- 1Laboratory of Materials for Renewable Energy (LMER), Basic Science Faculty (SB), Institute of Chemical Sciences and Engineering (ISIC), École Polytechnique Fédérale de Lausanne (EPFL) Valais/Wallis, Sion, Switzerland
- 2Empa Materials Science and Technology, Dübendorf, Switzerland
Methanation of CO2 is an important reaction for reducing CO2 emissions in a power-to-gas system. Compared to cobalt supported on gamma-Al2O3, cobalt supported on graphene nanoplatelets (GNPs) showed significantly better performance for CO2 methanation. Cobalt supported on GNPs was capable of 15% conversion of CO2 to CH4 at temperatures below 250°C, compared to 5% for cobalt supported on Al2O3. In situ thermogravimetric analysis (TGA) demonstrated that the Co/GNP catalyst was stable to 400°C. The maximum catalyst mass-specific CH4 yield was obtained at a Co loading of 5wt% on GNPs; however, high Co loading on GNPs deactivated the reactivity of the Co/GNP catalyst. Transmission electron microscopy (TEM) demonstrated that 5wt% Co/GNPs had the smallest and most dispersed cobalt nanoparticles. Excessive loading of cobalt tended to form isolated large Co nanoparticles. X-ray photoelectron spectroscopy (XPS) and Raman spectrometry revealed that more CoO phases were maintained on the surface of 5wt% Co/GNPs, indicating that the interaction between the Co and the GNPs had more of an impact on cobalt’s redox capacity than did particle size, which ultimately affected cobalt’s active phase during the CO2 reduction process. Furthermore, Raman spectrometry demonstrated that Co loading led to an increase in graphene defects. Higher Co loading on GNPs resulted in fewer interfaces between Co and GNPs due to the agglomeration of Co nanoparticles.
1 Introduction
Researchers agree that global warming is mostly caused by CO2 emissions from excessive use of fossil fuels (Johnsson et al., 2019). Over the decades, processes for CO2 reduction have been extensively studied and tested but with little real progress. For example, CO2 can be converted into other chemicals, such as CH4, CH3OH, and CH3COOH, via various techniques such as thermal, electrochemical, photochemical, biochemical, and plasmatic techniques (Jimenez et al., 2011; Mateo et al., 2018; Ojelade and Zaman, 2021; Pham et al., 2022). Among these, the thermal catalytic methanation process, also known as the Sabatier reaction, has been considered the most competitive CO2 reduction process for commercial application due to the high energy yield and conversion efficiency. In a power-to-gas system, CH4 produced from CO2 can be directly fed into the power plant for producing electricity, reducing the demand for natural gas (Bailera et al., 2017). Commercialization of the CO2 methanation process benefits society not only through the reduction of CO2 but also the replacement of fossil fuel by a renewable energy resource (Bacariza et al., 2020). However, the efficiency of the CO2 methanation process is critically dependent on the activity of the catalyst.
The CO2 conversion rate and CH4 yield are limited by the thermodynamic equilibrium of CO2 methanation at high temperatures; an ideal catalyst should be selective and function at relatively low temperatures (Kuśmierz, 2008; Wang et al., 2016). The transition metals Ru, Rh, Co, and Ni, supported on porous materials such as Al2O3, CeO2, and TiO2, have been reported to be active for CO2 methanation (Frontera et al., 2017). The noble metal ruthenium- and rhenium-based catalysts have shown excellent activity and selectivity over a wide temperature range, but their high market price has stimulated the development of cobalt and nickel catalysts as replacements. Ni/CeO2 has been shown to be comparable to the noble metal catalysts, but only at elevated temperatures, where it tends to be deactivated because of the agglomeration of Ni particles and coking on the catalyst’s surface (Vogt et al., 2018). In contrast, cobalt-based catalysts have demonstrated better reactivity at low temperatures (Ci et al., 2010; Liu et al., 2018; Parastaev et al., 2020; Tu et al., 2021). The active structure of cobalt-based catalysts has been determined to be the CoO phase (ten Have et al., 2022), (Zhao et al., 2020). However, this transition phase tends to be reduced to metallic Co by the reductive mixture of CO2 and H2, leading to studies on stabilizing the active phase for long-term reactivity (Zhong et al., 2019; Zhong et al., 2021a; Zhong et al., 2021b).
In order to maintain the stability of the catalytic CoO phase during the CO2 methanation reaction, it is essential to tune the redox ability of Co. Conventionally, the redox ability of Co can be adjusted by changing particle size, interaction with the support, or with a second promoter (Wigzell and Jackson, 2017; Qi et al., 2020; Rahmati et al., 2020). As reported previously, the insertion of a graphene layer into a Co/ZnO system results in a more reductive surface in an oxidative environment. Graphene is also able to tune Co particles (He et al., 2013; Karimi et al., 2015; Cañón and Teplyakov, 2021). Inspired by those results, we used graphene nanoplatelets as supports for cobalt-based CO2 methanation catalysts. Bulk and surface characterization methods were used to investigate the promotion mechanism of the GNP support. To the best of our knowledge, this is the first time that a carbonaceous material like GNP, has been used as a catalyst support for the thermal CO2 reduction process.
2 Experimental methods
2.1 Materials and catalyst preparation
The Co-based catalysts were prepared by the incipient impregnation wetness method. For the Co/Al2O3 sample, Co(NO)3·6H2O (Alfa Aesar, CAS#10026-22-9) was dissolved in deionized water (DIW). Al2O3 (2 g) was impregnated into the cobalt nitrate solution, and the mixture was dried at 80°C in air. For the Co/GNP samples, the cobalt nitrate was dissolved in a mixture of DIW and ethanol (1:1, vol%) because of the hydrophobic nature of the GNP (Sigma-Aldrich, CAS#7782-42-5) surface. The solution was then applied to the GNPs based on the saturated water absorption of the support, and this was repeated three times. For the gamma Al2O3 support (Jiangxi, Pingxiang, Alibaba.com), the cobalt nitrate was dissolved in DIW and the solution was impregnated three times. After the impregnation step, the samples were dried in air at 80°C for around 12 h. After drying, the as-prepared sample was directly loaded into a fixed-bed reactor. Before starting the CO2 methanation, in-situ reduction of the sample with 5% H2 (by vol) at 400°C for 2 h was performed to anneal the Co precursor to the metallic Co. For characterizations, the samples were reduced in 5% H2 (by vol) balanced with N2 at 400°C for 2 h, and then collected for different measurements.
2.2 Material characterization
The Brunauer–Emmett–Teller (BET) surface area of the supports was obtained by the N2 adsorption–desorption method using a BELSORP MAX II analyzer from Microtract MRB. The loading content of Co in each sample was determined with an Agilent 5110 inductively coupled plasma optical emission spectrometer (ICP-OES). X-ray diffraction patterns were obtained on a Bruker D8 Advance instrument (40 kV, 40 mA, Cu Kα radiation, and λ = 0.154 nm). X-ray photoelectron spectroscopy (XPS) and Raman spectroscopy were used for surface characterization. The XPS spectra of Co 2p were collected under ultra-high-vacuum (UHV) on a Kratos Axis Supra XPS system using the monochromatic Kα line of an aluminum X-ray source (1486.6 eV) with the analyzer set at a pass energy of 20 eV. The deconvolution of the Al 2p peak was completed with CasaXPS software. Raman spectroscopy was obtained under ambient conditions using a 632-nm laser. Scanning electron microscopy (SEM) images were acquired on a Thermo-Fisher Teneo FE-SEM. Transmission electron microscopy images were acquired with Thermo-Fisher Tecnai Osiris (200 kV) and Thermo-Fisher Tecnai Spirit (120 kV) EMs. Thermo-gravimetric analysis (TGA) was performed using a TG 209 F1 Libra instrument by Netzsch.
2.3 Catalytic activity evaluation
The reactivity of catalysts for CO2 methanation was evaluated in a microscale fixed-bed flow reactor. The reactor consists of a 5-cm-long stainless steel tube with an inner diameter of 8 mm for the catalyst bed zone and a temperature control system. The catalyst sample was loaded as pellets with a size of 40–60 mesh. The catalyst bed zone was heated in a furnace with the reaction temperature measured by a K-type thermocouple running through the catalyst bed. For each test, 200 mg of sample pellets were loaded into the reactor and reduced with 10% (by vol) H2 (2 ml/min H2 + 8 ml/min N2) at 400°C for 3 h. After the temperature had cooled to 50°C, the gas mixture was switched to 3 ml/min of CO2, 4.5 ml/min of N2, and 12 ml/min of H2. For each reaction at a specific temperature, a dwell of 2 h was set, after which the outlet gas was analyzed on a gas chromatograph (SRI 83670) with a thermal conductivity detector (TCD). The CO2 conversion (XCO2), CO selectivity (SCO), and CH4 selectivity (SCH4) rate were determined separately by Eqs 1–3:
where CO2, out/in, CH4 out, and COout are the molar concentrations of the reactor inlet/outlet gas stream. For each gas, the molar concentration was determined by external calibration curves with standard gases. For correction of the total volume change from inlet to outlet due to the reaction, N2 was used as an internal standard calibration gas. In order to compare the intrinsic activity of Co in each sample, we have calculated the Co mass-specific CH4 formation rate by Eq. (4):
where Vm is the molar volume of ideal gas at standard conditions, which is 22.4 L/mol, while mcatalyst is the mass of the catalyst loaded for each test. Co% is the cobalt content in weight percentage, as defined by ICP-OES analysis.
3 Results and discussion
3.1 Catalytic reactivity
The reactivity of supported Co catalysts for the hydrogenation of CO2 to methane is shown in Figure 1. For the same Co loading (5wt%), Co supported on GNPs exhibited a much higher CO2 conversion rate than on Al2O3. For example, only 5% CO2 conversion is obtained with 5wt% Co/Al2O3 at 235°C, while the conversion rate is 15% for 5wt% Co/GNPs. Unexpectedly, the increased Co loading on GNPs did not equate to a comparable rise in CO2 conversion. Much less CO2 was converted by 10wt% Co/GNPs than by 5wt% Co/GNPs. Figure 1B also compares the Co mass-specific CH4 production of four catalysts at kinetic-dominated temperatures to directly assess the intrinsic reactivity of Co in each sample. The Co mass-specific yield of CH4 of 10wt% Co/GNPs was lower than that of 5wt% Co/Al2O3. The results clearly indicate that a catalyst with 5wt% Co supported on GNPs was the most reactive, while the 10wt% Co loaded on GNPs had the lowest reactivity. The difference in mass-specific reactivity can likely be attributed to the different active phases of Co in each sample. The characteristics of this Co phase are revealed in the following results and analysis.
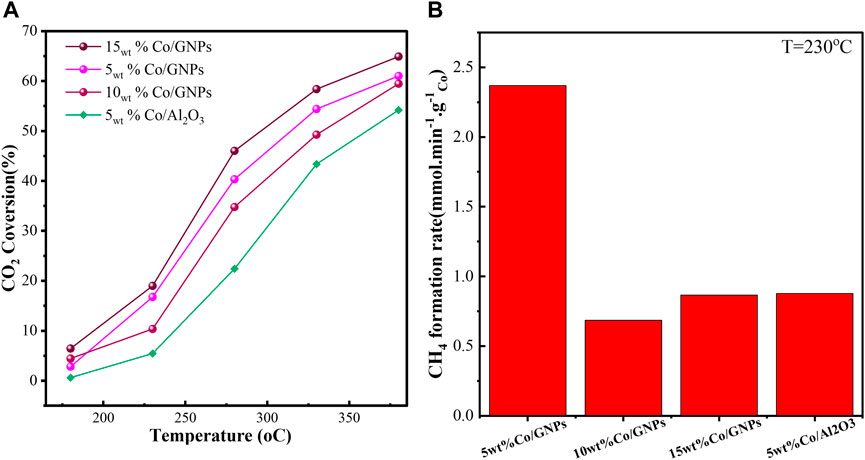
FIGURE 1. CO2 conversion of catalysts at different temperatures (A) and the mass-specific CH4 formation rate of cobalt in each catalyst at 230°C (B). Reaction conditions: 1 bar, 19.5 ml/min total flow rate with reaction mixtures of 15vol% of CO2, 60vol% of H2, and 25vol% of N2.
3.2 Bulk and morphology properties
To investigate the intrinsic effects of GNPs on Co catalysis for CO2 conversion, we tested commercial GNPs and compared them to porous Al2O3 supports with the same surface area (300 m2/g) as a reference. The BET surface area was determined, and results can be found in supporting information (Supplementary Table S1; Supplementary Figure S1). The porous Al2O3 material is commonly used as a support in commercial and industrial-scale operations for fabricating supported-metal catalysts because of its relatively low price and compatibility with different application conditions. Even though other reducible oxides, like CeO2, ZrO2, and ZnO, have been reported as promising supports for cobalt-based CO2 methanation, the intrinsic reactivity of those oxides for CO2 conversion complicates the determination of the specific influences of the cobalt–support interaction. In this study, the selected pure Al2O3 and GNPs are both inert in the CO2 methanation reaction at the tested temperatures. The Co content in the tested catalysts was confirmed by IPC-OES, and all the catalysts had the designated Co content (Table 1). The small discrepancies between nominal content and content in synthesized samples were probably due to deviations occurring during synthesis.
SEM-EDX mapping results (Figure 2) demonstrate that the 5wt% of Co applied is distributed uniformly onto both Al2O3 and GNP supports. Only a few regions in the map show a heavier Co deposit, indicating that most Co clusters were resistant to agglomeration during reduction at 400°C. TEM images (Figure 3) of Co/GNP samples show that increasing Co loading to 15wt% leads to formation of large Co aggregates. Cobalt particles of about 1 nm are formed and are well dispersed in the 5wt% Co/GNP sample, but their average size rose to 3.8 nm in the 10wt% Co/GNP sample. Large cobalt particles up to 30 nm were formed when Co content was increased to 15wt%. Large particles typically have a relatively low surface area, which results in fewer sites for reaction. This may help explain why larger loading results in a lower metal-mass-specific yield of CH4. Although the sample with 10wt% loading had smaller cobalt particles than that with 15wt% loading, which is believed to have a higher active surface area of Co, it exhibited lower CH4 yield than the 15wt% catalyst. The discrepancy between the Co surface area and reactivity may be explained by the finding, which is supported by numerous studies, that the catalytic reactivity of Co is dependent on its particle size. In those investigations, it was discovered that the size of Co affects its redox capacity and defines the active phase during reaction (Ci et al., 2010; Sadasivan et al., 2013; Torshizi et al., 2021).
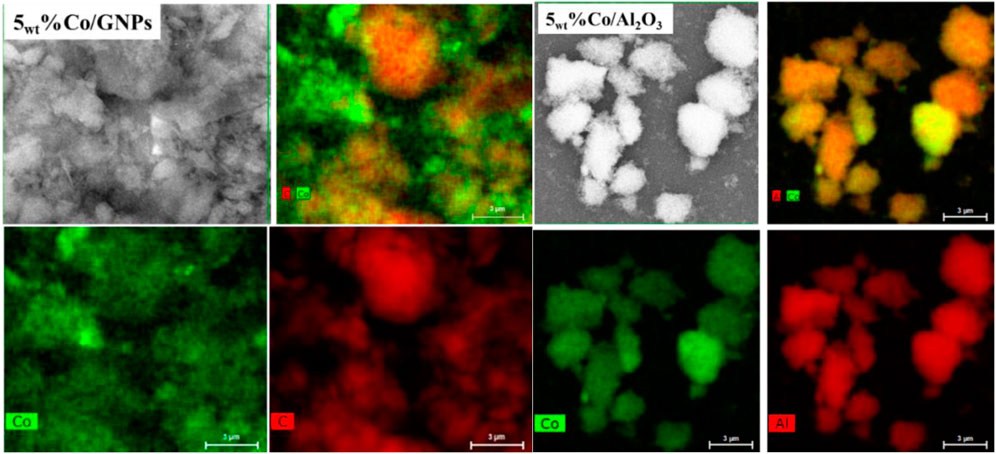
FIGURE 2. Representative SEM-EDX mapping images of cobalt supported on GNPs (left) and Al2O3 (right) after reduction by H2.
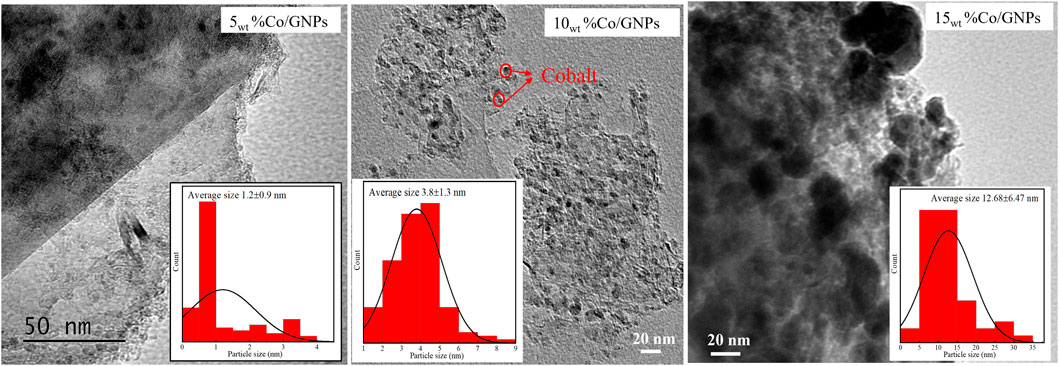
FIGURE 3. TEM images and cobalt particle size distribution of Co/GNP samples after reduction at 400°C by H2.
Figure 4 shows the crystalline structure of Co and GNPs. A wide peak around 2θ = 36° is attributed to the CoO (220) crystalline phase (PDF#00-048-1719) in all Co/GNP samples. The Co3O4 phase (PDF#04-001-8014) was only detected in the 15wt% Co/GNPs, while the metallic Co crystalline phase was found in both the 10wt% Co/GNPs and 15wt% Co/GNPs. Although all samples were reduced under the same conditions, they were oxidized during the ex situ measurement and transition due to exposure to air. The differences in the crystalline phase of Co in the three samples indicate that its redox ability might be different, suggesting some mechanistic aspects of the different Co active sites. This redox ability is dependent on Co particle size and interactions between Co and the GNP support (Sadasivan et al., 2013). It has been reported that smaller Co metal particles were oxidized more readily than bigger particles. According to TEM size analysis, the 5wt% Co catalyst had the smallest particle size. Although it was oxidized under the same conditions, the XRD pattern showed no Co3O4 phase in this sample. In addition to particle size, the interaction between the Co and the support plays a key role in determining its redox activity. We hypothesized that the metal–support interaction plays an essential role in Co’s redox ability, eventually affecting the Co active phase for CO2 methanation.
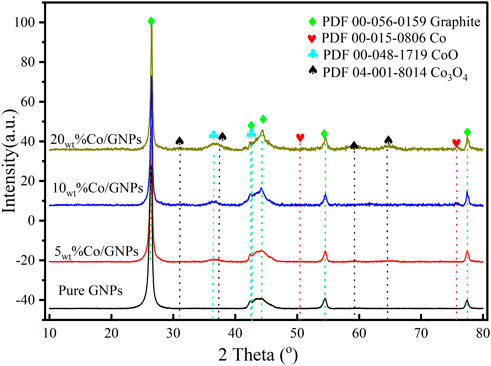
FIGURE 4. Full XRD patterns of GNP-based samples. Samples were first reduced with H2 at 400°C and measured ex situ in air.
3.3 Surface properties
Structural analysis of Co catalysts has revealed that the size of Co particles was dominated by the Co loading content. The size of the Co particles has always been considered a key aspect in the reactivity of Co, but the variation in reactivity with particle size did not agree with the findings in our study. Co’s crystalline phase also varies with the loading content, demonstrating that Co’s active phase in Co/GNPs catalysts might be different. To further define the active phase of Co, XPS and Raman spectrometry, which are powerful surface-sensitive techniques, have been used for verifying the surface phase of catalysts. In Figure 5, two satellite peaks at 785.8eV and 789.8eV indicate the coexistence of CoO (referred to as Co2+) and Co3O4 (referred to as Co3+) phases, respectively; meanwhile, the peak of metallic Co (about 778 eV) was not observed (Khassin, 2001; Lahijani et al., 2015; Cañón and Teplyakov, 2021). The deconvolution of the Co 2p peak based on previous literature studies allowed calculation of the ratio of Co2+/Co3+ on the surface. As summarized in Table 2, the ratio of Co2+/Co3+ of the tested catalysts followed the trend of 5wt%Co/GNPs > 5wt%Co/Al2O3 > 15wt%Co/GNPs >10wt%Co/GNPs, which is consistent with the trend of their intrinsic Co activity (Figure 1B). This trend indicates that CoO is the preferred phase for CO2 conversion, as reported. The different amounts of CoO formed on the catalyst’s surface were related to the redox ability of Co which is normally related to Co particle size and metal–support interactions (Torshizi et al., 2021; Díez-Ramírez et al., 2017; Parastaev et al., 2020). The GNP support was more conducive to the formation of the CoO phase for the reaction compared to the influence of particle size.
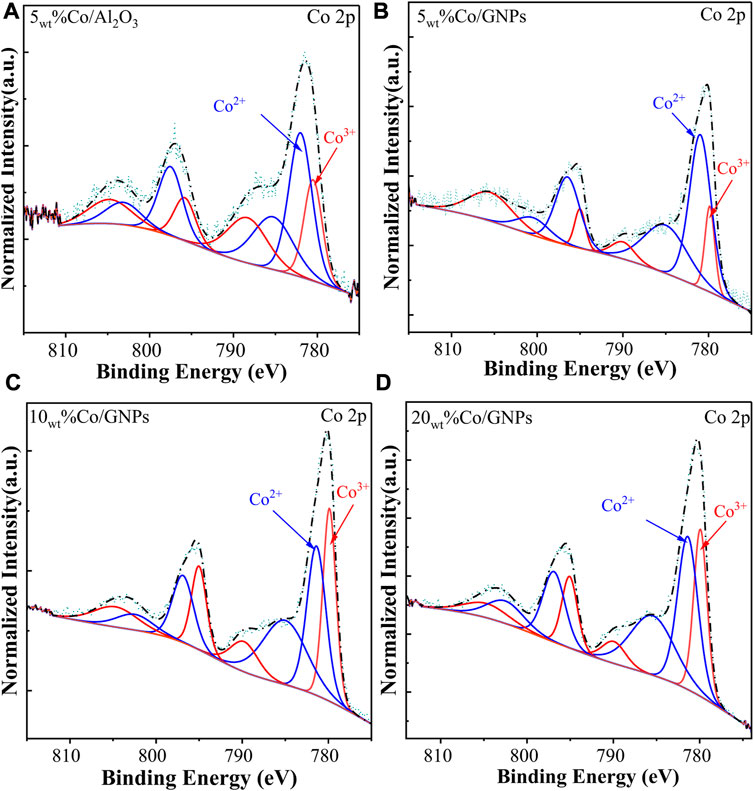
FIGURE 5. High-resolution XPS spectra of Co 2p for cobalt-based catalysts. All samples were reduced at 400°C with 5% H2 in N2 before measurement.
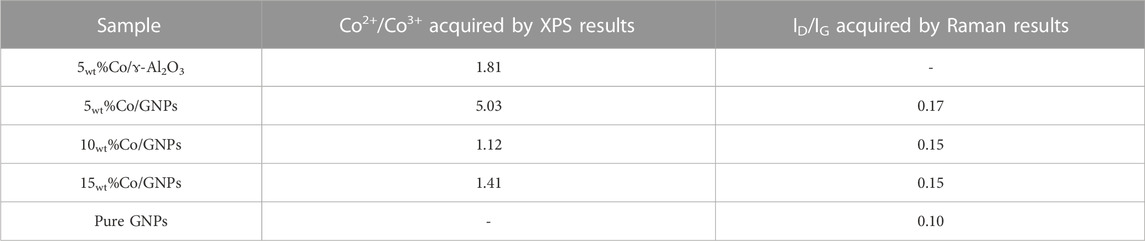
TABLE 2. Ratio of different cobalt species and the graphene phase on the surface of the catalyst and support analyzed by XPS and Raman spectrometry, respectively.
Figure 6A shows the typical Raman spectra of GNPs in catalysts and as a support only. A strong G-band peak appears at about 1583 cm−1 attributed to the E2g mode, which is caused by stretching of the C-C bond in all carbon materials. A 2D band peak located around 2700 cm−1 confirms the graphitic structure of GNPs. (Ci et al., 2010), (Luo et al., 2017) The relative low intensity of the 2D band together with a red shift location indicates that GNPs contain multiple graphene layers. Another peak at about 1350 cm−1 is the signature of the D band caused by disorder in the graphene structure. It is known that doping with transition metals, Ni, Co or Pt, leads to extrinsic disorder in graphene structure with formation of covalent bonds between C-atoms (Krasheninnikov et al., 2009; Xin et al., 2016). The quantification of the relative ratio between the intensities of the D band and the G band (ID/IG) provides information on the degree of disorder in the graphene layers after Co loading (Cançado et al., 2011) (Table 2). The 5wt% Co/GNPs catalyst has the highest ID/IG ratio at 0.17, while the support alone has the lowest ID/IG ratio at 0.10. However, loading more Co does not increase the disorder but results in less disorder. According to theoretical calculations (Krasheninnikov et al., 2009), the addition of a dopant to graphene shifts the Fermi-level of carbon, leading to the enhancement of conductivity and catalytic activity. This helps explain why the 5wt% Co/GNP sample had the highest reactivity for CO2 conversion. In this sample, it is also possible that more Co interacted with graphene, leading to a more stable electronic structure resisting the redox transformation of the CoO phase under reaction conditions. The agglomeration of Co clusters results in lower formation of interfaces or boundaries between graphene and Co.
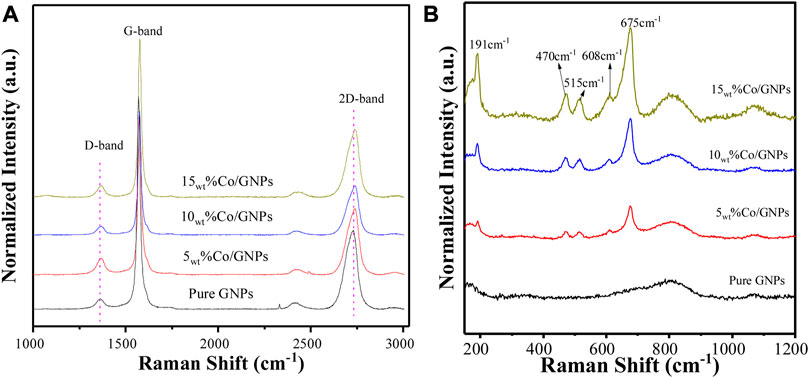
FIGURE 6. Raman spectra of catalysts and pure GNPs; the range of carbon features is shown in (A), while the cobalt features are depicted in (B). The Co/GNP samples were reduced before measurement, while the pure GNPs were measured directly without any pretreatment.
The Raman spectra of the Co phase are shown in Figure 6B. Five intense peaks located at 191, 470, 515, 608, and 675 cm−1 were found in all the Co/GNP samples. Based on previous results, a CoO octahedron-shaped sample registers three Raman peaks around 515 cm−1, 555 cm−1, and 680 cm−1 (Ravindra et al., 2014), while Co3O4 with a spinel structure has five Raman-active modes: A1g, Eg, and three T2g modes located around 194 cm−1, 479 cm−1, 515 cm−1, 617 cm−1, and 686 cm−1, respectively. Among the five modes, the A1g mode at 194cm-1 is the most intense (Hadjiev et al., 1988). For the stretching of Co-O, the nanoscale size of the sample and distortions of the lattice due to defects leads to the shift of position and change in intensity (Li et al., 2016). These characteristic peaks were observed in all samples with slight shifts, indicating the co-existence of CoO and Co3O4 oxide on the sample surface. This agrees with the XPS results, which always depict a face with a mixture of Co3+ and Co2+. It should be noted that the applied laser could also cause CoO oxidation, resulting in more Co3O4 phase, which complicates the discussion [44]. However, the relative ratio of Co3O4 in the sample can be derived indirectly from the intensity of the peak at 191cm-1 that corresponds to the A1g mode of the Co3O4 phase. The spectra demonstrate that 5wt% Co/GNP has the weakest peak at 191cm-1 after normalization, indicating that this catalyst has the highest CoO ratio, but the smallest amount of the Co3O4 phase. This finding is consistent with the XPS results, highlighting the CoO phase’s established reactivity for CO2 methanation.
3.4 Stability
The stability of carbon materials is always a concern for application in thermal CO2 conversion reactions due to the reverse Boudouard reaction of CO2 + C – 2CO (Lahijani et al., 2015). This reaction, also known as the CO2 gasification reaction, is thermodynamically supported at temperatures above 350°C, and at ambient pressure. In the case of a GNP-supported catalytic system for CO2 methanation, if the GNPs interact with the CO2 reactants, the support lifetime will be limited. Herein, we have used CO2-TGA to determine the stability of a synthesized GNP-supported Co catalyst. The test was performed at higher temperatures than the ideal CO2 methanation temperature of 200–300°C. Figure 7A demonstrates that the sample mass remains steady in CO2 at 400°C up to 2 h. The time-on-stream CO2 conversion of this catalyst running at 350°C shows a lifetime up to 24 h with only a slight decrease. This decrease is perhaps caused by the agglomeration of cobalt particles, as indicated in TEM images (Figure 3); stabilization of the Co particles should obviate this problem.
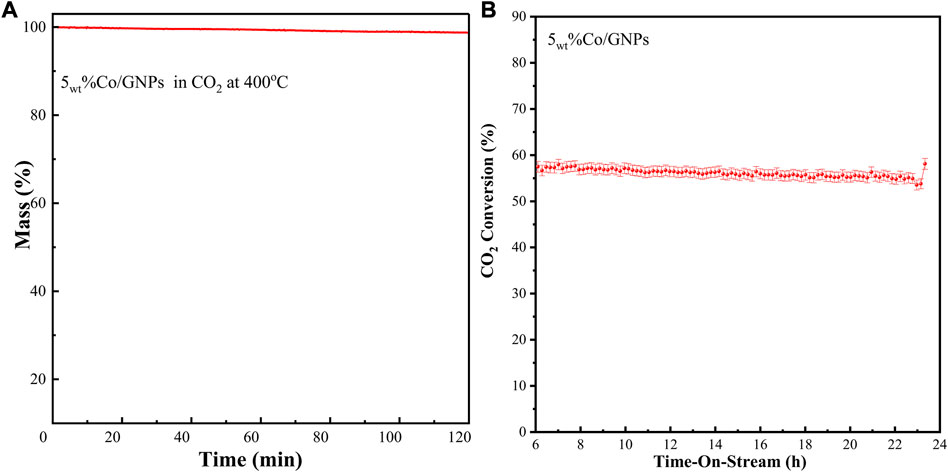
FIGURE 7. (A) TGA curve of the 5wt% Co/GNP sample after reduction in a continuous CO2 stream; (B) time-on-stream of CO2 conversion by a 5wt% Co/GNPs catalyst tested at 350°C under constant reaction conditions: 1 bar, 19.5 ml/min total flow rate with a reaction mixture containing 15vol% of CO2, 60vol% of H2, and 25vol% of N2.
4 Conclusions
Commercial GNP material was used as a support of a cobalt catalyst for CO2 methanation. The as-synthesized Co/GNP catalysts exhibited higher intrinsic reactivity than the Al2O3-supported Co catalyst. The best Co/GNPs, according to the reactivity tests, were obtained with a loading of 5wt% Co. TEM size analysis showed that larger Co particles were formed with increased Co loading, while Raman spectra showed that the growth of cobalt nanoparticles reduced the formation of interfaces between Co and the graphene surface, ultimately influencing the total number of Co active sites. As a result, fewer surface defects of graphene were produced in high-loading (10wt% and 15wt%) Co/GNP samples, which corresponded to lower catalytic reactivity and CH4 yield. Cobalt surface analysis using XPS and Raman spectra showed that a higher oxidative phase of Co (Co3+) was observed on the surface of materials with high Co loading. With the same loading, the sample supported by GNPs had more of the Co2+ phase than the cobalt supported by Al2O3. Accordingly, we conclude that the GNP support promotes the formation and maintenance of more of the reactive Co2+ phase on the catalyst surface for CO2 methanation. Modest Co loading (5wt%) promoted the formation of graphene defects while simultaneously forming more CoO active phases for increased reactivity. In conclusion, this research has been the first to demonstrate the potential reactivity of a carbon-supported Co catalyst and the synergistic role of graphene support for CO2 thermal methanation. Further efforts need to be devoted to improving the lifetime of this catalyst system.
Data availability statement
The original contributions presented in the study are included in the article/Supplementary Material. Further inquiries can be directed to the corresponding author.
Author contributions
LZ performed the synthesis, tests, characterization, data analysis, and paper drafting. TM performed the TEM and SEM microscopy measurements. YK measured the TGA and ICP-OES catalysts. LZ wrote the paper, with inputs from other authors. AZ reviewed the paper. All authors contributed to the article and approved the submitted version.
Funding
This research was supported by GAZNAT and the Swiss Federal Office of Energy (SFOE) under contract SI/502228-0.
Acknowledgments
The authors acknowledge the financial support of EPFL and Empa in Switzerland.
Conflict of interest
The authors declare that the research was conducted in the absence of any commercial or financial relationships that could be construed as a potential conflict of interest.
Publisher’s note
All claims expressed in this article are solely those of the authors and do not necessarily represent those of their affiliated organizations, or those of the publisher, the editors, and the reviewers. Any product that may be evaluated in this article, or claim that may be made by its manufacturer, is not guaranteed or endorsed by the publisher.
Supplementary material
The Supplementary Material for this article can be found online at: https://www.frontiersin.org/articles/10.3389/fceng.2023.1160254/full#supplementary-material
References
Bacariza, M. C., Spataru, D., Karam, L., Lopes, J. M., and Henriques, C. (2020). Promising catalytic systems for CO2 hydrogenation into CH4: A review of recent studies. Processes 8 (12), 1–45. doi:10.3390/pr8121646
Bailera, M., Lisbona, P., Romeo, L. M., and Espatolero, S. (2017). Power to Gas projects review: Lab, pilot and demo plants for storing renewable energy and CO2. Renew. Sustain. Energy Rev. 69, 292–312. doi:10.1016/J.RSER.2016.11.130
Cançado, L. G., Jorio, A., Ferreira, E. H. M., Stavale, F., Achete, C. A., Capaz, R. B., et al. (2011). Quantifying defects in graphene via Raman spectroscopy at different excitation energies. Nano Lett. 11 (8), 3190–3196. doi:10.1021/nl201432g
Cañón, J., and Teplyakov, A. v. (2021). XPS characterization of cobalt impregnated SiO2 and γ-Al2O3. Surf. Interface Analysis 53 (5), 475–481. doi:10.1002/sia.6935
Ci, L., Song, L., Jin, C., Jariwala, D., Wu, D., Li, Y., et al. (2010). Atomic layers of hybridized boron nitride and graphene domains. Nat. Mater 9 (5), 430–435. doi:10.1038/nmat2711
Díez-Ramírez, J., Sanchez, P., Kyriakou, V., Zafeiratos, S., Marnellos, G., Konsolakis, M., et al. (2017). Effect of support nature on the cobalt-catalyzed CO2 hydrogenation. J. CO2 Util. 21, 562–571. doi:10.1016/j.jcou.2017.08.019
Frontera, P., Macario, A., Ferraro, M., and Antonucci, P. L. (2017). Supported catalysts for CO2 methanation: A review. Catalysts 7, 59. doi:10.3390/catal7020059
Hadjiev, v. G., Iliev, M. N., and Vergilov, I. V. (1988). The Raman spectra of Co3O4. J. Phys. C Solid State Phys. 21 (7), L199–L201. doi:10.1088/0022-3719/21/7/007
He, F., Niu, N., Qu, F., Wei, S., Chen, Y., Gai, S., et al. (2013). Synthesis of three-dimensional reduced graphene oxide layer supported cobalt nanocrystals and their high catalytic activity in F-T CO2 hydrogenation. Nanoscale 5 (18), 8507–8516. doi:10.1039/c3nr03038e
Jimenez, V., Jimenez-Borja, C., Sanchez, P., Romero, A., Papaioannou, E. I., Theleritis, D., et al. (2011). Electrochemical promotion of the CO2 hydrogenation reaction on composite Ni or Ru impregnated carbon nanofiber catalyst-electrodes deposited on YSZ. Appl. Catal. B-ENVIRONMENTAL 107 (1–2), 210–220. doi:10.1016/j.apcatb.2011.07.016
Johnsson, F., Kjärstad, J., and Rootzén, J. (2019). The threat to climate change mitigation posed by the abundance of fossil fuels. Clim. Policy 19 (2), 258–274. doi:10.1080/14693062.2018.1483885
Karimi, S., Tavasoli, A., Mortazavi, Y., and Karimi, A. (2015). Cobalt supported on Graphene - a promising novel Fischer-Tropsch synthesis catalyst. Appl. Catal. A Gen. 499, 188–196. doi:10.1016/j.apcata.2015.04.024
Khassin, A. A. (2001). Metal-support interactions in cobalt-aluminum co-precipitated catalysts. XPS and CO adsorption studies’.
Krasheninnikov, A. v., Lehtinen, P. O., Foster, A. S., Pyykkö, P., and Nieminen, R. M. (2009). Embedding transition-metal atoms in graphene: Structure, bonding, and magnetism. Phys. Rev. Lett. 102 (12), 126807. doi:10.1103/PhysRevLett.102.126807
Kuśmierz, M. (2008). Kinetic study on carbon dioxide hydrogenation over Ru/γ-Al2O3 catalysts. Catal. Today 137 (2–4), 429–432. doi:10.1016/j.cattod.2008.03.003
Lahijani, P., Zainal, Z. A., Mohammadi, M., and Mohamed, A. R. (2015). Conversion of the greenhouse gas CO2 to the fuel gas CO via the boudouard reaction: A review. Renew. Sustain. Energy Rev. 41, 615–632. doi:10.1016/j.rser.2014.08.034
Li, Y., Qiu, W., Qin, F., Fang, H., Hadjiev, V. G., Litvinov, D., et al. (2016). Identification of cobalt oxides with Raman scattering and fourier transform infrared spectroscopy. J. Phys. Chem. C 120 (8), 4511–4516. doi:10.1021/acs.jpcc.5b11185
Liu, Q., Bian, B., Fan, J., and Yang, J. (2018). Cobalt doped Ni based ordered mesoporous catalysts for CO2 methanation with enhanced catalytic performance. Int. J. Hydrogen Energy 43 (10), 4893–4901. doi:10.1016/j.ijhydene.2018.01.132
Luo, W., Zafeiratos, S., and Zafeiratos, S. (2017). A brief review of the synthesis and catalytic applications of graphene-coated oxides. ChemCatChem 9 (13), 2432–2442. doi:10.1002/cctc.201700178
Mateo, D., Albero, J., and García, H. (2018). Graphene supported NiO/Ni nanoparticles as efficient photocatalyst for gas phase CO2 reduction with hydrogen. Appl. Catal. B 224, 563–571. doi:10.1016/j.apcatb.2017.10.071
Ojelade, O. A., and Zaman, S. F. (2021). A review on CO2hydrogenation to lower olefins: Understanding the structure-property relationships in heterogeneous catalytic systems. J. CO2 Util. 47, 101506. doi:10.1016/j.jcou.2021.101506
Parastaev, A., Muravev, V., Huertas Osta, E., van Hoof, A. J. F., Kimpel, T. F., Kosinov, N., et al. (2020). Boosting CO2 hydrogenation via size-dependent metal–support interactions in cobalt/ceria-based catalysts. Nat. Catal. 3 (6), 526–533. doi:10.1038/s41929-020-0459-4
Pham, T. H. M., Zhang, J., Li, M., Shen, T., Ko, Y., Tileli, V., et al. (2022). Enhanced electrocatalytic CO2 reduction to C2+ products by adjusting the local reaction environment with polymer binders. Adv. Energy Mater 12 (9), 2103663. doi:10.1002/aenm.202103663
Qi, Z., Chen, L., Zhang, S., Su, J., and Somorjai, G. A. (2020). A mini review of cobalt-based nanocatalyst in Fischer-Tropsch synthesis. Appl. Catal. A Gen. 602, 117701. doi:10.1016/j.apcata.2020.117701
Rahmati, M., Safdari, M. S., Fletcher, T. H., Argyle, M. D., and Bartholomew, C. H. (2020). Chemical and thermal sintering of supported metals with emphasis on cobalt catalysts during fischer-tropsch synthesis. Chem. Rev. 120 (10), 4455–4533. doi:10.1021/acs.chemrev.9b00417
Ravindra, A. v., Behera, B. C., and Padhan, P. (2014). Laser induced structural phase transformation of cobalt oxides nanostructures. J. Nanosci. Nanotechnol. 14 (7), 5591–5595. doi:10.1166/jnn.2014.9023
Sadasivan, S., Bellabarba, R. M., and Tooze, R. P. (2013). Size dependent reduction-oxidation-reduction behaviour of cobalt oxide nanocrystals. Nanoscale 5 (22), 11139–11146. doi:10.1039/c3nr02877a
ten Have, I. C., Kromwijk, J. J. G., Monai, M., Ferri, D., Sterk, E. B., Meirer, F., et al. (2022). Uncovering the reaction mechanism behind CoO as active phase for CO2 hydrogenation. Nat. Commun. 13 (1), 324. doi:10.1038/s41467-022-27981-x
Torshizi, H. O., Nakhaei Pour, A., Mohammadi, A., Zamani, Y., and Kamali Shahri, S. M. (2021). Fischer-Tropsch synthesis by reduced graphene oxide nanosheets supported cobalt catalysts: Role of support and metal nanoparticle size on catalyst activity and products selectivity. Front. Chem. Sci. Eng. 15 (2), 299–309. doi:10.1007/s11705-020-1925-x
Tu, J., Wu, H., Qian, Q., Han, S., Chu, M., Jia, S., et al. (2021). Low temperature methanation of CO2over an amorphous cobalt-based catalyst. Chem. Sci. 12 (11), 3937–3943. doi:10.1039/d0sc06414a
Vogt, C., Groeneveld, E., Kamsma, G., Nachtegaal, M., Lu, L., Kiely, C. J., et al. (2018). Unravelling structure sensitivity in CO2 hydrogenation over nickel. Nat. Catal. 1 (2), 127–134. doi:10.1038/S41929-017-0016-Y
Wang, X., Hong, Y., Shi, H., and Szanyi, J. (2016). Kinetic modeling and transient DRIFTS–MS studies of CO2 methanation over Ru/Al2O3 catalysts. J. Catal. 343, 185–195. doi:10.1016/j.jcat.2016.02.001
Wigzell, F. A., and Jackson, S. D. (2017). The Genesis of supported cobalt catalysts. Appl. Petrochem Res. 7 (1), 9–21. doi:10.1007/s13203-016-0175-9
Xin, L., Yang, F., Rasouli, S., Qiu, Y., Uzunoglu, A., et al. (2016). Understanding Pt nanoparticle anchoring on graphene supports through surface functionalization. ACS Catal. 6 (4), 2642–2653. doi:10.1021/acscatal.5b02722
Zhao, K., Calizzi, M., Moioli, E., Li, M., Borsay, A., Lombardo, L., et al. (2020). Unraveling and optimizing the metal-metal oxide synergistic effect in a highly active Co (CoO)1– catalyst for CO2 hydrogenation. J. Energy Chem. 53, 241–250. doi:10.1016/j.jechem.2020.05.025
Zhong, L., Barreau, M., Caps, V., Papaefthimiou, V., Haevecker, M., Teschner, D., et al. (2021). Improving the catalytic performance of cobalt for CO preferential oxidation by stabilizing the active phase through vanadium promotion. ACS Catal. 11 (9), 5369–5385. doi:10.1021/acscatal.0c05482
Zhong, L., Barreau, M., Chen, D., Caps, V., Haevecker, M., Teschner, D., et al. (2021). Effect of manganese promotion on the activity and selectivity of cobalt catalysts for CO preferential oxidation. Appl. Catal. B 297, 120397. doi:10.1016/j.apcatb.2021.120397
Keywords: CO2 reduction, CoO catalyst, graphene support, methanation, metal–support interaction
Citation: Zhong L, Pham THM, Ko Y and Züttel A (2023) Graphene nanoplatelets promoted CoO-based catalyst for low temperature CO2 methanation reaction. Front. Chem. Eng. 5:1160254. doi: 10.3389/fceng.2023.1160254
Received: 06 February 2023; Accepted: 20 March 2023;
Published: 15 May 2023.
Edited by:
Wen Luo, Shanghai University, ChinaReviewed by:
Lole Jurado, Institut Català d'Investigació Química, SpainJingjie Luo, Dalian University of Technology, China
Copyright © 2023 Zhong, Pham, Ko and Züttel. This is an open-access article distributed under the terms of the Creative Commons Attribution License (CC BY). The use, distribution or reproduction in other forums is permitted, provided the original author(s) and the copyright owner(s) are credited and that the original publication in this journal is cited, in accordance with accepted academic practice. No use, distribution or reproduction is permitted which does not comply with these terms.
*Correspondence: Liping Zhong, bGlwaW5nLnpob25nQGVwZmwuY2g=