a steady concentration whereas, plasma total dopamine declined after the peak. The AUC of L-DOPA and total dopamine increased quadratically (Figures 2B, F) while the AUC of plasma free dopamine was best described by a logistic model (Figure 2D) indicating a plateau in the increase. The plasma L-DOPA increased 46, 902, 2289 and 3707% 1h after infusion and feeding for the 0, 0.5, 1 and 2 mg/kg BW doses, respectively. Moreover, 2h after infusion the plasma L-DOPA concentration had a drastic reduction and the increase in plasma L-DOPA in relation to the concentration before infusion was 36, 90, 72 and 180% for the doses 0, 0.5, 1 and 2 mg/kg BW, respectively. ()
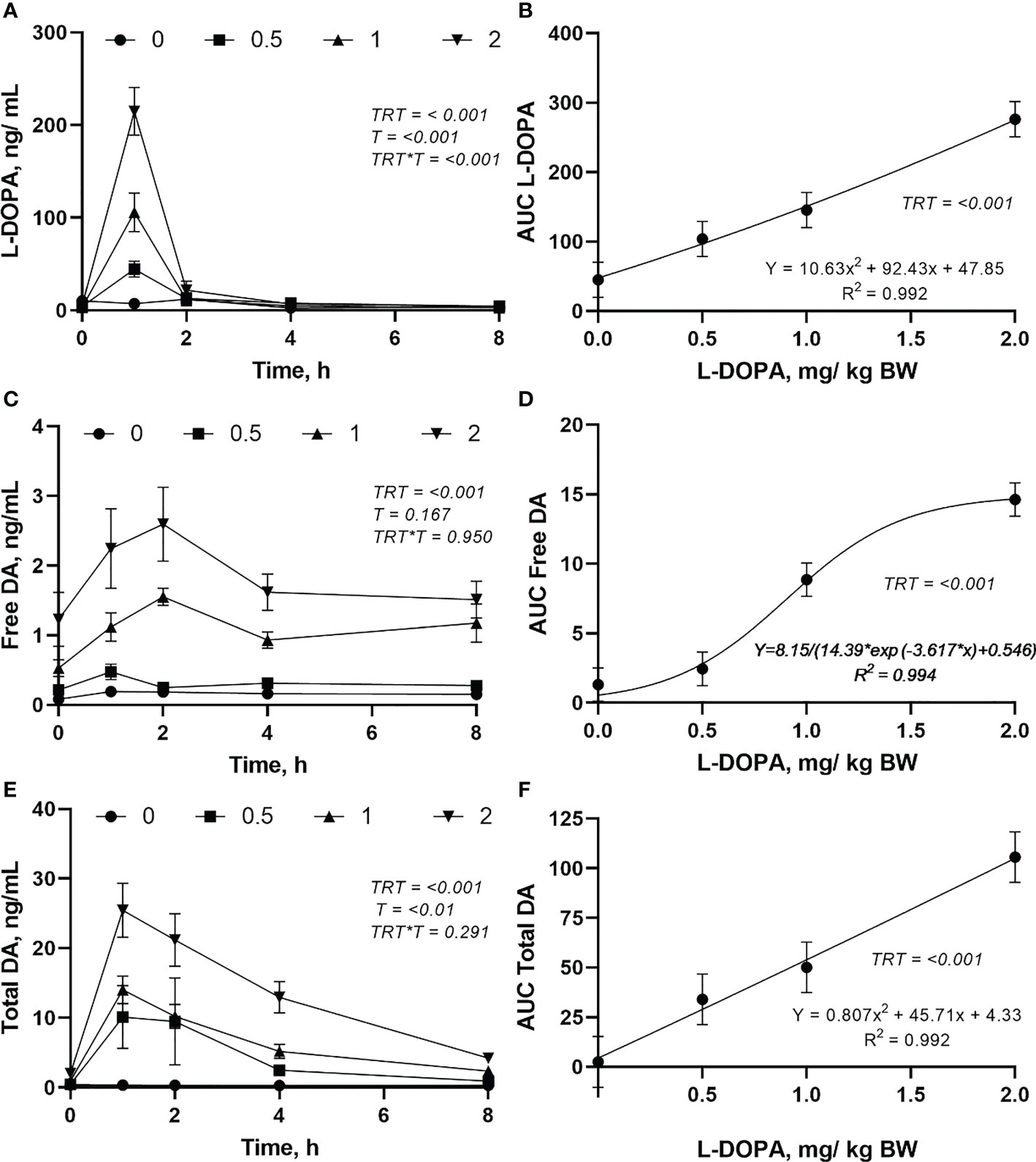
Figure 2 Changes in plasma L-DOPA overtime (A), area under curve (AUC) of L-DOPA (B), free dopamine (DA) over time (C), AUC of DA (D), total DA over time (E) and AUC of total DA (F) following intra-abomasal infusion of L-DOPA at 0, 0.5, 1 and 2 mg/kg BW. TRT is the effect of treatment, T is the time effect and TRT*T is the interaction. Each value is expressed as the mean ± standard error (n = 8).
The circulating concentration of epinephrine and norepinephrine after L-DOPA administration did not change (P > 0.05) (Figures 3A, B). Plasma serotonin increased (P< 0.001) 2 h after feeding and returned to baseline after 4 h (Figure 4A). However, neither plasma or serum serotonin were affected (P > 0.05) by L-DOPA infusion (Figures 4A, B).
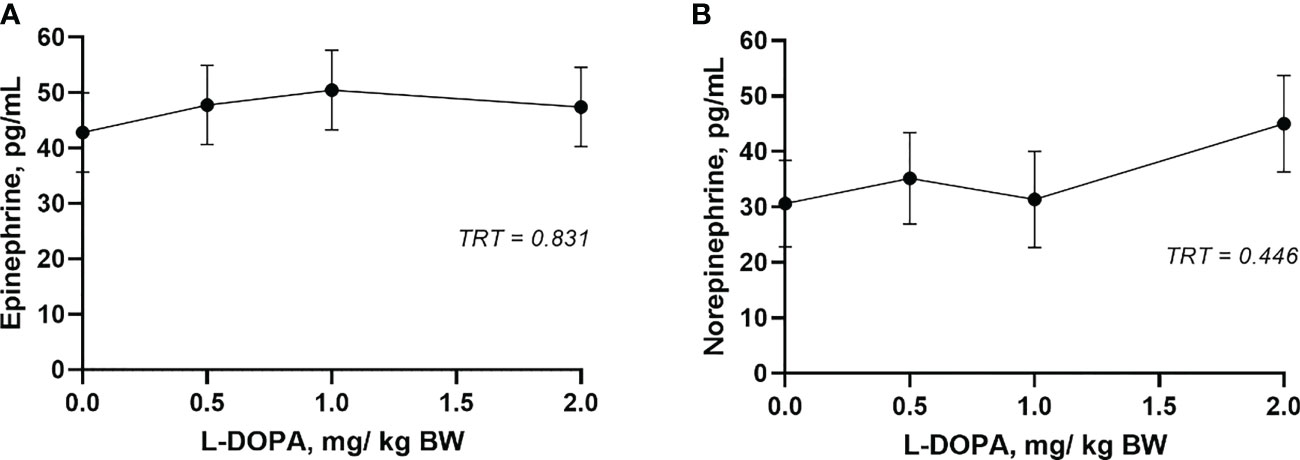
Figure 3 Plasma epinephrine (A) and norepinephrine (B) 4 h after administration of L-DOPA at 0, 0.5, 1 and 2 mg/kg BW. TRT is the effect of treatment. Each value is expressed as the mean ± standard error (n = 8).
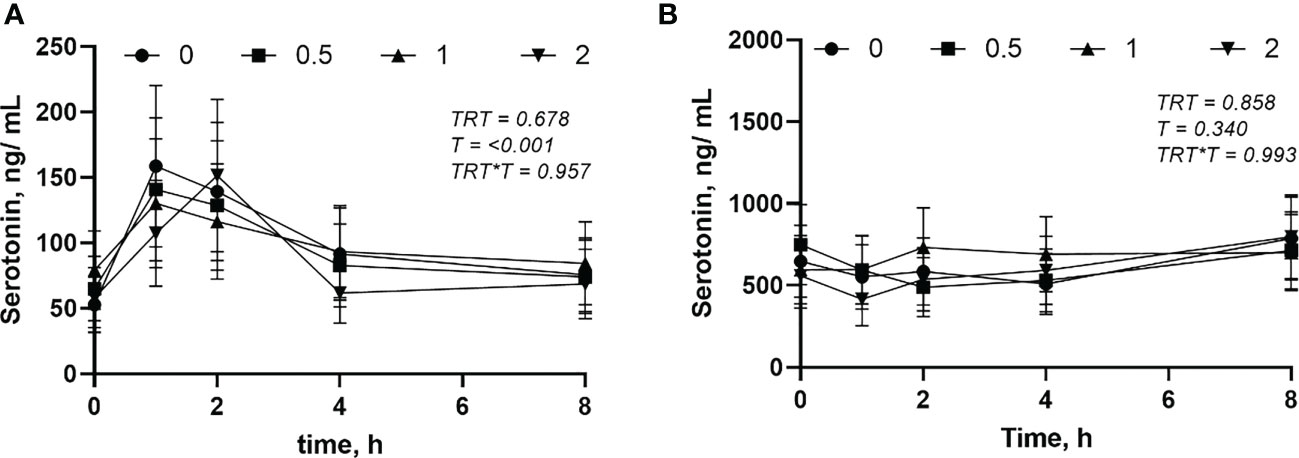
Figure 4 Plasma (A) and serum (B) serotonin after administration of L-DOPA at 0, 0.5, 1 and 2 mg/kg BW. TRT is the effect of treatment, T is the time effect and TRT*T is the interaction. Each value is expressed as the mean ± standard error (n = 8).
The plasma glucose and FFA were not affected (P > 0.05) by the L-DOPA doses (Figures 5A, B). However, the glucose increased (P< 0.001) and FFA decreased (P< 0.001) after feeding.
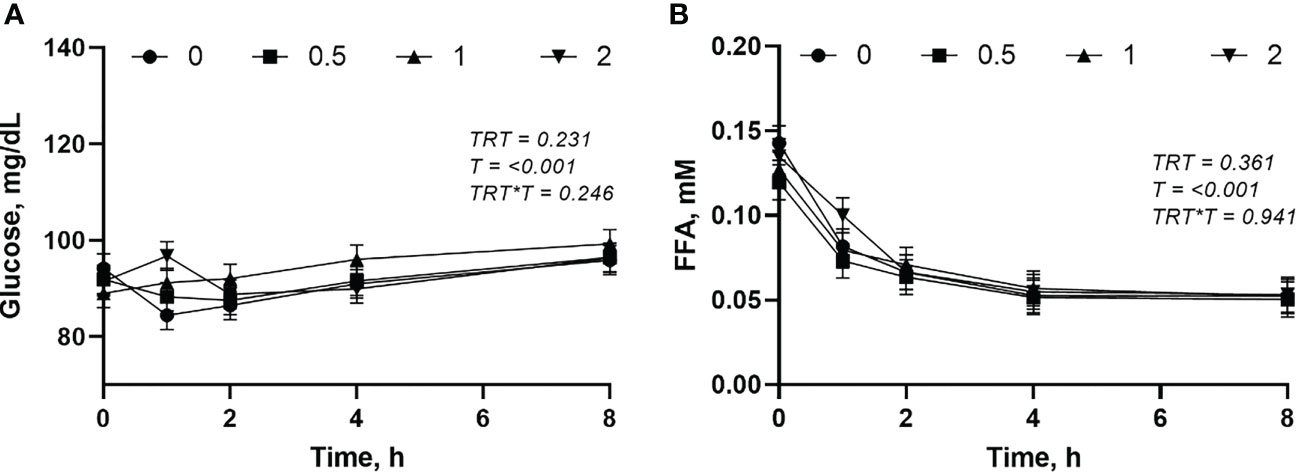
Figure 5 Changes in plasma glucose (A) and free fatty acid (FFA) (B) following intra-abomasal infusion of L-DOPA at 0, 0.5, 1 and 2 mg/kg BW. TRT is the effect of treatment, T is the time effect and TRT*T is the interaction. Each value is expressed as the mean ± standard error (n = 8).
Although there was no interaction between treatment and time (P = 0.150) for urinary L-DOPA, free and total urinary dopamine had an interaction (Figures 6A, C, E). Most of the L-DOPA, free and total dopamine were excreted in the first 4 h after the administration of L-DOPA (Figures 6A, C, E). There was no difference (P > 0.05) between treatments in the urinary excretion of L-DOPA after 4h post-administration of L-DOPA. The excretion of free and total dopamine were similar (P > 0.05) between the doses of L-DOPA after 8 h post-infusion. Daily excretion of L-DOPA, free and total dopamine were increased (P< 0.05) by administration of L-DOPA (Figures 6B, D, F). While urinary L-DOPA and total dopamine responded quadratically (P< 0.05), the free dopamine in urine increased linearly (0.018). The daily urinary dopamine was 10 to 40-fold higher than urinary L-DOPA with the highest ratio at the highest dose.
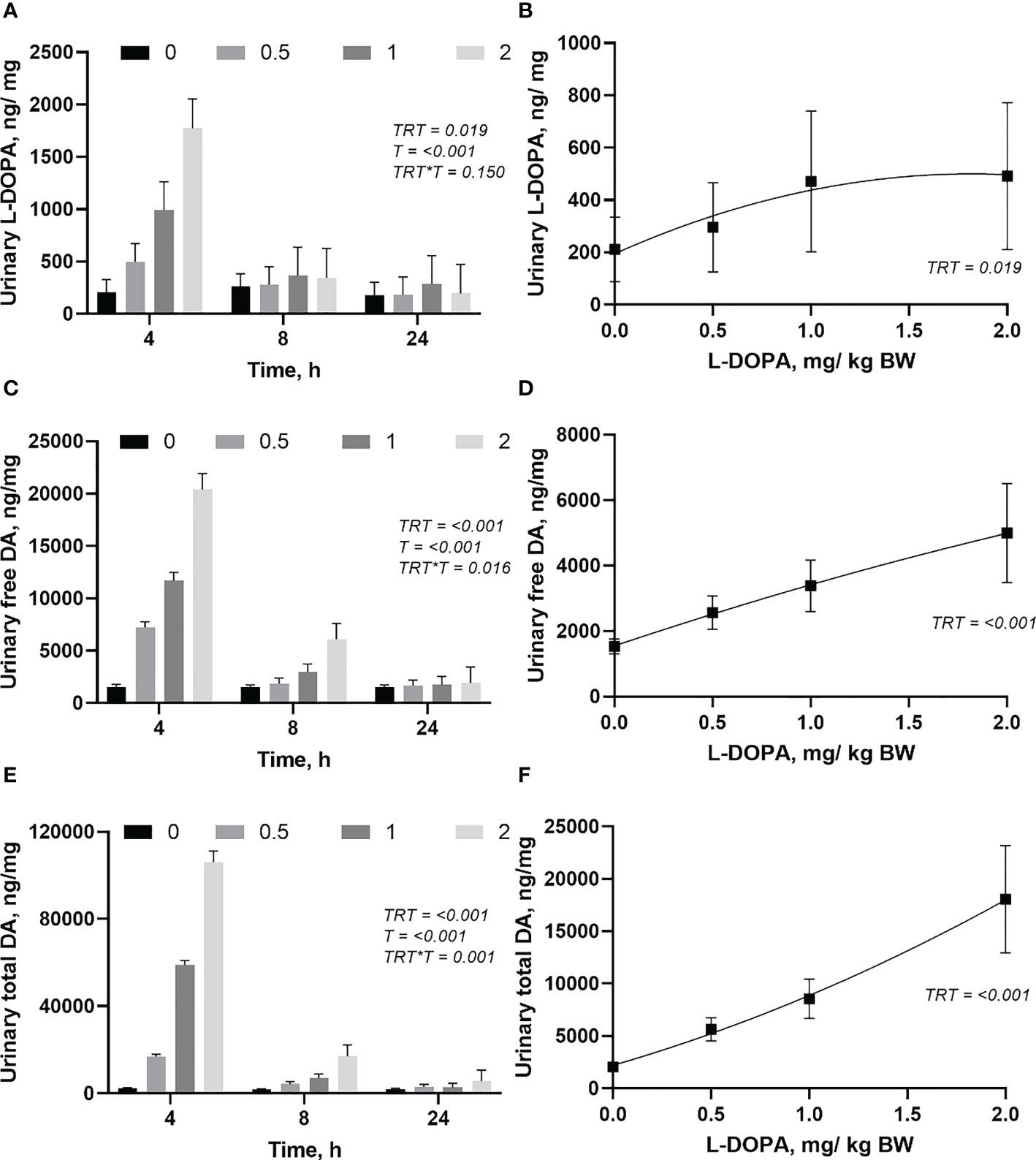
Figure 6 Changes in urinary (ng/mg of creatinine) L-DOPA over time (A) and daily (B), free dopamine (DA) over time (C) and daily (D), and total DA over time (E) and daily (F) following intra-abomasal infusion of L-DOPA at 0, 0.5, 1 and 2 mg/kg BW. TRT is the effect of treatment, T is the time effect and TRT*T is the interaction. Each value is expressed as the mean ± standard error (n = 8). Urinary creatinine was 6.78, 7.21, 6.82 and 7.01 for the 0, 0.5, 1, and 2 L-DOPA doses, respectively.
The DMI was high (over 30 g/kg BW) indicating a high-quality diet and a high palatability of the alfalfa cubes. L-DOPA infusion did not affect (P = 0.125) the daily DMI. There was no effect (P > 0.05) of L-DOPA administration on duration, size or number of meals.
4. Discussion
Dopamine has multiple physiological functions in both central and peripheral systems with most of the actions determined by vesicular exocytosis (Liu and Kaeser, 2019). L-DOPA has been used for several decades as a drug for normalizing brain dopamine and controlling motor symptoms in Parkinson’s disease (Boelens Keun et al., 2021). However, there is a lack of information about its use in livestock production. Although dopamine metabolism has been extensively studied in humans and lab animals, it is not well established how intestinally supplied L-DOPA affects dopamine metabolism in cattle. Many dopamine roles such as feed intake (Mills et al., 2020), energy metabolism (Mietlicki-Baase et al., 2015), immune system (Broome et al., 2020), and behavior (Ginane et al., 2015) could be points to improve animal health and production. This study showed that circulating dopamine may be increased several fold after intra-abomasal infusion of L-DOPA without adverse effects in cattle.
The strong increase in circulating L-DOPA at the first hour after administration followed by a rapid decline in the next hour indicates an efficient absorption and clearance system. The half-life of L-DOPA is about 1 h in humans (Murata, 2006) which is similar to that observed in this study. Tyrosine hydroxylase is the rate-limiting enzyme for catecholamine biosynthesis from tyrosine, and dopamine, epinephrine and norepinephrine are the products of the pathway. This enzyme is used as a feedback mechanism for controlling synthesis of the catecholamine neurotransmitters (Daubner et al., 2011). Conversely, aromatic amino acid decarboxylase is not a rate-limiting enzyme with a wide distribution in the body and it rapidly converts L-DOPA to dopamine, post-absorption (Ayano, 2016). Therefore, the fast disappearance of L-DOPA after absorption, which was evidenced by circulating L-DOPA returning to basal concentration around 2 h after administration, is associated with a rapid synthesis of dopamine by this enzyme. It is thought that dopamine synthesis primarily occurs in dopaminergic neurons, but it also can occur in cells from peripheral tissues (Matt and Gaskill, 2020) increasing circulating dopamine.
The different response between free and total dopamine (plateau vs non-plateau) in the peripheral system can be considered a protective mechanism against overstimulation of the dopaminergic neurons. It seems that free dopamine is allowed to increase rapidly with the availability of L-DOPA until a certain level, afterwards a different control mechanism, e.g., increase of dopamine conjugation, occurs resulting in a plateau phase. This type of dose-dependent response may be associated with differing Km of the enzymes used for dopamine synthesis and conjugation. Most of the circulating dopamine is conjugated (Merbel et al., 2011), which is not the active form. This sequestration of free dopamine is a protective mechanism. For example, dopamine sulfate has a half-life of a few hours, compared to a few minutes for free dopamine (Eldrup, 2004). Taken together, the longer half-life of conjugated dopamine and the fact the conjugation can be reversible (Pellock and Redinbo, 2017), suggests the conjugation of dopamine can prolong the viability of circulating dopamine.
Although the plasma dopamine was consistently increased with administration of L-DOPA, norepinephrine and epinephrine were not affected. Norepinephrine is synthesized from dopamine by the enzyme dopamine β-hydroxylase and can be converted to epinephrine by the enzyme phenylethanolamine N-methyltransferase (Meiser et al., 2013). The results of this study suggest that circulating norepinephrine and epinephrine are closely regulated and independent of plasma dopamine in the bovine. Circulating epinephrine is found in low concentrations and can strongly increase during acute stress (Ziegler et al., 2012; Capellino et al., 2020). The peripheral norepinephrine can communicate with the central nervous system by activating the vagal nerve β-adrenergic receptors (Wong et al., 2012). Because epinephrine is associated with short-term responses to stressors by initiating behavioral and physiological changes that permit an organism to confront the stressful stimulus and overcome it, the classic “fight or flight” mechanism (Wong et al., 2012), the stimulation of this neurotransmitter by a nutraceutical is not desired. Over activation of adrenergic receptors by epinephrine is important to survival in certain occasions. The dopamine has potential to be used to improve feed intake and reduce stress in cattle and the independency between circulating dopamine and epinephrine in bovines can be beneficial for the modulation of dopamine because there is a low risk of undesirable responses on production provoked by epinephrine.
It has been suggested that some serotonin and dopamine systems may act as mutual opponents (Daw et al., 2002). Conversely, dopamine and serotonin can also have joint activity carrying essential reward information (Fischer and Ullsperger, 2017). Administration of high doses of L-DOPA, intra-peritoneally (200 mg/kg) but not orally (250 mg/kg), decreases brain serotonin in rats (Algeri and Cerletti, 1974). The reduction of serotonin after L-DOPA administration observed in some studies with lab animals is believed to be induced by competition with tryptophan for the amino-acid transporter on serotonin neurons, which can also synthesize dopamine, and subsequently for aromatic amino-acid decarboxylase (Stansley and Yamamoto, 2015). The absence of changes in serotonin in this study may be explained by the moderate L-DOPA doses tested (0-2 mg/kg BW). However, to the best of our knowledge, this is the first study that evaluated the interaction of dopamine and serotonin in cattle; therefore, it is not possible infer about possible specie differences. There is a lack of information about the effect of L-DOPA on peripheral serotonin and most of the negative effects on brain serotonin were caused by doses several fold higher than those used in this study.
The direct effect of dopamine on energy metabolism, plasma glucose and free fatty acid content, was not evident in this study. Dopamine was found to modulate endocrine pancreatic hormones and may provoke hyperglycemia in both lab animals and humans (Rubí and Maechler, 2010). It is established in humans that dopamine has direct inhibitory effects on the secretion of the adipogenic hormone insulin and modifies the action of insulin in peripheral tissues and fatty acid metabolism (Rubí and Maechler, 2010). There is direct evidence that dopamine release is stimulated by glucose delivery to the gut (Ren et al., 2010). Moreover, taste and caloric density can increase extracellular dopamine release (Araujo et al., 2012). However, most of those effects are linked to peripheral sensors with the response in the central nervous system and they are associated with food intake. Also, there is not any available data describing how this would be different in a ruminant where little glucose enters the small intestine. Postprandial plasma L-DOPA and dopamine can increase plasma glucose in rats (Korner et al., 2019). Activation of the dopamine D1 receptor–expressing neurons in the nucleus accumbens (region involving in motivation and reward) increases glucose tolerance and insulin sensitivity in mice (Ter Horst et al., 2018). The lack of response of dopamine on glucose may be associated with the pattern of dopamine stimulation which may not evoke a reward stimulus.
The urinary concentration of L-DOPA was much smaller than free and total dopamine indicating that the main pathway to excretion of L-DOPA is through dopamine metabolism. Dopamine disappears from the circulation by oxidative deamination, O-methylation (Matt and Gaskill, 2020) or excretion in urine in the free and conjugated forms (Tuomainen and Männistö, 1997). Most of L-DOPA, free and total dopamine were excreted in the first 4 h indicating a rapid metabolism. The higher concentration of dopamine than L-DOPA (10 to 40-fold) is evidence that the disappearance of L-DOPA in plasma was caused by a rapid metabolism to dopamine rather than excretion.
In the present study, the increase of circulating dopamine did not cause a measurable change in daily DMI or meal distribution throughout the day showing that food intake control is a sophisticated system and it is dependent on several mechanisms rather than only the availability of dopamine in the blood. Dopamine has been shown to be important to feed control, as it has been shown that dopamine-deficient mice die of starvation unless dopamine is restored (Hnasko et al., 2004). In ruminants, feed intake is controlled by many mechanisms including the reward system (Ginane et al., 2015). In humans, eating disorders and obesity are associated with the dopamine role in the central nervous system on the reward system (Opmeer et al., 2010). However, a recent study of eating disorders suggests it is also related to peripheral dopamine levels (Mills et al., 2020).
The results showed that high circulating dopamine did not reduce feed intake or change feeding behavior in cattle. This information should be considered when evaluating L-DOPA as potential nutraceutical because dopamine can act in both stimulatory and inhibitory effects on feed intake. It should be noted that the diet in the present study was highly palatable and feed intake was high perhaps offering a limited chance to see increased intake. Injection of L-DOPA into the perifornical hypothalamus produced a dose-dependent suppression of feeding in hungry rats (Leibowitz and Rossakis, 1979). However, rats eating seeds containing L-DOPA did not change the daily feed intake (Huisden et al., 2019). Although dopamine may be associated with increasing food intake triggered by the reward mechanism, dopamine also has a feedback control on food seeking in both central and peripheral neural systems. It is unclear whether the lack of effect of L-DOPA on feeding behavior occurred because peripheral dopamine does not modulate intake in cattle or whether the pattern of L-DOPA administration did not evoke a reward mechanism on feed intake. Blockade of peripheral dopamine D2 receptors with dopamine antagonist or knockdown of striatal D2 increases food intake in rats (Reinholz et al., 2008; Johnson and Kenny, 2010). Conversely, treatment with the D2-like receptor agonist reduced hyperphagia in rats (Davis et al., 2009) and goats (Kaya et al., 1994). The results suggest that the increase of circulating dopamine did not overstimulate peripheral dopamine D2 receptors and consequently, did not activate negative feedback on feed intake.
The thermoneutral environment and the diet based on alfalfa cubes, a high processed feedstuff with high nutritional quality, used in this study contributed to a dry matter intake possibly close to a maximum potential for growing steers (over 30 g/kg BW). Although the increasing of activity of D1 neurons in the prefrontal cortex increases feed intake in rats through motivated feeding behavior (Land et al., 2014), the stimulus to feed intake triggered by a dopaminergic reward mechanism is expected to be less intense in animals that already have a high feed intake. When the homeostatic needs are attended, the reward mechanisms on feed intake became blunted (Ginane et al., 2015b).
5. Conclusion
Intra-abomasal L-DOPA administration increases circulating dopamine with no corresponding change in feed intake or feeding behavior, circulating serotonin or energy metabolites. L-DOPA absorption and metabolism is rapid with a peak at 1 h after abomasal administration and clearance in the blood after 2 h. However, the dopamine clearance is much slower, and circulating dopamine is increased through the day. Future studies should evaluate the pattern of L-DOPA administration with different diets to trigger the reward mechanism by dopamine and its potential stimulation of feed intake.
Data availability statement
The raw data supporting the conclusions of this article will be made available by the authors, without undue reservation.
Ethics statement
The animal study was reviewed and approved by University of Kentucky IACUC.
Author contributions
EV: Conceptualization, Methodology, Formal analysis, Investigation, Writing - Original Draft. JK: Methodology, Writing - Review & Editing. AE-M: Data analysis, Writing - Review & Editing. GC: Review and Editing. JM: Chemical Analysis, Review and Editing. DH: Conceptualization, Methodology, Formal analysis, Writing, Funding acquisition. All authors contributed to the article and approved the submitted version.
Funding
The authors would like to acknowledge the support of K. Vanzant, B. Cotton, and W. Lin in the conduct of this research. This work is funded by Hatch Capacity Grant Project no. KY007088 from the USDA National Institute of Food and Agriculture and Project 201807121511 from USDA/ARS and the University of Kentucky Agricultural Experiment Station.
Conflict of interest
The authors declare that the research was conducted in the absence of any commercial or financial relationships that could be construed as a potential conflict of interest.
Publisher’s note
All claims expressed in this article are solely those of the authors and do not necessarily represent those of their affiliated organizations, or those of the publisher, the editors and the reviewers. Any product that may be evaluated in this article, or claim that may be made by its manufacturer, is not guaranteed or endorsed by the publisher.
References
Algeri S., Cerletti C. (1974). Effects of l-dopa administration on the serotonergic system in rat brain: Correlation between levels of l-dopa accumulated in the brain and depletion of serotonin and tryptophan. Eur. J. Pharmacol. 27, 191–197. doi: 10.1016/0014-2999(74)90145-9
Andrews Z. B., Erion D., Beiler R., Liu Z.-W., Abizaid A., Zigman J., et al. (2009). Ghrelin promotes and protects nigrostriatal dopamine function via a UCP2-dependent mitochondrial mechanism. J. Neurosci. 29, 14057–14065. doi: 10.1523/JNEUROSCI.3890-09.2009
Araujo I. E., Ferreira J. G., Tellez L. A., Ren X., Yeckel C. W. (2012). The gut–brain dopamine axis: A regulatory system for caloric intake. Physiol. Behav. 106, 394–399. doi: 10.1016/j.physbeh.2012.02.026
Aslanoglou D., Bertera S., Sánchez-Soto M., Benjamin Free R., Lee J., Zong W., et al. (2021). Dopamine regulates pancreatic glucagon and insulin secretion via adrenergic and dopaminergic receptors. Transl. Psychiatry 11, 59. doi: 10.1038/s41398-020-01171-z
Ayano G. (2016). Dopamine: Receptors, functions, synthesis, pathways, locations and mental disorders: Review of literatures. J. Ment. Disord. Treat. 2, 2. doi: 10.4172/2471-271X.1000120
Billes S. K., Simonds S. E., Cowley M. A. (2012). Leptin reduces food intake via a dopamine D2 receptor-dependent mechanism. Mol. Metab. 1, 86–93. doi: 10.1016/j.molmet.2012.07.003
Biskup C. S., Sánchez C. L., Arrant A., van Swearingen A. E. D., Kuhn C., Zepf F. D. (2012). Effects of acute tryptophan depletion on brain serotonin function and concentrations of dopamine and norepinephrine in C57BL/6J and BALB/cJ mice. PloS One 7, e35916. doi: 10.1371/journal.pone.0035916
Boelens Keun J. T., Arnoldussen I. A., Vriend C., van de Rest O. (2021). Dietary approaches to improve efficacy and control side effects of levodopa therapy in parkinson’s disease: A systematic review. Adv. Nutr. 12, 2265–2287. doi: 10.1093/advances/nmab060
Boomsma F., Alberts G., van der Hoorn F. A. J., Man in ‘t Veld A. J., Schalekamp M. A. D. H. (1992). Simultaneous determination of free catecholamines and epinine and estimation of total epinine and dopamine in plasma and urine by high-performance liquid chromatography with fluorimetric detection. J. Chromatogr. 574, 109–117. doi: 10.1016/0378-4347(92)80104-X
Box G. E. P., Cox D. R. (1964). An analysis of transformations. J. R Stat. Soc. Ser. B (Methodological) 26, 211–243. doi: 10.1111/j.2517-6161.1964.tb00553.x
Broome S. T., Louangaphay K., Keay K., Leggio G., Musumeci G., Castorina A. (2020). Dopamine: an immune transmitter. Neural Regen. Res. 15, 2173. doi: 10.4103/1673-5374.284976
Capellino S., Claus M., Watzl C. (2020). Regulation of natural killer cell activity by glucocorticoids, serotonin, dopamine, and epinephrine. Cell Mol. Immunol. 17, 705–711. doi: 10.1038/s41423-020-0477-9
Daubner S. C., Le T., Wang S. (2011). Tyrosine hydroxylase and regulation of dopamine synthesis. Arch. Biochem. .Biophys. 508, 1–12. doi: 10.1016/j.abb.2010.12.017
Davis L. M., Michaelides M., Cheskin L. J., Moran T. H., Aja S., Watkins P. A., et al. (2009). Bromocriptine administration reduces hyperphagia and adiposity and differentially affects dopamine D2 receptor and transporter binding in leptin-Receptor-Deficient zucker rats and rats with diet-induced obesity. Neuroendocrinology 89, 152–162. doi: 10.1159/000170586
Daw N. D., Kakade S., Dayan P. (2002). Opponent interactions between serotonin and dopamine. Neural Netw. 15, 603–616. doi: 10.1016/S0893-6080(02)00052-7
Dayan L. (2003). L-DOPA increases noradrenaline turnover in central and peripheral nervous systems. Neuropharmacology 45, 524–533. doi: 10.1016/S0028-3908(03)00190-4
Dhanani T., Singh R., Shah S., Kumari P., Kumar S. (2015). Comparison of green extraction methods with conventional extraction method for extract yield, l-DOPA concentration and antioxidant activity of Mucuna pruriens seed. Green Chem. Lett. Ver. 8, 43–48. doi: 10.1080/17518253.2015.1075070
Egert-McLean A. M., Sama M. P., Klotz J. L., McLeod K. R., Kristensen N. B., Harmon D. L. (2019). Automated system for characterizing short-term feeding behavior and real-time forestomach motility in cattle. Comput. Electron. Agric. 167, 105037. doi: 10.1016/j.compag.2019.105037
Eldrup E. (2004). Significance and origin of DOPA, DOPAC, and dopamine-sulphate in plasma, tissues and cerebrospinal fluid. Dan. Med. Bull. 1, 34–62.
Fernandes A. B., Alves da Silva J., Almeida J., Cui G., Gerfen C. R., Costa R. M., et al. (2020). Postingestive modulation of food seeking depends on vagus-mediated dopamine neuron activity. Neuron 106, 778–788.e6. doi: 10.1016/j.neuron.2020.03.009
Fischer A. G., Ullsperger M. (2017). An update on the role of serotonin and its interplay with dopamine for reward. Front. Hum. Neurosci. 11. doi: 10.3389/fnhum.2017.00484
Frank G. K., Bailer U. F., Henry S. E., Drevets W., Meltzer C. C., Price J. C., et al. (2005). Increased dopamine D2/D3 receptor binding after recovery from anorexia nervosa measured by positron emission tomography and [11C]Raclopride. Biol. Psychiatry 58, 908–912. doi: 10.1016/j.biopsych.2005.05.003
García-Tornadú I., Ornstein A. M., Chamson-Reig A., Wheeler M. B., Hill D. J., Arany E., et al. (2010). Disruption of the dopamine D2 receptor impairs insulin secretion and causes glucose intolerance. Endocrinology 151, 1441–1450. doi: 10.1210/en.2009-0996
Ginane C., Bonnet M., Baumont R., Revell D. K. (2015). Feeding behaviour in ruminants: a consequence of interactions between a reward system and the regulation of metabolic homeostasis. Anim. Prod. Sci. 55, 247. doi: 10.1071/AN14481
González-Grajales L. A., Pieper L., Mengel S., Staufenbiel R. (2018). Evaluation of glucose dose on intravenous glucose tolerance test traits in Holstein-friesian heifers. J. Dairy. Sci. 101, 774–782. doi: 10.3168/jds.2017-13215
González-Sepúlveda M., Omar M. Y., Hamdon S., Ma G., Rosell-Vilar S., Raivio N., et al (2022). Spontaneous changes in brain striatal dopamine synthesis and storage dynamics ex vivo reveal end-product feedback-inhibition of tyrosine hydroxylase. Neuropharmacology 212, 109058. doi: 10.1016/j.neuropharm.2022.109058
Hajnal A., Smith G. P., Norgren R. (2004). Oral sucrose stimulation increases accumbens dopamine in the rat. Am. J. Physiol. Regul. Integr. Comp. Physiol. 286, R31–R37. doi: 10.1152/ajpregu.00282.2003
Hnasko T. S., Szczypka M. S., Alaynick W. A., During M. J., Palmiter R. D. (2004). A role for dopamine in feeding responses produced by orexigenic agents. Brain. Res. 1023, 309–318. doi: 10.1016/j.brainres.2004.07.051
Horst K. W. T., Lammers N. M., Trinko R., Opland D. M., Figee M., Ackermans M. T., et al. (2018). Striatal dopamine regulates systemic glucose metabolism in humans and mice. Sci. Transl. Med. 10. doi: 10.1126/scitranslmed.aar3752
Huisden C. M., Butterweck V., Szabo N. J., Gaskind J. M., Arriola K. G., Raji A., et al. (2019). Effects of detoxification of mucuna pruriens on the feed intake, behavior, organ weights, blood cell counts and metabolites of rats. Trop. Subtrop. Agroecosyst. 22, 379–389.
Jennings K. A. (2013). A comparison of the subsecond dynamics of neurotransmission of dopamine and serotonin. ACS Chem. Neurosci. 4, 704–714. doi: 10.1021/cn4000605
Johnson P. M., Kenny P. J. (2010). Dopamine D2 receptors in addictionlike reward dysfunction and compulsive eating in obese rats. Nat. Neurosci. 13, 635–641. doi: 10.1038/nn.2519
Joshi A., Schott M., la Fleur S. E., Barrot M. (2022). Role of the striatal dopamine, GABA and opioid systems in mediating feeding and fat intake. Neurosci. Biobehav. Rev. 139, 104726. doi: 10.1016/j.neubiorev.2022.104726
Kasuya E., Sutoh M., Yayou K. (2017). The effects of l-DOPA and sulpiride on growth hormone secretion at different injection times in Holstein steers. Anim.Sci. J. 88, 1842–1848. doi: 10.1111/asj.12850
Kaya F., Van Duin C. T. M., Van Miert A. S. (1994). Effects of dopamine receptor agonists on food intake and rumen motility in dwarf goats. J. Vet. Pharmacol. Ther. 17, 120–126. doi: 10.1111/j.1365-2885.1994.tb00221.x
Klein M. O., Battagello D. S., Cardoso A. R., Hauser D. N., Bittencourt J. C., Correa R. G. (2019). Dopamine: Functions, signaling, and association with neurological diseases. Cell Mol. Neurobiol. 39, 31–59. doi: 10.1007/s10571-018-0632-3
Korner J., Cline G. W., Slifstein M., Barba P., Rayat G. R., Febres G., et al. (2019). A role for foregut tyrosine metabolism in glucose tolerance. Mol. Metab. 23, 37–50. doi: 10.1016/j.molmet.2019.02.008
Kuo D.-Y. (2002). Co-Administration of dopamine D1 and D2 agonists additively decreases daily food intake, body weight and hypothalamic neuropeptide y level in rats. J. Biomed. Sci. 9, 126–132. doi: 10.1159/000048208
Land B. B., Narayanan N. S., Liu R.-J., Gianessi C. A., Brayton C. E., M Grimaldi D., et al. (2014). Medial prefrontal D1 dopamine neurons control food intake. Nat. Neurosci. 17, 248–253. doi: 10.1038/nn.3625
Leibowitz S. F., Rossakis C. (1979). L-dopa feeding suppression: Effect on catecholamine neurons of the perifornical lateral hypothalamus. Psychopharmacol. (Berl) 61, 273–280. doi: 10.1007/BF00432272
Lenka A., di Maria G., Lamotte G., Bahroo L., Jankovic J. (2022). Practical pearls to improve the efficacy and tolerability of levodopa in parkinson’s disease. Expert. Rev. Neurother. 22, 489–498. doi: 10.1080/14737175.2022.2091436
Liu C., Kaeser P. S. (2019). Mechanisms and regulation of dopamine release. Curr. Opin. Neurobiol. 57, 46–53. doi: 10.1016/j.conb.2019.01.001
Liu J., Perez S. M., Zhang W., Lodge D. J., Lu X.-Y. (2011). Selective deletion of the leptin receptor in dopamine neurons produces anxiogenic-like behavior and increases dopaminergic activity in amygdala. Mol. Psychiatry 16, 1024–1038. doi: 10.1038/mp.2011.36
Madzimure J., Mutema N., Chimonyo M., Bakare A. G., Mapiye C. (2014). Performance of mashona doelings supplemented with different levels of velvet bean (Mucuna pruriens l. DC. var. utilis) seed meal. Trop. Anim. Health Prod. 46, 901–904. doi: 10.1007/s11250-014-0578-5
Matt S. M., Gaskill P. J. (2020). Where is dopamine and how do immune cells see it?: dopamine-mediated immune cell function in health and disease. J. Neuroimmune Pharmacol. 15, 114–164. doi: 10.1007/s11481-019-09851-4
Meiser J., Weindl D., Hiller K. (2013). Complexity of dopamine metabolism. Cell Commun.and Signal. 11, 34. doi: 10.1186/1478-811X-11-34
Melkersson K., Jansson E. (2007). Effects of the atypical antipsychotic clozapine on insulin release in vitro. neuro. Endocrinol. Lett. 28, 854–860.
Merbel N. C., Hendriks G., Imbos R., Tuunainen J., Rouru J., Nikkanen H. (2011). Quantitative determination of free and total dopamine in human plasma by LC–MS/MS: the importance of sample preparation. Bioanalysis 3, 1949–1961. doi: 10.4155/bio.11.170
Mietlicki-Baase E. G., Reiner D. J., Cone J. J., Olivos D. R., McGrath L. E., Zimmer D. J., et al. (2015). Amylin modulates the mesolimbic dopamine system to control energy balance. Neuropsychopharmacology 40, 372–385. doi: 10.1038/npp.2014.180
Mills J. G., Thomas S. J., Larkin T. A., Deng C. (2020). Overeating and food addiction in major depressive disorder: Links to peripheral dopamine. Appetite 148, 104586. doi: 10.1016/j.appet.2020.104586
Morales I., Berridge K. C. (2020). ‘Liking’ and ‘wanting’ in eating and food reward: Brain mechanisms and clinical implications. Physiol. Behav. 227, 113152. doi: 10.1016/j.physbeh.2020.113152
Müller S. J., Teckentrup V., Rebollo I., Hallschmid M., Kroemer N. B. (2022). Vagus nerve stimulation increases stomach-brain coupling via a vagal afferent pathway. Brain Stimul. 15, 1279–1289. doi: 10.1016/j.brs.2022.08.019
Murata M. (2006). Pharmacokinetics of l-dopa. J. Neurol. 253, iii47–iii52. doi: 10.1007/s00415-006-3009-3
Omrani A., de Vrind V. A. J., Lodder B., Stoltenborg I., Kooij K., Wolterink-Donselaar I. G., et al. (2021). Identification of novel neurocircuitry through which leptin targets multiple inputs to the dopamine system to reduce food reward seeking. Biol. Psychiatry 90, 843–852. doi: 10.1016/j.biopsych.2021.02.017
Opmeer E. M., Kortekaas R., Aleman A. (2010). Depression and the role of genes involved in dopamine metabolism and signalling. Prog. Neurobiol. 92, 112–133. doi: 10.1016/j.pneurobio.2010.06.003
Ott T., Nieder A. (2019). Dopamine and cognitive control in prefrontal cortex. Trends Cogn. Sci. 23, 213–234. doi: 10.1016/j.tics.2018.12.006
Pellock S. J., Redinbo M. R. (2017). Glucuronides in the gut: Sugar-driven symbioses between microbe and host. J.Biol. Chem. 292, 8569–8576. doi: 10.1074/jbc.R116.767434
Peniche-Gonzalez I. N., Sarmiento-Franco L. A., Santos-Ricalde R. H. (2018). Utilization of mucuna pruriens whole pods to feed lactating hair ewes. Trop. Anim. Health Prod. 50, 1455–1461. doi: 10.1007/s11250-018-1580-0
Reinholz J., Skopp O., Breitenstein C., Bohr I., Winterhoff H., Knecht S. (2008). Compensatory weight gain due to dopaminergic hypofunction: new evidence and own incidental observations. Nutr. Metab. (Lond) 5, 35. doi: 10.1186/1743-7075-5-35
Ren X., Ferreira J. G., Zhou L., Shammah-Lagnado S. J., Yeckel C. W., de Araujo I. E. (2010). Nutrient selection in the absence of taste receptor signaling. J. Neurosci. 30, 8012–8023. doi: 10.1523/JNEUROSCI.5749-09.2010
Rubí B., Maechler P. (2010). Minireview: New roles for peripheral dopamine on metabolic control and tumor growth: Let’s seek the balance. Endocrinology 151, 5570–5581. doi: 10.1210/en.2010-0745
Shapiro S. S., Wilk M. B. (1965). An analysis of variance test for normality (Complete samples). Biometrika 52, 591. doi: 10.2307/2333709
Stansley B., Yamamoto B. (2015). L-dopa and brain serotonin system dysfunction. Toxics 3, 75–88. doi: 10.3390/toxics3010075
Szczypka M. S., Rainey M. A., Palmiter R. D. (2000). Dopamine is required for hyperphagia in lepob/ob mice. Nat. Genet. 25, 102–104. doi: 10.1038/75484
Tuomainen P., Männistö P. T. (1997). Norepinephrine (NE), epinephrine (E), and dopamine (DA) are catecholamines (CAs) that play important roles as neurotransmitters or hormones, and they are excreted in urine in free and conjugated forms. J. Clin. Chem. Clin. Biochem. 35, 229–235.
Vadivel V., Pugalenthi M. (2010). Studies on the incorporation of velvet bean (Mucuna pruriens var. utilis) as an alternative protein source in poultry feed and its effect on growth performance of broiler chickens. Trop. Anim. Health Prod. 42, 1367–1376. doi: 10.1007/s11250-010-9594-2
Valente E. E. L., Damasceno M. L., Klotz J. L., Harmon D. L. (2021). Residual effects of abomasal 5-hydroxytryptophan administration on serotonin metabolism in cattle. Domest. Anim. Endocrinol. 76, 106627. doi: 10.1016/j.domaniend.2021.106627
Volkow N. D., Wise R. A., Baler R. (2017). The dopamine motive system: implications for drug and food addiction. Nat. Rev. Neurosci. 18, 741–752. doi: 10.1038/nrn.2017.130
Wallace C. W., Fordahl S. C. (2022). Obesity and dietary fat influence dopamine neurotransmission: Exploring the convergence of metabolic state, physiological stress, and inflammation on dopaminergic control of food intake. Nutr. Res. Rev. 35, 236–251. doi: 10.1017/S0954422421000196
Wong D. L., Tai T. C., Wong-Faull D. C., Claycomb R., Meloni E. G., Myers K. M., et al. (2012). Epinephrine: A short- and long-term regulator of stress and development of illness. Cell Mol. Neurobiol. 32, 737–748. doi: 10.1007/s10571-011-9768-0
Keywords: dopamine, levodopa, metabolism, neurotransmitter, serotonin
Citation: Valente EEL, Klotz JL, Egert-McLean AM, Costa GW, May JB and Harmon DL (2023) Influence of intra-abomasal administration of L-DOPA on circulating catecholamines and feed intake in cattle. Front. Anim. Sci. 4:1127575. doi: 10.3389/fanim.2023.1127575
Received: 19 December 2022; Accepted: 31 January 2023;
Published: 15 February 2023.
Edited by:
Anna Katharine Shoveller, University of Guelph, CanadaReviewed by:
Hatem Eltahan, Agricultural Research Center, EgyptAnna Wessels, Freie Universität Berlin, Germany
Copyright © 2023 Valente, Klotz, Egert-McLean, Costa, May and Harmon. This is an open-access article distributed under the terms of the Creative Commons Attribution License (CC BY). The use, distribution or reproduction in other forums is permitted, provided the original author(s) and the copyright owner(s) are credited and that the original publication in this journal is cited, in accordance with accepted academic practice. No use, distribution or reproduction is permitted which does not comply with these terms.
*Correspondence: David L. Harmon, ZGF2aWQuaGFybW9uQHVreS5lZHU=
†USDA is an equal opportunity provider and employer