- Sustainable Biofuels and Co-Products Research Unit, Eastern Regional Research Center, USDA-ARS, Wyndmoor, PA, United States
2,3-butanediol (2,3-BDO) is a platform chemical that can be converted to a wide array of products ranging from bio-based materials to sustainable aviation fuel. This chemical can be produced by a variety of microorganisms in fermentation processes. Challenges remain for high titer 2,3-BDO production during fermentation due to several parameters, but controlling oxygen is one of the most relevant processing parameters to ensure viable product output. This work investigated the fermentation of plant biomass sugars by the 2,3-BDO producer Paenibacillus polymyxa. Aerobic and oxygen limited fermentation conditions were initially evaluated using molasses-based media to determine cell growth and 2,3-BDO output. Similar conditions were then evaluated on hydrolysate from pretreated sweet sorghum bagasse (SSB) that contained fermentable sugars from structural polysaccharides. Fermentations in molasses media under aerobic conditions found that 2,3-BDO could be generated, but over time the amount of 2,3-BDO decreased due to conversion back into acetoin. Oxygen limited fermentation conditions exhibited improved biomass growth, but only limited suppression of 2,3-BDO conversion to acetoin occurred. Glucose depletion appeared to have a greater role influencing 2,3-BDO conversion back into acetoin. Further improvements in 2,3-BDO yields were found by utilizing detoxified SSB hydrolysate.
1 Introduction
Concerns over the detrimental effects of greenhouse gas emissions associated with petroleum based-fuels has influenced governmental policies and market priorities to look for alternative bio-based sources for similar liquid transportation fuels. In the first decade of the 21st Century the United States implemented the Renewable Fuel Standard (RFS) which sets both bio-ethanol production targets from grain and lignocellulosic biomass and blending requirements for bio-ethanol with gasoline (Bracmort, 2018). By implementing the RFS the United States has reached 16.06 billion gallons of bio-ethanol produced in 2018, which accounted for 56% of the total ethanol production worldwide (Renewable Fuels Association, 2019). Most of the bio-ethanol output in the United States comes from corn grain and the success of the industry is in part driven by the production of additional co-products from corn that improve process economics by increasing revenue (McAloon et al., 2000; Rausch et al., 2019). The RFS also stipulates that other biofuel types must be generated over the coming decades from non-grain crops such as lignocellulosic feedstocks. This will allow further expansion of the bioeconomy to include more agricultural producers to increase biomass cultivation. These producers can also improve cropland environmental outcomes through the implementation of regenerative farming practices. These practices allow a farm site to grow a diverse rotation of annual crops (e.g., corn, wheat, oats) to provide cover on croplands to protect soil and retain nutrients, while more environmentally sensitive soil is utilized to grow perennial grasses (Schulte et al., 2021). This application of crop diversity is beneficial for agricultural land rotation, soil conditions, and allows farmers greater flexibility to plant grain or lignocellulosic crops that correspond to the regional climate while meeting the demands for producing feedstocks needed to generate biofuels.
Recently, more emphasis is being placed on producing other types of biofuels alongside ethanol. Transportation sectors such as the commercial aviation industry are looking to reduce their CO2 emissions by converting fleets to run on sustainable aviation fuel. This will be challenging given that larger production volumes are required. In the United States domestic production of sustainable aviation fuel has only approached 4.6 million gallons as of 2020 (United States Energy Information Administration, 2022). A more ambitious goal has been set by the United States to produce 35 billion gallons of sustainable aviation fuel by 2050 (Bettenhausen, 2022). This benchmark indicates that policy makers and commercial partners are intending to increase output within this decade. The American Society for Testing and Materials (ASTM) has already approved seven fuel annexes and two co-processing routes to produce sustainable aviation fuel (Oldani and Hileman, 2022). The approved processing routes include chemical conversion strategies such as alcohol to jet (ATJ), hydrotreated esters and fatty acids (HEFA), catalytic hydrothermolysis (CH), and Fischer-Tropsch (FT) conversion that allow up to 50% of the fuel volume in a commercial airplane to be from a sustainable source (Bettenhausen, 2022). To meet the proposed volume goal for sustainable aviation fuels set for 2050, more processing routes will need to be developed and approved.
One promising processing route that can be utilized to produce sustainable aviation fuel is combining a fermentation step with downstream chemical modification. The fermentation route can utilize recovered sugars from lignocellulosic biomass to produce an intermediate chemical that can then be chemically converted to hydrocarbons. A common fermentation product from a multitude of microorganisms that can be utilized for this goal is 2,3-butanediol (2,3-BDO). This fermentation product is a prominent bio-based platform chemical that can be upgraded through chemical catalysis to generate materials such as a form of synthetic rubber, or food-grade and pharmaceutical products (Xiao and Xu, 2007; Haveren et al., 2008). By taking advantage of downstream chemical catalysis 2,3-BDO can also be converted to produce hydrocarbons for use as sustainable aviation fuel (Ji et al., 2011). This processing route using 2,3-BDO is promising for sustainable aviation fuel, but challenges remain at the fermentation level due to a variety of factors. One prominent factor that needs to be optimized for 2,3-BDO fermentation is the level of oxygen supplied. As with most fermentation processes oxygen is required to promote biomass growth, but the level of oxygen has to be controlled at later stages as too much dissolved oxygen in the fermentation media will inactivate the enzyme α-acetolactate synthase thereby shutting down the pathway to generate 2,3-BDO (Kosaric et al., 1992; Ji et al., 2011). However, eliminating all oxygen sources once biomass growth has been achieved will force the microorganism to produce ethanol and organic acids (Celińska and Grajek, 2009). These observations necessitate that a balance between aerobic and oxygen limited conditions must be achieved to reach high 2,3-BDO titers.
This aspect of aerobic versus oxygen limited fermentation conditions is explored in this work to determine overall 2,3-BDO output. The bacteria strain Paenibacillus polymyxa B-4317 was chosen due to its natural ability to produce 2,3-BDO along with being one of the few 2,3-BDO producing strains that is classified as a biosafety level 1 (BSL-1) microorganism. This strain of bacteria also has the ability to consume pentose sugars such as xylose. Prior fermentation research using P. polymyxa has indicated that the organism produces high xylanase activity for growth on both xylans or whole corn fiber, but very little 2,3-BDO could be produced on xylose sugars alone (Hespell, 1996). Fermentation media containing a mixture of both glucose and xylose were able to produce higher 2,3-BDO titers which demonstrates the importance of starting with a medium enriched with hexose sugars (Marwoto et al., 2002). Compared to the more commonly utilized 2,3-BDO producers in the Klebsiella or Enterobacter family, more fermentation process research on P. polymyxa is required. More recent work on P. polymyxa has focused on novel fermentation processing to generate high 2,3-BDO titers (around 50 g/L) while limiting 2,3-BDO mediated toxicity to the organism that would reverse 2,3-BDO back into acetoin (Okonkwo et al., 2017b). Other research on P. polymyxa has focused not on 2,3-BDO production but instead utilizing the organism as a biofertilizer, or as a producer of xylanse and mannase enzymes for the paper industry (Chantorn et al., 2022). This research looks to identify other fermentation process parameters to improve 2,3-BDO production by P. polymyxa. P. polymyxa was cultivated in both an aerobic environment and an oxygen limited environment using capped anaerobic bottles. Initial studies were performed using molasses media with additional nutrient supplementation to evaluate biomass growth, sugar consumption, and product generation. Hydrolysate from pretreated sweet sorghum bagasse also served as a medium to determine biomass growth and 2,3-BDO generation from a lignocellulosic source.
2 Materials and Methods
2.1 Strain culturing and chemicals
The 2,3-butanediol producer Paenibacillus polymyxa B-4317 was utilized in this work. A frozen seed culture was obtained from the USDA-ARS Culture Collection located at the National Center for Agricultural Utilization in Peoria, IL, United States. Luria-Bertani (LB) broth (Sigma-Aldrich, St. Louis, MO, United States) was prepared by dissolving 20 g of LB broth powder in 1 L DI water. The LB broth solution was autoclaved for 15 min at 121°C. The seed culture was thawed, and the entire contents were added to 50 ml of LB broth in a sterilized Erlenmeyer flask that was capped with a foam plug. The inoculum was placed in a New Brunswick Innova 42R (Edison, NJ, United States) incubator and cultivated at 39°C and 150 RPM for 20 h. After cultivating for 20 h a 1 ml volume of the grown P. polymyxa inoculum was transferred to cryovials and mixed with 0.5 ml of a sterilized 30% (w/v) glycerol solution. The cryovials containing the P. polymyxa inoculum were stored in a −80 °C freezer until needed for experiments. All other chemicals utilized in this research were of microbiological grade and obtained from Sigma-Aldrich (St. Louis, MO, United States).
2.2 Buffer, mineral, and nutrient media preparation
Additional fermentation supplementation media was prepared based on previous work (Okonkwo et al., 2017b). A phosphate buffer stock solution was prepared containing 1.75 g KH2PO4 and 1.375 g K2HPO4 in 500 ml DI water. The phosphate buffer pH was adjusted to 6.5 and sterilized by using a VWR (Radnor, PA, United States) filter cup with a 0.2 μm filter. A second stock solution containing additional minerals and vitamins was also prepared. This mineral/vitamin solution was prepared by dissolving 0.4 g FeSO4 in 3 ml of 25% (w/v) HCl, followed by dilution to a total volume of 500 ml. To this solution the other components dissolved included: 0.8 g H3BO3, 0.04 g CuSO4 pentahydrate, 0.04 g NaMoO4 dihydrate, 5.0 g MnCl2 tetrahydrate, 0.1 g ZnSO4 heptahydrate, 0.08 Co.(NO3)2 hexahydrate, 1.0 g CaCl2 dihydrate, and 0.01 g biotin. The mineral/vitamin solution was made up to 1 L with DI water and filter sterilized. Both the phosphate buffer and mineral/vitamin stock solutions were stored in a refrigerator until needed to prepare fermentation media. All media samples prepared for P. polymyxa fermentation contained roughly 3% (v/v) phosphate buffer and 0.3% (v/v) mineral/vitamin solution.
2.3 Molasses media preparation for fermentation
Molasses media was prepared at the following concentration: 12.5, 25, 50, and 100 g/L. Sugarcane molasses was graciously donated by the USDA-ARS Southern Regional Research Center (SRRC) located in New Orleans, LA, United States. The molasses was procured from the LaFourche Sugar Corporation located in Thibodaux, LA, United States, on 4 December 2019. The molasses was carefully added to a large beaker and mixed with 500 ml of DI water. Using a hot plate, the molasses/water solution was gently heated to allow the molasses to dissolve. The dissolved molasses was then supplemented with yeast extract at 4 g/L and urea at 0.8 g/L. The pH was adjusted to 6.5 with 1.0 M NaOH or 1.0 M HCl if necessary. The solution was centrifuged at 4000 g for 10 min in a Beckman Coulter (Brea, CA, United States) Avanti J-E centrifuge to remove any undissolved solids. The centrifuged molasses media was sterilized by autoclaving at 121°C for 15 min. A 1 ml sample was taken from each media for HPLC analysis. The molasses media was stored in refrigerator until use.
All fermentation trials were performed in duplicate. Shake flask fermentation was performed using either Erlenmeyer flasks with foam plugs for aerobic conditions, or in capped anaerobic bottles for oxygen limited cultivation. P. polymyxa seed culture was used to inoculate 125 ml LB broth and allowed to ferment for 24 h at 39°C at 160 RPM in an Innova 42R incubator. Aerobic flask fermentation was carried out by adding 50 ml of molasses media to the flask along with 1.0 ml of P. polymyxa inoculum to each flask. Fermentation was carried out at 39°C and 160 RPM for 1 week. A 0.75 ml sample was taken at 4 h and after that, each day. Optical density (OD) was immediately read after each sampling on a Shimadzu UV-1800 UV/vis spectrophotometer (Shimadzu Scientific Instruments, Somerset, NJ, United States) at 600 nm. OD measurements were made with a 10x dilution of the fermentation broth. The sample was then stored in a freezer until HPLC analysis.
Oxygen limited fermentations were conducted in 100 ml glass anaerobic bottles from Chemglass Life Sciences (Vineland, NJ, United States). As stated above, 50 ml of molasses media was dispensed into a sterilized anerobic bottle followed by a 1 ml aliquot of the P. polymyxa inoculum. The anaerobic bottles were then closed at the top opening of the bottle with a butyl rubber stopper that was held in place by a crimping an aluminum seal. An 18 gauge syringe needle with 40 mm length from BD (Franklin Lakes, NJ, United States) was inserted into the stopper. The end of the needle exposed to the outer environment had a 0.2 μm polyvinylidene (PVDF) syringe filter attached. This needle would allow a minimal amount of air flow into the sealed aerobic bottle to produce an oxygen limited environment. Samples were taken on the same schedule as described above and evaluated for OD. The remaining sample was frozen before HPLC analysis.
2.4 Lignocellulosic hydrolysate preparation for fermentation
A hydrolysate produced from pretreated sweet sorghum bagasse (SSB) was also utilized as an additional fermentation media to evaluate aerobic and oxygen limited cultivation of P. polymyxa. Sweet sorghum bagasse (SSB) was obtained from Delta BioRenewables (Memphis, TN, United States). The SSB was pretreated by the low-moisture anhydrous ammonia (LMAA) method that has been previously described (Stoklosa et al., 2019). The pretreated SSB was then enzymatically hydrolyzed with Novozymes (Franklinton, NC, United States) CTec2 and HTec2 enzymes according to a previous method (Stoklosa et al., 2021). Fermentation experiments were performed on the raw hydrolysate, and on a detoxified hydrolysate subjected to treatment with activated carbon at a 10% (w/v) loading. The detoxified hydrolysate was mixed with activated carbon in a capped Erlenmeyer flask and placed in an incubator for 2 h at 50°C and 200 RPM. The detoxified hydrolysate was then recovered by filtering through Whatman 50 filter paper. The hydrolysate was then supplemented with 4 g/L yeast extract, 0.8 g/L urea, and the same volume concentration of phosphate buffer and mineral/vitamin solution as stated above. The pH of the media was adjusted to 6.5 and sterilized through a 0.2 μm filter. Fermentation with the pretreated SSB hydrolysate media was conducted aerobically in Erlenmeyer flasks with foam plug stoppers, and with oxygen limitation in capped anaerobic bottles. Both fermentation methods were carried out as described under the section titled Molasses Media Fermentation.
2.5 Analytical methods
All sugars, organic acids, acetoin, and 2,3-BDO from fermentation samples were separated and quantified on an Agilent (Santa Clara, CA, United States) Infinity II 1260 HPLC system. Fermentation samples were centrifuged in an Eppendorf (Hauppauge, NY, United States) Mini-Spin Plus centrifuge at 9600 g for 10 min. The sample was then syringed filtered through 0.2 μm filter into HPLC vials. A Bio-Rad (Hercules, CA, United States) Aminex HPX-87P separation column with a de-ashing guard column was utilized to separate molasses samples that contained the sugars sucrose, glucose, and fructose. Ultrapure water from a EMD Millipore (Billerica, MA, United States) Simplicity filtration system was used as the mobile phase. Molasses media and lignocellulosic hydrolysate media samples containing glucose, xylose, organic acids, and 2,3-BDO were separated using a Bio-Rad Aminex HPX-87H column with a cation H+ guard column. The mobile phase for this analysis consisted of a 5 mM H2SO4 in ultrapure water. Sugars and 2,3-BDO were quantified by a refractive index detector (RID), while organic acids and acetoin were quantified by a diode array detector (DAD). The sample injection volume was set at 5 μL. A five-point calibration set of standards were analyzed for each HPLC sequence.
3 Results/discussions
3.1 Fermentation with molasses media
Most microorganisms that are relevant to industrial fermentation processes require oxygen to perform metabolic functions whether for growth or product generation. Bacteria strains that naturally produce 2,3-BDO function similarly, but the metabolic pathway that controls 2,3-BDO production is susceptible to decreased output that is correlated to increased oxygen uptake by the organism (Converti et al., 2003). However, oxygen is vital for the organism to grow enough biomass that is needed for 2,3-BDO production in the first place (Beronio Jr. and Tsao, 1993). To further understand the role of oxygen in a fermentation process using Paenibacillus polymyxa, both aerobic conditions and an oxygen limiting environment were studied. Media prepared with molasses was fermented under these conditions and Table 1 lists the initial sugar concentrations for each molasses sample. Sucrose is abundant followed by lower quantities of glucose and fructose, which is expected. High concentrations of sucrose might impede fermentation performance due to high initial carbon loadings, but prior work has shown that even sucrose concentrations above 200 g/L could be consumed by this class of microbes (Wang et al., 2021).
Figure 1 displays P. polymyxa biomass growth during fermentation using molasses media for both conditions. Under aerobic conditions in Figure 1A, P. polymyxa grows rapidly within the first 24 h of fermentation followed by a decline in biomass levels which is likely due to cell death. An OD reading of 11.0 was achieved within 24 h for the 50 g/L molasses media but decreased to around 4.0 starting at 72 h and remained constant until 144 h. At the highest molasses concentration of 100 g/L biomass growth was significantly hindered which might be attributed to the high carbon loading of sugars at that condition. The oxygen limited fermentation in Figure 1B indicates a slightly different biomass growth profile. All molasses media samples reached around a 4.0 OD reading within 24 h of cultivation. Samples containing 12.5–50 g/L then saw a decrease in biomass growth output as fermentation continued. Alternatively, the high molasses concentration at 100 g/L provided a more suitable growth environment under oxygen limitation. A fast growth of P. polymyxa occurred within 4 h of cultivation followed by an increase in biomass output until the end of fermentation. Under an aerobic environment with adequate mixing P. polymyxa should have enough access to oxygen allowing for continual biomass growth (Heyman et al., 2019). However, biomass growth decreases after 24 h for all molasses loadings except the 100 g/L condition. As will be discussed later, the 100 g/L molasses loading was the only condition with incomplete sugar utilization. Previous work has shown that biomass dry cell weight decreases once glucose is fully consumed during fermentation with the 2,3-BDO producing strain Bacillus licheniformis (Heyman et al., 2019). At the 100 g/L molasses loading a relatively high amount of sugar is left unconsumed, however, the residual sugar remaining does provide a carbon source for the organism to utilize and continue biomass growth until the end of fermentation. The effect of oxygen on biomass growth by P. polymyxa will be shown to play a role in the overall output of 2,3-BDO.
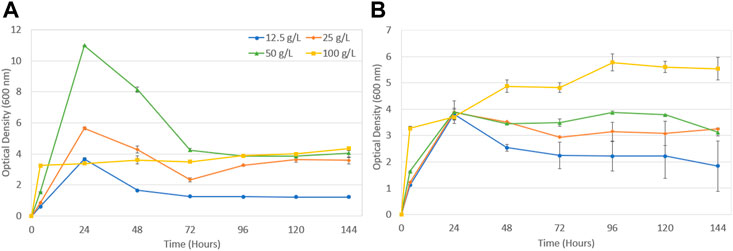
FIGURE 1. Biomass growth during fermentation with Paenibacillus polymyxa in molasses media under (A) aerobic conditions and (B) oxygen limitation.
Product generation during P. polymyxa fermentation, in particular 2,3-BDO output, is inherently tied to both oxygen availability and biomass growth by the organism. Figure 2 displays the sugar consumption and product generation for P. polymyxa during aerobic fermentation of molasses media. In Figures 2A–C sugar consumption was complete within 24 or 48 h for the 12.5, 25, and 50 g/L molasses, respectively. Figure 2D shows that at 100 g/L molasses loading only around 45% of the initial sugars were consumed within 144 h. Figure 2 also displays the product formation during fermentation. Although 2,3-BDO is the product of interest any bacteria strain that generates 2,3-BDO will also generate acetoin. The component acetoin is produced within the same pathway as 2,3-BDO production and is catalyzed by three enzymes that work on pyruvate to first produce α-acetolactate followed by conversion to acetoin through α-acetolactate decarboxylase and concluding with 2,3-BDO generation through 2,3-butanediol dehydrogenase or acetoin reductase (Ji et al., 2011; Tinôco et al., 2021b). Figures 2A,B show that acetoin generation exceeds 2,3-BDO production reaching about 16 and 36.9 g/L, respectively. Also shown in Figure 2B is that 2,3-BDO output tracks with acetoin production for the first 24 h, but then decreases with remaining fermentation time. 2,3-BDO has been generally understood to be generated by bacteria for carbon and energy storage (Xiao and Xu, 2007). These same bacteria strains will consume 2,3-BDO as a defense mechanism and reconvert it into acetoin (Okonkwo et al., 2017a). In Figure 2C at the 50 g/L molasses loading 2,3-BDO production reaches a maximum concentration of 31.9 g/L in 24 h but decreases to about 26 g/L for the remainder of the fermentation. Acetoin production increases up to 48 h and stabilizes at a consistent 25 g/L level. At this condition it is likely that P. polymyxa fermentation is lacking a nutritional component and the process is unable to generate additional 2,3-BDO or acetoin. Ethanol was also produced during aerobic fermentation with molasses media, but the concentration never exceeded 3 g/L. At 100 g/L molasses loading no 2,3-BDO was observed and only acetoin generation occurred reaching about 16 g/L.
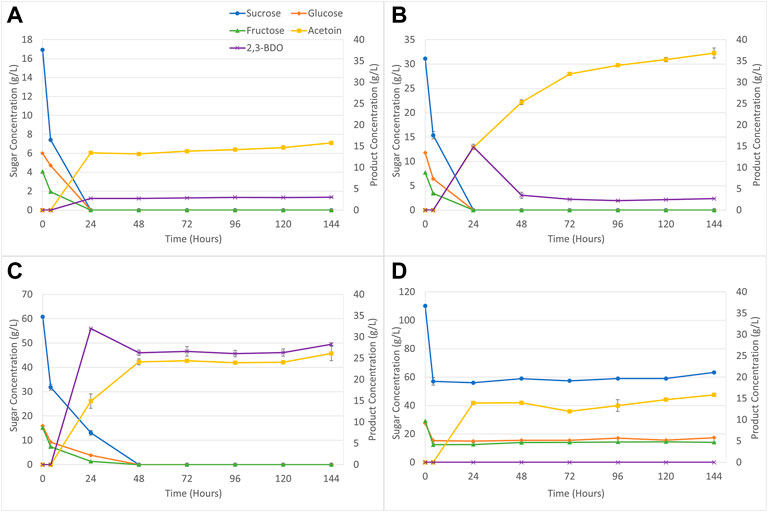
FIGURE 2. P. polymyxa sugar consumption and product generation during aerobic fermentation for (A) 12.5 g/L molasses loading, (B) 25 g/L molasses loading, (C) 50 g/L molasses loading, and (D) 100 g/L molasses loading.
The highest yields for 2,3-BDO and volumetric productivities were observed within 24 h of fermentation and are listed in Table 2. The 2,3-BDO yield obtained using 50 g/L molasses media is close to the theoretical yield of 0.50 g 2,3-BDO/g sugar consumed for 2,3-BDO production based on glucose consumption alone (Tinôco et al., 2021b).

TABLE 2. 2,3-Butanediol product yield and volumetric productivity at 24 h of aerobic fermentation of molasses media.
Figure 3 shows the sugar consumption and product generation for fermentation conducted on molasses media in an oxygen limited environment. As in Figure 2, the graphs in Figure 3 show complete sugar consumption occurs within 24–48 h at all molasses loadings except for the 100 g/L molasses condition. Even at this condition the sugar consumption in Figure 3D is around 60% of the initial concentration of sugars for fermentation which is more than the aerobic condition. The oxygen limited fermentation appears to have the effect of producing higher titers of 2,3-BDO at 12.5 and 25 g/L molasses compared to the aerobic fermentations. Table 3 shows the highest 2,3-BDO yields and volumetric productivity obtained during oxygen limited fermentation of molasses media.
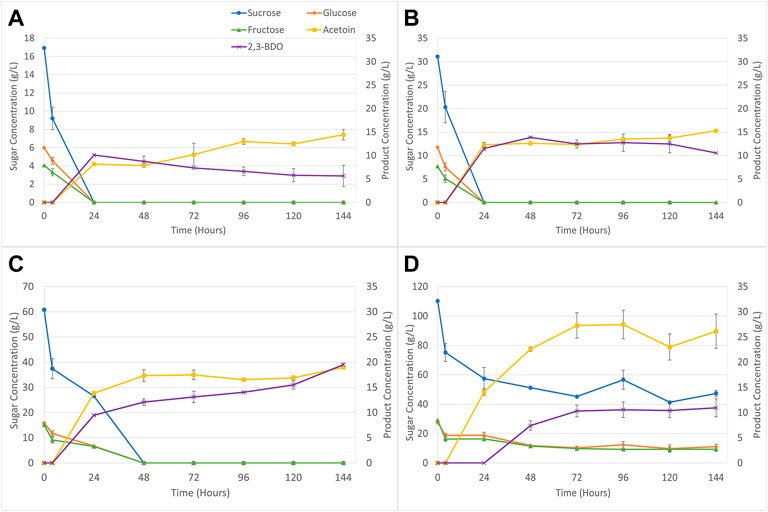
FIGURE 3. P. polymyxa sugar consumption and product generation during oxygen limited fermentation for (A) 12.5 g/L molasses loading, (B) 25 g/L molasses loading, (C) 50 g/L molasses loading, and (D) 100 g/L molasses loading.

TABLE 3. Highest 2,3-butanediol product yield and volumetric productivity obtained during oxygen limited fermentation of molasses media.
The highest 2,3-BDO yield was 0.423 g 2,3-BDO/g sugar consumed at 48 h for the 25 g/L molasses loading which corresponded to a volumetric productivity of 0.479 g/L hr. This yield for 2,3-BDO using 25.0 g/L of molasses media was found to be slightly higher than the yield obtained for 2,3-BDO produced from P. polymyxa PM 3605 that was cultivated on glycerol with 20 g/L of supplemented molasses (Tinôco et al., 2021a). As opposed to the aerobic fermentation using 100 g/L molasses, the oxygen limited fermentation of 100 g/L molasses produced a greater titer of acetoin at 26 g/L and around 10 g/L of 2,3-BDO. At this high loading of sugar with limited oxygen P. polymyxa was able to overcome any inhibition and produce 2,3-BDO. Molasses media fermentation with P. polymyxa under aerobic or oxygen limited conditions were able to produce adequate biomass and 2,3-BDO, but the byproduct acetoin was typically higher in titer for most conditions. Fermentations conducted in a limited oxygen environment should favor 2,3-BDO generation over acetoin by P. polymyxa (Qiu et al., 2016). As shown in Figure 3, for the most part acetoin generation still outpaces 2,3-BDO production in this oxygen limited environment. It is probable that the acetoin production by P. polymyxa is being influenced by exhausting or being in the presence of limited glucose content which allows the microbe to consume 2,3-BDO as a carbon source under this stress (Tsigoriyna et al., 2021). To evaluate whether greater 2,3-BDO titers could be achieved a hydrolysate from lignocellulosic biomass was produced and fermented under similar conditions.
3.2 Fermentation with lignocellulosic hydrolysate media
As opposed to molasses media which contains high loadings of sucrose that is easily metabolized, hydrolysates produced from pretreated lignocellulose material contain more moderate quantities of sugar in the form of glucose and xylose. A hydrolysate from low-moisture anhydrous ammonia (LMAA) pretreatment of sweet sorghum bagasse (SSB) was generated to conduct aerobic and oxygen limited fermentation with P. polymyxa. This hydrolysate was previously reported to contain 30 g/L of glucose accounting for a 70% theoretical yield, and 15 g/L of xylose at a 53.4% theoretical yield (Stoklosa et al., 2021). Aerobic fermentations with pretreated SSB hydrolysates produced less generation of both biomass and products compared to molasses media. Figure 4 displays sugar consumption, product generation, and cell biomass growth for the aerobic fermentation of a combined 1:1 (v/v) raw and detoxified pretreated SSB hydrolysate. The detoxification process did not alter or remove the components of glucose and xylose from the media which was confirmed by HPLC analysis. Cell biomass growth is also observed in Figure 4 for just raw hydrolysate at the same fermentation conditions.
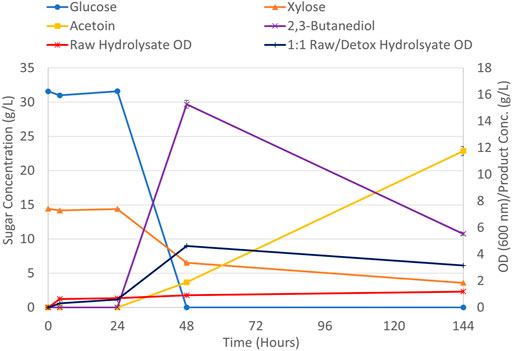
FIGURE 4. Sugar consumption, product generation, and biomass growth for the aerobic fermentation of a 1:1 (v/v) raw/detoxified pretreated SSB hydrolysate.
Glucose consumption was complete within 48 h, but about 20% of the initial of xylose remains after 144 h as shown in Figure 4. P. polymyxa has shown a natural ability to consume xylose in previous studies. A xylose-enriched hydrolysate from sulfuric acid pretreated wheat straw was fermented with P. polymyxa DSM 365 where the pentose sugar was consumed and 23.4 g/l of 2,3-BDO was generated (Narisetty et al., 2022). Incomplete xylose consumption in Figure 4 is indicative that the organism is lacking a specific nutrient to continue growth. Additionally, after 48 h of fermentation up to 15.3 g/L of 2,3-BDO was generated but this titer decreased to around 5.5 g/L at 144 h. In this same sampling interval, the acetoin titer increases from 1.9 g/L at 48 h to 11.8 g/L at 144 h. This trend in reduced 2,3-BDO output with increased acetoin production has been previously reported. Both components in the production pathway for the Bacillus family serve to maintain a redox balance between the NADH/NAD+ levels by undergoing reversible transformation to acetoin or 2,3-BDO (Petrov and Petrova, 2021). Starvation conditions also lead the cells to generate more ATP by consuming the produced 2,3-BDO which is oxidized back into acetoin thus yielding more NADH (Boecker et al., 2021).
Figure 4 also shows the OD biomass growth for the aerobic fermentation of only the raw pretreated SSB hydrolysate. This OD level only reached a level of 1.2 after 144 h of fermentation with no observable sugar consumption or product generation (data not shown). The pretreated SSB hydrolysate utilized here is known to contain soluble aromatic compounds from lignin that can be inhibitory to certain strains of bacteria and other yeasts (Guo et al., 2018; Stoklosa et al., 2019). The results presented in Figure 4 for the aerobic fermentation of the SSB hydrolysate show suboptimal biomass growth and product generation compared to the similar conditions evaluated for molasses media. With nitrogen and nutrient supplementation held constant between both media sets the driving factor for the fermentation performance between both sets appears to be dissolved inhibitory compounds in the SSB hydrolysate.
Pretreated SSB hydrolysate was next fermented with P. polymyxa under an oxygen limited environment. Figure 5 presents the biomass growth for this experimental condition. Compared to the biomass growth for the aerobic fermentation of the raw hydrolysate shown in Figure 4, the growth observed under oxygen limitation was improved. Both the 1:1 (v/v) raw/detox hydrolysate combination and fully detoxified hydrolysate produced rapid growth within 24 h. The raw hydrolysate without detoxification generated a lag in biomass growth for the first 24 h, but the growth rate improved to 5.6 after 48 h. At the completion of fermentation at 144 h the OD decreased for all hydrolysates, which was expected given the same result was observed for the molasses media fermentation. Figure 6 shows the sugar consumption and product generation for the oxygen limited fermentation conducted on pretreated SSB hydrolysate.
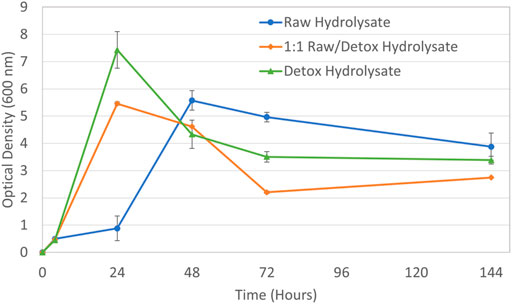
FIGURE 5. Biomass growth for P. polymyxa under oxygen limited fermentation with pretreated SSB hydrolysate media.
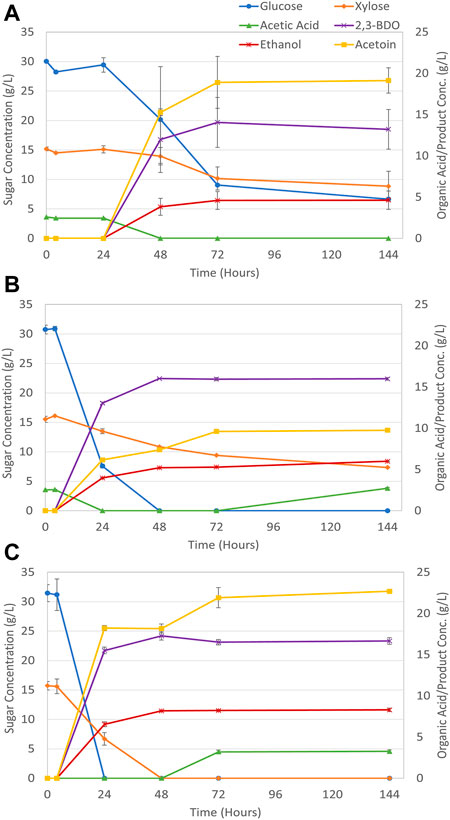
FIGURE 6. P. polymyxa sugar consumption and product generation during oxygen limited fermentation of (A) raw SSB hydrolysate, (B) 1:1 (v/v) raw/detox SSB hydrolysate, (C) detoxified SSB hydrolysate.
As Figure 6 shows the oxygen limited fermentation with P. polymyxa produces higher product titers with the SSB hydrolysate media compared to aerobic fermentation. Moreover, detoxified SSB hydrolysate can provide a more hospitable medium environment for both overall sugar consumption and higher product output. Figure 6A for the raw hydrolysate medium shows both incomplete sugar consumption for both glucose and xylose, but with 14.1 g/L of 2,3-BDO and 18.9 g/L of acetoin generated after 72 h. For the 1:1 (v/v) raw/detoxified SSB hydrolysate glucose was consumed within 48 h but about 7.3 g/L of xylose remains after 144 h. This condition also did not hinder 2,3-BDO production as 15.9 g/L of 2,3-BDO was generated at the end of fermentation which exceeded the 9.8 g/L of acetoin produced. The detoxified SSB hydrolysate shown in Figure 6C produced complete sugar utilization within 48 h and around 16.6 g/L of 2,3-BDO upon fermentation completion at 144 h along with 22.7 g/L of acetoin. Ethanol is also produced during fermentation at titers above 5 g/L for the SSB hydrolysate media. This concentration is slightly greater when compared to the molasses media, but the concentration of ethanol remains constant at 48 h and until the fermentation is stopped at 144 h. Table 4 lists the maximum 2,3-BDO yields and productivities obtained for the oxygen limited fermentation using pretreated SSB hydrolysate media.

TABLE 4. Highest 2,3-butanediol product yield and volumetric productivity obtained during oxygen limited fermentation of pretreated SSB hydrolysate media.
All 2,3-BDO yields obtained from pretreated SSB hydrolysate media were found to be higher compared to the yields for molasses media. Although the detoxified hydrolysate produced the lowest yield for the SSB hydrolysate, the volumetric productivity was found to be highest out of all three conditions. Most of the titers for 2,3-BDO produced by P. polymyxa fermentation in SSB hydrolysate media under oxygen limitation were only slightly lower than the 18.8 g/L of 2,3-BDO produced from corn stover hydrolysate using a cell recycling fermentation (Ma et al., 2018).
4 Conclusion
As demands for advanced biofuels increases, more bio-based production routes using lignocellulose sugars will be attractive processing options. The chemical 2,3-butanediol (2,3-BDO) can be fermented by a variety of bacterial organisms and can serve as a platform chemical for upgrading to hydrocarbons for use as a sustainable aviation fuel. 2,3-BDO still faces production challenges through fermentation due to several factors, but oxygen supply conditions are one of the most critical. Paenibacillus polymyxa, a natural 2,3-BDO producer, was fermented in both molasses media and pretreated sweet sorghum bagasse (SSB) hydrolysate media. The primary conditions evaluated showed that oxygen limited fermentation produced higher titers of 2,3-BDO compared to a fully aerobic fermentation. Although P. polymyxa growth was fast under fully aerobic conditions, 2,3-BDO production was hindered under these conditions and acetoin generation was favored. This finding was driven by glucose depletion and the microbe consuming 2,3-BDO for additional acetoin production. Utilizing an oxygen limited environment in sealed anaerobic bottles slowed biomass growth for both molasses media and SSB hydrolysate, but the overall production concentrations of 2,3-BDO were improved compared to aerobic fermentation. Further experiments are being conducted to determine if a limiting nutrient is influencing the overall fermentation performance by P. polymyxa along with larger scale studies in bioreactors using improved oxygen controls.
Data availability statement
The raw data supporting the conclusion of this article will be made available by the authors, without undue reservation.
Author contributions
RS conceptualized the research, planned and conducted experiments, collected and analyzed data, and contributed to writing and revising the manuscript; RL conducted experiments, collected and analyzed data, and contributed writing to the “Materials and Methods” section; DJ assisted with conceptualization, provided guidance on fermentation experiments, assisted with data interpretation, and revised the manuscript. All authors approve of the final version of the manuscript.
Acknowledgments
The authors would like to thank Delta Biorenewables LLC for providing sweet sorghum bagasse used in this work, Novozymes for providing CTec 2 and HTec 2 enzymes, and Dr. K. Thomas Klasson at the USDA-ARS Southern Regional Research Center for providing the molasses.
Conflict of interest
The authors declare that the research was conducted in the absence of any commercial or financial relationships that could be construed as a potential conflict of interest.
Publisher’s note
All claims expressed in this article are solely those of the authors and do not necessarily represent those of their affiliated organizations, or those of the publisher, the editors and the reviewers. Any product that may be evaluated in this article, or claim that may be made by its manufacturer, is not guaranteed or endorsed by the publisher.
Author disclaimer
Mention of trade names or commercial products in this publication is solely for providing specific information and does not imply recommendation or endorsement by the U.S. Department of Agriculture. USDA is an equal opportunity provider and employer.
References
Beronio, P. B., and Tsao, G. T. (1993). Optimization of 2, 3-butanediol production by Klebsiella oxytoca through oxygen transfer rate control. Biotechnol. Bioeng. 42 (11), 1263–1269. doi:10.1002/bit.260421102
Bettenhausen, C. A. (2022). “Flying the low-carbon skies,” in Chemical & engineering news (Washington, United States: American Chemical Society), 100, 16–19.
Boecker, S., Harder, B.-J., Kutscha, R., Pflügl, S., and Klamt, S. (2021). Increasing ATP turnover boosts productivity of 2, 3-butanediol synthesis in Escherichia coli. Microb. Cell Fact. 20 (1), 63. doi:10.1186/s12934-021-01554-x
Bracmort, K. (2018). The renewable fuel standard (RFS): An overview. Washington, DC, US: Congressional Research Service.
Celińska, E., and Grajek, W. (2009). Biotechnological production of 2, 3-butanediol—current state and prospects. Biotechnol. Adv. 27 (6), 715–725. doi:10.1016/j.biotechadv.2009.05.002
Chantorn, S., Aekkawatchai, N., Kasinsak, K., and Oontawee, S. (2022). Preservation of Paenibacillus polymyxa BTK01 and Bacillus subtilis BTK07 as lignocellulolytic bacterial starters for industrial applications: Physicochemical conditions, enzyme stability, freeze-drying processes and cryoprotection. Biocatal. Agric. Biotechnol. 42, 102326. doi:10.1016/j.bcab.2022.102326
Converti, A., Perego, P., and Del Borghi, M. (2003). Effect of specific oxygen uptake rate on Enterobacter aerogenes energetics: Carbon and reduction degree balances in batch cultivations. Biotechnol. Bioeng. 82 (3), 370–377. doi:10.1002/bit.10570
Guo, M., Jin, T., Nghiem, N. P., Fan, X., Qi, P. X., Jang, C. H., et al. (2018). Assessment of antioxidant and antimicrobial properties of lignin from corn stover residue pretreated with low-moisture anhydrous ammonia and enzymatic hydrolysis process. Appl. Biochem. Biotechnol. 184 (1), 350–365. doi:10.1007/s12010-017-2550-0
Haveren, J. V., Scott, E. L., and Sanders, J. (2008). Bulk chemicals from biomass. Biofuel. Bioprod. Biorefin. 2 (1), 41–57. doi:10.1002/bbb.43
Hespell, R. B. (1996). Fermentation of xylan, corn fiber, or sugars to acetoin and butanediol by Bacillus polymyxa strains. Curr. Microbiol. 32, 291–296. doi:10.1007/s002849900052
Heyman, B., Lamm, R., Tulke, H., Regestein, L., and Büchs, J. (2019). Shake flask methodology for assessing the influence of the maximum oxygen transfer capacity on 2, 3-butanediol production. Microb. Cell Fact. 18 (1), 78. doi:10.1186/s12934-019-1126-9
Ji, X.-J., Huang, H., and Ouyang, P.-K. (2011). Microbial 2, 3-butanediol production: A state-of-the-art review. Biotechnol. Adv. 29 (3), 351–364. doi:10.1016/j.biotechadv.2011.01.007
Kosaric, N., Magee, R., and Blaszczyk, R. (1992). Redox potential measurement for monitoring glucose and xylose conversion by K. pneumoniae. Chem. Biochem. Eng. Q. 6 (3), 145–151.
Ma, K., He, M., You, H., Pan, L., Wang, Z., Wang, Y., et al. (2018). Improvement of (R, R)-2, 3-butanediol production from corn stover hydrolysate by cell recycling continuous fermentation. Chem. Eng. J. 332, 361–369. doi:10.1016/j.cej.2017.09.097
Marwoto, B., Nakashimada, Y., Kakizono, T., and Nishio, N. (2002). Enchancement of (R, R)-2, 3-butanediol production from xylose by Paenibacillus polymyxa at elevated temperatures. Biotechnol. Lett. 24, 109–114. doi:10.1023/A:1013894403987
McAloon, A., Taylor, F., Yee, W., Ibsen, K., and Wooley, R. (2000). Determining the cost of producing ethanol from corn starch and lignocellulosic feedstocks. Golden, CO (US)): National Renewable Energy Lab.
Narisetty, V., Cox, R., Bommareddy, R., Agrawal, D., Ahmad, E., Pant, K. K., et al. (2022). Valorisation of xylose to renewable fuels and chemicals, an essential step in augmenting the commercial viability of lignocellulosic biorefineries. Sustain. Energy Fuels 6 (1), 29–65. doi:10.1039/d1se00927c
Okonkwo, C. C., Ujor, V. C., Mishra, P. K., and Ezeji, T. C. (2017b). Process development for enhanced 2, 3-butanediol production by Paenibacillus polymyxa DSM 365. Fermentation 3 (2), 18. doi:10.3390/fermentation3020018
Okonkwo, C. C., Ujor, V., and Ezeji, T. C. (2017a). Investigation of relationship between 2, 3-butanediol toxicity and production during growth of Paenibacillus polymyxa. New Biotechnol. 34, 23–31. doi:10.1016/j.nbt.2016.10.006
Oldani, A., and Hileman, J. (2022). “Decarbonizing aviation with sustainable aviation fuels,” in Chemical engineering progress (New York, United States: American Institute of Chemical Engineers), 118, 41–46.
Petrov, K., and Petrova, P. (2021). Current advances in microbial production of acetoin and 2, 3-butanediol by Bacillus spp. Fermentation 7 (4), 307. doi:10.3390/fermentation7040307
Qiu, Y., Zhang, J., Li, L., Wen, Z., Nomura, C. T., Wu, S., et al. (2016). Engineering Bacillus licheniformis for the production of meso-2, 3-butanediol. Biotechnol. Biofuels 9 (1), 117. doi:10.1186/s13068-016-0522-1
Rausch, K. D., Hummel, D., Johnson, L. A., and May, J. B. (2019). “Wet milling: The basis for corn biorefineries,” Corn, 2019, 501–535. doi:10.1016/B978-0-12-811971-6.00018-8
Renewable Fuels Association (2019). Annual ethanol production: U.S. and world ethanol production. [Online]. Available: at: https://ethanolrfa.org/markets-and-statistics/annual-ethanol-production (Accessed October 10, 2019).
Schulte, L. A., Dale, B. E., Bozzetto, S., Liebman, M., Souza, G. M., Haddad, N., et al. (2021). Meeting global challenges with regenerative agriculture producing food and energy. Nat. Sustain. 5, 384–388. doi:10.1038/s41893-021-00827-y
Stoklosa, R. J., Johnston, D. B., and Nghiem, N. P. (2019). Phaffia rhodozyma cultivation on structural and non-structural sugars from sweet sorghum for astaxanthin generation. Process Biochem. 83, 9–17. doi:10.1016/j.procbio.2019.04.005
Stoklosa, R. J., Moore, C., Latona, R. J., and Nghiem, N. P. (2021). Butyric acid generation by Clostridium tyrobutyricum from low-moisture anhydrous ammonia (LMAA) pretreated sweet sorghum bagasse. Appl. Biochem. Biotechnol. 193 (3), 761–776. doi:10.1007/s12010-020-03449-w
Tinôco, D., de Castro, A. M., Seldin, L., and Freire, D. M. G. (2021a). Production of (2R, 3R)-butanediol by Paenibacillus polymyxa PM 3605 from crude glycerol supplemented with sugarcane molasses. Process Biochem. 106, 88–95. doi:10.1016/j.procbio.2021.03.030
Tinôco, D., Pateraki, C., Koutinas, A. A., and Freire, D. M. G. (2021b). Bioprocess development for 2, 3-butanediol production by Paenibacillus strains. ChemBioEng Rev. 8 (1), 44–62. doi:10.1002/cben.202000022
Tsigoriyna, L., Ganchev, D., Petrova, P., and Petrov, K. (2021). Highly efficient 2, 3-butanediol production by Bacillus licheniformis via complex optimization of nutritional and technological parameters. Fermentation 7 (3), 118. doi:10.3390/fermentation7030118
United States Energy Information Administration (2022). Biofuels explained: Biodiesel. Renewable Diesel, and Other Biofuels [Online]. Available at: https://www.eia.gov/energyexplained/biofuels/biodiesel-rd-other-use-supply.php (Accessed July 27, 2022).
Wang, D., Oh, B.-R., Lee, S., Kim, D.-H., and Joe, M.-H. (2021). Process optimization for mass production of 2, 3-butanediol by Bacillus subtilis CS13. Biotechnol. Biofuels 14 (1), 15. doi:10.1186/s13068-020-01859-w
Keywords: lignocellulose, molasses, platform chemical, acetoin, 2,3-butanediol
Citation: Stoklosa RJ, Latona RJ and Johnston DB (2022) Assessing oxygen limiting fermentation conditions for 2,3-butanediol production from Paenibacillus polymyxa. Front. Chem. Eng. 4:1038311. doi: 10.3389/fceng.2022.1038311
Received: 06 September 2022; Accepted: 21 October 2022;
Published: 04 November 2022.
Edited by:
Daehwan Kim, Hood College, United StatesReviewed by:
Somnath D. Shinde, Independent researcher, Boston, MA, United StatesZul Ilham, University of Malaya, Malaysia
Meng Wang, Beijing University of Chemical Technology, China
Copyright © 2022 Stoklosa, Latona and Johnston. This is an open-access article distributed under the terms of the Creative Commons Attribution License (CC BY). The use, distribution or reproduction in other forums is permitted, provided the original author(s) and the copyright owner(s) are credited and that the original publication in this journal is cited, in accordance with accepted academic practice. No use, distribution or reproduction is permitted which does not comply with these terms.
*Correspondence: Ryan J. Stoklosa, cnlhbi5zdG9rbG9zYUB1c2RhLmdvdg==