- 1Centro de Investigación Tecnológica de Agua en el Desierto (CEITSAZA), Universidad Católica del Norte, Antofagasta, Chile
- 2Departamento de Química, Universidad Católica del Norte, Antofagasta, Chile
- 3Departamento de Ingeniería Química, Universidad Católica del Norte, Antofagasta, Chile
Agricultural wastes are considered as green adsorbents that can work as an alternative to recover critical and scarce metals from secondary sources. Critical elements as rare-earth elements (REEs) can be obtained from electronic wastes or tailings and could be recovered using these green alternatives. In this study, walnut shell (WS) was tested to determine whether several REEs can be efficiently retained by this green adsorbent. Fourier transform infrared spectroscopy (FTIR), scanning electron microscopy (SEM), thermogravimetry, and differential scanning calorimetry (TG-DSC) were used to characterize WS before and after REE adsorption. Analytical performance of REE quantification was evaluated, besides adsorption capacity and isotherms that were calculated in order to determine the model that fit well to REE adsorption. ICP-OES results indicated the lowest limit of detection and quantification (LOD and LOQ) with Eu (0.08 and 0.23 ppb, respectively); nevertheless, quantification of other elements was also at the ppb level. In order to obtain the highest adsorption of metals, 75- and 2,000-μm particle sizes were studied, reaching >80% of adsorption with both sizes. Additionally, several pH values were tested in order to determine the optimum condition for maximal adsorption and adsorption capacity, noticing that pH 4 showed the best adsorption percentage (>85%, qe = 6.5–8 mg/g). The Langmuir isotherm model fitted well for the Eu, La, Sm, and Gd adsorption equilibrium. Characterization of WS was done using FTIR, TG-DSC, and SEM. FTIR analysis showed several changes in the spectra after adsorption of REE tested, but major changes were observed at the OH group, which shifted up to 31 cm−1 of wavelength. Additionally, TG-DSC showed that WS pyrolysis was divided in three stages: vaporization of moisture (about 10% of weight loss); thermal decomposition of hemicellulose, cellulose, and lignin (higher than 60% of weight loss); and high-temperature calcination of residues (<25%). Finally, SEM characterization showed empty and filled pores of different sizes in WS after metal adsorption, and a more rugged aspect was observed. This study reveals that WS is an efficient low-cost adsorbent for REEs and can be used for future recovery of these elements from secondary sources.
Introduction
The rare-earth elements (REEs) consist of lanthanum, scandium, yttrium, and fourteen other REEs. REEs are known for their exceptional chemical, catalytic, electrical, magnetic, and optical characteristics, which have received particular attention due to their industrial applications in electronics, superconductors, and catalysts, among others. In addition, REEs have been shown to promote the growth of plants and the body weight gain of animals; therefore, agricultural and animal production industries are also interested in these elements (Hu et al., 2006; Xu and Wang, 2007; Abdelnour et al., 2019; Agathokleous et al., 2019). Due to the transition to a green, low-carbon economy, REEs are considered the most critical raw material groups with the highest supply risk, as explained by the European commission in 2010, which is reviewed in Binnemans et al. (2013). Alternative methods are necessary to recover REEs from aqueous solutions, either from lixiviation of electronic waste or from mining tailing.
The biosorption process involves a solid phase (sorbent or biosorbent) and a liquid phase containing a dissolved species to be sorbed (metal ions). Biosorption is a fast and reversible reaction used commonly for heavy metal recovery (Tripathi and Rawat Ranjan, 2015). Several materials have been used to recover heavy metals, such as peat, lignite and humic acids, fly ash, microbial biomass, and agricultural by-products such as soya bean hulls, walnut shells, and others (Laszlo and Dintzis, 1994; Alvarez Puebla et al., 2006; Pehlivan and Altun, 2008; Pehlivan et al., 2009; Módenes et al., 2015; Suresh Kumar et al., 2015; Darmayanti et al., 2017; Cheng et al., 2018; Ennoukh et al., 2019; Neris et al., 2019). The advantages of using a low-cost bioadsorbent are high efficiency at low cost, minimization of chemical and/or biological sludge regeneration of the biosorbent, and the possibility of metal recovery (Dai et al., 2018).
Walnut shells (WS) are abundant agricultural residues with great stability, wide specific surface area, and high mechanical strength, and they have been used to recover mainly heavy metals (Banerjee et al., 2018) but also some pharmaceutical contaminants and pesticides (Zuhra Memon et al., 2014; Ahmed and Hameed, 2018; Bayat et al., 2018). This raw material is mainly composed of lignin and polysaccharides, specifically 10.6% extractives (dichloromethane, ethanol and water), 29.9% lignin (Klason lignin, soluble lignin), 49.7% polysaccharides, and mineral composition (Zhu et al., 2016; Queirós et al., 2020). Moreover, the reuse or regeneration of WS has been studied mainly in the adsorption of heavy metal and organic compounds (such a naphthalene, oils, among others), showing that sequential biosorption–desorption cycles ranged approximately from 1 to 6 (Altun and Pehlivan, 2012; Zhu et al., 2016; Banerjee et al., 2018). So far, a single article showed the reuse of WS for REE adsorption, indicating that it is possible to use the adsorbent about 1,000 times (Li et al., 2012). Then, the adsorption of REEs onto WS has been poorly studied; therefore, this study aims to determine the adsorption capacity of natural WS at different particle sizes to retain several REEs under different pH solutions, in order to evaluate its performance for future applications to recover these elements either from electronic waste or from mine tailings.
Materials and Methods
Adsorbent and Chemical Reagents
All solutions were prepared from analytical-grade chemicals purchased from Sigma-Aldrich and Merck and deionized water. Stock solutions with a concentration of 1 mM of rare-earth elements (REEs), including La+3, Sm+3, Eu+3, Gd+3, and Nd+3, were prepared by dissolving a known amount of La(NO3)3, Sm(NO3)3, Eu(NO3)3, Gd(NO3)3, and Nd(NO3)3 in deionized water. From the stock solution, working solutions of 200 μg L−1 REEs were prepared by serial dilution immediately prior to their use. These solutions were pH adjusted from 3 to 7 with 1 N NaOH or HNO3. The pH of the solutions was determined using a digital pH meter.
For the sorbent material, residues of WS were used and acquired from the city of Illapel located in the IV region of Coquimbo in Chile. WS was ground in a disk mill, and the resulting crumbs were sieved to obtain particle sizes of 2,000 and 75 μm. Before use, all materials were washed thoroughly with deionized water and oven dried at 105°C for 24 h. Dried WS was stored in a desiccator until use.
Batch Adsorption Experiments
Three independent replicates for batch adsorption experiments were carried out at several pH values in 50-mL canonical flasks to examine the adsorption of REEs in WS, in order to determine the precision of experiments by calculating standard deviation. Briefly, 20 mL of 200-ppb REEs adjusted previously at pH values of 3, 4, 5.5, and 7 was mixed with 500 mg of WS. Two different particle sizes of 2,000 and 75 μm were used to identify the particle size effect in the adsorption of REEs. Thereafter, flasks were shook at 120 rpm at a constant temperature of 20°C for 12 h. After incubation, tubes containing the samples were centrifuged at 4,000 rpm for 5 min and filtered first with 1-μm filter paper (Microlab Scientific Inc.) followed by a second round of filtration using 0.45-μm filter paper (Microlab Scientific Inc.). MCE (mixed cellulose esters) filters were used for both filtration processes due to that it was shown to have the best performance in adsorption of metals, between 1 and 5% at the ppb and ppm level (Darren et al., 2014).
Quantification of REEs by ICP-OES
A multielement calibration curve for the five REEs was obtained using Perkin Elmer Optima 7,000 DV model inductively coupled plasma optical emission spectrometry (ICP-OES). Standards of 10, 25, 50, 100, 250, and 500 ppb were prepared immediately prior to their use. The wavelengths used for the La, Nd, Eu, Gd, and Sm elements were 398.852, 430.358, 381.967, 342.247, and 359.260 nm, respectively. Three analytical replicates were carried out. The REE removal efficiency and adsorption capacity of WS at different pH values was calculated according to previous works (Yi et al., 2015; Çelebi and Gök, 2017) using the following equations:
where Ad% is the REE removal efficiency and qe is the adsorption capacity. Ci and Ce are the initial REE and residual concentrations, respectively. V is the volume of the aqueous solution, and m is the mass of the WS used.
Adsorption Isotherms
The sorption isotherms for the REEs on WS were studied using the abovementioned batch technique. Two different particle sizes for the sorbents were allowed to equilibrate in independent experiments with solutions of 200 ppb La, Nd, Sm, Gd, and Eu at pH values of 3, 4, 5.5, and 7. Considering the adsorption capacity (Equation 2), sorption isotherms were described by Freundlich, Langmuir and Temkin isotherm models, which are represented in Equations (3–5), respectively, as shown in Table 1.
Characterization of Walnut Shell
To study the surface chemistry and morphology changes of WS before and after adsorption of REEs, Fourier-transform infrared (FT-IR) spectroscopy analysis was used as well as thermogravimetric and differential scanning calorimetric (TG-DSC) analysis and scanning electron microscopy (SEM).
FT-IR analysis (Perkin Elmer Spectrum 100 spectrometer) was used for identifying the functional groups on the surface that were present in the adsorbent and involved in the adsorption process of the raw material (WS) and WS containing REEs (WS-REEs). Bare WS and WS-REEs were oven dried at 30°C, and analysis of their active functional groups was carried out over the wavenumber region of 700 to 4,000 cm−1 using a KBr translucent disc.
The surface morphology of WS and WS-REEs was investigated using a field-emission scanning electron microscope (SEM) FE-SEM SU5000 Hitachi. The thermal behavior of 10 mg of WS and WS-REEs at several pH values was studied by means of thermal gravimetric analysis and differential scanning calorimetry, TGA-DSC (Netzsch Jupiter, heating and cooling rates 20 K min−1, purge gas flow: N2 50 mL min−1), in the temperature range of 20–900°C.
Results and Discussion
Analytical Performance of ICP-OES for REE Quantification
The calibration curve for all five REEs was obtained at a higher linearity of >0.98 (data not shown). Limits of detection and quantification (LOD and LOQ) were calculated as 3.3 σ/slope and 10 σ/slope, respectively (Shrivastava and Gupta, 2011). LOD and LOQ were determined to be 0.38 and 1.16 ppb for La, 1.61 and 4.90 ppb for Nd, 0.08 and 0.23 ppb for Eu, 8.2 and 24.7 ppb for Gd, and 13.4 and 40.5 ppb for Sm, respectively.
Adsorption Capacity of Natural WS
The pH adsorption is a significant parameter for the evaluation of adsorption performance (Çelebi and Gök, 2017). The adsorption was studied as a function of pH ranging from 3 to 7 by adjusting with HNO3 and NaOH, as required. Higher pH values were not studied since REE ions would majorly exist in the form of precipitate (Li et al., 2012). Lower pH values were also not analyzed, since functional groups of WS are vulnerable to be protonated triggering REE-ion repulsion by electrostatic interactions (Li et al., 2012). Sorbents were allowed to equilibrate with 200 ppb for 12 h at the abovementioned pH. After centrifugation and filtration, the supernatant was analyzed by ICP-OES to quantify the residual REEs. Removal (% adsorption) and adsorption capacity (qe in mg/g) were higher at pH 4 in most of the REEs (Figure 1 and Table S1), and only lanthanum showed slightly higher adsorption at pH 5.5 (Figure 1D). The adsorption at pH 4 and a 75-μm particle size was 87% for Eu, 84% for Gd, 93% for Sm, and 85% for La, while at a 2,000-μm particle size, the adsorption of REEs at the same pH was 89% for Eu, 97% for Gd, 99% for Sm, and 85% for La (Figure 1). The high adsorption might be explained by the high carbon content of walnut shell compared to O, H, and N, which according to previous reports is composed by more than 50% of C (Wang et al., 2010; Zabihi et al., 2010; Ghasemi et al., 2015). In some studies, it was reported the use of commercial activated carbon (CAC) as an adsorbent that is effective in heavy metal removal was reported, showing an adsorption efficiency of ~90% Cd and Cr removal (Babel and Kurniawan, 2004; Hydari et al., 2012). Although CAC is an efficient adsorbent, it has the disadvantage to be a high-cost and difficult regeneration procedure, becoming a limited method (Babel and Kurniawan, 2003). For that reason, chemical and physical activations of natural adsorbents have been done for some researchers as a cost-effective alternative. For instance, it was reported that chemical activation of adsorbents (such as sunflower, potato, canola, and walnut shell residues) with NaOH improved Ni adsorption, indicating that WS capacity was about 8 mg/g (Feizi and Jalali, 2015). Additionally, activation of WS with ZnCl2 to remove Hg(II) was shown, reaching a removal efficiency between 70 and 90% of adsorption percentage (Zabihi et al., 2009). However, and as explained before, in this article the uptake of REEs by natural WS was investigated in order to use this data for future work, reaching similar removal efficiency values to activated adsorbents (mentioned above). An additional work showed similar results, where WS packed microcolumn was described to retain 15 REEs at a pH value of 3.2; however, the removal efficiency was not reported (Li et al., 2012). So far, few studies have been reported regarding REE adsorption by WS; however, investigations of heavy metal removal by carbonaceous materials such as WS are more common. For instance, removal of Pb(II) by natural walnut shell was optimum at pH 5.5 with 90% adsorption (Yi et al., 2015) and for Cr(VI) at pH 2 with more than 75% adsorption. Therefore, according to our results, this low-cost material shows high capacity to retain, among other metals, REEs at pH 4. As shown in Table S1, both particle sizes improved the adsorption of the REEs, since there is enough surface area for the interaction of metals. A few reported results show that higher particle size shows lower adsorption of Ni(II); however, these studies used particle sizes much larger than those in our study. For instance, a particle size of 900 μm showed an adsorption capacity of 5.7 mg/g, while a particle size of 1,800 μm showed an adsorption capacity of 3.9 mg/g (Demirbaş et al., 2002).
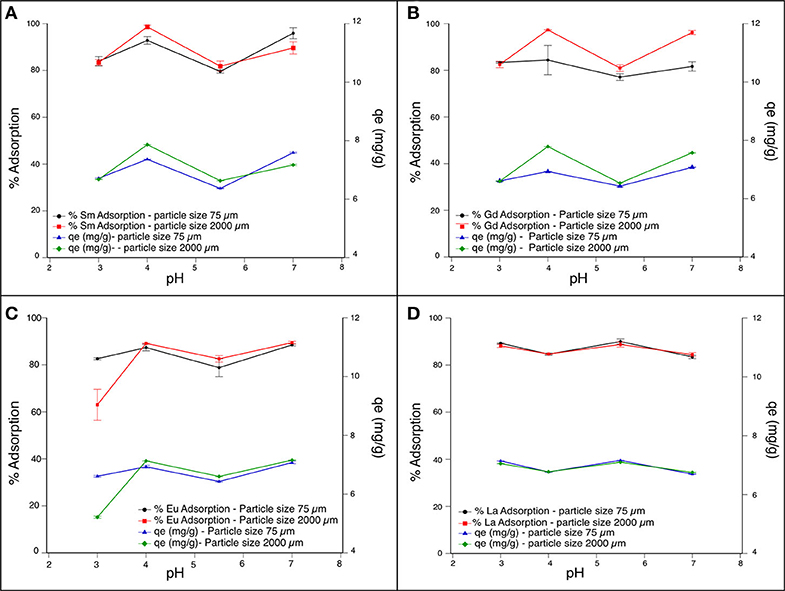
Figure 1. Effect of pH in the rare-earth removal efficiency and adsorption capacity using two particle size. (A) Sm (III); (B) Gd (III); (C) Eu (III); (D) La (III). Error bars correspond to standard deviation of three independent replicates.
A recent study compared three adsorbent mixtures to retain several rare-earth elements, such as Ce, Dy, Eu, La, Nd, and Y. These mixtures were composed by combinations of residual microalgae biomass, chitosan, thermally expanded graphite, and/or millet husk. The maximum capacity of these adsorbent was about 5 mg/g according to the Langmuir model, which is a very similar value obtained for WS in our study (Kosheleva et al., 2018). Grapefruit peel has also been used to adsorb La and Ce, observing similarities with our data since between pH 4 and 5 the highest adsorption was reached (between 80 and 90%) (Torab-Mostaedi et al., 2015). Additionally, it was reported that corn style, pineapple crown, orange peel, neem saw dust, prawn carapace, egg shell, fish scales, and crab shell also can adsorb cerium(III). It was shown that the highest adsorption was reached at pH values between 4 and 6, and corn style plus prawn carapace had the best performance in Ce adsorption (Jaya and Nilanjana, 2014). Another low-cost adsorbent that has been studied was malt spent rootlets (MSR), which showed to have the maximum adsorption of europium at pH between 4 and 5 with a removal percentage of ~70% (Jaya and Nilanjana, 2014). Additionally, durian rind sorbent was used to adsorb La and Y, showing that at pH between 4 and 5 the adsorption was about 73% for both elements (Kusrini et al., 2019). Moreover, the adsorption efficiency of several low-cost adsorbents to remove heavy metals such as Pb was also described. For instance, hazelnut shell (90%), pistachio shell (83%), and walnut shell (96, 95, and 99%) (Çelebi and Gök, 2017). The reuse or regeneration of low-cost materials has been studied in the adsorption of heavy metal and organic compounds (such a naphthalene, oils, among others), mainly showing that regeneration might range approximately from 1 to 6 cycles (Altun and Pehlivan, 2012; Zhou et al., 2015; Zhu et al., 2016; Banerjee et al., 2018). As mentioned before, a single article showed the reuse of WS for REE adsorption only, which indicated that it is possible to use WS as adsorbent about 1,000 times (Li et al., 2012).
Finally, it was not possible to quantify residual Nd (after adsorption experiments) by ICP-OES. Interferences might affect the quantification; however, no matrix effects or spectral overlaps were detected in the Nd standard. The wavelength used during ICP-OES analysis was 430.3 nm, as indicated by a previous report, which is a value that shows high sensitivity and performance for Nd quantification (Krachler et al., 2015). In our samples, the matrix has an effect on Nd quantification, and so far, no other studies have reported this effect. Therefore, the adsorption percentage and capacity for Nd were not determined.
Adsorption Isotherms
Equilibrium correlations between adsorbent and adsorbate are defined by adsorption isotherms (Dada et al., 2012). The sorption isotherms for the REEs were studied using a batch technique. Considering the calculated adsorption capacity (qe) of WS for every REE, the experimental sorption isotherms were calculated by the Freundlich, Langmuir, and Temkin isotherm models, as represented by Equations (3–5), respectively. Calculated isotherm parameters for the adsorption of REEs onto WS at 20°C are shown in Figure 2, and details for other parameters are found in Table S2. The best fit was obtained using the Langmuir model, as shown by the highest coefficient value 0.999. The Langmuir model suggests that REEs are adsorbed onto the WS in a monolayer, and considering that the best results were obtained with a particle size of 75 μm, it can be assumed that the maximum monolayer adsorption capacities for WS were 5.7 mg/g for Eu, 5.1 mg/g for Gd, 6.1 mg/g for Sm, and 6.0 mg/g for La (as shown in Table S2). Additionally, the separation factor RL was calculated for every metal, which helps to predict the affinity between the adsorbent and adsorbate. Every REE (at different pH values and both WS particle sizes) showed an RL below 1 and higher than 0, which indicates that the shape of the adsorption system is favorable (Kilic et al., 2011; Çelebi and Gök, 2017). Today, the single report in which WS is used for REE adsorption does not present adsorption isotherm analysis, since the aim of that article is to concentrate REEs with a microcolumn filled with WS to coupled it to ICP-MS (Li et al., 2012). Nevertheless, the authors presented the adsorption percentages for REEs onto WS (>90%), which are similar to our results (higher than 80%). Additional study indicated that natural walnut shells (NWS) can be used to efficiently remove Pb(II). In these experiments, the researchers determined that the adsorption capacity of the NWS for Pb(II) was 9.9 mg/g and that the adsorption model was well-described with a Langmuir isotherm (Çelebi and Gök, 2017). Moreover, the adsorption of Cr(VI) by walnut shell was Cr(VI) reported with an adsorption capacity of between 16.73 and 40.99 mg/g. Researchers indicated that WS has the potential to be used as a green adsorbent and it can be used in small-scale industries (Banerjee et al., 2018). Since little information is available regarding REE adsorption onto WS, our study provides valuable data, such as the high adsorption capacity of WS to retain these elements, and the ranges at which the adsorbent is most efficient for REE adsorption, in addition to the adsorption isotherm that fits better to this adsorption model.
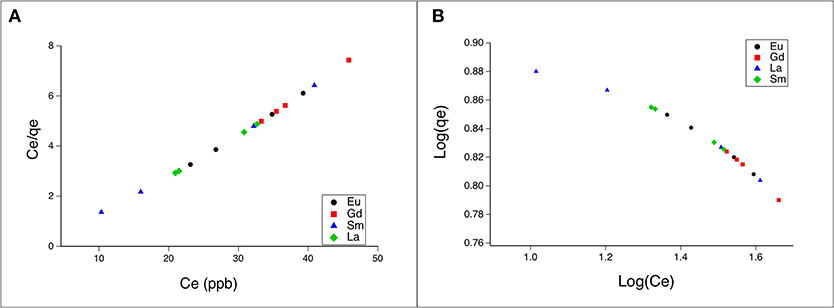
Figure 2. Experimental sorption isotherms of rare-earth elements onto 75-μm particle size WS. (A) Sorption isotherm represented by the Langmuir isotherm model of REEs by WS; (B) sorption isotherm represented by the Freundlich isotherm model of REEs by WS.
Adsorbent Characterization
Thermogravimetry and Differential Scanning Calorimetry (TG-DSC) Characterization
The thermal behavior of natural WS and WS-REEs was evaluated at pH 4, as it was the optimal pH. Nevertheless, TG and DSC for La were also evaluated at different pH values, as shown in Figures S1A,B. Figures 3A,B show the TG and derivative of TG (DTG), respectively, for the five elements (La, Eu, Sm, Gd, and Nd) adsorbed onto WS at pH 4. Pyrolysis of WS can be divided into three stages, as shown in Figures 3A,B: the first stage can be attributed to the vaporization of moisture, which contributes ~10% of the weight loss. The second stage, observed in more detail in Figure 3B, refers to the thermal decomposition of hemicellulose, cellulose, and lignin, which contributes more than 60% of the weight loss. The last stage involves the high-temperature calcination of residues, and the weight loss is >25%. As reported by several authors (Burhenne et al., 2013), TG analysis suggests that WS comprises hemicellulose, which is a group of biopolymers such as branched polysaccharides, and decomposes faster and at lower temperatures than cellulose and lignin, because hemicellulose is thermally more unstable (Burhenne et al., 2013). In agreement with the literature, the negative DTG curve for WS and REE-WS and DSC-DDSC (Figure 3) showed the hemicellulose decomposition in a temperature range between 200 and 300°C. The next decomposition might be attributed to cellulose, which ranged between 300 and 400°C, and finally, lignin decomposition can be observed at higher temperatures (>400°C). Similar results were reported before, where decomposition of hemicellulose, cellulose, and lignin ranged from 225 to 325°C, 337 to 407°C, and 417 to 607°C, respectively, for WS (Burhenne et al., 2013). Recently, it was described that pyrolysis of WS led to an ~90% weight loss in a temperature range of 200–467°C, which corresponds to rapid thermal decomposition of cellulose, hemicellulose, and part of lignin, confirming that lignin is pyrolyzed at temperatures above 400°C (Fan et al., 2018). Therefore, we can assume that metal adsorption onto WS showed no major changes in the thermal decomposition of WS.
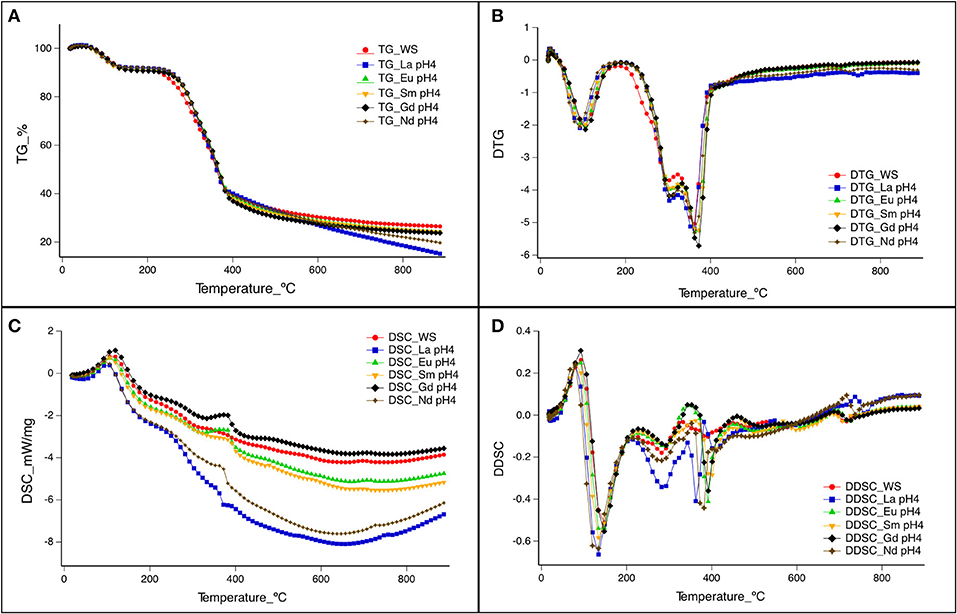
Figure 3. Thermogravimetry (TG) and differential scann ing calorimetry (DSC) of rare-earth element adsorption to WS at pH 4. (A,B) correspond to TG and the derivative of TG of Lanthanum adsorption to WS at pH 4. (C,D) correspond to DSC and derivative DSC of Lanthanum adsorption to WS at pH 4.
Fourier-Transform Infrared Spectroscopy (FT-IR) Characterization
WS, as agricultural waste, has the potential to be a good bioadsorbent that might retain heavy metals (Kazemipour et al., 2008), pesticides (Ahmad et al., 2010), and pharmaceutical residues (Ahmed and Hameed, 2018), and now it is reported that WS can efficiently retain rare-earth elements. Then, complementing our previous results, IR spectra for natural WS (control) and several REEs adsorbed at different pH values were acquired. Figure 4 shows the FTIR spectra for the control, Eu, Sm, La, Nd, and Gd adsorption at pH 4 (optimum condition, as defined previously). FTIR spectra at pH 5.5, 3, and 7 were also acquired, and they are shown in Figures S3–S5, respectively. Regarding Figure 4 and Table S3, the peak at 3,370 cm−1 in the control, which corresponds to the stretching vibration of O–H, showed larger spectral changes in the FTIR spectra measured for REEs for all studied pH values. Fewer spectral changes were observed at 1,328 and 1,049.18 cm−1, which correspond to carboxylate, C=O stretching, and stretching vibrations of C–O–C groups. Minor changes detected in other functional groups are detailed in Table S3. Similar results have been reported before, but the functional groups changed when REEs were adsorbed onto WS, as shown in Table S3E (Li et al., 2012). Instead, we detected two additional peaks, 1,428.58 and 1,328.66 cm−1, which were not previously reported by Li and coworkers. Previous report showed that for different adsorbents, both peaks might be related to alkane group stretching and carboxylate anion C=O stretching, respectively (Singha et al., 2011). Moreover, in several studies concerning different bioadsorbents, such as residual microalgae biomass, chitosan, millet husk, grapefruit peel, and eggshell, among others, it was indicated that chelation of rare-earth elements was facilitated by OH, NH, and CO functional groups according to FTIR analysis (Jaya and Nilanjana, 2014; Torab-Mostaedi et al., 2015; Kosheleva et al., 2018; Kusrini et al., 2019).
Then, according to our data, it can be suggested that OH is mainly responsible for REE adsorption onto WS, since at different pH values, all tested metals showed a larger wavenumber shift after adsorption.
Scanning Electron Microscopy (SEM)
The surface physical morphology of WS was characterized before and after REE adsorption by SEM analysis. Figures 5A–F shows SEM images with a magnification of 700×, while Figures S5A–F shows data obtained at a magnification of 4,500×. Empty and filled pores of different sizes were observed after metal adsorption, in addition to a more roughened aspect. Similar results were observed previously, where WS before adsorption of Cr(VI) was shown to be more porous, and it was observed that pores were partially blocked after metal adsorption (Singha et al., 2011). Moreover, it has been described previously that the external surface of WS (before and after metal adsorption) has a highly porous structure with round edges (Çelebi and Gök, 2017), which is similar to our results. Additionally, Çelebi and collaborators indicated that WS presented two types of pores: open and closed. The open pore is a slit-shaped pore or slit, and the closed pores are cylindrical (Çelebi and Gök, 2017). It was described that optimal activation of micropores can be achieved at 375°C. At this temperature, uniform micropores are developed, while at higher temperatures, such as 425°C, the structure of activated carbon collapses causing the extinction of micropores (Kim et al., 2001). Even though the effective adsorption of REEs onto WS was observed in this study, such adsorption might be improved by activating the adsorbent at 375°C for 1 h, as reported previously.
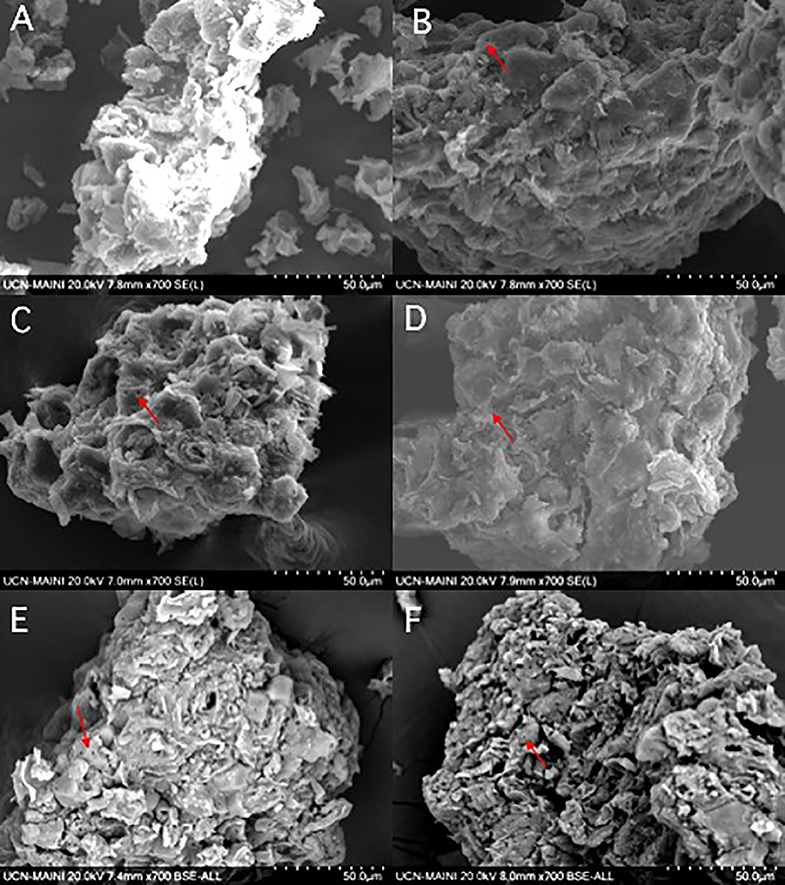
Figure 5. SEM micrograph of natural walnut shell before and after rare-earth elements adsorption at 700×. (A) Natural walnut shell (WS); (B) Nd adsorption onto WS; (C) La adsorption onto WS; (D) Gd adsorption onto WS; (E) Sm adsorption onto WS; (F) Eu adsorption onto WS. Arrows indicate the presence of porous.
Conclusions
Employment of walnut shell as a raw material for the adsorption of metals is a useful recycling process. The good adsorption capacity and removal efficiency of WS might be successfully used for adsorbing Eu, La, Sm, and Gd from aqueous solution. The present work found that REE adsorption is pH dependent, and the optimum pH value was determined to be 4. Additionally, REE ions were adsorbed onto the WS in a monolayer, since a best fit was obtained by using a Langmuir model, as shown by the highest coefficient value of 0.999. The maximum capacity based on Langmuir isotherm REE adsorption was defined at a particle size of 75 and 2,000 μm. A maximum retention of 93% was obtained for Sm at a particle size of 75 μm at pH 4. Characterization of walnut shell before and after adsorption by FTIR indicated that, among several functional groups that showed changes in wavenumber, the OH functional group showed the largest shift, which might indicate that it is involved in the retention of the REEs. TG-DSC characterization showed the thermal decomposition of WS and indicated that more than 60% of the weight corresponds to hemicellulose, cellulose, and lignin, which might be involved in the adsorption process of REEs. Finally, SEM characterization showed filled pores of different sizes after metal adsorption and a more roughened aspect, which might be improved by calcination at high temperatures such as 375°C. It is concluded that WS is a green and environmentally friendly biomaterial with high capacity toward retaining several metals, specifically REEs. Moreover, although no activation was done, we could obtain high adsorption with natural WS, becoming in excellent results for upcoming work. Then, WS has the potential to be used in the future to recover REEs from different secondary sources, such as waste electrical and electronic equipment (WEEE) or mine tailing, and to contribute to circular economy.
Data Availability Statement
All datasets presented in this study are included in the article/Supplementary Material.
Author Contributions
All authors listed have contributed equally and made a substantial, direct and intellectual contribution to the work, and approved it for publication.
Funding
We gratefully acknowledge to project CORFO ING2030 16EN12-71940 for the financial support to KG to develop this research. The authors acknowledge to Fondequip EQM130135 for TG-DSC, to Scientific Equipment Unit-MAINI of the Universidad Católica del Norte for FE-SEM SU5000, to Ceitsaza of the Universidad Católica del Norte for FT-IR and ICP-OES. The authors also acknowledge to project Fondecyt 3170076 for the financial support to RC. Finally, we thank to PMI 1795 project (Programa de Mejoramiento Institucional en Recursos Hídricos 1795) for financial support during experiments.
Conflict of Interest
The authors declare that the research was conducted in the absence of any commercial or financial relationships that could be construed as a potential conflict of interest.
Supplementary Material
The Supplementary Material for this article can be found online at: https://www.frontiersin.org/articles/10.3389/.2020.00004/full#supplementary-material
Abbreviations
REEs, rare-earth elements; WS, walnut shell; WS-REEs, walnut shell containing REEs; FTIR, Fourier transformed infrared spectroscopy; TG-DSC, thermogravimetry and differential scanning calorimetry; LOD and LOQ, limit of detection and quantification; ICP-OES, inductively coupled plasma optical emission spectrometry; qe, adsorption capacity; Kf and n, Freundlich constants; Kl, Langmuir sorption constant; WEEE, waste electrical and electronic equipment.
References
Abdelnour, S. A., Abd El-Hack, M. E., Khafaga, A. F., Noreldin, A. E., Arif, M., Chaudhry, M. T., et al. (2019). Impacts of rare earth elements on animal health and production: highlights of cerium and lanthanum. Sci. Total Environ. 672, 1021–1032. doi: 10.1016/j.scitotenv.2019.02.270
Agathokleous, E., Kitao, M., and Calabrese, E. J. (2019). Hormetic dose responses induced by lanthanum in plants. Environ. Pollut. 244, 332–341. doi: 10.1016/j.envpol.2018.10.007
Ahmad, T., Rafatullah, M., Ghazali, A., Sulaiman, O., Hashim, R., and Ahmad, A. (2010). Removal of pesticides from water and wastewater by different adsorbents: a review. J. Environ. Sci. Health Part C Environ. Carcinog. Ecotoxicol. Rev. 28, 231–271. doi: 10.1080/10590501.2010.525782
Ahmed, M. J., and Hameed, B. H. (2018). Removal of emerging pharmaceutical contaminants by adsorption in a fixed-bed column: a review. Ecotoxicol. Environ. Saf. 149, 257–266. doi: 10.1016/j.ecoenv.2017.12.012
Altun, T., and Pehlivan, E. (2012). Removal of Cr(VI) from aqueous solutions by modified walnut shells. Food Chem. 132, 693–700. doi: 10.1016/j.foodchem.2011.10.099
Alvarez Puebla, R., Aroca, R., Valenzuela Calahorro, C., and Garrido, J. (2006). Retention of cobalt on a humin derived from brown coal. J. Hazard. Mater. 135, 122–128. doi: 10.1016/j.jhazmat.2005.11.041
Babel, S., and Kurniawan, T. A. (2003). Low-cost adsorbents for heavy metals uptake from contaminated water: a review. J. Hazard. Mater. 97, 219–243. doi: 10.1016/S0304-3894(02)00263-7
Babel, S., and Kurniawan, T. A. (2004). Cr(VI) removal from synthetic wastewater using coconut shell charcoal and commercial activated carbon modified with oxidizing agents and/or chitosan. Chemosphere 54, 951–967. doi: 10.1016/j.chemosphere.2003.10.001
Banerjee, M., Basu, R. K., and Das, S. K. (2018). Cr(VI) adsorption by a green adsorbent walnut shell: adsorption studies, regeneration studies, scale-up design and economic feasibility. Process Saf. Environ. Prot. 116, 693–702. doi: 10.1016/j.psep.2018.03.037
Bayat, M., Alighardashi, A., and Sadeghasadi, A. (2018). Fixed-bed column and batch reactors performance in removal of diazinon pesticide from aqueous solutions by using walnut shell-modified activated carbon. Environ. Technol. Innov. 12, 148–159. doi: 10.1016/j.eti.2018.08.008
Binnemans, K., Jones, P. T., Blanpain, B., Van Gerven, T., Yang, Y., Walton, A., et al. (2013). Recycling of rare earths: a critical review. J. Clean. Prod. 51, 1–22. doi: 10.1016/j.jclepro.2012.12.037
Burhenne, L., Messmer, J., Aicher, T., and Laborie, M. P. (2013). The effect of the biomass components lignin, cellulose and hemicellulose on TGA and fixed bed pyrolysis. J. Anal. Appl. Pyrolysis 101, 177–184. doi: 10.1016/j.jaap.2013.01.012
Çelebi, H., and Gök, O. (2017). Evaluation of lead adsorption kinetics and isotherms from aqueous solution using natural walnut shell. Int. J. Environ. Res. 11, 83–90. doi: 10.1007/s41742-017-0009-3
Cheng, Y., Zhang, L., Bian, X., Zuo, H., and Dong, H. (2018). Adsorption and mineralization of REE—lanthanum onto bacterial cell surface. Environ. Sci. Pollut. Res. 25, 22334–22339. doi: 10.1007/s11356-017-9691-0
Dada, A. O., Olalekan, A. P., Olatunya, A. M., and Dada, A. O. (2012). Langmuir, freundlich, temkin and dubinin–radushkevich isotherms studies of equilibrium sorption of Zn 2+ unto phosphoric acid modified rice husk. IOSR J. Appl. Chem. 3, 38–45. doi: 10.9790/5736-0313845
Dai, Y., Sun, Q., Wang, W., Lu, L., Liu, M., Li, J., et al. (2018). Utilizations of agricultural waste as adsorbent for the removal of contaminants: a review. Chemosphere 211, 235–253. doi: 10.1016/j.chemosphere.2018.06.179
Darmayanti, L., Notodarmodjo, S., and Damanhuri, E. (2017). Removal of Copper (II) Ions in aqueous solutions by sorption onto fly ash. J. Eng. Technol. Sci. 49:546. doi: 10.5614/j.eng.technol.sci.2017.49.4.9
Darren, L., Keith, K., Pham, M., and Michael, S. (2014). A Systematic Evaluation of Dissolved Metals Loss during Water Sample Filtration Regional Applied Research Effort - Addressing Challenges through Science and Innovation. Cincinnati.
Demirbaş, E., Kobya, M., Öncel, S., and Encan, S. (2002). Removal of Ni(II) from aqueous solution by adsorption onto hazelnut shell activated carbon: equilibrium studies. Bioresour. Technol. 84, 291–293. doi: 10.1016/S0960-8524(02)00052-4
Ennoukh, F., Brini, L., Chafik, D., Bouhaouss, A., and Bchitou, R. (2019). Removal of hexavalent chromium from aqueous solutions using Argania spinosa leaves. Desalin. Water Treat. 151, 273–279. doi: 10.5004/dwt.2019.23886
Fan, F., Li, H., Xu, Y., Liu, Y., Zheng, Z., and Kan, H. (2018). Thermal behaviour of walnut shells by thermogravimetry with gas chromatography-mass spectrometry analysis. R. Soc. Open Sci. 5:180331. doi: 10.1098/rsos.180331
Feizi, M., and Jalali, M. (2015). Removal of heavy metals from aqueous solutions using sunflower, potato, canola and walnut shell residues. J. Taiwan Inst. Chem. Eng. 54, 125–136. doi: 10.1016/j.jtice.2015.03.027
Ghasemi, M., Ghoreyshi, A. A., Younesi, H., and Khoshhal, S. K. (2015). Synthesis of a high characteristics activated carbon from walnut shell for the removal of Cr (VI) and Fe (II) from aqueous solution: single and binary solutes adsorption. Iran. J. Chem. Eng. 12, 28–51.
Hu, Z., Haneklaus, S., Sparovek, G., and Schnug, E. (2006). Rare earth elements in soils. Commun. Soil Sci. Plant Anal. 37, 1381–1420. doi: 10.1080/00103620600628680
Hydari, S., Sharififard, H., Nabavinia, M., and Parvizi, M. (2012). A comparative investigation on removal performances of commercial activated carbon, chitosan biosorbent and chitosan/activated carbon composite for cadmium. Chem. Eng. J. 193–194, 276–282. doi: 10.1016/j.cej.2012.04.057
Ijagbemi, C. O., Baek, M. H., and Kim, D. S. (2009). Montmorillonite surface properties and sorption characteristics for heavy metal removal from aqueous solutions. J. Hazard. Mater. 166, 538–546. doi: 10.1016/j.jhazmat.2008.11.085
Jaya, S. V. C., and Nilanjana, D. (2014). Screening of biowaste materials for the sorption of cerium (III) from aqueous environment. Res. J. Pharm. Biol. Chem. Sci. 5, 402–408. Available online at: http://rjpbcs.com/pdf/2014_5(5)/[54].pdf
Kazemipour, M., Ansari, M., Tajrobehkar, S., Majdzadeh, M., and Kermani, H. R. (2008). Removal of lead, cadmium, zinc, and copper from industrial wastewater by carbon developed from walnut, hazelnut, almond, pistachio shell, and apricot stone. J. Hazard. Mater. 150, 322–327. doi: 10.1016/j.jhazmat.2007.04.118
Kilic, M., Apaydin-Varol, E., and Pütün, A. E. (2011). Adsorptive removal of phenol from aqueous solutions on activated carbon prepared from tobacco residues: equilibrium, kinetics and thermodynamics. J. Hazard. Mater. 189, 397–403. doi: 10.1016/j.jhazmat.2011.02.051
Kim, J. W., Sohn, M. H., Kim, D. S., Sohn, S. M., and Kwon, Y. S. (2001). Production of granular activated carbon from waste walnut shell and its adsorption characteristics for Cu2+ ion. J. Hazard. Mater. 85, 301–315. doi: 10.1016/S0304-3894(01)00239-4
Kosheleva, A., Atamaniuk, I., Politaeva, N., and Kuchta, K. (2018). Adsorption of rare earth elements using bio-based sorbents. MATEC Web Conf. 245:18001. doi: 10.1051/matecconf/201824518001
Krachler, M., Alvarez-Sarandes, R., and Van Winckel, S. (2015). Challenges in the quality assurance of elemental and isotopic analyses in the nuclear domain benefitting from high resolution ICP-OES and sector field ICP-MS. J. Radioanal. Nucl. Chem. 304, 1201–1209. doi: 10.1007/s10967-015-3952-5
Kusrini, E., Usman, A., Sani, F. A., Wilson, L. D., and Abdullah, M. A. A. (2019). Simultaneous adsorption of lanthanum and yttrium from aqueous solution by durian rind biosorbent. Environ. Monit. Assess. 191:488. doi: 10.1007/s10661-019-7634-6
Laszlo, J. A., and Dintzis, F. R. (1994). Crop resides as lon-exchange materials. Treatment of soybean hull and sugar beet fiber (pulp) with epichlorohydrin to improve cation-exchange capacity and physical stability. J. Appl. Polym. Sci. 52, 531–538. doi: 10.1002/app.1994.070520408
Li, Y., Yang, J. L., Jiang, Y., Yan, L., JiaLin, Y., and Yan, J. (2012). Trace rare earth element detection in food and agricultural products based on flow injection walnut shell packed microcolumn preconcentration coupled with inductively coupled plasma mass spectrometry. J. Agric. Food Chem. 60, 3033–3041. doi: 10.1021/jf2049646
Módenes, A. N., Espinoza-Quiñones, F. R., Colombo, A., Geraldi, C. L., and Trigueros, D. E. G. (2015). Inhibitory effect on the uptake and diffusion of Cd2+ onto soybean hull sorbent in Cd–Pb binary sorption systems. J. Environ. Manage. 154, 22–32. doi: 10.1016/j.jenvman.2015.02.022
Neris, J. B., Luzardo, F. H. M., da Silva, E. G. P., and Velasco, F. G. (2019). Evaluation of adsorption processes of metal ions in multi-element aqueous systems by lignocellulosic adsorbents applying different isotherms: a critical review. Chem. Eng. J. 357, 404–420. doi: 10.1016/j.cej.2018.09.125
Pehlivan, E., and Altun, T. (2008). Biosorption of chromium(VI) ion from aqueous solutions using walnut, hazelnut and almond shell. J. Hazard. Mater. 155, 378–384. doi: 10.1016/j.jhazmat.2007.11.071
Pehlivan, E., Altun, T., Cetin, S., and Iqbal Bhanger, M. (2009). Lead sorption by waste biomass of hazelnut and almond shell. J. Hazard. Mater. 167, 1203–1208. doi: 10.1016/j.jhazmat.2009.01.126
Queirós, C. S. G. P., Cardoso, S., Lourenço, A., Ferreira, J., Miranda, I., Lourenço, M. J. V., et al. (2020). Characterization of walnut, almond, and pine nut shells regarding chemical composition and extract composition. Biomass Convers. Biorefin. 10, 175–188. doi: 10.1007/s13399-019-00424-2
Shrivastava, A., and Gupta, V. (2011). Methods for the determination of limit of detection and limit of quantitation of the analytical methods. Chronicles Young Sci. 2, 21–25. doi: 10.4103/2229-5186.79345
Singha, B., Naiya, T. K., Bhattacharya, A., and kumar Das, S. K. (2011). Cr(VI) ions removal from aqueous solutions using natural adsorbents – FTIR Studies. J. Environ. Prot. 2, 729–735. doi: 10.4236/jep.2011.26084
Suresh Kumar, K., Dahms, H. U., Won, E. J., Lee, J. S., and Shin, K. H. (2015). Microalgae - a promising tool for heavy metal remediation. Ecotoxicol. Environ. Saf. 113, 329–352. doi: 10.1016/j.ecoenv.2014.12.019
Torab-Mostaedi, M., Asadollahzadeh, M., Hemmati, A., and Khosravi, A. (2015). Biosorption of lanthanum and cerium from aqueous solutions by grapefruit peel: equilibrium, kinetic and thermodynamic studies. Res. Chem. Intermed. 41, 559–573. doi: 10.1007/s11164-013-1210-4
Tripathi, A., and Rawat Ranjan, M. (2015). Heavy metal removal from wastewater using low cost adsorbents. J. Bioremed. Biodegrad. 6:315. doi: 10.4172/2155-6199.1000315
Wang, G., Li, A., and Li, M. (2010). Sorption of nickel ions from aqueous solutions using activated carbon derived from walnut shell waste. Desalin. Water Treat. 16, 282–289. doi: 10.5004/dwt.2010.1863
Xu, X., and Wang, Z. (2007). Phosphorus uptake and translocation in field-grown maize after application of rare earth-containing fertilizer. J. Plant Nutr. 30, 557–568. doi: 10.1080/01904160701209287
Yi, Z. J., Yao, J., Kuang, Y. F., Chen, H. L., Wang, F., and Yuan, Z. M. (2015). Removal of Pb(II) by adsorption onto Chinese walnut shell activated carbon. Water Sci. Technol. 72, 983–989. doi: 10.2166/wst.2015.305
Zabihi, M., Ahmadpour, A., and Haghighi Asl, A. (2009). Removal of mercury from water by carbonaceous sorbents derived from walnut shell. J. Hazard. Mater. 167, 230–236. doi: 10.1016/j.jhazmat.2008.12.108
Zabihi, M., Haghighi Asl, A., and Ahmadpour, A. (2010). Studies on adsorption of mercury from aqueous solution on activated carbons prepared from walnut shell. J. Hazard. Mater. 174, 251–256. doi: 10.1016/j.jhazmat.2009.09.044
Zhou, Y., Zhang, L., and Cheng, Z. (2015). Removal of organic pollutants from aqueous solution using agricultural wastes: a review. J. Mol. Liq. 212, 739–762. doi: 10.1016/j.molliq.2015.10.023
Zhu, M., Yao, J., Dong, L., and Sun, J. (2016). Adsorption of naphthalene from aqueous solution onto fatty acid modified walnut shells. Chemosphere 144, 1639–1645. doi: 10.1016/j.chemosphere.2015.10.050
Keywords: walnut shell, rare earth elements, green and low-cost adsorbents, adsorption isotherms, adsorption capacity
Citation: Gallardo K, Castillo R, Mancilla N and Remonsellez F (2020) Biosorption of Rare-Earth Elements From Aqueous Solutions Using Walnut Shell. Front. Chem. Eng. 2:4. doi: 10.3389/fceng.2020.00004
Received: 27 March 2020; Accepted: 29 May 2020;
Published: 22 July 2020.
Edited by:
Kun-Yi Andrew Lin, National Chung Hsing University, TaiwanReviewed by:
Harekrushna Sahoo, National Institute of Technology Rourkela, IndiaMin-Hao Yuan, China Medical University, Taiwan
Copyright © 2020 Gallardo, Castillo, Mancilla and Remonsellez. This is an open-access article distributed under the terms of the Creative Commons Attribution License (CC BY). The use, distribution or reproduction in other forums is permitted, provided the original author(s) and the copyright owner(s) are credited and that the original publication in this journal is cited, in accordance with accepted academic practice. No use, distribution or reproduction is permitted which does not comply with these terms.
*Correspondence: Karem Gallardo, a2dhbGxhcmRvQHVjbi5jbA==