- 1Department of Chemical and Biomolecular Engineering, University of California, Irvine, Irvine, CA, United States
- 2Sue & Bill Gross Stem Cell Research Center, University of California, Irvine, Irvine, CA, United States
The inherent self-organizing capacity of pluripotent and adult stem cell populations has advanced our fundamental understanding of processes that drive human development, homeostasis, regeneration, and disease progression. Translating these principles into in vitro model systems has been achieved with the advent of organoid technology, driving innovation to harness patient-specific, cell-laden regenerative constructs that can be engineered to augment or replace diseased tissue. While developmental organization and regenerative adult stem cell niches are tightly regulated in vivo, in vitro analogs lack defined architecture and presentation of physicochemical cues, leading to the unhindered arrangement of mini-tissues that lack complete physiological mimicry. This review aims to highlight the recent integrative engineering approaches that elicit spatio-temporal control of the extracellular niche to direct the structural and functional maturation of pluripotent and adult stem cell derivatives. While the advances presented here leverage multi-pronged strategies ranging from synthetic biology to microfabrication technologies, the methods converge on recreating the biochemical and biophysical milieu of the native tissue to be modeled or regenerated.
Introduction
A stem cell can be broadly defined by its ability to self-renew and differentiate into other cell types. Pluripotent populations, such as human embryonic stem cells (hESCs) or human induced-pluripotent stem cells (iPSCs), inherently have the largest regenerative potential, can be expanded indefinitely, and have the capacity to form any adult tissue. iPSCs, which were first described in 2007, circumvent ethical concerns associated with hESCs as they can be generated from somatic cells via transcriptional reprogramming (Takahashi et al., 2007; Yu et al., 2007). This technological breakthrough enables the generation of cells that have the potential to augment or replace diseased tissue in vivo and has already made clinical headway in the treatment of type 1 diabetes (Shapiro et al., 2021) and macular degeneration (da Cruz et al., 2018). Aside from their potential in a regenerative setting, patient-specific iPSCs pose as a powerful tool to model diseases in vitro, which can lead to personalized medicine (Schwank et al., 2013; Fujimori et al., 2018).
Adult stem cells can also indefinitely self-renew, which allows for homeostatic tissue maintenance and repair after injury. They do however have a lineage-restricted differentiation capacity. Multipotent populations including hematopoietic stem cells (HSCs) have been widely utilized in clinical settings for the treatment of blood disorders (Copelan, 2006). Mesenchymal stem cells (MSCs) also show clinical promise, and over 80 clinical trials are ongoing due to their attractive immune regulatory functions and pleiotropic effects (Clinical Trials, 2021). However, there is limited evidence that MSCs in a regenerative environment differentiate or replace resident cells in settings outside cartilage or bone regeneration. Rather, their therapeutic benefit rests in their pleiotropic bystander phenotypes, namely their ability to modulate the host tissue by secreting bioactive proteins, matrix, and extracellular vesicles (Savukinas et al., 2016; Lukomska et al., 2019). To this end, the expansion of tissue-specific adult stem cells as organoids has garnished enthusiasm as potential cellular therapeutics and has shown promise in the regeneration of a variety of tissues including the skin, intestines, liver, kidney, and pancreas (Toyohara et al., 2015; Cruz-Acuña et al., 2017; Hirsch et al., 2017; Hogrebe et al., 2020; de Koning and Carlotti, 2021; Sampaziotis et al., 2021).
Stem cells and their progeny have exciting therapeutic promise, however their application in a regenerative setting is limited in part by a difficulty in controlling the regulatory factors that drive their maintenance and differentiation for robust in vitro culture and expansion. The stem cell niche is a tissue-specific physiological microenvironment which is specialized to govern the maintenance and function of resident stem cell populations. Stem cells depend on interactions with their niche to survive, proliferate, and maintain homeostasis. This extracellular milieu includes juxtacrine cellular interactions such as gap junctions and Notch-ligand interactions, soluble chemical factors (oxygen tension, growth factors, chemokines, signal proteins), physical cues (topography, shear stress, interstitial flow), and engagement with the extracellular matrix (ECM) (Lane et al., 2014). The three-dimensional (3D) extracellular matrix, organized as a mixture of regulatory proteins, glycosaminoglycans (GAGs), and tethered small molecules, provides the necessary mechanical, architectural, and signaling support needed to sustain stem cell function (Figure 1). Dysregulation of the interplay between stem cells and their microenvironment has been implicated across disease settings, where stem cells no longer harbor the capacity to respond to regenerative cues. Depletion of factors that sustain the stem cell niche has been increasingly recognized to promote aging (Carlson and Conboy, 2007), tissue deterioration, and cancer (Rossi et al., 2008). This is evidenced by a large body of work, showing that heterochronic parabiosis and transplantation can promote the regenerative capacity of aged stem cell populations when put in a “young” niche (Conboy et al., 2005; Rebo et al., 2016; Poulos et al., 2017; Wlodarek et al., 2020). As such, it is critical to develop in vitro model systems that allow controlled insight into the parameters that govern stem cell survival, self-renewal, and differentiation.
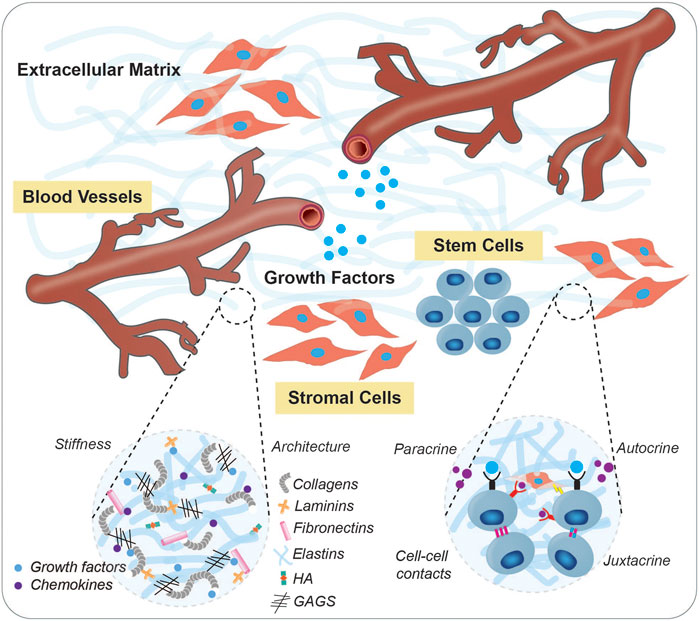
FIGURE 1. The stem cell niche. Stem cells require specialized microenvironments that facilitate their maintenance and functions via structural support, dynamic signaling mechanisms, trophic networks, topographical information, and physiological cues. Shown is a schematic of a typical stem cell niche which includes humoral cue delivering-vascular networks, supporting stroma, extracellular matrix, and soluble factors. Insets depict specific components of the ECM, as well as interactions between neighboring cells.
Various attempts have been made to recreate the native stem cell niche in vitro. Biomaterial-based approaches have been employed to recapitulate the physiological microenvironment and mimic native ECM interplay. The synergy of material science, microfabrication techniques, and cellular engineering have enabled the assembly of artificial niches that can be perturbed with high fidelity, providing new insight into how microenvironmental cues dictate stem cell behavior. Interdisciplinary approaches have been utilized to recapitulate the microenvironmental cues that regulate stem cell maintenance and downstream differentiation. These include the synthesis of novel biomaterial scaffolds, directed co-culture, and introduction of spatial cues. These feats have been realized through the advent of three-dimensional hydrogel culture systems, biological microelectromechanical (Bio-MEMs) devices, and 3D bioprinting. In this review, we discuss recent advancements in controlling the stem niche, with particular focus on iPSC models of early human development, and highlight how engineered biomaterial strategies are being used to study adult stem cell populations.
Engineering the Pluripotent Stem Cell Niche
Aggregation Tools
The 3D organization and components of physiological stem cell microenvironments are critical for the self-renewal and maintenance of stem cells. The in vivo stem cell microenvironment is defined by various factors including the direct interactions with neighboring cells, indirect interactions through autocrine or paracrine secreted factors, extracellular matrix composition, immunological components, physical shear stress, tissue rigidity, fluid flow and environmental exposures (Hall and Watt, 1989; Watt and Hogan, 2000; Lane et al., 2014). With the advent of stem cells, it was established that pluripotent stem cells aggregate in suspension to form embryoid bodies, which can differentiate into any cell type originating from the endoderm, mesoderm, or ectoderm lineage in addition to forming simple cell aggregates called spheroids. Unlike the mammalian embryo, these embryoid bodies or spheroids lack spatial organization, possess inconsistent tissue architecture, and include a variable mixture of cell types (Zeevaert et al., 2020; Rossant and Tam, 2021). Embryoid bodies or spheroids are formed through numerous techniques including non-adherent surfaces, hanging drop method, spinner flasks, rotating wall vessels, concave microwells, programmable droplet merging, micro-molded hydrogels, and microfluidic systems. Over time, these techniques have been developed to overcome some limitations associated with mass production, reproducibility, spheroid size, and shape control. 3D spheroids possess paracrine activity, therefore culturing them on bioreactor-based platforms enables the mimicking of the metabolic, mechanical, and geometric aspects of the biological niche (Chimenti et al., 2017; Cui et al., 2021). Spheroid engineering has been initiated to include multiple cell types, standardized oxygen concentration, defined media supplements, optimal physiochemical cues, and supporting biomaterials. This was done with consideration of the technical feasibility of large-scale production and the physiological relevance of 3D systems (Laschke and Menger, 2017; Tseng et al., 2017; Oliveira et al., 2018; Song et al., 2019; Lee et al., 2020). Hence, spheroids have been incorporated into natural and synthetic biomaterials to enhance the clinical translatability of these model systems by culturing them in appropriate microenvironments.
Novel digital trapping platforms have also been developed leveraging acoustofluidics to spatiotemporally manipulate scaffold-free spheroids in microfluidic or open chambers (Cai et al., 2020; Wu et al., 2021). Spheroids can also be bioprinted to obtain precisely organized arrays (Yu et al., 2016; Daly and Kelly, 2019). While co-cultured spheroids promote intracellular communication, dynamic conditions such as those on microfluidic devices were found to better support these cells (Lee et al., 2013). While conventional methods to generate spheroids can be easily scaled-up, there is limited control over the diverse shapes obtained as well as the size of spheroids produced. More recent techniques have focused on modifying the cell seeding density, using specialized aggregation plates and automated techniques, to obtain large-scale reproducible spheroids in terms of shape and size. Furthermore, most spheroids lack cellular heterogeneity unless they are co-cultured or fused with other spheroids comprised of different cell types. The lack of multicellular and organized structures in spheroids has been addressed to some extent since the generation of self-assembled organoids.
Organoids are complex three-dimensional clusters of organ-specific stem cells comprising of initially homogenous cells that break symmetry, undergo self-assembly and demonstrate functionality (Brassard and Lutolf, 2019). With increased physiological relevance and functionality over their previously generated counterparts, these organoids have established themselves as ideal models for studying cellular interactions, signaling mechanisms, disease modeling, toxicity, and drug testing (Dutta et al., 2017; Lancaster and Huch, 2019; Mead and Karp, 2019). Scaffolds, hydrogels, and micropatterning techniques have been used to mimic the biological niche to expand, differentiate, and mature these cells (Ho et al., 2016; Zhao et al., 2019; Tenje et al., 2020). However, the challenge to control cellular composition and reproducibility in self-organizing organoids remains. Bioprinting approaches do offer an alternative by generating identical structures with multiple cell types in an engineered microenvironment. Although efficient, these processes are mechanically or biochemically guided and do not occur through self-organization. In contrast to micropatterning and microfluidics, obtaining spheroids or organoids from hanging drop, spinner flasks, microwells, magnetic levitation, scaffolds, and hydrogels for further experimental processes is relatively straight forward. Therefore, all these aggregation methods have their own benefits and limitations. In the absence of any supporting matrix in suspension cultures, cells aggregate to form large compact or small loose clusters under the influence of physical forces or spatial proximity. This mainly depends on cell-cell interactions and cellular ECM secretion. Modified microwell shape such as U-bottom and pyramidal culture dishes have demonstrated improved stem cell aggregation as opposed to flat-bottom plates (Thomsen et al., 2018). Although numerous studies have focused on cell density, aggregate size, shape, aggregation techniques, medium composition, and formation kinetics to study the cell expansion, stability, and differentiation of stem cell aggregates, the dynamic process of 3D aggregate formation needs further exploration. It has been suggested that this process is primarily driven by cell adhesion molecules and matrix synthesis and remodeling (Kim et al., 2019). Adhesion molecules including cadherins mediate self-assembly by regulating interactions between cells and integrins and facilitate cellular-ECM protein crosstalk, thereby promoting aggregation (Chen et al., 2007; Weber et al., 2011; Li et al., 2012; Vitillo et al., 2016; Grenier et al., 2021). In addition to providing structural and biochemical support, the diffused ECM (laminin, collagen and fibronectin) between cells in aggregates triggers biomechanical signaling networks between the intracellular cytoskeleton and the extracellular microenvironment, hence regulating the survival, stability, and compactness (Farhadifar et al., 2007; Row et al., 2016; Kim et al., 2019). Depending on the application and desired outcome, the specific cell aggregation techniques can be selected (Figure 2).
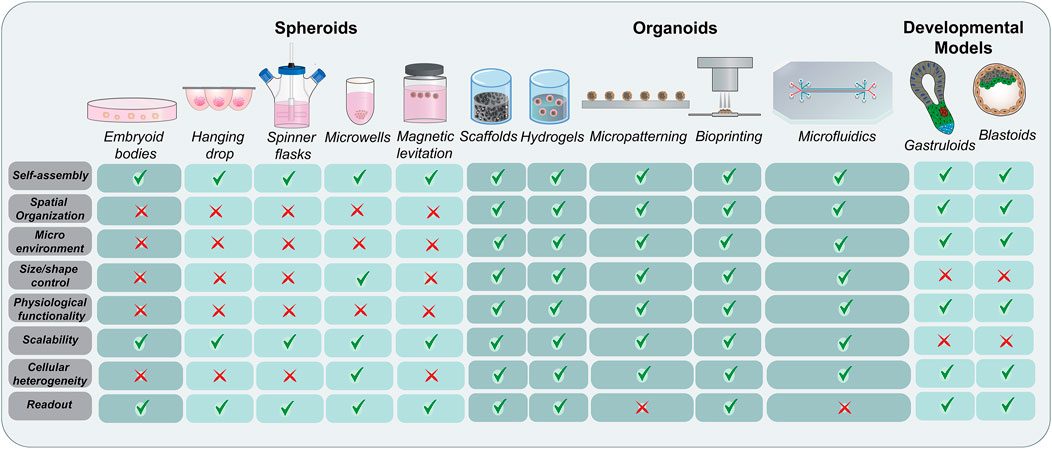
FIGURE 2. Evolution of stem cell organization techniques. A comprehensive schematic representing the methods used for the aggregation and self-organization of stem cells starting with embryoid bodies and spheroids and moving to more complex organoids and developmental models. While the conventional methods rely more on aggregation techniques, the advanced bioengineered models focus on physiological relevance and complex spatial organization. The major advantages and limitations of these techniques have been highlighted.
Synthetic morphogens mimicking the receptor-ligand interactions have been developed in engineered gradient systems to amplify the paracrine cues and enhance cell adhesion (Toda et al., 2020; Stapornwongkul and Vincent, 2021). Adhesion ligands like RGD, YIGSR peptides, or full-length proteins such as laminins have been incorporated into biomaterials to stimulate the adhesion, proliferation, and differentiation of stem cells (Antfolk and Jensen, 2020). In addition, surfaces of biomaterials have been modified with molecules supporting direct cell-cell interactions such as cadherins, selectins, nectin, and contactin to mimic the ECM (Zhang et al., 2020). Another strategy involved the immobilization of cell surface signaling ligands of the Notch pathway in order to direct differentiation of stem cells as well as Shh pathway to stimulate angiogenesis (Beckstead et al., 2006; Taqvi et al., 2006; Wall et al., 2008). Engineering techniques have also led to the development of biomaterials comprising of an extracellular cadherin domain fused to the Fc domain of IgG. Similarly, several other proteins have been conjugated to IgG to modify the surface of biomaterials and promote adhesion, cell growth, differentiation, and cellular matrix mechanosensing (Zhang et al., 2020). Furthermore, the chemical conjugation of cadherin-mimicking peptides to hydrogels has supported stem cell differentiation by replicating cell-cell interactions (Li et al., 2017). While these engineering techniques are useful for improving cell-cell interactions, they can also be leveraged to promote cell-ECM communication.
Mimicking the Extracellular Matrix
Biomaterials can either be natural, synthetic, or composites of both, and have properties that mirror tissue ECM including degradability, viscoelasticity, porosity, hydrophilicity, and stiffness. These materials have a range of malleability and can be functionalized with binding motifs, signaling molecules, and other bioactive moieties. Synthetic materials are desirable for their highly tunable properties and customizability, but often lack the functionality required for cytocompatibility. This bioactivity can be achieved through the chemical addition of niche-specific functional groups and proteins into the polymer system. Polyethylene Glycol (PEG) is a commonly used biocompatible synthetic polymer known for its adjustable rigidity and degradation kinetics. PEG is not biologically active on its own but can be coupled with biochemical factors to improve cell interaction. Considerable work has been done to show that synthetic hydrogel systems can be engineered to recapitulate particular stem cell niches and improve their culture in vitro (Gilbert et al., 2010; Musah et al., 2014; Sun et al., 2018; Chrisnandy et al., 2021). While synthetic materials provide many benefits, there are still advantages to using natural biomaterials for stem cell and organoid culture. Natural matrices such as Matrigel, hyaluronan, chitosan, fibrin, and collagen vary in their exact compositions and are not as readily modifiable as synthetic materials, but they often display improved cytocompatibility and degradability due to the presence of naturally occurring cell adhesion ligands (Wong et al., 2018; Chang et al., 2021; Poorna et al., 2021). These materials are less toxic and typically don’t require harsh chemical reactions for crosslinking or functionalization but can be immunogenic given their derivation from animal sources. While natural biomaterials are not strictly engineered in the way that many synthetic materials are, they can show promise when harnessed in conjunction with other biomaterials and engineered systems such as microfluidic chips or bioprinted constructs. The intrinsic cytocompatibility afforded by natural biomaterials can be critical for the support of pluripotent or multipotent cells within an engineered system, which could be supplemented with specially designed synthetic materials to improve the physical properties (Figure 3).
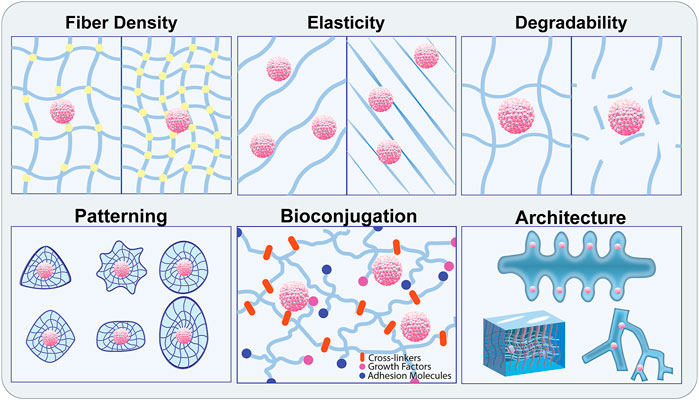
FIGURE 3. Modification of biomaterials to recapitulate the physiological stem cell niche. Biomaterial engineering approaches enable precise control over the biophysical properties of the ECM components leveraged to recreate the physiological stem cell niche. Material properties include fiber density, crosslinking, stiffness, elasticity, and degradability. These can be adjusted individually or in combination with patterning, bioconjugation, and pre-defined architecture to promote specialized organoid assembly and stem cell maintenance.
Biomaterial-assisted engineering techniques ensure that hydrogels provide a protective self-renewal environment. For example, nano- or micro-particles deliver essential bioactive molecules, and functional moieties such as adhesion ligands and immunomodulatory proteins are incorporated to facilitate growth and adhesion. This provides both exterior as well as interior cues (Lee et al., 2021). Other approaches employ biofunctional materials such as magnetic nanoparticles, polymer microspheres, and nanofiber particles, which have accelerated spheroid formation and enhance the mechanical properties, viability, and directed differentiation of stem cells (Kim et al., 2020). Spheroids-on-a chip were generated by combining bioprinting approaches with controlled cell seeding on concave hydrogels (Ling et al., 2015). In addition, engineering techniques using synthetic thermally expandable hydrogels and micropatterned surfaces have derived spheroids by self-assembly of monolayer stem cells to potentiate the interactions between cells and the surrounding ECM (Lee et al., 2018). High precision 3D bio-dot printing has enabled micropatterning and demonstrated improved paracrine interactions between various cell types including hepatocyte spheroids and endothelial cells along with long-term ability to sustain hepatic function and drug metabolism (Jeon et al., 2020). Stiffness-based biomaterial approaches have also been used to improve stem cell culture and differentiation. Identification and modulation of specific signaling cascades driven by resident support cells, growth factors, and adhesion molecules are also crucial in designing physiologically relevant microenvironments for stem cells. For instance, Wnt signaling, a critical pathway for mesodermal induction, is driven by Yes-associated protein (YAP) activation in response to mechanical sensation. Using polydimethylsiloxane (PDMS) as a tunable matrix, nuclear YAP localization was shown to increase when iPSCs were cultured on compliant substrates compared to stiff polystyrene plastic dishes. Additionally, mesodermal markers were upregulated, and endothelial cell specification was improved when iPSCs were seeded on soft substrates, independent of small molecule and growth factor addition (Smith et al., 2017). iPSC derived intestinal stem cell (ISC) maintenance has also been shown to be supported using a 4-arm PEG based hydrogel system, when functionalized with fibronectin (RGD) binding motifs rather than collagen 1 (GFOGER), or laminin a-1 derivatives (AG73, IKVAV) suggesting that specific adhesive moieties are needed in the stem cell niche (Cruz-Acuña et al., 2017).
Naturally occurring biomaterials have also been used to maintain and differentiate pluripotent stem cells. Cellulose, a common non-toxic, biocompatible polymer that is not of animal origin, has been used to investigate the efficiency of hepatocyte-like-cell (HLC) specification from iPSCs in a nanofibril form (Poorna et al., 2021). Expression of endoderm, hepatoblast, and mature hepatocyte markers, including lipid and albumin production, was demonstrated to be similar to culture conditions containing Matrigel (Poorna et al., 2021). Surface coating of chitosan, a polysaccharide derived from the exoskeleton of fungi and crustaceans, improves iPSC proliferation and differentiation potential compared to tissue-culture polystyrene dishes, while maintaining a biocompatible, feeder free system (Wong et al., 2018). When compared to vitronectin, chitosan substrates support high quality iPSC pluripotency and colony proliferation up to a year. Additionally, chitosan membranes support the formation of iPSC derived 3D spheroids and promote a naive-like expression profile. These naive-state iPSCs have a higher capacity for self-renewal, increased differentiation potential, and are optimal for genomic editing compared to traditional monolayer-cultured iPSCs (Weinberger et al., 2016). The recapitulation of 3D organization is an important biomimetic strategy for iPSC culture and has been found to induce improved naive gene expression compared to the vitronectin control (Chang et al., 2021). In addition, synthetic biomaterials such as polyacrylamide gels with amine-functionalization have improved the incorporation of crosslinking agents and biomolecule conjugation. With this system, iPSC attachment and proliferation are enhanced, leading to stiffness-dependent cytoskeletal organization and YAP translocation (Lee S. et al., 2019). While the physiologically relevant microenvironment and cellular interactions facilitate stem cell maintenance, self-renewal, and self-organization, the current spheroid and organoid models provide limited insights on the processes of tissue morphogenesis and embryogenesis.
Gastruloids
Recent innovations have led to the formation of self-organizing embryo-like structures from pluripotent stem cells that can provide insights on embryonic development leading to organogenesis (Shahbazi et al., 2019; Simunovic et al., 2019; Hyun et al., 2020). Gastruloids are three-dimensional aggregates of stem cells possessing axial organization and precisely distributed derivatives of the three germ layers, recapitulating post-implantation embryos in vitro (van den Brink and van Oudenaarden, 2021). A series of lineage decisions coax the embryonic cells to develop into cells from the three germ layers during fetal development. Previous 2D studies using embryonic stem cells or micropatterned pluripotent stem cells exposed to various intracellular signaling microenvironments have successfully recapitulated the early fate decisions and basic cellular rearrangement (Sharon et al., 2011; Warmflash et al., 2014; Etoc et al., 2016; Martyn et al., 2018; Morgani et al., 2018; Smith et al., 2018; Martyn et al., 2019). These micropatterned cultures enhance the understanding of the critical signaling cues. Additionally, early spatial organization, mechanics, epithelial-mesenchymal transition, and geometry influence the developmentally significant cell fate decisions. These include symmetry-breaking and the emergence of the primitive streak and subsequent gastrulation, suggesting that the 3D environment and interacting signaling pathways play an important role in these processes (Gattazzo et al., 2014; Ahmed, 2016; Blin et al., 2018; Xue et al., 2018; Yoney et al., 2018; Britton et al., 2019; Chhabra et al., 2019; Heemskerk et al., 2019; Srivastava and Kilian, 2019). In vivo, the response of embryonic cells to chemical signals from growth factors, morphogens, metabolites, and biomechanical signals including stretch, pressure, and adhesion result in the proper organization of the embryo (Srivastava and Kilian, 2019; Rossant and Tam, 2021). Various studies have attempted to replicate the gastrulation-like events in vitro with external developmental cues and self-organizing embryonic stem cells, iPSCs, and epiblast-like cells (Warmflash et al., 2014; Deglincerti et al., 2016; Shao et al., 2017). Elongated gastruloids consisting of the anterior and posterior-resembling regions have been generated by the mechanistic control of spatio-temporal signaling cues, in addition to modulating the extracellular matrix (Beccari et al., 2018; Moris et al., 2020; van den Brink et al., 2020; Girgin M. U. et al., 2021; Rossi et al., 2021). Recent gastrulation models represent symmetry-breaking epiblast structures (Simunovic et al., 2019). These gastruloid models represent structural changes, patterning, transcriptional signatures, and signaling of the human embryo in developmental stages that are beyond the legal constraints of in vivo human embryos. However, they lack the ability to mimic the pre-gastrulation phase of human development.
Blastoids
Embryonic and extra-embryonic tissue interaction is critical for mammalian embryogenesis to generate spatially organized tissues during development (Harrison et al., 2017; Shao et al., 2017; Rivron et al., 2018; Sozen et al., 2019; Zheng et al., 2019; Baillie-Benson et al., 2020). Pluripotent stem cells have been employed to generate blastocyst-like structures modeling early embryonic development (Liu et al., 2021; Yanagida et al., 2021; Yu et al., 2021). Blastoids are stem cell-derived models of blastocyst-stage conceptus, which recapitulate the early developmental events such as implantation, cell fate determination, and morphogenesis (Kagawa et al., 2021). Blastocyst-derived stem cells in mice demonstrated the ability to form spatially ordered implantable embryoids exhibiting lumenogenesis, expression markers of mesoderm, primordial germ cell precursors, and endoderm-like tissues in controlled microfluidic systems (Zheng et al., 2019). The inability of these structures to develop further has limited their application in developmental biology. Human iPSCs self-organize in an implantation-like niche environment to model the process of amniogenesis in the absence of maternal or extraembryonic cues. These findings reiterate the importance of the physical signals from the niche and show that the activation of BMP signaling cascades is critical in the development of peri-implantation amnion-like tissue (Shao et al., 2017). Since the interaction of embryonic and extraembryonic tissues is known to be crucial in self-organization, recent bioengineered models have led to the independent modulation of the physical as well as biological aspects of these compartments to direct specific trajectory (Girgin M. et al., 2021). CRISPRi techniques have been implicated in modifying cell behavior and controlling symmetry-breaking (Libby et al., 2021). Although various discrete stages are demonstrated in vitro, none of these models mimic the sequential events occurring in human embryonic development. Overall, bioengineering tools promote multicellular interactions and self-organization in in vitro embryo models by the manipulation of embryoid shape, initial cell aggregation, molecular signaling, and extracellular matrix (Fu et al., 2021; Xu et al., 2021). In comparison to the other self-assembled model systems, these embryonic models have emerged as promising platforms, demonstrating an increased level of spatial multicellular organization and similarity to the native tissue developmental trajectory. In addition to providing valuable insights into embryonic development, bioengineering techniques have highlighted the essential components of the adult stem cell niche.
Recreating the Adult Stem Cell Niche
Adult stem cells present exciting opportunities for understanding and recapitulating tissue regeneration and repair. Mimicry of their native niche is an important aspect of properly culturing adult stem cells, and as such requires an understanding of their physiological microenvironment. A combination of biochemical cues as well as biophysical factors play a role in determining cell fate and tissue organization. The ECM provides structural support and initiates biochemical signaling in the cells (Hussey et al., 2018). The following section outlines advances in the realm of adult stem cell niche engineering, with examples of multiple types of adult stem cells and their native niches.
Muscle Stem Cells
Muscle stem cells reside within muscle fibers, adjacent to the basal lamina and sarcolemma. They are accompanied by myocytes, resident inflammatory cells, fibroblasts, and blood vessel mesoangioblasts. Myocytes and inflammatory cells release soluble factors in response to tissue damage which activate muscle stem cells. The stem cells proliferate and differentiate to form new myofibers in addition to remodeling scar tissue laid down by fibroblasts after injury (Kwee and Mooney, 2017). The interplay of cells and chemical/physical cues makes up the regenerative muscle niche. Muscle stem cells (MuSCs) remain quiescent until injury-induced activation. In the native niche, MuSCs maintain physical connections with their surrounding myofibers, mediated by N- and M-cadherin. These connections play an important role in maintaining MuSC quiescence, and evidence has shown that diminishment of these adhesive junctions causes a break in quiescence (Goel et al., 2017). Conversely, local macrophages serve to activate MuSCs at the site of injury. These macrophages have been found to hyper express Adamts1 metalloproteinase which targets NOTCH1 proteins and decreases Notch signaling. Adamts1-positive macrophages have been shown to improve MuSC activation and subsequent muscle regeneration in mice (Du et al., 2017).
A large challenge in adult stem cell culture lies in their propensity to lose the capacity for self-renewal when plated in vitro. Muscle stem cells for example, aid in skeletal muscle regeneration when transplanted in mice directly after isolation. It has been shown that plating MuSCs in plastic dishes causes a decrease in their viability and differentiation potential, also known as “stemness”. Therefore, there is a need for an engineered biomaterial surface which can mimic the native stem cell niche and interact with the cells such that their survival and stem cell properties are not compromised. Elastic moduli have a great impact on the ability of an MuSC population to self-renew and retain their multipotency. The elastic modulus of polystyrene plastic is approximately 106 kPa, whereas skeletal muscle is five orders of magnitude softer. The use of a PEG hydrogel provides control over the stiffness of the substrate while also introducing niche-specific binding ligands. The PEG network can be functionalized with extracellular matrix proteins such as laminin, an important ligand native to the MuSC niche, via covalent crosslinking (Gilbert et al., 2010).
Surface stiffness indeed affects the differentiation capacity and regenerative potential of stem cells. It was found that MuSCs cultured on biomimetic, compliant hydrogels did not experience the previously reported levels of cell death. After 1-week, clonal expansion rate was doubled on soft plates compared to rigid plastic. Gene expression analysis showed that MuSCs cultured on rigid substrates demonstrated increased expression of differentiation markers compared to cells on soft hydrogels. These results indicate that a surface with stiffness closely resembling skeletal muscle can preserve the viability, self-renewal, and stemness of MuSCs. While cell culture on compliant, biomimetic substrates provides a marked improvement over polystyrene plastic, there may still be other niche factors to consider in this system to match the efficacy of freshly isolated stem cells in vivo (Gilbert et al., 2010). This example introduces the importance of mechanical stiffness for stem cell maintenance. It has also been found that MuSC-mediated ECM remodeling and laminin deposition is indispensable for their own expansion and self-renewal post-activation. The cells engage laminin through integrin signaling and depend on matrix metalloproteinase activity to remodel their environment (Rayagiri et al., 2018). Additionally, MuSCs depend on Notch signaling and interactions with native collagen V to maintain quiescence prior to activation (Baghdadi et al., 2018). Several reports outlined in the following sections expand on this work by investigating several adult stem cell types across various tunable hydrogel platforms and other biomaterial-based approaches.
Neural Stem Cells
The neural stem cell niche is localized in the subventricular zone and the dentate gyrus of the brain. These niches house a range of cells, including ependymal cells, transit-amplifying cells, neuroblasts, and astrocytes. These areas of the brain are also supported by a dense vascular network, and many proliferating progenitor cells reside proximal to blood vessels. Because of this proximity to vasculature, neural stem cells are dependent on soluble factors released by vascular endothelial cells for growth and proliferation (Gómez-Gaviro et al., 2012).
Neural cell development and specification are dependent on mechanical factors in addition to endothelial cell-secreted molecules. Soft polyacrylamide (0.7 kPa), functionalized separately with GAG binding peptide and RGD, supported efficient neuronal specification of iPSCs independently of soluble neurogenic factors. YAP is implicated in this process, with non-differentiating cells displaying nuclear localization of YAP on rigid substrates and differentiating cells displaying cytoplasmic YAP on compliant gels. This low-stress mediated YAP inactivation is an important factor in driving neuronal specification from iPSCs (Musah et al., 2014). Interestingly, specified lineage differentiation from human neural stem/progenitor cells (hNSPCs) has been shown to depend on stiff substrates as well. The hNSPCs express Piezo1 stretch-activated calcium channels which play an important role in mechanically induced lineage specification. Cell-mediated traction forces generated in response to substrate stiffness activate Piezo1 channels, which results in increased neuron specification. Inhibition of Piezo1 (pharmacological or soft substrate-induced) reduced neuron formation and improved astrocyte specification (Pathak et al., 2014). Understanding the relationship between mechanical signals and stem cell specification is useful for improving the analysis of mechanisms involving neural cells. For example, iPSC-derived motor neuronal spheroids were harnessed in conjunction with iPSC-derived muscle fibers on a 3D PDMS chip system. This device, supplemented with collagen I and Matrigel, was used to recapitulate the neuromuscular junction and analyze pathological mechanisms associated with neuromuscular diseases such as lateral sclerosis and muscular dystrophy (Osaki et al., 2020). These models exemplify the utility and importance of recapitulating niche factors for clinically relevant research.
Mesenchymal Stem Cells
Bone marrow-derived mesenchymal stem cells (BMMSCs) plated on fibronectin-functionalized polyacrylamide hydrogels displayed high dependence on mechanical stiffness. In this case, BMMSC adhesion, proliferation, and osteogenic expression were improved on gels of higher stiffness which mimicked the mechanics of their native environment (Sun et al., 2018). Mesenchymal stem cell survival and differentiation potential could be modulated by varying the concentration of RGD-functionalized adamantane in β-cyclodextrin hydrogels (Hong and Song, 2019). This further illustrates the importance of niche-specific binding ligands such as RGD and laminin for the propagation of stem cells in culture. Additionally, MSCs have been shown to respond to their mechanical environment and retain a memory of past physical signals when moved to a softer substrate. MSCs cultured in specially designed photocleavable diacrylate-PEG hydrogels retained nuclear YAP localization after induction of in situ matrix softening. This localization was transient, but positively correlated with the amount of culture time in the stiff gel prior to photo-initiated softening. Cells cultured on solely soft gels expressed markers of adipogenic and osteogenic differentiation, while longer periods of mechanical dosing in the stiff gel led to an exclusive increase in osteogenic lineage specification even after matrix softening was initiated (Yang et al., 2014). This illustrates the importance of the mechanical environment for directing MSC fate, as well as their ability to retain information about their mechanical history. Differences in hydrogel crosslinking kinetics can also impact MSC fate. Rapid crosslinking gels made from hyaluronic acid-adamantane bound with RGD facilitated MSC mechanosensation and subsequently promoted osteogenic differentiation. Slow crosslinking hyaluronic acid-cholic acid gels favored adipogenic differentiation as a result of decreased mechanosensation (Yang et al., 2021). These specially designed variable-kinetic hydrogels highlight yet another tunable property which can be leveraged to control stem cell behavior. Viscoelastic hydrogels made from low and high molecular weight alginate were used to subject MSCs to fast and slow stress relaxation, respectively. MSCs in fast relaxing gels displayed an increase in cell volume, mediated by the force-sensitive membrane ion channel TRPV4. This greater volume expansion also correlated with increased osteogenesis, compared to slow relaxing gels which diminished osteogenesis (Lee H.-P. et al., 2019). Viscoelastic and fluid properties not only impact cell fate but are also important to consider for fabrication techniques such as 3D bioprinting. Shear-thinning and self-healing HA-adamantane and HA-cyclodextrin hydrogels support low surface tension bioprinting of MSC spheroids, allowing for improved cell viability and controlled spatial patterning (Daly and Kelly, 2019). This can help with high precision disease and tissue mimicry, building towards highly applicable models for the translation from lab to clinic.
Hematopoietic Stem Cells
Hematopoietic stem cells differentiate and supply the body with all of the immune cells and cells of the blood. HSCs reside in the trabeculae of long bones within the bone marrow. These stem cells are supported by a range of signal-secreting niche cells including osteoblasts, endothelial cells, and mesenchymal stem cells. Additionally, ligand engagement afforded by ECM proteins such as collagen I, fibronectin, and laminin is indispensable for in vivo HSC development, proliferation, and lineage specification. HSCs offer promising therapeutics to patients with immune or hematologic disorders but are limited in number and can be difficult to transplant. Additionally, it has been shown that culture of HSCs in vitro, outside of the bone marrow niche, is very challenging (Zhang et al., 2019). Thus, there is a need for an engineered platform to recapitulate aspects of the native niche to improve HSC culture in vitro.
A bio-inspired polyacrylamide system was developed to investigate the effects of ECM engagement and biophysical properties on HSCs cultured in vitro. The gels were made to consist of regions with a combination of 3.7 and 44 kPa stiffnesses, to mimic the spatially variable stiffness of the native bone marrow niche. The surface was coated with combinations of three important ECM ligands, collagen I, fibronectin, and laminin. It was found that stiff, fibronectin-rich substrates maintained hematopoietic myeloid progenitor populations. Softer, vascular-like zones rich with laminin promoted differentiation towards the erythrocyte lineage (Choi and Harley, 2017). These results indicate that varying combinations of stiffness and ECM engagement can have a profound effect on the behavior of HSCs in vitro and gives further insight into how these cells can be adapted for systems outside of their native niche. Another hydrogel system leveraged methacrylate-functionalized gelatin (GelMA) and MSC co-culture to improve in vitro HSC culture. Degradable GelMA hydrogels are susceptible to MSC-driven remodeling which results in a dynamic matrix environment. HSCs cultured in a 1:1 ratio with MSCs had significantly improved maintenance over time, and the stiffest hydrogels (30.06 ± 12.35 kPa) showed the highest percentage of quiescent HSCs compared to HSC monocultures. The stiffest gels initially had low levels of porosity and biotransport, but at the end-point displayed MMP-9 expression, a hallmark of MSC remodeling. These quiescent cells are optimal for long-term homeostasis and clinical applications (Gilchrist et al., 2019). This suggests that niche cells such as MSCs not only support HSC maintenance through paracrine signaling, but also through physiological matrix remodeling.
Further study into the niche-supportive role of MSCs revealed a downregulation of niche factors following in vitro culture, which contributes to a reduced capacity for HSC maintenance. MSCs were revitalized with five niche-restoring transcription factors (identified via RNA-sequencing) through lentiviral gene transduction. Reprogrammed MSCs expressed HSC niche factors and promoted a sevenfold increase in functional HSC expansion compared to control MSCs in vitro. Culture with reprogrammed MSC-conditioned media yielded a similar increase in HSC expansion, indicating that the mechanism by which these MSCs support HSCs is largely mediated by soluble factors (Nakahara et al., 2019). These results highlight the importance of MSC-secreted factors and demonstrate an innovative approach to improving the supportive capacity of niche cells in vitro.
These studies show remarkable potential for HSC niche recapitulation in vitro which can be tuned to control stem cell maintenance and lineage specification. Other methods such as perfusion-integrated bone marrow-on-a-chip microfluidic systems show promise in combining many aspects of the native niche. These include ECM and cell-cell engagement, circulation, 3D organization, and stromal cell support (Aleman et al., 2019). The complex arrangement of these factors in a single organ-on-a-chip model allows for better mimicry of the in vivo environment and can be highly beneficial for improving our understanding of hematopoietic stem cell behavior in vitro.
Facultative Liver Stem Cells
The liver possesses a unique regenerative capacity, and yet does not house a well-defined nor agreed upon population of resident stem cells. Evidence has shown that differentiated cells of the liver display plasticity in response to injury and mediate regeneration rather than adult stem cells. It has been proposed that oval cells, a type of potential liver progenitor cell, can promote liver regeneration in response to injury. Oval cells were shown to generate hepatocytes and biliary epithelial cells in vitro, however there is not significant evidence indicating that oval cells differentiate into mature hepatocytes in vivo (Lázaro et al., 1998; Li et al., 2020). Recent work has indeed shown that the differentiated cells of the liver play a large role in mediating regeneration in response to various types of liver injury.
Many common adult stem cells express LGR5 and AXIN2 in addition to activating the WNT pathway to promote regeneration in their tissue and maintain their high proliferation (Sun et al., 2020). It has been shown that in the healthy liver, a population of pericentral hepatocytes co-express both LGR5 and AXIN2 (Planas-Paz et al., 2016). This suggests that this zone of cells would act as a liver stem cell/progenitor-like population and would dominate the regeneration effort in response to injury and normal hepatocyte proliferation during homeostasis. However, it was found that these LGR5+AXIN2+ cells did not especially contribute to liver repopulation. Hepatocytes from all zones of the liver proliferated equally to maintain homeostasis, diminishing the idea that any single population of cells is responsible. Furthermore, data shows that during a regeneration phase, all zones of hepatocytes upregulate AXIN2 and proliferate equally to promote liver regrowth (Sun et al., 2020). These findings demonstrate the inherent proliferative flexibility of differentiated hepatocytes and elucidates their role in homeostatic regulation and regeneration.
Additionally, mature hepatocytes have a documented potential for transdifferentiation. Shown in biliary-deficient mice, hepatocytes were targeted through TGF-β signaling and developed into cholangiocytes which formed physiologically active bile ducts (Schaub et al., 2018). Hepatocytes not only have the ability to increase their proliferation in response to injury but have been shown here to convert into another liver cell type when the signaling conditions and need for the cells are present. Hepatocytes are not the only plastic cells in the liver. In cases of severe liver injury hepatocyte regeneration can be impaired, resulting in a need for a new cell source. Using two different models of induced hepatocyte impairment, it was found that biliary cholangiocytes reacted and formed clusters of cholangiocyte-derived hepatocytes (Raven et al., 2017). After the conversion from biliary epithelial cells in response to severe liver injury, the resulting cells expressed markers of mature hepatocytes, stored glycogen, and were binucleated. These are all hallmarks of functional hepatocytes (Deng et al., 2018). This ability for both cholangiocytes and hepatocytes, two major functional cells of the liver, to transdifferentiate depending on the specific conditions in the liver further demonstrates the overall plasticity of the tissue. These cellular populations work together and react to their environment to act as the functional stem cells of the liver to maintain homeostasis and drive regeneration.
Self-assembled liver-organoids have been used to study hepatocyte proliferation, transdifferentiation, and the potential existence and function of liver stem cells/oval cells. Clonal organoids derived from small LGR5+ liver cells located near bile ducts after injury were shown to generate hepatocytes in vitro and proliferate into functional tissue once transplanted into a mouse model (Huch et al., 2013). Organoids originating from mature hepatocytes were found to retain hepatocyte expression and mimic in vivo hepatocyte proliferation in response to injury. Additionally, hepatocyte organoids formed transdifferentiated cystic structures under the right conditions and repopulated damaged liver when transplanted into a mouse model (Hu et al., 2018). The culture of functional liver cells in these organoid platforms allows for a more biomimetic environment in vitro and could shed insight on some of the processes which are normally only visible in vivo. These types of approaches can aid in the development of new regenerative therapies and procedures by allowing the cells to develop and self-assemble outside of the body, prior to transplantation.
Putative Lung Stem Cells
The lungs are composed of many types of cells including secretory goblet cells and alveolar epithelial cells. Tissues such as the lungs, which are in contact with the external environment, rely on rapid cellular regeneration to preserve their functionality. Studies have shown that certain differentiated cells such as club cells and type-II alveolar cells can self-renew, but there is speculation that these cells themselves are derived from a resident stem cell population (Rawlins et al., 2009; Barkauskas et al., 2013). These stem cells reportedly reside at bronchioalveolar-duct junctions and are appropriately named bronchioalveolar stem cells (BASCs). The nature of BASCs is under debate, with incomplete evidence to fully describe their role in lung regeneration. Recent RNA-sequencing and lineage tracing studies have found that BASCs activate in response to lung injury and can differentiate into the major cell types of the lung including both type-I and type-II alveolar cells to promote regeneration (Liu et al., 2019). Additionally, while BASCs are shown to regenerate lung airway cells in response to injury, they are only moderately implicated in homeostatic cell turnover (Salwig et al., 2019). This finding fits in with the notion that certain somatic lung cells self-renew to maintain homeostatic conditions, while the resident stem cells are activated in response to tissue damage to rapidly replenish lost somatic cells. These studies provide evidence for the function of bronchioalveolar stem cells in lung regeneration, but further work is required to extensively characterize them and explore approaches for an appropriately engineered ex vivo niche. Focus has also been put on the generation of self-renewing lung cells and organoids derived from human pluripotent stem cells. This work is promising because it allows for the study of lung tissue regeneration in vitro without reliance on adult stem cells isolated from the human lung.
Self-renewing type-II alveolar epithelial cells can be derived from human pluripotent stem cells by mimicking the milestones of their native development. Pluripotent stem cells are directed to the definitive endoderm lineage, which is then specified to anterior foregut endoderm, followed by lung progenitor cells, and terminating at the distal lung alveolar epithelium. Cells at this point can be cultured in Matrigel with growth-factor supplemented expansion media and will form 3D epithelial spheres capable of surviving many passages, secreting surfactant, and responding to immune signals (Jacob et al., 2019). Foregut spheroids derived from a similar stepwise differentiation protocol were shown to give rise to human lung organoids when cultured in Matrigel with FGF10. Organoids cultured for over 2 months developed airway-like structures, multiple lung cell populations, and formed basal cell cystic structures. RNA-sequencing data showed that these organoids express a transcriptional profile similar to that of human fetal lung (Dye et al., 2015). Foregut spheroids similarly grown in Matrigel but with FGF7, CHIR-99021, and ATRA instead of FGF10 generate bud-tip progenitor organoids. These organoids form patterned branch structures and mimic fetal branching morphogenesis. They can be coaxed in vitro via specific growth factors to differentiate into bronchiolar and alveolar epithelial populations (Miller et al., 2019). The implications of these findings are vast, with the in vitro recapitulation of fetal lung structures allowing for the study of various disease states, cellular interactions, and lung regeneration. Instead of relying on resident adult stem cells, these approaches focus on re-engineering the developmental process starting from germ layer specification. While this proves difficult to accomplish, there is a level of tunability to this technique. The exact organoid makeup depends on growth factor conditions and culture time and could likely be further refined using engineered synthetic matrices. Control over developmental progression and results in vitro is a powerful tool for better understanding the processes which affect the native lung niche in injury and disease.
Intestinal Stem Cells
The intestinal stem cell niche harbors a unique architecture which acts to support adult epithelial stem cells and their progeny in a crypt and villus configuration. Active intestinal stem cells reside at the base of the crypts, supported by a plethora of juxtacrine-factor secreting cells. Several of these signaling pathways work in conjunction to support ISC activity, including Wnt, R-spondin, Notch, and BMP. In addition to chemical signaling, there are also important ECM components which exist in the ISC niche such as fibronectin and laminin-III, which provide sites for adhesion and physical signaling that contribute to their viability and proliferation (Sun et al., 2018). Furthermore, cell-cell junctions including tight junctions, adherens junctions, gap junctions, and desmosomes play a critical role in the homeostasis of intestinal epithelia. These junctions form connections between adjacent cells and engage with intracellular cytoskeletal elements, thereby forming an essential part of the niche. These junctions facilitate barrier support, mechanical integrity, and signal transduction, thereby improving cellular survival, proliferation, and migration (Garcia et al., 2018).
The intestinal stem cell niche maintains LGR5+ ISCs such that they self-renew and self-organize into the crypt-villus architecture. Mouse ISC culture was first tested in crosslinked PEG gels containing RGD, laminin-III, collagen IV, hyaluronic acid, and perlecan (cells cultured in PEG gels without niche factors did not proliferate). ISCs cultured in stiffer gels displayed improved proliferation compared to those in soft matrices, with the intermediate (1.3 kPa) stiffness resulting in optimal ISC expansion. Cells grown on 1.3 kPa PEG gels featured mostly nuclear localization of YAP, indicating higher activity (Gjorevski et al., 2016). Stable, non-degradable vinyl sulfone-functionalized 8-arm PEG (sPEG), mixed with dynamic acrylate-functionalized PEG (dPEG), were used to induce controlled dynamic matrix softening to further study the ISC niche and organoid formation. The dPEG additions introduced ester linkages, which undergo hydrolytic cleavage, resulting in sustained matrix softening. In the stable state, gels supported ISC proliferation. This transient support of ISC maintenance transitioned to support intestinal organoid formation once the stiffness dropped to approximately 190 Pa. Immunofluorescence imaging confirmed the presence of differentiated cells and Lgr5+ stem cells in the organoids, a finding typical of native niche development. Additionally, YAP activation was higher and sustained in the softening gels compared to gels of 100% sPEG. Through genome-wide RNA interference in normal and neoplastic epidermal cells, YAP was shown to be a primary regulator of proliferation (Gjorevski et al., 2016). 1 kPa hyaluronan/elastin-based matrices functionalized with RGD were also shown to maintain the undifferentiated state of intestinal epithelial enteroids compared to softer 400 Pa gels (Hunt et al., 2021). Organoid crypt formation is a function of matrix softening evidenced by photocleavable allyl sulfide hydrogels (Hushka et al., 2020). Translating the tissue specific matrisome into synthetic materials highlights nuanced differences in ECM composition and can be tailored to support the expansion of healthy and tumor derived organoids (Below et al., 2021). Intercellular adhesion mediates YAP activation in reconstituted epidermis, and defects in YAP inhibition can contribute to uncontrolled cutaneous squamous cell carcinoma growth (Walko et al., 2017). These studies confirm the important mechanistic role of YAP in mechanosensation and subsequent cellular behavior. Synthetic hydrogel systems with controlled stiffness can modulate YAP and Hippo signaling cascades.
There have since been numerous different approaches to develop hydrogel platforms with tunable degradation and softening profiles. Multi-arm PEG monomers can be used to induce controlled stress relaxation in PEG hydrogels. One monomer is covalently crosslinked using Michael addition, and the other is reversibly linked with triple hydrogen bonding between cytosine moieties. These hybrid PEG gels display stress relaxation characteristics similar to those of Matrigel and soft tissues (Chrisnandy et al., 2021). A co-culture-hydrogel system was developed to investigate the effects of innate lymphoid cells (ILC1) and matrix degradation/deposition on intestinal organoid formation. A metalloproteinase sensitive A4+B4 PEG polymer was used to encapsulate intestinal organoids in addition to ILC1. ILC1 express MMP9, causing an increase in matrix degradation and substrate softening while also inducing fibroblast-mediated ECM deposition and substrate stiffening. Therefore, the balance between ILC1 induced degradation and deposition can be controlled to tune the stiffness in these PEG hydrogels (Jowett et al., 2021). These reports depict the customizable nature of the synthetic hydrogel platform, which make use of a wide array of chemistries and conjugatable biomolecules to support stem cell maintenance and differentiation.
Collectively, substrate mechanics, architecture, and degradation kinetics impact adult stem cell populations. PEG hydrogel systems are a pivotal example of tunable systems that can recapitulate aspects of that natural extracellular matrix. These types of experiments are expandable across different adult stem cell populations and niches, with varying stiffnesses and ligands to support specific tissue types. As exemplified by the MuSCs, optimal engraftment and behavior was achieved with the intermediate stiffness which resembled skeletal muscle. These approaches have been used to study the sensitivity of adult stem cells to substrate mechanics and elucidate the importance of biomimicry in stem cell culture. Further advances in tissue engineering and clinical relevance will require more materials with compound properties as demonstrated by the softening hydrocleavable sPEG/dPEG gels and photocleavable allyl sulfide gels.
Discussion
The directed expansion of single adult stem cells or induced pluripotent stem cells into self-organizing tissue-mimicking organoids or mini-organs has enabled the dissection of critical components of the stem cell niche sufficient to recapitulate the tissue microenvironment. These 3D organoids represent their in vivo counterparts in terms of cellular diversity, architectural organization, and function (Clevers, 2016). Also, the regulated generation of stem cell-based organoids requires appropriate external stimuli from other cells as well as the extracellular tissue microenvironment (Hoang and Ma, 2021). The development of organoid models has overcome the major limitations of earlier models with regard to the lack of organization and functionality, while enhancing our understanding of the 3D organization of stem cells in vitro and disease progression. In addition, the classic scaffold-based tissue engineering techniques are not on par with the level of tissue organization and functionality obtained by self-organized organoids. At the same time, complete reliance on autonomous cell organization results in varied organoid shape, size, and cell composition. Recent reports point towards the engineering of the self-organization process through spatiotemporal control of the intercellular and cell-ECM interactions (Brassard and Lutolf, 2019). Manipulating the initial culture conditions such as the cell type, pluripotency state, degree of aggregation, geometry, cellular density, and engineering the ECM microenvironment is one aspect of organoid engineering. Other points of control include directed symmetry breaking through the control of intrinsic and extrinsic physicochemical cues, imposing biophysical boundaries, biochemical signaling, and systemic stimuli to guide the process of self-patterning. In addition to these techniques, manipulation of endogenous stem cell genes by targeted genome editing via CRISPR or TALEN tools not only enhances niche function but also directs the self-assembly, disease modeling, and regulation of organoid structures (Schwank et al., 2013; Matano et al., 2015; Broutier et al., 2016; Guye et al., 2016) (Woo et al., 2016; Yin et al., 2016; Fujii et al., 2019; Fleischer et al., 2020). These genome-editing techniques are usually used to knock-down or knock-in specific genes or for correction of disease-causing genes. In some cases, using synthetic biology, genetic circuits are integrated to cause multicellular responses and self-organization (Artegiani et al., 2020; Hofer and Lutolf, 2021) (Figure 4).
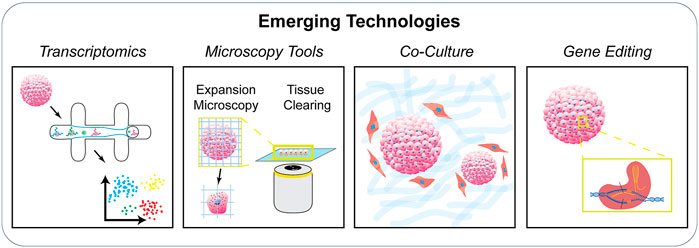
FIGURE 4. Emerging technologies to study the stem cell niche. There is an increasing need to characterize stem cell populations organized in engineered systems. Techniques including single cell transcriptomics, advanced microscopy techniques, co-culture systems, and gene editing tools provide new avenues to study stem cells in controlled niches.
Characterizing the resulting populations in engineered stem cell niches is also a challenge. For example, tissue clearing and expansion allows 3D characterization of entire immunolabeled organoids which has been applied to a wide range of organoid types including colonic, intestinal, breast tumor, hepatic, and airway organoids (Dekkers et al., 2019; Wassie et al., 2019). Single-cell RNA sequencing has shown to be a promising tool to not only uncover the cellular and molecular aspects of the stem cell niche but also to identify rare cell populations. Developments in single-cell genomics facilitates identification of transcription factor combinations and microenvironmental signals, while enabling reverse engineering of complex tissues to track and understand differentiation trajectories (Camp et al., 2018; Meistermann et al., 2021). However, to exploit the complete potential of such high throughput technologies, the capture efficiency, sequence coverage, sequencing depth, and overall experimental cost-effectiveness must be appropriately improved. Although the current single-cell RNA sequencing data provides insights into the niche architecture, the collective information involving multi-cellular crosstalk that drive processes in the stem cell niche can only be achieved by developing techniques simultaneously combining data extracted from multiple levels such as the genomic, protein, and spatial levels as well as effective computational analysis (Crosse et al., 2020; Savulescu et al., 2020; Tikhonova et al., 2020). Based on single-cell RNA sequencing-derived molecular maps, spatially resolved transcriptomics data enables the cellular and spatial allocation of niche factors along with knowledge of intracellular interactions (Baccin et al., 2020). Other current approaches also involve coupling single-cell RNA sequencing with multiplexed fluorescent in situ hybridization techniques that allow the mapping and counting of numerous RNA species, thereby elucidating both the spatial organization and transcriptional profile (Lu et al., 2021). Apart from the characterization of all the cell types located in the niche, the estimation of the exact proportions of each cell type is another important aspect that needs to be addressed in order to accurately recreate the stem cell niche. Given the dynamic nature of most tissues, this indeed becomes difficult. To achieve this, recent studies have adopted an untargeted approach in contrast to previous single-cell RNA sequencing wherein marker-based preselection of cell types occurred. Genome-wide expression profiling can be used to cluster cell types based on their transcriptome similarity and specific cell populations can be identified based on multiple gene sets. Furthermore, such approaches provide additional information regarding changes in cell populations, proliferation potential, signaling pathways and cell-type specific mutations (Zywitza et al., 2018). It is currently possible to synchronously visualize and computationally map stem cells on an organ-wide scale with high resolution (Gomariz et al., 2020). Also, stem cell niches can be comprehensively visualized by incorporating the latest tissue clearing methods with advanced high resolution imaging techniques such as expansion microscopy (Gallagher and Zhao, 2021; Nowzari et al., 2021).
Organoid size and long-term maintenance will be possible through the introduction of perfusable vasculature using microfluidics. Another limitation of organoids is associated with their maturity, which can be overcome by patterning essential physical, chemical, or biological cues. It has also been reported that microthermoformation of these structures can optimize the gradients of the endogenous as well as the exogenous cues (Sthijns et al., 2019). However, owing to their microscale nature, organoid structures are unable to reproduce the spatial organization of complex organs. The high level of heterogeneity in organoids can be addressed via specialized methods of aggregation or by surface engineering the cell membrane to promote clustering. Peptides, proteins, nanoparticles, polymers, or bio-orthogonal chemical species are introduced to the cell membranes through liposomal delivery or electrostatic forces. Membrane-binding nanoparticles and synthetic DNA fragments are other methods that have been widely used for the same purpose (Hofer and Lutolf, 2021). Therefore, there is a need for advanced engineered models that can recapitulate the biological niche as well as spatio-temporal structural organization.
Biomaterials, both natural and synthetic, represent an important arm of recapitulating the stem cell niche. They are harnessed for iPSC and adult stem cell culture and have been shown in different conformations to support both differentiation and maintenance of stemness. Also, since the biophysical properties of the material used to recreate the biological niche affect the stemness, developmental stages, and maturation of these cells, an improved understanding of the signaling mechanisms and complete niche components is imperative to design specialized biomaterials to accommodate different types of cells at various stages. A plethora of different materials have been described, ranging from synthetic materials such as polyethylene glycol and polyacrylamide to natural ones such as chitosan and cellulose. Specially engineered synthetic biomaterials such as sPEG/dPEG and light sensitive allyl sulfide boast superior mechanical tunability but rely on biomolecule additions such as RGD, laminin, GAG, collagen, and others to adequately support cellular adhesion and proliferation. A challenge with natural materials lies in overcoming their often-subpar mechanical properties. One potential solution depends on specific blends between synthetic and natural materials or even between two natural materials. Silk fibroin is a protein derived from silkworm cocoons which has excellent mechanical strength and customizability but lacks cytocompatibility. Gelatin, another common natural material, lacks mechanical stability but does support cell adhesion and proliferation. Blends of silk and gelatin (75/25 and 50/50) supported adhesion and survival of rat mesenchymal stem cells. These gels also promoted MSC differentiation to osteoblasts following induction with osteogenic medium by providing differing levels of stiffness depending on the ratio of the two materials (Luetchford et al., 2020). These results show the promise of harnessing blends of natural materials to improve their mechanical tunability.
Another approach to engineering materials with controllable properties is to incorporate stimuli responsive hydrogels. These are materials whose compositions are specifically engineered to react upon induction of a certain external stimulus. This reaction subsequently alters the physical properties of the gel, where both stiffening and softening are possible as a result. Temperature sensitive hydrogels contain polymers with certain chemical groups which are hydrophobic at high temperatures and hydrophilic at low temperatures. The binding or lack thereof with water alters the conformation of the polymer and impacts the compressive modulus. Alternatively, glucose-responsive, and other ligand-based hydrogels lose crosslinking density when glucose or other specified molecules are added (Valuev et al., 2011). Finally, pH sensitive hydrogels gain and lose hydrogen atoms depending on the pH of the surrounding solution, and the resulting change in electrostatic repulsion and hydrostatic pressure impact the expansion and physical properties of the gel (Cha et al., 2012). While these specialized hydrogels do present a way to reversibly alter the mechanical properties of the gel without degradation or covalent additions, they are not without their own limitations. One major challenge is the consideration that external stimuli may be harmful to or otherwise affect the cells which are cultured on or within the gel. When performing carefully controlled experiments, introducing an additional stimulus can possibly confound the results by causing unwanted cellular behavior or at worst, cell death. Cells commonly thrive within a tight range of pH and temperature, and significant alterations to this can be detrimental. Similarly, certain cells may respond to glucose or other molecular additions made to a ligand-sensitive gel.
The literature described herein spans a wide range of specific topics, each contributing differently to the regenerative stem cell niche. Spheroids and organoids give insight into self-assembly and some spatial organization, while complex developmental models such as gastruloids and blastoids give a deeper look into naive tissue architectural mimicry. These particularly shed light on the processes that drive cell fate decisions and precise lineage trajectories. Biomaterials recapitulate native ECM engagement as well as mechanical and chemical cues, which can be combined with other techniques such as microfluidics or bioprinting to create robust, biomimetic models for tissues and disease. Simultaneous multilevel investigation of the niche processes will pave the way for advanced technologies that can dissect out individual niche parameters in real-time and enable the physiological and functional recreation of the stem cell niche. Certainly, future models will need to incorporate multiple biomimetic strategies as researchers continue to study niche interactions and tissue assembly to more closely recapitulate the complex array of signals, cellular heterogeneity, and architecture related to the stem cell niche. Successful clinical applications of stem cells and their derivatives will depend on the integration of these multi-pronged strategies within a singular model, built from the current approaches.
Author Contributions
QS conceived the manuscript. PB, MA, and QS wrote the manuscript.
Funding
QS acknowledges support from the Howard Hughes Medical Institute Hanna Gray Fellowship Program.
Conflict of Interest
The authors declare that the research was conducted in the absence of any commercial or financial relationships that could be construed as a potential conflict of interest.
Publisher’s Note
All claims expressed in this article are solely those of the authors and do not necessarily represent those of their affiliated organizations, or those of the publisher, the editors and the reviewers. Any product that may be evaluated in this article, or claim that may be made by its manufacturer, is not guaranteed or endorsed by the publisher.
References
Ahmed, M., and ffrench-Constant, C. (2016). Extracellular Matrix Regulation of Stem Cell Behavior. Curr. Stem Cell Rep 2, 197–206. doi:10.1007/s40778-016-0056-2
Aleman, J., George, S. K., Herberg, S., Devarasetty, M., Porada, C. D., Skardal, A., et al. (2019). Deconstructed Microfluidic Bone Marrow On‐A‐Chip to Study Normal and Malignant Hemopoietic Cell-Niche Interactions. Small 15, 1902971. doi:10.1002/smll.201902971
Antfolk, M., and Jensen, K. B. (2020). A Bioengineering Perspective on Modelling the Intestinal Epithelial Physiology In Vitro. Nat. Commun. 11 (1), 1–11. doi:10.1038/s41467-020-20052-z
Artegiani, B., Hendriks, D., Beumer, J., Kok, R., Zheng, X., Joore, I., et al. (2020). Fast and Efficient Generation of Knock-In Human Organoids Using Homology-independent CRISPR-Cas9 Precision Genome Editing. Nat. Cell Biol 22, 321–331. doi:10.1038/s41556-020-0472-5
Baccin, C., Al-Sabah, J., Velten, L., Helbling, P. M., Grünschläger, F., Hernández-Malmierca, P., et al. (2020). Combined Single-Cell and Spatial Transcriptomics Reveal the Molecular, Cellular and Spatial Bone Marrow Niche Organization. Nat. Cell Biol 22, 38–48. doi:10.1038/s41556-019-0439-6
Baghdadi, M. B., Castel, D., Machado, L., Fukada, S.-I., Birk, D. E., Relaix, F., et al. (2018). Reciprocal Signalling by Notch-Collagen V-CALCR Retains Muscle Stem Cells in Their Niche. Nature 557, 714–718. doi:10.1038/s41586-018-0144-9
Baillie-Benson, P., Moris, N., and Martinez Arias, A. (2020). Pluripotent Stem Cell Models of Early Mammalian Development. Curr. Opin. Cell Biol. 66, 89–96. doi:10.1016/j.ceb.2020.05.010
Barkauskas, C. E., Cronce, M. J., Rackley, C. R., Bowie, E. J., Keene, D. R., Stripp, B. R., et al. (2013). Type 2 Alveolar Cells Are Stem Cells in Adult Lung. J. Clin. Invest. 123, 3025–3036. doi:10.1172/jci68782
Beccari, L., Moris, N., Girgin, M., Turner, D. A., Baillie-Johnson, P., Cossy, A.-C., et al. (2018). Multi-axial Self-Organization Properties of Mouse Embryonic Stem Cells into Gastruloids. Nature 562, 272–276. doi:10.1038/s41586-018-0578-0
Beckstead, B. L., Santosa, D. M., and Giachelli, C. M. (2006). Mimicking Cell-Cell Interactions at the Biomaterial-Cell Interface for Control of Stem Cell Differentiation. J. Biomed. Mater. Res. A. 79, 94–103. doi:10.1002/jbm.a.30760
Below, C. R., Kelly, J., Brown, A., Humphries, J. D., Hutton, C., Xu, J., et al. (2021). A Microenvironment-Inspired Synthetic Three-Dimensional Model for Pancreatic Ductal Adenocarcinoma Organoids. Nat. Mater. 21 (1), 110–119. doi:10.1038/s41563-021-01085-1
Blin, G., Wisniewski, D., Picart, C., Thery, M., Puceat, M., and Lowell, S. (2018). Geometrical Confinement Controls the Asymmetric Patterning of Brachyury in Cultures of Pluripotent Cells. Development 145, dev166025. doi:10.1242/dev.166025
Brassard, J. A., and Lutolf, M. P. (2019). Engineering Stem Cell Self-Organization to Build Better Organoids. Cell stem cell 24, 860–876. doi:10.1016/j.stem.2019.05.005
Britton, G., Heemskerk, I., Hodge, R., Qutub, A. A., and Warmflash, A. (2019). A Novel Self-Organizing Embryonic Stem Cell System Reveals Signaling Logic Underlying the Patterning of Human Ectoderm. Development 146, dev179093. doi:10.1242/dev.179093
Broutier, L., Andersson-Rolf, A., Hindley, C. J., Boj, S. F., Clevers, H., Koo, B.-K., et al. (2016). Culture and Establishment of Self-Renewing Human and Mouse Adult Liver and Pancreas 3D Organoids and Their Genetic Manipulation. Nat. Protoc. 11, 1724–1743. doi:10.1038/nprot.2016.097
Cai, H., Wu, Z., Ao, Z., Nunez, A., Chen, B., Jiang, L., et al. (2020). Trapping Cell Spheroids and Organoids Using Digital Acoustofluidics. Biofabrication 12, 035025. doi:10.1088/1758-5090/ab9582
Camp, J. G., Wollny, D., and Treutlein, B. (2018). Single-cell Genomics to Guide Human Stem Cell and Tissue Engineering. Nat. Methods 15, 661–667. doi:10.1038/s41592-018-0113-0
Carlson, M. E., and Conboy, I. M. (2007). Loss of Stem Cell Regenerative Capacity within Aged Niches. Aging cell 6, 371–382. doi:10.1111/j.1474-9726.2007.00286.x
Cha, R., He, Z., and Ni, Y. (2012). Preparation and Characterization of thermal/pH-sensitive Hydrogel from Carboxylated Nanocrystalline Cellulose. Carbohydr. Polym. 88, 713–718. doi:10.1016/j.carbpol.2012.01.026
Chang, P.-H., Chao, H.-M., Chern, E., and Hsu, S.-H. (2021). Chitosan 3D Cell Culture System Promotes Naïve-like Features of Human Induced Pluripotent Stem Cells: A Novel Tool to Sustain Pluripotency and Facilitate Differentiation. Biomaterials 268, 120575. doi:10.1016/j.biomaterials.2020.120575
Chen, S. S., Fitzgerald, W., Zimmerberg, J., Kleinman, H. K., and Margolis, L. (2007). Cell-Cell and Cell-Extracellular Matrix Interactions Regulate Embryonic Stem Cell Differentiation. Stem cells 25, 553–561. doi:10.1634/stemcells.2006-0419
Chhabra, S., Liu, L., Goh, R., Kong, X., and Warmflash, A. (2019). Dissecting the Dynamics of Signaling Events in the BMP, WNT, and NODAL cascade during Self-Organized Fate Patterning in Human Gastruloids. Plos Biol. 17, e3000498. doi:10.1371/journal.pbio.3000498
Chimenti, I., Massai, D., Morbiducci, U., Beltrami, A. P., Pesce, M., and Messina, E. (2017). Stem Cell Spheroids and Ex Vivo Niche Modeling: Rationalization and Scaling-Up. J. Cardiovasc. Trans. Res. 10, 150–166. doi:10.1007/s12265-017-9741-5
Choi, J. S., and Harley, B. A. (2017). Marrow-inspired Matrix Cues Rapidly Affect Early Fate Decisions of Hematopoietic Stem and Progenitor Cells. Sci. Adv. 3, e1600455. doi:10.1126/sciadv.1600455
Chrisnandy, A., Blondel, D., Rezakhani, S., Broguiere, N., and Lutolf, M. P. (2021). Synthetic Dynamic Hydrogels Promote Degradation-independent In Vitro Organogenesis. Nat. Mater, 1–9. doi:10.1038/s41563-021-01136-7
Clevers, H. (2016). Modeling Development and Disease with Organoids. Cell 165, 1586–1597. doi:10.1016/j.cell.2016.05.082
Clinical Trials (2021). Gov Mesenchymal Stem Cells [Online]. Available: https://clinicaltrials.gov.
Conboy, I. M., Conboy, M. J., Wagers, A. J., Girma, E. R., Weissman, I. L., and Rando, T. A. (2005). Rejuvenation of Aged Progenitor Cells by Exposure to a Young Systemic Environment. Nature 433, 760–764. doi:10.1038/nature03260
Copelan, E. A. (2006). Hematopoietic Stem-Cell Transplantation. N. Engl. J. Med. 354, 1813–1826. doi:10.1056/nejmra052638
Crosse, E. I., Gordon-Keylock, S., Rybtsov, S., Binagui-Casas, A., Felchle, H., Nnadi, N. C., et al. (2020). Multi-layered Spatial Transcriptomics Identify Secretory Factors Promoting Human Hematopoietic Stem Cell Development. Cell stem cell 27, 822–839. e828. doi:10.1016/j.stem.2020.08.004
Cruz-Acuña, R., Quirós, M., Farkas, A. E., Dedhia, P. H., Huang, S., Siuda, D., et al. (2017). Synthetic Hydrogels for Human Intestinal Organoid Generation and Colonic Wound Repair. Nat. Cell Biol. 19, 1326–1335.
Cui, H., Wang, X., Wesslowski, J., Tronser, T., Rosenbauer, J., Schug, A., et al. (2021). Assembly of Multi‐Spheroid Cellular Architectures by Programmable Droplet Merging. Adv. Mater. 33, 2006434. doi:10.1002/adma.202006434
da Cruz, L., Fynes, K., Georgiadis, O., Kerby, J., Luo, Y. H., Ahmado, A., et al. (2018). Phase 1 Clinical Study of an Embryonic Stem Cell-Derived Retinal Pigment Epithelium Patch in Age-Related Macular Degeneration. Nat. Biotechnol. 36, 328–337. doi:10.1038/nbt.4114
Daly, A. C., and Kelly, D. J. (2019). Biofabrication of Spatially Organised Tissues by Directing the Growth of Cellular Spheroids within 3D Printed Polymeric Microchambers. Biomaterials 197, 194–206. doi:10.1016/j.biomaterials.2018.12.028
de Koning, E. J. P., and Carlotti, F. (2021). Stem Cell-Based Islet Replacement Therapy in Diabetes: A Road Trip that Reached the Clinic. Cell Stem Cell 28, 2044–2046. doi:10.1016/j.stem.2021.11.008
Deglincerti, A., Etoc, F., Guerra, M. C., Martyn, I., Metzger, J., Ruzo, A., et al. (2016). Self-organization of Human Embryonic Stem Cells on Micropatterns. Nat. Protoc. 11, 2223–2232. doi:10.1038/nprot.2016.131
Dekkers, J. F., Alieva, M., Wellens, L. M., Ariese, H. C. R., Jamieson, P. R., Vonk, A. M., et al. (2019). High-resolution 3D Imaging of Fixed and Cleared Organoids. Nat. Protoc. 14, 1756–1771. doi:10.1038/s41596-019-0160-8
Deng, X., Zhang, X., Li, W., Feng, R.-X., Li, L., Yi, G.-R., et al. (2018). Chronic Liver Injury Induces Conversion of Biliary Epithelial Cells into Hepatocytes. Cell stem cell 23, 114–122. e113. doi:10.1016/j.stem.2018.05.022
Du, H., Shih, C.-H., Wosczyna, M. N., Mueller, A. A., Cho, J., Aggarwal, A., et al. (2017). Macrophage-released ADAMTS1 Promotes Muscle Stem Cell Activation. Nat. Commun. 8, 669. doi:10.1038/s41467-017-00522-7
Dutta, D., Heo, I., and Clevers, H. (2017). Disease Modeling in Stem Cell-Derived 3D Organoid Systems. Trends Mol. Med. 23, 393–410. doi:10.1016/j.molmed.2017.02.007
Dye, B. R., Hill, D. R., Ferguson, M. A., Tsai, Y. H., Nagy, M. S., Dyal, R., et al. (2015). In Vitro generation of Human Pluripotent Stem Cell Derived Lung Organoids. elife 4, e05098. doi:10.7554/eLife.05098
Etoc, F., Metzger, J., Ruzo, A., Kirst, C., Yoney, A., Ozair, M. Z., et al. (2016). A Balance between Secreted Inhibitors and Edge Sensing Controls Gastruloid Self-Organization. Dev. Cel. 39, 302–315. doi:10.1016/j.devcel.2016.09.016
Farhadifar, R., Röper, J.-C., Aigouy, B., Eaton, S., and Jülicher, F. (2007). The Influence of Cell Mechanics, Cell-Cell Interactions, and Proliferation on Epithelial Packing. Curr. Biol. 17, 2095–2104. doi:10.1016/j.cub.2007.11.049
Fleischer, A., Vallejo-Díez, S., Martín-Fernández, J. M., Sánchez-Gilabert, A., Castresana, M., Del Pozo, A., et al. (2020). iPSC-Derived Intestinal Organoids from Cystic Fibrosis Patients Acquire CFTR Activity upon TALEN-Mediated Repair of the p.F508del Mutation. Mol. Ther. - Methods Clin. Dev. 17, 858–870. doi:10.1016/j.omtm.2020.04.005
Fu, J., Warmflash, A., and Lutolf, M. P. (2021). Stem-cell-based Embryo Models for Fundamental Research and Translation. Nat. Mater. 20, 132–144. doi:10.1038/s41563-020-00829-9
Fujii, M., Clevers, H., and Sato, T. (2019). Modeling Human Digestive Diseases with CRISPR-Cas9-Modified Organoids. Gastroenterology 156, 562–576. doi:10.1053/j.gastro.2018.11.048
Fujimori, K., Ishikawa, M., Otomo, A., Atsuta, N., Nakamura, R., Akiyama, T., et al. (2018). Modeling Sporadic ALS in iPSC-Derived Motor Neurons Identifies a Potential Therapeutic Agent. Nat. Med. 24, 1579–1589. doi:10.1038/s41591-018-0140-5
Gallagher, B. R., and Zhao, Y. (2021). Expansion Microscopy: A Powerful Nanoscale Imaging Tool for Neuroscientists. Neurobiol. Dis. 154, 105362. doi:10.1016/j.nbd.2021.105362
Garcia, M. A., Nelson, W. J., and Chavez, N. (2018). Cell-Cell Junctions Organize Structural and Signaling Networks. Cold Spring Harb Perspect. Biol. 10, a029181. doi:10.1101/cshperspect.a029181
Gattazzo, F., Urciuolo, A., and Bonaldo, P. (2014). Extracellular Matrix: a Dynamic Microenvironment for Stem Cell Niche. Biochim. Biophys. Acta (Bba) - Gen. Subjects 1840, 2506–2519. doi:10.1016/j.bbagen.2014.01.010
Gilbert, P. M., Havenstrite, K. L., Magnusson, K. E. G., Sacco, A., Leonardi, N. A., Kraft, P., et al. (2010). Substrate Elasticity Regulates Skeletal Muscle Stem Cell Self-Renewal in Culture. Science 329, 1078–1081. doi:10.1126/science.1191035
Gilchrist, A. E., Lee, S., Hu, Y., and Harley, B. A. C. (2019). Soluble Signals and Remodeling in a Synthetic Gelatin‐Based Hematopoietic Stem Cell Niche. Adv. Healthc. Mater. 8, 1900751. doi:10.1002/adhm.201900751
Girgin, M. U., Broguiere, N., Hoehnel, S., Brandenberg, N., Mercier, B., Arias, A. M., et al. (2021a). Bioengineered Embryoids Mimic Post-Implantation Development in Vitro. Nat. Comm. 12 (1), 1–15.
Girgin, M. U., Broguiere, N., Mattolini, L., and Lutolf, M. P. (2021b). Gastruloids Generated without Exogenous Wnt Activation Develop Anterior Neural Tissues. Stem Cel. Rep. 16, 1143–1155. doi:10.1016/j.stemcr.2021.03.017
Gjorevski, N., Sachs, N., Manfrin, A., Giger, S., Bragina, M. E., Ordóñez-Morán, P., et al. (2016). Designer Matrices for Intestinal Stem Cell and Organoid Culture. Nature 539, 560–564. doi:10.1038/nature20168
Goel, A. J., Rieder, M.-K., Arnold, H.-H., Radice, G. L., and Krauss, R. S. (2017). Niche Cadherins Control the Quiescence-To-Activation Transition in Muscle Stem Cells. Cell Rep. 21, 2236–2250. doi:10.1016/j.celrep.2017.10.102
Gomariz, A., Isringhausen, S., Helbling, P. M., and Nombela‐Arrieta, C. (2020). Imaging and Spatial Analysis of Hematopoietic Stem Cell Niches. Ann. N.Y. Acad. Sci. 1466, 5–16. doi:10.1111/nyas.14184
Gómez-Gaviro, M. V., Scott, C. E., Sesay, A. K., Matheu, A., Booth, S., Galichet, C., et al. (2012). Betacellulin Promotes Cell Proliferation in the Neural Stem Cell Niche and Stimulates Neurogenesis. Proc. Natl. Acad. Sci. U S A. 109, 1317–1322. doi:10.1073/pnas.1016199109
Grenier, J. M. P., Testut, C., Fauriat, C., Mancini, S. J. C., and Aurrand-Lions, M. (2021). Adhesion Molecules Involved in Stem Cell Niche Retention during Normal Haematopoiesis and in Acute Myeloid Leukaemia. Front. Immunol. 12, 756231. doi:10.3389/fimmu.2021.756231
Guye, P., Ebrahimkhani, M. R., Kipniss, N., Velazquez, J. J., Schoenfeld, E., Kiani, S., et al. (2016). Genetically Engineering Self-Organization of Human Pluripotent Stem Cells into a Liver Bud-like Tissue Using Gata6. Nat. Commun. 7 (1), 1–12. doi:10.1038/ncomms10243
Hall, P. A., and Watt, F. M. (1989). Stem Cells: the Generation and Maintenance of Cellular Diversity. Development 106, 619–633. doi:10.1242/dev.106.4.619
Harrison, S. E., Sozen, B., Christodoulou, N., Kyprianou, C., and Zernicka-Goetz, M. (2017). Assembly of Embryonic and Extraembryonic Stem Cells to Mimic Embryogenesis In Vitro. Science 356, 1. doi:10.1126/science.aal1810
Heemskerk, I., Burt, K., Miller, M., Chhabra, S., Guerra, M. C., Liu, L., et al. (2019). Rapid Changes in Morphogen Concentration Control Self-Organized Patterning in Human Embryonic Stem Cells. Elife 8, e40526. doi:10.7554/eLife.40526
Hirsch, T., Rothoeft, T., Teig, N., Bauer, J. W., Pellegrini, G., De Rosa, L., et al. (2017). Regeneration of the Entire Human Epidermis Using Transgenic Stem Cells. Nature 551, 327–332. doi:10.1038/nature24487
Ho, S. S., Murphy, K. C., Binder, B. Y. K., Vissers, C. B., and Leach, J. K. (2016). Increased Survival and Function of Mesenchymal Stem Cell Spheroids Entrapped in Instructive Alginate Hydrogels. Stem Cell translational Med. 5, 773–781. doi:10.5966/sctm.2015-0211
Hoang, P., and Ma, Z. (2021). Biomaterial-guided Stem Cell Organoid Engineering for Modeling Development and Diseases. Acta Biomater. 132, 23–36. doi:10.1016/j.actbio.2021.01.026
Hofer, M., and Lutolf, M. P. (2021). Engineering Organoids. Nat. Rev. Mater. 6, 402–420. doi:10.1038/s41578-021-00279-y
Hogrebe, N. J., Augsornworawat, P., Maxwell, K. G., Velazco-Cruz, L., and Millman, J. R. (2020). Targeting the Cytoskeleton to Direct Pancreatic Differentiation of Human Pluripotent Stem Cells. Nat. Biotechnol. 38, 460–470. doi:10.1038/s41587-020-0430-6
Hong, K. H., and Song, S.-C. (2019). 3D Hydrogel Stem Cell Niche Controlled by Host-Guest Interaction Affects Stem Cell Fate and Survival Rate. Biomaterials 218, 119338. doi:10.1016/j.biomaterials.2019.119338
Hu, H., Gehart, H., Artegiani, B., LÖpez-Iglesias, C., Dekkers, F., Basak, O., et al. (2018). Long-term Expansion of Functional Mouse and Human Hepatocytes as 3D Organoids. Cell 175, 1591–1606. e1519. doi:10.1016/j.cell.2018.11.013
Huch, M., Dorrell, C., Boj, S. F., Van Es, J. H., Li, V. S. W., Van De Wetering, M., et al. (2013). In Vitro expansion of Single Lgr5+ Liver Stem Cells Induced by Wnt-Driven Regeneration. Nature 494, 247–250. doi:10.1038/nature11826
Hunt, D. R., Klett, K. C., Mascharak, S., Wang, H., Gong, D., Lou, J., et al. (2021). Engineered Matrices Enable the Culture of Human Patient‐Derived Intestinal Organoids. Adv. Sci. 8, 2004705. doi:10.1002/advs.202004705
Hushka, E. A., Yavitt, F. M., Brown, T. E., Dempsey, P. J., and Anseth, K. S. (2020). Relaxation of Extracellular Matrix Forces Directs Crypt Formation and Architecture in Intestinal Organoids. Adv. Healthc. Mater. 9, 1901214. doi:10.1002/adhm.201901214
Hussey, G. S., Dziki, J. L., and Badylak, S. F. (2018). Extracellular Matrix-Based Materials for Regenerative Medicine. Nat. Rev. Mater. 3, 159–173. doi:10.1038/s41578-018-0023-x
Hyun, I., Munsie, M., Pera, M. F., Rivron, N. C., and Rossant, J. (2020). Toward Guidelines for Research on Human Embryo Models Formed from Stem Cells. Stem Cell Rep. 14, 169–174. doi:10.1016/j.stemcr.2019.12.008
Jacob, A., Vedaie, M., Roberts, D. A., Thomas, D. C., Villacorta-Martin, C., Alysandratos, K.-D., et al. (2019). Derivation of Self-Renewing Lung Alveolar Epithelial Type II Cells from Human Pluripotent Stem Cells. Nat. Protoc. 14, 3303–3332. doi:10.1038/s41596-019-0220-0
Jeon, S., Heo, J. H., Kim, M. K., Jeong, W., and Kang, H. W. (2020). High‐Precision 3D Bio‐Dot Printing to Improve Paracrine Interaction between Multiple Types of Cell Spheroids. Adv. Funct. Mater. 30, 2005324. doi:10.1002/adfm.202005324
Jowett, G. M., Norman, M. D. A., Yu, T. T. L., Rosell Arévalo, P., Hoogland, D., Lust, S. T., et al. (2021). ILC1 Drive Intestinal Epithelial and Matrix Remodelling. Nat. Mater. 20, 250–259. doi:10.1038/s41563-020-0783-8
Kagawa, H., Javali, A., Khoei, H. H., Sommer, T. M., Sestini, G., Novatchkova, M., et al. (2021). Human Blastoids Model Blastocyst Development and Implantation. Nature 601, 1–9. doi:10.1038/s41586-021-04267-8
Kim, M.-H., Takeuchi, K., and Kino-Oka, M. (2019). Role of Cell-Secreted Extracellular Matrix Formation in Aggregate Formation and Stability of Human Induced Pluripotent Stem Cells in Suspension Culture. J. Biosci. Bioeng. 127, 372–380. doi:10.1016/j.jbiosc.2018.08.010
Kim, S. j., Kim, E. M., Yamamoto, M., Park, H., and Shin, H. (2020). Engineering Multi‐Cellular Spheroids for Tissue Engineering and Regenerative Medicine. Adv. Healthc. Mater. 9, 2000608. doi:10.1002/adhm.202000608
Kwee, B. J., and Mooney, D. J. (2017). Biomaterials for Skeletal Muscle Tissue Engineering. Curr. Opin. Biotechnol. 47, 16–22. doi:10.1016/j.copbio.2017.05.003
Lancaster, M. A., and Huch, M. (2019). Disease Modelling in Human Organoids. Dis. Model. Mech. 12, dmm039347. doi:10.1242/dmm.039347
Lane, S. W., Williams, D. A., and Watt, F. M. (2014). Modulating the Stem Cell Niche for Tissue Regeneration. Nat. Biotechnol. 32, 795–803. doi:10.1038/nbt.2978
Laschke, M. W., and Menger, M. D. (2017). Life Is 3D: Boosting Spheroid Function for Tissue Engineering. Trends Biotechnology 35, 133–144. doi:10.1016/j.tibtech.2016.08.004
Lázaro, C. A., Rhim, J. A., Yamada, Y., and Fausto, N. (1998). Generation of Hepatocytes from Oval Cell Precursors in Culture. Cancer Res. 58, 5514–5522.
Lee, H.-P., Stowers, R., and Chaudhuri, O. (2019a). Volume Expansion and TRPV4 Activation Regulate Stem Cell Fate in Three-Dimensional Microenvironments. Nat. Commun. 10, 529. doi:10.1038/s41467-019-08465-x
Lee, J., Lee, S., Ahmad, T., Madhurakkat Perikamana, S. K., Lee, J., Kim, E. M., et al. (2020). Human Adipose-Derived Stem Cell Spheroids Incorporating Platelet-Derived Growth Factor (PDGF) and Bio-Minerals for Vascularized Bone Tissue Engineering. Biomaterials 255, 120192. doi:10.1016/j.biomaterials.2020.120192
Lee, N.-H., Bayaraa, O., Zechu, Z., and Kim, H. S. (2021). Biomaterials-assisted Spheroid Engineering for Regenerative Therapy. BMB Rep. 54, 356–367. doi:10.5483/bmbrep.2021.54.7.059
Lee, S.-A., No, D. Y., Kang, E., Ju, J., Kim, D.-S., and Lee, S.-H. (2013). Spheroid-based Three-Dimensional Liver-On-A-Chip to Investigate Hepatocyte-Hepatic Stellate Cell Interactions and Flow Effects. Lab. Chip 13, 3529–3537. doi:10.1039/c3lc50197c
Lee, S., Stanton, A. E., Tong, X., and Yang, F. (2019b). Hydrogels with Enhanced Protein Conjugation Efficiency Reveal Stiffness-Induced YAP Localization in Stem Cells Depends on Biochemical Cues. Biomaterials 202, 26–34. doi:10.1016/j.biomaterials.2019.02.021
Lee, Y. B., Kim, E. M., Byun, H., Chang, H.-K., Jeong, K., Aman, Z. M., et al. (2018). Engineering Spheroids Potentiating Cell-Cell and Cell-ECM Interactions by Self-Assembly of Stem Cell Microlayer. Biomaterials 165, 105–120. doi:10.1016/j.biomaterials.2018.02.049
Li, L., Bennett, S. A. L., and Wang, L. (2012). Role of E-Cadherin and Other Cell Adhesion Molecules in Survival and Differentiation of Human Pluripotent Stem Cells. Cell Adhes. Migration 6, 59–73. doi:10.4161/cam.19583
Li, R., Xu, J., Wong, D. S. H., Li, J., Zhao, P., and Bian, L. (2017). Self-assembled N-Cadherin Mimetic Peptide Hydrogels Promote the Chondrogenesis of Mesenchymal Stem Cells through Inhibition of Canonical Wnt/β-Catenin Signaling. Biomaterials 145, 33–43. doi:10.1016/j.biomaterials.2017.08.031
Li, W., Li, L., and Hui, L. (2020). Cell Plasticity in Liver Regeneration. Trends Cell Biology 30, 329–338. doi:10.1016/j.tcb.2020.01.007
Libby, A. R. G., Joy, D. A., and Mcdevitt, T. C. (2021). “Engineering the Spatiotemporal Mosaic Self-Patterning of Pluripotent Stem Cells,” in Programmed Morphogenesis (Springer), 105–116. doi:10.1007/978-1-0716-1174-6_8
Ling, K., Huang, G., Liu, J., Zhang, X., Ma, Y., Lu, T., et al. (2015). Bioprinting-based High-Throughput Fabrication of Three-Dimensional MCF-7 Human Breast Cancer Cellular Spheroids. Engineering 1, 269–274. doi:10.15302/j-eng-2015062
Liu, Q., Liu, K., Cui, G., Huang, X., Yao, S., Guo, W., et al. (2019). Lung Regeneration by Multipotent Stem Cells Residing at the Bronchioalveolar-Duct junction. Nat. Genet. 51, 728–738. doi:10.1038/s41588-019-0346-6
Liu, X., Tan, J. P., Schröder, J., Aberkane, A., Ouyang, J. F., Mohenska, M., et al. (2021). Modelling Human Blastocysts by Reprogramming Fibroblasts into iBlastoids. Nature 591, 627–632. doi:10.1038/s41586-021-03372-y
Lu, Y., Liu, M., Yang, J., Weissman, S. M., Pan, X., Katz, S. G., et al. (2021). Spatial Transcriptome Profiling by MERFISH Reveals Fetal Liver Hematopoietic Stem Cell Niche Architecture. Cell Discov. 7, 1–17. doi:10.1038/s41421-021-00266-1
Luetchford, K. A., Chaudhuri, J. B., and De Bank, P. A. (2020). Silk Fibroin/gelatin Microcarriers as Scaffolds for Bone Tissue Engineering. Mater. Sci. Eng. C 106, 110116. doi:10.1016/j.msec.2019.110116
Lukomska, B., Stanaszek, L., Zuba-Surma, E., Legosz, P., Sarzynska, S., and Drela, K. (2019). Challenges and Controversies in Human Mesenchymal Stem Cell Therapy. Stem Cell Int 2019, 9628536. doi:10.1155/2019/9628536
Martyn, I., Siggia, E. D., and Brivanlou, A. H. (2019). Mapping Cell Migrations and Fates in a Gastruloid Model to the Human Primitive Streak. Development 146, dev179564. doi:10.1242/dev.179564
Martyn, I., Kanno, T. Y., Ruzo, A., Siggia, E. D., and Brivanlou, A. H. (2018). Self-organization of a Human Organizer by Combined Wnt and Nodal Signalling. Nature 558, 132–135. doi:10.1038/s41586-018-0150-y
Matano, M., Date, S., Shimokawa, M., Takano, A., Fujii, M., Ohta, Y., et al. (2015). Modeling Colorectal Cancer Using CRISPR-Cas9-Mediated Engineering of Human Intestinal Organoids. Nat. Med. 21, 256–262. doi:10.1038/nm.3802
Mead, B. E., and Karp, J. M. (2019). All Models Are Wrong, but Some Organoids May Be Useful. Genome Biol. 20, 66. doi:10.1186/s13059-019-1677-4
Meistermann, D., Bruneau, A., Loubersac, S., Reignier, A., Firmin, J., François-Campion, V., et al. (2021). Integrated Pseudotime Analysis of Human Pre-implantation Embryo Single-Cell Transcriptomes Reveals the Dynamics of Lineage Specification. Cell Stem Cell 28 (9), 1625–1640. doi:10.1016/j.stem.2021.04.027
Miller, A. J., Dye, B. R., Ferrer-Torres, D., Hill, D. R., Overeem, A. W., Shea, L. D., et al. (2019). Generation of Lung Organoids from Human Pluripotent Stem Cells In Vitro. Nat. Protoc. 14, 518–540. doi:10.1038/s41596-018-0104-8
Morgani, S. M., Metzger, J. J., Nichols, J., Siggia, E. D., and Hadjantonakis, A. K. (2018). Micropattern Differentiation of Mouse Pluripotent Stem Cells Recapitulates Embryo Regionalized Cell Fate Patterning. Elife 7, e32839. doi:10.7554/eLife.32839
Moris, N., Martinez Arias, A., and Steventon, B. (2020). Experimental Embryology of Gastrulation: Pluripotent Stem Cells as a New Model System. Curr. Opin. Genet. Dev. 64, 78–83. doi:10.1016/j.gde.2020.05.031
Musah, S., Wrighton, P. J., Zaltsman, Y., Zhong, X., Zorn, S., Parlato, M. B., et al. (2014). Substratum-induced Differentiation of Human Pluripotent Stem Cells Reveals the Coactivator YAP Is a Potent Regulator of Neuronal Specification. Proc. Natl. Acad. Sci. 111, 13805–13810. doi:10.1073/pnas.1415330111
Nakahara, F., Borger, D. K., Wei, Q., Pinho, S., Maryanovich, M., Zahalka, A. H., et al. (2019). Engineering a Haematopoietic Stem Cell Niche by Revitalizing Mesenchymal Stromal Cells. Nat. Cel Biol 21, 560–567. doi:10.1038/s41556-019-0308-3
Nowzari, F., Wang, H., Khoradmehr, A., Baghban, M., Baghban, N., Arandian, A., et al. (2021). Three-dimensional Imaging in Stem Cell-Based Researches. Front. Vet. Sci. 8, 657525. doi:10.3389/fvets.2021.657525
Oliveira, N. M., Martins-Cruz, C., Oliveira, M. B., Reis, R. L., and Mano, J. F. (2018). Coculture of Spheroids/2D Cell Layers Using a Miniaturized Patterned Platform as a Versatile Method to Produce Scaffold-free Tissue Engineering Building Blocks. Adv. Biosys. 2, 1700069. doi:10.1002/adbi.201700069
Osaki, T., Uzel, S. G. M., and Kamm, R. D. (2020). On-chip 3D Neuromuscular Model for Drug Screening and Precision Medicine in Neuromuscular Disease. Nat. Protoc. 15, 421–449. doi:10.1038/s41596-019-0248-1
Pathak, M. M., Nourse, J. L., Tran, T., Hwe, J., Arulmoli, J., Le, D. T. T., et al. (2014). Stretch-activated Ion Channel Piezo1 Directs Lineage Choice in Human Neural Stem Cells. Proc. Natl. Acad. Sci. USA 111, 16148–16153. doi:10.1073/pnas.1409802111
Planas-Paz, L., Orsini, V., Boulter, L., Calabrese, D., Pikiolek, M., Nigsch, F., et al. (2016). The RSPO-Lgr4/5-Znrf3/rnf43 Module Controls Liver Zonation and Size. Nat. Cell Biol 18, 467–479. doi:10.1038/ncb3337
Poorna, M. R., Sudhindran, S., Thampi, M. V., and Mony, U. (2021). Differentiation of Induced Pluripotent Stem Cells to Hepatocyte-like Cells on Cellulose Nanofibril Substrate. Colloids Surf. B: Biointerfaces 198, 111466. doi:10.1016/j.colsurfb.2020.111466
Poulos, M. G., Ramalingam, P., Gutkin, M. C., Llanos, P., Gilleran, K., Rabbany, S. Y., et al. (2017). Endothelial Transplantation Rejuvenates Aged Hematopoietic Stem Cell Function. J. Clin. Invest. 127, 4163–4178. doi:10.1172/jci93940
Raven, A., Lu, W.-Y., Man, T. Y., Ferreira-Gonzalez, S., O’Duibhir, E., Dwyer, B. J., et al. (2017). Cholangiocytes Act as Facultative Liver Stem Cells during Impaired Hepatocyte Regeneration. Nature 547, 350–354. doi:10.1038/nature23015
Rawlins, E. L., Okubo, T., Xue, Y., Brass, D. M., Auten, R. L., Hasegawa, H., et al. (2009). The Role of Scgb1a1+ Clara Cells in the Long-Term Maintenance and Repair of Lung Airway, but Not Alveolar, Epithelium. Cell Stem Cell 4, 525–534. doi:10.1016/j.stem.2009.04.002
Rayagiri, S. S., Ranaldi, D., Raven, A., Mohamad Azhar, N. I. F., Lefebvre, O., Zammit, P. S., et al. (2018). Basal Lamina Remodeling at the Skeletal Muscle Stem Cell Niche Mediates Stem Cell Self-Renewal. Nat. Commun. 9, 1075. doi:10.1038/s41467-018-03425-3
Rebo, J., Mehdipour, M., Gathwala, R., Causey, K., Liu, Y., Conboy, M. J., et al. (2016). A Single Heterochronic Blood Exchange Reveals Rapid Inhibition of Multiple Tissues by Old Blood. Nat. Commun. 7, 13363. doi:10.1038/ncomms13363
Rivron, N. C., Frias-Aldeguer, J., Vrij, E. J., Boisset, J.-C., Korving, J., Vivié, J., et al. (2018). Blastocyst-like Structures Generated Solely from Stem Cells. Nature 557, 106–111. doi:10.1038/s41586-018-0051-0
Rossant, J., and Tam, P. P. (2021). Opportunities and Challenges with Stem Cell-Based Embryo Models. Stem Cel Rep. 16, 1031–1038. doi:10.1016/j.stemcr.2021.02.002
Rossi, D. J., Jamieson, C. H. M., and Weissman, I. L. (2008). Stems Cells and the Pathways to Aging and Cancer. Cell 132, 681–696. doi:10.1016/j.cell.2008.01.036
Rossi, G., Broguiere, N., Miyamoto, M., Boni, A., Guiet, R., Girgin, M., et al. (2021). Capturing Cardiogenesis in Gastruloids. Cell stem cell 28, 230–240. e236. doi:10.1016/j.stem.2020.10.013
Row, S., Liu, Y., Alimperti, S., Agarwal, S. K., and Andreadis, S. T. (2016). Cadherin-11 Is a Novel Regulator of Extracellular Matrix Synthesis and Tissue Mechanics. J. Cell Sci 129, 2950–2961. doi:10.1242/jcs.183772
Salwig, I., Spitznagel, B., Vazquez-Armendariz, A. I., Khalooghi, K., Guenther, S., Herold, S., et al. (2019). Bronchioalveolar Stem Cells Are a Main Source for Regeneration of Distal Lung Epithelia In Vivo. EMBO J. 38, e102099. doi:10.15252/embj.2019102099
Sampaziotis, F., Muraro, D., Tysoe, O. C., Sawiak, S., Beach, T. E., Godfrey, E. M., et al. (2021). Cholangiocyte Organoids Can Repair Bile Ducts after Transplantation in the Human Liver. Science 371, 839–846. doi:10.1126/science.aaz6964
Savukinas, U. B., Enes, S. R., Sjöland, A. A., and Westergren-Thorsson, G. (2016). Concise Review: The Bystander Effect: Mesenchymal Stem Cell-Mediated Lung Repair. Stem cells 34, 1437–1444. doi:10.1002/stem.2357
Savulescu, A. F., Jacobs, C., Negishi, Y., Davignon, L., and Mhlanga, M. M. (2020). Pinpointing Cell Identity in Time and Space. Front. Mol. Biosci. 7, 209. doi:10.3389/fmolb.2020.00209
Schaub, J. R., Huppert, K. A., Kurial, S. N. T., Hsu, B. Y., Cast, A. E., Donnelly, B., et al. (2018). De Novo formation of the Biliary System by TGFβ-Mediated Hepatocyte Transdifferentiation. Nature 557, 247–251. doi:10.1038/s41586-018-0075-5
Schwank, G., Koo, B.-K., Sasselli, V., Dekkers, J. F., Heo, I., Demircan, T., et al. (2013). Functional Repair of CFTR by CRISPR/Cas9 in Intestinal Stem Cell Organoids of Cystic Fibrosis Patients. Cell stem cell 13, 653–658. doi:10.1016/j.stem.2013.11.002
Shahbazi, M. N., Siggia, E. D., and Zernicka-Goetz, M. (2019). Self-organization of Stem Cells into Embryos: A Window on Early Mammalian Development. Science 364, 948–951. doi:10.1126/science.aax0164
Shao, Y., Taniguchi, K., Gurdziel, K., Townshend, R. F., Xue, X., Yong, K. M. A., et al. (2017). Self-organized Amniogenesis by Human Pluripotent Stem Cells in a Biomimetic Implantation-like Niche. Nat. Mater 16, 419–425. doi:10.1038/nmat4829
Shapiro, A. M. J., Thompson, D., Donner, T. W., Bellin, M. D., Hsueh, W., Pettus, J., et al. (2021). Insulin Expression and C-Peptide in Type 1 Diabetes Subjects Implanted with Stem Cell-Derived Pancreatic Endoderm Cells in an Encapsulation Device. Cell Rep. Med. 2, 100466. doi:10.1016/j.xcrm.2021.100466
Sharon, N., Mor, I., Golan-lev, T., Fainsod, A., and Benvenisty, N. (2011). Molecular and Functional Characterizations of Gastrula Organizer Cells Derived from Human Embryonic Stem Cells. Stem cells 29, 600–608. doi:10.1002/stem.621
Simunovic, M., Metzger, J. J., Etoc, F., Yoney, A., Ruzo, A., Martyn, I., et al. (2019). A 3D Model of a Human Epiblast Reveals BMP4-Driven Symmetry Breaking. Nat. Cell Biol 21, 900–910. doi:10.1038/s41556-019-0349-7
Smith, Q., Chan, X. Y., Carmo, A. M., Trempel, M., Saunders, M., and Gerecht, S. (2017). Compliant Substratum Guides Endothelial Commitment from Human Pluripotent Stem Cells. Sci. Adv. 3, e1602883. doi:10.1126/sciadv.1602883
Smith, Q., Rochman, N., Carmo, A. M., Vig, D., Chan, X. Y., Sun, S., et al. (2018). Cytoskeletal Tension Regulates Mesodermal Spatial Organization and Subsequent Vascular Fate. Proc. Natl. Acad. Sci. USA 115, 8167–8172. doi:10.1073/pnas.1808021115
Song, L., Yuan, X., Jones, Z., Griffin, K., Zhou, Y., Ma, T., et al. (2019). Assembly of Human Stem Cell-Derived Cortical Spheroids and Vascular Spheroids to Model 3-D Brain-like Tissues. Sci. Rep. 9 (1), 1–16. doi:10.1038/s41598-019-42439-9
Sozen, B., Cox, A. L., De Jonghe, J., Bao, M., Hollfelder, F., Glover, D. M., et al. (2019). Self-organization of Mouse Stem Cells into an Extended Potential Blastoid. Dev. Cel. 51, 698–712. e698. doi:10.1016/j.devcel.2019.11.014
Srivastava, P., and Kilian, K. A. (2019). Micro-engineered Models of Development Using Induced Pluripotent Stem Cells. Front. Bioeng. Biotechnol. 7, 357. doi:10.3389/fbioe.2019.00357
Stapornwongkul, K. S., and Vincent, J.-P. (2021). Generation of Extracellular Morphogen Gradients: the Case for Diffusion. Nat. Rev. Genet. 22, 393–411. doi:10.1038/s41576-021-00342-y
Sthijns, M. M. J. P. E., Lapointe, V. L. S., and Van Blitterswijk, C. A. (2019). Building Complex Life through Self-Organization. Tissue Eng. A 25, 1341–1346. doi:10.1089/ten.tea.2019.0208
Sun, M., Chi, G., Li, P., Lv, S., Xu, J., Xu, Z., et al. (2018). Effects of Matrix Stiffness on the Morphology, Adhesion, Proliferation and Osteogenic Differentiation of Mesenchymal Stem Cells. Int. J. Med. Sci. 15, 257–268. doi:10.7150/ijms.21620
Sun, T., Pikiolek, M., Orsini, V., Bergling, S., Holwerda, S., Morelli, L., et al. (2020). AXIN2+ Pericentral Hepatocytes Have Limited Contributions to Liver Homeostasis and Regeneration. Cell Stem Cell 26, 97–107. e106. doi:10.1016/j.stem.2019.10.011
Takahashi, K., Tanabe, K., Ohnuki, M., Narita, M., Ichisaka, T., Tomoda, K., et al. (2007). Induction of Pluripotent Stem Cells from Adult Human Fibroblasts by Defined Factors. cell 131, 861–872. doi:10.1016/j.cell.2007.11.019
Taqvi, S., Dixit, L., and Roy, K. (2006). Biomaterial-based Notch Signaling for the Differentiation of Hematopoietic Stem Cells into T Cells. J. Biomed. Mater. Res. A. 79, 689–697. doi:10.1002/jbm.a.30916
Tenje, M., Cantoni, F., Porras Hernández, A. M., Searle, S. S., Johansson, S., Barbe, L., et al. (2020). A Practical Guide to Microfabrication and Patterning of Hydrogels for Biomimetic Cell Culture Scaffolds. Organs-on-a-Chip 2, 100003. doi:10.1016/j.ooc.2020.100003
Thomsen, A. R., Aldrian, C., Bronsert, P., Thomann, Y., Nanko, N., Melin, N., et al. (2018). A Deep Conical Agarose Microwell Array for Adhesion Independent Three-Dimensional Cell Culture and Dynamic Volume Measurement. Lab. Chip 18, 179–189. doi:10.1039/c7lc00832e
Tikhonova, A. N., Lasry, A., Austin, R., and Aifantis, I. (2020). Cell-by-cell Deconstruction of Stem Cell Niches. Cell stem cell 27, 19–34. doi:10.1016/j.stem.2020.06.013
Toda, S., Mckeithan, W. L., Hakkinen, T. J., Lopez, P., Klein, O. D., and Lim, W. A. (2020). Engineering Synthetic Morphogen Systems that Can Program Multicellular Patterning. Science 370, 327–331. doi:10.1126/science.abc0033
Toyohara, T., Mae, S.-I., Sueta, S.-I., Inoue, T., Yamagishi, Y., Kawamoto, T., et al. (2015). Cell Therapy Using Human Induced Pluripotent Stem Cell-Derived Renal Progenitors Ameliorates Acute Kidney Injury in Mice. Stem Cell Transl Med 4, 980–992. doi:10.5966/sctm.2014-0219
Tseng, T.-C., Wong, C.-W., Hsieh, F.-Y., and Hsu, S.-h. (2017). Biomaterial Substrate-Mediated Multicellular Spheroid Formation and Their Applications in Tissue Engineering. Biotechnol. J. 12, 1700064. doi:10.1002/biot.201700064
Valuev, I. L., Vanchugova, L. V., and Valuev, L. I. (2011). Glucose-sensitive Hydrogel Systems. Polym. Sci. Ser. A. 53, 385–389. doi:10.1134/s0965545x11050099
van den Brink, S. C., Alemany, A., Van Batenburg, V., Moris, N., Blotenburg, M., Vivié, J., et al. (2020). Single-cell and Spatial Transcriptomics Reveal Somitogenesis in Gastruloids. Nature 582, 405–409. doi:10.1038/s41586-020-2024-3
van den Brink, S. C., and van Oudenaarden, A. (2021). 3D Gastruloids: a Novel Frontier in Stem Cell-Based In Vitro Modeling of Mammalian Gastrulation. Trends Cell Biology 31, 747–759. doi:10.1016/j.tcb.2021.06.007
Vitillo, L., Baxter, M., Iskender, B., Whiting, P., and Kimber, S. J. (2016). Integrin-associated Focal Adhesion Kinase Protects Human Embryonic Stem Cells from Apoptosis, Detachment, and Differentiation. Stem Cel. Rep. 7, 167–176. doi:10.1016/j.stemcr.2016.07.006
Walko, G., Woodhouse, S., Pisco, A. O., Rognoni, E., Liakath-Ali, K., Lichtenberger, B. M., et al. (2017). A Genome-wide Screen Identifies YAP/WBP2 Interplay Conferring Growth Advantage on Human Epidermal Stem Cells. Nat. Commun. 8, 14744. doi:10.1038/ncomms14744
Wall, S. T., Saha, K., Ashton, R. S., Kam, K. R., Schaffer, D. V., and Healy, K. E. (2008). Multivalency of Sonic Hedgehog Conjugated to Linear Polymer Chains Modulates Protein Potency. Bioconjug. Chem. 19, 806–812. doi:10.1021/bc700265k
Warmflash, A., Sorre, B., Etoc, F., Siggia, E. D., and Brivanlou, A. H. (2014). A Method to Recapitulate Early Embryonic Spatial Patterning in Human Embryonic Stem Cells. Nat. Methods 11, 847–854. doi:10.1038/nmeth.3016
Wassie, A. T., Zhao, Y., and Boyden, E. S. (2019). Expansion Microscopy: Principles and Uses in Biological Research. Nat. Methods 16, 33–41. doi:10.1038/s41592-018-0219-4
Watt, F. M., and Hogan, a. B. L. M. (2000). Out of Eden: Stem Cells and Their Niches. Science 287, 1427–1430. doi:10.1126/science.287.5457.1427
Weber, G. F., Bjerke, M. A., and Desimone, D. W. (2011). Integrins and Cadherins Join Forces to Form Adhesive Networks. J. Cel. Sci. 124, 1183–1193. doi:10.1242/jcs.064618
Weinberger, L., Ayyash, M., Novershtern, N., and Hanna, J. H. (2016). Dynamic Stem Cell States: Naive to Primed Pluripotency in Rodents and Humans. Nat. Rev. Mol. Cell Biol 17, 155–169. doi:10.1038/nrm.2015.28
Wlodarek, L., Cao, F., Alibhai, F. J., Fekete, A., Noyan, N., Tobin, S. W., et al. (2020). Rectification of Radiotherapy-Induced Cognitive Impairments in Aged Mice by Reconstituted Sca-1+ Stem Cells from Young Donors. J. Neuroinflammation 17, 51. doi:10.1186/s12974-019-1681-3
Wong, C.-W., Chen, Y.-T., Chien, C.-L., Yu, T.-Y., Rwei, S.-P., and Hsu, S.-H. (2018). A Simple and Efficient Feeder-free Culture System to Up-Scale iPSCs on Polymeric Material Surface for Use in 3D Bioprinting. Mater. Sci. Eng. C 82, 69–79. doi:10.1016/j.msec.2017.08.050
Woo, D.-H., Chen, Q., Yang, T.-L. B., Glineburg, M. R., Hoge, C., Leu, N. A., et al. (2016). Enhancing a Wnt-Telomere Feedback Loop Restores Intestinal Stem Cell Function in a Human Organotypic Model of Dyskeratosis Congenita. Cell Stem Cell 19, 397–405. doi:10.1016/j.stem.2016.05.024
Wu, Z., Chen, B., Wu, Y., Xia, Y., Chen, H., Gong, Z., et al. (2021). Scaffold-free Generation of Heterotypic Cell Spheroids Using Acoustofluidics. Lab. Chip 21, 3498–3508. doi:10.1039/d1lc00496d
Xu, P. F., Borges, R. M., Fillatre, J., De Oliveira-Melo, M., Cheng, T., Thisse, B., et al. (2021). Construction of a Mammalian Embryo Model from Stem Cells Organized by a Morphogen Signalling centre. Nat. Commun. 12 (1), 1–22. doi:10.1038/s41467-021-23653-4
Xue, X., Sun, Y., Resto-Irizarry, A. M., Yuan, Y., Aw Yong, K. M., Zheng, Y., et al. (2018). Mechanics-guided Embryonic Patterning of Neuroectoderm Tissue from Human Pluripotent Stem Cells. Nat. Mater 17, 633–641. doi:10.1038/s41563-018-0082-9
Yanagida, A., Spindlow, D., Nichols, J., Dattani, A., Smith, A., and Guo, G. (2021). Naive Stem Cell Blastocyst Model Captures Human Embryo Lineage Segregation. Cell stem cell 28, 1016–1022. e1014. doi:10.1016/j.stem.2021.04.031
Yang, B., Wei, K., Loebel, C., Zhang, K., Feng, Q., Li, R., et al. (2021). Enhanced Mechanosensing of Cells in Synthetic 3D Matrix with Controlled Biophysical Dynamics. Nat. Commun. 12, 3514. doi:10.1038/s41467-021-23120-0
Yang, C., Tibbitt, M. W., Basta, L., and Anseth, K. S. (2014). Mechanical Memory and Dosing Influence Stem Cell Fate. Nat. Mater 13, 645–652. doi:10.1038/nmat3889
Yin, X., Mead, B. E., Safaee, H., Langer, R., Karp, J. M., and Levy, O. (2016). Engineering Stem Cell Organoids. Cell stem cell 18, 25–38. doi:10.1016/j.stem.2015.12.005
Yoney, A., Etoc, F., Ruzo, A., Carroll, T., Metzger, J. J., Martyn, I., et al. (2018). WNT Signaling Memory Is Required for ACTIVIN to Function as a Morphogen in Human Gastruloids. Elife 7, e38279. doi:10.7554/eLife.38279
Yu, J., Vodyanik, M. A., Smuga-Otto, K., Antosiewicz-Bourget, J., Frane, J. L., Tian, S., et al. (2007). Induced Pluripotent Stem Cell Lines Derived from Human Somatic Cells. science 318, 1917–1920. doi:10.1126/science.1151526
Yu, L., Wei, Y., Duan, J., Schmitz, D. A., Sakurai, M., Wang, L., et al. (2021). Blastocyst-like Structures Generated from Human Pluripotent Stem Cells. Nature 591, 620–626. doi:10.1038/s41586-021-03356-y
Yu, Y., Moncal, K. K., Li, J., Peng, W., Rivero, I., Martin, J. A., et al. (2016). Three-dimensional Bioprinting Using Self-Assembling Scalable Scaffold-free "tissue Strands" as a New Bioink. Sci. Rep. 6 (1), 1–11. doi:10.1038/srep28714
Zeevaert, K., Elsafi Mabrouk, M. H., Wagner, W., and Goetzke, R. (2020). Cell Mechanics in Embryoid Bodies. Cells 9, 2270. doi:10.3390/cells9102270
Zhang, P., Zhang, C., Li, J., Han, J., Liu, X., and Yang, H. (2019). The Physical Microenvironment of Hematopoietic Stem Cells and its Emerging Roles in Engineering Applications. Stem Cell Res Ther 10, 327. doi:10.1186/s13287-019-1422-7
Zhang, Y., Qin, Z., Qu, Z., Ge, M., and Yang, J. (2020). Cadherin-based Biomaterials: Inducing Stem Cell Fate towards Tissue Construction and Therapeutics. Prog. Nat. Sci. Mater. Int. 30, 597–608. doi:10.1016/j.pnsc.2020.09.001
Zhao, X., Cui, K., and Li, Z. (2019). The Role of Biomaterials in Stem Cell-Based Regenerative Medicine. Future Med. Chem. 11, 1777–1790. doi:10.4155/fmc-2018-0347
Zheng, Y., Xue, X., Shao, Y., Wang, S., Esfahani, S. N., Li, Z., et al. (2019). Controlled Modelling of Human Epiblast and Amnion Development Using Stem Cells. Nature 573, 421–425. doi:10.1038/s41586-019-1535-2
Keywords: stem cells, tissue engineering, biomaterials, organ-on-chip, development, regeneration
Citation: Barhouse PS, Andrade MJ and Smith Q (2022) Home Away From Home: Bioengineering Advancements to Mimic the Developmental and Adult Stem Cell Niche. Front. Chem. Eng. 4:832754. doi: 10.3389/fceng.2022.832754
Received: 10 December 2021; Accepted: 31 January 2022;
Published: 17 February 2022.
Edited by:
Panagiotis Mistriotis, Auburn University, United StatesReviewed by:
Xiaoping Bao, Purdue University, United StatesNatesh Parashurama, University at Buffalo, United States
Copyright © 2022 Barhouse, Andrade and Smith. This is an open-access article distributed under the terms of the Creative Commons Attribution License (CC BY). The use, distribution or reproduction in other forums is permitted, provided the original author(s) and the copyright owner(s) are credited and that the original publication in this journal is cited, in accordance with accepted academic practice. No use, distribution or reproduction is permitted which does not comply with these terms.
*Correspondence: Quinton Smith, cXVpbnRvbnNAdWNpLmVkdQ==
†These authors have contributed equally to this work